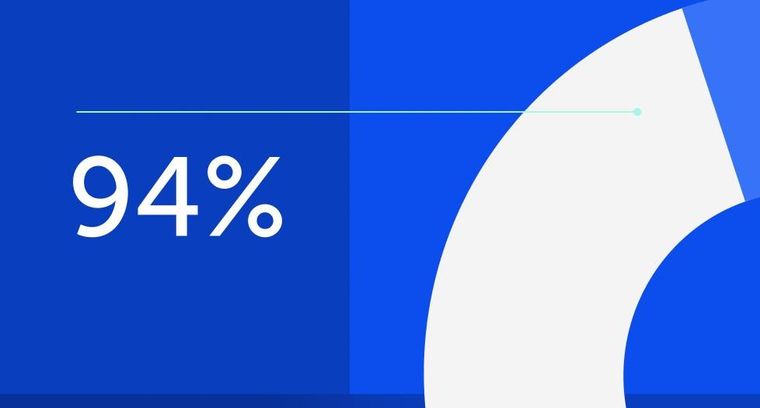
94% of researchers rate our articles as excellent or good
Learn more about the work of our research integrity team to safeguard the quality of each article we publish.
Find out more
REVIEW article
Front. Endocrinol., 09 August 2023
Sec. Bone Research
Volume 14 - 2023 | https://doi.org/10.3389/fendo.2023.1190762
This article is part of the Research TopicCellular and Molecular Repair of Fracture Non-UnionView all 4 articles
Background: Alzheimer’s disease (AD) is a neurodegenerative disorder that is the major cause of dementia in the aged population. Recent researches indicate that patients with AD have a significantly increased fracture risk, but the pathological mechanisms are still unclear.
Objective: We systematically reviewed studies regarding bone fracture risk in AD to uncover links between the pathologies of osteoporosis and AD.
Methods: We searched the literature using the databases of PubMed, Web of Science, Embase and Cochrane Library. Studies were included if they evaluated bone fracture risk in AD patients and if they explored the pathogenesis and prevention of bone fractures in these patients.
Results: AD patients had a significantly higher risk of bone fractures than age-matched controls. Multiple factors contributed to the increased risk of bone fractures in AD patients, including the direct effects of amyloid pathology on bone cells, abnormal brain-bone interconnection, Wnt/β-catenin signalling deficits, reduced activity, high risk of falls and frailty, and chronic immune activity. Exercise, prevention of falls and fortified nutrition were beneficial for reducing the fracture risk in AD patients. However, the efficacy of anti-osteoporotic agents in preventing bone fractures should be further evaluated in AD patients as corresponding clinical studies are very scarce.
Conclusion: Alzheimer’s disease patients have increased bone fracture risk and decreased bone mineral density owing to multiple factors. Assessment of anti-osteoporotic agents’ efficacy in preventing bone fractures of AD patients is urgently needed.
● Bone fractures risk is significantly increased in patients with Alzheimer’s disease (AD)
● The mechanisms include the effects of amyloid pathology on bone cells
● Brain-bone interconnections and Wnt/β-catenin signalling deficits play important roles
● Reduced activity, high risk of falls and frailty increase fracture risk
● Reducing fracture risk is important for patients with AD
Alzheimer’s disease (AD) is a neurodegenerative disease that causes cognitive impairment, of which short-time period memory deficits are a common manifestation (1). Deficits in speech, visuospatial processing and executive function can be presented in AD patients (1). In recent years, the quantity of patients with AD has been growing substantially, accounting for greater than 50% of dementia cases (2). The prevalence of dementia is predicted to increase from 57.4 million individuals worldwide in 2019 to 152.8 million individuals by 2050 (3). The global economic burden of AD and related dementias (ADRDs) is expected to grow rapidly from an estimated $2.8 trillion in 2019 to $16.9 trillion in 2050 (4).
Recently, studies indicate that AD patients have a higher risk of bone fracture, particularly hip fracture, than the older populations without AD (5–7). A meta-analysis including five studies indicated that AD was correlated with a 2.5-fold increased risk of hip fractures (8), which would lead to devastating consequences in AD patients, including loss of function and mobility, reduced quality of life(QOL), prolonged stay for hospitalization, and increased morbidity and mortality (6, 9). The increased falls owing to gait disturbances and postural instability may partially explain the increased risk of bone fractures in AD patients (10, 11). However, other reasons should also be considered for the increased bone fracture risk in AD patients, which deserves further in-depth investigation.
Therefore, we systematically review the studies regarding bone fractures in AD patients and focus on progress in pathological mechanisms and prevention of bone fractures in AD patients.
We systematically reviewed studies about the risk of bone fractures in AD patients, and we conducted a literature search using the databases of PubMed, Web of Science, Embase and Cochrane Library from January 1990 to December 2022, using the keywords ‘fractures’, ‘bone fractures’, ‘broken bones’, ‘osteoporotic fractures’, ‘hip fracture’, ‘Alzheimer’s disease’, ‘Alzheimer-type dementia’, ‘Alzheimer sclerosis’ and ‘Alzheimer syndrome’. In addition, the reference lists of included studies were checked and the names of authors were searched for additional studies. All articles were screened based on their title and abstract. Studies were included if they assessed the risk of bone fractures in AD patients, and studies were also included if they explored the pathogenesis and prevention of bone fractures in AD patients. Articles written in languages other than English, expert opinions, case reports and articles without full texts were excluded.
Our search yielded a total of 316 related studies, and 12 papers met the eligibility criteria (5–7, 12–20) (Figure 1). One study was a prospective matched-cohort study, and the others were retrospective matched-cohort studies, with follow-up durations ranging from 1 to 11 years. The characteristics of these studies were summarized in Table 1.
Data from prospective and retrospective matched-cohort studies indicated an independent relationship between AD and an increased incidence of bone fractures. Three studies evaluated the incident fracture rate in AD patients (5, 14, 17). They observed that patients with AD had a higher risk of fracture than those without AD. The most frequent fractures were hip fractures and vertebral fractures in AD patients (14). Seven studies used the incidence of hip fracture as the outcome (6, 7, 13, 15, 16, 18, 19), which indicated that AD patients had an increased risk of hip fractures than patients without AD, regardless of age, gender or AD duration. An independent correlation between AD and hip fractures was found, and AD was an independent risk factor for hip fractures. Another study focused on the reasons for hospitalizations in AD patients and discovered that patients with hip fracture were more likely to be hospitalized (12). A matched-cohort study exploring comorbidities and the risk of mortality in AD patients showed that hip fracture was more prevalent in the AD cohort than in the non-AD cohort and that hip fracture was correlated to the mortality risk of AD patients (20).
