- 1Laboratory of Fish Physiology and Genomics, UR1037, Institut National de Recherche pour l'Agriculture, l'Alimentation et l'Environnement (INRAE), Rennes, France
- 2Departament de Biologia Cel·lular, Fisiologia i Immunologia, Facultat de Biologia, Universitat de Barcelona, Barcelona, Spain
Control of tissue metabolism and growth involves interactions between organs, tissues, and cell types, mediated by cytokines or direct communication through cellular exchanges. Indeed, over the past decades, many peptides produced by adipose tissue, skeletal muscle and bone named adipokines, myokines and osteokines respectively, have been identified in mammals playing key roles in organ/tissue development and function. Some of them are released into the circulation acting as classical hormones, but they can also act locally showing autocrine/paracrine effects. In recent years, some of these cytokines have been identified in fish models of biomedical or agronomic interest. In this review, we will present their state of the art focusing on local actions and inter-tissue effects. Adipokines reported in fish adipocytes include adiponectin and leptin among others. We will focus on their structure characteristics, gene expression, receptors, and effects, in the adipose tissue itself, mainly regulating cell differentiation and metabolism, but in muscle and bone as target tissues too. Moreover, lipid metabolites, named lipokines, can also act as signaling molecules regulating metabolic homeostasis. Regarding myokines, the best documented in fish are myostatin and the insulin-like growth factors. This review summarizes their characteristics at a molecular level, and describes both, autocrine effects and interactions with adipose tissue and bone. Nonetheless, our understanding of the functions and mechanisms of action of many of these cytokines is still largely incomplete in fish, especially concerning osteokines (i.e., osteocalcin), whose potential cross talking roles remain to be elucidated. Furthermore, by using selective breeding or genetic tools, the formation of a specific tissue can be altered, highlighting the consequences on other tissues, and allowing the identification of communication signals. The specific effects of identified cytokines validated through in vitro models or in vivo trials will be described. Moreover, future scientific fronts (i.e., exosomes) and tools (i.e., co-cultures, organoids) for a better understanding of inter-organ crosstalk in fish will also be presented. As a final consideration, further identification of molecules involved in inter-tissue communication will open new avenues of knowledge in the control of fish homeostasis, as well as possible strategies to be applied in aquaculture or biomedicine.
1 Introduction
Our understanding of the relationship and communication between skeletal muscle, bone and adipose tissue has undergone a major resurgence in the past decade, with an increasing number of published papers on the subject, especially in mammalian models (1). Nonetheless, a growing interest in fish species exists considering they are the largest and most diverse group of vertebrates. Nowadays, skeletal muscle, bone and adipose tissue are no longer viewed from the simple mechanical and metabolic perspective. These tissues can produce a high variety of molecules with autocrine, paracrine, and endocrine functions that mediate a complicated network of cross talking to communicate each other their specific physiological status. Moreover, lipid metabolites, either de novo synthesized or derived from dietary fats, named lipokines, could also act as signaling molecules between organs to coordinate energy substrates’ use (2).
Some of the earliest and current evidence for tissues crosstalk comes out from the literature involving the role of cytokines in different human pathologies and during physical activity (3, 4). The use of zebrafish (Danio rerio) as an advantageous animal model in biomedicine, together with the increasing interest in the integrative knowledge of endocrine control of growth and metabolism in cultured fish species, has focused the attention to the crosstalk between bone, muscle, and adipose tissues in piscine models. Nevertheless, the information in fish inter-tissue communication is still limited compared with that of mammals.
Based on this background, the aim of this review is to give an overview about the most up-to-date knowledge in the field of adipokines, myokines and osteokines in fish, and their possible roles in tissues crosstalk.
2 Adipokines
The main function of vertebrate adipose tissue is the storage of triglycerides under conditions of excess of calories and their release during periods of energy demand. In addition, adipose tissue is now recognized as a complex endocrine organ. Adipose tissue is primarily composed of adipocytes, as well as pre-adipocytes, stem cells, endothelial cells, or macrophages, which contribute to the release of metabolites, lipids, and bioactive peptides, so-called adipokines. These secreted factors can regulate the tissue locally, but also many other tissues, thus controlling the whole organism’s physiology. In this section, we will focus on the best-known adipokines in teleost fish, keeping in mind their described effects in the adipose tissue itself, but also at a systemic level, including the regulation of different biological processes especially in muscle and bone as target organs (Figure 1; Table 1). Nevertheless, it is important to note that our understanding of the function and mechanisms of action of many of these adipokines is still largely incomplete in fish.
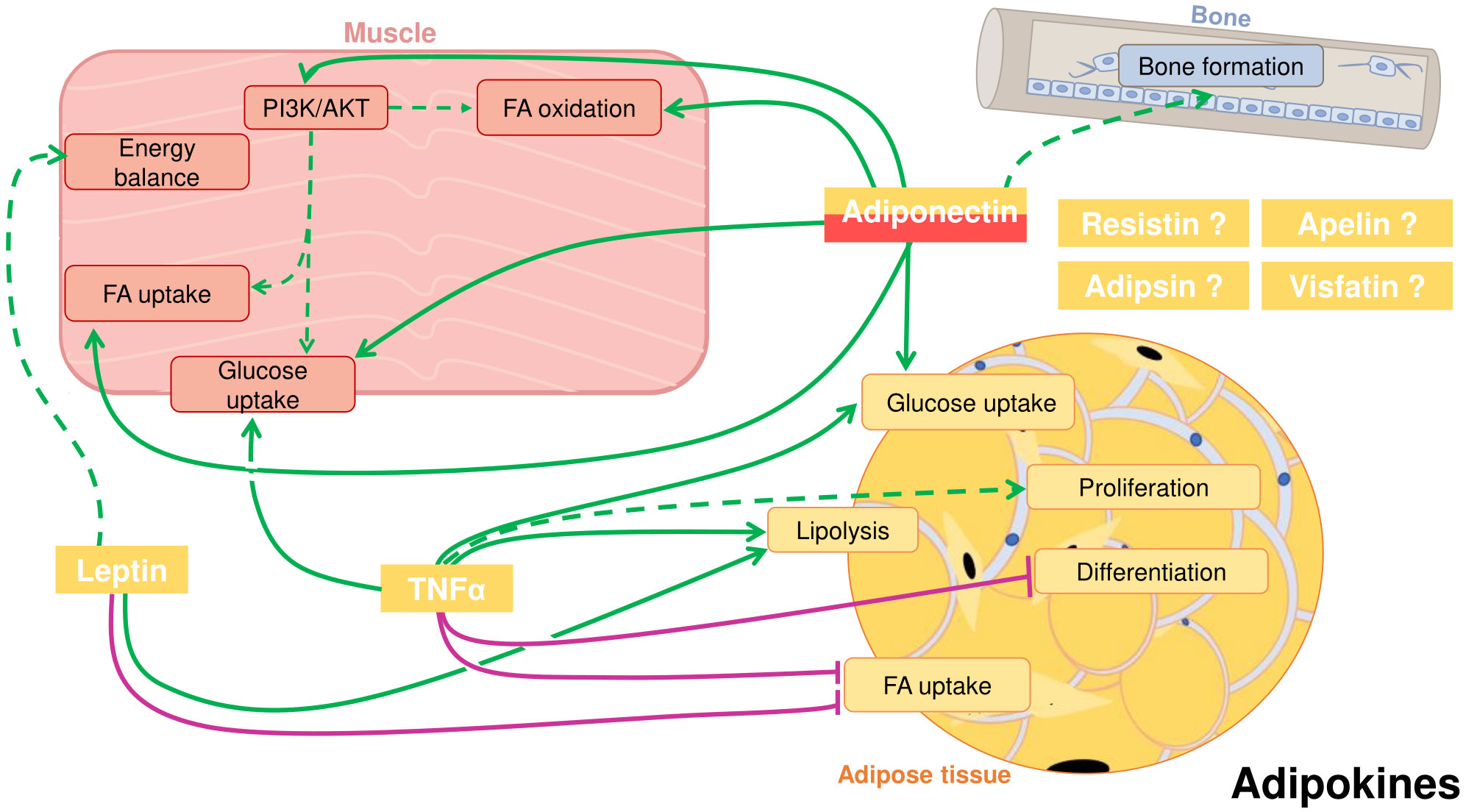
Figure 1 Crosstalk between adipose tissue, muscle, and bone in fish through cytokines secreted by the adipose tissue, also known as adipokines. Adiponectin, that can also be secreted by skeletal muscle, can positively influence in muscle fatty acid (FA) and glucose uptake, FA oxidation and activate the PI3K/AKT pathway. Studies suggest a role for adiponectin inducing bone formation. Adiponectin has also autocrine functions in the adipose tissue, where it can promote glucose uptake. Leptin in muscle can presumably influence energy balance. In the adipose tissue, leptin induces lipolysis and inhibits FA uptake. Tumor necrosis factor α (TNFα) can stimulate glucose uptake in muscle and has an important autocrine role in adipose tissue. There, TNFα stimulates glucose uptake and lipolysis, and probably adipocyte proliferation, whereas it inhibits differentiation and FA uptake. Other potential adipokines are resistin, apelin, adipsin and visfatin. Positive proved effects: green solid line; Positive suggested effects; green dotted line; Negative proved effects: purple solid line.
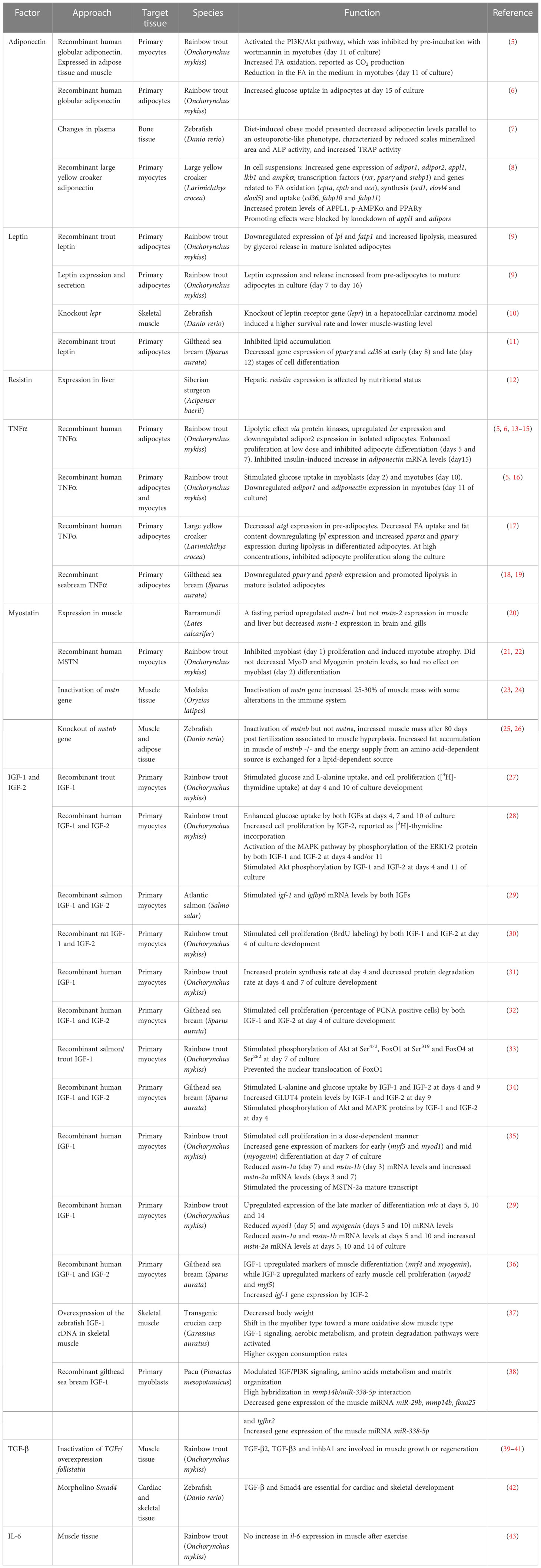
Table 1 List of reported adipokines and myokines in fish with summarized function in the crosstalk between tissues.
2.1 Adiponectin
Adiponectin is a cytokine-like peptide produced primarily by adipose tissue in mammals, which plays a crucial role in lipid and glucose metabolism regulation. The first identification of adiponectin in fish was reported in zebrafish (44). Since then, this adipokine has been identified in other fish species, such as rainbow trout (Oncorhynchus mykiss), ayu (Plecoglossus altivelis), large yellow croaker (Larimichthys crocea) and common carp (Cyprinus carpio) (8, 45–47). The main regions of mammalian adiponectin have been analyzed in fish, and the globular domain, which is involved in receptor binding, is the most conserved one. However, although mammalian adiponectin can be found in monomeric or multimeric forms, until now there is no evidence on multimer formation in teleost species (reviewed by Ji et al. (8)).
Yet, the adiponectin gene might have been lost in some fish species throughout evolution since it was not found in some published genome databases. This is the case of Nile tilapia (Oreochromis niloticus), black carp (Mylopharyngodon piceus), Japanese puffer (Takifugu rubripes), medaka (Oryzias latipes), three-spined stickleback (Gasterosteus aculeatus) and green spotted puffer (Tetraodon nigroviridis) (8). On the other hand, there are two isoforms of adiponectin genes, namely adipoqa and adipoqb, in cyprinid fishes, including grass carp (Ctenopharyngodon idella) (48) and zebrafish (44). For perciform fishes, only one adiponectin gene has been reported in the literature.
The highest expression level of adiponectin in ayu is found in the adipose tissue, as reported in mammals (49). In zebrafish, rainbow trout, large yellow croaker, common carp, and grass carp, the expression in the muscle is higher than that in the adipose tissue (5, 8, 44, 45, 47, 48), but still remains to be evaluated whether this peptide could be also considered a myokine in these species. adiponectin is also expressed in other tissues, such as brain, liver, kidney or heart with variable levels depending on the species (8). In fish, there are almost no studies on adiponectin plasma levels (7), neither on peptide secretion, nor on peptide release into the circulation, which makes difficult to understand its role in inter-organ communication.
Regarding adiponectin receptors, and similarly to mammals, some fish species have two forms (AdipoR1 and AdipoR2), while zebrafish, grass carp and common carp have two isoforms of AdipoR1 besides AdipoR2 (44, 47, 48). The homologies with mammalian sequences are between 70 and 80%. AdipoR1 is mainly located in muscle and AdipoR2 in liver of humans or mice, but a wider distribution is observed in fish. In particular, in zebrafish and rainbow trout, they are widely distributed and can be considered as ubiquitous (5, 44). AdipoRs are also widespread in other teleosts, although the pattern of expression varies among species, suggesting this scenario possible inter-species differences in the function of adiponectin in the communication between tissues.
Concerning the factors regulating gene expression, insulin was able to stimulate adiponectin, and to decrease adipor1 mRNA levels in rainbow trout cultured adipocytes (6) and in vivo in adipose tissue (5), in agreement with reports in various mammalian models (46, 50–52).
In mammals, adiponectin stimulates fatty acid oxidation, decreases plasma triglycerides and improves glucose metabolism by increasing insulin sensitivity (53, 54). Considering that many of its beneficial effects in mammals occur through the interaction with the skeletal muscle, it is not surprising that some physiological studies have focused on the role of adiponectin in fish muscle, specifically in trout cultured myocytes (5). In this in vitro model, no changes in glucose or fatty acids uptake were observed after a short period of incubation with adiponectin. Nevertheless, after 2 days of treatment, the reduced presence of fatty acids in the culture medium, and the increase in intracellular triglycerides, suggested an increase in fatty acid uptake. These effects were not accompanied by an increase in fatty acid transporters’ expression in trout, but in large yellow croaker myocytes, adiponectin upregulated the gene expression of the fatty acid transporters cd36, fabp10 and fabp11 (8).
Adiponectin also increased fatty acid oxidation in trout myocytes measured as CO2 production (5), one of the main actions of this adipokine in mammalian models, that helps to maintain lipid homeostasis and insulin sensitivity. Moreover, in trout myocytes, adiponectin activated the PI3K/AKT pathway but not the signaling through p44/42 mitogen-activated protein kinases (MAPK), similarly as it occurs in mouse myocytes (55). Indeed, in that study, wortmannin blocked the stimulatory effect of adiponectin demonstrating the specificity of the pathway. Activation of PI3K/AKT has been associated to increases in glucose and fatty acid uptake and beta-oxidation in mammals (56). The relevance of this molecular pathway in fatty acid oxidation in fish remains to be elucidated, but it appears that the regulation of muscle lipid metabolism by adiponectin and its mechanisms of action would be both conserved.
With regards to the effects of adiponectin regulating bone mass, controversial results were reported in mammals. From the available literature, it seems that adiponectin directly influences skeletal health. To identify the mechanisms that underlie the activity of adiponectin in bone, many studies in vitro and in mice models over-expressing or lacking adiponectin have been carried out. Although the in vitro data showed an osteogenic role of adiponectin, supported by pro-osteoblastic and anti-osteoclastic effects, data from transgenic mice or human studies failed to demonstrate unambiguously a beneficial effect of adiponectin on bone development (57). The inconsistency of the results may be partly due to the variation among experimental systems, the use of different structural forms and the complex nature of adiponectin signaling, which involves multiple direct and indirect mechanisms (58).
In fish, limited information exists on the role of adiponectin in bone. Zebrafish fed a high fat diet showed decreased plasma levels of adiponectin accompanied by a reduction of mineralized areas in scales and an alteration of growth tissue markers, such as a decrease of alkaline phosphatase and an increase in tartrate-resistant acid phosphatase activity respect to control fish, suggesting a positive effect of adiponectin on bone growth (7). Nevertheless, as far as we know, no other studies about the communication of adipose tissue and bone mediated by adiponectin have been addressed until now in fish.
Adiponectin has also local effects on the adipose tissue, stimulating glucose uptake and increasing lipid content in mammalian adipocytes (59). In fish, adiponectin increased glucose uptake in cultured rainbow trout adipocytes without modification of AKT or TOR phosphorylation (6), suggesting other signaling pathways involved. Nevertheless, due to the high levels of adiponectin expression in trout muscle, it is unknown whether an autocrine effect could exist in vivo, or whether adiponectin from muscle could contribute to regulate adipose tissue glucose metabolism in an endocrine or paracrine way. The future development of specific methods to measure circulating adiponectin levels and tissue secretion would help to better understand its role in fish.
Finally, it is remarkable that adiponectin and its receptors are modulated by nutritional status. For example, feed restriction affects their expression in a different way in muscle and adipose tissue in rainbow trout, Atlantic salmon (Salmo salar) and zebrafish, with also variations between species, underlining its key role in the communication between tissues (5, 44, 60). Nevertheless, going into detail about these changes is beyond the scope of this review.
Although the information regarding fish adiponectin is limited to very few species, taken together the reviewed results, a general conservation of structure and functions of adiponectin could be suggested at least bearing in mind its effects in muscle, bone, and adipose tissue metabolism. However, specific features in teleost adiponectin system should be considered to fully understand its physiological role.
2.2 Leptin
Leptin is a 16 kDa pleiotropic cytokine with an important role in food intake control and energy homeostasis in vertebrates. Fish leptin was first identified in 2005, when the leptin gene ortholog was cloned in pufferfish (61). Since then, leptin sequences were described in many fish species (reviewed by Blanco and Soengas (62)): common carp (63), zebrafish (64), medaka (65), Atlantic salmon (66), striped bass (Morone saxatilis) (67), yellow catfish, (Pelteobagrus fulvidraco) (68), mandarin fish (Siniperca chuatsi) (69), goldfish (Carassius auratus) (70), European and Japanese eel (Anguilla anguilla and Anguilla japonica, respectively) (71) and, more recently, in Northern snakehead (Channa argus) (72), among others.
Teleosts share very low primary sequence identity (~13-25%) with human leptin (73). A great number of fish species have two copies of this gene, named differently in various species (lep-a/I/1 and lep-b/II/2), due to the whole-genome duplication (WGD) event that occurred during the evolution of the teleost lineage (73). For instance, two copies are found in tongue sole (Cynoglossus semilaevis), Nile tilapia, orange-spotted grouper (Epinephelus coioides), chub mackerel (Scomber japonicus), Northern snakehead, zebrafish and medaka (62). Both leptin sequences show variable identity and even duplicate leptin genes described within fish species may be very different in primary amino acid sequence conservation (e.g., zebrafish) (64). lep-a/I/1 is the predominant form expressed in many of the teleost fish analyzed (74). On the contrary, other fish species (e.g., mandarin fish, pufferfish) retained only one leptin gene, but the existence of a second paralog cannot be discarded.
In mammals, leptin is an adipostat, is produced by, and circulates in proportion to the amount of white adipose tissue (75), but this scenario has not been confirmed yet in fish. In contrast to mammals, fish leptin shows differential distribution patterns, and is commonly highly expressed in the liver (62). Only in some species, the expression of leptin in adipose tissue is relatively high (76–78). Besides, its expression is found in some other tissues such as brain, gonads, kidney, gill, muscle and spleen, but the transcriptional patterns are very different between species (69, 79, 80). leptin paralogs also showed different transcriptional features within the same species, with lepA being more abundant in the liver whereas lepB in the gonads, thus indicating divergent roles (65, 76).
The development of different methods for measuring leptin in fish has resulted in abundant literature regarding plasma levels and their regulation, especially by nutritional status. In many fish species, and in contrast with mammals, circulating leptin was significantly increased after different periods of food deprivation, and subsequently decreased after refeeding in rainbow trout (68, 81, 82), or fine flounder Paralichthys adspersus (83). On the contrary, circulating leptin levels in other species were decreased after food deprivation (e.g., burbot, Lota lota) (84). Moreover, leptin expression in liver increased after refeeding in goldfish (70), common carp (63) or the cyprinid Labeo rohita (85). In addition, other studies revealed that leptin levels were not affected by fasting, but displayed peri-prandial changes in pacu (Piaractus mesopotamicus) (80). These findings suggested that leptin is clearly involved in energy homeostasis regulation but might exhibit different functional properties depending on the fish species. However, in all the species tested, leptin acted as an anorexigenic peptide; thus, increased circulating levels of leptin during fasting, reflected a possible strategy to avoid wasting energy foraging in those situations (62, 74).
