- 1Department of Endocrinology, Guang’anmen Hospital, China Academy of Chinese Medical Sciences, Beijing, China
- 2Department of Emergency, Guang’anmen Hospital, China Academy of Chinese Medical Sciences, Beijing, China
- 3Department of Traditional Chinese Medicine, The Seventh Hospital of Xingtai, Xingtai, Heibei, China
Diabetic nephropathy (DN) is a serious microvascular complication of diabetes. It has become a leading cause of death in patients with diabetes and end-stage renal disease. Ferroptosis is a newly discovered pattern of programmed cell death. Its main manifestation is the excessive accumulation of intracellular iron ion-dependent lipid peroxides. Recent studies have shown that ferroptosis is an important driving factor in the onset and development of DN. Ferroptosis is closely associated with renal intrinsic cell (including renal tubular epithelial cells, podocytes, and mesangial cells) damage in diabetes. Chinese herbal medicine is widely used in the treatment of DN, with a long history and definite curative effect. Accumulating evidence suggests that Chinese herbal medicine can modulate ferroptosis in renal intrinsic cells and show great potential for improving DN. In this review, we outline the key regulators and pathways of ferroptosis in DN and summarize the herbs, mainly monomers and extracts, that target the inhibition of ferroptosis.
1 Introduction
Diabetes mellitus is one of the major diseases that seriously endanger human health. According to the International Diabetes Federation, 536.6 million adults aged 20 to 79 had diabetes mellitus worldwide in 2021, and that number is expected to rise to 783.2 million by 2045 (1). Diabetic nephropathy (DN) is one of the major microvascular complications of diabetes mellitus and the most common cause of end-stage renal disease (2). According to statistics, DN occurs in 20% to 40% of patients with type 1 and type 2 diabetes mellitus (3). About 30% to 50% of end-stage renal disease worldwide is caused by DN (4). Previous studies have shown that hyperglycemia, hypertension, dyslipidemia, and being overweight or obese are common risk factors for DN (5). Therefore, lifestyle changes, aggressive glycemic control, blood pressure reduction, lipid regulation, and reduction of proteinuria are currently the main measures in the clinical management of DN. In the past twenty years, angiotensin-converting enzyme inhibitors (ACEI) and angiotensin receptor blockers (ARB) have shown good renoprotective effects in patients with DN. In recent years, newer drugs, including sodium-glucose co-transporter 2 inhibitors, glucagon-like peptide-1 receptor agonists, mineralocorticoid receptor antagonists, and endothelin antagonists, have also provided more options for the treatment of DN (6). However, some DN patients still progress to end-stage renal disease. Hence, finding new targets for the prevention and treatment of DN has become an urgent problem in this field.
The pathogenesis of DN has not been fully elucidated. Various pathogenic mechanisms such as oxidative stress (7), inflammatory response (8), the accumulation of advanced glycation end products (9, 10), endoplasmic reticulum stress (11), autophagy (12), and pyroptosis (13) are involved in the pathological process of DN. Ferroptosis is a newly discovered iron-dependent novel form of programmed cell death that differs morphologically, biochemically, and genetically from apoptosis, autophagy, and necrosis (14). The occurrence of ferroptosis in cells is usually accompanied by a large accumulation of iron and lipid peroxidation (15). Increased iron accumulation, free radical production, fatty acid supply, and lipid peroxidation are currently thought to be key to the induction of ferroptosis. Previous studies have shown that renal iron overload is prevalent in diabetic animal models, and even serum creatinine and urine protein levels are positively correlated with renal iron and ferritin levels (16, 17). Excess iron not only increased oxidative/nitrative stress and decreased antioxidant capacity but also activated the renin-angiotensin system, thereby promoting the progression of DN (17, 18). Since the presence of ferroptosis in DN was first reported by Wang et al. (19) in 2020. With the advancement of research, accumulating evidence suggests that ferroptosis is an important factor in promoting the occurrence and development of DN (20). Therefore, targeted inhibition of ferroptosis is expected to be a new tool for the treatment of DN. In recent years, Chinese herbal medicine has played an increasingly important role in the prevention and treatment of DN. Several clinical studies have shown that either the use of Chinese herbal medicine alone or in combination with ACEI or ARB helps to improve renal function and reduce proteinuria in patients with DN (21–23). More importantly, there is a growing body of research on Chinese herbal medicine to prevent and treat DN by regulating ferroptosis. This paper reviews the key regulators and signaling pathways of ferroptosis in DN and the research progress of Chinese herbal medicine in the treatment of DN by regulating ferroptosis, in order to provide a new research direction for the treatment of DN.
2 Key regulators and signaling pathways of ferroptosis in diabetic nephropathy
Ferroptosis in renal intrinsic cells is prevalent in the pathological process of DN. Previous studies have demonstrated that it is regulated by a variety of regulatory factors and signal transduction pathways (Figure 1; Supplementary Table 1). We have summarized these in detail.
2.1 Non-coding RNAs
It is well known that although non-coding RNAs are not directly involved in the protein translation process, they can produce non-coding transcripts that regulate gene expression and protein function (24). The most studied non-coding RNAs are microRNA, long non-coding RNA, and circular RNA. Previous studies have found that non-coding RNAs are widely involved in the biological processes of renal intrinsic cells, including cell proliferation (25), apoptosis (26), cellular senescence (27, 28), autophagy (29, 30), and pyroptosis (31, 32). Clinical data have confirmed that non-coding RNAs are important biomarkers for the early diagnosis and prognosis of patients with diabetic kidney disease (33, 34). The emerging evidence has shown that non-coding RNAs are important regulators of ferroptosis (35, 36). Li et al. (37) found that circASAP2 expression was downregulated and miR-770-5p expression was upregulated in DN mouse serum, kidney, and high-glucose cultured HK-2 cells. Upregulation of miR-770-5p suppressed the target gene SOX2 and its induced SLC7A11 expression, thereby promoting inflammation and ferroptosis. Xiong et al. (38) observed that hsa_circ_0006370 expression was significantly upregulated in HK-2 cells cultured with high glucose, while interference with hsa_circ_0006370 expression significantly increased the survival of HK-2 cells. Mechanistically, hsa_circ_0006370 silencing attenuated ferroptosis by inhibiting intracellular reactive oxygen species (ROS) production, enhancing antioxidant capacity, reducing iron ion levels, and regulating ferroptosis-associated protein expression. Therefore, circASAP2 and hsa_circ_0006370 may be potential intervention targets for DN ferroptosis.
2.2 Cyclooxygenase-2
Cyclooxygenase (COX), also known as prostaglandin synthase, is the key rate-limiting enzyme that catalyzes the conversion of arachidonic acid to prostaglandins. At least three isoforms of COX have been identified, namely COX-1, COX-2, and COX-3 (39). COX-3 is poorly studied, and COX-1 is constitutively expressed in most tissues and cells (40). COX-2 is an inducible enzyme that is less expressed in normal tissues. However, COX-2 is abundantly expressed in certain inflammatory stimuli, such as cytokines, tumor inducers, oncogenes, etc. (41, 42). In recent years, numerous studies have confirmed that COX-2 expression is significantly increased in mesangial cells, podocytes, and renal tubular epithelial cells induced by high glucose, as well as in the kidney of animal models of diabetes (43–47). Wei et al. (43) pointed out that activation of the c-Src/NF-κB/COX-2 pathway in the high-glucose state promoted extracellular matrix accumulation and mesangial proliferation, which exacerbated glomerulosclerosis. Ma et al. (48) found a positive correlation between COX-2 expression levels and low-density lipoprotein receptor expression levels in the kidney of diabetic rats. Increased COX-2 expression exacerbated renal lipid accumulation and inflammatory cytokine production, including interleukin-1beta (IL-1β), interleukin 6 (IL-6), tumor necrosis factor alpha (TNF-α), and monocyte chemoattractant protein-1 (MCP-1). In addition, COX-2 also contributes to diabetic nephropathy through the glomerular EP4 receptor (49). The latest report showed that increased COX-2 expression was accompanied by ferroptosis, while COX-2 knockdown reduced the sensitivity of renal tubular cells to ferroptosis in the high glucose state (47).
2.3 Arachidonate 15-lipoxygenase
Lipoxygenases are non heme-iron containing dioxygenases, and arachidonate 15-lipoxygenase (ALOX15) is a member of its family (50). Several studies have shown that ALOX15 is a key mediator in the formation of lipid peroxidation products and ultimately leads to ferroptosis (51, 52). In tert-butyl hydroperoxide-induced primary cortical neurons, Zhao et al. (53) observed that ALOX15 inhibition reversed SSAT1 upregulation-triggered neuronal loss and ferroptosis. Similarly, Gao et al. (54) found that inhibition of lipid peroxidation by downregulating the expression of ALOX15 contributed to the attenuation of ferroptosis in microglia and endothelial cells after subarachnoid hemorrhage. In addition, both the selective ALOX15 inhibitor PD146176 and siRNA-mediated silencing of ALOX15 significantly reduced ferroptosis in erastin-induced and RSL3-induced cancer cells (e.g., HT1080, Calu-1). Conversely, ALOX15 activators enhanced the sensitivity of cancer cells to these compounds (52). In recent years, ALOX15 has been extensively studied in diabetes and its complications (55–58). In the field of DN, Kim et al. (59) found that the 12/15-lipoxygenase and TGF-β pathways can cross-talk and activate each other. Another study showed that 12/15-lipoxygenase knockout reduced the expression of SET7 and profibrotic genes, which in turn improved proteinuria and renal pathological changes in diabetic mice (60). It was recently reported that ALOX15 protein expression was significantly increased in the renal tissues of streptozotocin (STZ)-induced diabetic mice, db/db mice, and DN patients. Correlation analysis suggested that ALOX15 protein expression was closely associated with renal pathological changes in DN patients, such as glomerular lesions, interstitial fibrosis and tubular atrophy, and interstitial inflammation. More importantly, immunofluorescence double staining also observed glutathione peroxidase 4 (GPX4) and ALOX15 expression in the same region. This study confirmed that ALOX15 is an important factor involved in DN ferroptosis (58). Therefore, targeting ALOX15 provides a new idea for the intervention of DN ferroptosis.
2.4 Peroxiredoxin 6
Peroxidases (Prdxs) are a family of antioxidant proteins widely found in prokaryotes and eukaryotes. Peroxidase 6 (Prdx6) is the only member of the Prdxs family that belongs to the 1-cysteine subclass. Unlike other Prdxs, Prdx6 is a bifunctional enzyme with both glutathione peroxidase and phospholipase A2 activities (61). It has been found that Prdx6 is widely expressed in human and mouse kidneys. Moreover, Prdx6 is involved in the maintenance of PH homeostasis by interacting with anion exchanger 1 (62). Another study showed that Prdx6 overexpression attenuated lipopolysaccharide-induced apoptosis in primary renal proximal tubule cells by scavenging ROS (63). Bioinformatics analysis showed that seven hub ferroptosis-related genes, including Prdx6, could accurately distinguish between diabetic kidney disease and control samples (64). Zhang et al. (65) found that Prdx6 was significantly downregulated in high glucose-induced podocytes and in the kidneys of DN mice. Overexpression of Prdx6 in vivo reduced urinary protein, blood creatinine, and urea nitrogen in DN mice. In vitro, Prdx6 overexpression ameliorated podocyte dysfunction by attenuating high glucose-induced oxidative stress and ferroptosis. Therefore, enhancing the activity of Prdx6 is an important way to alleviate ferroptosis in high glucose-induced podocytes.
2.5 ZRT/IRT-like protein 14
ZRT/IRT-like protein 14 (ZIP14, also known as SLC39A14), a member of the solute-linked carrier 39 family, is a transporter protein that mediates cellular uptake of zinc (66), manganese (67), and iron (68, 69). As an essential trace element, zinc and its carrier protein are closely related to the synthesis, secretion, and biological activity of insulin (70). Previous studies have shown the presence of ZIP14 in pancreatic β-cells, and modulation of ZIP14 activity is expected to be an important target for improving β-cell dysfunction (71). Lawson et al. (72) discovered that prolonged high glucose stimulation disrupted the expression of several ZIP transporter proteins, including SLC39A6, SLC39A7, and SLC39A14, depleted zinc from pancreatic β-cells, and resulted in the loss of β-cell markers, thereby promoting β-cell dedifferentiation. Maxel et al. (71) demonstrated that silencing of ZIP14 in pancreatic β-cells affects insulin processing and disturbs mitochondrial function. Recent studies have found that ZIP14 can be detected in both the proximal and distal tubules of the human kidney (73). In human proximal tubule epithelial cells, ZIP14 is involved not only in non-transferrin-bound iron uptake but also in transferrin-bound iron-derived iron uptake (74). Tubular iron deposition is common in chronic kidney disease, with glomerular dysfunction as the primary pathology, accompanied by increased ZIP14 expression in the proximal and distal tubules (73). Iron overload is both a major source of ROS and a key participant in ferroptosis (75). The emerging evidence has shown that ZIP14 expression was upregulated in the renal tubules of both DN patients and DN rats, as well as in HK-2 cells cultured with high glucose. Increased ZIP14 promotes iron deposition and is involved in diabetic kidney injury via ferroptosis (76). This study confirms a novel role of ZIP14 in ferroptosis in DN. Thus, ZIP14 may become an important pharmacological target for the treatment of DN.
2.6 High mobility group box 1
High mobility group box 1 (HMGB1), a highly conserved nuclear protein, is widely distributed in mammalian cells. As a DNA binding protein, nuclear HMBG1 is involved in a series of key nuclear events, such as nucleosome stability and sliding, gene transcription, DNA replication, DNA repair, gene transfer, gene delivery, etc. (77–79). Extracellularly, HMGB1 acts as a damage-associated molecular pattern molecule involved in cell differentiation, inflammation, immunity, cell migration, tissue regeneration, and other biological processes (77, 79, 80). Additionally, cytoplasmic HMBG1 is important for autophagy regulation (81). DN is a chronic inflammatory disease in which low-grade inflammation and the immune response are important mechanisms in its pathogenesis (82–85). HMGB1, an important mediator that can participate in the inflammatory response, drives the development of DN. Clinical data have shown that serum HMGB1 is closely associated with urinary albumin excretion and renal tubular damage in patients with type 2 diabetes (86). Preclinical studies have demonstrated that HMGB1 promotes the expression of markers of oxidative stress, inflammation, and fibrosis in DN cell models (87, 88). Knockdown of HMGB1 or inhibition of HMGB1-mediated inflammatory signaling pathways (e.g., HMGB1-RAGE-NF-κB and HMGB1-TLR4) significantly attenuated podocyte apoptosis, epithelial mesenchymal transition, renal inflammation, and fibrotic processes (87, 89, 90). Recent studies have revealed that HMGB1 is also an important regulator of ferroptosis (91, 92). Both type I and type II ferroptosis activators, including erastin, sorafenib, RSL3, and FIN56, induced the release of HMGB1 (93). HMGB1 could regulate ferroptosis through the RAS-JNK/p38 pathway (94). Zhao et al. (95) found that cytoplasmic HMGB1 induced renal tubular ferroptosis through regulation of acyl-CoA synthetase long-chain family member 4 (ACSL4). In HL-60/NRASQ61L cells, Ye et al. (94) observed that knockdown of HMGB1 inhibited erastin-induced ROS and malondialdehyde (MDA) production, degradation of GPX4, and cell death in an iron-mediated lysosomal pathway. It has been recently reported that serum HMGB1 expression is elevated in DN patients compared to healthy controls, accompanied by an increase in ferroptosis. Further studies revealed that knockdown of HMGB1 significantly inhibited high glucose-induced ferroptosis in mesangial cells. Mechanistically, silencing of HMGB1 not only decreased the expression of ACSL4, prostaglandin-endoperoxide synthase 2 (PTGS2), and NADPH oxidase 1 (NOX1), but also increased the level of GPX4 (96). This study suggests that HMGB1 inhibition may have potential therapeutic value for ferroptosis-associated DN.
