- 1School of Life and Health Technology, Dongguan University of Technology, Dongguan, China
- 2Department of Food and Nutrition, University of Helsinki, Helsinki, Finland
- 3Human Microbiome Research Program, Faculty of Medicine, University of Helsinki, Helsinki, Finland
Globally, excess weight during childhood and adolescence has become a public health crisis with limited treatment options. Emerging evidence suggesting the involvement of gut microbial dysbiosis in obesity instills hope that targeting the gut microbiota could help prevent or treat obesity. In pre-clinical models and adults, prebiotic consumption has been shown to reduce adiposity partially via restoring symbiosis. However, there is a dearth of clinical research into its potential metabolic benefits in the pediatric population. Here, we provide a succinct overview of the common characteristics of the gut microbiota in childhood obesity and mechanisms of action of prebiotics conferring metabolic benefits. We then summarize available clinical trials in children with overweight or obesity investigating the effects of prebiotics on weight management. This review highlights several controversial aspects in the microbiota-dependent mechanisms by which prebiotics are thought to affect host metabolism that warrant future investigation in order to design efficacious interventions for pediatric obesity.
Introduction
Excess weight during childhood and adolescence remains one of the most challenging issues in global health. In Europe, overweight and obesity currently affect more than one quarter of children (29% of boys and 27% of girls) (1). The global rate of overweight increased from 10.3% in 2000 to 18.4% in 2018, especially in the 5-19 age group (2). This surge in childhood overweight and obesity is more severe in low-income and middle-income countries over the past four decades (3), and has been exacerbated by the COVID-19 pandemic (4). Approximately three-quarters of children with overweight or obesity carry the weight status into adulthood, and therefore childhood obesity increases both immediate and imminent co-morbidity risks for several non-communicable diseases, such as cardiovascular diseases, non-alcoholic fatty liver disease (NAFLD), diabetes, cancer, and some immune-related disorders (5).
The etiology of obesity involves complex interactions between a compendium of environmental and genetic factors that result in an energy imbalance, leading to the deposition of excess adipose tissue. While genome wide association studies have identified several gene loci associated with obesity, genetic susceptibility accounts for only a small percentage of the variance in body weight (6, 7). Importantly, the rapid rise in obesity rates in recent years cannot be attributed to any genetic changes occurring on such short evolutionary time frame, suggesting that environmental factors such as diet and levels of physical activity play a larger role (8). As a metabolic gateway between the outer environment and the host (9), the gut microbiota (i.e., the trillions of microorganisms residing within the human gastrointestinal (GI) tract) has come under the spotlight for its potential contribution to the childhood obesity epidemic (10).
Current treatment strategies for childhood obesity center around lifestyle counseling with multicomponent approaches based on dietary changes, behavior therapy, physical activity, and to a lesser extent, pharmacotherapies, with limited success (4, 11, 12). Some evidence suggests that interventions that only focus on diet are less effective in older children (12). In general, lifestyle changes are difficult to implement over the long term. Although bariatric surgery is the most effective and durable treatment for inducing weight loss in adolescents with severe obesity, it is rarely performed in the pediatric/adolescent population (ca. less than 1% of all weight loss procedures) due to the perceived invasiveness, complications and irreversibility of the procedures as well as high costs (4). Consequently, research efforts have focused on identifying alternative strategies for long-term prevention and treatment of childhood obesity. Prebiotics, a term first coined in 1995 and currently rereferred to as “substrates that can be selectively utilized by host microorganisms conferring health benefits” (13), have been increasingly touted as one of such alternative approaches.
The definition and mechanisms of action of prebiotics (reviewed elsewhere in detail (13–15)) partly overlap with that of dietary fiber. The main difference is that prebiotics selectively stimulate certain commensals, while not all fibers show prebiotic properties. Current prebiotics are predominantly carbohydrate-based, with fructans (e.g., inulin and fructooligosaccharides (FOS)) and galactooligosaccharides (GOS) produced from lactose being the most common prebiotics studied to date (15). Although evidence from animal models suggests beneficial effects of prebiotics on metabolic parameters related to obesity (16), few clinical studies have been conducted in the pediatric population. In this article, we summarize the available clinical trials on childhood obesity using prebiotics, focusing on the 5-19 age group. We also highlight some controversial aspects in the microbiota-dependent mechanisms by which prebiotics are thought to affect host metabolism and discuss how prebiotics can help reduce the burden of childhood obesity.
Features of the gut microbiota in childhood obesity
Over the past two decades, differences in gut microbiota composition and its metabolic activity between individuals with and without obesity have been reported (17, 18). Through investigation into these transmissible and rapidly modifiable features of the gut microbiota, multiple mechanisms for the link between obesity and the gut microbiota have been proposed, including increased energy extraction from the diet, regulation of satiety and other gut hormones e.g., through activation of enteroendocrine signaling pathways by short-chain fatty acids (SCFAs; acetate, propionate and butyrate) and other microbial metabolites, modulation of glucose and energy homeostasis through bile acid and other signaling, and increased local and systemic inflammation caused by a variety of microbially derived molecules especially lipopolysaccharides (LPS) (19, 20). SCFAs produced through colonic fermentation of non-digestible carbohydrates have represented a focal point in the obesity-microbiome research field owing to their seemingly contrasting properties i.e., acting as an energy source as well as a diverse signaling moiety (21). The latter modulates intestinal barrier function, glucose homeostasis, lipid metabolism, appetite, and immune function (21, 22). Children and adults with obesity appear to have higher fecal concentrations of SCFAs compared to lean controls (23), suggestive of increased production. It should be noted that gut epithelia have the capacity to absorb up to 95% of SCFA before excretion (21). Therefore, the SCFA concentrations measured from feces are prone to variation, since SCFA absorption efficiency depends on the type of substrate and site of fermentation (more time to absorb for readily fermented substrates in the proximal than distal colon) and also likely differs between individuals (24, 25). Moreover, the contribution of colonic fermentation to daily energy supplies, initially calculated to be up to 10% (26), is likely an overestimation. By depleting the gut microbiota using calorie restriction and antibiotic treatment in healthy volunteers, Basolo et al. recently estimated that the gut microbiota harvests an additional ~2.5% of absolute ingested calories that would translate to a mere 1.2-kg weight gain in a 100-kg subject over one year (27). In this regard, it remains unclear to what extent the energy extraction capacity of the microbiota differs between individuals with obesity and those who are lean.
A recent systematic review of case-control studies comparing the gut microbiota of individuals with and without obesity concluded that LPS-producing Proteobacteria was the most consistently reported obesity-associated phylum, and Faecalibacterium, Akkermansia, and Alistipes as lean-associated genera in both adults and children (17). However, in childhood obesity discrepancies were observed for several bacterial taxa, notably the genus Bifidobacterium (17), likely reflecting the transition of Bifidobacterium species and dominance in children toward adulthood (28). Hence, the higher heterogenicity of the gut microbiota features in childhood obesity compared to that in adults may result from a synergic effect of age and obesity on the gut microbiota (17, 29). To date, no studies have employed metagenomic sequencing that could unravel specific bacterial species, strains and functions as well as potential viruses or fungi specifically involved in the development of pediatric obesity in a sufficiently large cohort (10, 17).