Studies have found that AD patients often had lower bone mineral density (BMD) than healthy individuals. Low BMD, impaired bone strength and microarchitecture could increase bone fracture risk, which was mainly led by disturbances in bone resorption and bone formation. Multiple factors could contribute to bone loss in AD patients. The pathogenesis of bone fractures in patients with AD was shown in Figure 2.
Figure 2 The pathogenesis of increased fracture risk in AD patients. Multiple factors may contribute to bone loss in AD, including direct effects of amyloid pathology on bone cells, abnormal brain-bone interconnection, Wnt/β-catenin signaling deficits, chronic inflammatory status and reduced activity, increased falls and frailty in AD patients. AD, Alzheimer’s disease; Aβ, amyloid-β peptide; SNS, sympathetic nervous systems; PNS, parasympathetic nervous systems; TNF-α, tumor necrosis factor α; IL 1β, interleukin 1β; IL 6, interleukin 6; IL 10, interleukin 10; RANKL, receptor activator of nuclear factorkappa B ligand; RUNX2, runt-related transcription factor 2; OCN, osteocalcin; DKK1, Dickkopf-1.
Two main pathological hallmarks of AD are the presence of extracellular aggregates of amyloid-beta peptide (Aβ) derived from the transmembrane amyloid precursor protein (APP) and the presence of neurofibrillary protein tangles, which might be composed of hyperphosphorylated tau, withinside the temporal lobe and different cortical areas in the brain which might be related to the death of neuronal cells and synaptic depletion (21). There is developing proof of the involvement of advanced glycation end products (AGEs) in the pathogenesis of AD and their feature as seeds for the aggregation of Aβ (22). The receptor for AGEs (RAGEs) acting as an Aβ binding partner are also included in the pathogenesis of AD (23). Thus, APP/Aβ plays a significant role in the pathogenesis of AD.
Moreover, investigations have suggested that APP/Aβ can directly damage skeletal remodelling by impacting bone cells. A previous study observed that APP and Aβ could regulate in-vitro and in-vivo osteoclast (OC) differentiation (24). Tg2576 mice, an AD mice model expressing the Swedish mutation of APP (APPswe) below the manipulation of a prion promoter, exhibited biphasic outcomes on OC activation, with an increase in OCs in younger mice but a decrease in older Tg2576 mice (24). In younger Tg2576 mice, the Aβ-RAGE–mediated increase in OCs had a function in selling the discharge of cytokines and elements from the bone matrix, thus included in the pathogenesis of bone fractures. However, in older Tg2576 mice, there was an increase in soluble RAGE (sRAGE) and osteoprotegerin (OPG), causing a decrease in OC formation, which may delay bone remodelling in an unbalanced way and thus might be associated with a higher rate of bone fractures (24). Another study confirmed that a decrease in osteoblastogenesis and loss of trabecular bone mass was resulted from the selective expression of APPswe in mature osteoblast-lineage cells (25). The bone loss was accompanied by elevated adipogenesis and increased bone marrow fat, showing a skeletal aging-like osteoporotic deficit (25). APP could play a physiological role in promoting osteoblast survival and bone formation by preventing oxidative stress and regulating mitochondrial function (26). Mice with knocked out APP gene (APP-/-) exhibited osteoporotic-like deficits, including decreased trabecular and cortical bone mass (26). Aβ42 is a key amyloidogenic peptide that is highly associated with AD. Aβ42 can potently enhance osteoclast differentiation and activation but does not affect osteoclast cell viability or number (27). One study observed that 5XFAD mice had decreased volumetric BMD, elevated endocortical bone loss and reduced mineralization with smaller mineral crystals (28). Two pathways were found to be contributing to skeletal fragility in AD via alteration of bone quality: accumulation of AGEs and lack of crystallinity, reduced crystal size, and lack of mineralization (28). Another animal study showed that endogenous Aβ might induce osteoporosis via mTOR-dependent inhibition of autophagy in bone marrow mesenchymal stem cells (BMSCs) (29). Therefore, APP/Aβ has direct effects on bone cells and may play a vital function in the pathogenesis of bone fractures in AD patients.
Recently, the roles of neural control in bone have been found (30). Both the sympathetic nervous system (SNS) and parasympathetic nervous system (PNS) can influence bone via numerous pathways, in which circadian genes, neuropeptide Y, serotonin, leptin, adiponectin, muscarinic receptors, nicotinic receptors, beta-adrenergic receptors, and sensory nerve innervation of bone are involved (31, 32). The nervous system can produce specific neurotransmitters and process peripheral hormonal signals, hence affecting bone homeostasis. This increases the probability that the ability of the brain to regulate bone in AD patients could be compromised and consequently reduce bone mass (33). AD patients usually show increased sympathetic tone and decreased parasympathetic flow, and reduced cholinergic innervation in the elderly (34). AD patients treated with AChE inhibitors exhibited a lower risk of hip fracture and stepped forward bone healing, indicating the damaged parasympathetic signalling affects bone homeostasis and might be a target to improve the bone health of AD patients (35).
An understanding of bone’s effects on the brain has also emerged as studies have revealed the possible underlying mechanisms regarding the brain’s influence on bone. Bone act as an endocrine organ that plays important roles by secreting proteins, including osteocalcin (OCN), osteopontin (OPN), and sclerostin (SOST). Blood bone turnover biomarkers, such as C-terminal fragments of collagen, osteoprotegerin (OPG), and OCN were found to be increased in AD patients, showing their association with osteoporosis (36). The bone-derived OCN can impact the production of neurotransmitters, which affect cognitive function (37). Furthermore, a small number of bone marrow-derived stem cells may have the capacity to migrate to the brain, differentiate into microglia-like cells and accelerate Aβ clearance (38). Thus, the brain and bones could be closely interconnected, and bidirectional signalling between brain and bone tissue may have been involved in an increased risk of fracture in AD patients.