2.7 Nuclear factor erythroid 2-related factor 2
Nuclear factor erythroid 2-related factor 2 (Nrf2) is a key transcription factor in the body’s antioxidant stress response. In addition to antioxidant responses, Nrf2 is involved in the regulation of a variety of other biological processes, including drug detoxification, amino acid metabolism, lipid metabolism, heme and iron metabolism, autophagy, unfolded protein response, inflammation, and immunity (97). Several studies have found significantly lower circulating Nrf2 levels in DN patients compared to healthy controls (96, 98). Nrf2 deficiency resulted in more severe diabetic symptoms, renal inflammation, and interstitial renal fibrosis in Akita diabetic model mice (99). Conversely, Nrf2 activation delayed DN progression by increasing the expression of the downstream antioxidant enzymes HO-1 and NADPH quinone oxidoreductase-1 (NQO-1) (100, 101). Ferroptosis is a new regulatory cell death mode. Three key events are required for its occurrence: iron deposition, lipid peroxidation, and glutathione depletion (102). Accumulating studies confirm that Nrf2 is an important regulator of ferroptosis (103, 104). Many components that are involved in the prevention of lipid peroxidation and the regulation of cellular iron homeostasis are target genes of Nrf2 (105–107). New evidence suggests that specific knockdown of Nrf2 enhanced the susceptibility of HK-2 cells to ferroptosis under high glucose conditions, while upregulation of Nrf2 expression contributed to the inhibition of ferroptosis and thus reduced kidney injury in diabetic mice (108). Another study found that high glucose promoted salusin-β expression in HK-2 cells in a time- and dose-dependent manner. Upregulated salusin-β mediated ferroptosis in renal tubular epithelial cells by inhibiting the Nrf2 expression (109). Taken together, the above results demonstrate that Nrf2 is an important regulator of ferroptosis in DN.
2.8 GPX4
GPX4 is a unique antioxidant enzyme in mammals. Functionally, GPX4 converts lipid hydroperoxides to non-toxic lipid alcohols. This limits the propagation of lipid peroxidation in the membrane (110). GPX4 is a key and central regulator of ferroptosis, as is now generally accepted (111, 112). Overexpressing and knocking down GPX4 modulates the lethality of 12 ferroptosis inducers (113). In addition, GPX4 is closely associated with kidney damage. Inducible GPX4 disruption was reported to cause acute renal failure and death in mice as early as 2014 (114). In recent years, GPX4 has gradually become the star molecule in the DN field. A number of studies have shown that the expression of GPX4 is significantly lower in kidney biopsy samples from patients with diabetic kidney disease (115–117). Moreover, reduced GPX4 levels were associated with the severity of diabetic kidney disease and an increased risk of progression to end-stage renal disease (117). A recent study found that activation of the SIRT3-SOD2-GPX4 signaling pathway, including upregulation of sirtuin 3 (SIRT3) expression, inhibition of superoxide dismutase 2 (SOD2) acetylation, and consequently promotion of GPX4 expression, helped restore mitochondrial redox homeostasis, thereby alleviating ferroptosis in DN models (118).
2.9 HIF-1α/HO-1 pathway
Hypoxia-inducible factor-1alpha (HIF-1α) is an important regulator of cellular oxygen homeostasis. Previous studies have found that HIF-1α is aberrantly expressed in the serum of diabetic patients and in the kidneys of DN patients and is significantly associated with the progression of interstitial renal fibrosis (119–121). HIF-1α, as a transcription factor, is able to regulate the expression of its downstream target, heme oxygenase-1 (HO-1) (122). It has been reported that deletion of HIF-1α in proximal renal tubule cells exacerbates mitochondrial dysfunction and renal injury in diabetic mice via the HO-1 pathway (123). A number of studies have also shown that the HIF-1α/HO-1 signaling pathway plays an important role in the regulation of ferroptosis (124–126). Destabilization of HIF-1α enhanced the sensitivity of gastric cancer cells to erastin and RSL3 (127). Furthermore, HO-1 regulates ROS production and iron metabolism (128). HO-1 deficiency promotes erastin-induced ferroptosis (129). Another study showed that HO-1 in renal proximal tubule cells is similarly antiferroptotic (130). Diabetes promoted renal ROS formation, lipid peroxidation, and renal tubular iron overload. However, this phenomenon can be blocked by the ferroptosis inhibitor Ferrostatin-1. Further studies revealed that ferroptosis may exacerbate proteinuria, tubular injury, and renal fibrosis in db/db mice via the HIF-1α/HO-1 pathway (131). Therefore, targeting the HIF-1α/HO-1 signaling pathway and regulating ferroptosis may be an effective strategy for improving DN.
The above findings suggest that ferroptosis is closely associated with renal intrinsic cell death in diabetic conditions, mainly in renal tubular epithelial cells, mesangial cells, and podocytes. Non-coding RNAs (e.g., circASAP2, hsa_circ_0006370), COX-2, ALOX15, Prdx6, etc. are important regulators of ferroptosis in renal cells. HIF-1α/HO-1 is a critical regulatory pathway. Therefore, an in-depth exploration of the role of these regulators and signaling pathways in ferroptosis is important for both understanding the pathogenesis of DN and finding new drug targets.
3 Modulatory role of Chinese herbal medicine in ferroptosis in diabetic nephropathy
A variety of natural compounds and extracts from Chinese herbal medicine have been reported to delay the progression of DN by inhibiting ferroptosis (Figure 2; Supplementary Table 2). Common natural compounds include flavonoids, terpenoids, anthraquinones, alkaloids, etc. (Figure 3). We will comprehensively review the anti-ferroptotic properties of Chinese herbal medicine and its potential in the treatment of DN.
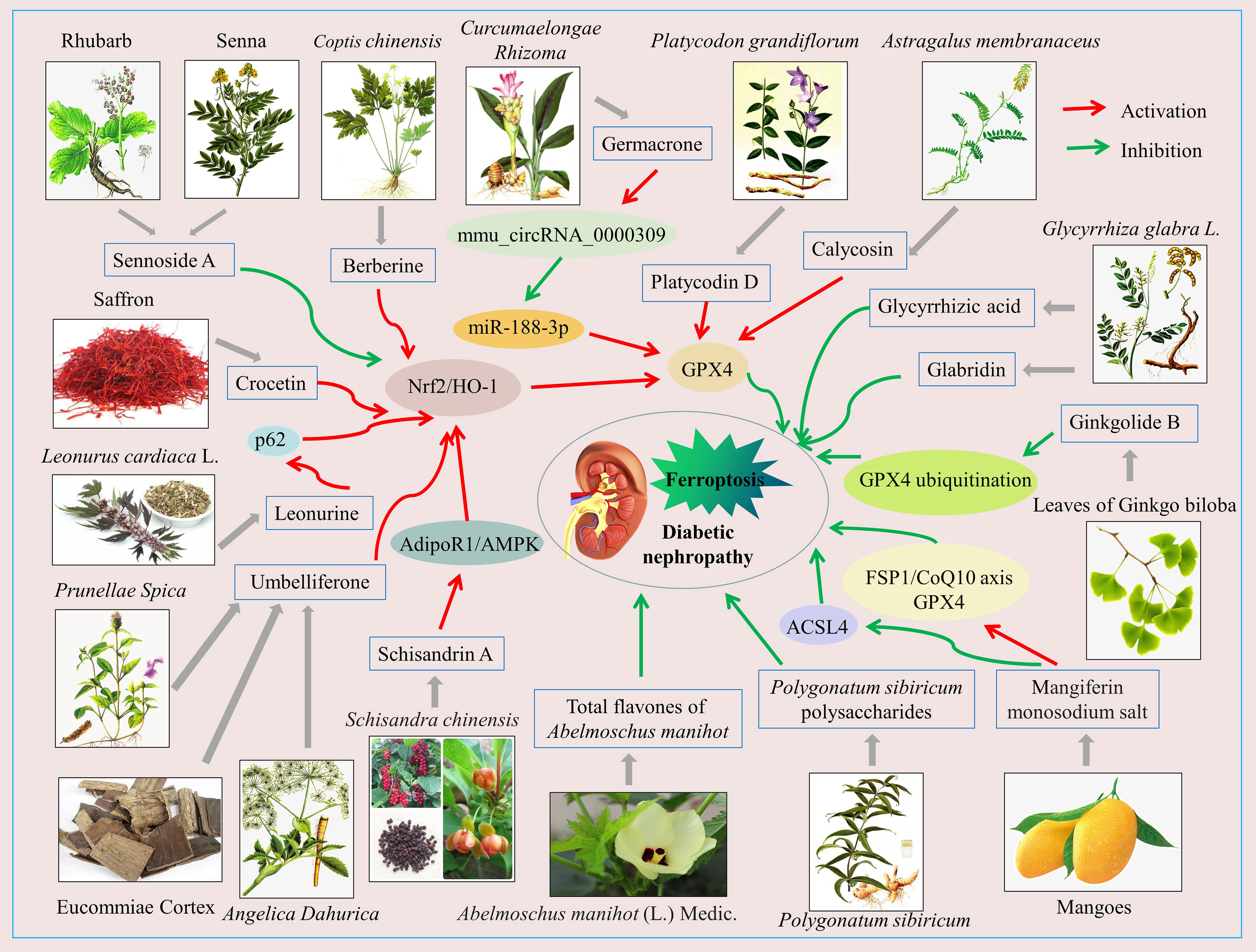
Figure 2 Schematic of Chinese herbal medicine against diabetic nephropathy by inhibiting ferroptosis. The activation of Nrf2/HO-1, GPX4, FSP1/CoQ10, AdipoR1/AMPK signaling pathways and so on, or the inhibition of the ASCL4 signaling pathway, can inhibit ferroptosis in renal intrinsic cells, which benefits diabetic nephropathy treatment.
3.1 Flavonoids
3.1.1 Glabridin
Glabridin is a prenylated isoflavone extracted from the roots and rhizomes of Glycyrrhiza glabra L (132). Previous studies have found that glabridin has hepatoprotective, neuroprotective, antidiabetic, and antioxidant effects in animal models of diabetes (133–135). Tan et al. (136) found that glabridin not only reversed hyperglycemia and insulin resistance in diabetic rats but also attenuated diabetes-induced damage to kidney function and structure. Mechanistically, glabridin increased SOD, glutathione (GSH), and catalase (CAT) activities; up-regulated GPX4, SLC7A11, and SLC3A2 expression; down-regulated transferrin receptor 1 (TfR1), vascular endothelial growth factor (VEGF), p-AKT, and p-ERK1/2 expression; and decreased MDA content and iron concentration in vitro and in vivo. These results suggest that glabridin delays DN progression in association with inhibition of oxidative stress, ferroptosis, and the VEGF/Akt/ERK signaling pathway.
3.1.2 Calycosin
Calycosin is an isoflavone phytoestrogen extracted from the Chinese herb Astragalus membranaceus, which has various pharmacological activities such as anti-inflammatory, antioxidant, anti-diabetic, anti-osteoporosis, anti-tumor, hepatoprotective, neuroprotective, and cardioprotective (137). In recent years, an increasing number of studies have explored the role and underlying mechanisms of calycosin in the treatment of DN (138–140). Huang et al. (141) observed that calycosin improved renal function, attenuated tubular injury, inhibited renal fibrosis in db/db mice in vivo, and increased the viability of high glucose-induced HK-2 cells in vitro. This protective effect of calycosin was associated with its reduction of lactate dehydrogenase (LDH), MDA, and lipid ROS levels; increase of GSH activity; promotion of GPX4 expression; and inhibition of nuclear receptor coactivator 4 (NCOA4) expression in DN models. Moreover, the above effects of calycosin were reversed when co-treated with the ferroptosis inducer erastin. These results suggest that calycosin reduces diabetes-induced kidney injury by inhibiting ferroptosis.
3.1.3 Total flavones of Abelmoschus manihot
Total flavones of Abelmoschus manihot (TFA) is a total flavonoid component extracted from the flowers of Abelmoschus manihot (L.) Medic. There are eight flavone glycosides whose chemical structures have been identified in TFA, including quercetin-3-O-robinobioside, hyperoside, quercetin, myricetin, quercetin-3′-O-glucoside, gossypetin, gossypetin-3-O-glucoside, and isoquercetin (142). Previous studies have shown that TFA has significant anti-inflammatory, endoplasmic reticulum stress-inhibiting, and podocyte-protective activities and is therefore widely used in the treatment of inflammatory bowel disease, DN, and chronic renal failure (142–146). Recent studies have found that TFA has effects similar to those of ferrostatin-1, a ferroptosis inhibitor. TFA inhibited ROS production, upregulated GPX4 and SLC7A11 expression, downregulated TfR1 and ACSL4 expression, and decreased MDA levels and iron content in the DN model (147). These data suggest that inhibition of ferroptosis in renal tubular cells by reducing iron deposition and improving antioxidant capacity may be an important mechanism by which TFA attenuates diabetic tubulopathy.
