- 1Department of Pharmaceutical Biotechnology, School of Pharmacy and Pharmaceutical Sciences, Isfahan University of Medical Sciences, Isfahan, Iran
- 2Department of Biomedical Engineering, Faculty of Engineering and Natural Sciences, Istinye University, Istanbul, Türkiye
- 3Department of Applied Mathematics, Faculty of Mathematics, University of Waterloo, Waterloo, ON, Canada
- 4Department of Prosthodontics Dentistry, Dental Materials Research Center, Dental Research Institute, School of Dentistry, Isfahan University of Medical Sciences, Isfahan, Iran
- 5Belfer Center for Applied Cancer Science, Dana Farber Cancer Institute, Boston, MA, United States
- 6Translational Sciences, Xsphera Biosciences Inc., Boston, MA, United States
- 7School of Pharmacy and Pharmaceutical Sciences, Isfahan University of Medical Sciences, Isfahan, Iran
- 8Department of Medical Laboratory Sciences, School of Allied Medical Sciences, Lovely Professional University, Phagwara, Punjab, India
- 9Division of Research and Development, Lovely Professional University, Phagwara Punjab, India
- 10Institute of Research and Development, Duy Tan University, Da Nang, Vietnam
- 11School of Medicine and Pharmacy, Duy Tan University, Da Nang, Vietnam
Type 2 Diabetes Mellitus (T2DM) has been the main category of metabolic diseases in recent years due to changes in lifestyle and environmental conditions such as diet and physical activity. On the other hand, the circadian rhythm is one of the most significant biological pathways in humans and other mammals, which is affected by light, sleep, and human activity. However, this cycle is controlled via complicated cellular pathways with feedback loops. It is widely known that changes in the circadian rhythm can alter some metabolic pathways of body cells and could affect the treatment process, particularly for metabolic diseases like T2DM. The aim of this study is to explore the importance of the circadian rhythm in the occurrence of T2DM via reviewing the metabolic pathways involved, their relationship with the circadian rhythm from two perspectives, lifestyle and molecular pathways, and their effect on T2DM pathophysiology. These impacts have been demonstrated in a variety of studies and led to the development of approaches such as time-restricted feeding, chronotherapy (time-specific therapies), and circadian molecule stabilizers.
1 Introduction
According to the World Health Organization (WHO), diabetes mellitus (DM) is a metabolic disorder characterized by high level of blood glucose (1) that could damage most of the vital organs including the cardiovascular system (2), eyes (3), kidneys (4), and nervous system (5, 6). More than 90% of all instances of diabetes mellitus are type 2 diabetes mellitus (T2DM) (7), which is characterized by a combination of two different factors: inability of the pancreatic β-cells in producing sufficient amounts of insulin and lack of insulin adsorption by its targeted cells (8). Type 2 Diabetes Mellitus patients are normally obese people with accumulation of body fat, especially in the abdominal area (9). The worldwide changes in the lifestyle patterns including consumption of high-calorie foods (10), and the aging population (11) are the primary causes of the T2DM epidemic.
Fine-tuning the molecular pathways involved in the synthesis and release of insulin and their response in tissues is required to ensure that insulin would meet the body’s metabolic demands (12). Therefore, the smallest metabolic imbalance in any of the relevant pathways could lead to the pathogenesis of T2DM (13). For instance, Adipokine dysregulation and increased free fatty acid release from adipose tissue are two of the inflammatory events that contribute to the development of insulin resistance in the whole body (14).
According to the epidemiological statistics, the predicted rates of T2DM are alarmingly high. The International Diabetes Federation estimates that by 2045, there will be 700 million people worldwide with diabetes (7). In 2019, 4.2 million deaths occurred among people with diabetes between the ages of 20-79 years old. Moreover, in the same year, more than $720 billion was paid for the treatments related to the diabetes, showing its extreme economic burden. The true burden of disease is likely underreported as one in three people with diabetes is underdiagnosed. Most patients with diabetes are middle-aged (40-59 years old) (15) and more than 80% of them live in low- to middle-income countries, which presents additional treatment challenges. Cardiovascular disease (CVD) is the main cause of mortality in T2DM patients compared to those without diabetes, while it could also lead to other vascular-related outcomes and stroke (2). Besides, diabetes can affect other organs such as pancreas (both β and α cells) (16), liver (17), muscle (18), kidneys (19), brain (20), gut (21), and adipose tissue (22). Furthermore, changes in gut microbiota (23), immunological diseases (24), and inflammation (25) are increasingly being recognized as important pathophysiological variables in the onset of metabolic syndrome (26).
Type 2 diabetes can result from the interaction of numerous inherited and environmental risk factors (27, 28). One of the interesting factors that has been proven to have a role in prevalence of T2DM is genetic and environmental changes in circadian rhythm. For example, T2DM is significantly more common in those who work in shifts, such as night and evening shifts (29). It has been demonstrated that days and/or weeks- long exposure to acute circadian disruption leads to changes in glucose metabolism as well (30).
2 Pathogenesis of T2DM
Pathogenesis of T2DM is fueled by inducing insulin resistance in skeletal muscle, liver, and fat so that insulin signaling is disturbed (31). Insulin resistance leads to a significant reduction in the glucose clearance, especially through the skeletal muscles that are the primary organ responsible for the postprandial glucose reduction (32). Muscle insulin resistance is due to a combination of factors, including but not limited to 1) insufficient insulin-mediated transport of glucose transporter type 4 (GLUT4) to the plasma membrane; 2) reduced glycogen accumulation; 3) decreased oxidation of glucose; and 4) altered the activity of mitochondria (32, 33). Fasting hepatic glucose production is elevated in an insulin resistance caused by impaired regulation of gluconeogenesis. In a hepatic insulin resistance state, livers produce more glucose after a meal since their ability to metabolize glucose is impaired (34). Finally, reducing the insulin-mediated glucose transport, lipid absorption, lipolysis, and inflammation in adipose tissue can lead to an increase in the plasma free fatty acids (FFAs) (35, 36).
Cellular insulin resistance is resulted from the accumulation of abnormal lipids in insulin-sensitive tissues like liver, muscles, and adipose tissue (36). Indeed, activation of insulin signaling cascade in muscle and liver cells is inhibited by the accumulation and trafficking of lipid messengers such as ceramides and diacylglycerols that are elevated in obesity (37). Besides, cumulation of intracellular diacylglycerols and ceramides could induce the inactivation of insulin receptor substrates 1 and 2 (IRS-1 and IRS-2) and create insulin resistance (38, 39). Protein kinase C and other atypical serine/threonine kinases trigger this pathway (40). Poor adipocyte metabolism has been linked to both obesity and T2DM, leading to an increase in lipolysis and FFA levels and an elevation in the expression level of inflammatory cytokines such as tumor necrosis factor-α (TNF-α) and interleukin-6 (IL-6) from activated adipose tissue macrophages (41–43). As a result, abnormal metabolism in adipose tissue of patients with T2DM is attributed to the excessive fat storage in target tissues via mediating alterations in the insulin signaling cascade in the skeletal muscle and liver.
Hyperglycemia cannot occur without the presence of both insulin resistance and pancreatic islet failure, both of which are characteristic of T2DM (44). Patients with T2DM who suffer from islet failure have impaired glucose-stimulated insulin production and uncontrolled glucagon release. Reduced β-cell populations and impaired β-cell secretory capacity contribute to the impaired glucose-stimulated insulin secretion (45). Increased apoptosis of β-cells along with the development of dedifferentiated α-cells have been linked to the β-cell mass reduction observed in type 2 diabetes (45). Moreover, exposure to islet amyloid peptide oligomers (IAPP), hyperlipidemia, and hyperglycemia is associated with the activation of intracellular oxidative and/or endoplasmic reticulum stress (46, 47). There is also limited evidence that changes in β-cells number and activity is the underlying reason of the defected glucagon suppression observed in diabetes; however, these possibilities need further investigation (48, 49).
2.1 Glucose homeostasis mechanism
Signals from organs such as pancreas, brain, gut, and liver exert considerable control on blood glucose homeostasis. The rise in blood glucose levels following a meal is the primary stimulus for insulin release from β-cells (50–52). The glucose transporter 2 (GLUT2) facilitative glucose transporter, found on the surface of β-cells, is responsible for glucose uptake from the circulation (53). During glycolysis, as glucose enters the cells and its level raises, the ATP/ADP ratio and ATP production increase (54). The increased ATP leads to the inhibition of ATP-sensitive K+ channels (KATP), channels that are responsible for the constant resting potential in the absence of stimuli via pumping K+ ions (55, 56). This leads to the reduced outward K+ current, followed by membrane depolarization, and thus, opening the voltage-gated Ca+ channels (VDCC) (57, 58). Insulin is secreted when granules containing the hormone fuse with the cell membrane, a process triggered by the increased intracellular calcium concentration.
The action of insulin on cells is mediated by its attachment to the extracellular subunits of insulin receptors on the cell surface; that leads to the phosphorylation of this receptor, the tyrosine receptor kinases (RTKs), followed by the activation of downstream pathways mediating the cellular effects (59, 60).
Another hormone emitted by the pancreas is called glucagon, which is released by alpha cells in the pancreatic islets when blood glucose levels drop. It counteracts with the insulin role in glucose regulation via increasing the production and secretion of glucose. Ketogenesis and lipolysis in the liver are both boosted by glucagon (51). The liver senses glucose levels in the portal vein and sends a signal to the arcuate nucleus (ARC) via vagal afferents, leading to smaller meals and increased postprandial satiety (61, 62). In addition to activating the G-protein-coupled receptors (GPCR) pathways in the ARC, glucagon has also the ability to pass through the blood-brain barrier (BBB) in animal models which suggests that it may directly act on the central nervous system to control hunger (63, 64).
Amylin, which is released together with insulin by pancreatic cells, inhibits the activity of orexigenic neurons in the anterior cingulate cortex to reduce hunger (65). Amylin inhibits gastric secretions and postprandial glucagon release through stimulating the region postrema in the medulla of the brainstem. Somatostatin, the hormone secreted from the pancreatic cells, has roles in regulation of appetite, digestion, and glucose metabolism via exocrine, endocrine, and brain pathways (66–68). It could suppress the expression of different hormones, from glucagon and insulin to gastrin, prolactin, secretin, and thyroid stimulating hormone (69), digestive enzymes, stomach acid, and bile produced by the digestive system (70–73).
The adrenal glands produce corticosteroids, which regulate many bodily functions. The release of this class of hormones follows a 24-hour cycle, controlled by the circadian clock. They can be broken down into two primary groups: mineralocorticoids and glucocorticoids (74). Glucocorticoids have anti-inflammatory effects in addition to regulating carbohydrate, protein, and lipid metabolism, whereas mineralocorticoids such as aldosterone manage the balances of fluid and electrolyte through modifying the renal tubules activity (74). Cortisol, the primary endogenous glucocorticoid in human physiology that is related to the stress, stimulates gluconeogenesis to raise blood glucose levels under both stress and hypoglycemia (75, 76).
Postprandially, cells in the digestive tract secrete a class of peptide hormones called incretins, which play a role in controlling both blood glucose and nutrient absorption. Gastric inhibitory peptide (GIP) and Glucagon-like peptide-1 (GLP-1) are two primary incretins that lower blood glucose via increasing the insulin release from pancreatic cells (77, 78). In addition to controlling the adsorption rate of nutrients into the body, incretins reduce the speed of stomach emptying. Dipeptidyl peptidase-4 (DPP-4) could inhibit the GLP-1 and GIP activity, so the inhibitors of DPP-4 along with several types of GLP-1 and GIP analogues have been applied in clinical therapy of T2DM; however, more research is needed to clarify the therapeutic use of these medications for T2DM (79) (Figure 1).
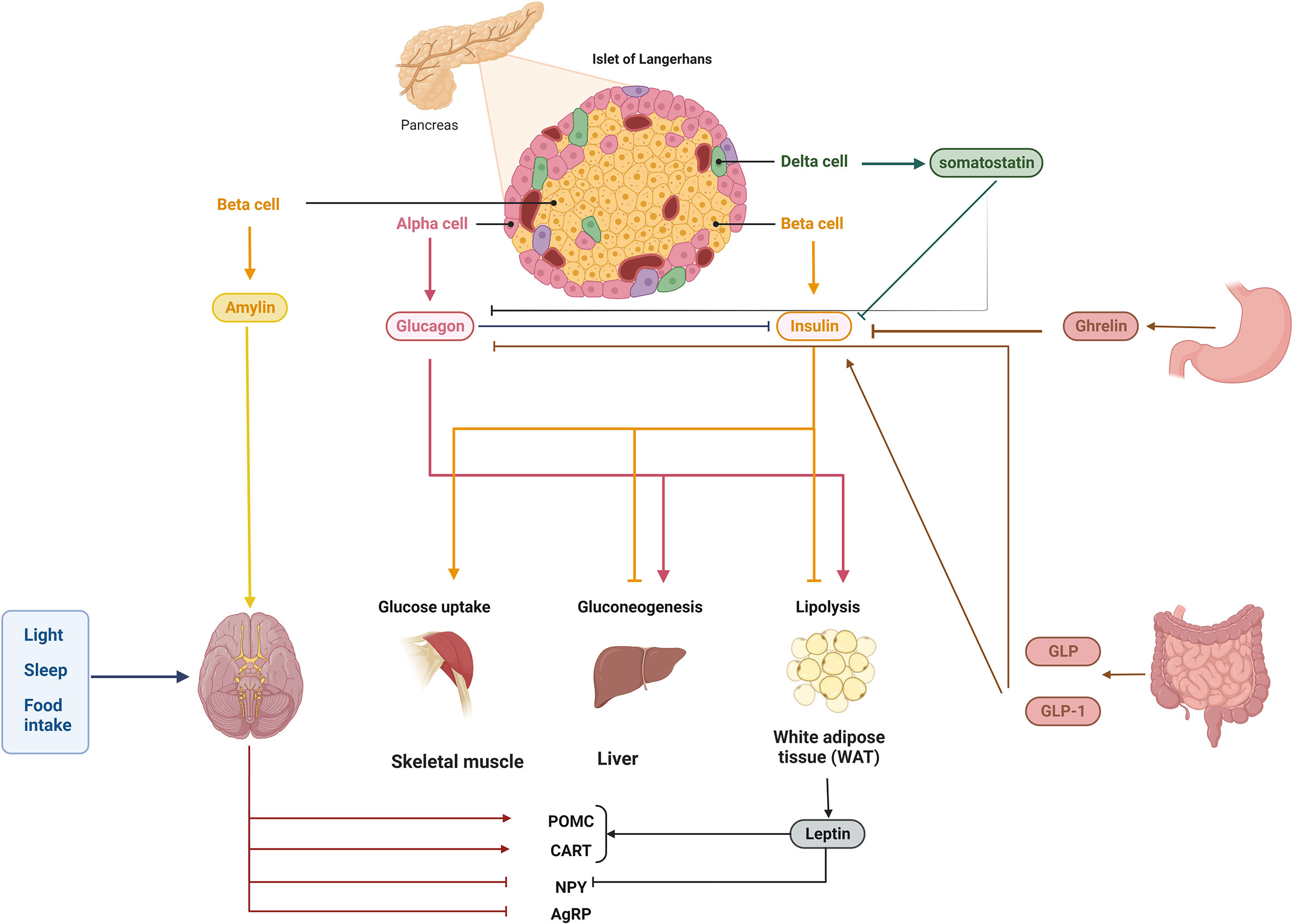
Figure 1 Homeostasis of blood glucose is controlled by a complex network of interactions. Multiple signals from the pancreas and further away from the brain, liver, muscle, intestine, stomach, and adipose tissue regulate blood glucose homeostasis. Glucagon, insulin (together with amylin), pancreatic polypeptide, and somatostatin are produced by cells in the pancreatic islets. Islet cells emit insulin in response to high glucose, such as from food intake; this insulin is detected by numerous peripheral tissues, which then synthesize or induce several molecules/pathways, such as lipogenesis and gluconeogenesis, and block others, such as glycogenolysis. Importantly, insulin secretion can be regulated both locally and at a distance, for example, by hormones and incretins in the colon and the stomach. Blood glucose homeostasis can be regulated by endogenous hormones like glucocorticoids, which also display rhythmicity, and by exogenous variables like light exposure and food consumption, which modulate circadian rhythms.