The ratio between the two predominant bacterial phyla, Firmicutes and Bacteroidetes, represents some of the most striking cross-study discordance in microbiota features of obesity (17, 30, 31). The discrepant findings may be due to differences in research methods and cohort-specific characteristics (e.g., ethnicity and diet) (32). By re-analyzing publicly available sequencing datasets derived from case-control studies using a uniform bioinformatic pipeline, the gut microbiota of children with obesity appeared to have an increased ratio of Firmicutes to Bacteroidetes primarily driven by the reduced relative abundance of Bacteroidaceae (Figure 1), suggesting common causal agents in the microbiota and/or similar physiological states in the intestinal milieu across continents and cultures. Of note, various Bacteroides spp. (dominant taxa in the family Bacteroidaceae) have been shown to inhibit or alleviate metabolic dysregulation by activating folate-mediated signaling pathways (35), reducing production of pro-inflammatory LPS (36) and modulating circulating amino acids (37, 38). On the other hand, Bacteroides spp. with the bile salt hydrolase (BSH) activity selectively hydrolyze conjugated bile acid substrates to alter bile acid compositions, resulting in worsened metabolic phenotypes in animal models (39).
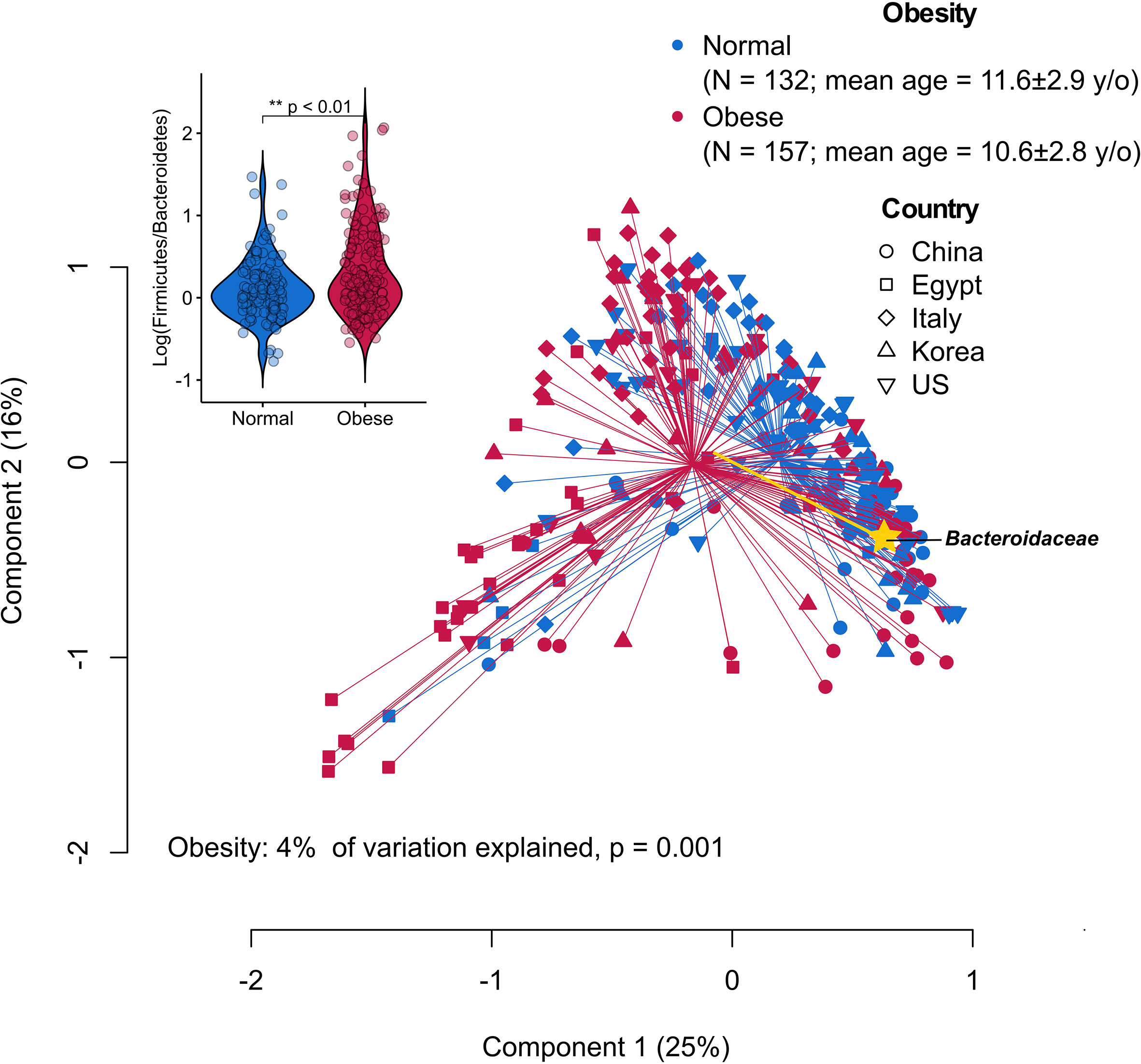
Figure 1 Principal coordinate analysis (PCoA) plot of family-level microbiota variation based on the Bray-Curtis dissimilarity matrix from five publicly available datasets of childhood obesity generated by comparable sequencing technology (PRJCA002952, PRJNA637782, PRJNA647465, PRJNA774503, PRJNA317290). Raw read sequence extraction, filtering and processing were performed as described previously (33). The processed sequences were analyzed at the family level that can capture most of the information while avoiding potential between-study technical variation (34). Obesity status explained 4% of the microbiota variation (P = 0.001, permutational multivariate analysis of variance). Yellow biplot indicates Bacteroidaceae that drives sample clustering on the plot. Box plot at the upper-left corner shows the logarithmic values of the Firmicutes-to-Bacteroidetes ratio from all samples according to obesity status.
Taken together, the altered gut microbial community in obesity and the evidence suggesting its connection to early-life perturbations (10) instill hope that targeting these disruptions could help prevent or treat childhood obesity. Nevertheless, observational studies, especially those employing shotgun metagenomic sequencing, on the associations between the gut microbiota and childhood obesity remain instrumental in understanding what caused the cross-study inconsistencies and identifying relevant targets and timing for rationally designed interventions. For example, few longitudinal studies in the pediatric population have examined the time of obesity onset and development of an obese-like gut microbiota, which would inform the right timing for potential interventions.
Potential mechanisms of action of prebiotics in metabolic improvements
The modulation of the enteroendocrine function by prebiotics and their fermentation products is often cited as the main mechanism behind the systemic effects on lipid and glucose homeostasis, as well as on satiety control (Figure 2) (16). For instance, circulating levels of glucagon like peptide-1 (GLP-1) and anorexigenic peptide YY (PYY) have been shown to be elevated by SCFAs following prebiotic supplementation in both mice and humans (16). Moreover, early murine studies suggest that the prebiotic-induced proliferation of commensal bacteria altered the mucosal architecture (40). The altered mucus composition and/or regular turnover of mucin glycoproteins may help maintain the integrity of the mucosal barrier and attenuate inflammation in the context of obesity (41, 42). It should be note that some prebiotics may directly interact with the host to promote barrier function and improve glucose metabolism, the identification of which from metabolic studies in humans is nevertheless extremely difficult (13). The prebiotic effect may be further enhanced by increasing the endogenous production of intestinal glucagon like peptide-2 (GLP-2), which is known to reinforce gut barrier function through upregulation of critical tight junction proteins in the epithelium (43). Since several above-mentioned changes coincided with an increase in fecal bifidobacteria, the health-promoting effects of prebiotics have been attributed mainly to this bacterial population group. However, the role of bifidobacteria in mediating prebiotic-induced metabolic improvements has been questioned in some studies (44). Importantly, what constitutes a healthy gut microbial community is often age and/or population-dependent (45); it is now recognized that prebiotic effects extend beyond bifidobacteria and would likely require a consortium of gut microbes involved in the trophic interactions (14). In addition to the microbiota-dependent mechanisms, prebiotics also contribute to increased dietary fiber intake and decreased energy density of the diet that has been associated with reduced odds of visceral obesity in overweight adolescents (46).