3.1.4 Mangiferin monosodium salt
Mangiferin is a natural flavonoid extracted mainly from mangoes and has a wide range of pharmacological activities, including antioxidant (148), anti-inflammatory and immunomodulatory (149), anti-diabetic (150), anti-cancer (151), anti-fibrotic (152), nephroprotective (153), cardioprotective (154), and neuroprotective (155). However, the poor solubility and low bioavailability of mangiferin limit its wide application (156). The mangiferin monosodium salt (MGM) obtained by structural modification of mangiferin can improve the solubility and bioavailability of mangiferin to some extent (157). The emerging evidence has shown that MGM also has a good renoprotective effect in DN rats. Mechanistically, MGM not only attenuated systemic insulin resistance (IR)-induced renal inflammation by inhibiting the MAPK/NF-κB signaling axis, but also ameliorated podocyte IR by activating the p-IRS1 (Tyr608)/p-PI3K/p-Akt signaling pathway. More importantly, this study also revealed the mechanism of ferroptosis in MGM attenuated renal injury in DN rats. MGM enhanced mevalonate-mediated antioxidant capacity (FSP1/CoQ10 axis and GPX4) and attenuated ACSL4-mediated proferroptotic lipid drivers’ generation in the kidney, thereby improving renal ferroptosis in DN rats (158).
3.2 Terpenoids
3.2.1 Glycyrrhizic acid
Glycyrrhizic acid is a triterpene isolated from the traditional medicinal plant Glycyrrhiza glabra, which has various pharmacological properties such as anti-inflammatory, antibacterial, antioxidant, anti-fibrotic, antiviral, anti-diabetic, anti-obesity, anti-cancer, hepatoprotective, renoprotective, and immunomodulatory (159–162). Previous studies have demonstrated that glycyrrhizic acid is an effective inhibitor of HMGB1 and ROS production and that it can effectively attenuate renal injury in DN models (162, 163). Recent studies found that glycyrrhizic acid upregulated GPX4 expression and reduced ROS production in high glucose-induced mouse glomerular podocytes, suggesting that glycyrrhizic acid attenuates high glucose-induced podocyte injury in association with inhibition of ferroptosis (164).
3.2.2 Ginkgolide B
Ginkgolide B is a diterpenoid isolated from the leaves of Ginkgo biloba with a variety of pharmacological effects, including anti-inflammatory (165), antioxidant (166), anti-apoptotic (167), and anti-ferroptosis (168, 169), etc. Recently, Chen et al. (170) found that ginkgolide B significantly reduced blood glucose in DN model mice, improved dyslipidemia and renal lipid accumulation, and attenuated renal dysfunction and histopathological damage, such as glomerular basement membrane thickening, tubular dilatation, extracellular matrix deposition, and fibrosis. Mechanistically, ginkgolide B promoted the expression of ferroptosis-associated proteins ferritin heavy chain 1 (FTH1) and GPX4, inhibited TfR1 expression, and decreased ROS levels and iron content in the DN model. Of these, inhibiting the ubiquitinated degradation of GPX4, increasing the level of GPX4 and thus inhibiting ferroptosis is the key of ginkgolide B to delay the progression of DN.
3.2.3 Germacrone
Germacrone, a monocyclic sesquiterpene natural compound, is one of the main bioactive components of the Chinese medicine Curcumaelongae Rhizoma. Modern research has confirmed the significant anti-tumor (171), anti-viral (172), anti-fibrotic (173), anti-inflammatory (174), and antioxidant (174) effects of germacrone. The renoprotective effects of germacrone have also attracted the attention of researchers in recent years (175, 176). Jin et al. (177) found that germacrone reduced blood glucose and urinary protein in db/db mice and alleviated the structural damage to the kidney caused by hyperglycemia. Mechanistically, germacrone upregulated the expression of mmu_circRNA_0000309 in the DN model, followed by mmu_circRNA_0000309 sponge miR-188-3p which in turn promoted the expression of GPX4, thereby inhibiting ferroptosis and ultimately attenuating mitochondrial damage and podocyte apoptosis.
3.3 Anthraquinones
As we all know, Rhubarb and Senna are commonly used Chinese herbs for constipation. Sennoside A is an important bioactive component extracted from Rhubarb and Senna with a wide range of pharmacological activities, including laxative (178), anti-obesity (179, 180), anti-diabetic (181), anti-tumor (182), and hepatoprotective (183, 184). A recent study found that PTGS2 protein expression and MDA levels were significantly increased, while GPX4 protein expression and GSH activity were significantly decreased, in the kidneys of DN mice. Sennoside A treatment was able to reverse this phenomenon and reduce urinary protein levels. Mechanistically, sennoside A attenuated ferroptosis through inhibition of the Nrf2/HO-1 signaling pathway, thereby treating DN (185).
3.4 Alkaloids
3.4.1 Berberine
Berberine, an isoquinoline alkaloid, is mainly extracted from the Chinese herb Coptis chinensis. Previous studies found that berberine has strong pharmacological activities, such as antidiabetic (186), hypolipidemic (187), antiviral (188), antitumor (189), anti-inflammatory (190), antioxidant (191), hepatoprotective (192), neuroprotective (193), etc. Currently, berberine is widely used in the treatment of diabetes and its complications, including type 2 diabetes (194), diabetic nephropathy (195), diabetic retinopathy (196), diabetic encephalopathy (196), and diabetic cardiomyopathy (197). Accumulating evidence suggests that berberine has a significant anti-ferroptosis effect (198–200). Bao et al. (200) found that berberine improved the viability and proliferation of pancreatic β cells. Mechanistically, berberine attenuated ferroptosis in β cells by promoting GPX4 expression. Guan et al. (201) demonstrated that berberine blocked high glucose-induced ferroptosis in glomerular podocytes by activating the Nrf2/HO-1/GPX4 signaling pathway, decreasing ROS levels, and increasing GSH activity.
3.4.2 Leonurine
Leonurine is an alkaloid extracted from Leonurus cardiaca L. (motherwort), a plant in the Labiatae family, with various biological effects such as antioxidant (202–204), anti-inflammatory (204), regulating autophagy (205), improving mitochondrial dysfunction (203), and protecting pancreatic β cells (206). Huang et al. (207) thought that leonurine is a promising therapeutic agent to prevent diabetes and its complications by inhibiting the formation of advanced glycation end-products (AGEs). A recent study found that leonurine could reduce iron accumulation, lipid peroxidation, and ferroptosis by activating the Nrf2 antioxidant pathway (208). Similarly, Wu et al. (209) found that leonurine significantly reversed erastin-induced damage in HK-2 cells. Mechanistically, leonurine promoted p62 expression, activated Nrf2 entry into the nucleus, and upregulated HO-1 protein expression, thereby protecting HK-2 cells from ferroptosis injury. The above results suggest that leonurine may be a natural compound that effectively inhibits ferroptosis.
3.5 Coumarins
Umbelliferone is a natural coumarin compound extracted from Eucommiae Cortex, Prunellae Spica, and Angelica Dahurica. It has been reported to possess a variety of biological activities, including anti-diabetic and anti-hyperlipidemic (210), anti-diarrheal and anti-ulcerogenic (211), anti-rheumatic (212), anti-tumor (213), antivirulence (214), neuroprotective (215, 216), cardioprotective (217, 218), hepatoprotective (219), and renoprotective (220). Recent studies have found that umbelliferone shows great potential in DN treatment. Wang et al. (221) demonstrated that umbelliferone improved renal function in DN rats through modulation of inflammation and the TLR/NF-κB pathway. Jin et al. (222) observed that umbelliferone inhibited ROS production, increased GSH activity, decreased MDA levels and iron content, upregulated GPX4, Nrf2 and HO-1 expression, and downregulated ACSL4 expression in both db/db mice and high glucose-induced HK-2 cell models. This finding suggests that umbelliferone has inhibitory effects on oxidative stress and ferroptosis both in vitro and in vivo. However, this effect of umbelliferone was reversed when Nrf2 was knocked down. Thus, umbelliferone delays DN progression mainly by activating the Nrf-2/HO-1 pathway and inhibiting ferroptosis.
3.6 Lignans
Schisandrin A is the main bioactive component isolated from the traditional Chinese medicine Schisandra chinensis. Previous studies have shown that Schisandrin A has a variety of pharmacological effects, including anti-inflammatory, antioxidant, antitumor, antidiabetic, hepatoprotective, neuroprotective, and musculoskeletal protection (223). The emerging evidence has shown that Schisandrin A not only attenuated oxidative stress, the inflammatory response, mitochondrial damage, and ROS/TXNIP/NLRP3 signaling pathway-mediated pyroptosis in DN models but also inhibited high glucose-induced ferroptosis (224). Specifically, Schisandrin A reduced lipid ROS levels by activating AdipoR1/AMPK signaling, which in turn induced Nrf2, HO-1, GPX4, and SOD2 protein expression in the DN model. Therefore, Schisandrin A may be a potential therapeutic agent for DN.
3.7 Polysaccharides
In China, Polygonatum sibiricum is a medicinal and edible plant. Polygonatum sibiricum polysaccharides (PSP) are one of the main active ingredients of Polygonatum sibiricum, which is also the only content determination component of Polygonatum sibiricum prescribed by the Chinese Pharmacopoeia (2020 edition). Modern pharmacological studies have shown that PSP has antioxidant (225, 226), anti-aging (227, 228), anti-inflammatory (226), hepatoprotective (229), immunomodulatory (230, 231), and anti-diabetic (232) effects. A recent study has found that PSP significantly improved renal function parameters and attenuated renal histopathological damage in DN rats (233). Mechanistically, PSP reduced MAD and total iron content, increased GSH content, down-regulated transferrin and FTH1 expression, and up-regulated GPX4 expression in the renal tissues of DN rats. This study confirms that PSP can play a therapeutic role in DN by inhibiting ferroptosis.
3.8 Saponins
Platycodin D, a triterpenoid saponin, was isolated from the root of Platycodon grandiflorum. Previous studies have demonstrated that platycodin D has various pharmacological properties, such as anti-tumor (234, 235), anti-diabetic (236), anti-hypercholesterolemic (237), anti-inflammatory (238), anti-asthmatic (239), anti-obesity (240), and hepatoprotective effects (241). The emerging evidence has shown that platycodin D significantly reduced LDH release and increased cell viability in high glucose-induced HK-2 cells (242). Mechanistically, platycodin D inhibited lipid ROS production, decreased MDA levels and iron content, increased GSH levels, inhibited ACSL4 and TfR1 expression, and promoted FTH1, SLC7A11, and GPX4 expression. In addition, this effect was enhanced by the combination of platycodin D with the ferroptosis inhibitor deferasirox, while the effect was attenuated by GPX4 knockdown. Therefore, the reversal of high glucose-induced HK-2 cell injury by platycodin D was associated with upregulation of GPX4 expression and inhibition of ferroptosis.
3.9 Carotenoids
Crocetin, a natural carotenoid, is one of the main active ingredients in the traditional Chinese medicine saffron. Modern pharmacology has demonstrated that crocetin possesses a variety of pharmacological activities, including cholesterol-lowering (243), antidiabetic (244), antiatherosclerotic (245), antidepressant (246), cardioprotective (247), and nephroprotective (248, 249). In recent years, it has been found that crocetin could alleviate DN by inhibiting the expression of inflammatory cytokines and fibrotic factors in the kidney of diabetic rats (248). In high glucose-induced human glomerular mesangial cells, Lu et al. (250) observed that crocetin promoted Nrf2, GPX4, and HO-1 protein expression, reduced intracellular ROS production, and improved cell survival. Therefore, inhibition of ferroptosis in human glomerular mesangial cells is also an important mechanism for the prevention or treatment of DN by crocetin.
In conclusion, the active ingredients of some herbal medicines have shown good effects in the treatment of DN by targeting ferroptosis. Mechanistically, ferroptosis is inhibited mainly by enhancing antioxidant capacity, reducing lipid peroxidation levels, and reducing iron overload. Nrf2/HO-1 is a well-studied signaling pathway. GPX4 is a key signaling molecule for the antiferroptotic effect of Chinese herb medicine. Unlike other studies, Ding et al. (185) found that inhibition of excessive activation of the Nrf2/HO-1 signaling pathway was an important mechanism by which sennoside A attenuated ferroptosis in DN models. Therefore, scholars can explain this inconsistency in further studies.
4 Conclusion and prospects
As a newly discovered mode of cell death, ferroptosis has been a hot topic of research in the field of kidney disease in recent years. Throughout the past studies, great progress has been made in the study of ferroptosis in DN pathogenesis and in the prevention and treatment of DN through the regulation of ferroptosis by Chinese herbal medicine. From the available literature, multiple regulatory factors (non-coding RNAs, COX-2, ALOX15, Prdx6, ZIP14, HMGB1, Nrf2, and GPX4) and the HIF-1α/HO-1 pathway are involved in the regulation of ferroptosis in DN. A variety of herbal active ingredients such as glabridin, calycosin, glycyrrhizic acid, germacrone, berberine, etc. have been shown to reduce DN by targeting the inhibition of ferroptosis. However, the research on the regulation of ferroptosis in DN by Chinese herbal medicine is still in the preliminary exploration stage. There are still some limitations in the existing studies. Firstly, there is a lack of specific biomarkers for ferroptosis. Current studies can only indirectly demonstrate the occurrence of iron death by detecting ROS levels, lipid peroxidation products, iron levels, GPX4 activity, etc. Secondly, almost all studies are preclinical, and models are limited to cellular and animal models. Thirdly, most studies have only explored the molecular mechanism of Chinese herbal medicine to alleviate ferroptosis in DN via a single pathway. Finally, Chinese herbal medicine contains several components, such as Chinese herbal formulas, Chinese patent medicines, extractive compounds, etc. At the present stage, the research on Chinese herbal medicines is mostly limited to monomers and extractive compounds. Fewer studies have been conducted on Chinese herbal formulas and Chinese patent medicines that are used frequently in clinical practice. Therefore, in future research, we should focus on exploring the molecular mechanisms of ferroptosis regulation by Chinese herbal medicines from multiple pathways and perspectives, conducting relevant clinical trials, and further exploring new Chinese herbal medicines with anti-ferroptotic effects. We believe that as ferroptosis research progresses, it is expected to provide new effective therapeutic strategies for the treatment of DN.
Author contributions
MW designed the study and completed the first draft, so she is the first author. XL examined the literature. ZT revised English grammar. XT and ML contributed in the scientific writing of the manuscript. MW and JW revised the manuscript. All authors contributed to the article and approved the submitted version.
Funding
This work was supported by Beijing Municipal Natural Science Foundation (No. 7202172).
Conflict of interest
The authors declare that the research was conducted in the absence of any commercial or financial relationships that could be construed as a potential conflict of interest.
Publisher’s note
All claims expressed in this article are solely those of the authors and do not necessarily represent those of their affiliated organizations, or those of the publisher, the editors and the reviewers. Any product that may be evaluated in this article, or claim that may be made by its manufacturer, is not guaranteed or endorsed by the publisher.