Secretion studies are crucial to know which tissues might contribute to circulating leptin. Measurable levels of leptin A were found by homologous radioimmunoassay (81) in culture media from both, rainbow trout pre-adipocytes and mature adipocytes, with significantly higher levels in the latter (9). As far as we know, this study is unique demonstrating that leptin is produced and released by adipocytes and supporting that adipose tissue might contribute to circulating leptin levels in rainbow trout and that this can act as a hormone.
Nevertheless, as indicated in fish, leptin is highest expressed in liver. It is not surprising that leptin, as a regulator of energy expenditure, is controlled at least in part by glucose and key elements of the endocrine stress axis. In this sense, recently, Mankiewicz et al. (86), using tilapia (Oreochromis mossambicus) freshly isolated hepatocytes’ incubations and a homologous leptin A ELISA, showed that its secretion is modulated by high levels of glucose. In the same study, an in vivo injection of epinephrine stimulated a rapid rise in blood glucose, which was followed by a 4-fold increase in hepatic lepA mRNA and plasma levels. Interestingly, specific fatty acids could modulate fish leptin secretion since leptin mRNA expression was downregulated by saturated fatty acids, and upregulated by monounsaturated and long chain polyunsaturated fatty acids in rainbow trout liver slices (87).
Indeed, it would be very helpful to have more information on leptin secretion by hepatocytes and adipocytes and about its regulation especially in species where leptin is highly expressed in liver and adipose tissue. Furthermore, the co-culture of different types of fish cells, such as adipocytes-myocytes, may help to understand the interactions of their secretomes and the crosstalk between these tissues.
The physiological actions of leptin are mediated by a glycoprotein consisting of a single-membrane-spanning receptor (LepR), that belongs to the class-I cytokine receptor family (88). In fish, an orthologous gene for the mammalian lepr was identified in several species (62), typically present as a single ortholog; however, duplicate lepr paralogs have been identified in a few species, including Atlantic salmon (89), European eel (71) or rainbow trout (86). Furthermore, in some species more than one isoform is present, as it also occurs in mammals, generated by alternative mRNA splicing and/or proteolytic processing of the protein products (88). The mammalian long isoform, or leprb, is the only one with clearly demonstrated signaling capability (90). There is few information regarding the functionality of these isoforms, but the salmon LepR (long form) includes all functionally important domains conserved among vertebrate LepRs.
High expression of lepr is commonly found in the brain, pituitary, and gonads of many fish species; however, there are species-specific differences in its tissue distribution. In this sense, the muscle, head kidney, pituitary and pancreas are the major expressing tissues in Nile tilapia (78) and, the muscle, skin, gill, brain and eye in medaka (65). Nevertheless, the physiological role of these receptors is still unclear.
In vertebrates, adipocytes regulate energy metabolism of the whole body, including muscle and bone, indirectly through interaction of leptin with sophisticated brain circuits that maintain energy levels by affecting food intake and energy expenditure (91). In mammals, leptin increases sympathetic nervous system tone (92) and, through its interaction with the melanocortin system, activates thyrotropin-releasing hormone to increase thyroid hormone signaling and, thus, energy expenditure (93–95). As mentioned before, most of the studies of leptin administration in many fish species support an anorexigenic action and there is abundant literature regarding its role as inhibitor of food intake, which has been reviewed extensively (62, 73) and is out of the scope of this review. However, its role as a regulator of energy expenditure has been less explored in fish.
Regarding lipid homeostasis, autocrine effects of leptin on adipose tissue of rainbow trout have been suggested using recombinant trout leptin (96). Indeed, leptin increased lipolysis measured as glycerol release in mature freshly isolated adipocytes of this salmonid. Furthermore, leptin significantly suppressed the fatty acid transporter fatp1 expression, suggesting a decrease in fatty acid uptake and storage, but did not affect the expression of any of the lipogenesis or β-oxidation-related genes studied (9). More recently, Basto-Silva et al. (11), reported that leptin inhibits lipid accumulation, significantly reducing the peroxisome proliferator-activated receptor gamma (pparγ) and fatty acid transporter cd36 gene expression, both in early differentiating and mature adipocytes of gilthead sea bream (Sparus aurata).
Some other findings come from studies with lepr mutants. Indeed, hyperphagia in lepr-deficient medaka led to a higher growth rate at the post-juvenile stage, but not in adults, although adult lepr mutants possessed large depots of visceral fat, unlike the wild type fish (97). On the other hand, lepr knockout zebrafish did not develop obesity (98), which is in marked contrast to mammals, particularly mice (ob and db models) and barely affected metabolism and energy allocation (99). lepr knockout trout exhibited a hyperphagic phenotype, and increased energy stores in muscle as observed in mammalian models (100). Recently, it was reported that leptin induces muscle wasting in a zebrafish kras-driven hepatocellular carcinoma model (10). By using lepr knockout fish, it was found that these animals had a higher survival rate and significantly lower muscle-wasting level after tumor induction than the tumor-induced fish in the wild-type background. These results, besides having interest in cancer cachexia, also demonstrated direct effects of leptin in fish muscle physiology.
In mammals, leptin has intense effects on skeletal muscle fatty acid metabolism, resulting in an increase in the capacity of this tissue to oxidize fatty acids and reduce triacylglycerides stores. Therefore, the development of leptin resistance in skeletal muscle, characteristic of obesity, may lead to insulin resistance in that tissue by allowing the accumulation of intramuscular lipids and disruption of the insulin signaling pathway (101). This situation can be ameliorated by different lifestyle factors such as aerobic training and diet, establishing a homeostatic crosstalk. Although not being an obesity fish model, studies in energetically divergent rainbow trout lines selected for low (lean line, LL) and high (fat line, FL) muscle adiposity, revealed impaired central leptin signaling system in the FL fish, probably linked to high muscle fat, since no differences in appetite regulation and feed intake between the two rainbow trout lines were found (102).
Some studies suggested indirectly the regulation of muscle metabolism by leptin in fish. The increase in circulating leptin under food deprivation might trigger the activation of the AMP-activated protein kinase (AMPK) in the skeletal muscle of fine flounder and contribute to the negative effects on anabolic processes (83). Another indirect evidence of leptin actions on fish muscle came from studies on lepr expression, which is modulated by nutritional status. The two lepr paralogs identified in rainbow trout are differentially expressed across tissues under catabolic conditions, showing that during fasting, leptin is likely acting to promote energy mobilization in the muscle through lepra1 and in the liver through lepra2 (86).
Clearly, more studies are required to learn about leptin crosstalk. The use of fish models in which primary culture of myogenic cells is well established (27, 30) may provide further insights for understanding the mechanisms by which leptin regulates metabolism and growth in fish skeletal muscle.
Regarding bone as a target tissue of leptin, different studies with mammalian models have shown that it plays multiple crucial roles in skeletal growth and metabolism through both, central and peripheral pathways, and that it might be involved in many human bone diseases (103). In humans, leptin is likely to exert a positive effect on bone mass, and contribute to the balance between adiposity and bone density (104). Interestingly, administration of a high fat diet alters energy metabolism generating an osteoporosis-like phenotype in adult zebrafish scales (7), but no information regarding the possible role of leptin regulating these processes has been reported to date. In this sense, it would be very interesting to study the role of leptin regulating the plasticity of cells derived from gilthead sea bream vertebra bone, which can be differentiated into both, osteoblast and adipocyte phenotypes (105, 106), as this will shed more light into the possible role of leptin as a mediator of the communication between adipose tissue and bone in fish.
2.3 Resistin
Resistin is a peptide that was first identified as a factor produced exclusively by adipocytes (107). Indeed, in mice, resistin mRNA expression is abundant only in adipose tissue (107) while in humans, it is expressed in white adipose tissue but also in other tissues. In fact, peripheral blood mononuclear cells, macrophages, and bone marrow cells are the primary source of circulating human resistin (108). Resistin is widely found in other species and, for example, it is highly expressed in the lung in porcine, goat and yak (12).
The increase in plasma resistin concentration impairs insulin sensitivity and decreases glucose tolerance in mice, and plays a significant role in human obesity-induced insulin resistance (109). It also shows pleiotropic effects and could be involved in physiological and pathological processes of bone/rheumatological disorders. The resistin receptor is unknown but several proteins could do such function: toll-like receptor 4, decorin, orphan receptor-1, insulin-like growth factor receptor, and adenylyl cyclase-associated protein 1 (110, 111).
In 2015, Hu and co-workers analyzed available genome sequences of the resistin-like gene family of diverse vertebrates (112). Genes encoding resistin-like peptides were found in Actinistia (coelacanth, a lobe-finned fish), but not in fish from the Actinopterygii, Chondrichthyes, or Agnatha. More recently, a resistin full-length cDNA has been obtained, with an open reading frame encoding 106 amino acids in Siberian sturgeon (Acipenser baerii) (12), showing low identity with other vertebrates, except when compared with the sterlet (Acipenser ruthenus). However, the resistin C-terminal is well conserved among species, suggesting that this region may be important to its physiological roles.
Gene expression of resistin was very high in the liver of Siberian sturgeon in comparison with other tissues, as described previously also for leptin. Besides adipose tissue, the liver is an important organ of lipid accumulation in some species, and it is essential in metabolic homeostasis, suggesting that resistin, as other classical adipokines, may have a role in metabolism regulation. In fact, in Siberian sturgeon, resistin mRNA expression in the liver decreased after fasting and increased sharply after refeeding, confirming it is affected by nutritional status (12) supporting this hypothesis. Nonetheless, data is limited to this study; consequently, further studies are needed to investigate the role of resistin as a hormone involved in tissue crosstalk in fish.
2.4 Tumor necrosis factor
TNF is a cytokine belonging to the “TNF ligand superfamily” (113, 114) that plays crucial roles in regulating immune functions and metabolism in vertebrates. In mammals has two isoforms, TNFα and TNFβ, while in fish there is only one more similar in structure and organization to TNFα (115). Only a single copy of tfnα gene was found in mammals, otherwise, various copies of the same gene were found in fish, the number of which depends on the species (116–122). TNFα was first identified in the Japanese flounder, Paralichthys olivaceus (123), and posteriorly in zebrafish, rainbow trout, gilthead sea bream and common carp (120, 124, 125). TNF family signature is well conserved among mammals and fish (119, 126).
TNFα was first described as a cytokine secreted by macrophages regulating inflammation, apoptosis, cell proliferation and stimulation of the immune system (115).. TNFα is also secreted by adipose tissue or skeletal muscle (113, 127), and it is classically included in the list of mammalian adipokines. It was in 1999 when Bulló-Bonet et al. (128) suggested that TNFα can be produced by human adipocytes themselves and, more recently, tnfα expression has also been detected in fish cultured adipocytes (6).
TNFα receptors, TNFR1 and TNFR2, have been described for several fish species (129). The functions of TNFR1 are, among others, related to apoptosis or cellular death while TNFR2 is involved in the promotion of cell survival (129). tnfr1 is expressed in many tissues, but tnfr2 is more tissue specific, limited to immune, endothelial, microglia and nerve cells (129).
Either the mature adipocytes or the stromal vascular fraction of adipose tissue, which includes pre-adipocytes mixed with macrophages, can act as endocrine cells secreting adipokines including TNFα. In mammals, obese individuals produce more TNFα, in part due to the high number of infiltrated macrophages in the adipose tissue (6, 130, 131). TNFα has a clear paracrine and/or autocrine role within adipose tissue (17). In mammals and fish, TFNα promotes adipocyte lipolysis, modulates lipogenesis, and glucose transport and inhibits pre-adipocytes proliferation (129, 132). It can also interact with other cytokines and vice versa (115). In fish, as in mammals, besides modulating fat metabolism, it has been proved that TFNα can act as a pro-inflammatory, apoptotic or organ regenerator regulator (6, 133–136). In almost all fish studies conducted and described in this review, mammalian recombinant TNFs have been used (5, 13, 137), although few studies have reported the use of recombinant trout TNF1 and TNF2 in muscle or other cell models (16, 129, 138).
Most of the studies regarding the effects of TNFα as a crosstalk mediator in fish have been limited to its autocrine effect in adipose tissue. This cytokine induced lipolysis in rainbow trout (13), gilthead sea bream (18, 19) or large yellow croaker (17) in experiments conducted both in vitro and in vivo. In mammalian models, TNFα lipolytic action is usually mediated by modulation of hormone-sensitive lipase (HSL) and adipose triglyceride lipase (ATGL). Silencing of atgl expression in 3T3-L1 pre-adipocytes almost completely abolished TNFα-induced glycerol release, while TNFα-induced lipolysis under the same conditions was only partially decreased upon reduction of hsl expression (139). In contrast with these observations, TNFα decreased the expression of the key lipolytic enzyme atgl in large yellow croaker pre-adipocytes (17) although other mammalian studies showed a similar action (140–142). The expression level of atgl was increased in liver and muscle by lipopolysaccharide (LPS)-induced TNFα in blunt snout bream, but not in adipose tissue (143). Altogether, these data suggest that TNFα might modulate adipose tissue lipolysis at several levels and with differences between fish species. These variations might be explained in terms of differences in the doses and origin of the peptides used in the experiments.
Regarding mechanisms of action, TNFα can activate MAPK in human adipocytes, leading to a lipolysis increase though ERK1/2 and p38 kinase activation (144, 145). Accordingly, Albalat et al. (13) demonstrated that these protein kinases are partially involved in the lipolytic effects of human TNFα in rainbow trout adipocytes. On the other hand, TNFα increased PPARα during activation of adipocyte lipolysis in large yellow croaker, in agreement with the role of this transcription factor in fatty acid catabolism (17, 146, 147). The relevance of PPARγ is not so clear as TNFα downregulated pparγ expression, promoting lipolysis in gilthead sea bream adipocytes (19) as in mammals (148), but during TNFα-induced lipolysis, pparγ expression levels increased in large yellow croaker (17). With regards to other possible transcription factors involved, liver X receptor (lxr) was upregulated by TNFα in rainbow trout isolated adipocytes, in parallel to the pro-lipolytic actions of this factor (14). Nevertheless, this mechanism of action is not so clear in TNFα-induced lipolysis in gilthead sea bream isolated adipocytes (19), highlighting again the complexity of the signaling pathways of TNFα activating adipocyte lipolysis in fish.
Furthermore, TNFα can cause a decrease in adiposity by other mechanisms besides inducing lipolysis. In order to decrease the amount of triglycerides in adipocytes, TNFα minimizes fatty acid uptake reducing substrate availability, but also inhibiting the esterification of free fatty acids in mammals (132) or decreasing the lipogenic enzyme fatty acid synthase (fas) expression (149). Among fish studies, TNFα did not change the expression of fas, pparα or pparγ in large yellow croaker pre-adipocytes but decreased the expression of lipoprotein lipase (lpl) (17), in agreement with its role reducing fatty acid uptake. Supporting this fact, in rainbow trout, TNFα induced by LPS administration, decreased fatp1 mRNA expression in adipose tissue, as well as in isolated adipocytes (5).
Moreover, in mammals it is proved that TNFα induces insulin-resistance (12, 150), by impairing insulin-stimulated glucose uptake through the inhibition of insulin signaling at the insulin receptor substrate level (151, 152), but on the other hand, TNFα increases basal glucose uptake in adipose tissue (153). From studies in rainbow trout adipocytes, it was concluded that stimulation of basal glucose uptake by TNFα seems to be conserved from fish to mammals. Meanwhile, the interactions between insulin and TNFα are ambiguous in fish, namely: although an inhibitory effect compared to insulin treatment was observed on AKT and TOR phosphorylation when TNFα was added together with insulin in rainbow trout adipocytes, it was not reflected on glucose uptake (6). On the other hand, recombinant trout TNFα directly stimulated glucose uptake in rainbow trout myoblasts and myotubes providing evidence for a potential regulatory role of TNFα in skeletal muscle metabolism (16).
Concerning adipogenesis, a negative effect of TNFα has been well documented in several in vitro models (12, 154, 155), including fish pre-adipocytes and adipocytes (6, 15). In mammals, TNFα is both an inhibitor of adipocyte differentiation and a suppressor of some early stage genes responsible of pre-adipocyte conversion (156); although in rats or mice, low concentrations of TNFα have a promoting effect on adipocyte proliferation (155, 157). In large yellow croaker, high concentrations of TNFα inhibited adipocyte proliferation but low concentrations had no effect (17). Like in mammals, this cytokine slightly enhanced rainbow trout adipocyte proliferation, only at a low dose, and inhibited cell differentiation, as indicated by a decrease in glycerol-3-phosphate dehydrogenase activity (15). Additionally, lpl, proved to be an early marker of adipocyte differentiation, together with pparγ, were transcriptionally downregulated by TNFα in fish as in mammals (15, 17). Furthermore, in the primary rainbow trout adipocytes culture, incubation with TNFα downregulated the expression of fatp1 (5), which can be understood not only as a decrease in cell differentiation but also as a contribution to reduce fatty acid uptake and thus, the lipid content of the adipocyte. Moreover, TNFα could cause a decrease in adiposity in mammals inducing adipocyte death (132, 158, 159), but as far as we know, there is no data available on the cytotoxic effect of TNFα in fish adipose tissue. Overall, differences in the response of adipocyte cells to TNFα in fish seem to be related to dosage and species specificity as it occurs in some mammalian models as well.
Interactions between TNFα and other adipokines (i.e., adiponectin) seem to have specific features in fish. Indeed, it has been reported that adiponectin suppresses the expression and release of TNFα from human and 3T3-L1 adipocytes (160, 161). In rainbow trout adipocytes, TNFα increased the mRNA levels of adipor1 but neither adiponectin nor TNFα modulated each other gene’s expression, although TNFα inhibited the insulin-induced increase in adiponectin mRNA levels (6). Therefore, the reciprocal suppressive effect of both adipokines previously reported in mammals is not clearly present in fish. When tested in vivo, the absence of a mammalian-type regulation between adiponectin and TNFα was confirmed, since TNFα injections failed to regulate the expression of the adiponectin system in rainbow trout adipose tissue (5).
To sum up, TNFα functions seem to be conserved between fish and mammals, it is secreted by fish adipocytes and could act in the adipose tissue in an autocrine way, and in other tissues including skeletal muscle. Therefore, it is likely that a crosstalk exists among all the tissues in which TNFα is involved, but further studies are needed to definitively prove that.
2.5 Other adipokines
Another regulatory peptide produced by adipocytes to be considered is apelin. In mammals, apelin and its receptor, that belongs to the type 1 angiotensin G protein-coupled receptor family (162), are expressed in adipose tissue but also widely distributed in other peripheral tissues in goldfish (162), Ya-fish (Schizothorax prenanti) (163), and others (164). Apelin has multiple roles, according to the numerous locations where it is found. These include the regulation of cardiovascular functions, metabolism, feeding behavior and energy expenditure. In adipose tissue and muscle, apelin regulates AMPK and promotes glucose utilization. It was hypothesized that apelin contributes to obesity disorders by developing adipose tissue mass via angiogenesis or pre-adipocytes proliferation. Nevertheless, contradictory results among diverse studies in mammals have been described and the precise role of apelin needs further investigation (162, 163, 165, 166). In fish, very little is known regarding apelin. Apparently, the only role of apelin demonstrated in fish was as orexigenic factor (162, 163). Nonetheless, other studies have hypothesized that apelin might be involved in the regulation of many physiological functions in fish due to its expression in various tissues, as in mammals.
Concerning other adipokines, adipsin and visfatin are two recognized ones found in mammals and fish. Adipsin has been described in olive flounder (167) and visfatin in silver Prussian carp (Carassius auratus gibelio) (168). Both were identified in Atlantic salmon and expressed in differentiating adipocytes derived from the adipose-derived stromo-vascular fraction (169). These studies agreed with mammalian literature showing that, apart from being expressed in different tissues, they are found mainly in adipose tissue. In mammalian models, those cytokines are implicated in immunological and inflammatory functions and, could be also involved in the control of lipid metabolism and adipogenesis. The basis established in the literature about those two adipokines could help discovering in the future the true roles, pathways, and cross talking in which adipsin and visfatin are involved in fish.
3 Lipokines
Studies of mammalian adipose tissue secretome focused at first on adipokines (leptin and adiponectin, mainly); however, adipose-derived lipids or lipokines emerged as new endocrine factors (170). Provided by intracellular pathways of fatty acid metabolism, circulating in blood plasma and acting as mediators between adipose and non-adipose tissues (pancreas, liver or muscle), lipokines appeared as regulators of metabolic homeostasis, stress or inflammation (171). Among these lipokines, lysophosphatidic acid, palmitoleate, fatty acyl esters of hydroxy fatty acids, oxylipins and N-acyl amino acids, can act as autocrine or paracrine signals (114). Some lipokines increase fatty acids uptake in skeletal muscle (172), whereas others secreted by myocytes and stimulated through exercise (i.e., β-aminoisobutyric acid (173);), act upon white adipocytes and hepatocytes. Interestingly, lipidome atlases now appear to characterize adipose tissues: subcutaneous versus visceral depots (174).