2.2 Circadian rhythm of glucose homeostasis: Diabetic vs healthy
It has been known for nearly half a century that normal human subjects exhibit a diurnal cycle in glucose tolerance. The rise in plasma glucose concentrations is much lower in the evening than in the morning after ingesting glucose orally or receiving an intravenous infusion of glucose. Mice and rats, like humans, have been shown to have diurnal rhythms (80). They have a lower glucose tolerance during the day (resting phase) than at night since they are nocturnal (active at night). The reasons behind impaired glucose tolerance include insulin resistance, high hepatic glucose production (HGP) from the liver, and impaired β-cell function. Research shows that insulin sensitivity and β-cell responsiveness to glucose are both lower in healthy adults after dinner than before breakfast. In people with normal glucose levels, the function of HGP in the diurnal regulation of glucose homeostasis is unclear. Researchers have found conflicting results regarding the diurnal variation in HGP: some have found a decrease in HGP associated with sleep and an increase in HGP at dawn, while others have found no diurnal rhythm in 24-hour fasting HGP or a decrease in HGP before breakfast compared to the lunch and dinner. One possible explanation for the divergent findings is that the participants in these trials had diverse eating habits on the day of testing. Patients with T2DM commonly experience the dawn phenomenon (hyperglycemia in the morning), which may be explained by the diurnal cycle of HGP. The increased HGP in the morning before breakfast has been linked to both a circadian modulation of HGP, as demonstrated by rodent research, and a prolonged overnight fast, which causes a rush of counterregulatory hormones (such as cortisol, growth hormones, and norepinephrine) (81–83).
Patients with T2DM frequently have fasting/morning hyperglycemia, which can be partially attributed to an increase in the overnight production of endogenous glucose. Endless laboratory work has been prompted by the observation of the daily fluctuations of glucose regulation in an effort to pinpoint and characterize the underlying endogenous circadian rhythms of glucose regulation. With the need to better understand diabetes and its effects, more studies in differential glycemic control over the course of the day in people with and without diabetes is needed (84).
3 The circadian rhythm: an overview
Without any environmental cues, circadian rhythms are generated by an internal molecular clock that runs for about 24 hours. A “master” clock present in the suprachiasmatic nucleus (SCN) of the hypothalamus coordinates the molecular clocks in several peripheral tissues into a unified, hierarchical system. About 20,000 neurons make up the SCN to create a highly integrated circadian network (85). Light signals from the retina are processed by the master clock, which then relays the obtained information to the peripheral clocks through the endocrine and systemic cues (86, 87). The molecular architecture and the ability to generate sustained circadian rhythms are shared by the master and peripheral clocks situated in SCN neurons and across peripheral tissues, with the degree of intercellular connectivity between these clocks being the primary distinction.
The systemic changes (like body temperature), metabolic changes (for example by changes in exercise and food intake), changes in sleep pattern (e.g. shift work or jetlag) and the circulating hormones are the regulators of the phase of the SCN clock, while the phase of peripheral clocks can be adjusted independent of the SCN clock (85, 86, 88). This is related to the high degree of intercellular coupling of the SCN neurons, which create a neuronal network resistant to the phase perturbations of the internal cues. This networking logic makes sure that the master clock always stays on time by keeping its intrinsic 24-hour timing in sync with the solar cycle, while the peripheral clocks adjust to show the local metabolic status of the tissues in which they function (Figure 2) (85).
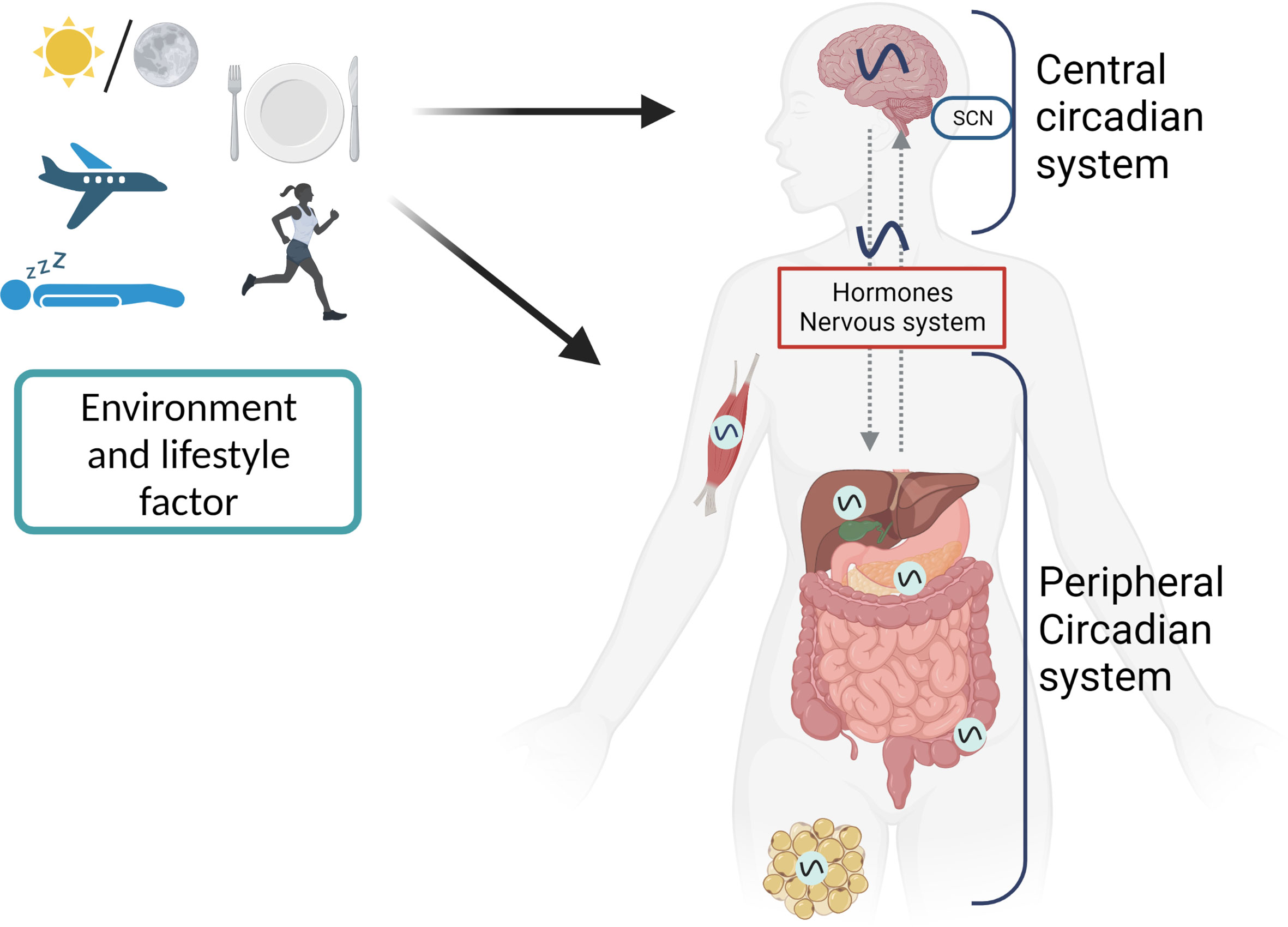
Figure 2 The first level of circadian disruption occurs when environmental cycles and/or behavioral cycles are out of sync with the SCN. Second, internal misalignment between the central clock and the peripheral clocks, generated by, for example, misaligned meals, can create circadian disruption at the organismal level. Another manifestation of this is an improper phase relationship between the peripheral clocks of various organs. Desynchronization between cells inside tissue can alter circadian rhythms on a cellular level.
Pancreatic islet cell subtypes, skeletal myocytes, adipocytes, and hepatocytes are all examples of metabolically important peripheral tissues/cells that have self-sustaining and cell-autonomous circadian oscillators (89–93). Without consistent stimulation from the SCN, these cell-autonomous oscillators can keep cellular processes rhythmic over the course of a 24-hour day (94). However, the SCN clock appears to integrate a complicated multi-level hierarchical oscillator network by coordinating and synchronizing tissue circadian oscillators in response to neuronal, humoral, and behavioral inputs (95, 96).
It was previously believed that the SCN only used a single biological mechanism for the regulation of the phase entrainment and circadian physiology of the peripheral tissue oscillators; however, recent research suggests otherwise (97). The autonomic nervous system, specifically the parasympathetic and sympathetic axons, aids in SCN-dependent entrainment of circadian rhythms. Autonomic regulation of the hepatic circadian clock, which is responsible for the circadian rhythms in the production of hepatic glucose and ambient glycemia, is mediated by the sleep-wake circadian network (98, 99). The SCN plays a pivotal role in cell types that are vulnerable to daily dietary challenges by indirectly managing the entrainment of peripheral oscillators via modification of the fasting/feeding cycles. It is suspected that a combination of food-related metabolites and gut-related hormonal factors mediates the circadian entrainment of peripheral oscillators in response to meals, although the specific processes involved remain unknown (100). The SCN also controls circadian cycles in temperature and hormones (e.g., glucocorticoids) (101). In mammals it manages peripheral oscillator entrainment and uses redundant communication channels to fine-tune the temporal regulation of a wide variety of circadian rhythms.
3.1 Molecular pathway of the circadian rhythm
The cell-autonomous molecular clock of the mammals consists of two transcription/translation feedback loops (TTFL) that interact with each other and generate strong 24-hour rhythms for gene expression. The core of TTFL is composed of four integral clock proteins: two activators (CLOCK and BMAL1) and two repressors (Period circadian protein homolog;PER1 and PER2) (102, 103). Their location and stability are regulated by kinases and phosphatases (kinases: CKI, phosphatases: PP1, PP5). CLOCK: BMAL1 is a type of transcription factor with basic helix-loop-helix-PAS (PER-ARNT-SIM) structure that stimulates the expression of the Per and Cry (Cryptochrome) repressor genes and other genes responsible for the clock-controlled output (104). The heterodimer formed in the cytoplasm between PER and CRY proteins is transported to the nucleus, so that it could inhibit further transcriptional activity via interacting by CLOCK: BMAL1. After about 24 hours, PER and CRY proteins are destructed through a ubiquitin-dependent processes, releasing the regulation of CLOCK: BMAL1. Phosphatases (PP1 and PP5) are used for preventing and regulating the activity of casein kinases (CKI and CKI, respectively), which is a critical factor for controlling the clock intrinsic period (Figure 3) (105, 106).
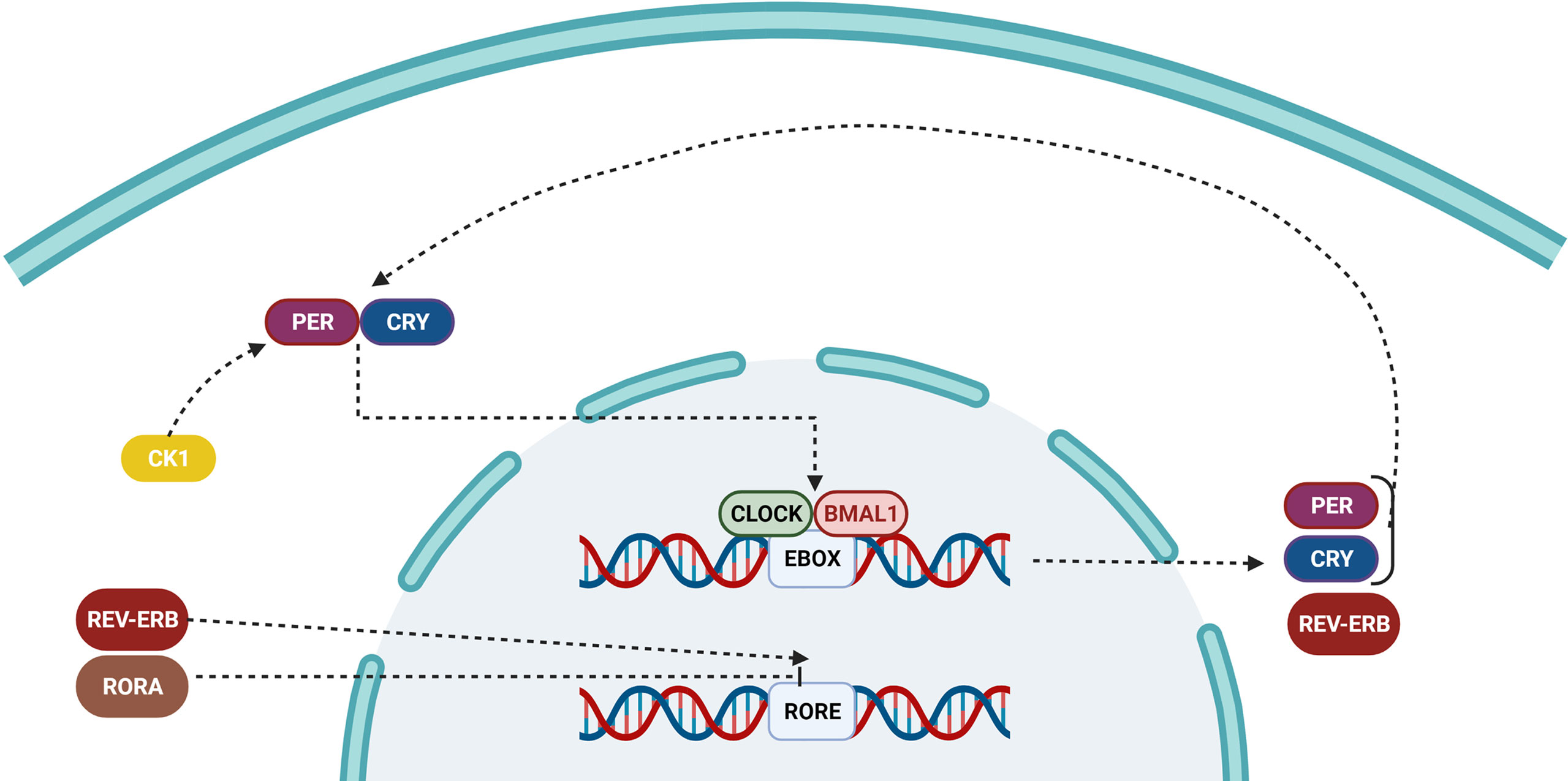
Figure 3 Pathway of the circadian clock inside a cell. Over time, the accumulation of PER and CRY proteins binds to CLOCK/BMAL1, and switches it from an active to an inactive state, preventing it from repressing the transcription of downstream genes. The ROR/REV-ERB complex controls the primary feedback loop by influencing RORE.
Sleep phase issues are shared by mice and humans when either the intrinsic duration of the clock is reduced (S662G) or a loss-of-function mutation occurs in CKI (T44A). By altering the location and stability of PER, pharmacological inhibition of casein kinases was shown to have a significant role in period length determination. Transcriptional activators (retinoid-related orphan receptors RORa, b, and c) and repressors (REV-ERBa and REV-ERBb) are involved in generation of the second TTFL (107, 108). ROR/REV-TTFL-induced delay in the expression of Cry1 is critical for the appropriate circadian timing; however, rhythmic changes in the abundance of BMAL1 are not necessary for driving the core loop of TTFL. Gene expression is appropriately timed for local physiology thanks to the cooperation and interlocking of feedback loops that induce phase delays in the output of the circadian transcription and make a robustness against the environmental disturbance and probable noises (109).
4 Diabetes and the circadian rhythm: a new frontier in diabetes research
4.1 Genetic signatures in the human genome
The metabolic risk of T2DM can be increased or decreased by specific single nucleotide polymorphisms (SNPs) in humans. Lipid metabolism can also cause SNPs in circadian genes (such as PER3), and on the other hand, the presence of SNPs in circadian genes (such as CLOCK and CRY1 in intestinal cholesterol absorption) can play a role in fat metabolism and increase the chance of T2DM (110–114). Risk factors for developing metabolic syndrome (such as hypertension) are also associated to a SNP presented in the structure of neuronal PAS domain protein 2 (NPAS2), a paralog of CLOCK with the ability of binding to the BMAL1 (115). Bmal1 SNPs are associated with high blood pressure in addition to T2DM, hyperglycemia, and gestational diabetes (116, 117). Patients with Cry2 and Per2 SNPs are prone to the glucose tolerance disorder, and individuals with Per2 SNPs, who overeat and experience stress-related eating, tend to acquire more weight (115, 118, 119).