Supplementary material
The Supplementary Material for this article can be found online at: https://www.frontiersin.org/articles/10.3389/fendo.2023.1188003/full#supplementary-material
References
1. Sun H, Saeedi P, Karuranga S, Pinkepank M, Ogurtsova K, Duncan BB, et al. IDF diabetes atlas: global, regional and country-level diabetes prevalence estimates for 2021 and projections for 2045. Diabetes Res Clin Pract (2022) 183:109119. doi: 10.1016/j.diabres.2021.109119
2. Tuttle KR, Bakris GL, Bilous RW, Chiang JL, de Boer IH, Goldstein-Fuchs J, et al. Diabetic kidney disease: a report from an ADA consensus conference. Diabetes Care (2014) 37(10):2864–83. doi: 10.2337/dc14-1296
3. Rossing P, Persson F and Frimodt-Møller M. Prognosis and treatment of diabetic nephropathy: recent advances and perspectives. Nephrol Ther (2018) 14 Suppl 1:S31–7. doi: 10.1016/j.nephro.2018.02.007
4. Ruiz-Ortega M, Rodrigues-Diez RR, Lavoz C, Rayego-Mateos S. Special issue "diabetic nephropathy: diagnosis, prevention and treatment". J Clin Med (2020) 9(3):813. doi: 10.3390/jcm9030813
5. Perkins BA, Bebu I, de Boer IH, Molitch M, Tamborlane W, Lorenzi G, et al. Risk factors for kidney disease in type 1 diabetes. Diabetes Care (2019) 42(5):883–90. doi: 10.2337/dc18-2062
6. Sawaf H, Thomas G, Taliercio JJ, Nakhoul G, Vachharajani TJ, Mehdi A. Therapeutic advances in diabetic nephropathy. J Clin Med (2022) 11(2):378. doi: 10.3390/jcm11020378
7. Østergaard JA, Cooper ME, Jandeleit-Dahm KAM. Targeting oxidative stress and anti-oxidant defence in diabetic kidney disease. J Nephrol (2020) 33(5):917–29. doi: 10.1007/s40620-020-00749-6
8. Jung SW, Moon JY. The role of inflammation in diabetic kidney disease. Korean J Intern Med (2021) 36(4):753–66. doi: 10.3904/kjim.2021.174
9. Koska J, Gerstein HC, Beisswenger PJ, Reaven PD. Advanced glycation end products predict loss of renal function and high-risk chronic kidney disease in type 2 diabetes. Diabetes Care (2022) 45(3):684–91. doi: 10.2337/dc21-2196
10. Parwani K, Mandal P. Role of advanced glycation end products and insulin resistance in diabetic nephropathy. Arch Physiol Biochem (2023) 129(1):95–107. doi: 10.1080/13813455.2020.1797106
11. Victor P, Umapathy D, George L, Juttada U, Ganesh GV, Amin KN, et al. Crosstalk between endoplasmic reticulum stress and oxidative stress in the progression of diabetic nephropathy. Cell Stress Chaperones (2021) 26(2):311–21. doi: 10.1007/s12192-020-01176-z
12. Cui J, Bai X and Chen X. Autophagy and diabetic nephropathy. Adv Exp Med Biol (2020) 1207:487–94. doi: 10.1007/978-981-15-4272-5_36
13. Wan J, Liu D, Pan S, Zhou S and Liu Z. NLRP3-mediated pyroptosis in diabetic nephropathy. Front Pharmacol (2022) 13:998574. doi: 10.3389/fphar.2022.998574
14. Dixon SJ, Lemberg KM, Lamprecht MR, Skouta R, Zaitsev EM, Gleason CE, et al. Ferroptosis: an iron-dependent form of nonapoptotic cell death. Cell (2012) 149(5):1060–72. doi: 10.1016/j.cell.2012.03.042
15. Li J, Cao F, Yin HL, Huang ZJ, Lin ZT, Mao N, et al. Ferroptosis: past, present and future. Cell Death Dis (2020) 11(2):88. doi: 10.1038/s41419-020-2298-2
16. Dominguez JH, Liu Y and Kelly KJ. Renal iron overload in rats with diabetic nephropathy. Physiol Rep (2015) 3(12):e12654. doi: 10.14814/phy2.12654
17. Chaudhary K, Chilakala A, Ananth S, Mandala A, Veeranan-Karmegam R, Powell FL, et al. Renal iron accelerates the progression of diabetic nephropathy in the HFE gene knockout mouse model of iron overload. Am J Physiol Renal Physiol (2019) 317(2):F512–7. doi: 10.1152/ajprenal.00184.2019
18. Gao W, Li X, Gao Z and Li H. Iron increases diabetes-induced kidney injury and oxidative stress in rats. Biol Trace Elem Res (2014) 160(3):368–75. doi: 10.1007/s12011-014-0021-9
19. Wang Y, Bi R, Quan F, Cao Q, Lin Y, Yue C, et al. Ferroptosis involves in renal tubular cell death in diabetic nephropathy. Eur J Pharmacol (2020) 888:173574. doi: 10.1016/j.ejphar.2020.173574
20. Wu Y, Chen Y. Research progress on ferroptosis in diabetic kidney disease. Front Endocrinol (Lausanne) (2022) 13:945976. doi: 10.3389/fendo.2022.945976
21. Liu J, Gao LD, Fu B, Yang HT, Zhang L, Che SQ, et al. Efficacy and safety of zicuiyin decoction on diabetic kidney disease: a multicenter, randomized controlled trial. Phytomedicine (2022) 100:154079. doi: 10.1016/j.phymed.2022.154079
22. Zhao J, Tostivint I, Xu L, Huang J, Gambotti L, Boffa JJ, et al. Efficacy of combined abelmoschus manihot and irbesartan for reduction of albuminuria in patients with type 2 diabetes and diabetic kidney disease: a multicenter randomized double-blind parallel controlled clinical trial. Diabetes Care (2022) 45(7):e113–5. doi: 10.2337/dc22-0607
23. Yan G, Chang T, Zhao Y, Yu M, Mi J, Wang G, et al. The effects of ophiocordyceps sinensis combined with ACEI/ARB on diabetic kidney disease: a systematic review and meta-analysis. Phytomedicine (2023) 108:154531. doi: 10.1016/j.phymed.2022.154531
24. Winkle M, El-Daly SM, Fabbri M and Calin GA. Noncoding RNA therapeutics - challenges and potential solutions. Nat Rev Drug Discov (2021) 20(8):629–51. doi: 10.1038/s41573-021-00219-z
25. Zhu D, Wu X and Xue Q. Long non-coding RNA CASC2 restrains high glucose-induced proliferation, inflammation and fibrosis in human glomerular mesangial cells through mediating miR-135a-5p/TIMP3 axis and JNK signaling. Diabetol Metab Syndr (2021) 13(1):89. doi: 10.1186/s13098-021-00709-5
26. Tsai YC, Kuo MC, Hung WW, Wu LY, Wu PH, Chang WA, et al. High glucose induces mesangial cell apoptosis through miR-15b-5p and promotes diabetic nephropathy by extracellular vesicle delivery. Mol Ther (2020) 28(3):963–74. doi: 10.1016/j.ymthe.2020.01.014
27. Jiang X, Ruan XL, Xue YX, Yang S, Shi M and Wang LN. Metformin reduces the senescence of renal tubular epithelial cells in diabetic nephropathy via the MBNL1/miR-130a-3p/STAT3 pathway. Oxid Med Cell Longev (2020) 2020:8708236. doi: 10.1155/2020/8708236
28. Cao D, Zhao M, Wan C, Zhang Q, Tang T, Liu J, et al. Role of tea polyphenols in delaying hyperglycemia-induced senescence in human glomerular mesangial cells via miR-126/Akt-p53-p21 pathways. Int Urol Nephrol (2019) 51(6):1071–8. doi: 10.1007/s11255-019-02165-7
29. Ma Z, Li L, Livingston MJ, Zhang D, Mi Q, Zhang M, et al. p53/microRNA-214/ULK1 axis impairs renal tubular autophagy in diabetic kidney disease. J Clin Invest (2020) 130(9):5011–26. doi: 10.1172/jci135536
30. Chen K, Yu B and Liao J. LncRNA SOX2OT alleviates mesangial cell proliferation and fibrosis in diabetic nephropathy via Akt/mTOR-mediated autophagy. Mol Med (2021) 27(1):71. doi: 10.1186/s10020-021-00310-6
31. Zhan JF, Huang HW, Huang C, Hu LL and Xu WW. Long non-coding RNA NEAT1 regulates pyroptosis in diabetic nephropathy via mediating the miR-34c/NLRP3 axis. Kidney Blood Press Res (2020) 45(4):589–602. doi: 10.1159/000508372
32. Wang J, Zhao SM. LncRNA-antisense non-coding RNA in the INK4 locus promotes pyroptosis via miR-497/thioredoxin-interacting protein axis in diabetic nephropathy. Life Sci (2021) 264:118728. doi: 10.1016/j.lfs.2020.118728
33. Han Q, Zhang Y, Jiao T, Li Q, Ding X, Zhang D, et al. Urinary sediment microRNAs can be used as potential noninvasive biomarkers for diagnosis, reflecting the severity and prognosis of diabetic nephropathy. Nutr Diabetes (2021) 11(1):24. doi: 10.1038/s41387-021-00166-z
34. An Y, Zhang C, Xu F, Li W, Zeng C, Xie L, et al. Increased urinary miR-196a level predicts the progression of renal injury in patients with diabetic nephropathy. Nephrol Dial Transplant (2020) 35(6):1009–16. doi: 10.1093/ndt/gfy326
35. Qi R, Bai Y, Wei Y, Liu N and Shi B. The role of non-coding RNAs in ferroptosis regulation. J Trace Elem Med Biol (2022) 70:126911. doi: 10.1016/j.jtemb.2021.126911
36. Farooqi AA, Kapanova G, Kalmakhanov S, Kussainov AZ, Datkhayeva Z. Regulation of ferroptosis by non-coding RNAs: mechanistic insights. J Pharmacol Exp Ther (2023) 384(1):20–7. doi: 10.1124/jpet.121.001225
37. Li Q, Meng X and Hua Q. Circ ASAP2 decreased inflammation and ferroptosis in diabetic nephropathy through SOX2/SLC7A11 by miR-770-5p. Acta Diabetol (2023) 60(1):29–42. doi: 10.1007/s00592-022-01961-5
38. Xiong X, Yan YH, Yang Q, Shen MR and Zhong XS. hsa_circ_0006370 promotes ferroptosis in high glucose treated renal tubular epithelial cells (HK-2). J Guangdong Pharm Univ (2022) 38(1):97–102. doi: 10.16809/j.cnki.2096-3653.2021120501
39. Tyagi A, Kamal MA and Poddar NK. Integrated pathways of COX-2 and mTOR: roles in cell sensing and alzheimer's disease. Front Neurosci (2020) 14:693. doi: 10.3389/fnins.2020.00693
40. Mitchell JA, Kirkby NS, Ahmetaj-Shala B, Armstrong PC, Crescente M, Ferreira P, et al. Cyclooxygenases and the cardiovascular system. Pharmacol Ther (2021) 217:107624. doi: 10.1016/j.pharmthera.2020.107624
41. Ji XK, Madhurapantula SV, He G, Wang KY, Song CH, Zhang JY, et al. Genetic variant of cyclooxygenase-2 in gastric cancer: more inflammation and susceptibility. World J Gastroenterol (2021) 27(28):4653–66. doi: 10.3748/wjg.v27.i28.4653
42. Zhou TJ, Zhang SL, He CY, Zhuang QY, Han PY, Jiang SW, et al. Downregulation of mitochondrial cyclooxygenase-2 inhibits the stemness of nasopharyngeal carcinoma by decreasing the activity of dynamin-related protein 1. Theranostics (2017) 7(5):1389–406. doi: 10.7150/thno.17647
43. Wei J, Deng X, Li Y, Li R, Yang Z, Li X, et al. PP2 ameliorates renal fibrosis by regulating the NF-κB/COX-2 and PPARγ/UCP2 pathway in diabetic mice. Oxid Med Cell Longev (2021) 2021:7394344. doi: 10.1155/2021/7394344
44. Quan X, Liu H, Ye D, Ding X, Su X. Forsythoside a alleviates high glucose-induced oxidative stress and inflammation in podocytes by inactivating MAPK signaling via MMP12 inhibition. Diabetes Metab Syndr Obes (2021) 14:1885–95. doi: 10.2147/dmso.S305092
45. Singh B, Kumar A, Singh H, Kaur S, Arora S, Singh B. Protective effect of vanillic acid against diabetes and diabetic nephropathy by attenuating oxidative stress and upregulation of NF-κB, TNF-α and COX-2 proteins in rats. Phytother Res (2022) 36(3):1338–52. doi: 10.1002/ptr.7392
46. Cai J, Liu B, Guo T, Zhang Y, Wu X, Leng J, et al. Effects of thromboxane prostanoid receptor deficiency on diabetic nephropathy induced by high fat diet and streptozotocin in mice. Eur J Pharmacol (2020) 882:173254. doi: 10.1016/j.ejphar.2020.173254
47. Wu Z, Li D, Tian D, Liu X, Wu Z. Aspirin mediates protection from diabetic kidney disease by inducing ferroptosis inhibition. PloS One (2022) 17(12):e0279010. doi: 10.1371/journal.pone.0279010