On fish so far, few lipidome data are available in relation to crosstalk between organs, maybe due to a key fat storage in liver in some species, or only the existence of white fat adipose tissue, whereas in mammals, brown adipose tissue is involved as well (175, 176). To our knowledge, lipidome reports rather appeared in relation to fish environment or diet, focusing more on lipid metabolism than on lipokines (177, 178). For instance, Dreier et al. (177) highlighted the impact of environmental factors on lipid metabolism referring to studies on largemouth bass (Micropterus salmoides), zebrafish embryos or adults, rainbow trout juveniles or Mediterranean cyprinidae. Therein, relationships between pollution and changes in transcripts related to lipid metabolism were evidenced, as well as chemical exposure and fish lipid composition, or polluted water and phosphatidyl-cholines/-ethanolamines alterations within skeletal muscle. Among changes, the cholesterol pathway appeared as the one exhibiting the highest number of altered metabolites in the study on zebrafish embryos, whereas limited phospholipid lipase activities affected brain tissues, and neurobehavioral responses, in the report on young trout. On the other side, Jin et al. (178) reported how diet composition affects the lipid uptake (gut), processing (liver) and deposition (muscle) in Atlantic salmon in freshwater and seawater stages.
4 Myokines
Skeletal muscle constitutes the largest part of the body weight, particularly in fish, where it accounts for up to 60-70% and is therefore the largest organ. Since the beginning of the 21st century, it is known that skeletal muscle secretes proteins called myokines, which influence the function of other tissues and organs (4, 179) through autocrine, paracrine and endocrine signaling. Although several hundreds of myokines have been identified in mammals from decades, few are known in fish. These myokines and their reported effects in the muscle itself, bone and adipose tissue in fish are summarized in Figure 2 and Table 1.
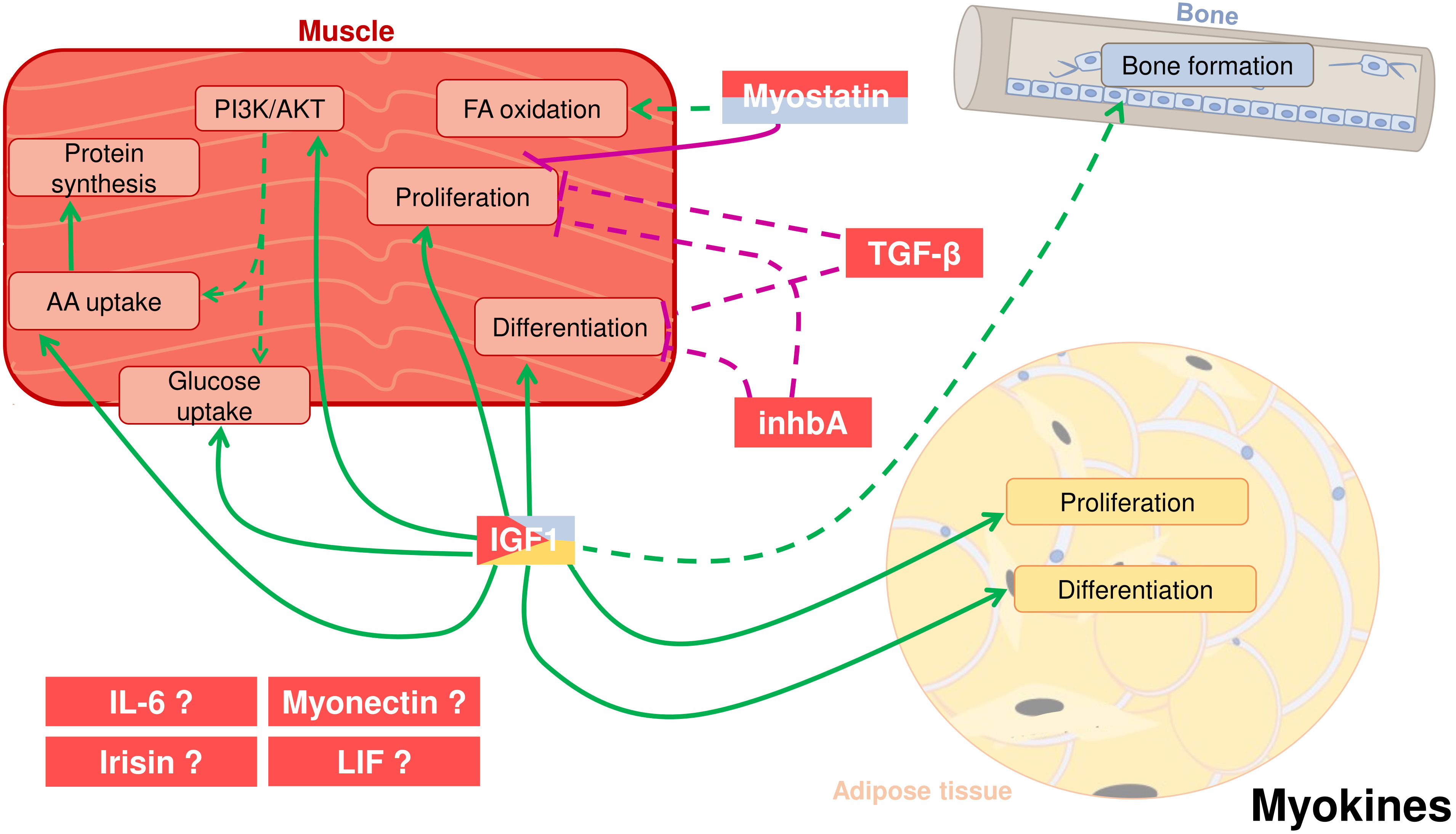
Figure 2 Crosstalk between adipose tissue, muscle, and bone in fish through cytokines secreted by the skeletal muscle, also known as myokines. Myostatin (MSTN) can be secreted by muscle but also from the bone. It has a potential positive effect on fatty acid (FA) oxidation and a proved negative effect on myocyte proliferation. Other two myokines most likely have autocrine roles: Transforming growth factor-β (TGF-β) and inhibin A (inhbA) inhibit cell proliferation and differentiation in muscle. Insulin-like growth factor 1 (IGF-1), produced mostly by muscle but also by other tissues like adipose and bone, enhances in muscle cell proliferation and differentiation, activation of the PI3K/AKT pathway, glucose and amino acid (AA) uptake and protein synthesis. IGF-1 also induces proliferation and differentiation of adipose cells. Moreover, it is also hypothesized that IGF-1 has an influence on bone formation. Other potential myokines are interleukin 6 (IL-6), irisin, myonectin, and leukemia inhibitory factor (LIF). Positive proved effects: green solid line; Positive suggested effects; green dotted line; Negative proved effects: purple solid line; Negative suggested effects: purple dotted line.
4.1 The transforming growth factors family members
The TFG beta (TGF-β) superfamily includes numerous structurally related growth factors known to regulate proliferation and differentiation of many cell types. These growth factors are classified into three subfamilies: TGF-β (including myostatin, MSTN), bone morphogenetic proteins and activin/inhibin.
4.2 Myostatin
The most famous and studied myokine is MSTN, also known as growth differentiation factor 8 (GDF-8) belonging to the TGF-β superfamily. MSTN is synthesized as a precursor protein containing a signal peptide, a large prodomain, and the bioactive peptide. After two cleavages, the mature MSTN dimer functions through binding to the activin receptor IIB that leads to the phosphorylation of the transcription factor Smad3 (180). Identified in 1997 in mouse by McPherron et al. (181), mstn is nearly expressed exclusively in skeletal muscle although it is also detectable in cardiac muscle and adipose tissue. The models of mstn knockout mouse or the natural null allele lead to the “double-muscle” phenotype (181, 182), being MSTN considered as the strongest endogenous inhibitor of muscle growth. Interestingly, the mstn knockout mouse beside the muscle phenotype, also exhibits decreased fat mass. Several works have shown that inactivation of MSTN increases the expression of key thermogenic genes (e.g., ucp1, prdm16) and brown adipose-related markers in white adipose tissues, leading to increased energy expenditure and loss of adipose mass (183, 184).
In fish, mstn genes were identified in numerous species revealing the presence of up to four paralogs coding for a well-conserved mature protein (~90% identity between mammals and fish) (reviewed by Gabillard et al. (185)). Fish mstn expression is not restricted to muscle but rather has a widespread pattern including white and red muscle, brain, liver, ovary… (20, 186–188). In addition, the expression of mstn paralogs is differently regulated according to tissues and physiological situation. In rainbow trout, mstn-1a is expressed in nearly all tissues whereas mstn-1b and mstn-2a are mainly expressed in brain, red and white muscle. In zebrafish and tilapia, mstn-1 is strongly expressed in brain and muscle whereas mstn-2 is mainly expressed in brain (185). Moreover, a fasting period upregulated mstn-1 but not mstn-2 expression in muscle and liver of barramundi (Lates calcarifer) but decreased mstn-1 expression in brain and gills (20). On the contrary, in zebrafish, no clear effect of fasting was observed for both mstn orthologs in muscle (189). Concerning the receptor, expression of activin receptor type IIB has been found in numerous tissues in salmon, goldfish, and zebrafish, suggesting that MSTN could have an effect in several fish tissues including the muscle, hence acting in an autocrine manner (35).
To decipher the mechanism of action of MSTN in fish muscle, several studies used cultured myoblasts. With appropriate proliferation and differentiation media, it was shown that human MSTN inhibited trout myoblast proliferation (21) and induced myotube atrophy (22). In contrast, MSTN had no effect on myoblast differentiation because it did not decrease MyoD and Myogenin protein levels (21) as in mammals (190). Another study (35) observed that myoblasts cultured in low serum concentration (2%) expressed higher levels of myogenic markers (myogenin, myod) in the presence of MSTN, suggesting an enhancement of differentiation. Nevertheless, low serum concentration and addition of MSTN for a long time, strongly inhibited cell proliferation and consequently promoted differentiation in a non-specific manner as suggested by the authors themselves.
To understand the function of MSTN better, several authors inactivated the gene and observed the muscle phenotype. In medaka, a null-mutation of the unique mstn gene induced a 30% increase of muscle mass 16 weeks post-hatching (23), associated to fiber hyperplasia during the first 5 weeks post-hatching and fiber hypertrophy at 16 weeks of age. In the last few years, several papers have reported an increase of muscle mass following mstn disruption in carp (191), blunt snout bream (192), olive flounder (193) and channel catfish (194), showing in this case a consistent response between species. Unfortunately, the consequences of mstn null mutation on other tissues were not studied in these works. TALEN-induced inactivation of mstn gene in medaka observed an increase of muscle mass (25%) but also an alteration of the immune system (24). In zebrafish, inactivation of mstnb but not mstna lead to an increase of muscle mass after 80 days post-fertilization associated to muscle hyperplasia (25, 150). Interestingly, the authors reported an increase of fat accumulation in muscle of mstnb -/- zebrafish and showed evidences for a transition of energy supply from an amino acid- to a lipid-dependent source (150). Recently, CRISPR/Cas9 inactivation of mstn in loach (Misgurnus anguillicaudatus) led to an increase of fibers number but also accumulation of lipids, especially in red muscle (195).
Regarding the impacts on bone metabolism, mstn null mice displayed in some studies, besides muscle hypertrophy, a significant increase in bone mineral density, which provides direct evidence of muscle to bone biochemical crosstalk (196, 197). Some evidence exists that inhibition of MSTN action increases bone formation in mice, suggesting that it exerts a negative effect on osteoblasts differentiation. In contrast, MSTN acts as a positive regulator of osteoclasts, increasing bone resorption (198). Hence, fish in vitro studies using osteoblast cultures (105) would be very advantageous to study the possible communication of MSTN with fish skeletal bone.
Together, these results suggest that MSTN could have a role in non-muscle tissues, but it is unknown whether these effects result from crosstalk between tissues or simply from a wide expression of MSTN, for example in adipose tissue or immune system organs. In fact, bone-derived MSTN has been also recently suggested to control muscle development in gilthead sea bream in two in vivo models, one of fasting and refeeding and another one of muscle regeneration after an injury, since mstn2 expression in bone was in all cases modulated, as proposed by the authors, to coordinate musculoskeletal growth (199, 200).
4.3 Other TGF-β family members
Whereas the function of MSTN is well studied in fish, data about the potential role of other TGF-β members on the crosstalk between adipose, muscle and bone tissues are very scarce. In trout, four tgf-β orthologs (tgf-β1, tgf-β2, tgf-β3, tgf-β6), three paralogs of tgf-β1, (tgf-β1a, tgf-β1b and tgf-β1c), and four inhibin βA (inhba) paralogs have been identified (201), expressed in red and white muscle and adipose tissue. It is noteworthy that tgf-β6, which is not present in tetrapods, is highly expressed in white muscle of gilthead sea bream (202) but not in trout (201). In addition, tgf-β6 expression was downregulated during a refeeding period following fasting in gilthead sea bream muscle (202). Under a comparable experimental condition in trout, tgf-β1a and tgf-β2 were quickly downregulated during refeeding whereas tgf-β3 increased up to 7 days post-refeeding (201). In contrast, the muscle expression of inhba1 but no other inhba, sharply dropped during refeeding being even undetectable in some fish samples. inhba was downregulated during in vitro differentiation of myogenic cells, and upregulated by IGF-1 (39). Similarly, inhba knockout mice exhibited enhancement of muscle development, showing a conserved role for this gene in growth regulation (203). Furthermore, Smad4, the central intracellular mediator of TGF-β signaling, has been shown to be essential for cardiac and skeletal development of zebrafish (42).
Moreover, TGF-β members and inhibin/activin bind to the activin type II receptor (AcvR) and overexpression of a dominant negative form of this receptor induced a strong development of trout muscle (40). In zebrafish, inactivation of both activin receptors (acvr2aa and acvr2ba) induced a strong increase of muscle mass due to fiber hypertrophy (204). Similarly, overexpression of follistatin, an antagonist of inhibin/activin and MSTN, specifically increased muscle growth (41). Together, despite the complexity of the TGFs superfamily reported in fish, the data suggest that some TGF-β members and inhbA could be inhibitors of muscle growth or regeneration in trout, even though these results need to be confirmed in other fish species.
4.4 Insulin-like growth factors
The growth factors IGF-1 and IGF-2 are polypeptides of 67-70 amino acids identified in mammals and fish 50 years ago, and well conserved across evolution (~70% amino acid identity between mammals and fish) (205). These growth factors are mainly produced and secreted from the liver, which accounts for 80% of the circulating IGF-1 (206), the peptide that exerts the major endocrine control of tissues growth. Nevertheless, igfs are expressed in almost all tissues, stimulating in an autocrine/paracrine manner, the proliferation and differentiation of cells. IGFs bind to a tyrosine kinase receptor, the IGF type 1 receptor (IGF1R) that mediates almost all the actions of IGF-1 and IGF-2 and has a ubiquitous expression. Another kind of receptor, the IGF type 2 receptor, exhibits greater affinity for IGF-2 than for IGF-1, but does not activate signaling pathways. In contrast, the binding of IGF-2 to its own receptor results in the degradation of the ligand, thus participating to the regulation of its activity. To define the effects of IGF-1 produced by muscle fibers, a transgenic construct was generated in which expression of a human igf-1 was driven by the avian skeletal α-actin gene (207). IGF-1 concentration in the serum was similar in wild type and transgenic mice, but the latter developed skeletal muscle hypertrophy and male mice had less adipose tissue (208). The IGFs produced by skeletal muscle were considered myokines and modulated by several hormonal and environmental factors to regulate muscle homeostasis but also bone and adipose tissues.
Regarding endocrine or possible autocrine effects, in fish cultured myoblasts, IGFs stimulate cell proliferation (27, 30, 32), amino acid and glucose uptake, protein synthesis (22, 31, 33, 34), and cell differentiation (28, 35, 35, 38) contributing to muscle growth. In addition, growth hormone (GH) and IGF-1 stimulate the expression of igf-1 in myoblasts of gilthead sea bream (209) and Atlantic salmon (29) supporting the promotion of autocrine functions.
From several decades, the effect of nutritional status on igfs expression has been studied in numerous fish species. For instance, food starvation results in suppressed growth associated with a decrease of liver igf-1 mRNA and circulating IGF-1 in trout (36), eel (210), salmon (211), barramundi (212), catfish (213) and tilapia (214). In muscle, food restriction decreases igf-1 but not igf-2 expression in trout (36, 215, 216) and tilapia (217, 218) showing that muscular expression of igf-2 is not related to muscle growth. According to these results, amino acids supplementation stimulated the expression of igf-1 and igf-2 in myoblasts/myotubes of salmon in culture (29). Another environmental parameter that influences expression of igfs is water temperature. Indeed, in juvenile trout, expression of igf-1 in muscle is higher at 16°C than 8°C (219) whereas no significant effect has been observed for igf-2 expression. Nevertheless, the induction of igf-1 expression in muscle was rather due to a temperature-induced increase of food intake than to the temperature itself (219).
Exercise in fish often induces better growth rate, especially in pelagic fish. Recently, it has been reported that gilthead sea bream under sustained swimming expressed more igf-1 in white muscle (220). More precisely, it was reported that the splice variant igf-1c was upregulated whereas no effect was observed for igf-1a and igf-1b. This effect was found in the anterior and the caudal part of the myotome, whereas igf-2 expression was upregulated only in the caudal part, where the muscle is heavily loaded during swimming. In zebrafish, exercise stimulated muscle growth without stimulation of IGF-1 (221) highlighting potential differences between fish species. These differences could be attributed not only to fish species characteristics but also to the type of exercise (intensity and duration). Unfortunately, in those studies bone growth was not analyzed to enlighten a possible role of muscle IGF-1 acting locally on skeletal bone development/metabolism. To our knowledge, only one paper in fish is available showing in vivo evidence of the functional role of IGF-1 produced by muscle in an autocrine or paracrine way. These data showed that transgenic carp overexpressing igf-1 in the white muscle exhibited lower growth and a fast-to-slow fiber switch associated to upregulation of the muscle genes involved in lipid metabolism (222). The authors suggested that the paradoxical lower growth rate reported could be related to deep changes in metabolism that would affect the growth of muscle fibers.
Notwithstanding, despite numerous data on IGFs actions and regulations, it remains unclear whether IGFs produced by the muscle have a function on the development of adipose tissue or bone as it occurs in mammals. In this sense, during bone repair, muscle-derived IGF-1 may signal to the osteoprogenitor cells in the periosteum to increase bone formation in a paracrine way (198). Supporting this hypothesis, increased proliferation was reported in response to IGF-1 in an in vitro model of bone-derived cells from gilthead sea bream (105). However, if this IGF-1 in vivo comes from the muscle or acts as an osteokine in an autocrine manner still must be deciphered.
Furthermore, we could also consider IGF-1 as an adipokine, as it is expressed together with its receptor in adipose tissue as in many other tissues in mammals and fish (43). Some studies have addressed this, and the mitogenic effect of IGF-1 in the adipose tissue has been confirmed. In this sense, IGF-1 increased proliferation of rainbow trout (15) and gilthead sea bream (37) pre-adipocytes and, it also stimulated differentiation in the early stages of gilthead sea bream cells in culture (37). Again, we cannot categorize what type of communication is really happening in vivo considering that this IGF-1 could be coming from the adipose tissue and act in an autocrine way, but also from contiguous tissues such as muscle or from the plasma being IGF-1 produced in the liver, thus representing paracrine or endocrine actions, respectively.
Finally, the activity of IGFs is regulated by six binding proteins (IGFBPs) in mammals and fish (223). Although some of the IGFBPs functions are IGF-independent, it has been clearly shown that they bind IGF-1 and IGF-2 with high affinity to prevent their degradation and to modulate their binding capacity to the IGF1R. igfbp1, igfbp2 and their paralogs are mainly expressed in the liver and involved in the regulation of metabolism. igfbp5 is readily expressed in muscle and associated to muscle growth, downregulated during fasting and upregulated during refeeding in trout in a similar manner than igf-1 and myogenin (36), as well as its expression paralleled that of igf-2 in a model of sustained exercise in gilthead sea bream (220). Due to the presence of numerous igfbp paralogs in the teleost genome, the functions of IGFBPs remain elusive. For a comprehensive overview of teleost IGFBPs, the reader is referred to the review by Garcia de la serrana and Macqueen (223).
4.5 Interleukin-6
IL-6 is a cytokine produced by immune cells, mesenchymal stem cells (MSCs), endothelial cells and fibroblasts (224). In addition, IL-6 was one of the first myokines identified in mammals due to its strong increase in muscle after exercise (225), leading to an increase in blood IL-6 levels (225, 226) that in turn, stimulates hepatic glucose production and lipolysis. In fish, IL-6 was identified in several species including rainbow trout (227) and zebrafish (228) but il-6 expression in muscle has only been observed in zebrafish and very recently, in gilthead sea bream (200). Although the literature on fish IL-6 focuses on its functions in the immune system, transcriptomic analysis of muscle after exercise failed to observe increased il-6 expression in zebrafish (221). Therefore, the function of IL-6 as a myokine in fish remains to be determined.
4.6 Others myokines
Although the number of identified myokines continues to increase in mammals, data in fish is still very scarce. For instance, the functions of irisin (229), myonectin (230) or the leukemia inhibitory factor (LIF) (231) on the crosstalk between fish adipose, muscle and/or bone tissues is still unknown arguing in favor of future work on this direction.
5 Osteokines
Bone is a specialized connective tissue composed of cells and a mineralized extracellular matrix (ECM). The skeleton serves as attachment of the muscle for locomotion, provides mechanical protection for internal organs, and constitutes a reservoir for hematopoietic stem cells and minerals contributing to calcium homeostasis. In mammals, it has been recently shown that the bone can act also as an endocrine organ through the secretion of specific hormones called ‘osteokines’ (232, 233). Evidence over the past decades have identified at least three bone-derived molecules showing endocrine functions. Osteocalcin and lipocalin-2 (LCN2) are secreted by osteoblasts and primarily involved in the control of energy metabolism and homeostasis (234), while fibroblast growth factor 23 (FGF23) is produced by osteoblasts and osteocytes and regulates phosphate homeostasis in the kidney (235). In this review, we will present the existing information about these bone-derived cytokines in fish.