These SNPs could also affect the expression level of several other genes. For example, the sleep/wake cycles are aided by the secretion of more melatonin in the evening and less during the day. Elevated fasting glucose levels could impair cellular functions and increase the risk of T2DM in the presence of two SNPs in the melatonin receptor 1B gene (Mtnr1b) (120, 121). Melatonin secretion patterns could be affected by these SNPs and lead to an increased risk in diabetes. Accordingly, it seems that the risk of metabolic syndrome, obesity, and T2DM could be significantly influenced by SNPs in the molecular circadian clock and the genes regulated by those SNPs (122).
In a 2021 study, researchers showed a genetic network between the circadian cycle and T2DM (123). In this study, integrating gene expression patterns with the biomolecular networks of the genome-scale in diabetes samples allowed for a meta-analysis of data pertaining to genes involved in T2DM and different types of cancers (including bladder, breast, pancreatic, liver, colon, and rectum cancers). They found that both of these disorders shared a common deregulation of a number of genes. For example, although the expression level of Arntl2 and Agrp were increased in T2DM and cancer samples, Usp2, Ezh2, Igf1, Klf10, and Ntrk3 were downregulated (123). Phenotypic changes in T2DM appear to be linked with the malignant transformation, which may partially be related to the alterations in mRNA. Using these genes for survival analysis, they discovered associations between only Arntl2, Usp2, and Igf1. Survival was poor in BLCA and BRCA cancer samples where Arntl2 was upregulated, while downregulation of Usp2 and Igf1 had a negative effect (123).
4.2 Peripheral circadian rhythm: a central role in diabetes
4.2.1 Pancreatic circadian rhythm
It has long been understood that individuals’ ability to maintain their blood glucose levels stable throughout the day is varied with the time. Indeed, improved glucose tolerance is related to higher effects of glucose and β-cell targeting secretagogues on insulin secretion, which is first observed at the start of the active circadian cycle (124, 125). Classic experiments using a 72h glucose clamp methodology corroborated these first data, showing that humans exhibit strong circadian rhythms of insulin secretion independent of feeding and glycemia (29). Moreover, the cell-autonomous circadian clock appears to oversee the diurnal rhythms in the glucose-induced insulin secretion. These diurnal rhythms are maintained in the isolated pancreatic islets and pure β-cell populations in human, animal, or in vitro models (29, 126). Besides, circadian fluctuations have also been seen in the glucagon production in vivo and in vitro (islet cultures and isolated α-cells). However, our knowledge of the circadian regulation of the α-cell glucagon response is considerably limited (127).
Many research groups have established the effects and roles of the autonomous circadian clock (e.g., Per1 and Per2) in pancreatic islets (127–130). The results of these studies demonstrated that clock gene expression in islet cells exhibits robust independent circadian rhythms with a clear 24h period, phase, and amplitude of circadian oscillations. Moreover, they showed that SCN entrains the circadian clocks phases of the islets via modulating the feeding behavior. It was also seen that shifts in photoperiod and/or obesogenic meals can jumble the islet circadian clock (131). Besides, the pure populations of α- and β-cells exhibit robust cell-autonomous circadian oscillators, with discrete phases in transcriptional and functional oscillations (89, 132).
Similar to other metabolically active cells such as skeletal muscle and liver, the transcriptional profile of islet cells is subject to circadian oscillations regulated by the cellular clocks. Around one-third of the transcripts in mouse islets show circadian cycles, which is consistent with previous findings. The biological pathways involved in the secretion and exocytosis of insulin (including SNARE interactions, vesicular transport, and exocytosis), mitochondrial function, processing and transporting of protein in endoplasmic reticulum, and cell turnover regulation, are enriched in circadian-expressed transcripts in islet cells (such as DNA damage and repair and DNA replication) (133, 134). Recent research has shown that each of the distinct islet cell subtypes have their own circadian transcripts, with expression peak during the feeding or fasting (activating or inactivating) of the circadian cycle, which corresponds to the glucagon and insulin release peak (130, 135).
4.2.2 Liver circadian rhythms
Liver plays a critical role in orchestrating the glucose homeostasis’s circadian regulation throughout the day. It is the diurnal change in the amount of hepatic glucose synthesis that regulates the circadian variations in blood glucose under fasting conditions (136). Because of this, circadian rhythms in the concentrations of blood glucose are lost if the circadian clock of SCN (or liver-specific) is disrupted (137). The liver also acts in the circadian modulation of postprandial glucose metabolism since the circadian changes in the amounts of glucose production by the liver could affect the diurnal cycles of meal tolerance and insulin sensitivity. In addition, both animal and human models have shown that the rates of gluconeogenesis and glycogenolysis, as well as glycogen storage strongly fluctuate during the day (29, 138).
Hepatocytes exhibit specific diurnal oscillations in gene expression and morphological properties such as volume and size of cells (139–141). Hepatocytes display patterns of circadian expression for a number of metabolic genes, including Glut2, Gck, Pepck, and Gcgr. These genes are critical for regulating hepatic glucose production, and lipid oxidation in hepatic cells, respectively. Both nuclear and cytoplasmic proteome of hepatocytes undergoes significant diurnal variation associated with rhythm in cellular metabolism, proliferation, DNA repair, ribosome synthesis, and intracellular protein trafficking. Multiple diurnal rhythms in liver function, as well as the corresponding of diurnal oscillations in the transcriptome and proteome of the liver, appear to be primarily regulated by fasting-feeding cycles. Consequently, while assessing the mechanisms regulating daily circadian rhythm in the liver, it is important to distinguish between clock-dependent and clock-independent factors (such as feeding-driven factors) (142). Besides it as important to add that feeding may be a stronger cue for hepatic circadian rhythm than light-dark cycle as feeding restricted to resting phase inverts metabolic rhythm of liver (143, 144).
Scientists have uncovered some of the processes and targeted molecules related to the clock-controlled transcriptional regulation of hepatocytes by utilizing chromatin immunoprecipitation (ChIP-Seq) and deep sequencing. For instance, Rey and coworkers mapped almost 2,000 BMAL1 binding sites across the entire genome in the liver (145). Additional evidence have shown that hepatic clocks are critical mediators of glucose homeostasis since most of the binding sites were located/mapped in promoter areas of genes related to the metabolism of hepatic glucose (like Glut2 and G6pc) and lipid (such as Agpat6 and Dgat2). This finding is supported by the in vivo test observation that showed mice with a liver-specific deletion of Bmal1 exhibit systemic glycemia and glucose intolerance due to the absence of rhythmic regulation of glucose metabolism related genes (e.g Glut2) (146, 147). Newer studies built on these observations, demonstrating that hepatic Bmal1 is essential for controlling hepatic oxidative capacity through orchestrating intracellular mitochondrial dynamics such as mytophagy and fission (146, 148).
Through the activation of different transcriptional and translational regulators, hepatic clocks coordinate the cellular metabolism regulation via an “indirect” method. By performing a ChIP-Seq analysis on binding sites of BMAL1 of the liver, they were able to show that “transcriptional control” was the most significantly enriched annotated functional cluster, included about 82 DNA transcription factors and 18 liver-expressed nuclear receptors. In lipid and cholesterol metabolism, hepatic clocks activate Kruppel-like 10 (Klf10) and the nuclear receptor Ppar (peroxisome proliferator-activated receptor) (149–151). The fact that both Klf10 and Ppar regulate Bmal1 promoter activity demonstrates the intricate relationship between the circadian clock and metabolism of liver (149, 150). Finally, research have shown that proteins involved in the circadian clock (such as CRY 1 and 2, and PER2) attune the regulation of circadian over hepatic glucose metabolism via modulating the signaling of cAMP and the post-translational function of nuclear receptor. Diabetic mice with overexpression of Cry1 in the liver had reduced gluconeogenesis and blood glucose levels. Researchers convincingly showed that Cry1 expression during the circadian clock inhibits the key enzymes of gluconeogenic activation through binding and blocking the Gs subunit of hepatic glucagon receptor (152, 153). Growing documents indicate that hepatic clocks play a critical role in the regulation of glucose production and uptake and insulin sensitivity in T2DM.
4.2.3 Muscle circadian rhythms
It was revealed by in vivo and in vitro studies skeletal myocytes contain functional, self-sustaining, and independently oscillating circadian rhythm. It is important to note that circadian fluctuations occur for almost every physiological factor involved in glucose homeostasis in skeletal muscle (92, 154, 155). Changes in protein and lipid metabolism, as well as glucose oxidation and uptake, deposition of glycogen, the oxidative capability of mitochondria, and expression and translocation of the GLUT4, occur in skeletal muscle on a daily basis (156–158). Consistent with its basic function, the circadian system increases metabolic flexibility, as evidenced by the fact that the maximum physiological activity of skeletal muscle in uptake and oxidation of glucose is coincided with the commencement of circadian cycles in both human and animal (mouse) tests. In accordance with the transcriptional control by the endogenous circadian clock mechanism, circadian rhythms in skeletal muscle activities (such as glucose absorption) are maintained in isolated muscle cells (158). In addition, T2DM is linked to alterations in the circadian modulation of glucose uptake and oxidation in muscle, suggesting that the disruption of the circadian clock is a factor that could affect the pathogenesis of insulin resistance in skeletal muscle of patients with diabetes (159, 160).
The strongest evidence that confirmed the critical role of biological clock in regulating the function of skeletal muscle is provided by the studies done on Bmal1 knockout mice models. The loss of Bmal1 is unique among clock genes because it eliminates circadian rhythms in behavior, physiology, and molecular processes. Notably, muscle-specific transgenic rescue can correct the profound decrease in the mass, function, and mitochondrial density of skeletal muscle resulted from a systemic loss of Bmal1 (161). Bmal1 knockout mice models have been established to clarify the function of this gene in the regulation of glucose metabolism in skeletal muscle. It is interesting to note that Bmal1 knockouts generally show a significant reduction in glucose and insulin tolerance, resulting from reduced insulin-stimulated glucose uptake, which is a major factor in T2DM (162, 163).
Disturbing the glucose uptake related to the Bmal1 deletion could also reduce the expression level of TBC1D1, a protein essential for GLUT4 translocation from the cytoplasm to the plasma membrane (164, 165). Notably, reduction in the amounts of glucose transport documented in patients with T2DM also affects skeletal muscle. Deletion of Bmal1 in muscle could change the cellular metabolism toward increasing the lipid usage and storage, and reduce the expression and activity of essential metabolic enzymes that are responsible in the breakdown and oxidation of glucose (164). Again, this is consistent with the phenotypic alterations in T2DM patients’ skeletal muscles. Extensive experimental data suggest that impaired insulin-mediated glucose transport, absorption, storage, and oxidation in skeletal muscle contribute to the onset and progression of T2DM (166).
4.2.4 Adipocyte circadian rhythm
In addition to act as an energy source, white adipose tissue (WAT) has metabolic and endocrine functions including blood glucose level regulation. The body utilizes the triglycerides (TGs) stored in WAT for energy through a process called lipolysis, in which the TGs are broken down into FFAs and glycerol (167). Lipolysis and lipogenesis, as two main paths of fat metabolism in the body, play a very important role in glucose homeostasis, since the excess fat are accumulated in different tissues especially liver and muscles, and cause insulin resistance and interfere with glucose homeostasis (168). Human plasma-free fatty acid concentrations have been shown to vary throughout the day, and this diurnal variation has been believed to be caused by the cyclic changes of the insulin signaling cascade activation in adipose tissue (169).
The circadian clock has been demonstrated to govern adipose tissue functions such as lipolysis, adipogenesis, and metabolic inflammation in mammals because of the dramatic daily variations in energy needs (170, 171). Obesity is reliably induced in Clock and/or Bmal1 mutant mice, likely as a result of elevated levels of plasma FFAs and triglycerides. Studies in rodents and humans have also uncovered the importance of circadian expression for many of the genes that are critical for the appropriate regulation of metabolism of adipocyte in different adipose tissue depots, suggesting that circadian clocks play a role in regulating adipocyte metabolism (91, 172). As an illustration, one study demonstrated that the heterodimer of BMAL1 and CLOCK had a transcriptional regulation on the activity of two important lipolysis pacemaker enzymes that lead to the clock-regulated release of FFA and glycerol (173). Adipocytes had a reduced ability to produce polyunsaturated fatty acids in Bmal1-deficient animals. This could be due to decreased expression of Elovl6 and Scd1, two critical genes involved in the elongation and desaturation of FFA (173, 174).
Adipose tissue is known as the energy stores as well as one of the largest endocrine organs due to its seemingly endless capacity to expand and produce a wide range of hormones and cytokines. Leptin, adiponectin, resistin, visfatin, omentin, and inflammatory cytokines (such as tumor necrosis factor-alpha (TNF-α), interleukin-6 (IL-6), and monocyte chemoattractant protein-1 (MCP-1)) are just some of the known hormones that are released by adipocytes in both obesity and diabetes (175, 176). Macrophages found in adipose tissue are one of the best-known contributors in the insulin resistance induced by obesity. Adipose tissue macrophages (ATMs) have been demonstrated to convert from anti-inflammatory M2 phase to pro-inflammatory M1 type in diabetes conditions, and release the pro-inflammatory cytokines such as TNF-α, IL-6) and IL-12 (43, 177, 178). Although the precise role of the circadian clock in regulating inflammation in adipose tissue remains unclear, there is mounting evidence that suggests disruptions in these pathways can trigger inflammatory reactions (179). For instance, the expression level of TNF-α and macrophage-1 antigen (MAC1) in WAT was increased in obese mice with a disrupted circadian clock (177, 178, 180). Macrophages with a disturbed circadian clock (Per2 mutant) showed higher M1 macrophage polarization in WAT and exhibited heightened pro-inflammatory activation in response to LPS. Downregulation of PPAR, an important clock-regulated transcription factor used for the polarization of M2 macrophage, in macrophages replicated the same results in obese mice with Per1/2-disrupted myeloid cells (41). Overwhelming evidence suggests that the circadian clock could regulate the biology of adipose tissue from multiple aspects (such as lipogenesis, lipolysis, insulin sensitivity, inflammatory pathways, etc.) so that the disruption of this clock could lead to an increase in the adiposity, insulin resistance, and susceptibility to the development of T2DM (176, 181).
4.3 Environmental and epidemiological factors
4.3.1 Light and circadian rhythm
Constant light exposure decreased the domain (the difference between the highest and lowest points) of the SCN rhythmicity in mice, as measured by electrophysiological monitoring. As a result, food consumption rose while energy expenditure fell (87, 182). In a study in mouse models, the effect of the light cycle on fat and glucose metabolism was investigated. Results demonstrated the effect of light on obesity and development of diabetes. Body weight gain, adipocyte area, glucose intolerance, and insulin resistance were all augmented in low-fat diet (LFD) and high-fat diet (HFD) mice exposed to the circadian-disrupting (CD) cycle under higher intensity (HI) but not lower intensities (LI) (183). In addition, LFD-HI therapy was associated with an increase in blood and hepatic triglyceride levels independent of the light cycle. Another benefit of CD cycle was reflected in the improvement of glucose and lipid metabolism in HFD-LI. Moreover, the deleterious effects of CD cycle on the metabolism were mitigated under the LI condition, particularly in HFD mice (183) (Figure 4).