48. Ma KL, Liu L, Zhang Y, Wang GH, Hu ZB, Chen PP, et al. Aspirin attenuates podocyte injury in diabetic rats through overriding cyclooxygenase-2-mediated dysregulation of LDL receptor pathway. Int Urol Nephrol (2019) 51(3):551–8. doi: 10.1007/s11255-018-2059-7
49. Guan Y, Davis L, Breyer MD, Hao CM. Cyclooxygenase-2 contributes to diabetic nephropathy through glomerular EP4 receptor. Prostaglandins Other Lipid Mediat (2022) 159:106621. doi: 10.1016/j.prostaglandins.2022.106621
50. Singh NK, Rao GN. Emerging role of 12/15-lipoxygenase (ALOX15) in human pathologies. Prog Lipid Res (2019) 73:28–45. doi: 10.1016/j.plipres.2018.11.001
51. Bromfield EG, Walters JLH, Cafe SL, Bernstein IR, Stanger SJ, Anderson AL, et al. Differential cell death decisions in the testis: evidence for an exclusive window of ferroptosis in round spermatids. Mol Hum Reprod (2019) 25(5):241–56. doi: 10.1093/molehr/gaz015
52. Shintoku R, Takigawa Y, Yamada K, Kubota C, Yoshimoto Y, Takeuchi T, et al. Lipoxygenase-mediated generation of lipid peroxides enhances ferroptosis induced by erastin and RSL3. Cancer Sci (2017) 108(11):2187–94. doi: 10.1111/cas.13380
53. Zhao J, Wu Y, Liang S, Piao X. Activation of SSAT1/ALOX15 axis aggravates cerebral ischemia/reperfusion injury via triggering neuronal ferroptosis. Neuroscience (2022) 485:78–90. doi: 10.1016/j.neuroscience.2022.01.017
54. Gao S, Zhou L, Lu J, Fang Y, Wu H, Xu W, et al. Cepharanthine attenuates early brain injury after subarachnoid hemorrhage in mice via inhibiting 15-lipoxygenase-1-mediated microglia and endothelial cell ferroptosis. Oxid Med Cell Longev (2022) 2022:4295208. doi: 10.1155/2022/4295208
55. Conteh AM, Reissaus CA, Hernandez-Perez M, Nakshatri S, Anderson RM, Mirmira RG, et al. Platelet-type 12-lipoxygenase deletion provokes a compensatory 12/15-lipoxygenase increase that exacerbates oxidative stress in mouse islet β cells. J Biol Chem (2019) 294(16):6612–20. doi: 10.1074/jbc.RA118.007102
56. Coppey L, Obrosov A, Shevalye H, Davidson E, Paradee W, Yorek MA. Characterization of mice ubiquitously overexpressing human 15-lipoxygenase-1: effect of diabetes on peripheral neuropathy and treatment with menhaden oil. J Diabetes Res (2021) 2021:5564477. doi: 10.1155/2021/5564477
57. Chen Q, Zheng Q, Yang Y, Luo Y, Wang H, Li H, et al. 12/15-lipoxygenase regulation of diabetic cognitive dysfunction is determined by interfering with inflammation and cell apoptosis. Int J Mol Sci (2022) 23(16):8997. doi: 10.3390/ijms23168997
58. Wang X, Jiang L, Liu XQ, Huang YB, Zhu W, Zeng HX, et al. Identification of genes reveals the mechanism of cell ferroptosis in diabetic nephropathy. Front Physiol (2022) 13:890566. doi: 10.3389/fphys.2022.890566
59. Kim YS, Xu ZG, Reddy MA, Li SL, Lanting L, Sharma K, et al. Novel interactions between TGF-β1 actions and the 12/15-lipoxygenase pathway in mesangial cells. J Am Soc Nephrol (2005) 16(2):352–62. doi: 10.1681/asn.2004070568
60. Yuan H, Reddy MA, Deshpande S, Jia Y, Park JT, Lanting LL, et al. Epigenetic histone modifications involved in profibrotic gene regulation by 12/15-lipoxygenase and its oxidized lipid products in diabetic nephropathy. Antioxid Redox Signal (2016) 24(7):361–75. doi: 10.1089/ars.2015.6372
61. Fisher AB. Peroxiredoxin 6: a bifunctional enzyme with glutathione peroxidase and phospholipase A2 activities. Antioxid Redox Signal (2011) 15(3):831–44. doi: 10.1089/ars.2010.3412
62. Sorrell SL, Golder ZJ, Johnstone DB, Frankl FEK. Renal peroxiredoxin 6 interacts with anion exchanger 1 and plays a novel role in pH homeostasis. Kidney Int (2016) 89(1):105–12. doi: 10.1038/ki.2015.277
63. Lee DH, Park JH, Han SB, Yoon DY, Jung YY, Hong JT. Peroxiredoxin 6 overexpression attenuates lipopolysaccharide-induced acute kidney injury. Oncotarget (2017) 8(31):51096–107. doi: 10.18632/oncotarget.17002
64. Ma J, Li C, Liu T, Zhang L, Wen X, Liu X, et al. Identification of markers for diagnosis and treatment of diabetic kidney disease based on the ferroptosis and immune. Oxid Med Cell Longev (2022) 2022:9957172. doi: 10.1155/2022/9957172
65. Zhang Q, Hu Y, Hu JE, Ding Y, Shen Y, Xu H, et al. Sp1-mediated upregulation of Prdx6 expression prevents podocyte injury in diabetic nephropathy via mitigation of oxidative stress and ferroptosis. Life Sci (2021) 278:119529. doi: 10.1016/j.lfs.2021.119529
66. Wang G, Biswas AK, Ma W, Kandpal M, Coker C, Grandgenett PM, et al. Metastatic cancers promote cachexia through ZIP14 upregulation in skeletal muscle. Nat Med (2018) 24(6):770–81. doi: 10.1038/s41591-018-0054-2
67. Scheiber IF, Alarcon NO, Zhao N. Manganese uptake by A549 cells is mediated by both ZIP8 and ZIP14. Nutrients (2019) 11(7):1473. doi: 10.3390/nu11071473
68. Prajapati M, Conboy HL, Hojyo S, Fukada T, Budnik B, Bartnikas TB. Biliary excretion of excess iron in mice requires hepatocyte iron import by Slc39a14. J Biol Chem (2021) 297(1):100835. doi: 10.1016/j.jbc.2021.100835
69. Routhe LJ, Andersen IK, Hauerslev LV, Issa II, Moos T, Thomsen MS. Astrocytic expression of ZIP14 (SLC39A14) is part of the inflammatory reaction in chronic neurodegeneration with iron overload. Glia (2020) 68(9):1810–23. doi: 10.1002/glia.23806
70. Cruz KJC, de Oliveira ARS, Morais JBS, Severo JS, Mendes PMV, de Sousa Melo SR, et al. Zinc and insulin resistance: biochemical and molecular aspects. Biol Trace Elem Res (2018) 186(2):407–12. doi: 10.1007/s12011-018-1308-z
71. Maxel T, Smidt K, Petersen CC, Honoré B, Christensen AK, Jeppesen PB, et al. The zinc transporter Zip14 (SLC39a14) affects beta-cell function: proteomics, gene expression, and insulin secretion studies in INS-1E cells. Sci Rep (2019) 9(1):8589. doi: 10.1038/s41598-019-44954-1
72. Lawson R, Maret W, Hogstrand C. Prolonged stimulation of insulin release from MIN6 cells causes zinc depletion and loss of β-cell markers. J Trace Elem Med Biol (2018) 49:51–9. doi: 10.1016/j.jtemb.2018.04.020
73. van Raaij S, van Swelm R, Bouman K, Cliteur M, van den Heuvel MC, Pertijs J, et al. Tubular iron deposition and iron handling proteins in human healthy kidney and chronic kidney disease. Sci Rep (2018) 8(1):9353. doi: 10.1038/s41598-018-27107-8
74. van Raaij SEG, Srai SKS, Swinkels DW, van Swelm RPL. Iron uptake by ZIP8 and ZIP14 in human proximal tubular epithelial cells. Biometals (2019) 32(2):211–26. doi: 10.1007/s10534-019-00183-7
75. Wan J, Ren H, Wang J. Iron toxicity, lipid peroxidation and ferroptosis after intracerebral haemorrhage. Stroke Vasc Neurol (2019) 4(2):93–5. doi: 10.1136/svn-2018-000205
76. Wu K, Fei L, Wang X, Lei Y, Yu L, Xu W, et al. ZIP14 is involved in iron deposition and triggers ferroptosis in diabetic nephropathy. Metallomics (2022) 14(7):mfac034. doi: 10.1093/mtomcs/mfac034
77. Kang R, Chen R, Zhang Q, Hou W, Wu S, Cao L, et al. HMGB1 in health and disease. Mol Aspects Med (2014) 40:1–116. doi: 10.1016/j.mam.2014.05.001
78. Wang S, Zhang Y. HMGB1 in inflammation and cancer. J Hematol Oncol (2020) 13(1):116. doi: 10.1186/s13045-020-00950-x
79. Mandke P, Vasquez KM. Interactions of high mobility group box protein 1 (HMGB1) with nucleic acids: implications in DNA repair and immune responses. DNA Repair (Amst) (2019) 83:102701. doi: 10.1016/j.dnarep.2019.102701
80. Rouillard ME, Hu J, Sutter PA, Kim HW, Huang JK, Crocker SJ. The cellular senescence factor extracellular HMGB1 directly inhibits oligodendrocyte progenitor cell differentiation and impairs CNS remyelination. Front Cell Neurosci (2022) 16:833186. doi: 10.3389/fncel.2022.833186
81. Khambu B, Yan S, Huda N, Yin XM. Role of high-mobility group box-1 in liver pathogenesis. Int J Mol Sci (2019) 20(21):5314. doi: 10.3390/ijms20215314
82. Pérez-Morales RE, Del Pino MD, Valdivielso JM, Ortiz A, Mora-Fernández C, Navarro-González JF. Inflammation in diabetic kidney disease. Nephron (2019) 143(1):12–6. doi: 10.1159/000493278
83. Matoba K, Takeda Y, Nagai Y, Kawanami D, Utsunomiya K, Nishimura R. Unraveling the role of inflammation in the pathogenesis of diabetic kidney disease. Int J Mol Sci (2019) 20(14):3393. doi: 10.3390/ijms20143393
84. Chen J, Liu Q, He J, Li Y. Immune responses in diabetic nephropathy: pathogenic mechanisms and therapeutic target. Front Immunol (2022) 13:958790. doi: 10.3389/fimmu.2022.958790
85. Tang SCW, Yiu WH. Innate immunity in diabetic kidney disease. Nat Rev Nephrol (2020) 16(4):206–22. doi: 10.1038/s41581-019-0234-4
86. Peng J, Zhou QY, Zhao SL, Wu S, Liao XY, Yi B. Correlation between high mobility group protein B1 and kidney injury in patients with type 2 diabetes mellitus. Chin J Diabetes (2022) 30(6):421–6.
87. Yao D, Wang S, Wang M, Lu W. Renoprotection of dapagliflozin in human renal proximal tubular cells via the inhibition of the high mobility group box 1−receptor for advanced glycation end products−nuclear factor−κB signaling pathway. Mol Med Rep (2018) 18(4):3625–30. doi: 10.3892/mmr.2018.9393
88. Wang J, Yang S, Li W, Zhao M, Li K. Circ_0000491 promotes apoptosis, inflammation, oxidative stress, and fibrosis in high glucose-induced mesangial cells by regulating miR-455-3p/hmgb1 axis. Nephron (2022) 146(1):72–83. doi: 10.1159/000516870
89. Jin J, Gong J, Zhao L, Zhang H, He Q, Jiang X. Inhibition of high mobility group box 1 (HMGB1) attenuates podocyte apoptosis and epithelial-mesenchymal transition by regulating autophagy flux. J Diabetes (2019) 11(10):826–36. doi: 10.1111/1753-0407.12914
90. Liu ZZ, Weng HB, Zhang LJ, Pan LY, Sun W, Chen HX, et al. Bupleurum polysaccharides ameliorated renal injury in diabetic mice associated with suppression of HMGB1-TLR4 signaling. Chin J Nat Med (2019) 17(9):641–9. doi: 10.1016/s1875-5364(19)30078-0
91. Zhang H, Wang Z, Liu Z, Du K, Lu X. Protective effects of dexazoxane on rat ferroptosis in doxorubicin-induced cardiomyopathy through regulating HMGB1. Front Cardiovasc Med (2021) 8:685434. doi: 10.3389/fcvm.2021.685434
92. Wang X, Simayi A, Fu J, Zhao X, Xu G. Resveratrol mediates the miR-149/HMGB1 axis and regulates the ferroptosis pathway to protect myocardium in endotoxemia mice. Am J Physiol Endocrinol Metab (2022) 323(1):E21–32. doi: 10.1152/ajpendo.00227.2021
93. Wen Q, Liu J, Kang R, Zhou B, Tang D. The release and activity of HMGB1 in ferroptosis. Biochem Biophys Res Commun (2019) 510(2):278–83. doi: 10.1016/j.bbrc.2019.01.090
94. Ye F, Chai W, Xie M, Yang M, Yu Y, Cao L, et al. HMGB1 regulates erastin-induced ferroptosis via RAS-JNK/p38 signaling in HL-60/NRAS(Q61L) cells. Am J Cancer Res (2019) 9(4):730–9.