5.1 Osteocalcin
Osteocalcin (a.k.a. bone Gla protein) is a small (45-50 amino acids) secreted molecule belonging to the vitamin K-dependent protein family and containing 3-4 γ-carboxyglutamic acid (Gla) residues, through which it interacts with calcium and hydroxyapatite. Produced by osteoblasts, osteocalcin is among the most abundant (10-20%) non-collagenous proteins found in the ECM of bone and dentin of most vertebrates, from bony fish to mammals (236). In fact, the appearance of osteocalcin seems to have paralleled hydroxyapatite-containing bone structures development, since it is not found in elasmobranchs, whose skeleton is composed of calcified cartilage (237). The mature γ-carboxylated form of osteocalcin has been associated with bone formation and mineralization, and it is required for the correct maturation of the hydroxyapatite crystals in mammalian bone (238). On the other hand, the uncarboxylated osteocalcin has been recently identified in mammals as a hormone regulating glucose metabolism, fat mass, angiogenesis, as well as male reproduction (239–241). Osteocalcin induces the production and secretion of insulin by pancreatic β cells, and promotes adaptation to exercise by stimulating the use of glucose and fatty acids by the skeletal muscle (242–244). In addition, osteocalcin controls cognition, and seems to be necessary for developing the acute stress response (189). However, none of these functions outside the skeleton have been postulated for a fish osteocalcin yet.
The osteocalcin gene originated before the division of the Teleostei and the Tetrapoda. In fish, the first osteocalcin characterized was that of the gilthead sea bream (245). Later on osteocalcin was identified in meagre (Argyrosomus regius), Adriatic sturgeon (Acipenser naccarii), common carp, pufferfish, medaka, Senegalese sole (Solea senegalensis), white sea bream (Diplodus sargus) and rainbow trout among others (246–249). The gene structure and primary sequences of teleosts’ osteocalcins show many conserved features with those of other vertebrates, especially in the central region of the molecule (250). Conserved elements include two invariant cysteine residues that form a single disulfide bond in the mature molecule, as well as three γ-carboxyglutamic acid residues in the Gla domain, key to facilitate binding of osteocalcin to hydroxyapatite (251, 252). Moreover, during the characterization of osteocalcin from the meagre, a fourth carboxylation was reported (250). This feature was identified in other fish osteocalcins through multiple-sequence analysis; as well as in mammals, although no post-translational modification has been reported in those; therefore, its relevance remains to be elucidated. In any case, all known osteocalcins encode polypeptide chains as pre-pro-precursors with well-conserved features, suggesting the assemblage of a similar functional three-dimensional structure. Hence, mature osteocalcin binds strongly to hydroxyapatite to control crystals maturation, but also, a small fraction of osteocalcin (i.e., unbound) is released into the circulation, serum osteocalcin levels being considered the most sensitive marker of bone formation (240, 253).
Although most fish species only have one osteocalcin gene as in mammals, the existence of a second osteocalcin has been reported in some teleosts including zebrafish, rainbow trout, or Atlantic cod (Gadus morhua) (247, 254). Comparative analysis of the mature peptides revealed a high sequence conservation, suggesting that both isoforms may have the same function concerning bone mineralization. Indeed, this second osteocalcin is characterized by a large acidic and serine-rich pro-domain that perhaps, may be highly phosphorylated thus, potentiating its calcium binding properties (247). This second osteocalcin is thought to have arisen from WGD in the Teleostei lineage about 400 million years ago (247, 255). Moreover, in rainbow trout, the reported presence of paralogs for this second osteocalcin is in agreement with the salmonid-specific genome duplication (256).
In terms of tissue expression, fish osteocalcin as in other vertebrates is located only in mineralized bone tissues, including branchial arches, jaw, vertebra, scales and teeth (245, 257). Osteocalcin is associated to osteoblast-like cells and its content in fish bone is not dependent on the origin of the tissue, since similar levels have been found in fish with either cellular or acellular bone (i.e., devoid of entombed osteocytes). However, lower levels of osteocalcin mRNA have been reported in scaleless fishes such as channel catfish (Ictalurus punctatus), probably due to their need of using bone as the primary source of calcium for homeostasis instead of the scales as other fish species (258). Furthermore, as previously observed in mammals (259, 260), osteocalcin mRNA levels vary depending on the stage of development, as it is only present in calcified bone structures also in fish (245, 248, 261). In gilthead sea bream, osteocalcin appearance at around 30 days post-hatching coincides with the onset of skeleton mineralization (245), and the levels of expression at that time are greatly induced in response to increased incubation temperature during embryogenesis (262), which can be related with accelerated growth and the appearance of skeletal abnormalities. Indeed, inverse correlation between malformation severity and osteocalcin levels have been reported in fish (263–265). These evidences are supported by the knockout mice study in which osteocalcin deficiency led to higher bone mass by increased and accelerated bone formation (266).
Osteocalcin functions in mammals seem to be mediated through GPRC6A, a G protein-coupled receptor family C group 6 member (267, 268). In fish, this receptor was first identified in silico in relation to the olfactory epithelium and vomeronasal organ in several species (269). More recently, the expression and functionality of GPCR6A as amino acid receptor and sensor in the gastrointestinal tract of rainbow trout has been demonstrated (270), as in mammalian cells (271); nevertheless, its potential involvement mediating the role of osteocalcin in fish bone or other extra-skeletal tissues like muscle and adipose remains to be elucidated.
5.2 Lipocalin-2
LCN2 (a.k.a. neutrophil gelatinase-associated lipocalin or siderocalin), belongs to the lipocalin family of small secreted proteins (160-200 amino acids), which contains up to 50 members generated after WGD and specific gene duplications (272). Lipocalins share a common folding pattern resulting in a tertiary structure with eight-stranded antiparallel β barrels that enclose a hydrophobic substrate-binding pocket (273). Lipocalins have many different functions depending on their ligands (e.g., retinol, steroids, odorants, pheromones, siderophores, etc.).
Specifically, LCN2 is an iron-sequestering protein, first described in mammals as secreted by neutrophils, and mostly involved in innate immunity functioning as an antimicrobial protein (273–275). The expression of lcn2 in rodents is also strongly induced in cartilage, embryo-derived hypertrophic chondrocytes and myocytes after stimulation with bacterial LPS, showing an inflammatory response during development (276). In humans, lcn2 is highly expressed among others in the liver and adipose tissue, and circulating LCN2 has been identified as an inflammatory marker closely related to obesity and its metabolic complications (277). More recently, bone-derived LCN2 has been identified, and demonstrated that it influences energy metabolism by activating anorexigenic signaling pathways in the brain (278). These actions of LCN2 occur through binding to the melanocortin 4 receptor at the level of the hypothalamic paraventricular and ventromedial neurons.
In fish, a recent phylogenetic study of the lipocalin family, showed six members, being the lipocalin-type prostaglandin D synthase the evolutionary precursor of LCN2. In triploid crucian carp, a lipocalin named 3nLcn2 was recently described, showing a lipocalin domain and moderate to high levels of sequence identity with other fish lipocalins, although low when compared with mammalian sequences (279). Furthermore, 3nLcn2 presented antimicrobial activity against bacterial infection, especially exerting a protective effect at intestinal level, thus playing a role in immune defense in fish, as previously described in mammals. However, LCN2 bone-secretion or function as an osteokine have not been described yet for any fish species.
5.3 Fibroblast growth factor 23
FGF23 is in mammals, primarily expressed and secreted from the bone and related to phosphorous regulation (280), although it has been also proposed to act controlling body calcium homeostasis (281).
FGF23 is a 32 kDa protein, phylogenetically grouped with members of a FGFs subfamily that act as hormones or systemic factors interacting with specific receptors in the presence of members of the Klotho family of proteins. Indeed, FGF23 binds to Klotho, which encodes a type I membrane β-glycosidase-like protein that is an essential cofactor for FGF23 binding to FGF receptors (280).
In teleosts, fgf23 and αklotho have been cloned in zebrafish among other species. Phylogenetic analysis showed as expected, that zebrafish fgf23 is more closely related to fgf23 from other fish species such as the rainbow smelt (Osmerus mordax) and the spotted green pufferfish, sharing 63 and 57% identity, respectively, than to the human gene (282). In adult zebrafish, fgf23 is specifically expressed in the corpuscles of Stannius (282), which are endocrine glands that lie in close proximity to the nephron and are thought to be involved in calcium homeostasis, and αklotho is expressed in the kidney. fgf23 expression is stimulated by ambient water with a high calcium level. Overexpression of fgf23 in zebrafish lead to inhibition of calcium uptake by downregulating the expression of the epithelium calcium channel (ECaC) suggesting that FGF23 functions as a hypocalcemic hormone (283). Besides, a previous study also using zebrafish, reported that FGF23 regulates phosphate homeostasis (284). Interestingly, fgf23 and αklotho mutations cause shortened lifespan in zebrafish, with early onset of behavioral and degenerative physical changes, and a specific calcification of vessels throughout the body (285). Altogether, these studies suggest a significant role of FGF23 and Klotho regulating fish mineral balance and aging-related processes, similarly as it occurs in mammals.
6 New approaches to specific analysis of tissue interactions
Other complementary studies that aim to decipher cellular interactions have been developed in mammalian models, including cells co-cultured, to try understanding in vitro how they communicate in vivo through contacts, secretions or both (286). For instance, adipocytes cultured in 2D as freshly isolated from tissues or immortalized cell-lines, evidenced some of these interactions: endocrine and paracrine mainly.
Indeed, due to adipose tissue evolution (175) and numerous adipose depots (visceral, subcutaneous, intra-muscular, brown…), adipocytes from endotherms were cultured with various cell types like those from blood vessels (287), cartilage (288), immune tissues (289), intestine (290) or even cancers (291). Herein, we focus on adipocyte-muscle co-cultures. Floating and fragile mature adipocytes used in co-cultures are rare as compared to cell-lines such as 3T3-L1, mouse fibroblasts that differentiate into adipocyte-like cells and the mouse myoblast cell line C2C12, themselves preferred to others (292). As an illustration for reciprocal dialogs among these cells: 3T3-L1 suppress muscle differentiation in C2C12 myocytes (293), while differentiated C2C12 inhibit proliferation and differentiation of 3T3-L1 adipocytes (294). Pre-adipocyte models, developed in vitro to provide differentiating adipocytes within 2 weeks of culture, did not resemble fully mature adipocytes with their large unilocular lipid droplet (295), but eased the co-culture in several species. Papers reporting on pre-adipocytes and muscle cells described different kinds of interactions and effects depending on the cell ratio, cell densities or length of co-culture time.
Studies with intra-muscular adipocytes in co-culture are rare, even though from their location they would be ideal partners to crosstalk with muscle MSCs. As an example, intra-muscular pre-adipocytes (IMPA) from chicken muscle impeded MSCs differentiation and promoted lipid deposition (26), proliferating MSCs inhibited IMPA differentiation and non-proliferating MSCs accelerated IMPA differentiation and lipid storage (296). Last, but not least, fibro-adipogenic progenitors, identified as multi-potent progenitors within the muscle, are highly influenced by the environment in their lineage choices and, conversely, influence muscle homeostasis by their secretions (297).
Based on single-cell or -nucleus sequencing, multiple adipocyte subtypes were recently reported (298, 299), opening the avenue to co-cultures with dedicated subpopulations depending on the cell-cell communications to be explored within adipose tissues or with distal organs (300). In addition, a renewed interest for the ECM: composition, physics and function (301) paves the way to the culture of fat cells or progenitors with autologous ECM, in vivo or in vitro derived (302).
Concerning other cell types, adipocyte stem cells stimulate 2D-co-cultured chondrocytes in a way that may contribute cartilage repair (303), whereas mature adipocytes modulate osteoclast differentiation (304), through the secretion of growth factors or adipokines: clear illustrations of an active bone-muscle crosstalk in (305). In the limits of our knowledge, no such co-cultures have been reported in fish species so far.
Thereafter, 3D-cultures appeared attractive to work out as a model since they include cell-cell contacts and so, putative juxtacrine signals (292, 306, 307). As an example, 3D in vitro structures were proposed for muscle/bone co-cultures using established cell lines for muscle (murine C2C12) and bone (human TE85 (308);). In optimal cases however, cultured cells look as closely as possible to those inside the tissues and keep this phenotype as long as possible to signal properly to the other cell types. For example, membrane mature adipocyte aggregate cultures helped maintaining adipogenic expression and long-term cultures with no functional loss, so that subcutaneous or visceral adipocytes retained depot-specific expression after 14 days (289) while mature adipocytes are reputed fragile and alive in vitro for only a few hours or days.
Although co-culture systems in fish remain to be developed, transgenic lines appeared in zebrafish that helped following in vivo adipocyte differentiation processes or adiposity dynamics (309), through the imaging of adipocyte- or lipid droplet-specific labels (fabp4-EGFP (310);; plin2-TOMATO (311);). Together with the use of a fasting-refeeding experiment (fabp11-EGFP (312);) or a high fat diet (311) these lines helped reporting on the metabolic regulations that impact on adipose tissues.
Furthermore, extracellular vesicles (EVs) isolated from various biological fluids (blood, lymph for instance) or cell culture media, mediate important communication between donor and recipient cells. In fact, EVs are released by a wide variety of cell types and are found in all organisms investigated so far (313). Recipient cells catch them by endocytosis, ligand binding or membrane fusion (313). From donor cells, large EVs or microvesicles (MVs) form by budding of the plasma membrane and vary in diameter from 250 to 500 nm. Small EVs or exosomes (40-100 nm) form inside the cell by fusion of intraluminal vesicles with the plasma membrane, and share with EVs common cargos such as proteins, lipids, RNAs (314). However, cargos of MVs or exosomes can also be selective, regulated by physiological or pathological states (315), their release thus resembling paracrine/endocrine signals. EVs secreted by the adipose tissue affect bone remodeling (via lipid transfer, circulating miRNAs or EV-linked-adipokines (316);). And, a reciprocal EV-based crosstalk between muscle and bone has also been reported (317), highlighting the role of such vesicles, conserved among eukaryotes (318). Interestingly, zebrafish embryo-expressing fluorescent proteins associated with exosomes (e.g., CD63-phluorin) have been demonstrated to be a relevant model to study endogenous EVs in vivo to unravel fundamental aspects in EVs physiology and their role in inter-organ communication (319, 320).
In mammals, interplays among cytokines and/or exosomes, also contribute to the metabolic situations altered in obesity or improved by exercise. As an example, metabolic organs defined as adipose tissue, gut, skeletal muscle and liver, can communicate with macrophages infiltrated into the adipose tissue in obesity cases (321). Similarly somehow, exercise-induced soluble factors among which adipokines, myokines, osteokines contribute to redistributed energy, appetite control, fat loss and reduced systemic inflammation (322, 323). Therein, the adipose tissue appears as an endocrine organ that interacts with others through cross talking with the endothelial cells of the blood vessels (287). Similar interplays might well be active in fish species as well, but few papers have addressed the question except one dealing with feeding, appetite regulating mechanisms, and energy homeostasis (324), proposing teleost fish as models to understand mammalian dysregulations.
7 Conclusions
To conclude, our current knowledge about adipokines, myokines, and osteokines physiologies relies, in a great part, on different findings regarding peptide structure, receptors, and expression patterns in a variety of fish species and tissues. The scenario is very complex in teleost fish, since these molecules are described in a wide variety of tissues and sometimes, different isoforms are identified. In addition, profiles of tissue distribution are different in many cases from those in mammals, probably associated with the specific fish physiology. That happens with some adipokines, which in fish, are rather expressed in liver, or in skeletal muscle, which are important organs of lipid deposition. Subsequently, the denomination of these factors as adipo-, myo- or osteo-kines could be more diffuse in fish.
Here we provide evidence of the existence of crosstalk between tissues from studies on the autocrine, paracrine, and endocrine effects of these cytokines and more specifically, findings from null mutations or knockouts of specific genes (i.e., mstn, lepr). Nevertheless, the importance of this communication between adipose, muscle and bone tissues during fish development, or along the life cycle, is not known yet.
Another unresolved question is the identity of the secretomes from these tissues in basal conditions or during fish exercise, fasting or aging, in order to adjust the whole organism’s homeostasis. The identification of molecular actors involved in inter-tissue communication opens new avenues of knowledge and strategies to improve growth and ameliorate possible metabolic disorders or skeletal anomalies in cultured fish.
Adipose tissue, muscle and bone are intimately linked, both in forms and in functions from embryogenesis to growth and development. Therefore, bone-muscle, muscle-adipose tissue, and bone-adipose tissue communication must be bi-directional with a complicated network of cytokines involved in such crosstalk. Research in this aspect could be greatly helped by the development of new experimental tools to determine whether these cytokines are responsible of a particular pathway of communication between tissues. New approaches such as the co-culture of different cell types, the study of exosomes or even the development of organoids will thus improve the understanding of the mechanisms underlying tissues crosstalk in fish.
Author contributions
IH, EC, J-CG, and IN contributed to conception and design of the review. ER-M, SB-P, and VG prepared the table and figures. IH, EC, ER-M, J-CG, and IN wrote sections of the manuscript. All authors contributed to manuscript revision, read, and approved the submitted version.
Funding
This work was supported by INRAE PHASE department (project API Adipo3D 2022) and the French “Agence Nationale de la Recherche” (ANR-20-CE20-0013-01; ANR-08-BLAN-0267). Moreover, this review is part of the R+D+I project PID2020-116172RB-I00 granted by the Spanish “Ministerio de Ciencia e Innovación” (MCIN/AEI/10.13039/501100011033/), and by the “Generalitat de Catalunya” (2021SGR-00713). ERM was supported by a predoctoral fellowship from the “Generalitat de Catalunya” (2021FISDU-00314), SBP by a predoctoral fellowship PRE2018-085580 from the MCIN/AEI/10.13039/501100011033 and by “ESF Investing in your future”, and VG by a fellowship from INRAE PHASE and the Région Bretagne.
Conflict of interest
The authors declare that the research was conducted in the absence of any commercial or financial relationships that could be construed as a potential conflict of interest.
Publisher’s note
All claims expressed in this article are solely those of the authors and do not necessarily represent those of their affiliated organizations, or those of the publisher, the editors and the reviewers. Any product that may be evaluated in this article, or claim that may be made by its manufacturer, is not guaranteed or endorsed by the publisher.