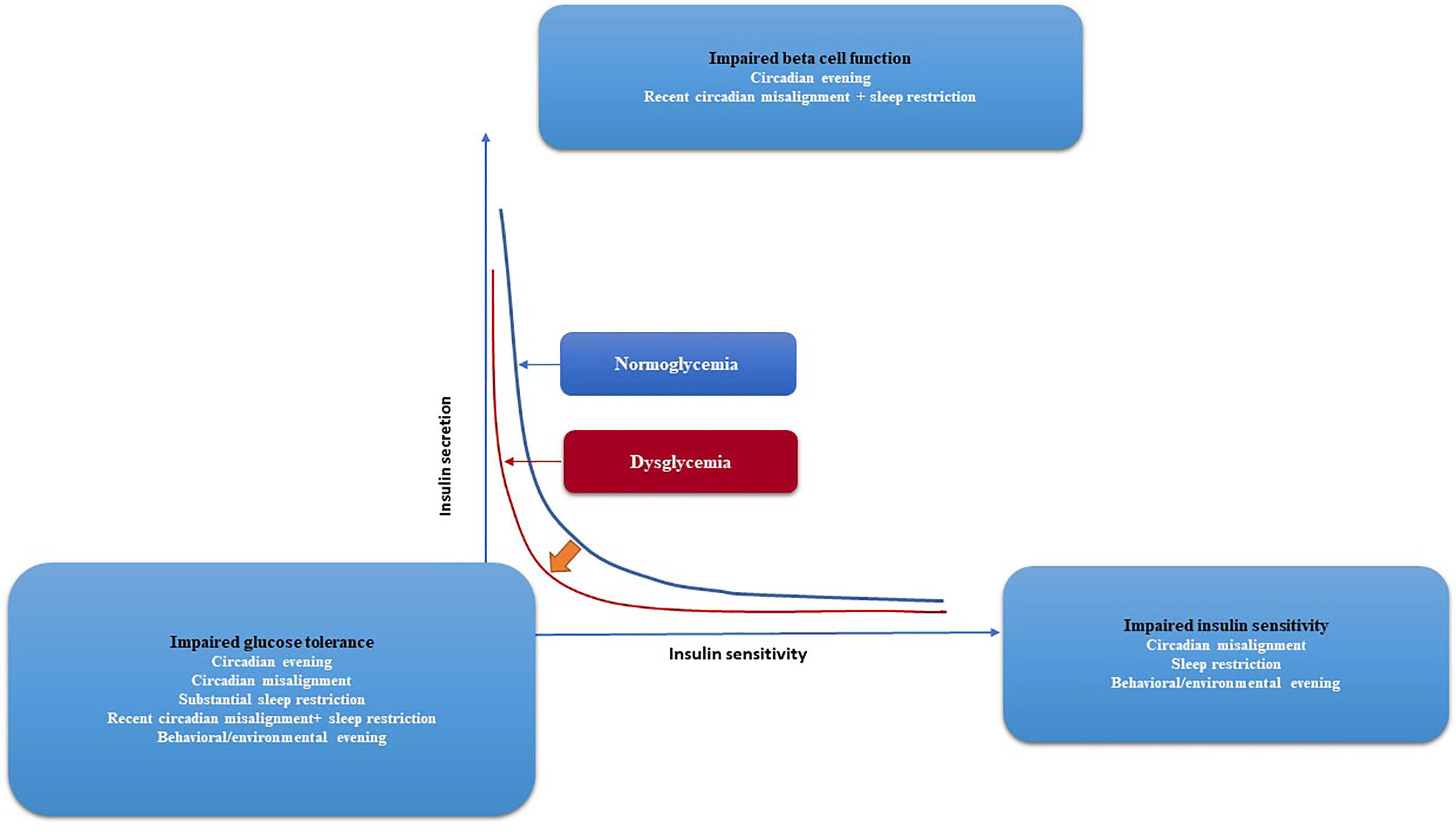
Figure 4 Glycemic control and the risk of developing diabetes, together with the effects of circadian and behavioral factors are depicted graphically. The two hyperbolae for normoglycaemia (blue line) and dysglycaemia (red line) illustrate the correlation between insulin secretion and insulin sensitivity (red line). Lower insulin sensitivity requires more insulin secretion for normal blood glocuse levels. Impaired glucose tolerance arises if insulin secretion is unable to compensate for diminished insulin sensitivity, as shown by a leftward shift of the curve. Circadian disarray, sleep restriction, and, to a lesser extent, behavioral/environmental lateness all have negative effects on insulin sensitivity, according to experiments. Both recent circadian misalignment and nocturnal awakening have negative effects on beta cell function. Evidence suggests that a number of factors—including behavioral/environmental evening, circadian misalignment, significant sleep restriction, recent circadian misalignment with sleep restriction, and severe sleep restriction—combine to reduce glucose tolerance. The progression from normoglycemia to dysglycemia and eventually diabetes may be influenced by circadian and behavioral factors that affect glycaemic regulation.
4.3.2 Food intake and circadian rhythm
The hypothalamus, adipose, and hepatic clocks are all affected by a high-fat diet in animal models. Circadian rhythms are also affected by the timing of meals. The postprandial glucose response to a meal may be affected by the time of day, which would have a profound impact on T2DM (184). Earlier mealtime consumption has been linked to lower levels of postprandial glucose in both observational and experimental investigations. Increasing the protein and fat content of evening meals has been found to be a straightforward method for lowering postprandial glucose levels (184). Foods with a low glycemic index (GI) have a negative impact on the glycemic response if they were taken in the morning than later (185). The glycaemic response can be lowered by carefully timing the consumption of fat and protein with carbohydrate items like bread and rice. There is interesting evidence that changing dietary habits such as time and number of meals, the interval between meals, and the amount of each meal can influence postmeal glucose levels and metabolism. Instead of solely considering the dietary content of a meal, people with diabetes can benefit from considering these recommendations to improve their glycaemic management (185).
The majority of food consumed by mice maintained on a 12h light/12h dark cycle (12:12h LD) is consumed during the dark phase. However, in a study that was conducted to determine the effects of the time of feeding on weight gain, it was concluded that mice that were only fed during the light phase acquired more weight compared to those that were only fed during the dark phase (186). In another experiment, the effect of time-restricted feeding (TRF) on preventing obesity and metabolic syndrome was investigated in the liver of Rev-erb α/β and Bmal1 knockout mice and all organs of Cry1;Cry2 knockout mice (187). When these mice were given free access to food, they quickly became obese and displayed metabolic abnormalities that were determined by their genetic makeup. However, when they were fed with the same diet but with TRF, they were in a healthy weight range with no metabolic disorders. It was discovered through transcriptome and metabolome analysis that TRF lowered hepatic fat buildup and improved cellular resistance to metabolic stress (187).
Same as what was seen in animal studies, the timing of meals has been proven to affect the risk of diabetes development in humans. A study in 2018 has shown that there is a direct link between eating dinner late and gene MTNR1B (melatonin receptor 1B), which affects glucose metabolism, increased body fat and weight gain (188). Previously, the relationship of this gene with type 2 diabetes has been proven (189). Since exogenous melatonin has also been demonstrated to cause decreased glucose tolerance, the suggested role of melatonin in this pathway is in line with that discovery (190). Time-restricted eating (TRE), eating within a specific daily window, has been shown to improve the metabolic indices such as atherogenic lipids, weight, visceral fat, and blood pressure in people with metabolic syndrome. In 2019, a randomized controlled clinical trial was conducted to evaluate the association of 9-hour TRF with diabetes risk in men. In this study, 15 men were randomly divided into two early (TRFe) or delayed (TRFd) groups, and during and before (to achieve baseline glucose) the study, the volunteers’ blood glucose and insulin were measured. The level of blood lipids and steroid hormones were measured in all volunteers. The results showed that TRF can improve glycemic responses regardless of whether it is early or delayed (191). The benefits of TRE were tested and reported by a small sample size (n=15–20) and a larger clinical study (192, 193).
4.3.3 Work shift and circadian rhythm
Shift work, and circadian misalignment more generally, are associated with the increased risk of metabolic syndrome, obesity, and T2DM. A real-world investigation comparing day-shift and night-shift workers supports the hypothesis that circadian misalignment is a risk factor for metabolic disorders (134). Results showed that compared to day-shift workers, night-shift workers had higher levels of glucose, insulin, and triacylglycerol after eating a meal. A meta-analysis of 12 observational studies reported that shift work is associated with an increased risk of T2DM (9%), compared to those who have never worked shifts (194). It’s important to note that workers whose shifts alternate throughout the week are at a greater risk than those whose schedules remain stable. This is probably because both food intake and light exposure are an inappropriate time when the body is not prepared for them. Also, similar to the human results, rats subjected by a simulated shift-work procedure (using rotating running wheels) became obese and experienced a flattening of their glucose rhythms. Accelerated cell dysfunction and loss along with impaired GSIS were observed in rats whose 12:12h LD cycles were disrupted by continuous light exposure (131, 195). Research on Bmal1 knockout mice has revealed the critical function of Bmal1 in mitigating oxidative stress and adjusting to circadian rhythm disturbances. Therefore, mice with a dysfunctional version of this gene are more likely to develop diabetes (196).
The timing of food consumption, light exposure, and nutrient content have all been recognized as important factors used for regulating the metabolic clock in both rodents and humans. These results suggest that time-specific therapy (chronotherapy) and other strategies that target the circadian system, like the analogs of synthetic circadian protein, could be useful for the management of metabolic syndrome and T2DM.
5 Chronotherapy; new weapon in a war against diabetes
Chronotherapy consists of maintaining an optimized circadian rhythm and regulating the timing of drugs to achieve more efficacy and fewer side effects (197). An example of medication timing is prescribing statins to be used at night. The reason is that statins target 3-hydroxy-3-methylglutaryl coenzyme A (HMG CoA) reductase. The activity of this enzyme is highest at night and during sleep; therefore, targeting it at night with statins can be more effective (198).
A dopamine agonist, bromocriptine, is an adjunctive therapy for T2DM. Following a 24h cycle, hypothalamic dopaminergic activity drives hepatic gluconeogenesis and adipocyte lipolysis (199). When taken within 2h of awakening, bromocriptine is expected to inhibit this drive, helping to prevent hyperglycemia and dyslipidemia (199). Despite FDA approval for the treatment of T2DM and evidence of the drug’s effectiveness in lowering blood glucose levels, bromocriptine is rarely used in actual clinical practice (199). In a study in 2021, results showed that in addition to greater effectiveness, the reduction of complications, especially cardiovascular complications, can be seen in the treatment with bromocriptine based on the circadian rhythm; however, with all this evidence, further preclinical and clinical studies are still needed to identify the exact relationship between circadian rhythm and bromocriptine (200, 201).
Despite laboratory evidence that showed the time-dependent effects of metformin on blood glucose and its interaction with the circadian rhythm molecular components, no clinical trials have looked at the optimal time of day to take this drug (202, 203). Scientists found that the time of day significantly affected the initial drop in blood glucose and the levels of lactate in the blood of healthy mice after metformin treatment (204). They further showed that circadian time has significant effects on the activation of AMP-activated protein kinase (AMPK) in the liver via affecting the kinetics of metformin. Loss of Bmal1 expression in the liver modifies the AMPK induction and blood glucose response to metformin, while can’t completely eliminate the diurnal variations. Combined, these findings highlight the complexity with which circadian rhythms influence physiologic responses to metformin (204). In addition to the effect of the circadian cycle on metformin, it has also had very significant effects on modifying the circadian cycle of different tissues (such as liver and muscles) in diabetes (205, 206).
The endocrine cells of pineal glands secrete a hormone called melatonin, which was the first circadian-based medication to be studied for its potential to treat diabetes (207, 208). Since its production and secretion are governed by direct neural inputs from the SCN, it has been argued that the rhythmic secretion of melatonin is a hormonal response to the central circadian clock (209). Indeed, the SCN and peripheral circadian oscillators are directly affected by exogenous melatonin treatment via the ubiquitously expressed melatonin receptors. Thus, it has been proven that supplementing with melatonin at the proper time improves the global circadian rhythms in both mice and humans (209, 210). By improving the insulin signaling cascade of the cells, melatonin treatment in animals has been found to reduce obesity and attenuate insulin resistance of the liver and skeletal muscle. In isolated T2DM islets, it was able to enhance the survival of β-cells and improve the insulin secretion (stimulated by glucose); therefore, reduce the oxidative and endoplasmic reticulum stress (211–213). Although many studies have pointed out the potential therapeutic effects of melatonin in the control and treatment of diabetes, there is still a need to conduct targeted clinical studies for determining the effectiveness of this drug in T2DM treatment (214–217). In another study, the effectiveness of melatonin and metformin at the same time in the treatment of diabetes was discussed in rat that had become diabetic due to circadian rhythm disorder and obesity (218). As a stand-alone therapy, melatonin improved the activity of circadian rhythms, suppressed the induction of β-cell failure, and enhanced the tolerance of glucose. On the other hand, metformin improved the sensitivity of insulin and glucose tolerance without affecting circadian rhythms; however, it was taken to help with sleep issues (218). Importantly, melatonin and metformin demonstrated synergistic activities to ameliorate obesity, insulin sensitivity, circadian activity, and suppression of islet cell failure in CDO rats, and so slowing the onset of metabolic dysfunction. Based on the results of this research, it seems reasonable to consider treating metabolic and circadian dysfunctions together as a means of preventing and treating obesity and T2DM (218).
More research on small-molecule pharmacological enhancers of the circadian system has been conducted in recent years to improve the clock-regulated physiological outputs. Subsequently, several pharmacological agents have been created with established efficacy in modifying metabolic function in pre-clinical investigations, all of which focus on the core circadian oscillator (219, 220). Initially, thiazolidinedione, a medication used to treat diabetes, was identified as the first synthetic agonist for ROR (Retinoic Acid-Related Orphan Receptors) and the only medication available for the treatment of insulin resistance (221, 222). Nobiletin, another ROR inhibitor and a naturally occurring flavonoid has been discovered to increase clock amplitude through activation of the ROR nuclear receptor (223). Specifically, administration of Nobiletin for an extended periods of time improved the glycemia, glucose tolerance, insulin resistance, and ectopic fat formation in two different mouse models of obesity and T2DM (224, 225). Rev-ERB agonist, a recently found pharmacological regulator of the circadian system was shown to have the capability of modifying the gene expression of circadian metabolic and ameliorate glycemia, lipidemia, and ectopic fat deposition in mice (226). One of these compounds is berberine, which intervenes in the circadian cycle through three pathways; 1) reducing the activity of the luciferase reporters Nlrp3 and Bmal1, 2) increasing the repressor activity of REV-ERB, and 3) reducing the expression levels of REV-ERB target genes (227). In another clinical trial about the effect of berberine in the treatment of diabetes, the results provided evidence that berberine is a safe and effective therapeutic agent for diabetes, particularly when used in combination with other medications. This may be useful in the future for directing the clinical application of berberine and the development of drugs to treat type 2 diabetes and dyslipidemia (228).
Cell-based phenotypic screening for drugs that modulated circadian rhythmicity led to the discovery of the first CRY agonist (KL001) (229, 230). The mechanism of action of KL001 involves blocking the interaction of the ubiquitin ligases that bind to the main binding pocket of CRY and tag them for destruction. In order to extend the repressive phase of the clock, CRY’s lifetime can be extended by binding tiny molecules in the binding pocket (229). Improvements in glucose tolerance in the db/db mice model of diabetes have been observed in vivo with compounds that were discovered through in silico drug design research began with KL001 (231).
6 In-silico approach: systematic study in biology and pharmacology
Understanding diseases, determining the relationship between various cellular pathways, and selecting an appropriate treatment all benefit greatly from the use of various computational and simulation technologies (such as artificial intelligence, molecular docking, etc.). Systems biology refers to the discipline that uses computational tools and models of complex biological systems to systematically investigate biological phenomena (232, 233).
These computational analyses and sophisticated models have also found application in the field of pharmacology. Therapeutic efficacy and side effect predictions (including organ toxicity and genetic toxicity) and medication repositioning could be improved with the help of these computational investigations. System pharmacology is the name given to this branch of pharmacology (234, 235).
6.1 Drug combination prediction
Because of their potential to boost efficacy or lessen unwanted side effects, combination treatments are increasingly being used in the treatment of a wide range of disorders. In addition, optimizing the inhibition of different cell pathways can enhance the process of treating a disease when several pathways contribute to its incidence or treatment. Given the large number of available medications as well as intricate physiological pathways, the use of computational methods to predict the ideal therapeutic combination is critical and can save time and money on various research (236–238).
For example, in 2021, Teng and et.al, used In silico method for predicting effective drug combinations for T2DM treatment. They compiled data on genes linked to T2DM and built a disease module for the condition. They then identified promising medications by assessing their spatial closeness to the disease module. Gene Set Enrichment Analysis (GSEA) was then used to narrow down the list of possible medications based on the drug-induced gene expression profiles. This network-based approach had also been used in predicting potential drug combinations for type 2 diabetes (237).