95. Zhao Z, Li G, Wang Y, Li Y, Xu H, Liu W, et al. Cytoplasmic HMGB1 induces renal tubular ferroptosis after ischemia/reperfusion. Int Immunopharmacol (2023) 116:109757. doi: 10.1016/j.intimp.2023.109757
96. Wu Y, Zhao Y, Yang HZ, Wang YJ, Chen Y. HMGB1 regulates ferroptosis through Nrf2 pathway in mesangial cells in response to high glucose. Biosci Rep (2021) 41(2):BSR20202924. doi: 10.1042/bsr20202924
97. He F, Ru X, Wen T. NRF2, a transcription factor for stress response and beyond. Int J Mol Sci (2020) 21(13):4777. doi: 10.3390/ijms21134777
98. Nie P, Lou Y, Bai X, Zhu Y, Guo Q, Luo P, et al. Influence of zinc levels and Nrf2 expression in the clinical and pathological changes in patients with diabetic nephropathy. Nutr Diabetes (2022) 12(1):37. doi: 10.1038/s41387-022-00212-4
99. Liu Y, Uruno A, Saito R, Matsukawa N, Hishinuma E, Saigusa D, et al. Nrf2 deficiency deteriorates diabetic kidney disease in akita model mice. Redox Biol (2022) 58:102525. doi: 10.1016/j.redox.2022.102525
100. Ma L, Wu F, Shao Q, Chen G, Xu L, Lu F. Baicalin alleviates oxidative stress and inflammation in diabetic nephropathy via Nrf2 and MAPK signaling pathway. Drug Des Devel Ther (2021) 15:3207–21. doi: 10.2147/dddt.S319260
101. Duan Q, Tian L, Feng J, Ping X, Li L, Yaigoub H, et al. Trametenolic acid ameliorates the progression of diabetic nephropathy in db/db mice via Nrf2/HO-1 and NF-κB-mediated pathways. J Immunol Res (2022) 2022:6151847. doi: 10.1155/2022/6151847
102. Bertrand RL. Iron accumulation, glutathione depletion, and lipid peroxidation must occur simultaneously during ferroptosis and are mutually amplifying events. Med Hypotheses (2017) 101:69–74. doi: 10.1016/j.mehy.2017.02.017
103. Anandhan A, Dodson M, Schmidlin CJ, Liu P, Zhang DD. Breakdown of an ironclad defense system: the critical role of NRF2 in mediating ferroptosis. Cell Chem Biol (2020) 27(4):436–47. doi: 10.1016/j.chembiol.2020.03.011
104. Lu J, Zhao Y, Liu M, Lu J, Guan S. Toward improved human health: Nrf2 plays a critical role in regulating ferroptosis. Food Funct (2021) 12(20):9583–606. doi: 10.1039/d1fo01036k
105. Dodson M, Castro-Portuguez R, Zhang DD. NRF2 plays a critical role in mitigating lipid peroxidation and ferroptosis. Redox Biol (2019) 23:101107. doi: 10.1016/j.redox.2019.101107
106. Tian H, Xiong Y, Zhang Y, Leng Y, Tao J, Li L, et al. Activation of NRF2/FPN1 pathway attenuates myocardial ischemia-reperfusion injury in diabetic rats by regulating iron homeostasis and ferroptosis. Cell Stress Chaperones (2021) 27(2):149–64. doi: 10.1007/s12192-022-01257-1
107. Kerins MJ, Ooi A. The roles of NRF2 in modulating cellular iron homeostasis. Antioxid Redox Signal (2018) 29(17):1756–73. doi: 10.1089/ars.2017.7176
108. Li S, Zheng L, Zhang J, Liu X, Wu Z. Inhibition of ferroptosis by up-regulating Nrf2 delayed the progression of diabetic nephropathy. Free Radic Biol Med (2021) 162:435–49. doi: 10.1016/j.freeradbiomed.2020.10.323
109. Wang WJ, Jiang X, Gao CC, Chen ZW. Salusin−β participates in high glucose−induced HK−2 cell ferroptosis in a Nrf−2−dependent manner. Mol Med Rep (2021) 24(3):674. doi: 10.3892/mmr.2021.12313
110. Forcina GC, Dixon SJ. GPX4 at the crossroads of lipid homeostasis and ferroptosis. Proteomics (2019) 19(18):e1800311. doi: 10.1002/pmic.201800311
111. Seibt TM, Proneth B, Conrad M. Role of GPX4 in ferroptosis and its pharmacological implication. Free Radic Biol Med (2019) 133:144–52. doi: 10.1016/j.freeradbiomed.2018.09.014
112. Nishida Xavier da Silva T, Friedmann Angeli JP, Ingold I. GPX4: old lessons, new features. Biochem Soc Trans (2022) 50(3):1205–13. doi: 10.1042/bst20220682
113. Yang WS, SriRamaratnam R, Welsch ME, Shimada K, Skouta R, Viswanathan VS, et al. Regulation of ferroptotic cancer cell death by GPX4. Cell (2014) 156(1-2):317–31. doi: 10.1016/j.cell.2013.12.010
114. Friedmann Angeli JP, Schneider M, Proneth B, Tyurina YY, Tyurin VA, Hammond VJ, et al. Inactivation of the ferroptosis regulator Gpx4 triggers acute renal failure in mice. Nat Cell Biol (2014) 16(12):1180–91. doi: 10.1038/ncb3064
115. Lu Q, Yang L, Xiao JJ, Liu Q, Ni L, Hu JW, et al. Empagliflozin attenuates the renal tubular ferroptosis in diabetic kidney disease through AMPK/NRF2 pathway. Free Radic Biol Med (2023) 195:89–102. doi: 10.1016/j.freeradbiomed.2022.12.088
116. Kim S, Kang SW, Joo J, Han SH, Shin H, Nam BY, et al. Characterization of ferroptosis in kidney tubular cell death under diabetic conditions. Cell Death Dis (2021) 12(2):160. doi: 10.1038/s41419-021-03452-x
117. Wang YH, Chang DY, Zhao MH, Chen M. Glutathione peroxidase 4 is a predictor of diabetic kidney disease progression in type 2 diabetes mellitus. Oxid Med Cell Longev (2022) 2022:2948248. doi: 10.1155/2022/2948248
118. Li Q, Liao J, Chen W, Zhang K, Li H, Ma F, et al. NAC alleviative ferroptosis in diabetic nephropathy via maintaining mitochondrial redox homeostasis through activating SIRT3-SOD2/Gpx4 pathway. Free Radic Biol Med (2022) 187:158–70. doi: 10.1016/j.freeradbiomed.2022.05.024
119. Gharib AF, Askary AE, Almehmadi M, Etewa RL, Althobaiti BB, Allam HH, et al. Vitamin d and hypoxia-inducible factor (HIF-1α) serum levels as markers for progression of nephropathy in type 2 diabetic patients. Clin Lab (2022) 68(4). doi: 10.7754/Clin.Lab.2021.210540
120. Sagar SK, Zhang C, Guo Q, Yi R. Role of expression of endothelin-1 and angiotensin-II and hypoxia-inducible factor-1α in the kidney tissues of patients with diabetic nephropathy. Saudi J Kidney Dis Transpl (2013) 24(5):959–64. doi: 10.4103/1319-2442.118098
121. Pang X, Zhang Y, Shi X, Peng Z, Xing Y, Jiarui H. Hirudin reduces the expression of markers of the extracellular matrix in renal tubular epithelial cells in a rat model of diabetic kidney disease through the hypoxia-inducible factor-1α (HIF-1α)/vascular endothelial growth factor (VEGF) signaling pathway. Med Sci Monit (2020) 26:e921894. doi: 10.12659/msm.921894
122. Nakashima M, Watanabe M, Nakano K, Uchimaru K, Horie R. Differentiation of Hodgkin lymphoma cells by reactive oxygen species and regulation by heme oxygenase-1 through HIF-1α. Cancer Sci (2021) 112(6):2542–55. doi: 10.1111/cas.14890
123. Jiang N, Zhao H, Han Y, Li L, Xiong S, Zeng L, et al. HIF-1α ameliorates tubular injury in diabetic nephropathy via HO-1-mediated control of mitochondrial dynamics. Cell Prolif (2020) 53(11):e12909. doi: 10.1111/cpr.12909
124. Wu Y, Wang J, Zhao T, Chen J, Kang L, Wei Y, et al. Di-(2-ethylhexyl) phthalate exposure leads to ferroptosis via the HIF-1α/HO-1 signaling pathway in mouse testes. J Hazard Mater (2022) 426:127807. doi: 10.1016/j.jhazmat.2021.127807
125. Zhou ZY, Wang W, Xiong J, Fan GH. Isoliquiritin apioside relieves intestinal ischemia/reperfusion-induced acute lung injury by blocking hif-1α-mediated ferroptosis. Int Immunopharmacol (2022) 108:108852. doi: 10.1016/j.intimp.2022.108852
126. Tang Z, Ju Y, Dai X, Ni N, Liu Y, Zhang D, et al. HO-1-mediated ferroptosis as a target for protection against retinal pigment epithelium degeneration. Redox Biol (2021) 43:101971. doi: 10.1016/j.redox.2021.101971
127. Li D, Wang T, Lai J, Zeng D, Chen W, Zhang X, et al. Silencing TRPM2 enhanced erastin- and RSL3-induced ferroptosis in gastric cancer cells through destabilizing HIF-1α and Nrf2 proteins. Cytotechnology (2022) 74(5):559–77. doi: 10.1007/s10616-022-00545-z
128. Ma C, Wu X, Zhang X, Liu X, Deng G. Heme oxygenase-1 modulates ferroptosis by fine-tuning levels of intracellular iron and reactive oxygen species of macrophages in response to bacillus calmette-guerin infection. Front Cell Infect Microbiol (2022) 12:1004148. doi: 10.3389/fcimb.2022.1004148
129. Kwon MY, Park E, Lee SJ, Chung SW. Heme oxygenase-1 accelerates erastin-induced ferroptotic cell death. Oncotarget (2015) 6(27):24393–403. doi: 10.18632/oncotarget.5162
130. Adedoyin O, Boddu R, Traylor A, Lever JM, Bolisetty S, George JF, et al. Heme oxygenase-1 mitigates ferroptosis in renal proximal tubule cells. Am J Physiol Renal Physiol (2018) 314(5):F702–14. doi: 10.1152/ajprenal.00044.2017
131. Feng X, Wang S, Sun Z, Dong H, Yu H, Huang M, et al. Ferroptosis enhanced diabetic renal tubular injury via HIF-1α/HO-1 pathway in db/db mice. Front Endocrinol (Lausanne) (2021) 12:626390. doi: 10.3389/fendo.2021.626390
132. Yoshioka Y, Kubota Y, Samukawa Y, Yamashita Y, Ashida H. Glabridin inhibits dexamethasone-induced muscle atrophy. Arch Biochem Biophys (2019) 664:157–66. doi: 10.1016/j.abb.2019.02.006
133. Komolkriengkrai M, Nopparat J, Vongvatcharanon U, Anupunpisit V, Khimmaktong W. Effect of glabridin on collagen deposition in liver and amelioration of hepatocyte destruction in diabetes rats. Exp Ther Med (2019) 18(2):1164–74. doi: 10.3892/etm.2019.7664
134. Hasanein P. Glabridin as a major active isoflavan from glycyrrhiza glabra (licorice) reverses learning and memory deficits in diabetic rats. Acta Physiol Hung (2011) 98(2):221–30. doi: 10.1556/APhysiol.98.2011.2.14
135. Wu F, Jin Z and Jin J. Hypoglycemic effects of glabridin, a polyphenolic flavonoid from licorice, in an animal model of diabetes mellitus. Mol Med Rep (2013) 7(4):1278–82. doi: 10.3892/mmr.2013.1330
136. Tan H, Chen J, Li Y, Li Y, Zhong Y, Li G, et al. Glabridin, a bioactive component of licorice, ameliorates diabetic nephropathy by regulating ferroptosis and the VEGF/Akt/ERK pathways. Mol Med (2022) 28(1):58. doi: 10.1186/s10020-022-00481-w
137. Deng M, Chen H, Long J, Song J, Xie L, Li X. Calycosin: a review of its pharmacological effects and application prospects. Expert Rev Anti Infect Ther (2021) 19(7):911–25. doi: 10.1080/14787210.2021.1863145
138. Yosri H, El-Kashef DH, El-Sherbiny M, Said E, Salem HA. Calycosin modulates NLRP3 and TXNIP-mediated pyroptotic signaling and attenuates diabetic nephropathy progression in diabetic rats; an insight. BioMed Pharmacother (2022) 155:113758. doi: 10.1016/j.biopha.2022.113758
139. Zhang YY, Tan RZ, Zhang XQ, Yu Y, Yu C. Calycosin ameliorates diabetes-induced renal inflammation via the NF-κB pathway in vitro and in vivo. Med Sci Monit (2019) 25:1671–8. doi: 10.12659/msm.915242
140. Elsherbiny NM, Said E, Atef H, Zaitone SA. Renoprotective effect of calycosin in high fat diet-fed/STZ injected rats: effect on IL-33/ST2 signaling, oxidative stress and fibrosis suppression. Chem Biol Interact (2020) 315:108897. doi: 10.1016/j.cbi.2019.108897
141. Huang D, Shen P, Wang C, Gao J, Ye C, Wu F. Calycosin plays a protective role in diabetic kidney disease through the regulation of ferroptosis. Pharm Biol (2022) 60(1):990–6. doi: 10.1080/13880209.2022.2067572
142. Zhang D, Zhu P, Liu Y, Shu Y, Zhou JY, Jiang F, et al. Total flavone of abelmoschus manihot ameliorates crohn's disease by regulating the NF−κB and MAPK signaling pathways. Int J Mol Med (2019) 44(1):324–34. doi: 10.3892/ijmm.2019.4180
143. Bu F, Ding Y, Chen T, Wang Q, Wang R, Zhou JY, et al. Total flavone of abelmoschus manihot improves colitis by promoting the growth of akkermansia in mice. Sci Rep (2021) 11(1):20787. doi: 10.1038/s41598-021-00070-7
144. Liu S, Ye L, Tao J, Ge C, Huang L, Yu J. Total flavones of abelmoschus manihot improve diabetic nephropathy by inhibiting the iRhom2/TACE signalling pathway activity in rats. Pharm Biol (2017) 56(1):1–11. doi: 10.1080/13880209.2017.1412467
145. Liu BH, Tu Y, Ni GX, Yan J, Yue L, Li ZL, et al. Total flavones of abelmoschus manihot ameliorates podocyte pyroptosis and injury in high glucose conditions by targeting METTL3-dependent m(6)A modification-mediated NLRP3-inflammasome activation and PTEN/PI3K/Akt signaling. Front Pharmacol (2021) 12:667644. doi: 10.3389/fphar.2021.667644
146. Tu Y, Fang QJ, Sun W, Liu BH, Liu YL, Wu W, et al. Total flavones of abelmoschus manihot remodels gut microbiota and inhibits microinflammation in chronic renal failure progression by targeting autophagy-mediated macrophage polarization. Front Pharmacol (2020) 11:566611. doi: 10.3389/fphar.2020.566611
147. Wang MZ, Cai YF, Fang QJ, Liu YL, Wang J, Chen JX, et al. Inhibition of ferroptosis of renal tubular cells with total flavones of abelmoschus manihot alleviates diabetic tubulopathy. Anat Rec (Hoboken) (2022). doi: 10.1002/ar.25123
148. Sha H, Zeng H, Zhao J, Jin H. Mangiferin ameliorates gestational diabetes mellitus-induced placental oxidative stress, inflammation and endoplasmic reticulum stress and improves fetal outcomes in mice. Eur J Pharmacol (2019) 859:172522. doi: 10.1016/j.ejphar.2019.172522