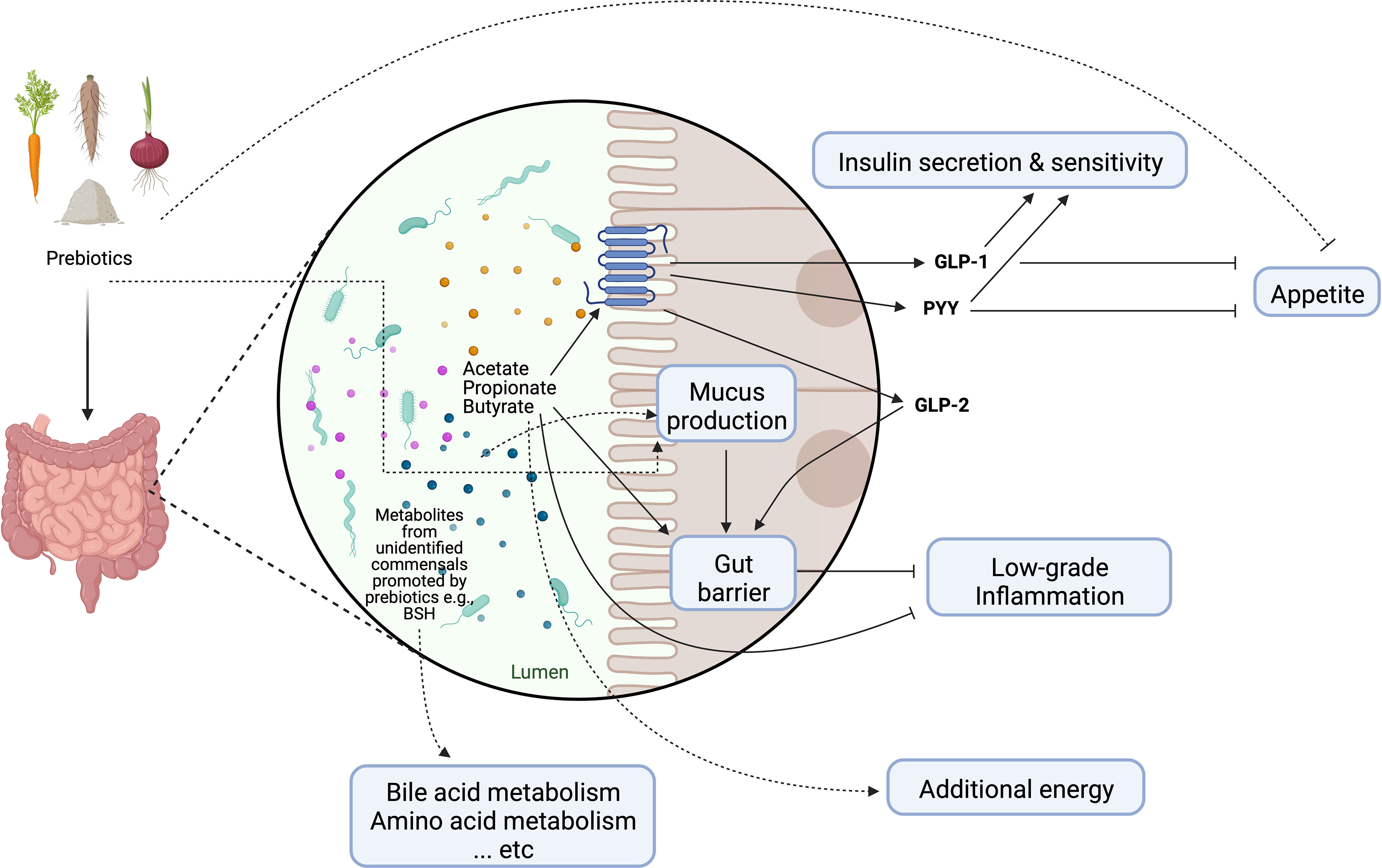
Figure 2 Schematic representation of main mechanisms of actions through which prebiotics may influence host metabolism. Dotted lines represent purported mechanisms under investigation. GLP-1, glucagon-like peptide-1; GLP-2, glucagon-like peptide-2; PYY, peptide YY; BSH, bile salt hydrolase. Created with Biorender.com.
Clinical evidence for prebiotics as a dietary intervention in childhood obesity
As summarized in Table 1, the few clinical studies in children with overweight or obesity to date showed no consistent effects of prebiotics on weight management or insulin sensitivity. Most of the studies empirically used inulin-type fructans (ITFs) as the choice of prebiotic supplementation likely based on their relatively well-studied metabolic benefits in adults (56, 57). Zalewski and Szajewska investigated the effect of glucomannan (a viscous dietary fiber derived from the plant Amorphophallus konjac with a bifidogenic effect (58)) and found decreased levels of total and low-density lipoprotein cholesterol with no body weight reduction after 12 weeks of supplementation, though the gut microbiota was not analyzed (53). Additionally, one ongoing trial is studying the effect of polylactose, a newly synthesized prebiotic derived from lactose, on the liver fat in children with obesity and NAFLD (see www.clinicaltrials.gov NCT04100109).
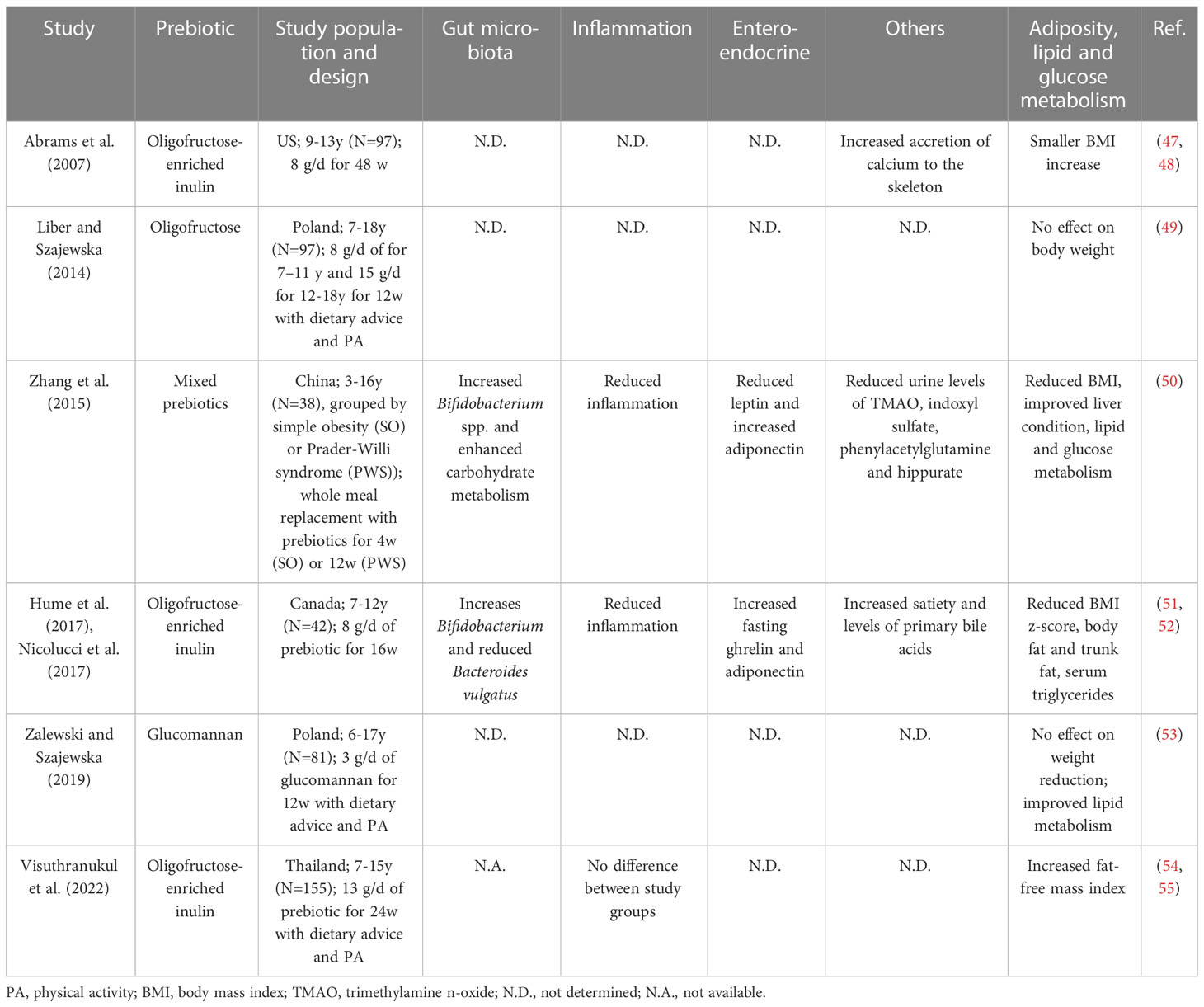
Table 1 Summary of clinical trials with prebiotics in children with overweight or simple and genetic obesity.