Considering the relationship between circadian cycle and type 2 diabetes and the specificity of different cellular pathways, the use of these computational methods can speed up the use of combination therapy based on circadian cycle in the treatment of diabetes.
6.2 Drug repositioning
To date, more than 5,000 drugs have been approved by the US Food and Drug Administration in various therapeutic indications, and many drugs are in different phases of clinical studies. This volume of drugs and available data on their effects on cells and different cellular pathways gives us the possibility to find new targets for drugs that have previously been approved and used for other targets without the need to design new drugs; This reuse of drugs is called drug repositioning (239). The use of drug repositioning can significantly reduce the cost and time of new treatments. A very indicative example of the application of drug repositioning was seen in the treatment of Covid-19. In addition to many computational and clinical studies, many drugs mentioned in computational studies could show appropriate effects in clinical studies. They were used as a treatment option in the treatment protocol for Covid-19 (240, 241).
Considering that no known drugs has yet been introduced as an effective intervention in the circadian cycle and approved in clinical trials, implementing drug repositioning to suggest effective medicinal compounds on different targets in the circadian cycle can be very practical and bring forth the treatment based on the circadian rhythm.
7 Conclusion
Various studies have shown the effect of circadian rhythm in the creation, progression, and treatment of diabetes, especially T2DM. These studies, which were mostly laboratory- and animal-based, have provided suitable evidence for designing and conducting further wider studies, especially at the clinical level. Moreover, chronotherapy has been proposed as one of the most trending areas of pharmacology, which is very important in making personalized medicine a reality in terms of more effectiveness and less side effects. Also, with a better understanding of chronotherapy, we can suggest combination treatments based on the combination of drugs with environmental and lifestyle factors. New laboratory tools and techniques such as organ-on-a-chip, microfluidic-based diagnostic tools, and new generation sequences allow us to conduct more studies on different aspects of the circadian rhythm and its relationship with diabetes. Also, with the progress of computational tools and biological modeling, as well as the huge growth of data science and artificial intelligence, chronotherapy appears to be a very promising approach in the treatment of different types of diseases.
Author contributions
Conceptualization: MM, AH, and PS. Methodology: MM and AA. Investigation: AH, MK, MA-P, and AT. Resources: MM and AZ. Writing—original draft preparation: AH, MK, MA-P, AT, and PP. Writing—review and editing: MM, AZ, AA, and PS. All authors contributed to the article and approved the submitted version.
Conflict of interest
Author AA was employed by Xsphera Biosciences Inc.
The remaining authors declare that the research was conducted in the absence of any commercial or financial relationships that could be construed as a potential conflict of interest.
Publisher’s note
All claims expressed in this article are solely those of the authors and do not necessarily represent those of their affiliated organizations, or those of the publisher, the editors and the reviewers. Any product that may be evaluated in this article, or claim that may be made by its manufacturer, is not guaranteed or endorsed by the publisher.
References
1. Scully T, Ettela A, LeRoith D, Gallagher EJ. Obesity, type 2 diabetes, and cancer risk. Front Oncol (2021) 10. doi: 10.3389/fonc.2020.615375
2. De Rosa S, Arcidiacono B, Chiefari E, Brunetti A, Indolfi C, Foti DP. Type 2 diabetes mellitus and cardiovascular disease: genetic and epigenetic links. Front Endocrinol (Lausanne) (2018) 9:2. doi: 10.3389/fendo.2018.00002
3. Wang W, Lo ACY. Diabetic retinopathy: pathophysiology and treatments. Int J Mol Sci (2018) 19. doi: 10.3390/ijms19061816
4. Nordheim E, Geir Jenssen T. Chronic kidney disease in patients with diabetes mellitus. Endocr Connect (2021) 10:R151–r159. doi: 10.1530/EC-21-0097
5. Grote CW. Wright DE. a role for insulin in diabetic neuropathy. . Front Neurosci (2016) 10. doi: 10.3389/fnins.2016.00581
6. Luna R, Talanki Manjunatha R, Bollu B, Jhaveri S, Avanthika C, Reddy N, et al. A comprehensive review of neuronal changes in diabetics. Cureus (2021) 13:e19142. doi: 10.7759/cureus.19142
7. Khan MAB, Hashim MJ, King JK, Govender RD, Mustafa H, Al Kaabi J. Epidemiology of type 2 diabetes - global burden of disease and forecasted trends. J Epidemiol Glob Health (2020) 10:107–11. doi: 10.2991/jegh.k.191028.001
8. Westman EC. Type 2 diabetes mellitus: a pathophysiologic perspective. Front Nutr (2021) 8. doi: 10.3389/fnut.2021.707371
9. Veit M, van Asten R, Olie A, Prinz P. The role of dietary sugars, overweight, and obesity in type 2 diabetes mellitus: a narrative review. Eur J Clin Nutr (2022) 76:1497–501. doi: 10.1038/s41430-022-01114-5
10. Sami W, Ansari T, Butt NS, Hamid MRA. Effect of diet on type 2 diabetes mellitus: a review. Int J Health Sci (Qassim) (2017) 11:65–71.
11. Longo M, Bellastella G, Maiorino MI, Meier JJ, Esposito K, Giugliano D. Diabetes and aging: from treatment goals to pharmacologic therapy. Front Endocrinol (2019) 10. doi: 10.3389/fendo.2019.00045
12. Brännmark C, Nyman E, Fagerholm S, Bergenholm L, Ekstrand EM, Cedersund G, et al. Insulin signaling in type 2 diabetes: experimental and modeling analyses reveal mechanisms of insulin resistance in human adipocytes. J Biol Chem (2013) 288:9867–80. doi: 10.1074/jbc.M112.432062
13. Hameed I, Masoodi SR, Mir SA, Nabi M, Ghazanfar K, Ganai BA. Type 2 diabetes mellitus: from a metabolic disorder to an inflammatory condition. World J Diabetes (2015) 6:598–612. doi: 10.4239/wjd.v6.i4.598
14. Kim WK, Bae KH, Lee SC, Oh KJ. The latest insights into adipokines in diabetes. J Clin Med (2019) 8. doi: 10.3390/jcm8111874
15. Safiri S, Karamzad N, Kaufman JS, Bell AW, Nejadghaderi SA, Sullman MJM, et al. Prevalence, deaths and disability-Adjusted-Life-Years (DALYs) due to type 2 diabetes and its attributable risk factors in 204 countries and territories, 1990-2019: results from the global burden of disease study 2019. Front Endocrinol (2022) 13. doi: 10.3389/fendo.2022.838027
16. Eizirik DL, Pasquali L, Cnop M. Pancreatic β-cells in type 1 and type 2 diabetes mellitus: different pathways to failure. Nat Rev Endocrinol (2020) 16:349–62. doi: 10.1038/s41574-020-0355-7
17. Guerra S, Gastaldelli A. The role of the liver in the modulation of glucose and insulin in non alcoholic fatty liver disease and type 2 diabetes. Curr Opin Pharmacol (2020) 55:165–74. doi: 10.1016/j.coph.2020.10.016
18. Maliszewska K, Adamska-Patruno E, Goscik J, Lipinska D, Citko A, Krahel A, et al. The role of muscle decline in type 2 diabetes development: a 5-year prospective observational cohort study. Nutrients (2019) 11. doi: 10.3390/nu11040834
19. Wilding JPH. The role of the kidneys in glucose homeostasis in type 2 diabetes: clinical implications and therapeutic significance through sodium glucose co-transporter 2 inhibitors. Metabolism (2014) 63:1228–37. doi: 10.1016/j.metabol.2014.06.018
20. Garcia-Serrano AM, Duarte JMN. Brain metabolism alterations in type 2 diabetes: what did we learn from diet-induced diabetes models? Front Neurosci (2020) 14. doi: 10.3389/fnins.2020.00229
21. Cunningham AL, Stephens JW, Harris DA. Gut microbiota influence in type 2 diabetes mellitus (T2DM). Gut Pathog (2021) 13:50. doi: 10.1186/s13099-021-00446-0
22. Daryabor G, Atashzar MR, Kabelitz D, Meri S, Kalantar K. The effects of type 2 diabetes mellitus on organ metabolism and the immune system. Front Immunol (2020) 11:1582. doi: 10.3389/fimmu.2020.01582
23. Zhou Z, Sun B, Yu D, Zhu C. Gut microbiota: an important player in type 2 diabetes mellitus. Front Cell Infection Microbiol (2022) 12. doi: 10.3389/fcimb.2022.834485
24. de Candia P, Prattichizzo F, Garavelli S, De Rosa V, Galgani M, Di Rella F, et al. Type 2 diabetes: how much of an autoimmune disease? Front Endocrinol (Lausanne) (2019) 10:451. doi: 10.3389/fendo.2019.00451
25. Tsalamandris S, Antonopoulos AS, Oikonomou E, Papamikroulis GA, Vogiatzi G, Papaioannou S, et al. The role of inflammation in diabetes: current concepts and future perspectives. Eur Cardiol (2019) 14:50–9. doi: 10.15420/ecr.2018.33.1
26. Regufe VMG, Pinto C, Perez P. Metabolic syndrome in type 2 diabetic patients: a review of current evidence. Porto BioMed J (2020) 5:e101. doi: 10.1097/j.pbj.0000000000000101
27. Ling C, Rönn T. Epigenetics in human obesity and type 2 diabetes. Cell Metab (2019) 29:1028–44. doi: 10.1016/j.cmet.2019.03.009
28. Ling C, Bacos K, Rönn T. Epigenetics of type 2 diabetes mellitus and weight change [[/amp]]mdash; a tool for precision medicine? Nat Rev Endocrinol (2022) 18:433–48. doi: 10.1038/s41574-022-00671-w
29. Kalsbeek A, la Fleur S, Fliers E. Circadian control of glucose metabolism. Mol Metab (2014) 3:372–83. doi: 10.1016/j.molmet.2014.03.002
30. Noh J. The effect of circadian and sleep disruptions on obesity risk. J Obes Metab Syndr (2018) 27:78–83. doi: 10.7570/jomes.2018.27.2.78
31. Galicia-Garcia U, Benito-Vicente A, Jebari S, Larrea-Sebal A, Siddiqi H, Uribe KB, et al. Pathophysiology of type 2 diabetes mellitus. Int J Mol Sci (2020) 21. doi: 10.3390/ijms21176275
32. Abdul-Ghani MA, DeFronzo RA. Pathogenesis of insulin resistance in skeletal muscle. J BioMed Biotechnol (2010) 2010:476279. doi: 10.1155/2010/476279
33. da Silva Rosa SC, Nayak N, Caymo AM, Gordon JW. Mechanisms of muscle insulin resistance and the cross-talk with liver and adipose tissue. Physiol Rep (2020) 8:e14607. doi: 10.14814/phy2.14607
34. Mu W, X-f C, Liu Y, Q-z Lv, G-l L, J-g Z, et al. Potential nexus of non-alcoholic fatty liver disease and type 2 diabetes mellitus: insulin resistance between hepatic and peripheral tissues. Front Pharmacol (2019) 9. doi: 10.3389/fphar.2018.01566
35. Petersen KS, Bowen KJ, Tindall AM, Sullivan VK, Johnston EA, Fleming JA, et al. The effect of inflammation and insulin resistance on lipid and lipoprotein responsiveness to dietary intervention. Curr Developments Nutr (2020) 4. doi: 10.1093/cdn/nzaa160
36. Metcalfe LK, Smith GC, Turner N. Defining lipid mediators of insulin resistance: controversies and challenges. J Mol Endocrinol (2019) 62:R65–82. doi: 10.1530/JME-18-0023
37. Hardy OT, Czech MP, Corvera S. What causes the insulin resistance underlying obesity? Curr Opin Endocrinol Diabetes Obes (2012) 19:81–7. doi: 10.1097/MED.0b013e3283514e13
38. Sokolowska E, Blachnio-Zabielska A. The role of ceramides in insulin resistance. Front Endocrinol (2019) 10. doi: 10.3389/fendo.2019.00577
39. Kolczynska K, Loza-Valdes A, Hawro I, Sumara G. Diacylglycerol-evoked activation of PKC and PKD isoforms in regulation of glucose and lipid metabolism: a review. Lipids Health Dis (2020) 19:113. doi: 10.1186/s12944-020-01286-8
40. Petersen MC, Shulman GI. Roles of diacylglycerols and ceramides in hepatic insulin resistance. Trends Pharmacol Sci (2017) 38:649–65. doi: 10.1016/j.tips.2017.04.004
41. Fujisaka S. The role of adipose tissue M1/M2 macrophages in type 2 diabetes mellitus. Diabetol Int (2021) 12:74–9. doi: 10.1007/s13340-020-00482-2
42. Kraakman MJ, Murphy AJ, Jandeleit-Dahm K, Kammoun HL. Macrophage polarization in obesity and type 2 diabetes: weighing down our understanding of macrophage function? Front Immunol (2014) 5. doi: 10.3389/fimmu.2014.00470
43. Banerjee A, Singh J. Remodeling adipose tissue inflammasome for type 2 diabetes mellitus treatment: current perspective and translational strategies. Bioeng Transl Med (2020) 5:e10150. doi: 10.1002/btm2.10150
44. Radlinger B, Ramoser G, Kaser S. Exocrine pancreatic insufficiency in type 1 and type 2 diabetes. Curr Diabetes Rep (2020) 20:18. doi: 10.1007/s11892-020-01304-0
45. Wysham C, Shubrook J. Beta-cell failure in type 2 diabetes: mechanisms, markers, and clinical implications. Postgraduate Med (2020) 132:676–86. doi: 10.1080/00325481.2020.1771047
46. Haataja L, Gurlo T, Huang CJ, Butler PC. Islet amyloid in type 2 diabetes, and the toxic oligomer hypothesis. Endocr Rev (2008) 29:303–16. doi: 10.1210/er.2007-0037
47. Bram Y, Frydman-Marom A, Yanai I, Gilead S, Shaltiel-Karyo R, Amdursky N, et al. Apoptosis induced by islet amyloid polypeptide soluble oligomers is neutralized by diabetes-associated specific antibodies. Sci Rep (2014) 4:4267. doi: 10.1038/srep04267
48. Capozzi ME, Wait JB, Koech J, Gordon AN, Coch RW, Svendsen B, et al. Glucagon lowers glycemia when β-cells are active. JCI Insight (2019) 5. doi: 10.1172/jci.insight.129954
49. Wang M-Y, Dean ED, Quittner-Strom E, Zhu Y, Chowdhury KH, Zhang Z, et al. Glucagon blockade restores functional β-cell mass in type 1 diabetic mice and enhances function of human islets. Proc Natl Acad Sci (2021) 118:e2022142118. doi: 10.1073/pnas.2022142118
50. Roh E, Song DK, Kim M-S. Emerging role of the brain in the homeostatic regulation of energy and glucose metabolism. Exp Mol Med (2016) 48:e216–6. doi: 10.1038/emm.2016.4
51. Röder PV, Wu B, Liu Y, Han W. Pancreatic regulation of glucose homeostasis. Exp Mol Med (2016) 48:e219. doi: 10.1038/emm.2016.6
52. Han HS, Kang G, Kim JS, Choi BH, Koo SH. Regulation of glucose metabolism from a liver-centric perspective. Exp Mol Med (2016) 48:e218. doi: 10.1038/emm.2015.122
