- Affiliated Hospital of Weifang Medical University, School of Clinical Medicine, Weifang Medical University, Weifang, SD, China
Introduction: Tissue-resident stem cells (TRSCs) have the ability to self-renew and differentiate throughout an individual’s lifespan, and they utilize both mechanisms to maintain homeostasis and regenerate damaged tissues. Several studies suggest that these stem cells can serve as a potential source for cell-replacement-based therapy by promoting differentiation or expansion. In recent years, low-intensity pulsed ultrasound (LIPUS) has been demonstrated to effectively stimulate stem cell proliferation and differentiation, promote tissue regeneration, and inhibit inflammatory responses.
Aims: To present a comprehensive overview of current application and mechanism of LIPUS on tissue resident stem cells.
Methods: We searched PubMed, Web of Science for articles on the effects of LIPUS on tissue resident stem cells and its application.
Results: The LIPUS could modulate cellular activities such as cell viability, proliferation and differentiation of tissue resident stem cells and related cells through various cellular signaling pathways. Currently, LIPUS, as the main therapeutic ultrasound, is being widely used in the treatment of preclinical and clinical diseases.
Conclusion: The stem cell research is the hot topic in the biological science, while in recent years, increasing evidence has shown that TRSCs are good targets for LIPUS-regulated regenerative medicine. LIPUS may be a novel and valuable therapeutic approach for the treatment of ophthalmic diseases. How to further improve its efficiency and accuracy, as well as the biological mechanism therein, will be the focus of future research.
1 Introduction
Stem cells are categorized based on their ability to differentiate into pluripotent, multipotent, and unipotent stem cells, with the latter also known as adult stem cells. Pluripotent stem cells include embryonic stem cells and induced pluripotent stem cells (1). Adult stem cells have a limited differentiation capacity but play a crucial role in tissue regeneration, as they are the endogenous stem cells in the tissue almost always.
The human body is comprised of various cell types, and the turnover rates of the cell types that construct each organ vary. As many as 1011 cells die in each adult every day (2). Rapidly turning over cells are replaced by the progeny of highly active stem cells, often referred to as “tissue-resident stem cells (TRSCs)” or “adult stem cells” (1, 3). These TRSCs play a critical role in maintaining tissue homeostasis and repairing injuries, which is dependent on interactions with stem cell niches (3, 4). Current research has identified TRSCs in important organs such as the brain, eyes, lung, heart, kidney, urethra, penis, bladder, muscle, and more (as shown in Figure 1). These cells are capable of self-renewal and differentiation throughout an individual’s lifespan, utilizing both mechanisms to maintain homeostasis and regenerate damaged tissues (4–6). It has been suggested that tissue-resident adult stem/progenitor cells offer the potential for in vivo differentiation stimulation or using their ex vivo expanded progenies for cell replacement-based therapies with multiple applications in humans (5). Such applications include Parkinson’s and Alzheimer’s diseases, Type 1 or 2 diabetes mellitus, as well as eye, liver, lung, skin, cardiovascular disorders, aggressive and metastatic cancers (4, 5). Each type of tissue resident stem cell has its own unique characteristics and functions, making them important for maintaining tissue homeostasis and regeneration (Table 1).
Low-intensity pulsed ultrasound (LIPUS) has recently been shown to be a novel approach for modulating stem cells. LIPUS is a specific type of therapeutic ultrasound that operates at low intensity and uses pulsed waves (7). Therapeutic ultrasound has the advantage of producing controlled biological effects noninvasively (8). The use of ultrasonic energy for therapy has continued to expand, with approved applications now including uterine fibroid ablation, cataract removal (phacoemulsification), surgical tissue cutting and hemostasis, transdermal drug delivery, and bone fracture healing, among others (9). LIPUS has minimal thermal effects while still delivering acoustic energy to the target tissue, allowing for noninvasive physical stimulation for therapeutic applications (10). The beneficial biological effects of LIPUS are the result of mechanotransduction, producing microturbulence within the intercellular and intracellular fluids in the vicinity of the wave (11).