The Wnt/β-catenin signalling pathway is a rich and complicated network that modulates cell proliferation, migration and differentiation, and Wnt proteins orchestrate numerous short-range cell-to-cell communication in mammals (39). In bone, Wnt/β-catenin signalling contributes to osteoblast differentiation and promotes bone formation (40). In brain, the Wnt/β-catenin signalling pathway promotes the formation of synaptic junctions between neurons and increases neuronal survival (41). The impaired Wnt/β-catenin pathway plays a critical role in the development of AD (42). Insufficient Wnt/β-catenin activation impaired bone remodelling and changed the gene expression associated with Wnt/β-catenin signalling in a mouse model (htau mice), which exhibited both low bone mass and AD-like tauopathy (43). Moreover, increased expression of Dickkopf-1 (DKK1) and SOST were found in the bone of htau mice (43). SOST can encode proteins that antagonize the Wnt/β-catenin pathway at preliminary tiers of signalling, which is associated with a reduction in bone formation (44). DKK1 additionally performs numerous roles in the pathogenesis of AD (45). The expression of DKK1 could be overactivated by the pathological protein Aβ, further suppressing the Wnt pathway and triggering a sequence of downstream consequences that enhance the tau hyperphosphorylation and increase extra poisonous Aβ fragments cleavage, thus perpetuating Wnt disorder and promoting the accumulation of toxic protein (45). These provocative data supported that a systemic motive force which includes Wnt signalling deficits would possibly relate to bone loss and brain pathology in patients with AD.
Neuroinflammation also plays essential roles in the pathogenesis and progression of AD (46). AD pathogenesis can be promoted by chronic neuroinflammation, which is driven by the overactivation of resident microglial cells, the infiltration of macrophages and the involvement of circulating immune cells (47). Numerous inflammatory markers were verified to be significantly altered in a comparison between AD patients and controls (48), and tumour necrosis factor-alpha (TNF-α) ranges were extensively increased in the cerebrospinal fluid (CSF) and the serum of AD patients than healthy controls and associated with disorder development (49), directly potentiating bone reformation in part through synergistic interactions with RANKL and upregulating osteoclast differentiation, thus promoting bone resorption (50). In addition, TNF-α could inhibit bone formation by indirectly suppressing osteoblast manufacturing and proliferation, which is via suppressing the expression of insulin-like growth factor-1, osterix (OSX), Wnt, and runt-related transcription factor 2 (RUNX2) signalling (51–53). The levels of interleukin 1β (IL1β), interleukin 6 (IL6), interleukin 10 (IL10) and TNFα were elevated in the cerebral tissue of AD mice, which indicated proinflammatory cytokines promoted the occurrence of AD (54). Inflammatory cytokines might also accelerate bone loss and increase fracture risks in AD patients (55).
AD patients usually have reduced activity in midlife (56). Bones are constantly motivated by weight-bearing movements and muscle contraction, and they are also sensitive to mechanical strain. Osteocytes and their dendritic connections can sense the fluid float driven by stresses placed upon bone. Osteocytes produce signalling molecules that activate bone remodelling in reaction to these stresses (57). Reduced mechanical strain because of immobilization accelerated bone loss in a time-, intense-dependent manner (58). There is an increased risk of falls and injuries in older patients with cognitive impairment compared with healthy controls. There was an up to eight times greater incidence of falls in patients with dementia than in those without cognitive impairment (59). Falls and fall-associated accidents could cause a two- to three-fold risk of hip fracture, slower recovery rates, increased likelihood of multiplied probability of being positioned into residential care and higher mortality than cognitively healthy peers (60). A study confirmed that cognitive impairment became a critical risk factor for falls in older people in Chinese communities (61).
The previous study showed that frailty is highly prevalent in AD patients with the pooled prevalence of 31.9% (62). Frailty is a clinically detectable syndrome associated with the ageing of multiple physiological systems, which could activate vulnerability, Additionally, frailty is associated with malnutrition, cognitive deterioration, atherosclerosis and sarcopenia, and their diverse metabolic alterations (63). A meta-analysis indicated that frailty was highly correlated with falls, bone fractures, hospitalization, disability, dementia, and death (64). Both frailty and decreased mobility lead to reduced muscle strength, and sarcopenia is common in elderly individuals with cognitive deterioration, which could be a vital risk factor for bone fractures in AD patients (65).
Exercise is beneficial to improve gait, balance, strength and mobility, and executive functions and further reduce falls in the older population (66), and it may postpone the progression of AD in a sustainable and cost-effective manner (67). Physical activity or exercise is also important for keeping bone health, since increasing muscle mass and mechanical stress can prevent bone loss (68, 69). Compared to wild-type mice, the AD mice (3xTg mice) had an increased risk for limb fracture. Treatment with resveratrol, exercise, or both in combination can improve fracture resistance and bone strength (70). A recent study showed that a multimodal exercise program reduced the incidence of falls and improved balance, gait, and BMD in institutionalized patients with AD (71). Falls are common in AD patients, and approximately two-thirds of AD patients fall annually, which is double the risk of falls in cognitively unimpaired elderly individuals (72).
Preventing falls is essential for AD patients, and these patients require multidisciplinary management. According to the World Guidelines for Fall Prevention and Management for Older Adults (73), there are wider benefits to reduce the risk factors for falls (e.g., gait and balance problems), including elevated intrinsic capacities (physical and mental health), functioning and the quality of life. Evaluation of the risk of future falls should be performed by experienced clinicians using existing resources. Multidomain interventions, which were a mixture of interventions tailored to the individual, including implementing strength and balance exercises, reviewing medications, optimizing vision and hearing, addressing foot problems and appropriate footwear, using interventions to cope with concerns about falling, making individual education and environmental modifications and so on, whilst delivered, were valid for downscaling the fall rates of high-risk community-dwelling elderly individuals (73).