Currently, only two clinical trials on prebiotic supplementation in pediatric obesity, both reporting positive outcomes in reducing inflammatory markers as well as BMI, assessed gut microbiota composition (50, 51). The two studies similarly reported an increase in bifidobacteria in the gut microbiota after the intervention (50, 51), but the increase appeared not to correlate with changes in metabolic or inflammatory markers (51). Instead, the reduction in Bacteroides vulgatus, a bacterial group linked to both positive (36, 37, 59, 60) and negative (61, 62) metabolic health previously, was associated with a reduction in percent trunk fat over the 16-week intervention (51). Notably, there is no data on the long-terms effects of prebiotics beyond one year, which may have been required for some microbiota-dependent mechanisms to appreciably affect body weight (27). Moreover, puberty can act as a confounder in pediatric clinical trials both in terms of weight development and the gut microbiota (63). Thus, there exists a need for clinical trials assessing long-term changes in metabolic, enteroendocrine and inflammatory markers as well as in the gut microbiota with the use of rationally designed/selected prebiotics in the pediatric population.
Discussion and perspectives
Unlike rodent models of obesity with a salient and transferrable microbiota component in the pathophysiology (64), in humans controversies exist regarding whether the difference between the gut microbiota of individuals with and without obesity is the cause or effect of altered diet and energy metabolism and, if the former, how much it influences pathophysiology. Despite this incomplete understanding, clinical trials have tested empirically numerous microbiota-targeting therapies, including prebiotics, to prevent or treat obesity with mixed results. Below, we discuss some important aspects of prebiotics that warrant future investigation in order to design efficacious interventions for pediatric obesity.
Human studies in pediatric obesity to date have predominantly used ITFs as the sole prebiotic supplement, which cannot correct the reduced relative abundance of Bacteroides spp. in the gut microbiota, characteristic in and potentially mechanistically linked to pediatric obesity as described previously. Recent studies have shown some dietary fibers, such as seaweed polysaccharides and yeast mannan, to selectively promote specific Bacteroides species (65, 66). By rationally designing prebiotic fibers to increase the abundances of targeted Bacteroides, it was found that prebiotic supplementation significantly altered plasma proteomes linked to microbiota changes in adults with overweight and obesity in a 3-week pilot trial (67). On the other hand, the degree of polymerization (DP) of prebiotics has a significant impact on their fermentation location in the colon; low (e.g., FOS) and high (e.g., inulin) DP fructans tend to be fermented more in the proximal and distal colon, respectively (68). This difference may influence the efficacy of prebiotics since distal SCFA infusion in vivo has been shown to induce more pronounced effects on biomarkers than proximal (69).
Administration of prebiotic interventions within the right window of time is critical, as the microbiota of children continues to develop and has higher interindividual variability compared to that of adults. While the optimal timing remains to be determined, it is likely that early interventions would exert a more pronounced effect similar to what was observed in other diet-based interventions for pediatric obesity (12). Although not covered in the scope of this article, human milk oligosaccharides (HMOs) abundant in breastmilk may possess anti-obesogenic properties partly through their prebiotic potential and have been formulated in some commercially available infant formula (70). However, the data on such supplementation, especially regarding its long-term effects, is currently lacking. Specific types of HMOs have been positively associated with child height and weight z scores in a recent cohort study (71), suggesting its growth-promoting effects. Thus, at least theoretically, if HMO content is a poor match to the age-depended needs, it could potentially promote overly fast growth or accumulation of fat mass over fat-free mass (71). On the other hand, animal studies using prebiotics found that prenatal supplementation successfully decreased the offspring’s obesity risk (72), likely via the effect of maternal SCFAs that are constantly supplied to the fetus (73). This raises the possibility of intervening an intergenerational cycle of obesity through maternal interventions. Finally, an underexplored area in the use of prebiotic supplementation is the correction of antibiotic-associated dysbiosis, which has been linked to increased childhood BMI (74). In a rat model, increased fat mass, hyperinsulinemia and insulin resistance following direct pulsed administration of antibiotics in early life were prevented by prebiotic co-administration in a sex-specific manner (75).
Overall, taking into consideration the interindividual and inter-population variability in microbiota responses to prebiotics is important to maximize benefits while avoiding potential harms. The variable metabolic improvements in adults with obesity in response to inulin supplementation have been demonstrated to depend on the pre-intervention gut microbiota (76). Holmes et al. showed substantial interindividual variation in the prebiotic effects on SCFA production from the microbiota of adolescents with obesity, which could contribute to the inconsistent results from prior studies of prebiotics in pediatric obesity (24). Thus, therapeutic efforts involving prebiotics in children with obesity may benefit from a priori patient stratification based on the gut microbiota. In the global context, one challenge to translating potential clinical benefits of prebiotics is the “uncharacterized” gut microbiota in the populations of developing countries where the rise of pediatric obesity is most alarming. For instance, Egyptian and American adolescents differed considerably in microbiota composition, functions and microbial metabolites (77). This inter-population difference in the gut microbiota may represent a limiting factor for successful prebiotic interventions in the context of pediatric undernutrition. Specifically, rice bran supplementation significantly improved the weight for age z-scores in the infants from Mali, where rice is the dominant staple food, compared to that of Nicaraguan infants who had different gut microbiota and metabolome composition (78). On the other hand, gastrointestinal tolerance differs with the type and amount of prebiotics consumed and is affected by the highly individualized response (79). For example, most of the highly fermentable ITFs may be tolerated in the generally healthy adult population at daily intakes of up to 15 g, depending on their DP (80). In contrast, resistant starches and soluble corn fiber have been consumed at the dose of 50 g/d without symptoms (81). Early studies suggest that infants and young children tolerate ITFs up to 0.8g/kg body weight per day (82), which has been corroborated by the interventions discussed herein (Table 1). The gastrointestinal tolerance of other types of prebiotics in children is less documented and warrants formal evaluation in interventional trials (79). Last but not least, prolonged consumption of inulin fiber enriched foods unexpectedly led to potentially dysregulated bile acid and/or SCFA metabolism in recent murine studies (83, 84), contributing to type 2 inflammation and increasing the risk of hepatocellular carcinoma in a microbiota-dependent manner. Although the translatability of these animal models to humans remains unclear, the findings emphasize the need to rigorously report safety data in prebiotic trials conducted in various populations and ethnic groups with different gut microbiota composition. Many of the concerns may also be circumvented by rationally designing locally sourced dietary approaches to target the gut microbiota.