References
1. Kirk B, Feehan J, Lombardi G, Duque G. Muscle, bone, and fat crosstalk: the biological role of myokines, osteokines, and adipokines. Curr Osteoporos Rep (2020) 18:388–400. doi: 10.1007/s11914-020-00599-y
2. Liu S, Alexander RK, Lee C-H. Lipid metabolites as metabolic messengers in inter-organ communication. Trends Endocrinol Metab (2014) 25:356–63. doi: 10.1016/j.tem.2014.05.002
3. Lombardi G, Sanchis-Gomar F, Perego S, Sansoni V, Banfi G. Implications of exercise-induced adipo-myokines in bone metabolism. Endocrine (2016) 54:284–305. doi: 10.1007/s12020-015-0834-0
4. Severinsen MCK, Pedersen BK. Muscle–organ crosstalk: The emerging roles of myokines. Endocrine Rev (2020) 41:594–609. doi: 10.1210/endrev/bnaa016
5. Sánchez-Gurmaches J, Cruz-Garcia L, Gutiérrez J, Navarro I. Adiponectin effects and gene expression in rainbow trout: an in vivo and in vitro approach. J Exp Biol (2012) 215:1373–83. doi: 10.1242/jeb.061697
6. Bou M, Todorčević M, Rodríguez J, Capilla E, Gutiérrez J, Navarro I. Interplay of adiponectin, TNFα and insulin on gene expression, glucose uptake and PPARγ, AKT and TOR pathways in rainbow trout cultured adipocytes. Gen Comp Endocrinol (2014) 205:218–25. doi: 10.1016/j.ygcen.2014.05.005
7. Carnovali M, Luzi L, Terruzzi I, Banfi G, Mariotti M. Metabolic and bone effects of high-fat diet in adult zebrafish. Endocrine (2018) 61:317–26. doi: 10.1007/s12020-017-1494-z
8. Ji R, Xu X, Xiang X, Zhu S, Li Y, Mai K, et al. Regulation of adiponectin on lipid metabolism in large yellow croaker (Larimichthys crocea). Biochim Biophys Acta (BBA) Mol Cell Biol Lipids (2020) 1865:158711. doi: 10.1016/j.bbalip.2020.158711
9. Salmerón C, Johansson M, Angotzi AR, Rønnestad I, Jönsson E, Björnsson BT, et al. Effects of nutritional status on plasma leptin levels and in vitro regulation of adipocyte leptin expression and secretion in rainbow trout. Gen Comp Endocrinol (2015) 210:114–23. doi: 10.1016/j.ygcen.2014.10.016
10. Yang Q, Yan C, Wang X, Gong Z. Leptin induces muscle wasting in a zebrafish kras-driven hepatocellular carcinoma (HCC) model. Dis Models Mech (2019) 12:dmm038240. doi: 10.1242/dmm.038240
11. Basto-Silva C, Balbuena-Pecino S, Oliva-Teles A, Riera-Heredia N, Navarro I, Guerreiro I, et al. Gilthead seabream (Sparus aurata) in vitro adipogenesis and its endocrine regulation by leptin, ghrelin, and insulin. Comp Biochem Physiol Part A: Mol Integr Physiol (2020) 249:110772. doi: 10.1016/j.cbpa.2020.110772
12. Tang N, Liu Y, Tian Z, Xu S, Wang M, Chen H, et al. Characterization, tissue distribution of resistin gene and the effect of fasting and refeeding on resistinmRNA expression in Siberian sturgeon ( Acipenser baerii ). J Fish Biol (2020) 97:508–14. doi: 10.1111/jfb.14406
13. Albalat A, Gutiérrez J, Navarro I. Regulation of lipolysis in isolated adipocytes of rainbow trout (Oncorhynchus mykiss): The role of insulin and glucagon. Comp Biochem Physiol Part A: Mol Integr Physiol (2005) 142:347–54. doi: 10.1016/j.cbpa.2005.08.006
14. Cruz-Garcia L, Sánchez-Gurmaches J, Gutiérrez J, Navarro I. Role of LXR in trout adipocytes: Target genes, hormonal regulation, adipocyte differentiation and relation to lipolysis. Comp Biochem Physiol Part A: Mol Integr Physiol (2012) 163:120–6. doi: 10.1016/j.cbpa.2012.05.193
15. Bouraoui L, Gutiérrez J, Navarro I. Regulation of proliferation and differentiation of adipocyte precursor cells in rainbow trout (Oncorhynchus mykiss). J Endocrinol (2008) 198:459–69. doi: 10.1677/JOE-08-0264
16. Vraskou Y, Roher N, Díaz M, Antonescu CN, MacKenzie SA, Planas JV. Direct involvement of tumor necrosis factor-α in the regulation of glucose uptake in rainbow trout muscle cells. Am J Physiol-Regulatory Integr Comp Physiol (2011) 300:R716–23. doi: 10.1152/ajpregu.00514.2010
17. Wang X, Huang M, Wang Y. The effect of insulin, TNFα and DHA on the proliferation, differentiation and lipolysis of preadipocytes isolated from Large yellow croaker (Pseudosciaena crocea r.). PloS One (2012) 7:e48069. doi: 10.1371/journal.pone.0048069
18. Saera-Vila A, Calduch-Giner JA, Navarro I, Pérez-Sánchez J. Tumour necrosis factor (TNF)α as a regulator of fat tissue mass in the Mediterranean gilthead sea bream (Sparus aurata l.). Comp Biochem Physiol Part B: Biochem Mol Biol (2007) 146:338–45. doi: 10.1016/j.cbpb.2006.11.003
19. Cruz-Garcia L, Saera-Vila A, Navarro I, Calduch-Giner J, Pérez-Sánchez J. Targets for TNFα-induced lipolysis in gilthead sea bream(Sparus aurata l.) adipocytes isolated from lean and fat juvenile fish. J Exp Biol (2009) 212:2254–60. doi: 10.1242/jeb.029025
20. De Santis C, Jerry DR. Differential tissue-regulation of myostatin genes in the teleost fish lates calcarifer in response to fasting. Evid Funct Diff Mol Cell Endocrinol (2011) 335:158–65. doi: 10.1016/j.mce.2011.01.011
21. Seiliez I, Sabin N, Gabillard J-C. Myostatin inhibits proliferation but not differentiation of trout myoblasts. Mol Cell Endocrinol (2012) 351:220–6. doi: 10.1016/j.mce.2011.12.011
22. Seiliez I, Taty Taty GC, Bugeon J, Dias K, Sabin N, Gabillard J-C. Myostatin induces atrophy of trout myotubes through inhibiting the TORC1 signaling and promoting ubiquitin–proteasome and autophagy-lysosome degradative pathways. Gen Comp Endocrinol (2013) 186:9–15. doi: 10.1016/j.ygcen.2013.02.008
23. Chisada S, Okamoto H, Taniguchi Y, Kimori Y, Toyoda A, Sakaki Y, et al. Myostatin-deficient medaka exhibit a double-muscling phenotype with hyperplasia and hypertrophy, which occur sequentially during post-hatch development. Dev Biol (2011) 359:82–94. doi: 10.1016/j.ydbio.2011.08.027
24. Chiang Y-A, Kinoshita M, Maekawa S, Kulkarni A, Lo C-F, Yoshiura Y, et al. TALENs-mediated gene disruption of myostatin produces a larger phenotype of medaka with an apparently compromised immune system. Fish Shellfish Immunol (2016) 48:212–20. doi: 10.1016/j.fsi.2015.11.016
25. Wang C, Chen Y-L, Bian W-P, Xie S-L, Qi G-L, Liu L, et al. Deletion of mstna and mstnb impairs the immune system and affects growth performance in zebrafish. Fish Shellfish Immunol (2018) 72:572–80. doi: 10.1016/j.fsi.2017.11.040
26. Guo L, Cui H, Zhao G, Liu R, Li Q, Zheng M, et al. Intramuscular preadipocytes impede differentiation and promote lipid deposition of muscle satellite cells in chickens. BMC Genomics (2018) 19:838. doi: 10.1186/s12864-018-5209-5
27. Castillo J, Codina M, Martínez ML, Navarro I, Gutiérrez J. Metabolic and mitogenic effects of IGF-I and insulin on muscle cells of rainbow trout. Am J Physiol-Regulatory Integr Comp Physiol (2004) 286:R935–41. doi: 10.1152/ajpregu.00459.2003
28. Duran BOS, Zanella BTT, Perez ES, Mareco EA, Blasco J, Dal-Pai-Silva M, et al. Amino acids and IGF1 regulation of fish muscle growth revealed by transcriptome and microRNAome integrative analyses of pacu (Piaractus mesopotamicus) myotubes. Int J Mol Sci (2022) 23:1180. doi: 10.3390/ijms23031180
29. Bower NI, Johnston IA. Transcriptional regulation of the IGF signaling pathway by amino acids and insulin-like growth factors during myogenesis in Atlantic salmon. PloS One (2010) 5:e11100. doi: 10.1371/journal.pone.0011100
30. Gabillard JC, Sabin N, Paboeuf G. In vitro characterization of proliferation and differentiation of trout satellite cells. Cell Tissue Res (2010) 342:471–7. doi: 10.1007/s00441-010-1071-8
31. Cleveland BM, Weber GM. Effects of insulin-like growth factor-I, insulin, and leucine on protein turnover and ubiquitin ligase expression in rainbow trout primary myocytes. Am J Physiol-Regulatory Integr Comp Physiol (2010) 298:R341–50. doi: 10.1152/ajpregu.00516.2009
32. Rius-Francino M, Acerete L, Jiménez-Amilburu V, Capilla E, Navarro I, Gutiérrez J. Differential effects on proliferation of GH and IGFs in sea bream (Sparus aurata) cultured myocytes. Gen Comp Endocrinol (2011) 172:44–9. doi: 10.1016/j.ygcen.2011.03.024
33. Seiliez I, Sabin N, Gabillard J-C. FoxO1 is not a key transcription factor in the regulation of myostatin (mstn-1a and mstn-1b) gene expression in trout myotubes. Am J Physiol-Regulatory Integr Comp Physiol (2011) 301:R97–R104. doi: 10.1152/ajpregu.00828.2010
34. Montserrat N, Capilla E, Navarro I, Gutiérrez J. Metabolic effects of insulin and IGFs on gilthead Sea bream (Sparus aurata) muscle cells. Front Endocrinol (2012) 3. doi: 10.3389/fendo.2012.00055
35. Garikipati DK, Rodgers BD. Myostatin stimulates myosatellite cell differentiation in a novel model system: evidence for gene subfunctionalization. Am J Physiol-Regulatory Integr Comp Physiol (2012) 302:R1059–66. doi: 10.1152/ajpregu.00523.2011
36. Gabillard J-C, Kamangar BB, Montserrat N. Coordinated regulation of the GH/IGF system genes during refeeding in rainbow trout (Oncorhynchus mykiss). J Endocrinol (2006) 191:15–24. doi: 10.1677/joe.1.06869
37. Salmerón C, Acerete L, Gutiérrez J, Navarro I, Capilla E. Characterization and endocrine regulation of proliferation and differentiation of primary cultured preadipocytes from gilthead sea bream (Sparus aurata). Domest Anim Endocrinol (2013) 45:1–10. doi: 10.1016/j.domaniend.2013.02.002
38. Kaiya H, Kojima M, Hosoda H, Riley LG, Hirano T, Grau EG, et al. Identification of tilapia ghrelin and its effects on growth hormone and prolactin release in the tilapia, oreochromis mossambicus. Comp Biochem Physiol Part B: Biochem Mol Biol (2003) 135:421–9. doi: 10.1016/S1096-4959(03)00109-X
39. de Mello F, Streit DP, Sabin N, Gabillard J-C. Dynamic expression of tgf-β2, tgf-β3 and inhibin βA during muscle growth resumption and satellite cell differentiation in rainbow trout (Oncorhynchus mykiss). Gen Comp Endocrinol (2015) 210:23–9. doi: 10.1016/j.ygcen.2014.10.011
40. Phelps MP, Jaffe IM, Bradley TM. Muscle growth in teleost fish is regulated by factors utilizing the activin II b receptor. J Exp Biol (2013) 216:3742–50. doi: 10.1242/jeb.086660
41. Medeiros EF, Phelps MP, Fuentes FD, Bradley TM. Overexpression of follistatin in trout stimulates increased muscling. Am J Physiol-Regulatory Integr Comp Physiol (2009) 297:R235–42. doi: 10.1152/ajpregu.91020.2008
42. Yang J, Wang J, Zeng Z, Qiao L, Zhuang L, Jiang L, et al. Smad4 is required for the development of cardiac and skeletal muscle in zebrafish. Differentiation (2016) 92:161–8. doi: 10.1016/j.diff.2016.06.005
43. Moriyama S, Ayson FG, Kawauchi H. Growth regulation by insulin-like growth factor-I in fish. Biosci Biotechnol Biochem (2000) 64:1553–62. doi: 10.1271/bbb.64.1553
44. Nishio S-I, Gibert Y, Bernard L, Brunet F, Triqueneaux G, Laudet V. Adiponectin and adiponectin receptor genes are coexpressed during zebrafish embryogenesis and regulated by food deprivation. Dev Dynamics (2008) 237:1682–90. doi: 10.1002/dvdy.21559
45. Kondo H, Suga R, Suda S, Nozaki R, Hirono I, Nagasaka R, et al. EST analysis on adipose tissue of rainbow trout oncorhynchus mykiss and tissue distribution of adiponectin. Gene (2011) 485:40–5. doi: 10.1016/j.gene.2011.05.035
46. Liu BH, Wang YC, Wu SC, Mersmann HJ, Cheng WTK, Ding ST. Insulin regulates the expression of adiponectin and adiponectin receptors in porcine adipocytes. Domest Anim Endocrinol (2008) 34:352–9. doi: 10.1016/j.domaniend.2007.10.003
47. Pi D, Wang J, Zhao M, Liu M, Zhang Y, Qin C, et al. Adiponectin and adiponectin receptors in common carp (Cyprinus carpio): Tissue distribution and their expressions in response to high-carbohydrate and high-lipid diets. Aquacult Rep (2022) 27:101341. doi: 10.1016/j.aqrep.2022.101341
48. Qin C, Zhao W, Yan X, Yang G, Yang L, Lu R, et al. Effects of adiponectin on glucose metabolism in the hepatopancreas of grass carp (Ctenopharyngodon idella). Aquacult Nutr (2022) 2022:e5699931. doi: 10.1155/2022/5699931
49. Liu H, Lu X-J, Chen J. Full-length and a smaller globular fragment of adiponectin have opposite roles in regulating monocyte/macrophage functions in ayu, plecoglossus altivelis. Fish Shellfish Immunol (2018) 82:319–29. doi: 10.1016/j.fsi.2018.08.041
50. Halleux CM, Takahashi M, Delporte ML, Detry R, Funahashi T, Matsuzawa Y, et al. Secretion of adiponectin and regulation of apM1 gene expression in human visceral adipose tissue. Biochem Biophys Res Commun (2001) 288:1102–7. doi: 10.1006/bbrc.2001.5904
51. Hajri T, Tao H, Wattacheril J, Marks-Shulman P, Abumrad NN. Regulation of adiponectin production by insulin: interactions with tumor necrosis factor-α and interleukin-6. Am J Physiol-Endocrinol Metab (2011) 300:E350–60. doi: 10.1152/ajpendo.00307.2010
52. Sun YG, Zan LS, Wang HB, Guo HF, Yang DP, Zhao XL, et al. Insulin inhibits the expression of adiponectin and AdipoR2 mRNA in cultured bovine adipocytes. Asian Australas J Anim Sci (2009) 22:1429–36. doi: 10.5713/ajas.2009.90159
53. Weyer C, Funahashi T, Tanaka S, Hotta K, Matsuzawa Y, Pratley RE, et al. Hypoadiponectinemia in obesity and type 2 diabetes: Close association with insulin resistance and hyperinsulinemia. J Clin Endocrinol Metabol (2001) 86:6. doi: 10.1210/jcem.86.5.7463
54. Maeda N, Shimomura I, Kishida K, Nishizawa H, Matsuda M, Nagaretani H, et al. Diet-induced insulin resistance in mice lacking adiponectin/ACRP30. Nat Med (2002) 8:731–7. doi: 10.1038/nm724
55. Yamauchi T, Kamon J, Ito Y, Tsuchida A, Yokomizo T, Kita S, et al. Cloning of adiponectin receptors that mediate antidiabetic metabolic effects. Nature (2003) 423:762–9. doi: 10.1038/nature01705
56. Yamauchi T, Kamon J, Minokoshi Y, Ito Y, Waki H, Uchida S, et al. Adiponectin stimulates glucose utilization and fatty-acid oxidation by activating AMP-activated protein kinase. Nat Med (2002) 8:1288–95. doi: 10.1038/nm788
57. Pal China S, Sanyal S, Chattopadhyay N. Adiponectin signaling and its role in bone metabolism. Cytokine (2018) 112:116–31. doi: 10.1016/j.cyto.2018.06.012
58. Naot D, Musson DS, Cornish J. The activity of adiponectin in bone. Calcif Tissue Int (2017) 100:486–99. doi: 10.1007/s00223-016-0216-5
59. Achari AE, Jain SK. Adiponectin, a therapeutic target for obesity, diabetes, and endothelial dysfunction. Int J Mol Sci (2017) 18:1321. doi: 10.3390/ijms18061321
60. Bower NI, Taylor RG, Johnston IA. Phasing of muscle gene expression with fasting-induced recovery growth in Atlantic salmon. Front Zool (2009) 6:18. doi: 10.1186/1742-9994-6-18
61. Kurokawa T, Uji S, Suzuki T. Identification of cDNA coding for a homologue to mammalian leptin from pufferfish, takifugu rubripes. Peptides (2005) 26:745–50. doi: 10.1016/j.peptides.2004.12.017
62. Blanco AM, Soengas JL. Leptin signalling in teleost fish with emphasis in food intake regulation. Mol Cell Endocrinol (2021) 526:111209. doi: 10.1016/j.mce.2021.111209
63. Huising MO, Geven EJW, Kruiswijk CP, Nabuurs SB, Stolte EH, Spanings FAT, et al. Increased leptin expression in common carp (Cyprinus carpio) after food intake but not after fasting or feeding to satiation. Endocrinology (2006) 147:5786–97. doi: 10.1210/en.2006-0824
64. Gorissen M, Bernier NJ, Nabuurs SB, Flik G, Huising MO. Two divergent leptin paralogues in zebrafish (Danio rerio) that originate early in teleostean evolution. J Endocrinol (2009) 201:329–39. doi: 10.1677/JOE-09-0034
65. Kurokawa T, Murashita K. Genomic characterization of multiple leptin genes and a leptin receptor gene in the Japanese medaka, oryzias latipes. Gen Comp Endocrinol (2009) 161:229–37. doi: 10.1016/j.ygcen.2009.01.008
66. Rønnestad I, Nilsen TO, Murashita K, Angotzi AR, Gamst Moen A-G, Stefansson SO, et al. Leptin and leptin receptor genes in Atlantic salmon: Cloning, phylogeny, tissue distribution and expression correlated to long-term feeding status. Gen Comp Endocrinol (2010) 168:55–70. doi: 10.1016/j.ygcen.2010.04.010
67. Won ET, Baltzegar DA, Picha ME, Borski RJ. Cloning and characterization of leptin in a perciform fish, the striped bass (Morone saxatilis): Control of feeding and regulation by nutritional state. Gen Comp Endocrinol (2012) 178:98–107. doi: 10.1016/j.ygcen.2012.04.019
68. Gong N, Einarsdottir IE, Johansson M, Björnsson BT. Alternative splice variants of the rainbow trout leptin receptor encode multiple circulating leptin-binding proteins. Endocrinology (2013) 154:2331–40. doi: 10.1210/en.2012-2082
69. He S, Liang X-F, Li L, Huang W, Shen D, Tao Y-X. Gene structure and expression of leptin in Chinese perch. Gen Comp Endocrinol (2013) 194:183–8. doi: 10.1016/j.ygcen.2013.09.008
70. Tinoco AB, Nisembaum LG, Isorna E, Delgado MJ, de Pedro N. Leptins and leptin receptor expression in the goldfish (Carassius auratus). regulation by food intake and fasting/overfeeding conditions. Peptides (2012) 34:329–35. doi: 10.1016/j.peptides.2012.02.001
71. Morini M, Pasquier J, Dirks R, van den TG, Tomkiewicz J, Rousseau K, et al. Duplicated leptin receptors in two species of eel bring new insights into the evolution of the leptin system in vertebrates. PloS One (2015) 10:e0126008. doi: 10.1371/journal.pone.0126008
72. Wen Z-Y, Qin C-J, Wang J, He Y, Li H-T, Li R, et al. Molecular characterization of two leptin genes and their transcriptional changes in response to fasting and refeeding in northern snakehead (Channa argus). Gene (2020) 736:144420. doi: 10.1016/j.gene.2020.144420
73. Gorissen M, Flik G. Leptin in teleostean fish, towards the origins of leptin physiology. J Chem Neuroanat (2014) 61–62:200–6. doi: 10.1016/j.jchemneu.2014.06.005
74. Deck CA, Honeycutt JL, Cheung E, Reynolds HM, Borski RJ. Assessing the functional role of leptin in energy homeostasis and the stress response in vertebrates. Front Endocrinol (2017) 8. doi: 10.3389/fendo.2017.00063
75. Ahima RS, Flier JS. Adipose tissue as an endocrine organ. Trends Endocrinol Metab (2000) 11:327–32. doi: 10.1016/S1043-2760(00)00301-5
76. Yuan X, Li A, Liang X-F, Huang W, Song Y, He S, et al. Leptin expression in mandarin fish siniperca chuatsi (Basilewsky): Regulation by postprandial and short-term fasting treatment. Comp Biochem Physiol Part A: Mol Integr Physiol (2016) 194:8–18. doi: 10.1016/j.cbpa.2016.01.014
77. Ohga H, Matsumori K, Kodama R, Kitano H, Nagano N, Yamaguchi A, et al. Two leptin genes and a leptin receptor gene of female chub mackerel (Scomber japonicus): Molecular cloning, tissue distribution and expression in different obesity indices and pubertal stages. Gen Comp Endocrinol (2015) 222:88–98. doi: 10.1016/j.ygcen.2015.06.002
78. Shpilman M, Hollander-Cohen L, Ventura T, Gertler A, Levavi-Sivan B. Production, gene structure and characterization of two orthologs of leptin and a leptin receptor in tilapia. Gen Comp Endocrinol (2014) 207:74–85. doi: 10.1016/j.ygcen.2014.05.006