AD patients are often malnourished because of physiological and psychological factors (74). Nutrition (e.g., via vegetables, fruit, and fish) is critical for optimizing cognition and decreasing fracture risk in AD patients (75). The importance of a balanced diet including protein, vegetables, fruit and minerals has been emphasized for bone health and the prevention of fractures (76). Healthy dietary patterns, such as the Mediterranean diet, could be helpful for AD patients and reduce fracture risk. It is beneficial for the prevention of osteoporosis through the supplementation of key micronutrients for bone, such as calcium and vitamin D (77). Additionally, some clinical studies showed that a higher vitamin D status was related to a decreased risk of AD and all-cause dementia, which has supported the significance of vitamin D in cognitive health (78, 79). Some randomized controlled trials found that biomeasures and cognitive function were improved in AD patients after supplementation of vitamin D (80). Thus, it is essential to maintain sufficient vitamin D concentrations to reduce bone loss and neurocognitive decline.
In recent years, great progress has been made in drug treatment for osteoporosis, and the effective agents include antiresorptive, anabolic and dual-action agents. However, there are few studies evaluating the effect of anti-osteoporotic agents on the risk of fracture in AD patients. The worry of side effects would possibly result in hesitation to prescribe these agents to the frailest elderly patients. For instance, patients with dementia were probably more sensitive to the serious side effects of bisphosphonates (BPs) (81).
BPs have been proven to be a key intervention for osteoporosis, and they are considered to be one of the first-line medicinal drugs for preventing osteoporotic fractures (82). Some clinical and preclinical studies have revealed that nitrogen-containing BPs (NBPs) may be potential to alleviate the symptoms of neurological disorders such as brain calcification, AD and Huntington’s disease by targeting the mevalonate pathway (83). In the MEDALZ-2005 cohort, the incidence of BP use was 11.2%, and the median period of BP use was 777 days among AD patients (84). But they didn’t evaluate the effects of BPs in that cohort (84). In a cohort comprised of nursing home residents aged 65 years or older, of which 51% patients had moderate-to-severe cognitive impairment, BPs treatment was correlated with a significant reduction in hip fracture among frail, elderly patients (85).
As a fully human monoclonal antibody targeting the bone resorption mediator RANKL, denosumab is effective in postmenopausal osteoporosis, male osteoporosis and glucocorticoid-induced osteoporosis (86–88). Teriparatide, a recombinant fragment of the human parathyroid hormone, is an anabolic drug that can substantially increase BMD and decrease the incidence of vertebral fractures (89, 90). The effects of denosumab or teriparatide on osteoporosis are still unclear in AD patients. The effects of zoledronic acid, denosumab, and teriparatide for preventing hip fractures were evaluated in frail older patients, and some patients with cognitive impairment were included in that study (91). The study showed that denosumab and zoledronic acid might be as effective as teriparatide for the prevention of hip fractures in frail older patients, but further investigation is required to evaluate their efficacy and safety in AD patients (91).
Romosozumab, a monoclonal antibody to sclerostin, is a new osteoanabolic drug that increases bone formation and decreases bone resorption, which is recommended as preliminary treatment in patients with a very high fracture risk without a history of stroke or myocardial infarction (92). Since circulating DKK1 level was significantly correlated with the annual rate of change in cognition (93), DKK1 could be a novel biomarker and promising therapeutic target for osteoporosis in AD patients. No reports have been made targeting sclerostin or DKK1 in osteoporosis in AD patients.
Above all, few studies have evaluated the effects and safety of anti-osteoporotic drugs on osteoporosis in AD patients. There is an urgent need to conduct research on treatment for osteoporosis in AD patients.
AD patients usually have a high fracture risk, which will further increase the morbidity and mortality of AD patients. Mechanisms of increased fracture risk are multifactorial in AD patients, including direct effects of amyloid pathology on bone cells, abnormal brain-bone interconnection, Wnt/β-catenin signalling deficits, reduced activity, increased falls and frailty, and chronic inflammatory status. Exercise, prevention of falls, and full nutrition are recommended in AD patients. Further studies are necessary to clarify the efficacy and safety of anti-osteoporotic agents in reducing fracture risk in AD patients.
ML contributed to conceptualization and study design of the research. B-NZ collected the data, analyzed data, and drafted the manuscript. QZ contributed to supervision and revision. All authors contributed to the article and approved the submitted version.
This study is supported by National Key R&D Program of China (2018YFA0800801, 2021YFC2501704), National Natural Science Foundation of China (No.82070908), CAMS Innovation Fund for Medical Sciences (CIFMS) (2021-I2M-C&T-B-007, 2021-I2M-1-051), and National High Level Hospital Clinical Research Funding (2022-PUMCH-B-014).
The authors declare that the research was conducted in the absence of any commercial or financial relationships that could be construed as a potential conflict of interest.
All claims expressed in this article are solely those of the authors and do not necessarily represent those of their affiliated organizations, or those of the publisher, the editors and the reviewers. Any product that may be evaluated in this article, or claim that may be made by its manufacturer, is not guaranteed or endorsed by the publisher.