149. Feng M, Wei S, Zhang S, Yang Y. Anti-inflammation and anti-pyroptosis activities of mangiferin via suppressing NF-κB/NLRP3/GSDMD signaling cascades. Int J Mol Sci (2022) 23(17):10124. doi: 10.3390/ijms231710124
150. Wang M, Liang Y, Chen K, Wang M, Long X, Liu H, et al. The management of diabetes mellitus by mangiferin: advances and prospects. Nanoscale (2022) 14(6):2119–35. doi: 10.1039/d1nr06690k
151. Morozkina SN, Nhung Vu TH, Generalova YE, Snetkov PP, Uspenskaya MV. Mangiferin as new potential anti-cancer agent and mangiferin-integrated polymer systems-a novel research direction. Biomolecules (2021) 11(1):79. doi: 10.3390/biom11010079
152. Zhang L, Huang C, Fan S. Mangiferin and organ fibrosis: a mini review. Biofactors (2021) 47(1):59–68. doi: 10.1002/biof.1693
153. Akter S, Moni A, Faisal GM, Uddin MR, Jahan N, Hannan MA, et al. Renoprotective effects of mangiferin: pharmacological advances and future perspectives. Int J Environ Res Public Health (2022) 19(3):1864. doi: 10.3390/ijerph19031864
154. Jiang T, Han F, Gao G, Liu M. Mangiferin exert cardioprotective and anti-apoptotic effects in heart failure induced rats. Life Sci (2020) 249:117476. doi: 10.1016/j.lfs.2020.117476
155. Liu T, Song Y, Hu A. Neuroprotective mechanisms of mangiferin in neurodegenerative diseases. Drug Dev Res (2021) 82(4):494–502. doi: 10.1002/ddr.21783
156. Mei S, Perumal M, Battino M, Kitts DD, Xiao J, Ma H, et al. Mangiferin: a review of dietary sources, absorption, metabolism, bioavailability, and safety. Crit Rev Food Sci Nutr (2021), 1–19. doi: 10.1080/10408398.2021.1983767
157. Guo HB, Chen MQ, Li MR, Hu MY, Chen BH, Zhou CY. Pharmacokinetic comparisons of mangiferin and mangiferin monosodium salt in rat plasma by UPLC-MS/MS. J Chem (2019) 2019:9272710. doi: 10.1155/2019/9272710
158. Zhao C, Pu Z, Gao J, Liu C, Xing J, Lang W, et al. "Multiomics" analyses combined with systems pharmacology reveal the renoprotection of mangiferin monosodium salt in rats with diabetic nephropathy: focus on improvements in renal ferroptosis, renal inflammation, and podocyte insulin resistance. J Agric Food Chem (2023) 71(1):358–81. doi: 10.1021/acs.jafc.2c05595
159. Sun ZG, Zhao TT, Lu N, Yang YA, Zhu HL. Research progress of glycyrrhizic acid on antiviral activity. Mini Rev Med Chem (2019) 19(10):826–32. doi: 10.2174/1389557519666190119111125
160. Cao ZY, Liu YZ, Li JM, Ruan YM, Yan WJ, Zhong SY, et al. Glycyrrhizic acid as an adjunctive treatment for depression through anti-inflammation: a randomized placebo-controlled clinical trial. J Affect Disord (2020) 265:247–54. doi: 10.1016/j.jad.2020.01.048
161. Rehman MU, Farooq A, Ali R, Bashir S, Bashir N, Majeed S, et al. Preclinical evidence for the pharmacological actions of glycyrrhizic acid: a comprehensive review. Curr Drug Metab (2020) 21(6):436–65. doi: 10.2174/1389200221666200620204914
162. Chen K, Yang R, Shen FQ, Zhu HL. Advances in pharmacological activities and mechanisms of glycyrrhizic acid. Curr Med Chem (2020) 27(36):6219–43. doi: 10.2174/0929867325666191011115407
163. Hou S, Zhang T, Li Y, Guo F, Jin X. Glycyrrhizic acid prevents diabetic nephropathy by activating AMPK/SIRT1/PGC-1α signaling in db/db mice. J Diabetes Res (2017) 2017:2865912. doi: 10.1155/2017/2865912
164. Wang YJ, Wang Z, Zhao TQ, Hou SZ. Effect of glycyrrhizic acid on high glucose induced podocyte injury in mice. J Ningxia Med Univ (2022) 44(3):267–71. doi: 10.16050/j.cnki.issn1674-6309.2022.03.009
165. Hu H, Li Y, Xin Z, Zhanga X. Ginkgolide b exerts anti-inflammatory and chondroprotective activity in LPS-induced chondrocytes. Adv Clin Exp Med (2018) 27(7):913–20. doi: 10.17219/acem/70414
166. Lin D, Wu H, Zhou Z, Tao Z, Jia T, Gao W. Ginkgolide b improves multiterritory perforator flap survival by inhibiting endoplasmic reticulum stress and oxidative stress. J Invest Surg (2021) 34(6):610–6. doi: 10.1080/08941939.2019.1676483
167. Liu J, Wu P, Xu Z, Zhang J, Liu J, Yang Z. Ginkgolide b inhibits hydrogen peroxide−induced apoptosis and attenuates cytotoxicity via activating the PI3K/Akt/mTOR signaling pathway in H9c2 cells. Mol Med Rep (2020) 22(1):310–6. doi: 10.3892/mmr.2020.11099
168. Yang Y, Chen J, Gao Q, Shan X, Wang J, Lv Z. Study on the attenuated effect of ginkgolide b on ferroptosis in high fat diet induced nonalcoholic fatty liver disease. Toxicology (2020) 445:152599. doi: 10.1016/j.tox.2020.152599
169. Shao L, Dong C, Geng D, He Q, Shi Y. Ginkgolide b protects against cognitive impairment in senescence-accelerated P8 mice by mitigating oxidative stress, inflammation and ferroptosis. Biochem Biophys Res Commun (2021) 572:7–14. doi: 10.1016/j.bbrc.2021.07.081
170. Chen J, Ou Z, Gao T, Yang Y, Shu A, Xu H, et al. Ginkgolide b alleviates oxidative stress and ferroptosis by inhibiting GPX4 ubiquitination to improve diabetic nephropathy. BioMed Pharmacother (2022) 156:113953. doi: 10.1016/j.biopha.2022.113953
171. Fang X, Tan T, Gao B, Zhao Y, Liu T, Xia Q. Germacrone regulates HBXIP-mediated cell cycle, apoptosis and promotes the formation of autophagosomes to inhibit the proliferation of gastric cancer cells. Front Oncol (2020) 10:537322. doi: 10.3389/fonc.2020.537322
172. He W, Zhai X, Su J, Ye R, Zheng Y, Su S. Antiviral activity of germacrone against pseudorabies virus in vitro. Pathogens (2019) 8(4):258. doi: 10.3390/pathogens8040258
173. Li Z, Wang Z, Dong F, Shi W, Dai W, Zhao J, et al. Germacrone attenuates hepatic stellate cells activation and liver fibrosis via regulating multiple signaling pathways. Front Pharmacol (2021) 12:745561. doi: 10.3389/fphar.2021.745561
174. Zhuang S, Liu B, Guo S, Xue Y, Wu L, Liu S, et al. Germacrone alleviates neurological deficits following traumatic brain injury by modulating neuroinflammation and oxidative stress. BMC Complement Med Ther (2021) 21(1):6. doi: 10.1186/s12906-020-03175-0
175. Soodvilai S, Meetam P, Siangjong L, Chokchaisiri R, Suksamrarn A, Soodvilai S. Germacrone reduces cisplatin-induced toxicity of renal proximal tubular cells via inhibition of organic cation transporter. Biol Pharm Bull (2020) 43(11):1693–8. doi: 10.1248/bpb.b20-00392
176. Wang Y, Feng F, He W, Sun L, He Q and Jin J. miR-188-3p abolishes germacrone-mediated podocyte protection in a mouse model of diabetic nephropathy in type I diabetes through triggering mitochondrial injury. Bioengineered (2022) 13(1):774–88. doi: 10.1080/21655979.2021.2012919
177. Jin J, Wang Y, Zheng D, Liang M, He Q. A novel identified circular RNA, mmu_mmu_circRNA_0000309, involves in germacrone-mediated improvement of diabetic nephropathy through regulating ferroptosis by targeting miR-188-3p/GPX4 signaling axis. Antioxid Redox Signal (2022) 36(10-12):740–59. doi: 10.1089/ars.2021.0063
178. Cao Y, He Y, Wei C, Li J, Qu L, Zhang H, et al. Aquaporins alteration profiles revealed different actions of senna, sennosides, and sennoside a in diarrhea-rats. Int J Mol Sci (2018) 19(10):3210. doi: 10.3390/ijms19103210
179. Wei Z, Shen P, Cheng P, Lu Y, Wang A, Sun Z. Gut bacteria selectively altered by sennoside a alleviate type 2 diabetes and obesity traits. Oxid Med Cell Longev (2020) 2020:2375676. doi: 10.1155/2020/2375676
180. Le J, Zhang X, Jia W, Zhang Y, Luo J, Sun Y, et al. Regulation of microbiota-GLP1 axis by sennoside a in diet-induced obese mice. Acta Pharm Sin B (2019) 9(4):758–68. doi: 10.1016/j.apsb.2019.01.014
181. Ma L, Cao X, Ye X, Ye J, Sun Y. Sennoside a induces GLP-1 secretion through activation of the ERK1/2 pathway in l-cells. Diabetes Metab Syndr Obes (2020) 13:1407–15. doi: 10.2147/dmso.S247251
182. Le J, Fu Y, Han Q, Ma Y, Ji H, Wei X, et al. Transcriptome analysis of the inhibitory effect of sennoside a on the metastasis of hepatocellular carcinoma cells. Front Pharmacol (2020) 11:566099. doi: 10.3389/fphar.2020.566099
183. Zhu H, Zhao H, Xu S, Zhang Y, Ding Y, Li J, et al. Sennoside a alleviates inflammatory responses by inhibiting the hypermethylation of SOCS1 in CCl(4)-induced liver fibrosis. Pharmacol Res (2021) 174:105926. doi: 10.1016/j.phrs.2021.105926
184. Zhu H, He C, Zhao H, Jiang W, Xu S, Li J, et al. Sennoside a prevents liver fibrosis by binding DNMT1 and suppressing DNMT1-mediated PTEN hypermethylation in HSC activation and proliferation. FASEB J (2020) 34(11):14558–71. doi: 10.1096/fj.202000494RR
185. Ding Y, Wang L. Mechanism exploration of sennoside a in treating DN based on Nrf2/HMOX-1 ferroptosis signaling pathway. Inf Traditional Chin Med (2021) 38(7):36–9. doi: 10.19656/j.cnki.1002-2406.20210706
186. Wang Y, Liu H, Zheng M, Yang Y, Ren H, Kong Y, et al. Berberine slows the progression of prediabetes to diabetes in zucker diabetic fatty rats by enhancing intestinal secretion of glucagon-like peptide-2 and improving the gut microbiota. Front Endocrinol (Lausanne) (2021) 12:609134. doi: 10.3389/fendo.2021.609134
187. Wang S, Ren H, Zhong H, Zhao X, Li C, Ma J, et al. Combined berberine and probiotic treatment as an effective regimen for improving postprandial hyperlipidemia in type 2 diabetes patients: a double blinded placebo controlled randomized study. Gut Microbes (2022) 14(1):2003176. doi: 10.1080/19490976.2021.2003176
188. Warowicka A, Nawrot R, Goździcka-Józefiak A. Antiviral activity of berberine. Arch Virol (2020) 165(9):1935–45. doi: 10.1007/s00705-020-04706-3
189. Rauf A, Abu-Izneid T, Khalil AA, Imran M, Shah ZA, Emran TB, et al. Berberine as a potential anticancer agent: a comprehensive review. Molecules (2021) 26(23):7368. doi: 10.3390/molecules26237368
190. Ehteshamfar SM, Akhbari M, Afshari JT, Seyedi M, Nikfar B, Shapouri-Moghaddam A, et al. Anti-inflammatory and immune-modulatory impacts of berberine on activation of autoreactive T cells in autoimmune inflammation. J Cell Mol Med (2020) 24(23):13573–88. doi: 10.1111/jcmm.16049
191. Ma X, Chen Z, Wang L, Wang G, Wang Z, Dong X, et al. The pathogenesis of diabetes mellitus by oxidative stress and inflammation: its inhibition by berberine. Front Pharmacol (2018) 9:782. doi: 10.3389/fphar.2018.00782
192. Zhou M, Deng Y, Liu M, Liao L, Dai X, Guo C, et al. The pharmacological activity of berberine, a review for liver protection. Eur J Pharmacol (2021) 890:173655. doi: 10.1016/j.ejphar.2020.173655
193. Akbar M, Shabbir A, Rehman K, Akash MSH, Shah MA. Neuroprotective potential of berberine in modulating alzheimer's disease via multiple signaling pathways. J Food Biochem (2021) 45(10):e13936. doi: 10.1111/jfbc.13936
194. Zhang Y, Gu Y, Ren H, Wang S, Zhong H, Zhao X, et al. Gut microbiome-related effects of berberine and probiotics on type 2 diabetes (the PREMOTE study). Nat Commun (2020) 11(1):5015. doi: 10.1038/s41467-020-18414-8
195. Hu S, Wang J, Liu E, Zhang X, Xiang J, Li W, et al. Protective effect of berberine in diabetic nephropathy: a systematic review and meta-analysis revealing the mechanism of action. Pharmacol Res (2022) 185:106481. doi: 10.1016/j.phrs.2022.106481
196. Wang N, Zhang C, Xu Y, Tan HY, Chen H, Feng Y. Berberine improves insulin-induced diabetic retinopathy through exclusively suppressing Akt/mTOR-mediated HIF-1α/VEGF activation in retina endothelial cells. Int J Biol Sci (2021) 17(15):4316–26. doi: 10.7150/ijbs.62868
197. Dong S, Zhang S, Chen Z, Zhang R, Tian L, Cheng L, et al. Berberine could ameliorate cardiac dysfunction via interfering myocardial lipidomic profiles in the rat model of diabetic cardiomyopathy. Front Physiol (2018) 9:1042. doi: 10.3389/fphys.2018.01042
198. Song C, Li D, Zhang J, Zhao X. Berberine hydrochloride alleviates imatinib mesylate - induced cardiotoxicity through the inhibition of Nrf2-dependent ferroptosis. Food Funct (2023) 14(2):1087–98. doi: 10.1039/d2fo03331c
199. Yi J, Wu S, Tan S, Qin Y, Wang X, Jiang J, et al. Berberine alleviates liver fibrosis through inducing ferrous redox to activate ROS-mediated hepatic stellate cells ferroptosis. Cell Death Discov (2021) 7(1):374. doi: 10.1038/s41420-021-00768-7
200. Bao L, Jin Y, Han J, Wang W, Qian L, Wu W. Berberine regulates GPX4 to inhibit ferroptosis of islet β cells. Planta Med (2023) 89(3):254–61. doi: 10.1055/a-1939-7417
201. Guan XM, Xie YS, Ni WJ, Tang LQ. Influence of Nrf2/HO-1/GPX4 signaling pathway on high glucose-induced podocyte ferroptosis and intervention of berberine. Chin Pharmacol Bull (2021) 37(3):396–403.