It has been shown that the variability of glycemic response to exercise in prediabetic adults depended on the gut microbiota via SCFA production and amino acid catabolism (85). Similarly, prebiotic supplementation and physical activity appeared to have a synergistic effect on BMI reduction in adults with obesity (86). While a recent study in children with obesity argued that the effects of inulin supplementation on body weight and adiposity reduction may be overshadowed by the intensive behavioral modification (55), it will be interesting to see whether prebiotic supplementation provides benefits to those who are the most refractory to exercise-induced weight loss.
Prebiotic fibers in appropriate concentrations improve the textural and sensory qualities of foods, primarily due to their moisture retention capacity and potential off-flavor masking properties (87). Inulin, for instance, has been formulated in sausages and cheese as a fat substitute (88, 89). In light of the recent reports linking certain food additives to metabolic dysregulation via dysbiosis (90, 91) as well as the growing clean-label trend, several types of exopolysaccharides naturally synthesized by lactic acid bacteria during fermentation have been proposed as substitutes for flavorants and emulsifiers used in bakery and dairy products (92). Many of these exopolysaccharides have shown potential prebiotic properties in pre-clinical and clinical studies (92). Taken together, the benefit of prebiotic-enriched foods in combatting childhood obesity is twofold. On one hand, prebiotic addition increases dietary fiber content and promotes growth of beneficial commensals. On the other hand, prebiotics also enhance physiochemical properties of the surrounding food matrix, allowing for reduction in fat and food additives as well as energy content without considerably compromising product taste and texture. These benefits can potentially bolster an overall improved sensory experience for the children, thereby promoting long-term adherence to a healthier diet. Importantly, it has been proposed that addition of 2.5-6.5 g prebiotics per serving in food may serve to double or triple one’s regular fiber intake and meet a threshold for beneficial effects with a single dietary modification (93).
Conclusions
While the available studies in the pediatric population generated mixed results potentially mired by interindividual variation, prebiotic supplementation has shown potential as an adjunctive therapy for improving several metabolic markers when rationally applied as well as reducing energy density of the diet. Their long-term effects and the mechanistic role of the gut microbiota in mediating the potential benefits need to be clarified in more clinical trials directly targeting childhood obesity.
Author contributions
YW and CJ conceptualized, reviewed the literature, visualized the data, and wrote the manuscript. AS critically revised the manuscript. All authors contributed to the article and approved the submitted version.
Funding
The open access was funded by Helsinki University Library.
Conflict of interest
The authors declare that the research was conducted in the absence of any commercial or financial relationships that could be construed as a potential conflict of interest.
Publisher’s note
All claims expressed in this article are solely those of the authors and do not necessarily represent those of their affiliated organizations, or those of the publisher, the editors and the reviewers. Any product that may be evaluated in this article, or claim that may be made by its manufacturer, is not guaranteed or endorsed by the publisher.
References
1. World Health Organization. WHO European regional obesity report. World Health Organization. Regional Office for Europe (2022).
2. Keeley B, Little C, Zuehlke E. The state of the world's children 2019: children, food and nutrition–growing well in a changing world. New York, NY: UNICEF (2019).
3. (NCD-RisC) NRFC. Worldwide trends in body-mass index, underweight, overweight, and obesity from 1975 to 2016: a pooled analysis of 2416 population-based measurement studies in 128·9 million children, adolescents, and adults. Lancet (2017) 390(10113):2627–42. doi: 10.1016/s0140-6736(17)32129-3
4. Jebeile H, Kelly AS, O'Malley G, Baur LA. Obesity in children and adolescents: epidemiology, causes, assessment, and management. Lancet Diabetes Endocrinol (2022) 10(5):351–65. doi: 10.1016/s2213-8587(22)00047-x
5. Di Cesare M, Sorić M, Bovet P, Miranda JJ, Bhutta Z, Stevens GA, et al. The epidemiological burden of obesity in childhood: a worldwide epidemic requiring urgent action. BMC Med (2019) 17(1):212. doi: 10.1186/s12916-019-1449-8
6. Meyre D, Delplanque J, Chèvre JC, Lecoeur C, Lobbens S, Gallina S, et al. Genome-wide association study for early-onset and morbid adult obesity identifies three new risk loci in European populations. Nat Genet (2009) 41(2):157–9. doi: 10.1038/ng.301
7. Walters RG, Jacquemont S, Valsesia A, de Smith AJ, Martinet D, Andersson J, et al. A new highly penetrant form of obesity due to deletions on chromosome 16p11.2. Nature (2010) 463(7281):671–5. doi: 10.1038/nature08727
8. Sonnenburg JL, Sonnenburg ED. Vulnerability of the industrialized microbiota. Science (2019) 366(6464):eaaw9255. doi: 10.1126/science.aaw9255
9. Cani PD, Van Hul M, Lefort C, Depommier C, Rastelli M, Everard A. Microbial regulation of organismal energy homeostasis. Nat Metab (2019) 1(1):34–46. doi: 10.1038/s42255-018-0017-4
10. Jian C, Carpén N, Helve O, de Vos WM, Korpela K, Salonen A. Early-life gut microbiota and its connection to metabolic health in children: perspective on ecological drivers and need for quantitative approach. EBioMedicine (2021) 69:103475. doi: 10.1016/j.ebiom.2021.103475
11. Pereira AR, Oliveira A. Dietary interventions to prevent childhood obesity: a literature review. Nutrients (2021) 13(10):3447. doi: 10.3390/nu13103447
12. Brown T, Moore TH, Hooper L, Gao Y, Zayegh A, Ijaz S, et al. Interventions for preventing obesity in children. Cochrane Database Syst Rev (2019) 7(7):Cd001871. doi: 10.1002/14651858.CD001871.pub4
13. Sanders ME, Merenstein DJ, Reid G, Gibson GR, Rastall RA. Probiotics and prebiotics in intestinal health and disease: from biology to the clinic. Nat Rev Gastroenterol Hepatol (2019) 16(10):605–16. doi: 10.1038/s41575-019-0173-3