2. Scheltens P, De Strooper B, Kivipelto M, Holstege H, Chételat G, Teunissen CE, et al. Alzheimer's disease. Lancet (London England) (2021) 397(10284):1577–90. doi: 10.1016/S0140-6736(20)32205-4
3. Collaborators GDF. Estimation of the global prevalence of dementia in 2019 and forecasted prevalence in 2050: an analysis for the Global Burden of Disease Study 2019. Lancet Public Health (2022) 7(2):e105–25. doi: 10.1016/S2468-2667(21)00249-8
4. Nandi A, Counts N, Chen S, Seligman B, Tortorice D, Vigo D, et al. Global and regional projections of the economic burden of Alzheimer's disease and related dementias from 2019 to 2050: A value of statistical life approach. EClinicalMedicine (2022) 51:101580. doi: 10.1016/j.eclinm.2022.101580
5. Melton LJ 3rd, Beard CM, Kokmen E, Atkinson EJ, O'Fallon WM. Fracture risk in patients with Alzheimer's disease. J Am Geriatrics Soc (1994) 42(6):614–9. doi: 10.1111/j.1532-5415.1994.tb06859.x
6. Baker NL, Cook MN, Arrighi HM, Bullock R. Hip fracture risk and subsequent mortality among Alzheimer's disease patients in the United Kingdom 1988-2007. Age Ageing (2011) 40(1):49–54. doi: 10.1093/ageing/afq146
7. Tolppanen AM, Taipale H, Tanskanen A, Tiihonen J, Hartikainen S. Comparison of predictors of hip fracture and mortality after hip fracture in community-dwellers with and without Alzheimer's disease - exposure-matched cohort study. BMC geriatrics (2016) 16(1):204. doi: 10.1186/s12877-016-0383-2
8. Liang Y, Wang L. Alzheimer's disease is an important risk factor of fractures: a meta-analysis of cohort studies. Mol Neurobiol (2017) 54(5):3230–5. doi: 10.1007/s12035-016-9841-2
9. Rajamaki B, Koponen M, Hartikainen S, Tolppanen AM. Length of hospital stay after hip fracture and readmission rates of persons with and without Alzheimer's disease: a matched cohort study. BMC geriatrics (2020) 20(1):214. doi: 10.1186/s12877-020-01609-5
10. Buchner DM, Larson EB. Falls and fractures in patients with Alzheimer-type dementia. Jama (1987) 257(11):1492–5. doi: 10.1001/jama.1987.03390110068028
11. Ansai JH, Andrade LP, Rossi PG, Takahashi ACM, Vale FAC, Rebelatto JR. Gait, dual task and history of falls in elderly with preserved cognition, mild cognitive impairment, and mild Alzheimer's disease. Braz J Phys Ther (2017) 21(2):144–51. doi: 10.1016/j.bjpt.2017.03.010
12. Sloan FA, Taylor DH Jr. Effect of Alzheimer disease on the cost of treating other diseases. Alzheimer Dis associated Disord (2002) 16(3):137–43. doi: 10.1097/00002093-200207000-00002
13. Weller I, Schatzker J. Hip fractures and Alzheimer's disease in elderly institutionalized Canadians. Ann Epidemiol (2004) 14(5):319–24. doi: 10.1016/j.annepidem.2003.08.005
14. Malone DC, McLaughlin TP, Wahl PM, Leibman C, Arrighi HM, Cziraky MJ, et al. Burden of Alzheimer's disease and association with negative health outcomes. Am J managed Care (2009) 15(8):481–8.
15. Lai SW, Chen YL, Lin CL, Liao KF. Alzheimer's disease correlates with greater risk of hip fracture in older people: a cohort in Taiwan. J Am Geriatrics Soc (2013) 61(7):1231–2. doi: 10.1111/jgs.12349
16. Tolppanen AM, Lavikainen P, Soininen H, Hartikainen S. Incident hip fractures among community dwelling persons with Alzheimer's disease in a Finnish nationwide register-based cohort. PloS One (2013) 8(3):e59124. doi: 10.1371/journal.pone.0059124
17. Jantzi M, Maher AC, Ioannidis G, Hirdes JP, Giangregorio LM, Papaioannou A. Individuals with neurological diseases are at increased risk of fractures within 180 days of admission to long-term care in Ontario. Age Ageing (2015) 44(2):252–7. doi: 10.1093/ageing/afu156
18. Saarelainen L, Tolppanen AM, Koponen M, Tanskanen A, Sund R, Tiihonen J, et al. Risk of hip fracture in benzodiazepine users with and without alzheimer disease. J Am Med Directors Assoc (2017) 18(1):87.e15–21. doi: 10.1016/j.jamda.2016.09.019
19. Torvinen-Kiiskinen S, Tolppanen AM, Koponen M, Tanskanen A, Tiihonen J, Hartikainen S, et al. Antidepressant use and risk of hip fractures among community-dwelling persons with and without Alzheimer's disease. Int J geriatric Psychiatry (2017) 32(12):e107–15. doi: 10.1002/gps.4667
20. Rajamaki B, Hartikainen S, Tolppanen AM. The effect of comorbidities on survival in persons with Alzheimer's disease: a matched cohort study. BMC geriatrics (2021) 21(1):173. doi: 10.1186/s12877-021-02130-z
21. Bloom GS. Amyloid-β and tau: the trigger and bullet in Alzheimer disease pathogenesis. JAMA Neurol (2014) 71(4):505–8. doi: 10.1001/jamaneurol.2013.5847
22. Lubitz I, Ricny J, Atrakchi-Baranes D, Shemesh C, Kravitz E, Liraz-Zaltsman S, et al. High dietary advanced glycation end products are associated with poorer spatial learning and accelerated Aβ deposition in an Alzheimer mouse model. Aging Cell (2016) 15(2):309–16. doi: 10.1111/acel.12436
23. Galasko D, Bell J, Mancuso JY, Kupiec JW, Sabbagh MN, van Dyck C, et al. Clinical trial of an inhibitor of RAGE-Aβ interactions in Alzheimer disease. Neurology (2014) 82(17):1536–42. doi: 10.1212/WNL.0000000000000364
24. Cui S, Xiong F, Hong Y, Jung JU, Li XS, Liu JZ, et al. APPswe/Aβ regulation of osteoclast activation and RAGE expression in an age-dependent manner. J Bone mineral Res (2011) 26(5):1084–98. doi: 10.1002/jbmr.299
25. Xia WF, Jung JU, Shun C, Xiong S, Xiong L, Shi XM, et al. Swedish mutant APP suppresses osteoblast differentiation and causes osteoporotic deficit, which are ameliorated by N-acetyl-L-cysteine. J Bone mineral Res (2013) 28(10):2122–35. doi: 10.1002/jbmr.1954
26. Pan JX, Tang F, Xiong F, Xiong L, Zeng P, Wang B, et al. APP promotes osteoblast survival and bone formation by regulating mitochondrial function and preventing oxidative stress. Cell Death Dis (2018) 9(11):1077. doi: 10.1038/s41419-018-1123-7
27. Li S, Liu B, Zhang L, Rong L. Amyloid beta peptide is elevated in osteoporotic bone tissues and enhances osteoclast function. Bone (2014) 61:164–75. doi: 10.1016/j.bone.2014.01.010
28. JE LL, Gil C, Amatya N, Lagalwar S, Possidente B, Vashishth D. Degradation of bone quality in a transgenic mouse model of alzheimer's disease. J Bone mineral Res (2022) 37(12):2548–65. doi: 10.1002/jbmr.4723
29. Lin Y, Chen T, Chen J, Fang Y, Zeng C. Endogenous Aβ induces osteoporosis through an mTOR-dependent inhibition of autophagy in bone marrow mesenchymal stem cells (BMSCs). Ann Trans Med (2021) 9(24):1794. doi: 10.21037/atm-21-6427
30. Huang S, Li Z, Liu Y, Gao D, Zhang X, Hao J, et al. Neural regulation of bone remodeling: Identifying novel neural molecules and pathways between brain and bone. J Cell Physiol (2019) 234(5):5466–77. doi: 10.1002/jcp.26502
31. Marenzana M, Chenu C. Sympathetic nervous system and bone adaptive response to its mechanical environment. J musculoskeletal neuronal Interact (2008) 8(2):111–20.