202. Liu X, Cao W, Qi J, Li Q, Zhao M, Chen Z, et al. Leonurine ameliorates adriamycin-induced podocyte injury via suppression of oxidative stress. Free Radic Res (2018) 52(9):952–60. doi: 10.1080/10715762.2018.1500021
203. Zhang Q, Sun Q, Tong Y, Bi X, Chen L, Lu J, et al. Leonurine attenuates cisplatin nephrotoxicity by suppressing the NLRP3 inflammasome, mitochondrial dysfunction, and endoplasmic reticulum stress. Int Urol Nephrol (2022) 54(9):2275–84. doi: 10.1007/s11255-021-03093-1
204. Han L, Chen A, Liu L, Wang F. Leonurine preconditioning attenuates ischemic acute kidney injury in rats by promoting Nrf2 nuclear translocation and suppressing TLR4/NF-κB pathway. Chem Pharm Bull (Tokyo) (2022) 70(1):66–73. doi: 10.1248/cpb.c21-00740
205. Zheng S, Zhuang T, Tang Y, Wu R, Xu T, Leng T, et al. Leonurine protects against ulcerative colitis by alleviating inflammation and modulating intestinal microflora in mouse models. Exp Ther Med (2021) 22(5):1199. doi: 10.3892/etm.2021.10633
206. Xu W, Cui J, Zhou F, Bai M, Deng R, Wang W. Leonurine protects against dexamethasone-induced cytotoxicity in pancreatic β-cells via PI3K/Akt signaling pathway. Biochem Biophys Res Commun (2020) 529(3):652–8. doi: 10.1016/j.bbrc.2020.05.184
207. Huang L, Yang X, Peng A, Wang H, Lei X, Zheng L, et al. Inhibitory effect of leonurine on the formation of advanced glycation end products. Food Funct (2015) 6(2):584–9. doi: 10.1039/c4fo00960f
208. Hu J, Gu W, Ma N, Fan X, Ci X. Leonurine alleviates ferroptosis in cisplatin-induced acute kidney injury by activating the Nrf2 signalling pathway. Br J Pharmacol (2022) 179(15):3991–4009. doi: 10.1111/bph.15834
209. Wu AJ, Chen NQ, Huang LH, Cheng R, Wang XW, Li C, et al. Leonurine inhibits ferroptosis in renal tubular epithelial cells by activating p62/Nrf2/HO-1 signaling pathway. China J Chin Materia Med (2023) 48(8):2176–83. doi: 10.19540/j.cnki.cjcmm.20221115.401
210. Ramesh B, Pugalendi KV. Antihyperlipidemic and antidiabetic effects of umbelliferone in streptozotocin diabetic rats. Yale J Biol Med (2005) 78(4):189–96.
211. Cruz LF, Figueiredo GF, Pedro LP, Amorin YM, Andrade JT, Passos TF, et al. Umbelliferone (7-hydroxycoumarin): a non-toxic antidiarrheal and antiulcerogenic coumarin. BioMed Pharmacother (2020) 129:110432. doi: 10.1016/j.biopha.2020.110432
212. Cai L, Zhou MY, Hu S, Liu FY, Wang MQ, Wang XH, et al. Umbelliferone inhibits migration, invasion and inflammation of rheumatoid arthritis fibroblast-like synoviocytes and relieves adjuvant-induced arthritis in rats by blockade of wnt/β-catenin signaling pathway. Am J Chin Med (2022) 50(7):1945–62. doi: 10.1142/s0192415x22500835
213. Yu SM, Hu DH, Zhang JJ. Umbelliferone exhibits anticancer activity via the induction of apoptosis and cell cycle arrest in HepG2 hepatocellular carcinoma cells. Mol Med Rep (2015) 12(3):3869–73. doi: 10.3892/mmr.2015.3797
214. Zhao L, Jin X, Xiong Z, Tang H, Guo H, Ye G, et al. The antivirulence activity of umbelliferone and its protective effect against a. hydrophila-infected grass carp. Int J Mol Sci (2022) 23(19):11119. doi: 10.3390/ijms231911119
215. Hindam MO, Sayed RH, Skalicka-Woźniak K, Budzyńska B, El Sayed NS. Xanthotoxin and umbelliferone attenuate cognitive dysfunction in a streptozotocin-induced rat model of sporadic alzheimer's disease: the role of JAK2/STAT3 and Nrf2/HO-1 signalling pathway modulation. Phytother Res (2020) 34(9):2351–65. doi: 10.1002/ptr.6686
216. Liang S, Chen Z, Li H, Cang Z, Yin K, Wu M, et al. Neuroprotective effect of umbelliferone against cerebral ischemia/reperfusion induced neurological deficits: in-vivo and in-silico studies. J Biomol Struct Dyn (2021) 39(13):4715–25. doi: 10.1080/07391102.2020.1780153
217. Althunibat OY, Abduh MS, Abukhalil MH, Aladaileh SH, Hanieh H, Mahmoud AM. Umbelliferone prevents isoproterenol-induced myocardial injury by upregulating Nrf2/HO-1 signaling, and attenuating oxidative stress, inflammation, and cell death in rats. BioMed Pharmacother (2022) 149:112900. doi: 10.1016/j.biopha.2022.112900
218. Khadrawy SM, El Sayed RA. Umbelliferone attenuates diabetic cardiomyopathy by suppression of JAK/STAT signaling pathway through amelioration of oxidative stress and inflammation in rats. J Biochem Mol Toxicol (2023) 37(4):e23296. doi: 10.1002/jbt.23296
219. Wu Z, Geng Y, Buist-Homan M, Moshage H. Scopoletin and umbelliferone protect hepatocytes against palmitate- and bile acid-induced cell death by reducing endoplasmic reticulum stress and oxidative stress. Toxicol Appl Pharmacol (2022) 436:115858. doi: 10.1016/j.taap.2021.115858
220. Hassanein EHM, Ali FEM, Kozman MR, Abd El-Ghafar OAM. Umbelliferone attenuates gentamicin-induced renal toxicity by suppression of TLR-4/NF-κB-p65/NLRP-3 and JAK1/STAT-3 signaling pathways. Environ Sci Pollut Res Int (2021) 28(9):11558–71. doi: 10.1007/s11356-020-11416-5
221. Wang HQ, Wang SS, Chiufai K, Wang Q, Cheng XL. Umbelliferone ameliorates renal function in diabetic nephropathy rats through regulating inflammation and TLR/NF-κB pathway. Chin J Nat Med (2019) 17(5):346–54. doi: 10.1016/s1875-5364(19)30040-8
222. Jin T, Chen C. Umbelliferone delays the progression of diabetic nephropathy by inhibiting ferroptosis through activation of the nrf-2/HO-1 pathway. Food Chem Toxicol (2022) 163:112892. doi: 10.1016/j.fct.2022.112892
223. Fu K, Zhou H, Wang C, Gong L, Ma C, Zhang Y, et al. A review: pharmacology and pharmacokinetics of schisandrin a. Phytother Res (2022) 36(6):2375–93. doi: 10.1002/ptr.7456
224. Wang X, Li Q, Sui B, Xu M, Pu Z, Qiu T. Schisandrin a from schisandra chinensis attenuates ferroptosis and NLRP3 inflammasome-mediated pyroptosis in diabetic nephropathy through mitochondrial damage by AdipoR1 ubiquitination. Oxid Med Cell Longev (2022) 2022:5411462. doi: 10.1155/2022/5411462
225. Wang S, Li G, Zhang X, Wang Y, Qiang Y, Wang B, et al. Structural characterization and antioxidant activity of polygonatum sibiricum polysaccharides. Carbohydr Polym (2022) 291:119524. doi: 10.1016/j.carbpol.2022.119524
226. Shen F, Song Z, Xie P, Li L, Wang B, Peng D, et al. Polygonatum sibiricum polysaccharide prevents depression-like behaviors by reducing oxidative stress, inflammation, and cellular and synaptic damage. J Ethnopharmacol (2021) 275:114164. doi: 10.1016/j.jep.2021.114164
227. Zheng S. Protective effect of polygonatum sibiricum polysaccharide on d-galactose-induced aging rats model. Sci Rep (2020) 10(1):2246. doi: 10.1038/s41598-020-59055-7
228. Zhang Z, Yang B, Huang J, Li W, Yi P, Yi M, et al. Identification of the protective effect of polygonatum sibiricum polysaccharide on d-galactose-induced brain ageing in mice by the systematic characterization of a circular RNA-associated ceRNA network. Pharm Biol (2021) 59(1):347–66. doi: 10.1080/13880209.2021.1893347
229. Xiao L, Qi L, Zhang G, Liu H, Gu Y, Zhang L, et al. Polygonatum sibiricum polysaccharides attenuate lipopoly-saccharide-induced septic liver injury by suppression of pyroptosis via NLRP3/GSDMD signals. Molecules (2022) 27(18):5999. doi: 10.3390/molecules27185999
230. Zhang J, Liu N, Sun C, Sun D, Wang Y. Polysaccharides from polygonatum sibiricum delar. ex redoute induce an immune response in the RAW264.7 cell line via an NF-κB/MAPK pathway. RSC Adv (2019) 9(31):17988–94. doi: 10.1039/c9ra03023a
231. Yelithao K, Surayot U, Park W, Lee S, Lee DH, You S. Effect of sulfation and partial hydrolysis of polysaccharides from polygonatum sibiricum on immune-enhancement. Int J Biol Macromol (2019) 122:10–8. doi: 10.1016/j.ijbiomac.2018.10.119
232. Liu D, Tang W, Han C, Nie S. Advances in polygonatum sibiricum polysaccharides: extraction, purification, structure, biosynthesis, and bioactivity. Front Nutr (2022) 9:1074671. doi: 10.3389/fnut.2022.1074671
233. Liu H, Li HZ, Zhang H, Lv SQ, Su XH, Pan HM. Effects of polygona-polysaccharose on ferroptosis in diabetic nephropathy rats. Chin J Inf Traditional Chin Med (2022). doi: 10.19879/j.cnki.1005-5304.202209207
234. Liu Y, Tian S, Yi B, Feng Z, Chu T, Liu J, et al. Platycodin d sensitizes KRAS-mutant colorectal cancer cells to cetuximab by inhibiting the PI3K/Akt signaling pathway. Front Oncol (2022) 12:1046143. doi: 10.3389/fonc.2022.1046143
235. Hsu WC, Ramesh S, Shibu MA, Chen MC, Wang TF, Day CH, et al. Platycodin d reverses histone deacetylase inhibitor resistance in hepatocellular carcinoma cells by repressing ERK1/2-mediated cofilin-1 phosphorylation. Phytomedicine (2021) 82:153442. doi: 10.1016/j.phymed.2020.153442
236. Shen Q, Zhong YT, Liu XX, Hu JN, Qi SM, Li K, et al. Platycodin d ameliorates hyperglycaemia and liver metabolic disturbance in HFD/STZ-induced type 2 diabetic mice. Food Funct (2023) 14(1):74–86. doi: 10.1039/d2fo03308a
237. Choi YJ, Lee SJ, Kim HI, Lee HJ, Kang SJ, Kim TY, et al. Platycodin d enhances LDLR expression and LDL uptake via down-regulation of IDOL mRNA in hepatic cells. Sci Rep (2020) 10(1):19834. doi: 10.1038/s41598-020-76224-w
238. Guo R, Meng Q, Wang B and Li F. Anti-inflammatory effects of platycodin d on dextran sulfate sodium (DSS) induced colitis and e. coli lipopolysaccharide (LPS) induced inflammation. Int Immunopharmacol (2021) 94:107474. doi: 10.1016/j.intimp.2021.107474
239. Lee EG, Kim KH, Hur J, Kang JY, Lee HY and Lee SY. Platycodin d attenuates airway inflammation via suppression Th2 transcription factor in a murine model of acute asthma. J Asthma (2022) 59(7):1279–89. doi: 10.1080/02770903.2021.1941084
240. Kim HL, Park J, Jung Y, Ahn KS, Um JY. Platycodin d, a novel activator of AMP-activated protein kinase, attenuates obesity in db/db mice via regulation of adipogenesis and thermogenesis. Phytomedicine (2019) 52:254–63. doi: 10.1016/j.phymed.2018.09.227
241. Liu YM, Cong S, Cheng Z, Hu YX, Lei Y, Zhu LL, et al. Platycodin d alleviates liver fibrosis and activation of hepatic stellate cells by regulating JNK/c-JUN signal pathway. Eur J Pharmacol (2020) 876:172946. doi: 10.1016/j.ejphar.2020.172946
242. Huang J, Chen G, Wang J, Liu S, Su J. Platycodin d regulates high glucose-induced ferroptosis of HK-2 cells through glutathione peroxidase 4 (GPX4). Bioengineered (2022) 13(3):6627–37. doi: 10.1080/21655979.2022.2045834
243. Siddiq AA, Martin A. Crocetin exerts hypocholesterolemic effect by inducing LDLR and inhibiting PCSK9 and sortilin in HepG2 cells. Nutr Res (2022) 98:41–9. doi: 10.1016/j.nutres.2021.08.005
244. Zheng Y, Zhu N, Wang J, Zhao N, Yuan C. Crocetin suppresses gestational diabetes in streptozotocin-induced diabetes mellitus rats via suppression of inflammatory reaction. J Food Biochem (2021) 459:e13857. doi: 10.1111/jfbc.13857
245. Abedimanesh S, Bathaie SZ, Ostadrahimi A, Asghari Jafarabadi M, Taban Sadeghi M. The effect of crocetin supplementation on markers of atherogenic risk in patients with coronary artery disease: a pilot, randomized, double-blind, placebo-controlled clinical trial. Food Funct (2019) 10(11):7461–75. doi: 10.1039/c9fo01166h
246. Lin S, Li Q, Jiang S, Xu Z, Jiang Y, Liu L, et al. Crocetin ameliorates chronic restraint stress-induced depression-like behaviors in mice by regulating MEK/ERK pathways and gut microbiota. J Ethnopharmacol (2021) 268:113608. doi: 10.1016/j.jep.2020.113608
247. Wang Y, Yu W, Shi C, Hu P. Crocetin attenuates sepsis-induced cardiac dysfunction via regulation of inflammatory response and mitochondrial function. Front Physiol (2020) 11:514. doi: 10.3389/fphys.2020.00514
248. Yang X. Design and optimization of crocetin loaded PLGA nanoparticles against diabetic nephropathy via suppression of inflammatory biomarkers: a formulation approach to preclinical study. Drug Deliv (2019) 26(1):849–59. doi: 10.1080/10717544.2019.1642417
249. Liu P, Xue Y, Zheng B, Liang Y, Zhang J, Shi J, et al. Crocetin attenuates the oxidative stress, inflammation and apoptosisin arsenic trioxide-induced nephrotoxic rats: implication of PI3K/AKT pathway. Int Immunopharmacol (2020) 88:106959. doi: 10.1016/j.intimp.2020.106959
Keywords: diabetic nephropathy, ferroptosis, Chinese herbal medicine, renal protection, mechanism
Citation: Wei M, Liu X, Tan Z, Tian X, Li M and Wei J (2023) Ferroptosis: a new strategy for Chinese herbal medicine treatment of diabetic nephropathy. Front. Endocrinol. 14:1188003. doi: 10.3389/fendo.2023.1188003
Received: 16 March 2023; Accepted: 23 May 2023;
Published: 09 June 2023.
Edited by:
Lixin Guo, Peking University, ChinaReviewed by:
Akira Sugawara, Tohoku University, JapanFeng Zhang, Nanjing University of Chinese Medicine, China
Copyright © 2023 Wei, Liu, Tan, Tian, Li and Wei. This is an open-access article distributed under the terms of the Creative Commons Attribution License (CC BY). The use, distribution or reproduction in other forums is permitted, provided the original author(s) and the copyright owner(s) are credited and that the original publication in this journal is cited, in accordance with accepted academic practice. No use, distribution or reproduction is permitted which does not comply with these terms.
*Correspondence: Junping Wei, d2VpanVucGluZ0AxMjYuY29t