79. Zhang H, Chen H, Zhang Y, Li S, Lu D, Zhang H, et al. Molecular cloning, characterization and expression profiles of multiple leptin genes and a leptin receptor gene in orange-spotted grouper (Epinephelus coioides). Gen Comp Endocrinol (2013) 181:295–305. doi: 10.1016/j.ygcen.2012.09.008
80. Volkoff H, Estevan Sabioni R, Coutinho LL, Cyrino JEP. Appetite regulating factors in pacu (Piaractus mesopotamicus): Tissue distribution and effects of food quantity and quality on gene expression. Comp Biochem Physiol Part A: Mol Integr Physiol (2017) 203:241–54. doi: 10.1016/j.cbpa.2016.09.022
81. Kling P, Rønnestad I, Stefansson SO, Murashita K, Kurokawa T, Björnsson BT. A homologous salmonid leptin radioimmunoassay indicates elevated plasma leptin levels during fasting of rainbow trout. Gen Comp Endocrinol (2009) 162:307–12. doi: 10.1016/j.ygcen.2009.04.003
82. Johansson M, Björnsson BT. Elevated plasma leptin levels of fasted rainbow trout decrease rapidly in response to feed intake. Gen Comp Endocrinol (2015) 214:24–9. doi: 10.1016/j.ygcen.2015.02.020
83. Fuentes EN, Kling P, Einarsdottir IE, Alvarez M, Valdés JA, Molina A, et al. Plasma leptin and growth hormone levels in the fine flounder (Paralichthys adspersus) increase gradually during fasting and decline rapidly after refeeding. Gen Comp Endocrinol (2012) 177:120–7. doi: 10.1016/j.ygcen.2012.02.019
84. Nieminen P, Mustonen A-M, Hyvärinen H. Fasting reduces plasma leptin- and ghrelin-immunoreactive peptide concentrations of the burbot (Lota lota) at 2°C but not at 10°C. jzoo (2003) 20:1109–15. doi: 10.2108/zsj.20.1109
85. Dar SA, Srivastava PP, Rather MA, Varghese T, Rasool SI, Gupta S. Molecular and computational analysis of ghrelin, growth hormone secretagogues receptor and mRNA expression of growth-related genes after exogenous administered ghrelin peptide in labeo rohita. Int J Biol Macromol (2020) 142:756–68. doi: 10.1016/j.ijbiomac.2019.10.016
86. Mankiewicz JL, Cleveland BM. Characterization of a leptin receptor paralog and its response to fasting in rainbow trout (Oncorhynchus mykiss). Int J Mol Sci (2021) 22:7732. doi: 10.3390/ijms22147732
87. Coccia E, Varricchio E, Vito P, Turchini GM, Francis DS, Paolucci M. Fatty acid-specific alterations in leptin, PPARα, and CPT-1 gene expression in the rainbow trout. Lipids (2014) 49:1033–46. doi: 10.1007/s11745-014-3939-y
89. Angotzi AR, Stefansson SO, Nilsen TO, JanI Ø, Andersson E, Taranger GL, et al. Identification of a novel leptin receptor duplicate in Atlantic salmon: Expression analyses in different life stages and in response to feeding status. Gen Comp Endocrinol (2016) 235:108–19. doi: 10.1016/j.ygcen.2016.06.004
90. Zabeau L, Lavens D, Peelman F, Eyckerman S, Vandekerckhove J, Tavernier J. The ins and outs of leptin receptor activation. FEBS Lett (2003) 546:45–50. doi: 10.1016/S0014-5793(03)00440-X
91. Stern JH, Rutkowski JM, Scherer PE. Adiponectin, leptin, and fatty acids in the maintenance of metabolic homeostasis through adipose tissue crosstalk. Cell Metab (2016) 23:770–84. doi: 10.1016/j.cmet.2016.04.011
92. Satoh-Asahara N, Ogawa Y, Katsuura G, Numata Y, Tsuji T, Hayase M, et al. Sympathetic activation of leptin via the ventromedial hypothalamus - leptin-induced increase in catecholamine secretion. Diabetes (1999) 48:1787–93. doi: 10.2337/diabetes.48.9.1787
93. Harris M, Aschkenasi C, Elias CF, Chandrankunnel A, Nillni EA, Bjørbæk C, et al. Transcriptional regulation of the thyrotropin-releasing hormone gene by leptin and melanocortin signaling. J Clin Invest (2001) 107:111–20. doi: 10.1172/JCI10741
94. Ortiga-Carvalho T, Oliveira K, Soares B, Pazos-Moura C. The role of leptin in the regulation of TSH secretion in the fed state: in vivo and in vitro studies. J Endocrinol (2002) 174:121–5. doi: 10.1677/joe.0.1740121
95. Seoane LM, Carro E, Tovar S, Casanueva FF, Dieguez C. Regulation of in vivo TSH secretion by leptin. Regul Peptides (2000) 92:25–9. doi: 10.1016/S0167-0115(00)00145-2
96. Murashita K, Uji S, Yamamoto T, Rønnestad I, Kurokawa T. Production of recombinant leptin and its effects on food intake in rainbow trout (Oncorhynchus mykiss). Comp Biochem Physiol Part B: Biochem Mol Biol (2008) 150:377–84. doi: 10.1016/j.cbpb.2008.04.007
97. Chisada S, Kurokawa T, Murashita K, Rønnestad I, Taniguchi Y, Toyoda A, et al. Leptin receptor-deficient (knockout) medaka, oryzias latipes, show chronical up-regulated levels of orexigenic neuropeptides, elevated food intake and stage specific effects on growth and fat allocation. Gen Comp Endocrinol (2014) 195:9–20. doi: 10.1016/j.ygcen.2013.10.008
98. Michel M, Page-McCaw PS, Chen W, Cone RD. Leptin signaling regulates glucose homeostasis, but not adipostasis, in the zebrafish. Proc Natl Acad Sci (2016) 113:3084–9. doi: 10.1073/pnas.1513212113
99. Del Vecchio G, Murashita K, Verri T, Gomes AS, Rønnestad I. Leptin receptor-deficient (knockout) zebrafish: Effects on nutrient acquisition. Gen Comp Endocrinol (2021) 310:113832. doi: 10.1016/j.ygcen.2021.113832
100. Mankiewicz JL, Picklo MJ, Idso J, Cleveland BM. Leptin receptor deficiency results in hyperphagia and increased fatty acid mobilization during fasting in rainbow trout (Oncorhynchus mykiss). Biomolecules (2022) 12:516. doi: 10.3390/biom12040516
101. Dyck DJ. Leptin sensitivity in skeletal muscle is modulated by diet and exercise. Exercise Sport Sci Rev (2005) 33:189–94. doi: 10.1097/00003677-200510000-00007
102. Gong N, Johansson M, Björnsson BT. Impaired central leptin signaling and sensitivity in rainbow trout with high muscle adiposity. Gen Comp Endocrinol (2016) 235:48–56. doi: 10.1016/j.ygcen.2016.06.013
103. Chen XX, Yang T. Roles of leptin in bone metabolism and bone diseases. J Bone Miner Metab (2015) 33:474–85. doi: 10.1007/s00774-014-0569-7
104. Reid IR, Baldock PA, Cornish J. Effects of leptin on the skeleton. Endocrine Rev (2018) 39:938–59. doi: 10.1210/er.2017-00226
105. Capilla E, Teles-García Á, Acerete L, Navarro I, Gutiérrez J. Insulin and IGF-I effects on the proliferation of an osteoblast primary culture from sea bream (Sparus aurata). Gen Comp Endocrinol (2011) 172:107–14. doi: 10.1016/j.ygcen.2011.03.020
106. Riera-Heredia N, Lutfi E, Gutiérrez J, Navarro I, Capilla E. Fatty acids from fish or vegetable oils promote the adipogenic fate of mesenchymal stem cells derived from gilthead sea bream bone potentially through different pathways. PloS One (2019) 14:e0215926. doi: 10.1371/journal.pone.0215926
107. Steppan CM, Lazar MA. The current biology of resistin. J Internal Med (2004) 255:439–47. doi: 10.1111/j.1365-2796.2004.01306.x
108. Codoñer-Franch P, Alonso-Iglesias E. Resistin: Insulin resistance to malignancy. Clin Chim Acta (2015) 438:46–54. doi: 10.1016/j.cca.2014.07.043
109. Galic S, Oakhill JS, Steinberg GR. Adipose tissue as an endocrine organ. Mol Cell Endocrinol (2010) 316:129–39. doi: 10.1016/j.mce.2009.08.018
110. Acquarone E, Monacelli F, Borghi R, Nencioni A, Odetti P. Resistin: A reappraisal. Mech Ageing Dev (2019) 178:46–63. doi: 10.1016/j.mad.2019.01.004
111. Taouis M, Benomar Y. Is resistin the master link between inflammation and inflammation-related chronic diseases? Mol Cell Endocrinol (2021) 533:111341. doi: 10.1016/j.mce.2021.111341
112. Hu Q, Tan H, Irwin DM. Evolution of the vertebrate resistin gene family. PloS One (2015) 10:e0130188. doi: 10.1371/journal.pone.0130188
113. Sethi JK, Hotamisligil GS. The role of TNFα in adipocyte metabolism. Semin Cell Dev Biol (1999) 10:19–29. doi: 10.1006/scdb.1998.0273
114. Li VL, Kim JT, Long JZ. Adipose tissue lipokines: Recent progress and future directions. Diabetes (2020) 69:2541–8. doi: 10.2337/dbi20-0012
115. Goetz FW, Planas JV, MacKenzie S. Tumor necrosis factors. Dev Comp Immunol (2004) 28:487–97. doi: 10.1016/j.dci.2003.09.008
116. Uenobe M, Kohchi C, Yoshioka N, Yuasa A, Inagawa H, Morii K, et al. Cloning and characterization of a TNF-like protein of plecoglossus altivelis (ayu fish). Mol Immunol (2007) 44:1115–22. doi: 10.1016/j.molimm.2006.07.281
117. Wiens GD, Glenney GW. Origin and evolution of TNF and TNF receptor superfamilies. Dev Comp Immunol (2011) 35:1324–35. doi: 10.1016/j.dci.2011.03.031
118. Kajungiro RA, Xue L, Aynealem M. Molecular cloning and expression patterns of two tumor necrosis factor alpha genes in crucian carp (Carassius carassius). Mol Biol (2015) 49:120–9. doi: 10.1134/S0026893315010021
119. Laing KJ, Wang T, Zou J, Holland J, Hong S, Bols N, et al. Cloning and expression analysis of rainbow trout Oncorhynchus mykiss tumour necrosis factor-α: Rainbow trout tumour necrosis factor-α. Eur J Biochem (2001) 268:1315–22. doi: 10.1046/j.1432-1327.2001.01996.x
120. Zou J, Wang T, Hirono I, Aoki T, Inagawa H, Honda T. Differential expression of two tumor necrosis factor genes in rainbow trout, oncorhynchus mykiss. Dev Comp Immunol (2002) 12:161‐72. doi: 10.1016/S0145-305X(01)00058-1
121. Savan R, Sakai M. Presence of multiple isoforms of TNF alpha in carp (Cyprinus carpio l.): genomic and expression analysis. Fish Shellfish Immunol (2004) 17:87–94. doi: 10.1016/j.fsi.2003.11.001
122. Hong S, Li R, Xu Q, Secombes CJ, Wang T. Two types of TNF-α exist in teleost fish: Phylogeny, expression, and bioactivity analysis of type-II TNF-α3 in rainbow trout. Oncorhynchus Mykiss JI (2013) 191:5959–72. doi: 10.4049/jimmunol.1301584
123. Hirono I, Nam B-H, Kurobe T, Aoki T. Molecular cloning, characterization, and expression of TNF cDNA and gene from Japanese flounder paralychthys olivaceus1. J Immunol (2000) 165:4423–7. doi: 10.4049/jimmunol.165.8.4423
124. Garca-Castillo J, Pelegrn P, Mulero V, Meseguer J. Molecular cloning and expression analysis of tumor necrosis factor from a marine fish reveal its constitutive expression and ubiquitous nature. Immunogenetics (2002) 3:200–7. doi: 10.1007/s00251-002-0451-y
125. Saeij JPJ, Stet RJM, de Vries BJ, van Muiswinkel WB, Wiegertjes GF. Molecular and functional characterization of carp TNF: a link between TNF polymorphism and trypanotolerance? Dev Comp Immunol (2003) 27:29–41. doi: 10.1016/S0145-305X(02)00064-2
126. Bobe J, Goetz FW. Molecular cloning and expression of a TNF receptor and two TNF ligands in the fish ovary. Comp Biochem Physiol Part B: Biochem Mol Biol (2001) 129:475–81. doi: 10.1016/S1096-4959(01)00353-0
127. Li F, Li Y, Duan Y, Hu C-AA, Tang Y, Yin Y. Myokines and adipokines: Involvement in the crosstalk between skeletal muscle and adipose tissue. Cytokine Growth Factor Rev (2017) 33:73–82. doi: 10.1016/j.cytogfr.2016.10.003
128. Bulló-Bonet M, García-Lorda P, López-Soriano FJ, Argilés JM, Salas-Salvadó J. Tumour necrosis factor, a key role in obesity? FEBS Lett (1999) 451:215–9. doi: 10.1016/S0014-5793(99)00540-2
129. Li Y, Xiao T, Zou J. Fish TNF and TNF receptors. Sci China Life Sci (2021) 64:196–220. doi: 10.1007/s11427-020-1712-4
130. Hotamisligil GS, Peraldi P, Budavari A, Ellis R, White MF, Spiegelman BM. IRS-1-Mediated inhibition of insulin receptor tyrosine kinase activity in TNF-α- and obesity-induced insulin resistance. Science (1996) 271:665–70. doi: 10.1126/science.271.5249.665
131. Weisberg SP, McCann D, Desai M, Rosenbaum M, Leibel RL, Ferrante AW. Obesity is associated with macrophage accumulation in adipose tissue. J Clin Invest (2003) 112:1796–808. doi: 10.1172/JCI19246
132. Sethi JK, Hotamisligil GS. Metabolic messengers: tumour necrosis factor. Nat Metab (2021) 3:1302–12. doi: 10.1038/s42255-021-00470-z
133. Secombes CJ, Wang T, Hong S, Peddie S, Crampe M, Laing KJ, et al. Cytokines and innate immunity of fish. Dev Comp Immunol (2001) 25:713–23. doi: 10.1016/S0145-305X(01)00032-5
134. Lam FW-S, Wu S-Y, Lin S-J, Lin C-C, Chen Y-M, Wang H-C, et al. The expression of two novel orange-spotted grouper (Epinephelus coioides) TNF genes in peripheral blood leukocytes, various organs, and fish larvae. Fish Shellfish Immunol (2011) 30:618–29. doi: 10.1016/j.fsi.2010.12.011
135. Nelson CM, Ackerman KM, O’Hayer P, Bailey TJ, Gorsuch RA, Hyde DR. Tumor necrosis factor-alpha is produced by dying retinal neurons and is required for müller glia proliferation during zebrafish retinal regeneration. J Neurosci (2013) 33:6524–39. doi: 10.1523/JNEUROSCI.3838-12.2013
136. Zou J, Secombes CJ. The function of fish cytokines. Biology (2016) 5:23. doi: 10.3390/biology5020023
137. Liu D, Mai K, Zhang Y, Xu W, Ai Q. Tumour necrosis factor-α inhibits hepatic lipid deposition through GSK-3β/β-catenin signaling in juvenile turbot (Scophthalmus maximus l.). Gen Comp Endocrinol (2016) 228:1–8. doi: 10.1016/j.ygcen.2015.12.027
138. Zou J, Peddie S, Scapigliati G, Zhang Y, Bols NC, Ellis AE, et al. Functional characterisation of the recombinant tumor necrosis factors in rainbow trout, oncorhynchus mykiss. Dev Comp Immunol (2003) 27:813–22. doi: 10.1016/S0145-305X(03)00077-6
139. Yang X, Zhang X, Heckmann BL, Lu X, Liu J. Relative contribution of adipose triglyceride lipase and hormone-sensitive lipase to tumor necrosis factor-α (TNF-α)-induced lipolysis in adipocytes *. J Biol Chem (2011) 286:40477–85. doi: 10.1074/jbc.M111.257923
140. Kralisch S, Klein J, Lossner U, Bluher M, Paschke R, Stumvoll M, et al. Hormonal regulation of the novel adipocytokine visfatin in 3T3-L1 adipocytes. J Endocrinol (2005) 185:R1–8. doi: 10.1677/joe.1.06211
141. Kim H-K, Della-Fera M, Lin J, Baile CA. Docosahexaenoic acid inhibits adipocyte differentiation and induces apoptosis in 3T3-L1 preadipocytes. J Nutr (2006) 136:2965–9. doi: 10.1093/jn/136.12.2965
142. Deiuliis JA, Shin J, Bae D, Azain MJ, Barb R, Lee K. Developmental, hormonal, and nutritional regulation of porcine adipose triglyceride lipase (ATGL). Lipids (2008) 43:215–25. doi: 10.1007/s11745-007-3146-1
143. Dai Y-J, Liu W-B, Li X-F, Zhou M, Xu C, Qian Y, et al. Molecular cloning of adipose triglyceride lipase (ATGL) gene from blunt snout bream and its expression after LPS-induced TNF-α factor. Fish Physiol Biochem (2018) 44:1143–57. doi: 10.1007/s10695-018-0502-4
144. Wallach D, Varfolomeev EE, Malinin NL, Goltsev YV, Kovalenko AV, Boldin MP. Tumor necrosis factor receptor and fas signaling. Annu Rev Immunol (1999) 17:331–67. doi: 10.1146/annurev.immunol.17.1.331
145. Greenberg AS, Shen W-J, Muliro K, Patel S, Souza SC, Roth RA, et al. Stimulation of lipolysis and hormone-sensitive lipase via the extracellular signal-regulated kinase pathway*. J Biol Chem (2001) 276:45456–61. doi: 10.1074/jbc.M104436200
146. Zhang F, Lavan B, Gregoire FM. Peroxisome proliferator-activated receptors as attractive antiobesity targets. Drug News Perspect (2004) 17:661–9. doi: 10.1358/dnp.2004.17.10.873918
147. Yoon M. The role of PPARα in lipid metabolism and obesity: Focusing on the effects of estrogen on PPARα actions. Pharmacol Res (2009) 60:151–9. doi: 10.1016/j.phrs.2009.02.004
148. Cawthorn WP, Sethi JK. TNF-α and adipocyte biology. FEBS Lett (2008) 582:117–31. doi: 10.1016/j.febslet.2007.11.051
149. Doerrler W, Feingold KR, Grunfeld C. Cytokines induce catabolic effects in cultured adipocytes by multiple mechanisms. Cytokine (1994) 6:478–84. doi: 10.1016/1043-4666(94)90074-4
150. Gao Y, Dai Z, Shi C, Zhai G, Jin X, He J, et al. Depletion of myostatin b promotes somatic growth and lipid metabolism in zebrafish. Front Endocrinol (2016) 7:88. doi: 10.3389/fendo.2016.00088
151. Porter MH, Cutchins A, Fine JB, Bai Y, Digirolamo M. Effects of TNF-α on glucose metabolism and lipolysis in adipose tissue and isolated fat-cell preparations. J Lab Clin Med (2002) 139:140–6. doi: 10.1067/mlc.2002.121552
152. Fernández-Veledo S, Vila-Bedmar R, Nieto-Vazquez I, Lorenzo M. C-jun n-terminal kinase 1/2 activation by tumor necrosis factor-α induces insulin resistance in human visceral but not subcutaneous adipocytes: Reversal by liver X receptor agonists. J Clin Endocrinol Metab (2009) 94:3583–93. doi: 10.1210/jc.2009-0558
153. Stephens JM, Lee J, Pilch PF. Tumor necrosis factor-α-induced insulin resistance in 3T3-L1 adipocytes is accompanied by a loss of insulin receptor substrate-1 and GLUT4 expression without a loss of insulin receptor-mediated signal transduction *. J Biol Chem (1997) 272:971–6. doi: 10.1074/jbc.272.2.971
154. Xu H, Sethi JK, Hotamisligil GS. Transmembrane tumor necrosis factor (TNF)-α inhibits adipocyte differentiation by selectively activating TNF receptor 1*. J Biol Chem (1999) 274:26287–95. doi: 10.1074/jbc.274.37.26287
155. Grohmann M, Sabin M, Holly J, Shield J, Crowne E, Stewart C. Characterization of differentiated subcutaneous and visceral adipose tissue from children: the influences of TNF-α and IGF-I. J Lipid Res (2005) 46:93–103. doi: 10.1194/jlr.M400295-JLR200
156. Xing H, Northrop JP, Grove JR, Kilpatrick KE, Su J-L, Ringold GM. TNFα-mediated inhibition and reversal of adipocyte differentiation is accompanied by suppressed expression of PPARγ without effects on pref-1 expression*. Endocrinology (1997) 138:2776–83. doi: 10.1210/endo.138.7.5242
157. Kras KM, Hausman DB, Martin RJ. Tumor necrosis factor-α stimulates cell proliferation in adipose tissue-derived stromal-vascular cell culture: Promotion of adipose tissue expansion by paracrine growth factors. Obes Res (2000) 8:186–93. doi: 10.1038/oby.2000.20
158. Nisoli E, Briscini L, Tonello C, De Giuli-Morghen C, Carruba MO. Tumor necrosis factor-α induces apoptosis in rat brown adipocytes. Cell Death Differ (1997) 4:771–8. doi: 10.1038/sj.cdd.4400292
159. Porras A, Álvarez AM, Valladares A, Benito M. TNF-α induces apoptosis in rat fetal brown adipocytes in primary culture. FEBS Lett (1997) 416:324–8. doi: 10.1016/S0014-5793(97)01204-0
160. Dietze-Schroeder D, Sell H, Uhlig M, Koenen M, Eckel J. Autocrine action of adiponectin on human fat cells prevents the release of insulin resistance-inducing factors. Diabetes (2005) 54:2003–11. doi: 10.2337/diabetes.54.7.2003
161. Bruun JM, Lihn AS, Verdich C, Pedersen SB, Toubro S, Astrup A, et al. Regulation of adiponectin by adipose tissue-derived cytokines: in vivo and in vitro investigations in humans. Am J Physiol-Endocrinol Metab (2003) 285:E527–33. doi: 10.1152/ajpendo.00110.2003
162. Volkoff H, Wyatt JL. Apelin in goldfish (Carassius auratus): Cloning, distribution and role in appetite regulation. Peptides (2009) 30:1434–40. doi: 10.1016/j.peptides.2009.04.020
163. Lin F, Wu H, Chen H, Xin Z, Yuan D, Wang T, et al. Molecular and physiological evidences for the role in appetite regulation of apelin and its receptor APJ in ya-fish (Schizothorax prenanti). Mol Cell Endocrinol (2014) 396:46–57. doi: 10.1016/j.mce.2014.08.009