32. Dimitri P, Rosen C. The central nervous system and bone metabolism: an evolving story. Calcif Tissue Int (2017) 100(5):476–85. doi: 10.1007/s00223-016-0179-6
33. Otto E, Knapstein PR, Jahn D, Appelt J, Frosch KH, Tsitsilonis S, et al. Crosstalk of brain and bone-clinical observations and their molecular bases. Int J Mol Sci (2020) 21(14):4946. doi: 10.3390/ijms21144946
34. Schliebs R, Arendt T. The cholinergic system in aging and neuronal degeneration. Behav Brain Res (2011) 221(2):555–63. doi: 10.1016/j.bbr.2010.11.058
35. Tamimi I, Ojea T, Sanchez-Siles JM, Rojas F, Martin I, Gormaz I, et al. Acetylcholinesterase inhibitors and the risk of hip fracture in Alzheimer's disease patients: a case-control study. J Bone mineral Res (2012) 27(7):1518–27. doi: 10.1002/jbmr.1616
36. Luckhaus C, Mahabadi B, Grass-Kapanke B, Jänner M, Willenberg H, Jäger M, et al. Blood biomarkers of osteoporosis in mild cognitive impairment and Alzheimer's disease. J Neural Transm (Vienna Austria 1996) (2009) 116(7):905–11. doi: 10.1007/s00702-009-0241-x
37. Obri A, Khrimian L, Karsenty G, Oury F. Osteocalcin in the brain: from embryonic development to age-related decline in cognition. Nat Rev Endocrinol (2018) 14(3):174–82. doi: 10.1038/nrendo.2017.181
38. Yuan J, Meloni BP, Shi T, Bonser A, Papadimitriou JM, Mastaglia FL, et al. The potential influence of bone-derived modulators on the progression of alzheimer's disease. J Alzheimer's Dis JAD (2019) 69(1):59–70. doi: 10.3233/JAD-181249
39. Nusse R, Clevers H. Wnt/β-catenin signaling, disease, and emerging therapeutic modalities. Cell (2017) 169(6):985–99. doi: 10.1016/j.cell.2017.05.016
40. Krishnan V, Bryant HU, Macdougald OA. Regulation of bone mass by Wnt signaling. J Clin Invest (2006) 116(5):1202–9. doi: 10.1172/JCI28551
41. Oliva CA, Vargas JY, Inestrosa NC. Wnts in adult brain: from synaptic plasticity to cognitive deficiencies. Front Cell Neurosci (2013) 7:224. doi: 10.3389/fncel.2013.00224
42. Inestrosa NC, Varela-Nallar L. Wnt signaling in the nervous system and in Alzheimer's disease. J Mol Cell Biol (2014) 6(1):64–74. doi: 10.1093/jmcb/mjt051
43. Dengler-Crish CM, Ball HC, Lin L, Novak KM, Cooper LN. Evidence of Wnt/β-catenin alterations in brain and bone of a tauopathy mouse model of Alzheimer's disease. Neurobiol Aging (2018) 67:148–58. doi: 10.1016/j.neurobiolaging.2018.03.021
44. Marini F, Giusti F, Palmini G, Brandi ML. Role of Wnt signaling and sclerostin in bone and as therapeutic targets in skeletal disorders. Osteoporos Int (2023) 34(2):213–38. doi: 10.1007/s00198-022-06523-7
45. Caricasole A, Copani A, Caraci F, Aronica E, Rozemuller AJ, Caruso A, et al. Induction of Dickkopf-1, a negative modulator of the Wnt pathway, is associated with neuronal degeneration in Alzheimer's brain. J Neurosci (2004) 24(26):6021–7. doi: 10.1523/JNEUROSCI.1381-04.2004
46. Hammond TR, Marsh SE, Stevens B. Immune signaling in neurodegeneration. Immunity (2019) 50(4):955–74. doi: 10.1016/j.immuni.2019.03.016
47. Zenaro E, Piacentino G, Constantin G. The blood-brain barrier in Alzheimer's disease. Neurobiol Dis (2017) 107:41–56. doi: 10.1016/j.nbd.2016.07.007
48. Shen XN, Niu LD, Wang YJ, Cao XP, Liu Q, Tan L, et al. Inflammatory markers in Alzheimer's disease and mild cognitive impairment: a meta-analysis and systematic review of 170 studies. J neurol neurosurgery Psychiatry (2019) 90(5):590–8. doi: 10.1136/jnnp-2018-319148
49. Chang R, Yee KL, Sumbria RK. Tumor necrosis factor α Inhibition for Alzheimer's Disease. J Cent nervous system Dis (2017) 9:1179573517709278. doi: 10.1177/1179573517709278
50. Dengler-Crish CM, Smith MA, Wilson GN. Early evidence of low bone density and decreased serotonergic synthesis in the dorsal raphe of a tauopathy model of alzheimer's disease. J Alzheimer's Dis JAD (2017) 55(4):1605–19. doi: 10.3233/JAD-160658
51. Gilbert L, He X, Farmer P, Boden S, Kozlowski M, Rubin J, et al. Inhibition of osteoblast differentiation by tumor necrosis factor-alpha. Endocrinology (2000) 141(11):3956–64. doi: 10.1210/endo.141.11.7739
52. Kaneki H, Guo R, Chen D, Yao Z, Schwarz EM, Zhang YE, et al. Tumor necrosis factor promotes Runx2 degradation through up-regulation of Smurf1 and Smurf2 in osteoblasts. J Biol Chem (2006) 281(7):4326–33. doi: 10.1074/jbc.M509430200
53. Gilbert LC, Chen H, Lu X, Nanes MS. Chronic low dose tumor necrosis factor-α (TNF) suppresses early bone accrual in young mice by inhibiting osteoblasts without affecting osteoclasts. Bone (2013) 56(1):174–83. doi: 10.1016/j.bone.2013.06.002
54. Rani V, Verma R, Kumar K, Chawla R. Role of pro-inflammatory cytokines in Alzheimer's disease and neuroprotective effects of pegylated self-assembled nanoscaffolds. Curr Res Pharmacol Drug Discovery (2023) 4:100149. doi: 10.1016/j.crphar.2022.100149
55. Mundy GR. Osteoporosis and inflammation. Nutr Rev (2007) 65(12 Pt 2):S147–151. doi: 10.1111/j.1753-4887.2007.tb00353.x