Currently, LIPUS has been successfully applied in clinical practices to promote fracture healing, accelerate soft tissue regeneration, and inhibit inflammatory responses. It has also become a tool for enhancing regeneration and tissue engineering by modulating tissue-resident stem cells. Recently, researchers discovered that LIPUS protects retinal ganglion cells (RGCs) from apoptosis and axonal atrophy after optic nerve crush injury and also protects the retina from degenerative thinning (12).
This review presents an overview of the research progress in the tissue resident stem cells, biological effects of LIPUS on stem cell, molecular signaling pathway, preclinical and clinical applications of LIPUS.
2 Effects of LIPUS on tissue resident stem cells and related cells
LIPUS is a non-invasive medical treatment that uses high-frequency sound waves to stimulate tissue healing and regeneration, proving to be a novel approach to modulate TRSCs. During LIPUS treatment, a small handheld device is placed on the skin over the affected area. The device emits high-frequency sound waves that penetrate the tissues and stimulate cellular activity. The sound waves cause microscopic vibrations in the tissues, which activate cell signaling pathways and promote the production of growth factors and other molecules involved in tissue repair and regeneration (7, 10, 11).
2.1 Key parameters of LIPUS
Some of the key parameter (13, 14) that can be adjusted to optimize the effectiveness of LIPUS for stem cells including: 1). Frequency: LIPUS typically uses frequencies in the range of 20 kHz to 1 MHz, but the optimal frequency can depend on the specific application and the tissue type. For example, bone tissue may respond best to frequencies in the range of 20-40 kHz, while cartilage tissue may respond best to frequencies in the range of 100-500 kHz. 2). Intensity: LIPUS can be delivered at different intensities, ranging from very low intensities (typically < 10 mW/cm2) to higher intensities (up to several hundred mW/cm2). The optimal intensity can depend on the specific application and the tissue type. For example, bone tissue may require higher intensities to achieve the desired effects, while cartilage tissue may respond better to lower intensities. 3). Duration: The duration of LIPUS exposure can also vary depending on the specific application and the tissue type. In general, longer exposure times may be required for tissues with lower metabolic rates or slower rates of cell proliferation. 4). Duty cycle: The duty cycle of LIPUS refers to the percentage of time that the ultrasound is on versus off. Duty cycles can range from continuous (100% on) to pulsed (less than 100% on). Pulsed duty cycles are often used in LIPUS applications for stem cells, as they have been shown to be more effective than continuous duty cycles. 5). Spatial distribution: The spatial distribution of LIPUS can also be important, as it can affect the distribution of acoustic pressure and the resulting effects on the tissue. This can be controlled by the size and shape of the ultrasound transducer, as well as the positioning of the transducer relative to the tissue being treated.
2.2 Ophthalmic tissue resident stem cells
Stem cells have potential therapeutic applications in ophthalmology due to their ability to regenerate damaged tissues and repair the eye. There are several types of stem cells that can be used in ophthalmology, including corneal epithelial stem cells, retinal pigment epithelium stem cells, and mesenchymal stem cells. Some potential therapeutic applications of stem cells in ophthalmology include corneal repair, retinal repair, glaucoma treatment, gene therapy and drug development.
Recently, studies have demonstrated the presence of several endogenous stem cell populations in multiple sites of the eye, which play a role in many eye diseases (15). Limbal stem cells exist in both the inner and outer limbus and are believed to generate short-term progenitor cells that can move concentrically to the central cornea or delaminate (16). Stem cells in the Schwalbe lineage region may constitute a population of adult stem cells with the ability to compensate for the loss of trabecular meshwork (TM) and/or corneal endothelial cells (17). Corneal stem cells are also present and maintain the integrity of the corneal epithelium, protect it from external physical or chemical damage, prevent invasion and damage from pathogenic microorganisms, and inhibit immune inflammation and corneal neovascularization, thereby maintaining the stability of the ocular surface.
Postnatal iris pigment epithelium (IPE) cells are characterized by neural stem/progenitor cells that can proliferate efficiently and exhibit remarkable plasticity. Under appropriate conditions, IPE can generate a variety of cell types, including retina-specific neurons and glia, and lenses (18). The ciliary body (CB) and retinal pigment epithelium (RPE) also contain pigment cells with neuronal progenitor-like characteristics (19). These stem cell populations offer new potential sources for basic stem cell biology and therapeutic applications in retinal diseases.