14. Gibson GR, Hutkins R, Sanders ME, Prescott SL, Reimer RA, Salminen SJ, et al. Expert consensus document: the international scientific association for probiotics and prebiotics (ISAPP) consensus statement on the definition and scope of prebiotics. Nat Rev Gastroenterol Hepatol (2017) 14(8):491–502. doi: 10.1038/nrgastro.2017.75
15. Davani-Davari D, Negahdaripour M, Karimzadeh I, Seifan M, Mohkam M, Masoumi SJ, et al. Prebiotics: definition, types, sources, mechanisms, and clinical applications. Foods (2019) 8(3):92. doi: 10.3390/foods8030092
16. Rodriguez J, Delzenne NM. Modulation of the gut microbiota-adipose tissue-muscle interactions by prebiotics. J Endocrinol (2021) 249(1):R1–r23. doi: 10.1530/joe-20-0499
17. Xu Z, Jiang W, Huang W, Lin Y, Chan FKL, Ng SC. Gut microbiota in patients with obesity and metabolic disorders - a systematic review. Genes Nutr (2022) 17(1):2. doi: 10.1186/s12263-021-00703-6
18. Turnbaugh PJ, Ley RE, Mahowald MA, Magrini V, Mardis ER, Gordon JI. An obesity-associated gut microbiome with increased capacity for energy harvest. Nature (2006) 444(7122):1027–31. doi: 10.1038/nature05414
19. de Vos WM, Tilg H, Van Hul M, Cani PD. Gut microbiome and health: mechanistic insights. Gut (2022) 71(5):1020–32. doi: 10.1136/gutjnl-2021-326789
20. Jardon KM, Canfora EE, Goossens GH, Blaak EE. Dietary macronutrients and the gut microbiome: a precision nutrition approach to improve cardiometabolic health. Gut (2022) 71(6):1214–26. doi: 10.1136/gutjnl-2020-323715
21. den Besten G, van Eunen K, Groen AK, Venema K, Reijngoud DJ, Bakker BM. The role of short-chain fatty acids in the interplay between diet, gut microbiota, and host energy metabolism. J Lipid Res (2013) 54(9):2325–40. doi: 10.1194/jlr.R036012
22. Canfora EE, Meex RCR, Venema K, Blaak EE. Gut microbial metabolites in obesity, NAFLD and T2DM. Nat Rev Endocrinol (2019) 15(5):261–73. doi: 10.1038/s41574-019-0156-z
23. Kim KN, Yao Y, Ju SY. Short chain fatty acids and fecal microbiota abundance in humans with obesity: a systematic review and meta-analysis. Nutrients (2019) 11(10):2512. doi: 10.3390/nu11102512
24. Holmes ZC, Silverman JD, Dressman HK, Wei Z, Dallow EP, Armstrong SC, et al. Short-chain fatty acid production by gut microbiota from children with obesity differs according to prebiotic choice and bacterial community composition. mBio (2020) 11(4):e00914-20. doi: 10.1128/mBio.00914-20
25. Müller M, Hernández MAG, Goossens GH, Reijnders D, Holst JJ, Jocken JWE, et al. Circulating but not faecal short-chain fatty acids are related to insulin sensitivity, lipolysis and GLP-1 concentrations in humans. Sci Rep (2019) 9(1):12515. doi: 10.1038/s41598-019-48775-0
26. McNeil NI. The contribution of the large intestine to energy supplies in man. Am J Clin Nutr (1984) 39(2):338–42. doi: 10.1093/ajcn/39.2.338
27. Basolo A, Hohenadel M, Ang QY, Piaggi P, Heinitz S, Walter M, et al. Effects of underfeeding and oral vancomycin on gut microbiome and nutrient absorption in humans. Nat Med (2020) 26(4):589–98. doi: 10.1038/s41591-020-0801-z
28. Derrien M, Turroni F, Ventura M, van Sinderen D. Insights into endogenous bifidobacterium species in the human gut microbiota during adulthood. Trends Microbiol (2022) 30(10):940–7. doi: 10.1016/j.tim.2022.04.004
29. Del Chierico F, Abbatini F, Russo A, Quagliariello A, Reddel S, Capoccia D, et al. Gut microbiota markers in obese adolescent and adult patients: age-dependent differential patterns. Front Microbiol (2018) 9:1210. doi: 10.3389/fmicb.2018.01210
30. Magne F, Gotteland M, Gauthier L, Zazueta A, Pesoa S, Navarrete P, et al. The Firmicutes/Bacteroidetes ratio: a relevant marker of gut dysbiosis in obese patients? Nutrients (2020) 12(5):1474. doi: 10.3390/nu12051474
31. Houtman TA, Eckermann HA, Smidt H, de Weerth C. Gut microbiota and BMI throughout childhood: the role of firmicutes, bacteroidetes, and short-chain fatty acid producers. Sci Rep (2022) 12(1):3140. doi: 10.1038/s41598-022-07176-6
32. Stanislawski MA, Dabelea D, Lange LA, Wagner BD, Lozupone CA. Gut microbiota phenotypes of obesity. NPJ Biofilms Microbiomes (2019) 5(1):18. doi: 10.1038/s41522-019-0091-8
33. Dwiyanto J, Ayub Q, Lee SM, Foo SC, Chong CW, Rahman S. Geographical separation and ethnic origin influence the human gut microbial composition: a meta-analysis from a Malaysian perspective. Microb Genom (2021) 7(8):000619. doi: 10.1099/mgen.0.000619
34. Smits SA, Leach J, Sonnenburg ED, Gonzalez CG, Lichtman JS, Reid G, et al. Seasonal cycling in the gut microbiome of the hadza hunter-gatherers of Tanzania. Science (2017) 357(6353):802–6. doi: 10.1126/science.aan4834
35. Qiao S, Bao L, Wang K, Sun S, Liao M, Liu C, et al. Activation of a specific gut bacteroides-Folate-Liver axis benefits for the alleviation of nonalcoholic hepatic steatosis. Cell Rep (2020) 32(6):108005. doi: 10.1016/j.celrep.2020.108005
36. Yoshida N, Emoto T, Yamashita T, Watanabe H, Hayashi T, Tabata T, et al. Bacteroides vulgatus and bacteroides dorei reduce gut microbial lipopolysaccharide production and inhibit atherosclerosis. Circulation (2018) 138(22):2486–98. doi: 10.1161/circulationaha.118.033714
37. Yoshida N, Yamashita T, Osone T, Hosooka T, Shinohara M, Kitahama S, et al. Bacteroides spp. promotes branched-chain amino acid catabolism in brown fat and inhibits obesity. iScience (2021) 24(11):103342. doi: 10.1016/j.isci.2021.103342
38. Liu R, Hong J, Xu X, Feng Q, Zhang D, Gu Y, et al. Gut microbiome and serum metabolome alterations in obesity and after weight-loss intervention. Nat Med (2017) 23(7):859–68. doi: 10.1038/nm.4358
39. Yao L, Seaton SC, Ndousse-Fetter S, Adhikari AA, DiBenedetto N, Mina AI, et al. A selective gut bacterial bile salt hydrolase alters host metabolism. eLife (2018) 7:e37182. doi: 10.7554/eLife.37182
40. Kleessen B, Hartmann L, Blaut M. Fructans in the diet cause alterations of intestinal mucosal architecture, released mucins and mucosa-associated bifidobacteria in gnotobiotic rats. Br J Nutr (2003) 89(5):597–606. doi: 10.1079/bjn2002827
41. Paone P, Cani PD. Mucus barrier, mucins and gut microbiota: the expected slimy partners? Gut (2020) 69(12):2232–43. doi: 10.1136/gutjnl-2020-322260
42. Paone P, Suriano F, Jian C, Korpela K, Delzenne NM, Van Hul M, et al. Prebiotic oligofructose protects against high-fat diet-induced obesity by changing the gut microbiota, intestinal mucus production, glycosylation and secretion. Gut Microbes (2022) 14(1):2152307. doi: 10.1080/19490976.2022.2152307