56. Friedland RP, Fritsch T, Smyth KA, Koss E, Lerner AJ, Chen CH, et al. Patients with Alzheimer's disease have reduced activities in midlife compared with healthy control-group members. Proc Natl Acad Sci United States America (2001) 98(6):3440–5. doi: 10.1073/pnas.061002998
57. Bikle DD. Integrins, insulin like growth factors, and the skeletal response to load. Osteoporos Int (2008) 19(9):1237–46. doi: 10.1007/s00198-008-0597-z
58. Rolvien T, Amling M. Disuse osteoporosis: clinical and mechanistic insights. Calcif Tissue Int (2022) 110(5):592–604. doi: 10.1007/s00223-021-00836-1
59. Allan LM, Ballard CG, Rowan EN, Kenny RA. Incidence and prediction of falls in dementia: a prospective study in older people. PloS One (2009) 4(5):e5521. doi: 10.1371/journal.pone.0005521
60. Harvey L, Mitchell R, Brodaty H, Draper B, Close J. The influence of dementia on injury-related hospitalisations and outcomes in older adults. Injury (2016) 47(1):226–34. doi: 10.1016/j.injury.2015.09.021
61. Wang C, Chong Y, Wang L, Wang Y. The correlation between falls and cognitive frailty in elderly individuals with hypertension in a chinese community. Front Aging Neurosci (2022) 14:783461. doi: 10.3389/fnagi.2022.783461
62. Kojima G, Liljas A, Iliffe S, Walters K. Prevalence of frailty in mild to moderate alzheimer's disease: A systematic review and meta-analysis. Curr Alzheimer Res (2017) 14(12):1256–63. doi: 10.2174/1567205014666170417104236
63. Gómez-Gómez ME, Zapico SC. Frailty, cognitive decline, neurodegenerative diseases and nutrition interventions. Int J Mol Sci (2019) 20(11):2842. doi: 10.3390/ijms20112842
64. Chu W, Chang SF, Ho HY. Adverse health effects of frailty: systematic review and meta-analysis of middle-aged and older adults with implications for evidence-based practice. Worldviews Evidence-Based Nurs (2021) 18(4):282–9. doi: 10.1111/wvn.12508
65. Abay RJY, Gold LS, Cawthon PM, Andrews JS. Lean mass, grip strength, and hospital-associated disability among older adults in Health ABC. Alzheimer's dementia (2022) 18(10):1898–906. doi: 10.1002/alz.12527
66. Burton E, Cavalheri V, Adams R, Browne CO, Bovery-Spencer P, Fenton AM, et al. Effectiveness of exercise programs to reduce falls in older people with dementia living in the community: a systematic review and meta-analysis. Clin Interventions Aging (2015) 10:421–34. doi: 10.2147/CIA.S71691
67. Jia RX, Liang JH, Xu Y, Wang YQ. Effects of physical activity and exercise on the cognitive function of patients with Alzheimer disease: a meta-analysis. BMC geriatrics (2019) 19(1):181. doi: 10.1186/s12877-019-1175-2
68. Palombaro KM, Black JD, Buchbinder R, Jette DU. Effectiveness of exercise for managing osteoporosis in women postmenopause. Phys Ther (2013) 93(8):1021–5. doi: 10.2522/ptj.20110476
69. Gonzalo-Encabo P, McNeil J, Boyne DJ, Courneya KS, Friedenreich CM. Dose-response effects of exercise on bone mineral density and content in post-menopausal women. Scandinavian J Med Sci sports (2019) 29(8):1121–9. doi: 10.1111/sms.13443
70. Alkhouli MF, Hung J, Squire M, Anderson M, Castro M, Babu JR, et al. Exercise and resveratrol increase fracture resistance in the 3xTg-AD mouse model of Alzheimer's disease. BMC complementary Altern Med (2019) 19(1):39. doi: 10.1186/s12906-019-2451-6
71. Puente-González AS, Sánchez-Sánchez MC, Fernández-Rodríguez EJ, Hernández-Xumet JE, Barbero-Iglesias FJ, Méndez-Sánchez R. Effects of 6-month multimodal physical exercise program on bone mineral density, fall risk, balance, and gait in patients with alzheimer's disease: A controlled clinical trial. Brain Sci (2021) 11(1):63. doi: 10.3390/brainsci11010063
72. Muir SW, Gopaul K, Montero Odasso MM. The role of cognitive impairment in fall risk among older adults: a systematic review and meta-analysis. Age Ageing (2012) 41(3):299–308. doi: 10.1093/ageing/afs012
73. Montero-Odasso M, van der Velde N, Martin FC, Petrovic M, Tan MP, Ryg J, et al. World guidelines for falls prevention and management for older adults: a global initiative. Age Ageing (2022) 51(9):afac205. doi: 10.1093/ageing/afac205
74. Lopes da Silva S, Vellas B, Elemans S, Luchsinger J, Kamphuis P, Yaffe K, et al. Plasma nutrient status of patients with Alzheimer's disease: Systematic review and meta-analysis. Alzheimer's dementia (2014) 10(4):485–502. doi: 10.1016/j.jalz.2013.05.1771
75. Power R, Prado-Cabrero A, Mulcahy R, Howard A, Nolan JM. The role of nutrition for the aging population: implications for cognition and alzheimer's disease. Annu Rev Food Sci Technol (2019) 10:619–39. doi: 10.1146/annurev-food-030216-030125
76. Rizzoli R, Biver E, Brennan-Speranza TC. Nutritional intake and bone health. Lancet Diabetes Endocrinol (2021) 9(9):606–21. doi: 10.1016/S2213-8587(21)00119-4
77. Compston JE, McClung MR, Leslie WD. Osteoporosis. Lancet (London England) (2019) 393(10169):364–76. doi: 10.1016/S0140-6736(18)32112-3
78. DeLuca GC, Kimball SM, Kolasinski J, Ramagopalan SV, Ebers GC. Review: the role of vitamin D in nervous system health and disease. Neuropathol Appl Neurobiol (2013) 39(5):458–84. doi: 10.1111/nan.12020