Trabecular meshwork stem cells (TMSCs) are homogeneous and pluripotent, with the ability to differentiate into phagocytic TM cells. These cells may be able to repair damaged trabecular meshwork and restore functional regulation of aqueous outflow, offering potential for novel stem cell-based therapies for glaucoma (15, 20, 21). Additionally, retinal Muller glia were recently discovered to differentiate into retinal ganglion cells (RGCs), providing a local source of endogenous cells for replacement therapy. Traumatic optic nerve (ON) degeneration and glaucoma-induced retinal ganglion cell death pose major challenges to vision loss. Therefore, preventing RGC apoptosis and promoting regeneration may provide opportunities for the treatment of glaucoma and other optic neuropathies (9, 22).
2.2.1 Effects of LIPUS on Glioma stem cells
Glioma stem cells (GSCs) are a type of cells resembling neural stem cells, with strong self-renewal, unlimited proliferation, and multi-directional differentiation potential. Song et al. (23) investigated the effect of LIPUS on GSCs by inducing glioblastoma cell-derived GSCs in vitro and stimulating them with LIPUS for three consecutive days. After measuring relevant markers on the cell surface, the researchers found that LIPUS can induce GSCs to lose stem cell-related characteristics and also “awaken” dormant GSCs from the quiescent G0 phase to the active G2/M phase, thus inducing GSC differentiation. Considering the clinical significance of GSCs, the researchers also observed that LIPUS can increase the sensitivity of GCSs to the chemotherapy drug temozolomide (TMZ) and reduce drug resistance compared to the blank control group.
2.2.2 Effects of LIPUS on Retinal ganglion cells
In ophthalmic diseases such as glaucoma and traumatic optic neuropathy, Retinal Ganglion Cells (RGCs) injury and apoptosis are significant factors affecting visual acuity in patients. Previous studies demonstrated that ultrasound can stimulate the retina of mice and rapidly modulate visual sensitivity (24). Recently, Zhou et al. (12) used LIPUS in optic nerve compression animal models and in vitro RGCs degeneration models to investigate the regulatory effect of LIPUS on retinal tissue-resident stem cells. Low-intensity LIPUS (Grade 1 and 2) was found to improve RGCs’ cell viability and prevent apoptosis in optic nerve compression. The optimal LIPUS parameters were a probe diameter of 1.3 cm, audio frequency of 1 MHz, duty ratio of 20%, and pulse repetition rate of 1 kHz. Moreover, LIPUS was shown to alleviate retinal degeneration and protect it from degenerative thinning. At the molecular level, it was found that LIPUS treatment induced dynamic transformation of YAP/p-YAP, which was enhanced by caspase-3 blotting, and protected both the retina and RGCs. These results suggest that LIPUS plays a key role in preventing RGCs’ apoptosis through YAP.
Currently, extensive research demonstrated that many other stem cells were also modulated by LIPUS both in vitro and in vivo (Table 2).
3 Mechanism of LIPUS in modulating TRSCs
At present, the mechanism of LIPUS regulating TRSCs has been extensively studied, and the relevant cell biology and molecular biology mechanisms have been initially discovered. Related cell signaling pathways were also explored. However, to thoroughly elucidate its mechanism, especially the regulatory mechanism of tissue-resident stem cells, a lot of in-depth basic and clinical research is still needed.
3.1 The cellular and molecular mechanisms involved in the interaction
LIPUS has been shown to have a positive effect on TRSCs, promoting their proliferation, differentiation, and migration. The cellular and molecular mechanisms involved in this interaction are complex and not fully understood, but several studies have provided insights into the potential mechanisms underlying the effects of LIPUS on TRSCs.
1) Activation of intracellular signaling pathways: LIPUS has been shown to activate various intracellular signaling pathways, including the MAPK and PI3K/AKT pathways (59), which are involved in cell proliferation, differentiation, and migration. These signaling pathways are thought to play a role in the beneficial effects of LIPUS on TRSCs.