53. Thorens B. GLUT2, glucose sensing and glucose homeostasis. Diabetologia (2015) 58:221–32. doi: 10.1007/s00125-014-3451-1
54. Deng D, Yan N. GLUT. SGLT, and SWEET: structural and mechanistic investigations of the glucose transporters. Protein Sci (2016) 25:546–58. doi: 10.1002/pro.2858
55. McTaggart JS, Clark RH, Ashcroft FM. The role of the KATP channel in glucose homeostasis in health and disease: more than meets the islet. J Physiol (2010) 588:3201–9. doi: 10.1113/jphysiol.2010.191767
56. Koster JC, Permutt MA, Nichols CG. Diabetes and insulin secretion: the ATP-sensitive k+ channel (K ATP) connection. Diabetes (2005) 54:3065–72. doi: 10.2337/diabetes.54.11.3065
57. Tuluc P, Theiner T, Jacobo-Piqueras N, Geisler SM. Role of high voltage-gated Ca(2+) channel subunits in pancreatic β-cell insulin release. From Structure to Funct Cells (2021) 10. doi: 10.3390/cells10082004
58. Rorsman P, Ramracheya R, Rorsman NJG, Zhang Q. ATP-regulated potassium channels and voltage-gated calcium channels in pancreatic alpha and beta cells: similar functions but reciprocal effects on secretion. Diabetologia (2014) 57:1749–61. doi: 10.1007/s00125-014-3279-8
59. Zhao M, Jung Y, Jiang Z, Svensson KJ. Regulation of energy metabolism by receptor tyrosine kinase ligands. Front Physiol (2020) 11. doi: 10.3389/fphys.2020.00354
60. Hubbard SR. The insulin receptor: both a prototypical and atypical receptor tyrosine kinase. Cold Spring Harb Perspect Biol (2013) 5:a008946. doi: 10.1101/cshperspect.a008946
61. Galsgaard KD. The vicious circle of hepatic glucagon resistance in non-alcoholic fatty liver disease. J Clin Med (2020) 9:4049. doi: 10.3390/jcm9124049
62. Ramnanan CJ, Edgerton DS, Kraft G, Cherrington AD. Physiologic action of glucagon on liver glucose metabolism. Diabetes Obes Metab (2011) 13 Suppl 1:118–25. doi: 10.1111/j.1463-1326.2011.01454.x
63. Wang Q, Liang X, Wang S. Intra-islet glucagon secretion and action in the regulation of glucose homeostasis. Front Physiol (2013) 3. doi: 10.3389/fphys.2012.00485
64. Abraham MA, Lam TKT. Glucagon action in the brain. Diabetologia (2016) 59:1367–71. doi: 10.1007/s00125-016-3950-3
65. Hieronymus L, Griffin S. Role of amylin in type 1 and type 2 diabetes. Diabetes Educ (2015) 41:47s–56s. doi: 10.1177/0145721715607642
66. Boyle CN, Zheng Y, Lutz TA. Mediators of amylin action in metabolic control. J Clin Med (2022) 11:2207. doi: 10.3390/jcm11082207
67. Moreno P, Acitores A, Gutiérrez-Rojas I, Nuche-Berenguer B, Assar ME, Rodriguez-Mañas L, et al. Amylin effect in extrapancreatic tissues participating in glucose homeostasis, in normal, insulin-resistant and type 2 diabetic state. Peptides (2011) 32:2077–85. doi: 10.1016/j.peptides.2011.09.007
68. Gedulin BR, Jodka CM, Herrmann K, Young AA. Role of endogenous amylin in glucagon secretion and gastric emptying in rats demonstrated with the selective antagonist, AC187. Regul Pept (2006) 137:121–7. doi: 10.1016/j.regpep.2006.06.004
69. Denwood G, Tarasov A, Salehi A, Vergari E, Ramracheya R, Takahashi H, et al. Glucose stimulates somatostatin secretion in pancreatic δ-cells by cAMP-dependent intracellular Ca2+ release. J Gen Physiol (2019) 151(9):1094–115. doi: 10.1085/jgp.201912351
70. Eom YS, Wilson JR, Bernet VJ. Links between thyroid disorders and glucose homeostasis. Diabetes Metab J (2022) 46:239–56. doi: 10.4093/dmj.2022.0013
71. Lutz TA, Meyer U. Amylin at the interface between metabolic and neurodegenerative disorders. Front Neurosci (2015) 9. doi: 10.3389/fnins.2015.00216
72. Pendharkar SA, Drury M, Walia M, Korc M, Petrov MS. Gastrin-releasing peptide and glucose metabolism following pancreatitis. Gastroenterol Res (2017) 10:224–34. doi: 10.14740/gr890w
73. Mathiesen DS, Lund A, Vilsbøll T, Knop FK, Bagger JI. Amylin and calcitonin: potential therapeutic strategies to reduce body weight and liver fat. Front Endocrinol (2021) 11. doi: 10.3389/fendo.2020.617400
74. Li J-X, Cummins CL. Fresh insights into glucocorticoid-induced diabetes mellitus and new therapeutic directions. Nat Rev Endocrinol (2022) 18:540–57. doi: 10.1038/s41574-022-00683-6
75. van der Kooij MA. The impact of chronic stress on energy metabolism. Mol Cell Neurosci (2020) 107:103525. doi: 10.1016/j.mcn.2020.103525
76. Seal SV, Turner JD. The 'Jekyll and hyde' of gluconeogenesis: early life adversity, later life stress, and metabolic disturbances. Int J Mol Sci (2021) 22. doi: 10.3390/ijms22073344
77. Nadkarni P, Chepurny OG, Holz GG. Regulation of glucose homeostasis by GLP-1. Prog Mol Biol Transl Sci (2014) 121:23–65. doi: 10.1016/B978-0-12-800101-1.00002-8
78. Deacon CF. Metabolism of GIP and the contribution of GIP to the glucose-lowering properties of DPP-4 inhibitors. Peptides (2020) 125:170196. doi: 10.1016/j.peptides.2019.170196
79. Grespan E, Giorgino T, Natali A, Ferrannini E, Mari A. Different mechanisms of GIP and GLP-1 action explain their different therapeutic efficacy in type 2 diabetes. Metabolism (2021) 114:154415. doi: 10.1016/j.metabol.2020.154415
80. Poggiogalle E, Jamshed H, Peterson CM. Circadian regulation of glucose, lipid, and energy metabolism in humans. Metabolism (2018) 84:11–27. doi: 10.1016/j.metabol.2017.11.017
81. Radziuk J, Pye S. Diurnal rhythm in endogenous glucose production is a major contributor to fasting hyperglycaemia in type 2 diabetes. Suprachiasmatic deficit limit Cycle behaviour? Diabetologia (2006) 49:1619–28. doi: 10.1007/s00125-006-0273-9
82. Mancilla R, Krook A, Schrauwen P, Hesselink MKC. Diurnal regulation of peripheral glucose metabolism: potential effects of exercise timing. Obesity (2020) 28:S38–45. doi: 10.1002/oby.22811
83. Kessler K, Hornemann S, Petzke KJ, Kemper M, Kramer A, Pfeiffer AFH, et al. The effect of diurnal distribution of carbohydrates and fat on glycaemic control in humans: a randomized controlled trial. Sci Rep (2017) 7:44170. doi: 10.1038/srep44170
84. Peng X, Fan R, Xie L, Shi X, Dong K, Zhang S, et al. Yang y. a growing link between circadian rhythms, type 2 diabetes mellitus and alzheimer's disease. . Int J Mol Sci (2022) 23. doi: 10.3390/ijms23010504
85. Hastings MH, Maywood ES, Brancaccio M. Generation of circadian rhythms in the suprachiasmatic nucleus. Nat Rev Neurosci (2018) 19:453–69. doi: 10.1038/s41583-018-0026-z
86. Ramkisoensing A, Meijer JH. Synchronization of biological clock neurons by light and peripheral feedback systems promotes circadian rhythms and health. Front Neurol (2015) 6. doi: 10.3389/fneur.2015.00128
87. LeGates TA, Fernandez DC, Hattar S. Light as a central modulator of circadian rhythms, sleep and affect. Nat Rev Neurosci (2014) 15:443–54. doi: 10.1038/nrn3743
88. Herzog ED, Hermanstyne T, Smyllie NJ, Hastings MH. Regulating the suprachiasmatic nucleus (SCN) circadian clockwork: interplay between cell-autonomous and circuit-level mechanisms. Cold Spring Harb Perspect Biol (2017) 9. doi: 10.1101/cshperspect.a027706
89. Chan K, Wong FS, Pearson JA. Circadian rhythms and pancreas physiology: a review. Front Endocrinol (2022) 13. doi: 10.3389/fendo.2022.920261
90. Man AWC, Xia N, Li H. Circadian rhythm in adipose tissue: novel antioxidant target for metabolic and cardiovascular diseases. Antioxidants (Basel) (2020) 9. doi: 10.3390/antiox9100968
91. Heyde I, Begemann K, Oster H. Contributions of white and brown adipose tissues to the circadian regulation of energy metabolism. Endocrinology (2021) 162:1–14. doi: 10.1210/endocr/bqab009
92. Aoyama S, Shibata S. The role of circadian rhythms in muscular and osseous physiology and their regulation by nutrition and exercise. Front Neurosci (2017) 11. doi: 10.3389/fnins.2017.00063
93. Lefta M, Wolff G, Esser KA. Circadian rhythms, the molecular clock, and skeletal muscle. Curr Top Dev Biol (2011) 96:231–71. doi: 10.1016/B978-0-12-385940-2.00009-7
94. Pickel L, Lee JH, Maughan H, Shi IQ, Verma N, Yeung C, et al. Circadian rhythms in metabolic organs and the microbiota during acute fasting in mice. Physiol Rep (2022) 10:e15393. doi: 10.14814/phy2.15393
95. Jones JR, Chaturvedi S, Granados-Fuentes D, Herzog ED. Circadian neurons in the paraventricular nucleus entrain and sustain daily rhythms in glucocorticoids. Nat Commun (2021) 12:5763. doi: 10.1038/s41467-021-25959-9
96. Gnocchi D, Bruscalupi G. Circadian rhythms and hormonal homeostasis: pathophysiological implications. Biol (Basel) (2017) 6. doi: 10.3390/biology6010010
97. Farhud D, Aryan Z. Circadian rhythm, lifestyle and health: a narrative review. Iran J Public Health (2018) 47:1068–76.
98. Potter GD, Skene DJ, Arendt J, Cade JE, Grant PJ, Hardie LJ. Circadian rhythm and sleep disruption: causes, metabolic consequences, and countermeasures. Endocr Rev (2016) 37:584–608. doi: 10.1210/er.2016-1083
99. Saran AR, Dave S, Zarrinpar A. Circadian rhythms in the pathogenesis and treatment of fatty liver disease. Gastroenterology (2020) 158:1948–1966.e1941. doi: 10.1053/j.gastro.2020.01.050
100. Pickel L, Sung H-K. Feeding rhythms and the circadian regulation of metabolism. Front Nutr (2020) 7. doi: 10.3389/fnut.2020.00039
101. Narasimamurthy R, Virshup DM. Molecular mechanisms regulating temperature compensation of the circadian clock. Front Neurol (2017) 8. doi: 10.3389/fneur.2017.00161
102. Partch CL, Green CB, Takahashi JS. Molecular architecture of the mammalian circadian clock. Trends Cell Biol (2014) 24:90–9. doi: 10.1016/j.tcb.2013.07.002
103. Yeom M, Lee H, Shin S, Park D, Jung E. PER, a circadian clock component, mediates the suppression of MMP-1 expression in HaCaT keratinocytes by cAMP. Molecules (2018) 23. doi: 10.3390/molecules23040745
104. Menet JS, Pescatore S, Rosbash M. CLOCK:BMAL1 is a pioneer-like transcription factor. Genes Dev (2014) 28:8–13. doi: 10.1101/gad.228536.113
105. Xie Y, Tang Q, Chen G, Xie M, Yu S, Zhao J, et al. New insights into the circadian rhythm and its related diseases. Front Physiol (2019) 10. doi: 10.3389/fphys.2019.00682
106. Mirian M, Hariri A, Yadollahi M, Kohandel M. Circadian and immunity cycle talk in cancer destination: from biological aspects to in silico analysis. Cancers (2022) 14:1578. doi: 10.3390/cancers14061578
107. Cook DN, Kang HS, Jetten AM. Retinoic acid-related orphan receptors (RORs): regulatory functions in immunity, development, circadian rhythm, and metabolism. Nucl Receptor Res (2015) 2. doi: 10.11131/2015/101185
108. Takeda Y, Jothi R, Birault V, Jetten AM. RORγ directly regulates the circadian expression of clock genes and downstream targets in vivo. Nucleic Acids Res (2012) 40:8519–35. doi: 10.1093/nar/gks630
109. Fagiani F, Di Marino D, Romagnoli A, Travelli C, Voltan D, Di Cesare Mannelli L, et al. Molecular regulations of circadian rhythm and implications for physiology and diseases. Signal Transduction Targeted Ther (2022) 7:41. doi: 10.1038/s41392-022-00899-y
110. Corella D, Asensio EM, Coltell O, Sorlí JV, Estruch R, Martínez-González M, et al. CLOCK gene variation is associated with incidence of type-2 diabetes and cardiovascular diseases in type-2 diabetic subjects: dietary modulation in the PREDIMED randomized trial. Cardiovasc Diabetol (2016) 15:4. doi: 10.1186/s12933-015-0327-8
111. Scott EM, Carter AM, Grant PJ. Association between polymorphisms in the clock gene, obesity and the metabolic syndrome in man. Int J Obes (Lond) (2008) 32:658–62. doi: 10.1038/sj.ijo.0803778
112. Garaulet M, Lee YC, Shen J, Parnell LD, Arnett DK, Tsai MY, et al. CLOCK genetic variation and metabolic syndrome risk: modulation by monounsaturated fatty acids. Am J Clin Nutr (2009) 90:1466–75. doi: 10.3945/ajcn.2009.27536
113. Sookoian S, Gemma C, Gianotti TF, Burgueño A, Castaño G, Pirola CJ. Genetic variants of clock transcription factor are associated with individual susceptibility to obesity. Am J Clin Nutr (2008) 87:1606–15. doi: 10.1093/ajcn/87.6.1606
114. Kovac U, Jasper EA, Smith CJ, Baer RJ, Bedell B, Donovan BM, et al. The association of polymorphisms in circadian clock and lipid metabolism genes with 2nd trimester lipid levels and preterm birth. Front Genet (2019) 10. doi: 10.3389/fgene.2019.00540
115. Englund A, Kovanen L, Saarikoski ST, Haukka J, Reunanen A, Aromaa A, et al. NPAS2 and PER2 are linked to risk factors of the metabolic syndrome. J Circadian Rhythms (2009) 7:5. doi: 10.1186/1740-3391-7-5
116. Richards J, Diaz AN, Gumz ML. Clock genes in hypertension: novel insights from rodent models. Blood Press Monit (2014) 19:249–54. doi: 10.1097/MBP.0000000000000060
117. Yang Y-D, Zeng Y, Li J, Zhou J-H, He Q-Y, Zheng C-J, et al. Association of BMAL1 clock gene polymorphisms with fasting glucose in children. Pediatr Res (2023). doi: 10.1038/s41390-023-02467-8
118. Pappa KI, Gazouli M, Anastasiou E, Iliodromiti Z, Antsaklis A, Anagnou NP. The major circadian pacemaker ARNT-like protein-1 (BMAL1) is associated with susceptibility to gestational diabetes mellitus. Diabetes Res Clin Pract (2013) 99:151–7. doi: 10.1016/j.diabres.2012.10.015
119. Vieira E G, Ruano E, Figueroa AL C, Aranda G, Momblan D, Carmona F, et al. Altered clock gene expression in obese visceral adipose tissue is associated with metabolic syndrome. PloS One (2014) 9:e111678. doi: 10.1371/journal.pone.0111678
120. Lyssenko V, Nagorny CL, Erdos MR, Wierup N, Jonsson A, Spégel P, et al. Common variant in MTNR1B associated with increased risk of type 2 diabetes and impaired early insulin secretion. Nat Genet (2009) 41:82–8. doi: 10.1038/ng.288
121. Kim JY, Cheong HS, Park B-L, Baik SH, Park S, Lee SW, et al. Melatonin receptor 1 b polymorphisms associated with the risk of gestational diabetes mellitus. BMC Med Genet (2011) 12:82. doi: 10.1186/1471-2350-12-82