43. Cani PD, Possemiers S, Van de Wiele T, Guiot Y, Everard A, Rottier O, et al. Changes in gut microbiota control inflammation in obese mice through a mechanism involving GLP-2-driven improvement of gut permeability. Gut (2009) 58(8):1091–103. doi: 10.1136/gut.2008.165886
44. Woting A, Pfeiffer N, Hanske L, Loh G, Klaus S, Blaut M. Alleviation of high fat diet-induced obesity by oligofructose in gnotobiotic mice is independent of presence of bifidobacterium longum. Mol Nutr Food Res (2015) 59(11):2267–78. doi: 10.1002/mnfr.201500249
45. Wilmanski T, Rappaport N, Diener C, Gibbons SM, Price ND. From taxonomy to metabolic output: what factors define gut microbiome health? Gut Microbes (2021) 13(1):1–20. doi: 10.1080/19490976.2021.1907270
46. Mollard RC, Sénéchal M, MacIntosh AC, Hay J, Wicklow BA, Wittmeier KD, et al. Dietary determinants of hepatic steatosis and visceral adiposity in overweight and obese youth at risk of type 2 diabetes. Am J Clin Nutr (2014) 99(4):804–12. doi: 10.3945/ajcn.113.079277
47. Abrams SA, Griffin IJ, Hawthorne KM, Ellis KJ. Effect of prebiotic supplementation and calcium intake on body mass index. J Pediatr (2007) 151(3):293–8. doi: 10.1016/j.jpeds.2007.03.043
48. Abrams SA, Griffin IJ, Hawthorne KM. Young adolescents who respond to an inulin-type fructan substantially increase total absorbed calcium and daily calcium accretion to the skeleton. J Nutr (2007) 137(11 Suppl):2524s–6s. doi: 10.1093/jn/137.11.2524S
49. Liber A, Szajewska H. Effect of oligofructose supplementation on body weight in overweight and obese children: a randomised, double-blind, placebo-controlled trial. Br J Nutr (2014) 112(12):2068–74. doi: 10.1017/s0007114514003110
50. Zhang C, Yin A, Li H, Wang R, Wu G, Shen J, et al. Dietary modulation of gut microbiota contributes to alleviation of both genetic and simple obesity in children. EBioMedicine (2015) 2(8):968–84. doi: 10.1016/j.ebiom.2015.07.007
51. Nicolucci AC, Hume MP, Martinez I, Mayengbam S, Walter J, Reimer RA. Prebiotics reduce body fat and alter intestinal microbiota in children who are overweight or with obesity. Gastroenterology (2017) 153(3):711–22. doi: 10.1053/j.gastro.2017.05.055
52. Hume MP, Nicolucci AC, Reimer RA. Prebiotic supplementation improves appetite control in children with overweight and obesity: a randomized controlled trial. Am J Clin Nutr (2017) 105(4):790–9. doi: 10.3945/ajcn.116.140947
53. Zalewski BM, Szajewska H. No effect of glucomannan on body weight reduction in children and adolescents with overweight and obesity: a randomized controlled trial. J Pediatr (2019) 211:85–91.e1. doi: 10.1016/j.jpeds.2019.03.044
54. Visuthranukul C, Kwanbunbumpen T, Chongpison Y, Chamni S, Panichsillaphakit E, Uaariyapanichkul J, et al. The impact of dietary fiber as a prebiotic on inflammation in children with obesity. Foods (2022) 11(18):2856. doi: 10.3390/foods11182856
55. Visuthranukul C, Chamni S, Kwanbunbumpen T, Saengpanit P, Chongpison Y, Tepaamorndech S, et al. Effects of inulin supplementation on body composition and metabolic outcomes in children with obesity. Sci Rep (2022) 12(1):13014. doi: 10.1038/s41598-022-17220-0
56. Cerdó T, García-Santos JA M, Campoy C. The role of probiotics and prebiotics in the prevention and treatment of obesity. Nutrients (2019) 11(3):635. doi: 10.3390/nu11030635
57. Chambers ES, Byrne CS, Morrison DJ, Murphy KG, Preston T, Tedford C, et al. Dietary supplementation with inulin-propionate ester or inulin improves insulin sensitivity in adults with overweight and obesity with distinct effects on the gut microbiota, plasma metabolome and systemic inflammatory responses: a randomised cross-over trial. Gut (2019) 68(8):1430–8. doi: 10.1136/gutjnl-2019-318424
58. Chen HL, Fan YH, Chen ME, Chan Y. Unhydrolyzed and hydrolyzed konjac glucomannans modulated cecal and fecal microflora in balb/c mice. Nutrition (2005) 21(10):1059–64. doi: 10.1016/j.nut.2005.02.008
59. You HJ, Si J, Kim J, Yoon S, Cha KH, Yoon HS, et al. Bacteroides vulgatus SNUG 40005 restores akkermansia depletion by metabolite modulation. Gastroenterology (2022) 164(1):103–16. doi: 10.1053/j.gastro.2022.09.040
60. Xu M, Lan R, Qiao L, Lin X, Hu D, Zhang S, et al. Bacteroides vulgatus ameliorates lipid metabolic disorders and modulates gut microbial composition in hyperlipidemic rats. Microbiol Spectr (2023) 11(1):e0251722. doi: 10.1128/spectrum.02517-22
61. Xu T, Wang X, Chen Y, Li H, Zhao L, Ding X, et al. Microbiome features differentiating unsupervised-Stratification-Based clusters of patients with abnormal glycometabolism. mBio (2023) 14(1):e0348722. doi: 10.1128/mbio.03487-22
62. Qi X, Yun C, Sun L, Xia J, Wu Q, Wang Y, et al. Gut microbiota-bile acid-interleukin-22 axis orchestrates polycystic ovary syndrome. Nat Med (2019) 25(8):1225–33. doi: 10.1038/s41591-019-0509-0
63. Korpela K, Kallio S, Salonen A, Hero M, Kukkonen AK, Miettinen PJ, et al. Gut microbiota develop towards an adult profile in a sex-specific manner during puberty. Sci Rep (2021) 11(1):23297. doi: 10.1038/s41598-021-02375-z
64. Bouter KE, van Raalte DH, Groen AK, Nieuwdorp M. Role of the gut microbiome in the pathogenesis of obesity and obesity-related metabolic dysfunction. Gastroenterology (2017) 152(7):1671–8. doi: 10.1053/j.gastro.2016.12.048
65. Wang S, Xiao Y, Tian F, Zhao J, Zhang H, Zhai Q, et al. Rational use of prebiotics for gut microbiota alterations: specific bacterial phylotypes and related mechanisms. J Funct Foods (2020) 66:103838. doi: 10.1016/j.jff.2020.103838
66. Oba S, Sunagawa T, Tanihiro R, Awashima K, Sugiyama H, Odani T, et al. Prebiotic effects of yeast mannan, which selectively promotes bacteroides thetaiotaomicron and bacteroides ovatus in a human colonic microbiota model. Sci Rep (2020) 10(1):17351. doi: 10.1038/s41598-020-74379-0
67. Delannoy-Bruno O, Desai C, Raman AS, Chen RY, Hibberd MC, Cheng J, et al. Evaluating microbiome-directed fibre snacks in gnotobiotic mice and humans. Nature (2021) 595(7865):91–5. doi: 10.1038/s41586-021-03671-4
68. Wegh CAM, Schoterman MHC, Vaughan EE, Belzer C, Benninga MA. The effect of fiber and prebiotics on children's gastrointestinal disorders and microbiome. Expert Rev Gastroenterol Hepatol (2017) 11(11):1031–45. doi: 10.1080/17474124.2017.1359539
69. Neis EP, van Eijk HM, Lenaerts K, Olde Damink SW, Blaak EE, Dejong CH, et al. Distal versus proximal intestinal short-chain fatty acid release in man. Gut (2019) 68(4):764–5. doi: 10.1136/gutjnl-2018-316161