2) Upregulation of growth factors and cytokines: LIPUS has been shown to upregulate the expression of growth factors and cytokines, such as VEGF, bFGF, and TGF-β, which are involved in the regulation of cell proliferation, differentiation, and migration (64, 65). These growth factors and cytokines are thought to stimulate the activity of TRSCs and promote their regenerative potential.
3) Enhancement of extracellular matrix (ECM) remodeling: LIPUS has been shown to promote the remodeling of the ECM (66), which is important for tissue regeneration. TRSCs rely on the ECM to provide physical support and biochemical signals for their proliferation, differentiation, and migration. LIPUS has been shown to stimulate the synthesis and remodeling of ECM components, such as collagen and fibronectin, which are important for tissue regeneration.
4) Induction of angiogenesis: LIPUS has been shown to promote the formation of new blood vessels, which is important for tissue regeneration (45). TRSCs have been shown to play a role in angiogenesis, and LIPUS has been shown to stimulate the production of angiogenic factors, such as VEGF.
Overall, the cellular and molecular mechanisms involved in the interaction between LIPUS and TRSCs are complex and multifactorial. The effects of LIPUS on TRSCs are likely mediated through multiple signaling pathways, growth factors, cytokines, and ECM components, all of which play a role in the regenerative potential of these cells.
3.2 Cellular signaling pathways for LIPUS modulate TRSCs
Since 2004 (67), researches have been carried out on the cellular signaling pathways mechanically stimulated by LIPUS, especially the pathways related to the stem cells. LIPUS has been shown to modulate the activity of TRSCs through various cellular signaling pathways (Figure 2), including 1). Wnt/β-catenin pathway: The Wnt/β-catenin pathway is involved in the regulation of cell proliferation, differentiation, and migration, and it plays an important role in the development and maintenance of ocular tissues. LIPUS has been shown to activate the Wnt/β-catenin pathway in tissue resident stem cells, leading to enhanced proliferation and differentiation (68, 69). 2). PI3K/Akt pathway: The PI3K/Akt pathway is a key regulator of cell survival and proliferation, and it plays an important role in the response to growth factors and cytokines. LIPUS has been shown to activate the PI3K/Akt pathway in tissue resident stem cells, leading to enhanced survival and proliferation (31, 59). 3). MAPK/ERK pathway: The MAPK/ERK pathway is involved in the regulation of cell proliferation, differentiation, and migration, and it plays an important role in the response to extracellular stimuli. LIPUS has been shown to activate the MAPK/ERK pathway in tissue resident stem cells, leading to enhanced proliferation, differentiation, and migration (26, 34, 70, 71). 4). Notch signaling pathway: The Notch signaling pathway is involved in the regulation of cell fate determination and differentiation, and it plays an important role in the development and maintenance of ocular tissues. LIPUS has been shown to activate the Notch signaling pathway in tissue resident stem cells, leading to enhanced differentiation and regeneration (53). 5). TGF-β signaling pathway: The TGF-β signaling pathway is involved in the regulation of cell proliferation, differentiation, and migration, and it plays an important role in the response to tissue injury and inflammation. LIPUS has been shown to activate the TGF-β signaling pathway in tissue resident stem cells, leading to enhanced proliferation, differentiation, and migration (27, 41, 72, 73).