79. Melo van Lent D, Egert S, Wolfsgruber S, Kleineidam L, Weinhold L, Wagner-Thelen H, et al. Low serum vitamin D status is associated with incident alzheimer's dementia in the oldest old. Nutrients (2022) 15(1):61. doi: 10.3390/nu15010061
80. Jia J, Hu J, Huo X, Miao R, Zhang Y, Ma F. Effects of vitamin D supplementation on cognitive function and blood Aβ-related biomarkers in older adults with Alzheimer's disease: a randomised, double-blind, placebo-controlled trial. J neurol neurosurgery Psychiatry (2019) 90(12):1347–52. doi: 10.1136/jnnp-2018-320199
81. Haasum Y, Fastbom J, Fratiglioni L, Johnell K. Undertreatment of osteoporosis in persons with dementia? A population-based study. Osteoporos Int (2012) 23(3):1061–8. doi: 10.1007/s00198-011-1636-8
82. Favus MJ. Bisphosphonates for osteoporosis. New Engl J Med (2010) 363(21):2027–35. doi: 10.1056/NEJMct1004903
83. Zameer S, Najmi AK, Vohora D, Akhtar M. Bisphosphonates: Future perspective for neurological disorders. Pharmacol Rep PR (2018) 70(5):900–7. doi: 10.1016/j.pharep.2018.03.011
84. Tiihonen M, Taipale H, Tanskanen A, Tiihonen J, Hartikainen S. Incidence and Duration of Cumulative Bisphosphonate Use among Community-Dwelling Persons with or without Alzheimer's Disease. J Alzheimer's Dis JAD (2016) 52(1):127–32. doi: 10.3233/JAD-151181
85. Zullo AR, Zhang T, Lee Y, McConeghy KW, Daiello LA, Kiel DP, et al. Effect of bisphosphonates on fracture outcomes among frail older adults. J Am Geriatrics Soc (2019) 67(4):768–76. doi: 10.1111/jgs.15725
86. Smith MR, Egerdie B, Hernández Toriz N, Feldman R, Tammela TL, Saad F, et al. Denosumab in men receiving androgen-deprivation therapy for prostate cancer. New Engl J Med (2009) 361(8):745–55. doi: 10.1056/NEJMoa0809003
87. Bone HG, Wagman RB, Brandi ML, Brown JP, Chapurlat R, Cummings SR, et al. 10 years of denosumab treatment in postmenopausal women with osteoporosis: results from the phase 3 randomised FREEDOM trial and open-label extension. Lancet Diabetes Endocrinol (2017) 5(7):513–23. doi: 10.1016/S2213-8587(17)30138-9
88. Saag KG, Pannacciulli N, Geusens P, Adachi JD, Messina OD, Morales-Torres J, et al. Denosumab versus risedronate in glucocorticoid-induced osteoporosis: final results of a twenty-four-month randomized, double-blind, double-dummy trial. Arthritis Rheumatol (Hoboken N.J.) (2019) 71(7):1174–84. doi: 10.1002/art.40874
89. Kendler DL, Marin F, Zerbini CAF, Russo LA, Greenspan SL, Zikan V, et al. Effects of teriparatide and risedronate on new fractures in post-menopausal women with severe osteoporosis (VERO): a multicentre, double-blind, double-dummy, randomised controlled trial. Lancet (London England) (2018) 391(10117):230–40. doi: 10.1016/S0140-6736(17)32137-2
90. Hagino H, Sugimoto T, Tanaka S, Sasaki K, Sone T, Nakamura T, et al. A randomized, controlled trial of once-weekly teriparatide injection versus alendronate in patients at high risk of osteoporotic fracture: primary results of the Japanese Osteoporosis Intervention Trial-05. Osteoporos Int (2021) 32(11):2301–11. doi: 10.1007/s00198-021-05996-2
91. Zullo AR, Lee Y, Lary C, Daiello LA, Kiel DP, Berry SD. Comparative effectiveness of denosumab, teriparatide, and zoledronic acid among frail older adults: a retrospective cohort study. Osteoporos Int (2021) 32(3):565–73. doi: 10.1007/s00198-020-05732-2
92. Geusens P, Appelman-Dijkstra N, Lems W, van den Bergh J. Romosozumab for the treatment of postmenopausal women at high risk of fracture. Expert Opin Biol Ther (2023) 23(1):11–9. doi: 10.1080/14712598.2022.2152320
Keywords: Alzheimer’s disease, bone fracture, pathological mechanisms, prevention, treatment
Citation: Zhou B-N, Zhang Q and Li M (2023) Alzheimer’s disease and its associated risk of bone fractures: a narrative review. Front. Endocrinol. 14:1190762. doi: 10.3389/fendo.2023.1190762
Received: 19 May 2023; Accepted: 21 July 2023;
Published: 09 August 2023.
Edited by:
Xingming Shi, Augusta University, United StatesReviewed by:
Ida Cariati, Università di Roma Tor Vergata, ItalyCopyright © 2023 Zhou, Zhang and Li. This is an open-access article distributed under the terms of the Creative Commons Attribution License (CC BY). The use, distribution or reproduction in other forums is permitted, provided the original author(s) and the copyright owner(s) are credited and that the original publication in this journal is cited, in accordance with accepted academic practice. No use, distribution or reproduction is permitted which does not comply with these terms.
*Correspondence: Mei Li, bGltZWlsemhAc2luYS5jb20=
†ORCID: Mei Li, orcid.org/0000-0001-7270-4902
Disclaimer: All claims expressed in this article are solely those of the authors and do not necessarily represent those of their affiliated organizations, or those of the publisher, the editors and the reviewers. Any product that may be evaluated in this article or claim that may be made by its manufacturer is not guaranteed or endorsed by the publisher.
Research integrity at Frontiers
Learn more about the work of our research integrity team to safeguard the quality of each article we publish.