122. Lane JM, Chang AM, Bjonnes AC, Aeschbach D, Anderson C, Cade BE, et al. Impact of common diabetes risk variant in MTNR1B on sleep, circadian, and melatonin physiology. Diabetes (2016) 65:1741–51. doi: 10.2337/db15-0999
123. Barbosa Vieira TK, Jurema da Rocha Leão M, Pereira LX, Alves da Silva LC, Pereira da Paz BB, Santos Ferreira RJ, et al. Correlation between circadian rhythm related genes, type 2 diabetes, and cancer: insights from metanalysis of transcriptomics data. Mol Cell Endocrinol (2021) 526:111214. doi: 10.1016/j.mce.2021.111214
124. Vieira E, Burris TP, Quesada I. Clock genes, pancreatic function, and diabetes. Trends Mol Med (2014) 20:685–93. doi: 10.1016/j.molmed.2014.10.007
125. Sadacca LA, Lamia KA, deLemos AS, Blum B, Weitz CJ. An intrinsic circadian clock of the pancreas is required for normal insulin release and glucose homeostasis in mice. Diabetologia (2011) 54:120–4. doi: 10.1007/s00125-010-1920-8
126. Rakshit K, Thomas AP, Matveyenko AV. Does disruption of circadian rhythms contribute to beta-cell failure in type 2 diabetes? Curr Diabetes Rep (2014) 14:474. doi: 10.1007/s11892-014-0474-4
127. Petrenko V, Saini C, Giovannoni L, Gobet C, Sage D, Unser M, et al. Pancreatic α- and β-cellular clocks have distinct molecular properties and impact on islet hormone secretion and gene expression. Genes Dev (2017) 31:383–98. doi: 10.1101/gad.290379.116
128. Zhen Y, Xi Z, Hu L, Chen Y, Ge L, Wei W, et al. Impacts of circadian gene Period2 knockout on intestinal metabolism and hepatic antioxidant and inflammation state in mice. Oxid Med Cell Longevity (2022) 2022:7896371. doi: 10.1155/2022/7896371
129. Husse J, Hintze SC, Eichele G, Lehnert H, Oster H. Circadian clock genes Per1 and Per2 regulate the response of metabolism-associated transcripts to sleep disruption. PloS One (2012) 7:e52983. doi: 10.1371/journal.pone.0052983
130. Seshadri N, Doucette CA. Circadian regulation of the pancreatic beta cell. Endocrinology (2021) 162:bqab089. doi: 10.1210/endocr/bqab089
131. Qian J, Block GD, Colwell CS, Matveyenko AV. Consequences of exposure to light at night on the pancreatic islet circadian clock and function in rats. Diabetes (2013) 62:3469–78. doi: 10.2337/db12-1543
132. Rakshit K, Qian J, Gaonkar KS, Dhawan S, Colwell CS, Matveyenko AV. Postnatal ontogenesis of the islet circadian clock plays a contributory role in β-cell maturation process. Diabetes (2018) 67:911–22. doi: 10.2337/db17-0850
133. Stenvers DJ, Scheer F, Schrauwen P, la Fleur SE, Kalsbeek A. Circadian clocks and insulin resistance. Nat Rev Endocrinol (2019) 15:75–89. doi: 10.1038/s41574-018-0122-1
134. Oosterman JE, Wopereis S, Kalsbeek A. The circadian clock, shift work, and tissue-specific insulin resistance. Endocrinology (2020) 161:1–11. doi: 10.1210/endocr/bqaa180
135. García-Costela M, Escudero-Feliú J, Puentes-Pardo JD, San Juán SM, Morales-Santana S, Ríos-Arrabal S, et al. Circadian genes as therapeutic targets in pancreatic cancer. Front Endocrinol (Lausanne) (2020) 11:638. doi: 10.3389/fendo.2020.00638
136. Mukherji A, Bailey SM, Staels B, Baumert TF. The circadian clock and liver function in health and disease. J Hepatol (2019) 71:200–11. doi: 10.1016/j.jhep.2019.03.020
137. Tahara Y, Shibata S. Circadian rhythms of liver physiology and disease: experimental and clinical evidence. Nat Rev Gastroenterol Hepatol (2016) 13:217–26. doi: 10.1038/nrgastro.2016.8
138. Ferrell JM, Chiang JYL. Circadian rhythms in liver metabolism and disease. Acta Pharm Sin B (2015) 5:113–22. doi: 10.1016/j.apsb.2015.01.003
139. Shi D, Chen J, Wang J, Yao J, Huang Y, Zhang G, et al. Circadian clock genes in the metabolism of non-alcoholic fatty liver disease. Front Physiol (2019) 10. doi: 10.3389/fphys.2019.00423
140. Ramanathan C, Xu H, Khan SK, Shen Y, Gitis PJ, Welsh DK, et al. Cell type-specific functions of period genes revealed by novel adipocyte and hepatocyte circadian clock models. PloS Genet (2014) 10:e1004244. doi: 10.1371/journal.pgen.1004244
141. Areshidze DA, Kozlova MA. Morphofunctional state and circadian rhythms of the liver of female rats under the influence of chronic alcohol intoxication and constant lighting. Int J Mol Sci (2022) 23. doi: 10.3390/ijms231810744
142. Guan D, Xiong Y, Trinh TM, Xiao Y, Hu W, Jiang C, et al. The hepatocyte clock and feeding control chronophysiology of multiple liver cell types. Science (2020) 369:1388–94. doi: 10.1126/science.aba8984
143. Vollmers C, Gill S, DiTacchio L, Pulivarthy SR, Le HD, Panda S. Time of feeding and the intrinsic circadian clock drive rhythms in hepatic gene expression. Proc Natl Acad Sci (2009) 106:21453–8. doi: 10.1073/pnas.0909591106
144. Greco CM, Koronowski KB, Smith JG, Shi J, Kunderfranco P, Carriero R, et al. Integration of feeding behavior by the liver circadian clock reveals network dependency of metabolic rhythms. Sci Adv (2021) 7:eabi7828. doi: 10.1126/sciadv.abi7828
145. Rey G, Cesbron F, Rougemont J, Reinke H, Brunner M, Naef F. Genome-wide and phase-specific DNA-binding rhythms of BMAL1 control circadian output functions in mouse liver. PloS Biol (2011) 9:e1000595. doi: 10.1371/journal.pbio.1000595
146. Jiang H, Garcia V, Yanum JA, Lee J, Dai G. Circadian clock core component Bmal1 dictates cell cycle rhythm of proliferating hepatocytes during liver regeneration. Am J Physiol Gastrointest Liver Physiol (2021) 321:G389–g399. doi: 10.1152/ajpgi.00204.2021
147. Gatfield D, Schibler U. Circadian glucose homeostasis requires compensatory interference between brain and liver clocks. Proc Natl Acad Sci USA (2008) 105:14753–4. doi: 10.1073/pnas.0807861105
148. Jacobi D, Liu S, Burkewitz K, Kory N, Knudsen NH, Alexander RK, et al. Hepatic Bmal1 regulates rhythmic mitochondrial dynamics and promotes metabolic fitness. Cell Metab (2015) 22:709–20. doi: 10.1016/j.cmet.2015.08.006
149. Ruberto AA, Gréchez-Cassiau A, Guérin S, Martin L, Revel JS, Mehiri M, et al. KLF10 integrates circadian timing and sugar signaling to coordinate hepatic metabolism. Elife (2021) 10. doi: 10.7554/eLife.65574
150. Guillaumond F, Gréchez-Cassiau A, Subramaniam M, Brangolo S, Peteri-Brünback B, Staels B, et al. Kruppel-like factor KLF10 is a link between the circadian clock and metabolism in liver. Mol Cell Biol (2010) 30:3059–70. doi: 10.1128/MCB.01141-09
151. Chen L, Yang G. PPARs integrate the mammalian clock and energy metabolism. PPAR Res (2014) 2014:653017. doi: 10.1155/2014/653017
152. Hatori M, Panda S. CRY links the circadian clock and CREB-mediated gluconeogenesis. Cell Res (2010) 20:1285–8. doi: 10.1038/cr.2010.152
153. Weger BD, Gobet C, David FPA, Atger F, Martin E, Phillips NE, et al. Systematic analysis of differential rhythmic liver gene expression mediated by the circadian clock and feeding rhythms. Proc Natl Acad Sci (2021) 118:e2015803118. doi: 10.1073/pnas.2015803118
154. Kelu JJ, Pipalia TG, Hughes SM. Circadian regulation of muscle growth independent of locomotor activity. Proc Natl Acad Sci (2020) 117:31208–18. doi: 10.1073/pnas.2012450117
155. Leong I. Muscle circadian clock regulates lipid storage. Nat Rev Endocrinol (2018) 14:563–3. doi: 10.1038/s41574-018-0089-y
156. Schiaffino S, Blaauw B, Dyar KA. The functional significance of the skeletal muscle clock: lessons from Bmal1 knockout models. Skeletal Muscle (2016) 6:33. doi: 10.1186/s13395-016-0107-5
157. Li Y, Chen W. Skeletal muscle clock and metabolism. Biol Rhythm Res (2022) 53:580–91. doi: 10.1080/09291016.2020.1816037
158. Dyar KA, Ciciliot S, Wright LE, Biensø RS, Tagliazucchi GM, Patel VR, et al. Muscle insulin sensitivity and glucose metabolism are controlled by the intrinsic muscle clock. Mol Metab (2014) 3:29–41. doi: 10.1016/j.molmet.2013.10.005
159. Sylow L, Kleinert M, Richter EA, Jensen TE. Exercise-stimulated glucose uptake [[/amp]]mdash; regulation and implications for glycaemic control. Nat Rev Endocrinol (2017) 13:133–48. doi: 10.1038/nrendo.2016.162
160. Merz KE, Thurmond DC. Role of skeletal muscle in insulin resistance and glucose uptake. Compr Physiol (2020) 10:785–809. doi: 10.1002/cphy.c190029
161. Ehlen JC, Brager AJ, Baggs J, Pinckney L, Gray CL, DeBruyne JP, et al. Bmal1 function in skeletal muscle regulates sleep. eLife (2017) 6:e26557. doi: 10.7554/eLife.26557
162. Harfmann BD, Schroder EA, Kachman MT, Hodge BA, Zhang X, Esser KA. Muscle-specific loss of Bmal1 leads to disrupted tissue glucose metabolism and systemic glucose homeostasis. Skelet Muscle (2016) 6:12. doi: 10.1186/s13395-016-0082-x
163. Liu J, Zhou B, Yan M, Huang R, Wang Y, He Z, et al. CLOCK and BMAL1 regulate muscle insulin sensitivity via SIRT1 in Male mice. Endocrinology (2016) 157:2259–69. doi: 10.1210/en.2015-2027
164. Basse AL, Dalbram E, Larsson L, Gerhart-Hines Z, Zierath JR, Treebak JT. Skeletal muscle insulin sensitivity show circadian rhythmicity which is independent of exercise training status. Front Physiol (2018) 9:1198. doi: 10.3389/fphys.2018.01198
165. Chavez JA, Roach WG, Keller SR, Lane WS, Lienhard GE. Inhibition of GLUT4 translocation by Tbc1d1, a rab GTPase-activating protein abundant in skeletal muscle, is partially relieved by AMP-activated protein kinase activation*. J Biol Chem (2008) 283:9187–95. doi: 10.1074/jbc.M708934200
166. Feneberg R, Lemmer B. Circadian rhythm of glucose uptake in cultures of skeletal muscle cells and adipocytes in wistar-Kyoto, wistar, goto-kakizaki, and spontaneously hypertensive rats. Chronobiol Int (2004) 21:521–38. doi: 10.1081/CBI-200026958
167. Neeland IJ, Turer AT, Ayers CR, Powell-Wiley TM, Vega GL, Farzaneh-Far R, et al. Dysfunctional adiposity and the risk of prediabetes and type 2 diabetes in obese adults. Jama (2012) 308:1150–9. doi: 10.1001/2012.jama.11132
168. Saponaro C, Gaggini M, Carli F, Gastaldelli A. The subtle balance between lipolysis and lipogenesis: a critical point in metabolic homeostasis. Nutrients (2015) 7:9453–74. doi: 10.3390/nu7115475
169. Cignarelli A, Genchi VA, Perrini S, Natalicchio A, Laviola L, Giorgino F. Insulin and insulin receptors in adipose tissue development. Int J Mol Sci (2019) 20. doi: 10.3390/ijms20030759
170. Gooley JJ, Chua EC-P. Diurnal regulation of lipid metabolism and applications of circadian lipidomics. J Genet Genomics (2014) 41:231–50. doi: 10.1016/j.jgg.2014.04.001
171. Gooley JJ. Circadian regulation of lipid metabolism. Proc Nutr Soc (2016) 75:440–50. doi: 10.1017/S0029665116000288
172. Otway DT, Frost G, Johnston JD. Circadian rhythmicity in murine pre-adipocyte and adipocyte cells. Chronobiol Int (2009) 26:1340–54. doi: 10.3109/07420520903412368
173. Pan X, Mota S, Zhang B. Circadian clock regulation on lipid metabolism and metabolic diseases. Adv Exp Med Biol (2020) 1276:53–66. doi: 10.1007/978-981-15-6082-8_5
174. Jouffe C, Weger BD, Martin E, Atger F, Weger M, Gobet C, et al. Disruption of the circadian clock component BMAL1 elicits an endocrine adaption impacting on insulin sensitivity and liver disease. Proc Natl Acad Sci (2022) 119:e2200083119. doi: 10.1073/pnas.2200083119
175. Lu HL, Wang HW, Wen Y, Zhang MX, Lin HH. Roles of adipocyte derived hormone adiponectin and resistin in insulin resistance of type 2 diabetes. World J Gastroenterol (2006) 12:1747–51. doi: 10.3748/wjg.v12.i11.1747
176. Biondi G, Marrano N, Borrelli A, Rella M, Palma G, Calderoni I, et al. Adipose tissue secretion pattern influences β-cell wellness in the transition from obesity to type 2 diabetes. Int J Mol Sci (2022) 23:5522. doi: 10.3390/ijms23105522
177. Rancourt RC, Ott R, Ziska T, Schellong K, Melchior K, Henrich W, et al. Visceral adipose tissue inflammatory factors (TNF-alpha, SOCS3) in gestational diabetes (GDM): epigenetics as a clue in GDM pathophysiology. Int J Mol Sci (2020) 21:479. doi: 10.3390/ijms21020479
178. Zatterale F, Longo M, Naderi J, Raciti GA, Desiderio A, Miele C, et al. Chronic adipose tissue inflammation linking obesity to insulin resistance and type 2 diabetes. Front Physiol (2019) 10:1607. doi: 10.3389/fphys.2019.01607
179. Xiong X, Lin Y, Lee J, Paul A, Yechoor V, Figueiro M, et al. Chronic circadian shift leads to adipose tissue inflammation and fibrosis. Mol Cell Endocrinol (2021) 521:111110. doi: 10.1016/j.mce.2020.111110
180. Nieto-Vazquez I, Fernández-Veledo S, Krämer DK, Vila-Bedmar R, Garcia-Guerra L, Lorenzo M. Insulin resistance associated to obesity: the link TNF-alpha. Arch Physiol Biochem (2008) 114:183–94. doi: 10.1080/13813450802181047
181. Guilherme A, Virbasius JV, Puri V, Czech MP. Adipocyte dysfunctions linking obesity to insulin resistance and type 2 diabetes. Nat Rev Mol Cell Biol (2008) 9:367–77. doi: 10.1038/nrm2391
182. Blume C, Garbazza C, Spitschan M. Effects of light on human circadian rhythms, sleep and mood. Somnologie (Berl) (2019) 23:147–56. doi: 10.1007/s11818-019-00215-x
183. Fan X, Chen D, Wang Y, Tan Y, Zhao H, Zeng J, et al. Light intensity alters the effects of light-induced circadian disruption on glucose and lipid metabolism in mice. Am J Physiology-Endocrinology Metab (2022) 322:E1–9. doi: 10.1152/ajpendo.00025.2021
184. Chamorro R, Jouffe C, Oster H, Uhlenhaut NH, Meyhöfer SM. When should I eat: a circadian view on food intake and metabolic regulation. Acta Physiol (Oxf) (2023) 237(3):e13936. doi: 10.1111/apha.13936
185. Henry CJ, Kaur B, Quek RYC. Chrononutrition in the management of diabetes. Nutr Diabetes (2020) 10:6. doi: 10.1038/s41387-020-0109-6