164. Assan D, Huang Y, Mustapha UF, Addah MN, Li G, Chen H. Fish feed intake, feeding behavior, and the physiological response of apelin to fasting and refeeding. Front Endocrinol (Lausanne) (2021) 12:798903. doi: 10.3389/fendo.2021.798903
165. Lee D-E, Kehlenbrink S, Lee H, Hawkins M, Yudkin JS. Getting the message across: mechanisms of physiological cross talk by adipose tissue. Am J Physiol-Endocrinol Metab (2009) 296:E1210–29. doi: 10.1152/ajpendo.00015.2009
166. Pham DV, Park P-H. Recent insights on modulation of inflammasomes by adipokines: a critical event for the pathogenesis of obesity and metabolism-associated diseases. Arch Pharmacal Res (2020) 43:997–1016. doi: 10.1007/s12272-020-01274-7
167. Kong HJ, Hong G-E, Nam B-H, Kim Y-O, Kim W-J, Lee S-J, et al. An immune responsive complement factor d/adipsin and kallikrein-like serine protease (PoDAK) from the olive flounder paralichthys olivaceus. Fish Shellfish Immunol (2009) 27:486–92. doi: 10.1016/j.fsi.2009.06.022
168. Xu D, Shiping Z, Xiaoxiong W, Hanchuan D, Cuiping Z, Xu W, et al. cDNA structure, genomic organization and expression patterns of visfatin in silver Prussian carp (Carassius auratus gibelio). Afr J Biotechnol (2011) 10:16703–106714. doi: 10.4314/%u.v10i74.%c
169. Todorčević M, Škugor S, Krasnov A, Ruyter B. Gene expression profiles in Atlantic salmon adipose-derived stromo-vascular fraction during differentiation into adipocytes. BMC Genomics (2010) 11:39. doi: 10.1186/1471-2164-11-39
170. Cao H, Gerhold K, Mayers JR, Wiest MM, Watkins SM, Hotamisligil GS. Identification of a lipokine, a lipid hormone linking adipose tissue to systemic metabolism. Cell (2008) 134:933–44. doi: 10.1016/j.cell.2008.07.048
171. Hernández-Saavedra D, Stanford KI. The regulation of lipokines by environmental factors. Nutrients (2019) 11:2422. doi: 10.3390/nu11102422
172. Stanford KI, Lynes MD, Takahashi H, Baer LA, Arts PJ, May FJ, et al. 12,13-diHOME: An exercise-induced lipokine that increases skeletal muscle fatty acid uptake. Cell Metab (2018) 27:1111–1120.e3. doi: 10.1016/j.cmet.2018.03.020
173. Roberts LD, Boström P, O’Sullivan JF, Schinzel RT, Lewis GD, Dejam A, et al. β-aminoisobutyric acid induces browning of white fat and hepatic β-oxidation and is inversely correlated with cardiometabolic risk factors. Cell Metab (2014) 19:96–108. doi: 10.1016/j.cmet.2013.12.003
174. Lange M, Angelidou G, Ni Z, Criscuolo A, Schiller J, Blüher M, et al. AdipoAtlas: A reference lipidome for human white adipose tissue. Cell Rep Med (2021) 2:100407. doi: 10.1016/j.xcrm.2021.100407
175. Gesta S, Tseng Y-H, Kahn CR. Developmental origin of fat: Tracking obesity to its source. Cell (2007) 131:242–56. doi: 10.1016/j.cell.2007.10.004
177. Dreier DA, Bowden JA, Aristizabal-Henao JJ, Denslow ND, Martyniuk CJ. Ecotoxico-lipidomics: An emerging concept to understand chemical-metabolic relationships in comparative fish models. Comp Biochem Physiol Part D: Genomics Proteomics (2020) 36:100742. doi: 10.1016/j.cbd.2020.100742
178. Jin Y, Harvey TN, Bartosova Z, Hassani S, Bruheim P, Sandve SR, et al. Diet and life stage-associated lipidome remodeling in Atlantic salmon. J Agric Food Chem (2021) 69:3787–96. doi: 10.1021/acs.jafc.0c07281
179. Giudice J, Taylor JM. Muscle as a paracrine and endocrine organ. Curr Opin Pharmacol (2017) 34:49–55. doi: 10.1016/j.coph.2017.05.005
180. Rodriguez J, Vernus B, Chelh I, Cassar-Malek I, Gabillard JC, Hadj Sassi A, et al. Myostatin and the skeletal muscle atrophy and hypertrophy signaling pathways. Cell Mol Life Sci (2014) 71:4361–71. doi: 10.1007/s00018-014-1689-x
181. McPherron AC, Lawler AM, Lee S-J. Regulation of skeletal muscle mass in mice by a new TGF-p superfamily member. Nature (1997) 387:83–90. doi: 10.1038/387083a0
182. Grobet L, Royo Martin LJ, Poncelet D, Pirottin D, Brouwers B, Riquet J, et al. A deletion in the bovine myostatin gene causes the double–muscled phenotype in cattle. Nat Genet (1997) 17:71–4. doi: 10.1038/ng0997-71
183. Kim WK, Choi H-R, Park SG, Ko Y, Bae K-H, Lee SC. Myostatin inhibits brown adipocyte differentiation via regulation of Smad3-mediated β-catenin stabilization. Int J Biochem Cell Biol (2012) 44:327–34. doi: 10.1016/j.biocel.2011.11.004
184. Braga M, Pervin S, Norris K, Bhasin S, Singh R. Inhibition of in vitro and in vivo brown fat differentiation program by myostatin. Obesity (2013) 21:1180–8. doi: 10.1002/oby.20117
185. Gabillard J-C, Biga PR, Rescan P-Y, Seiliez I. Revisiting the paradigm of myostatin in vertebrates: Insights from fishes. Gen Comp Endocrinol (2013) 194:45–54. doi: 10.1016/j.ygcen.2013.08.012
186. Rescan P-Y, Jutel I, Rallière C. Two myostatin genes are differentially expressed in myotomal muscles of the trout (Oncorhynchus mykiss). J Exp Biol (2001) 204:3523–9. doi: 10.1242/jeb.204.20.3523
187. Garikipati DK, Gahr SA, Roalson EH, Rodgers BD. Characterization of rainbow trout myostatin-2 genes (rtMSTN-2a and -2b): Genomic organization, differential expression, and pseudogenization. Endocrinology (2007) 148:2106–15. doi: 10.1210/en.2006-1299
188. Helterline DLI, Garikipati D, Stenkamp DL, Rodgers BD. Embryonic and tissue-specific regulation of myostatin-1 and -2 gene expression in zebrafish. Gen Comp Endocrinol (2007) 151:90–7. doi: 10.1016/j.ygcen.2006.12.023
189. Meyer Berger JM, Singh P, Khrimian L, Morgan DA, Chowdhury S, Arteaga-Solis E, et al. Mediation of the acute stress response by the skeleton. Cell Metab (2019) 30:890–902.e8. doi: 10.1016/j.cmet.2019.08.012
190. Langley B, Thomas M, Bishop A, Sharma M, Gilmour S, Kambadur R. Myostatin inhibits myoblast differentiation by down-regulating MyoD expression *. J Biol Chem (2002) 277:49831–40. doi: 10.1074/jbc.M204291200
191. Zhong Z, Niu P, Wang M, Huang G, Xu S, Sun Y, et al. Targeted disruption of sp7 and myostatin with CRISPR-Cas9 results in severe bone defects and more muscular cells in common carp. Sci Rep (2016) 6:22953. doi: 10.1038/srep22953
192. Sun Y, Zheng G-D, Nissa M, Chen J, Zou S-M. Disruption of mstna and mstnb gene through CRISPR/Cas9 leads to elevated muscle mass in blunt snout bream (Megalobrama amblycephala). Aquaculture (2020) 528:735597. doi: 10.1016/j.aquaculture.2020.735597
193. Kim J, Cho JY, Kim J-W, Kim H-C, Noh JK, Kim Y-O, et al. CRISPR/Cas9-mediated myostatin disruption enhances muscle mass in the olive flounder paralichthys olivaceus. Aquaculture (2019) 512:734336. doi: 10.1016/j.aquaculture.2019.734336
194. Coogan M, Alston V, Su B, Khalil K, Elaswad A, Khan M, et al. CRISPR/Cas-9 induced knockout of myostatin gene improves growth and disease resistance in channel catfish (Ictalurus punctatus). Aquaculture (2022) 557:738290. doi: 10.1016/j.aquaculture.2022.738290
195. Tao B, Tan J, Chen L, Xu Y, Liao X, Li Y, et al. CRISPR/Cas9 system-based myostatin-targeted disruption promotes somatic growth and adipogenesis in loach, misgurnus anguillicaudatus. Aquaculture (2021) 544:737097. doi: 10.1016/j.aquaculture.2021.737097
196. Hamrick M, Bowser M, Fulzele S, Ahsan S, Arounleut P, Isales CM. Changes in the activin a-myostatin-follistatin system within bone and muscle of aging mice. FASEB J (2012) 26:914.4–4. doi: 10.1096/fasebj.26.1_supplement.914.4
197. Bonewald L. Use it or lose it to age: A review of bone and muscle communication. Bone (2019) 120:212–8. doi: 10.1016/j.bone.2018.11.002
198. Li G, Zhang L, Wang D, AIQudsy L, Jiang JX, Xu H, et al. Muscle-bone crosstalk and potential therapies for sarco-osteoporosis. J Cell Biochem (2019) 120:14262–73. doi: 10.1002/jcb.28946
199. Lavajoo F, Perelló-Amorós M, Vélez EJ, Sánchez-Moya A, Balbuena-Pecino S, Riera-Heredia N, et al. Regulatory mechanisms involved in muscle and bone remodeling during refeeding in gilthead sea bream. Sci Rep (2020) 10:184. doi: 10.1038/s41598-019-57013-6
200. Otero-Tarrazón A, Perelló-Amorós M, Jorge-Pedraza V, Moshayedi F, Sánchez-Moya A, García-Pérez I, et al. Muscle regeneration in gilthead sea bream: implications of endocrine and local regulatory factors and the crosstalk with bone. Front Endocrinol (2023) 14:1101356. doi: 10.3389/fendo.2023.1101356
201. de Mello F, Streit DP, Sabin N, Gabillard J-C. Identification of TGF-β, inhibin βA and follistatin paralogs in the rainbow trout genome. Comp Biochem Physiol Part B: Biochem Mol Biol (2014) 177–178:46–55. doi: 10.1016/j.cbpb.2014.07.006
202. Funkenstein B, Olekh E, Jakowlew SB. Identification of a novel transforming growth factor-β (TGF-β6) gene in fish: regulation in skeletal muscle by nutritional state. BMC Mol Biol (2010) 11:37. doi: 10.1186/1471-2199-11-37
203. Lee S-J, Lee Y-S, Zimmers TA, Soleimani A, Matzuk MM, Tsuchida K, et al. Regulation of muscle mass by follistatin and activins. Mol Endocrinol (2010) 24:1998–2008. doi: 10.1210/me.2010-0127
204. Che J, Hu C, Wang Q, Fan C, Si Y, Gong X, et al. The double mutations of acvr2aa and acvr2ba leads to muscle hypertrophy in zebrafish. Aquacult Fisheries (2022). doi: 10.1016/j.aaf.2022.03.007
205. Wood A, Duan C, Bern H. Insulin-like growth factor signaling in fish. Int Rev Cytol (2005) 243:215. doi: 10.1016/S0074-7696(05)43004-1
206. Sjögren K, Liu J-L, Blad K, Skrtic S, Vidal O, Wallenius V, et al. Liver-derived insulin-like growth factor I (IGF-I) is the principal source of IGF-I in blood but is not required for postnatal body growth in mice. Proc Natl Acad Sci (1999) 96:7088–92. doi: 10.1073/pnas.96.12.7088
207. Coleman ME, DeMayo F, Yin KC, Lee HM, Geske R, Montgomery C, et al. Myogenic vector expression of insulin-like growth factor I stimulates muscle cell differentiation and myofiber hypertrophy in transgenic mice *. J Biol Chem (1995) 270:12109–16. doi: 10.1074/jbc.270.20.12109
208. Fiorotto ML, Schwartz RJ, Delaughter MC. Persistent IGF-I overexpression in skeletal muscle transiently enhances DNA accretion and growth1. FASEB J (2003) 17:59–60. doi: 10.1096/fj.02-0289fje
209. Jiménez-Amilburu V, Salmerón C, Codina M, Navarro I, Capilla E, Gutiérrez J. Insulin-like growth factors effects on the expression of myogenic regulatory factors in gilthead sea bream muscle cells. Gen Comp Endocrinol (2013) 188:151–8. doi: 10.1016/j.ygcen.2013.02.033
210. Duan C, Hirano T. Effects of insulin-like growth factor-I and insulin on the in-vitro uptake of sulphate by eel branchial cartilage: evidence for the presence of independent hepatic and pancreatic sulphation factors. J Endocrinol (1992) 133:211–9. doi: 10.1677/joe.0.1330211
211. Duan C, Plisetskaya EM. Nutritional regulation of insulin-like growth factor-I mRNA expression in salmon tissues. J Endocrinol (1993) 139:243–52. doi: 10.1677/joe.0.1390243
212. Matthews SJ, Kinhult AKK, Hoeben P, Sara VR, Anderson TA. Nutritional regulation of insulin-like growth factor-I mRNA expression in barramundi, lates calcarifer. J Mol Endocrinol (1997) 18:273–6. doi: 10.1677/jme.0.0180273
213. Peterson BC, Waldbieser GC. Effects of fasting on IGF-I, IGF-II, and IGF-binding protein mRNA concentrations in channel catfish (Ictalurus punctatus). Domest Anim Endocrinol (2009) 37:74–83. doi: 10.1016/j.domaniend.2009.03.004
214. Uchida K, Kajimura S, Riley LG, Hirano T, Aida K, Grau EG. Effects of fasting on growth hormone/insulin-like growth factor I axis in the tilapia, oreochromis mossambicus. Comp Biochem Physiol Part A: Mol Integr Physiol (2003) 134:429–39. doi: 10.1016/S1095-6433(02)00318-5
215. Chauvigné F, Gabillard JC, Weil C, Rescan PY. Effect of refeeding on IGFI, IGFII, IGF receptors, FGF2, FGF6, and myostatin mRNA expression in rainbow trout myotomal muscle. Gen Comp Endocrinol (2003) 132:209–15. doi: 10.1016/S0016-6480(03)00081-9
216. Montserrat N, Gabillard JC, Capilla E, Navarro MI, Gutiérrez J. Role of insulin, insulin-like growth factors, and muscle regulatory factors in the compensatory growth of the trout (Oncorhynchus mykiss). Gen Comp Endocrinol (2007) 150:462–72. doi: 10.1016/j.ygcen.2006.11.009
217. Fox BK, Breves JP, Davis LK, Pierce AL, Hirano T, Grau EG. Tissue-specific regulation of the growth hormone/insulin-like growth factor axis during fasting and re-feeding: Importance of muscle expression of IGF-I and IGF-II mRNA in the tilapia. Gen Comp Endocrinol (2010) 166:573–80. doi: 10.1016/j.ygcen.2009.11.012
218. Nebo C, Portella MC, Carani FR, de Almeida FLA, Padovani CR, Carvalho RF, et al. Short periods of fasting followed by refeeding change the expression of muscle growth-related genes in juvenile Nile tilapia (Oreochromis niloticus). Comp Biochem Physiol Part B: Biochem Mol Biol (2013) 164:268–74. doi: 10.1016/j.cbpb.2013.02.003
219. Gabillard J-C, Weil C, Rescan P-Y, Navarro I, Gutiérrez J, Le Bail P-Y. Effects of environmental temperature on IGF1, IGF2, and IGF type I receptor expression in rainbow trout (Oncorhynchus mykiss). Gen Comp Endocrinol (2003) 133:233–42. doi: 10.1016/S0016-6480(03)00167-9
220. Vélez EJ, Azizi S, Millán-Cubillo A, Fernández-Borràs J, Blasco J, Chan SJ, et al. Effects of sustained exercise on GH-IGFs axis in gilthead sea bream (Sparus aurata). Am J Physiol-Regulatory Integr Comp Physiol (2016) 310:R313–22. doi: 10.1152/ajpregu.00230.2015
221. Palstra AP, Tudorache C, Rovira M, Brittijn SA, Burgerhout E, van den TGEEJM, et al. Establishing zebrafish as a novel exercise model: Swimming economy, swimming-enhanced growth and muscle growth marker gene expression. PloS One (2010) 5:e14483. doi: 10.1371/journal.pone.0014483
222. Li D, Lou Q, Zhai G, Peng X, Cheng X, Dai X, et al. Hyperplasia and cellularity changes in IGF-1-Overexpressing skeletal muscle of crucian carp. Endocrinology (2014) 155:2199–212. doi: 10.1210/en.2013-1938
223. Garcia de la Serrana D, Macqueen DJ. Insulin-like growth factor-binding proteins of teleost fishes. Front Endocrinol (2018) 9. doi: 10.3389/fendo.2018.00080
224. Tanaka T, Narazaki M, Ogata A, Kishimoto T. A new era for the treatment of inflammatory autoimmune diseases by interleukin-6 blockade strategy. Semin Immunol (2014) 26:88–96. doi: 10.1016/j.smim.2014.01.009
225. Pedersen BK. Muscle as a secretory organ. In: Comprehensive physiology. John Wiley & Sons, Ltd (2013) 3:1337–62. doi: 10.1002/cphy.c120033
226. Steensberg A, van Hall G, Osada T, Sacchetti M, Saltin B, Pedersen BK. Production of interleukin-6 in contracting human skeletal muscles can account for the exercise-induced increase in plasma interleukin-6. J Physiol (2000) 529:237–42. doi: 10.1111/j.1469-7793.2000.00237.x
227. Iliev DB, Castellana B, MacKenzie S, Planas JV, Goetz FW. Cloning and expression analysis of an IL-6 homolog in rainbow trout (Oncorhynchus mykiss). Mol Immunol (2007) 44:1803–7. doi: 10.1016/j.molimm.2006.07.297
228. Varela M, Dios S, Novoa B, Figueras A. Characterisation, expression and ontogeny of interleukin-6 and its receptors in zebrafish (Danio rerio). Dev Comp Immunol (2012) 37:97–106. doi: 10.1016/j.dci.2011.11.004
229. Ferrante C, Orlando G, Recinella L, Leone S, Chiavaroli A, Di Nisio C, et al. Central inhibitory effects on feeding induced by the adipo-myokine irisin. Eur J Pharmacol (2016) 791:389–94. doi: 10.1016/j.ejphar.2016.09.011
230. Seldin MM, Peterson JM, Byerly MS, Wei Z, Wong GW. Myonectin (CTRP15), a novel myokine that links skeletal muscle to systemic lipid homeostasis*. J Biol Chem (2012) 287:11968–80. doi: 10.1074/jbc.M111.336834
231. Abe T, Mikekado T, Haga S, Kisara Y, Watanabe K, Kurokawa T, et al. Identification, cDNA cloning, and mRNA localization of a zebrafish ortholog of leukemia inhibitory factor. Comp Biochem Physiol Part B: Biochem Mol Biol (2007) 147:38–44. doi: 10.1016/j.cbpb.2006.12.019
232. Mera P, Ferron M, Mosialou I. Regulation of energy metabolism by bone-derived hormones. Cold Spring Harb Perspect Med (2017) 8:a031666. doi: 10.1101/cshperspect.a031666
233. Han Y, You X, Xing W, Zhang Z, Zou W. Paracrine and endocrine actions of bone–the functions of secretory proteins from osteoblasts, osteocytes, and osteoclasts. Bone Res (2018) 6:1–11. doi: 10.1038/s41413-018-0019-6
234. Dirckx N, Moorer MC, Clemens TL, Riddle RC. The role of osteoblasts in energy homeostasis. Nat Rev Endocrinol (2019) 15:651–65. doi: 10.1038/s41574-019-0246-y
235. Robling AG, Bonewald LF. The osteocyte: New insights. Annu Rev Physiol (2020) 82:485–506. doi: 10.1146/annurev-physiol-021119-034332
236. Cancela ML, Williamson MK, Price PA. Amino-acid sequence of bone gla protein from the African clawed toad xenopus laevis and the fish sparus aurata1. Int J Pept Protein Res (1995) 46:419–23. doi: 10.1111/j.1399-3011.1995.tb01076.x
237. Rice JS, Williamson MK, Price PA. Isolation and sequence of the vitamin K-dependent matrix gla protein from the calcified cartilage of the soupfin shark. J Bone Mineral Res (1994) 9:567–76. doi: 10.1002/jbmr.5650090417
238. Boskey AL, Gadaleta S, Gundberg C, Doty SB, Ducy P, Karsenty G. Fourier Transform infrared microspectroscopic analysis of bones of osteocalcin-deficient mice provides insight into the function of osteocalcin. Bone (1998) 23:187–96. doi: 10.1016/S8756-3282(98)00092-1
239. Karsenty G, Ferron M. The contribution of bone to whole-organism physiology. Nature (2012) 481:314–20. doi: 10.1038/nature10763
240. Neve A, Corrado A, Cantatore FP. Osteocalcin: Skeletal and extra-skeletal effects. J Cell Physiol (2013) 228:1149–53. doi: 10.1002/jcp.24278
241. Oury F, Ferron M, Huizhen W, Confavreux C, Xu L, Lacombe J, et al. Osteocalcin regulates murine and human fertility through a pancreas-bone-testis axis. J Clin Invest (2013) 123:2421–33. doi: 10.1172/JCI65952
242. Wei J, Hanna T, Suda N, Karsenty G, Ducy P. Osteocalcin promotes β-cell proliferation during development and adulthood through Gprc6a. Diabetes (2014) 63:1021–31. doi: 10.2337/db13-0887
243. Pi M, Kapoor K, Ye R, Nishimoto SK, Smith JC, Baudry J, et al. Evidence for osteocalcin binding and activation of GPRC6A in β-cells. Endocrinology (2016) 157:1866–80. doi: 10.1210/en.2015-2010
244. Mera P, Laue K, Wei J, Berger JM, Karsenty G. Osteocalcin is necessary and sufficient to maintain muscle mass in older mice. Mol Metab (2016) 5:1042–7. doi: 10.1016/j.molmet.2016.07.002
245. Pinto JP, Ohresser MCP, Cancela ML. Cloning of the bone gla protein gene from the teleost fish sparus aurata. evidence for overall conservation in gene organization and bone-specific expression from fish to man. Gene (2001) 270:77–91. doi: 10.1016/S0378-1119(01)00426-7