70. Maessen SE, Derraik JGB, Binia A, Cutfield WS. Perspective: human milk oligosaccharides: fuel for childhood obesity prevention? Adv Nutr (Bethesda Md) (2020) 11(1):35–40. doi: 10.1093/advances/nmz093
71. Lagström H, Rautava S, Ollila H, Kaljonen A, Turta O, Mäkelä J, et al. Associations between human milk oligosaccharides and growth in infancy and early childhood. Am J Clin Nutr (2020) 111(4):769–78. doi: 10.1093/ajcn/nqaa010
72. Wiedmer EB, Herter-Aeberli I. The potential of prebiotic and probiotic supplementation during obese pregnancy to improve maternal and offspring's metabolic health and reduce obesity risk-a narrative review. Front Nutr (2022) 9:819882. doi: 10.3389/fnut.2022.819882
73. Kimura I, Miyamoto J, Ohue-Kitano R, Watanabe K, Yamada T, Onuki M, et al. Maternal gut microbiota in pregnancy influences offspring metabolic phenotype in mice. Science (2020) 367(6481):eaaw8429. doi: 10.1126/science.aaw8429
74. Korpela K, Zijlmans MA, Kuitunen M, Kukkonen K, Savilahti E, Salonen A, et al. Childhood BMI in relation to microbiota in infancy and lifetime antibiotic use. Microbiome (2017) 5(1):26. doi: 10.1186/s40168-017-0245-y
75. Klancic T, Laforest-Lapointe I, Wong J, Choo A, Nettleton JE, Chleilat F, et al. Concurrent prebiotic intake reverses insulin resistance induced by early-life pulsed antibiotic in rats. Biomedicines. (2021) 9(1):66. doi: 10.3390/biomedicines9010066
76. Rodriguez J, Hiel S, Neyrinck AM, Le Roy T, Pötgens SA, Leyrolle Q, et al. Discovery of the gut microbial signature driving the efficacy of prebiotic intervention in obese patients. Gut (2020) 69(11):1975–87. doi: 10.1136/gutjnl-2019-319726
77. Shankar V, Gouda M, Moncivaiz J, Gordon A, Reo NV, Hussein L, et al. Differences in gut metabolites and microbial composition and functions between Egyptian and U.S. children are consistent with their diets. mSystems (2017) 2(1):e00169-16. doi: 10.1128/mSystems.00169-16
78. Zambrana LE, McKeen S, Ibrahim H, Zarei I, Borresen EC, Doumbia L, et al. Rice bran supplementation modulates growth, microbiota and metabolome in weaning infants: a clinical trial in Nicaragua and Mali. Sci Rep (2019) 9(1):13919. doi: 10.1038/s41598-019-50344-4
79. Holscher HD, Chumpitazi BP, Dahl WJ, Fahey GC, Liska DJ, Slavin JL, et al. Perspective: assessing tolerance to nondigestible carbohydrate consumption. Adv Nutr (Bethesda Md) (2022) 13(6):2084–97. doi: 10.1093/advances/nmac091
80. Le Bourgot C, Rigaudier F, Juhel C, Herpin F, Meunier C. Gastrointestinal tolerance of short-chain fructo-oligosaccharides from sugar beet: an observational, connected, dose-ranging study in healthy volunteers. Nutrients (2022) 14(7):1461. doi: 10.3390/nu14071461
81. Deehan EC, Walter J. The fiber gap and the disappearing gut microbiome: implications for human nutrition. Trends Endocrinol metabolism: TEM (2016) 27(5):239–42. doi: 10.1016/j.tem.2016.03.001
82. Saavedra JM, Tschernia A. Human studies with probiotics and prebiotics: clinical implications. Br J Nutr (2002) 87(Suppl 2):S241–6. doi: 10.1079/bjnbjn/2002543
83. Arifuzzaman M, Won TH, Li TT, Yano H, Digumarthi S, Heras AF, et al. Inulin fibre promotes microbiota-derived bile acids and type 2 inflammation. Nature (2022) 611(7936):578–84. doi: 10.1038/s41586-022-05380-y
84. Singh V, Yeoh BS, Chassaing B, Xiao X, Saha P, Aguilera Olvera R, et al. Dysregulated microbial fermentation of soluble fiber induces cholestatic liver cancer. Cell (2018) 175(3):679–94.e22. doi: 10.1016/j.cell.2018.09.004
85. Liu Y, Wang Y, Ni Y, Cheung CKY, Lam KSL, Wang Y, et al. Gut microbiome fermentation determines the efficacy of exercise for diabetes prevention. Cell Metab (2020) 31(1):77–91.e5. doi: 10.1016/j.cmet.2019.11.001
86. Rodriguez J, Neyrinck AM, Van Kerckhoven M, Gianfrancesco MA, Renguet E, Bertrand L, et al. Physical activity enhances the improvement of body mass index and metabolism by inulin: a multicenter randomized placebo-controlled trial performed in obese individuals. BMC Med (2022) 20(1):110. doi: 10.1186/s12916-022-02299-z
87. Wang Y, Maina NH, Coda R, Katina K. Challenges and opportunities for wheat alternative grains in breadmaking: ex-situ- versus in-situ-produced dextran. Trends Food Sci Technol (2021) 113:232–44. doi: 10.1016/j.tifs.2021.05.003
88. Alaei F, Hojjatoleslamy M, Hashemi Dehkordi SM. The effect of inulin as a fat substitute on the physicochemical and sensory properties of chicken sausages. Food Sci Nutr (2018) 6(2):512–9. doi: 10.1002/fsn3.585
89. Karimi R, Azizi MH, Ghasemlou M, Vaziri M. Application of inulin in cheese as prebiotic, fat replacer and texturizer: a review. Carbohydr Polym (2015) 119:85–100. doi: 10.1016/j.carbpol.2014.11.029
90. Chassaing B, Compher C, Bonhomme B, Liu Q, Tian Y, Walters W, et al. Randomized controlled-feeding study of dietary emulsifier carboxymethylcellulose reveals detrimental impacts on the gut microbiota and metabolome. Gastroenterology (2022) 162(3):743–56. doi: 10.1053/j.gastro.2021.11.006
91. Suez J, Cohen Y, Valdés-Mas R, Mor U, Dori-Bachash M, Federici S, et al. Personalized microbiome-driven effects of non-nutritive sweeteners on human glucose tolerance. Cell (2022) 185(18):3307–28.e19. doi: 10.1016/j.cell.2022.07.016
92. Wang Y, Jian C, Salonen A, Dong M, Yang Z. Designing healthier bread through the lens of the gut microbiota. Trends Food Sci Technol (2023) 134:13–28. doi: 10.1016/j.tifs.2023.02.007
Keywords: prebiotics (Sources: MeSH), childhood obesity, gut microbiota, short-chain fatty acids (SCFAs), bacteroides
Citation: Wang Y, Salonen A and Jian C (2023) Can prebiotics help tackle the childhood obesity epidemic? Front. Endocrinol. 14:1178155. doi: 10.3389/fendo.2023.1178155
Received: 02 March 2023; Accepted: 10 May 2023;
Published: 26 May 2023.
Edited by:
Solaleh Emamgholipour, Tehran University of Medical Sciences, IranReviewed by:
Raylene A. Reimer, University of Calgary, CanadaReyhane Ebrahimi, Max Planck Institute for Multidisciplinary Sciences, Germany
Copyright © 2023 Wang, Salonen and Jian. This is an open-access article distributed under the terms of the Creative Commons Attribution License (CC BY). The use, distribution or reproduction in other forums is permitted, provided the original author(s) and the copyright owner(s) are credited and that the original publication in this journal is cited, in accordance with accepted academic practice. No use, distribution or reproduction is permitted which does not comply with these terms.
*Correspondence: Ching Jian, Y2hpbmcuamlhbkBoZWxzaW5raS5maQ==