186. Hatori M, Vollmers C, Zarrinpar A, DiTacchio L, Bushong EA, Gill S, et al. Time-restricted feeding without reducing caloric intake prevents metabolic diseases in mice fed a high-fat diet. Cell Metab (2012) 15:848–60. doi: 10.1016/j.cmet.2012.04.019
187. Chaix A, Lin T, Le HD, Chang MW, Panda S. Time-restricted feeding prevents obesity and metabolic syndrome in mice lacking a circadian clock. Cell Metab (2019) 29:303–319.e304. doi: 10.1016/j.cmet.2018.08.004
188. Goni L, Sun D, Heianza Y, Wang T, Huang T, Martínez JA, et al. A circadian rhythm-related MTNR1B genetic variant modulates the effect of weight-loss diets on changes in adiposity and body composition: the POUNDS lost trial. Eur J Nutr (2019) 58:1381–9. doi: 10.1007/s00394-018-1660-y
189. Gaulton KJ, Ferreira T, Lee Y, Raimondo A, Mägi R, Reschen ME, et al. Genetic fine mapping and genomic annotation defines causal mechanisms at type 2 diabetes susceptibility loci. Nat Genet (2015) 47:1415–25. doi: 10.1038/ng.3437
190. Lopez-Minguez J, Saxena R, Bandín C, Scheer FA, Garaulet M. Late dinner impairs glucose tolerance in MTNR1B risk allele carriers: a randomized, cross-over study. Clin Nutr (2018) 37:1133–40. doi: 10.1016/j.clnu.2017.04.003
191. Hutchison AT, Regmi P, Manoogian ENC, Fleischer JG, Wittert GA, Panda S, et al. Time-restricted feeding improves glucose tolerance in men at risk for type 2 diabetes: a randomized crossover trial. Obes (Silver Spring) (2019) 27:724–32. doi: 10.1002/oby.22449
192. Quist JS, Jensen MM, Clemmensen KKB, Pedersen H, Bjerre N, Størling J, et al. Protocol for a single-centre, parallel-group, randomised, controlled, superiority trial on the effects of time-restricted eating on body weight, behaviour and metabolism in individuals at high risk of type 2 diabetes: the REStricted eating time (RESET) study. BMJ Open (2020) 10:e037166. doi: 10.1136/bmjopen-2020-037166
193. Świątkiewicz I, Mila-Kierzenkowska C, Szewczyk-Golec K, Nuszkiewicz J, Wróblewska J, Rajewski P, et al. Pilot clinical trial of time-restricted eating in patients with metabolic syndrome. Nutrients (2021) 13. doi: 10.3390/nu13020346
194. Gan Y, Yang C, Tong X, Sun H, Cong Y, Yin X, et al. Shift work and diabetes mellitus: a meta-analysis of observational studies. Occup Environ Med (2015) 72:72–8. doi: 10.1136/oemed-2014-102150
195. Knutsson A, Kempe A. Shift work and diabetes–a systematic review. Chronobiol Int (2014) 31:1146–51. doi: 10.3109/07420528.2014.957308
196. Lee J, Moulik M, Fang Z, Saha P, Zou F, Xu Y, et al. Bmal1 and β-cell clock are required for adaptation to circadian disruption, and their loss of function leads to oxidative stress-induced β-cell failure in mice. Mol Cell Biol (2013) 33:2327–38. doi: 10.1128/MCB.01421-12
197. Amiama-Roig A, Verdugo-Sivianes EM, Carnero A, Blanco J-R. Chronotherapy: circadian rhythms and their influence in cancer therapy. Cancers (2022) 14:5071. doi: 10.3390/cancers14205071
198. Fauler G, Abletshauser C, Erwa W, Löser R, Witschital K, März W. Time-of-intake (morning versus evening) of extended-release fluvastatin in hyperlipemic patients is without influence on the pharmacodynamics (mevalonic acid excretion) and pharmacokinetics. Int J Clin Pharmacol Ther (2007) 45:328–34. doi: 10.5414/CPP45328
199. Raskin P, Cincotta AH. Bromocriptine-QR therapy for the management of type 2 diabetes mellitus: developmental basis and therapeutic profile summary. Expert Rev Endocrinol Metab (2016) 11:113–48. doi: 10.1586/17446651.2016.1131119
200. Ezrokhi M, Zhang Y, Luo S, Cincotta AH. Time-of-Day-Dependent effects of bromocriptine to ameliorate vascular pathology and metabolic syndrome in SHR rats held on high fat diet. Int J Mol Sci (2021) 22. doi: 10.3390/ijms22116142
201. Framnes-DeBoer SN, Bakke E, Yalamanchili S, Peterson H, Sandoval DA, Seeley RJ, et al. Bromocriptine improves glucose tolerance independent of circadian timing, prolactin, or the melanocortin-4 receptor. Am J Physiol Endocrinol Metab (2020) 318:E62–e71. doi: 10.1152/ajpendo.00325.2019
202. Sanchez-Rangel E, Inzucchi SE. Metformin: clinical use in type 2 diabetes. Diabetologia (2017) 60:1586–93. doi: 10.1007/s00125-017-4336-x
203. Aroda VR, Ratner RE. Metformin and type 2 diabetes prevention. Diabetes Spectr (2018) 31:336–42. doi: 10.2337/ds18-0020
204. Henriksson E, Huber A-L, Soto EK, Kriebs A, Vaughan ME, Duglan D, et al. The liver circadian clock modulates biochemical and physiological responses to metformin. J Biol Rhythms (2017) 32:345–58. doi: 10.1177/0748730417710348
205. Alex A, Luo Q, Mathew D, Di R, Bhatwadekar AD. Metformin corrects abnormal circadian rhythm and Kir4. 1 Channels Diabetes. Invest Ophthalmol Vis Sci (2020) 61:46. doi: 10.1167/iovs.61.6.46
206. Barnea M, Haviv L, Gutman R, Chapnik N, Madar Z, Froy O. Metformin affects the circadian clock and metabolic rhythms in a tissue-specific manner. Biochim Biophys Acta (2012) 1822:1796–806. doi: 10.1016/j.bbadis.2012.08.005
207. Zisapel N. New perspectives on the role of melatonin in human sleep, circadian rhythms and their regulation. Br J Pharmacol (2018) 175:3190–9. doi: 10.1111/bph.14116
208. Espino J, Pariente JA, Rodríguez AB. Role of melatonin on diabetes-related metabolic disorders. World J Diabetes (2011) 2:82–91. doi: 10.4239/wjd.v2.i6.82
209. Arendt J. Melatonin: countering chaotic time cues. Front Endocrinol (2019) 10. doi: 10.3389/fendo.2019.00391
210. Schwartz MD, Wotus C, Liu T, Friesen WO, Borjigin J, Oda GA, et al. Dissociation of circadian and light inhibition of melatonin release through forced desynchronization in the rat. Proc Natl Acad Sci (2009) 106:17540–5. doi: 10.1073/pnas.0906382106
211. Costes S, Boss M, Thomas AP, Matveyenko AV. Activation of melatonin signaling promotes β-cell survival and function. Mol Endocrinol (2015) 29:682–92. doi: 10.1210/me.2014-1293
212. Peschke E, Bähr I, Mühlbauer E. Melatonin and pancreatic islets: interrelationships between melatonin, insulin and glucagon. Int J Mol Sci (2013) 14:6981–7015. doi: 10.3390/ijms14046981
213. Lee YH, Jung HS, Kwon MJ, Jang JE, Kim TN, Lee SH, et al. Melatonin protects INS-1 pancreatic β-cells from apoptosis and senescence induced by glucotoxicity and glucolipotoxicity. Islets (2020) 12:87–98. doi: 10.1080/19382014.2020.1783162
214. McMullan CJ, Schernhammer ES, Rimm EB, Hu FB, Forman JP. Melatonin secretion and the incidence of type 2 diabetes. Jama (2013) 309:1388–96. doi: 10.1001/jama.2013.2710
215. Pourhanifeh MH, Hosseinzadeh A, Dehdashtian E, Hemati K, Mehrzadi S. Melatonin: new insights on its therapeutic properties in diabetic complications. Diabetol Metab Syndrome (2020) 12:30. doi: 10.1186/s13098-020-00537-z
216. Patel R, Parmar N, Pramanik Palit S, Rathwa N, Ramachandran AV, Begum R. Diabetes mellitus and melatonin: where are we? Biochimie (2022) 202:2–14. doi: 10.1016/j.biochi.2022.01.001
217. Forrestel AC, Miedlich SU, Yurcheshen M, Wittlin SD, Sellix MT. Chronomedicine and type 2 diabetes: shining some light on melatonin. Diabetologia (2017) 60:808–22. doi: 10.1007/s00125-016-4175-1
218. Thomas AP, Hoang J, Vongbunyong K, Nguyen A, Rakshit K, Matveyenko AV. Administration of melatonin and metformin prevents deleterious effects of circadian disruption and obesity in Male rats. Endocrinology (2016) 157:4720–31. doi: 10.1210/en.2016-1309
219. Rasmussen ES, Takahashi JS, Green CB. Time to target the circadian clock for drug discovery. Trends Biochem Sci (2022) 47:745–58. doi: 10.1016/j.tibs.2022.04.009
220. Ruan W, Yuan X, Eltzschig HK. Circadian rhythm as a therapeutic target. Nat Rev Drug Discovery (2021) 20:287–307. doi: 10.1038/s41573-020-00109-w
221. Lebovitz HE. Thiazolidinediones: the forgotten diabetes medications. Curr Diabetes Rep (2019) 19:151. doi: 10.1007/s11892-019-1270-y
222. Missbach M, Jagher B, Sigg I, Nayeri S, Carlberg C, Wiesenberg I. Thiazolidine diones, specific ligands of the nuclear receptor retinoid z Receptor/Retinoid acid receptor-related orphan receptor α with potent antiarthritic activity*. J Biol Chem (1996) 271:13515–22. doi: 10.1074/jbc.271.23.13515
223. Shinozaki A, Misawa K, Ikeda Y, Haraguchi A, Kamagata M, Tahara Y, et al. Potent effects of flavonoid nobiletin on amplitude, period, and phase of the circadian clock rhythm in PER2::LUCIFERASE mouse embryonic fibroblasts. PloS One (2017) 12:e0170904. doi: 10.1371/journal.pone.0170904
224. Parkar NA, Bhatt LK, Addepalli V. Efficacy of nobiletin, a citrus flavonoid, in the treatment of the cardiovascular dysfunction of diabetes in rats. Food Funct (2016) 7:3121–9. doi: 10.1039/C6FO00294C
225. Lee YS, Cha BY, Saito K, Yamakawa H, Choi SS, Yamaguchi K, et al. Nobiletin improves hyperglycemia and insulin resistance in obese diabetic ob/ob mice. Biochem Pharmacol (2010) 79:1674–83. doi: 10.1016/j.bcp.2010.01.034
226. Wang S, Li F, Lin Y, Wu B. Targeting REV-ERBα for therapeutic purposes: promises and challenges. Theranostics (2020) 10:4168–82. doi: 10.7150/thno.43834
227. Zhou Z, Lin Y, Gao L, Yang Z, Wang S, Wu B. Circadian pharmacological effects of berberine on chronic colitis in mice: role of the clock component rev-erbα. Biochem Pharmacol (2020) 172:113773. doi: 10.1016/j.bcp.2019.113773
228. Guo J, Chen H, Zhang X, Lou W, Zhang P, Qiu Y, et al. The effect of berberine on metabolic profiles in type 2 diabetic patients: a systematic review and meta-analysis of randomized controlled trials. Oxid Med Cell Longev (2021) 2021:2074610. doi: 10.1155/2021/2074610
229. Hirota T, Lee JW, St John PC, Sawa M, Iwaisako K, Noguchi T, et al. Identification of small molecule activators of cryptochrome. Science (2012) 337:1094–7. doi: 10.1126/science.1223710
230. Solovev IA, Shaposhnikov MV, Moskalev AA. Chronobiotics KL001 and KS15 extend lifespan and modify circadian rhythms of drosophila melanogaster. Clocks Sleep (2021) 3:429–41. doi: 10.3390/clockssleep3030030
231. Humphries PS, Bersot R, Kincaid J, Mabery E, McCluskie K, Park T, et al. Carbazole-containing amides and ureas: discovery of cryptochrome modulators as antihyperglycemic agents. Bioorganic Medicinal Chem Lett (2018) 28:293–7. doi: 10.1016/j.bmcl.2017.12.051
232. Stéphanou A, Fanchon E, Innominato PF, Ballesta A. Systems biology, systems medicine, systems pharmacology: the what and the why. Acta Biotheor (2018) 66:345–65. doi: 10.1007/s10441-018-9330-2
233. Segú-Vergés C, Coma M, Kessel C, Smeets S, Foell D, Aldea A. Application of systems biology-based in silico tools to optimize treatment strategy identification in still’s disease. Arthritis Res Ther (2021) 23:126. doi: 10.1186/s13075-021-02507-w
234. Azer K, Kaddi CD, Barrett JS, Bai JPF, McQuade ST, Merrill NJ, et al. History and future perspectives on the discipline of quantitative systems pharmacology modeling and its applications. Front Physiol (2021) 12. doi: 10.3389/fphys.2021.637999
235. Aguayo-Orozco A, Audouze K, Brunak S, Taboureau O. In silico systems pharmacology to assess drug's therapeutic and toxic effects. Curr Pharm Des (2016) 22:6895–902. doi: 10.2174/1381612822666160907093215
236. Jeon M, Kim S, Park S, Lee H, Kang J. In silico drug combination discovery for personalized cancer therapy. BMC Syst Biol (2018) 12:16. doi: 10.1186/s12918-018-0546-1
237. Teng D, Gong Y, Wu Z, Li W, Tang Y, Liu G. In silico prediction of potential drug combinations for type 2 diabetes mellitus by an integrated network and transcriptome analysis. ChemMedChem (2022) 17:e202100620. doi: 10.1002/cmdc.202100620
238. Sidorov P, Naulaerts S, Ariey-Bonnet J, Pasquier E, Ballester PJ. Predicting synergism of cancer drug combinations using NCI-ALMANAC data. Front Chem (2019) 7. doi: 10.3389/fchem.2019.00509
239. Zamami Y, Hamano H, Niimura T, Aizawa F, Yagi K, Goda M, et al. Drug-repositioning approaches based on medical and life science databases. Front Pharmacol (2021) 12. doi: 10.3389/fphar.2021.752174
240. Rodrigues L, Bento Cunha R, Vassilevskaia T, Viveiros M, Cunha C. Drug repurposing for COVID-19: a review and a novel strategy to identify new targets and potential drug candidates. Molecules (2022) 27. doi: 10.3390/molecules27092723
Keywords: diabetes mellitus, circadian rhythm, chronotherapy, metabolic syndrome, sleep disorder, lifestyle
Citation: Hariri A, Mirian M, Zarrabi A, Kohandel M, Amini-Pozveh M, Aref AR, Tabatabaee A, Prabhakar PK and Sivakumar PM (2023) The circadian rhythm: an influential soundtrack in the diabetes story. Front. Endocrinol. 14:1156757. doi: 10.3389/fendo.2023.1156757
Received: 01 February 2023; Accepted: 03 May 2023;
Published: 27 June 2023.
Edited by:
Pål Olof Westermark, Leibniz Institute for Farm Animal Biology (FBN), GermanyReviewed by:
Jill Kanaley, University of Missouri, United StatesKam Ghaedi, University of La Verne, United States
Ekaterina Gubareva, N.N. Petrov National Medical Research Center of Oncology, Russia
Copyright © 2023 Hariri, Mirian, Zarrabi, Kohandel, Amini-Pozveh, Aref, Tabatabaee, Prabhakar and Sivakumar. This is an open-access article distributed under the terms of the Creative Commons Attribution License (CC BY). The use, distribution or reproduction in other forums is permitted, provided the original author(s) and the copyright owner(s) are credited and that the original publication in this journal is cited, in accordance with accepted academic practice. No use, distribution or reproduction is permitted which does not comply with these terms.
*Correspondence: Mina Mirian, TWluYS5taXJpYW5AcGhhcm0ubXVpLmFjLmly; Ponnurengam Malliappan Sivakumar, c2l2YWt1bWFyQGR1eXRhbi5lZHUudm4=
†ORCID: Mina Mirian, orcid.org/0000-0002-9322-2707orcid.org/0000-0002-9322-2707
Ponnurengam Malliappan Sivakumar, orcid.org/0000-0002-5880-9596orcid.org/0000-0002-5880-9596