246. Nishimoto SK, Waite JH, Nishimoto M, Kriwacki RW. Structure, activity, and distribution of fish osteocalcin *. J Biol Chem (2003) 278:11843–8. doi: 10.1074/jbc.M211449200
247. Laizé V, Viegas CSB, Price PA, Cancela ML. Identification of an osteocalcin isoform in fish with a Large acidic prodomain *. J Biol Chem (2006) 281:15037–43. doi: 10.1074/jbc.M600373200
248. Renn J, Winkler C. Characterization of collagen type 10a1 and osteocalcin in early and mature osteoblasts during skeleton formation in medaka. J Appl Ichthyol (2010) 26:196–201. doi: 10.1111/j.1439-0426.2010.01404.x
249. Viegas CSB, Simes DC, Williamson MK, Cavaco S, Laizé V, Price PA, et al. Sturgeon osteocalcin shares structural features with matrix gla protein: Evolutionary relationship and functional implications. J Biol Chem (2013) 288:27801–11. doi: 10.1074/jbc.M113.450213
250. Frazão C, Simes DC, Coelho R, Alves D, Williamson MK, Price PA, et al. Structural evidence of a fourth gla residue in fish osteocalcin: biological implications. Biochemistry (2005) 44:1234–42. doi: 10.1021/bi048336z
251. Hauschka PV, Carr SA. Calcium-dependent .alpha.-helical structure in osteocalcin. Biochemistry (1982) 21:2538–47. doi: 10.1021/bi00539a038
252. Hauschka PV, Wians FH Jr. Osteocalcin-hydroxyapatite interaction in the extracellular organic matrix of bone. Anatomical Rec (1989) 224:180–8. doi: 10.1002/ar.1092240208
253. Cantatore FP, Corrado A, Grano M, Quarta L, Colucci S, Melillo N. Osteocalcin synthesis by human osteoblasts from normal and osteoarthritic bone after vitamin D3 stimulation. Clin Rheumatol (2004) 23:490–5. doi: 10.1007/s10067-004-0928-1
254. Lie KK, Moren M. Retinoic acid induces two osteocalcin isoforms and inhibits markers of osteoclast activity in Atlantic cod (Gadus morhua) ex vivo cultured craniofacial tissues. Comp Biochem Physiol Part A: Mol Integr Physiol (2012) 161:174–84. doi: 10.1016/j.cbpa.2011.10.023
255. Jaillon O, Aury J-M, Brunet F, Petit J-L, Stange-Thomann N, Mauceli E, et al. Genome duplication in the teleost fish tetraodon nigroviridis reveals the early vertebrate proto-karyotype. Nature (2004) 431:946–57. doi: 10.1038/nature03025
256. Moghadam HK, Ferguson MM, Danzmann RG. Evolution of hox clusters in salmonidae: A comparative analysis between Atlantic salmon (Salmo salar) and rainbow trout (Oncorhynchus mykiss). J Mol Evol (2005) 61:636–49. doi: 10.1007/s00239-004-0338-7
257. Ortiz-Delgado JB, Simes DC, Gavaia P, Sarasquete C, Cancela ML. Osteocalcin and matrix GLA protein in developing teleost teeth: identification of sites of mRNA and protein accumulation at single cell resolution. Histochem Cell Biol (2005) 124:123–30. doi: 10.1007/s00418-005-0015-y
258. Nishimoto SK, Araki N, Robinson FD, Waite JH. Discovery of bone gamma-carboxyglutamic acid protein in mineralized scales. the abundance and structure of lepomis macrochirus bone gamma-carboxyglutamic acid protein. J Biol Chem (1992) 267:11600–5. doi: 10.1016/S0021-9258(19)49953-5
259. Price PA, Lothringer JW, Baukol SA, Reddi AH. Developmental appearance of the vitamin K-dependent protein of bone during calcification. analysis of mineralizing tissues in human, calf, and rat. J Biol Chem (1981) 256:3781–4. doi: 10.1016/S0021-9258(19)69522-0
260. Desbois C, Hogue DA, Karsenty G. The mouse osteocalcin gene cluster contains three genes with two separate spatial and temporal patterns of expression. J Biol Chem (1994) 269:1183–90. doi: 10.1016/S0021-9258(17)42240-X
261. Gavaia PJ, Simes DC, Ortiz-Delgado JB, Viegas CSB, Pinto JP, Kelsh RN, et al. Osteocalcin and matrix gla protein in zebrafish (Danio rerio) and Senegal sole (Solea senegalensis): Comparative gene and protein expression during larval development through adulthood. Gene Expression Patterns (2006) 6:637–52. doi: 10.1016/j.modgep.2005.11.010
262. Riera-Heredia N, Martins R, Mateus AP, Costa RA, Gisbert E, Navarro I, et al. Temperature responsiveness of gilthead sea bream bone; an in vitro and in vivo approach. Sci Rep (2018) 8:11211. doi: 10.1038/s41598-018-29570-9
263. Costa JM, Sartori MMP, Nascimento NFD, Kadri SM, Ribolla PEM, Pinhal D, et al. Inadequate dietary phosphorus levels cause skeletal anomalies and alter osteocalcin gene expression in zebrafish. Int J Mol Sci (2018) 19:364. doi: 10.3390/ijms19020364
264. Mazurais D, Darias MJ, Gouillou-Coustans MF, Le Gall MM, Huelvan C, Desbruyères E, et al. Dietary vitamin mix levels influence the ossification process in European sea bass (Dicentrarchus labrax) larvae. Am J Physiol-Regulatory Integr Comp Physiol (2008) 294:R520–7. doi: 10.1152/ajpregu.00659.2007
265. Riera-Heredia N, Vélez EJ, Gutiérrez J, Navarro I, Capilla E. Gene expression analyses in malformed skeletal structures of gilthead sea bream (Sparus aurata). J Fish Dis (2019) 42:1169–80. doi: 10.1111/jfd.13019
266. Ducy P, Desbois C, Boyce B, Pinero G, Story B, Dunstan C, et al. Increased bone formation in osteocalcin-deficient mice. Nature (1996) 382:448–52. doi: 10.1038/382448a0
267. Bodine PVN, Komm BS. Evidence that conditionally immortalized human osteoblasts express an osteocalcin receptor. Bone (1999) 25:535–43. doi: 10.1016/S8756-3282(99)00213-6
268. Oury F, Sumara G, Sumara O, Ferron M, Chang H, Smith CE, et al. Endocrine regulation of Male fertility by the skeleton. Cell (2011) 144:796–809. doi: 10.1016/j.cell.2011.02.004
269. Hashiguchi Y, Nishida M. Evolution and origin of vomeronasal-type odorant receptor gene repertoire in fishes. BMC Evol Biol (2006) 6:76. doi: 10.1186/1471-2148-6-76
270. Calo J, Blanco AM, Comesaña S, Conde-Sieira M, Morais S, Soengas JL. First evidence for the presence of amino acid sensing mechanisms in the fish gastrointestinal tract. Sci Rep (2021) 11:4933. doi: 10.1038/s41598-021-84303-9
271. Oya M, Kitaguchi T, Pais R, Reimann F, Gribble F, Tsuboi T. The G protein-coupled receptor family c group 6 subtype a (GPRC6A) receptor is involved in amino acid-induced glucagon-like peptide-1 secretion from GLUTag cells *. J Biol Chem (2013) 288:4513–21. doi: 10.1074/jbc.M112.402677
272. Diez-Hermano S, Ganfornina MD, Skerra A, Gutiérrez G, Sanchez D. An evolutionary perspective of the lipocalin protein family. Front Physiol (2021) 12. doi: 10.3389/fphys.2021.718983
273. Kitamura K. Chapter 55 - lipocalin-2. In: Ando H, Ukena K, Nagata S, editors. Handbook of hormones, 2nd ed. San Diego: Academic Press (2021). p. 613–5. doi: 10.1016/B978-0-12-820649-2.00156-X
274. Goetz DH, Holmes MA, Borregaard N, Bluhm ME, Raymond KN, Strong RK. The neutrophil lipocalin NGAL is a bacteriostatic agent that interferes with siderophore-mediated iron acquisition. Mol Cell (2002) 10:1033–43. doi: 10.1016/S1097-2765(02)00708-6
275. Flo TH, Smith KD, Sato S, Rodriguez DJ, Holmes MA, Strong RK, et al. Lipocalin 2 mediates an innate immune response to bacterial infection by sequestrating iron. Nature (2004) 432:917–21. doi: 10.1038/nature03104
276. Zerega B, Cermelli S, Michelis B, Cancedda R, Descalzi Cancedda F. Expression of NRL/NGAL (neu-related lipocalin/neutrophil gelatinase-associated lipocalin) during mammalian embryonic development and in inflammation. Eur J Cell Biol (2000) 79:165–72. doi: 10.1078/S0171-9335(04)70019-9
277. Wang Y, Lam KSL, Kraegen EW, Sweeney G, Zhang J, Tso AW, et al. Lipocalin-2 is an inflammatory marker closely associated with obesity, insulin resistance, and hyperglycemia in humans. Clin Chem (2007) 53:34–41. doi: 10.1373/clinchem.2006.075614
278. Mosialou I, Shikhel S, Liu J-M, Maurizi A, Luo N, He Z, et al. MC4R-dependent suppression of appetite by bone-derived lipocalin 2. Nature (2017) 543:385–90. doi: 10.1038/nature21697
279. Zhou Z, Feng C, Liu X, Liu S. 3nLcn2, a teleost lipocalin 2 that possesses antimicrobial activity and inhibits bacterial infection in triploid crucian carp. Fish Shellfish Immunol (2020) 102:47–55. doi: 10.1016/j.fsi.2020.04.015
280. Hu MC, Shiizaki K, Kuro-o M, Moe OW. Fibroblast growth factor 23 and klotho: Physiology and pathophysiology of an endocrine network of mineral metabolism. Annu Rev Physiol (2013) 75:503–33. doi: 10.1146/annurev-physiol-030212-183727
281. Quinn SJ, Thomsen ARB, Pang JL, Kantham L, Bräuner-Osborne H, Pollak M, et al. Interactions between calcium and phosphorus in the regulation of the production of fibroblast growth factor 23 in vivo. Am J Physiol-Endocrinol Metab (2013) 304:E310–20. doi: 10.1152/ajpendo.00460.2012
282. Mangos S, Amaral AP, Faul C, Jüppner H, Reiser J, Wolf M. Expression of fgf23 and αklotho in developing embryonic tissues and adult kidney of the zebrafish, danio rerio. Nephrol Dialysis Transplant (2012) 27:4314–22. doi: 10.1093/ndt/gfs335
283. Lin C-H, Hu H-J, Hwang P-P. Molecular physiology of the hypocalcemic action of fibroblast growth factor 23 in zebrafish (Danio rerio). Endocrinology (2017) 158:1347–58. doi: 10.1210/en.2016-1883
284. Elizondo MR, Budi EH, Parichy DM. trpm7 regulation of in vivo cation homeostasis and kidney function involves stanniocalcin 1 and fgf23. Endocrinology (2010) 151:5700–9. doi: 10.1210/en.2010-0853
285. Singh AP, Sosa MX, Fang J, Shanmukhappa SK, Hubaud A, Fawcett CH, et al. αKlotho regulates age-associated vascular calcification and lifespan in zebrafish. Cell Rep (2019) 28:2767–2776.e5. doi: 10.1016/j.celrep.2019.08.013
286. Armingol E, Officer A, Harismendy O, Lewis NE. Deciphering cell–cell interactions and communication from gene expression. Nat Rev Genet (2021) 22:71–88. doi: 10.1038/s41576-020-00292-x
287. Herold J, Kalucka J. Angiogenesis in adipose tissue: The interplay between adipose and endothelial cells. Front Physiol (2021) 11. doi: 10.3389/fphys.2020.624903
288. Collins KH, Lenz KL, Pollitt EN, Ferguson D, Hutson I, Springer LE, et al. Adipose tissue is a critical regulator of osteoarthritis. Proc Natl Acad Sci (2021) 118:e2021096118. doi: 10.1073/pnas.2021096118
289. Harms MJ, Li Q, Lee S, Zhang C, Kull B, Hallen S, et al. Mature human white adipocytes cultured under membranes maintain identity, function, and can transdifferentiate into brown-like adipocytes. Cell Rep (2019) 27:213–225.e5. doi: 10.1016/j.celrep.2019.03.026
290. Takahashi Y, Sato S, Kurashima Y, Lai C-Y, Otsu M, Hayashi M, et al. Reciprocal inflammatory signaling between intestinal epithelial cells and adipocytes in the absence of immune cells. EBioMedicine (2017) 23:34–45. doi: 10.1016/j.ebiom.2017.07.027
291. Zhao C, Wu M, Zeng N, Xiong M, Hu W, Lv W, et al. Cancer-associated adipocytes: emerging supporters in breast cancer. J Exp Clin Cancer Res (2020) 39:156. doi: 10.1186/s13046-020-01666-z
292. Dufau J, Shen JX, Couchet M, De Castro Barbosa T, Mejhert N, Massier L, et al. In vitro and ex vivo models of adipocytes. Am J Physiol-Cell Physiol (2020) 320:C822–41. doi: 10.1152/ajpcell.00519.2020
293. Seo K, Suzuki T, Kobayashi K, Nishimura T. Adipocytes suppress differentiation of muscle cells in a co-culture system. Anim Sci J (2019) 90:423–34. doi: 10.1111/asj.13145
294. Chu W, Wei W, Yu S, Han H, Shi X, Sun W, et al. C2C12 myotubes inhibit the proliferation and differentiation of 3T3-L1 preadipocytes by reducing the expression of glucocorticoid receptor gene. Biochem Biophys Res Commun (2016) 472:68–74. doi: 10.1016/j.bbrc.2016.02.063
295. Pellegrinelli V, Rouault C, Rodriguez-Cuenca S, Albert V, Edom-Vovard F, Vidal-Puig A, et al. Human adipocytes induce inflammation and atrophy in muscle cells during obesity. Diabetes (2015) 64:3121–34. doi: 10.2337/db14-0796
296. Cui HX, Guo LP, Zhao GP, Liu RR, Li QH, Zheng MQ, et al. Method using a co-culture system with high-purity intramuscular preadipocytes and satellite cells from chicken pectoralis major muscle. Poultry Sci (2018) 97:3691–7. doi: 10.3382/ps/pey023
297. Biferali B, Proietti D, Mozzetta C, Madaro L. Fibro–adipogenic progenitors cross-talk in skeletal muscle: The social network. Front Physiol (2019) 10:1074. doi: 10.3389/fphys.2019.01074
298. Sun W, Dong H, Balaz M, Slyper M, Drokhlyansky E, Colleluori G, et al. snRNA-seq reveals a subpopulation of adipocytes that regulates thermogenesis. Nature (2020) 587:98–102. doi: 10.1038/s41586-020-2856-x
299. Yang Loureiro Z, Solivan-Rivera J, Corvera S. Adipocyte heterogeneity underlying adipose tissue functions. Endocrinology (2022) 163:bqab138. doi: 10.1210/endocr/bqab138
300. Zhu Q, An YA, Scherer PE. Mitochondrial regulation and white adipose tissue homeostasis. Trends Cell Biol (2022) 32:351–64. doi: 10.1016/j.tcb.2021.10.008
301. Johnston EK, Abbott RD. Adipose tissue development relies on coordinated extracellular matrix remodeling, angiogenesis, and adipogenesis. Biomedicines (2022) 10:2227. doi: 10.3390/biomedicines10092227
302. Chan WW, Yu F, Le QB, Chen S, Yee M, Choudhury D. Towards biomanufacturing of cell-derived matrices. Int J Mol Sci (2021) 22:11929. doi: 10.3390/ijms222111929
303. Zhong J, Guo B, Xie J, Deng S, Fu N, Lin S, et al. Crosstalk between adipose-derived stem cells and chondrocytes: when growth factors matter. Bone Res (2016) 4:1–10. doi: 10.1038/boneres.2015.36
304. Montalvany-Antonucci CC, Zicker MC, Ferreira AVM, Macari S, Ramos-Junior ES, Gomez RS, et al. High-fat diet disrupts bone remodeling by inducing local and systemic alterations. J Nutr Biochem (2018) 59:93–103. doi: 10.1016/j.jnutbio.2018.06.006
305. Battafarano G, Rossi M, Marampon F, Minisola S, Del Fattore A. Bone control of muscle function. Int J Mol Sci (2020) 21:1178. doi: 10.3390/ijms21041178
306. Shahin-Shamsabadi A, Selvaganapathy PR. A 3D self-assembled In vitro model to simulate direct and indirect interactions between adipocytes and skeletal muscle cells. Adv Biosyst (2020) 4:2000034. doi: 10.1002/adbi.202000034
307. Suarez-Martinez E, Suazo-Sanchez I, Celis-Romero M, Carnero A. 3D and organoid culture in research: physiology, hereditary genetic diseases and cancer. Cell Biosci (2022) 12:39. doi: 10.1186/s13578-022-00775-w
308. Wragg NM, Mosqueira D, Blokpeol-Ferreras L, Capel A, Player DJ, Martin NRW, et al. Development of a 3D tissue-engineered skeletal muscle and bone Co-culture system. Biotechnol J (2019) 15:1900106. doi: 10.1002/biot.201900106
309. Minchin JEN, Rawls JF. A classification system for zebrafish adipose tissues. Dis Models Mech (2017) 10:797–809. doi: 10.1242/dmm.025759
310. Lepanto P, Levin-Ferreyra F, Koziol U, Malacrida L, Badano JL. Insights into in vivo adipocyte differentiation through cell-specific labeling in zebrafish. Biol Open (2021) 10:bio058734. doi: 10.1242/bio.058734
311. Lumaquin D, Johns E, Montal E, Weiss JM, Ola D, Abuhashem A, et al. An in vivo reporter for tracking lipid droplet dynamics in transparent zebrafish. eLife (2021) 10:e64744. doi: 10.7554/eLife.64744
312. Mao Y, Hong K-H, Liao W, Li L, Kim S-J, Xiong Y, et al. Generation of a novel transgenic zebrafish for studying adipocyte development and metabolic control. Int J Mol Sci (2021) 22:3994. doi: 10.3390/ijms22083994
313. van Niel G, D’Angelo G, Raposo G. Shedding light on the cell biology of extracellular vesicles. Nat Rev Mol Cell Biol (2018) 19:213–28. doi: 10.1038/nrm.2017.125
314. O’Brien K, Breyne K, Ughetto S, Laurent LC, Breakefield XO. RNA Delivery by extracellular vesicles in mammalian cells and its applications. Nat Rev Mol Cell Biol (2020) 21:585–606. doi: 10.1038/s41580-020-0251-y
315. Isaac R, Reis FCG, Ying W, Olefsky JM. Exosomes as mediators of intercellular crosstalk in metabolism. Cell Metab (2021) 33:1744–62. doi: 10.1016/j.cmet.2021.08.006
316. Zhang Y, Zhang C, Wang J, Liu H, Wang M. Bone-adipose tissue crosstalk: Role of adipose tissue derived extracellular vesicles in bone diseases. J Cell Physiol (2020) 236:7874–86. doi: 10.1002/jcp.30414
317. He C, He W, Hou J, Chen K, Huang M, Yang M, et al. Bone and muscle crosstalk in aging. Front Cell Dev Biol (2020) 8:585644. doi: 10.3389/fcell.2020.585644
318. Kita S, Maeda N, Shimomura I. Interorgan communication by exosomes, adipose tissue, and adiponectin in metabolic syndrome. J Clin Invest (2019) 129:4041–9. doi: 10.1172/JCI129193
319. Verweij FJ, Revenu C, Arras G, Dingli F, Loew D, Pegtel DM, et al. Live tracking of inter-organ communication by endogenous exosomes in vivo. Dev Cell (2019) 48:573–589.e4. doi: 10.1016/j.devcel.2019.01.004
320. Verdi V, Bécot A, van Niel G, Verweij FJ. In vivo imaging of EVs in zebrafish: New perspectives from “the waterside”. FASEB BioAdvances (2021) 3:918–29. doi: 10.1096/fba.2021-00081
321. Wang Y-Y, Wang Y-D, Qi X-Y, Liao Z-Z, Mai Y-N, Xiao X-H. Organokines and exosomes: Integrators of adipose tissue macrophage polarization and recruitment in obesity. Front Endocrinol (Lausanne) (2022) 13:839849. doi: 10.3389/fendo.2022.839849
322. Gonzalez-Gil AM, Elizondo-Montemayor L. The role of exercise in the interplay between myokines, hepatokines, osteokines, adipokines, and modulation of inflammation for energy substrate redistribution and fat mass loss: A review. Nutrients (2020) 12:1899. doi: 10.3390/nu12061899
323. Ren Y, Zhao H, Yin C, Lan X, Wu L, Du X, et al. Adipokines, hepatokines and myokines: Focus on their role and molecular mechanisms in adipose tissue inflammation. Front Endocrinol (Lausanne) (2022) 13:873699. doi: 10.3389/fendo.2022.873699
Keywords: inter-tissue communication, adiponectin, leptin, TNF, TGFs, myostatin, IGFs, osteocalcin
Citation: Hue I, Capilla E, Rosell-Moll E, Balbuena-Pecino S, Goffette V, Gabillard J-C and Navarro I (2023) Recent advances in the crosstalk between adipose, muscle and bone tissues in fish. Front. Endocrinol. 14:1155202. doi: 10.3389/fendo.2023.1155202
Received: 31 January 2023; Accepted: 27 February 2023;
Published: 14 March 2023.
Edited by:
Anderson O. L. Wong, The University of Hong Kong, Hong Kong SAR, ChinaReviewed by:
Hiroyasu Kamei, Kanazawa University, JapanSuraj Unniappan, University of Saskatchewan, Canada
Hélène Volkoff, Memorial University of Newfoundland, Canada
Copyright © 2023 Hue, Capilla, Rosell-Moll, Balbuena-Pecino, Goffette, Gabillard and Navarro. This is an open-access article distributed under the terms of the Creative Commons Attribution License (CC BY). The use, distribution or reproduction in other forums is permitted, provided the original author(s) and the copyright owner(s) are credited and that the original publication in this journal is cited, in accordance with accepted academic practice. No use, distribution or reproduction is permitted which does not comply with these terms.
*Correspondence: Isabel Navarro, bW5hdmFycm9AdWIuZWR1