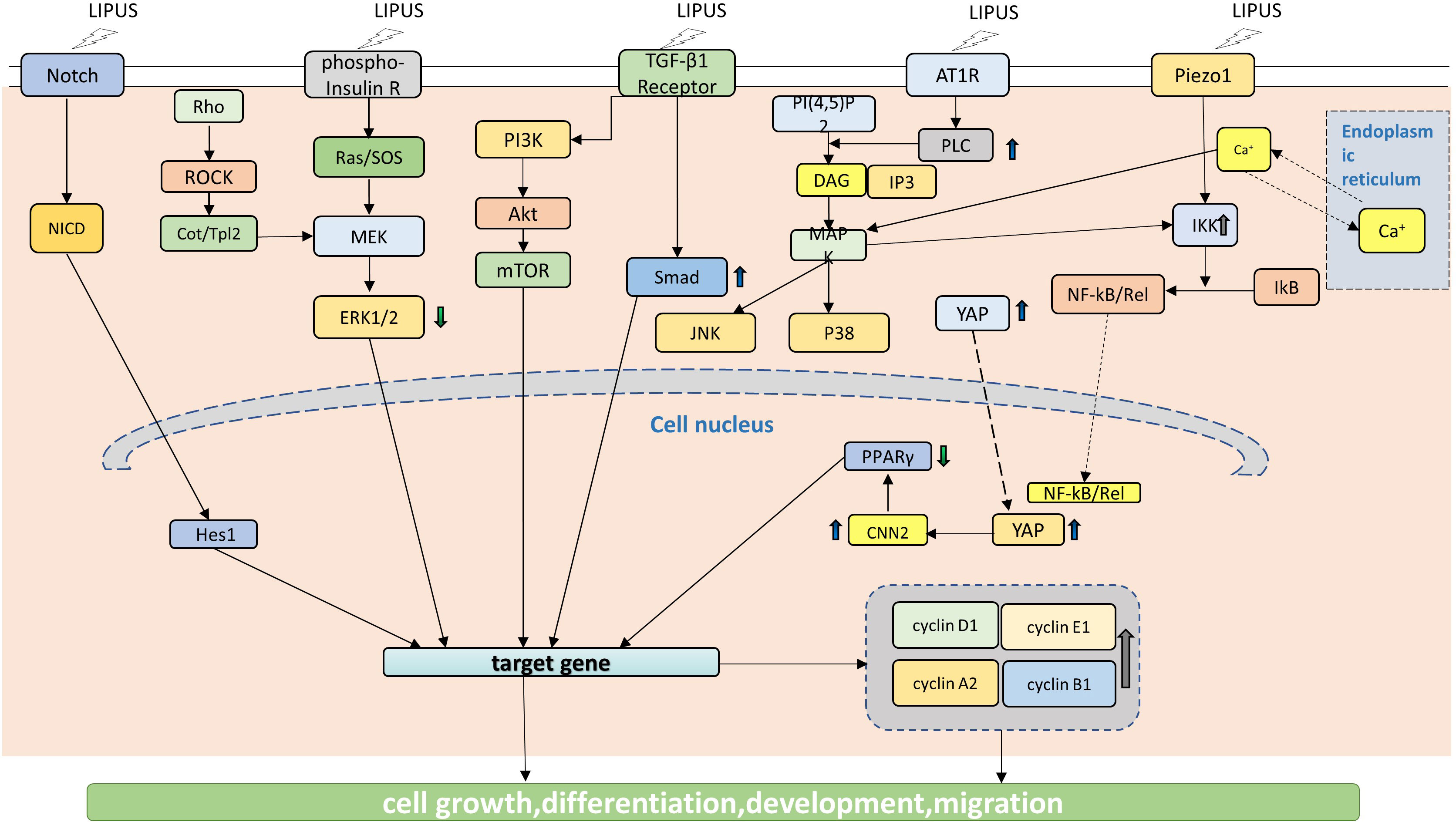
Figure 2 LIPUS acts on signaling pathways in TRSCS. LIPUS triggers the proliferation and differentiation of TRSCs by regulating Notch signaling pathway, which is manifested by the significant up-regulation of Hesl expression level. LIPUS can activate the TGF-B and PI3K/Akt pathways, which are involved in the regulation of cell proliferation, differentiation, and migration. LIPUS also regulates MAPK/ERK pathway, including Rho-Cou/Tpl2-MEK-ERK signaling pathway, Ras/SOS-MEK-ERK signaling pathway, JNK MAPK signaling pathway and p38 MAPK signaling pathway. By activating the TGF-B and PI3K/Akt signaling pathways, LIPUS enhances cell survival and proliferation. Furthermore, LIPUS promotes the translocation of YAP to the nucleus, resulting in the up-regulation of CCN2. The forced expression of CCN2, however, can reduce the expression of PPAR y gene.
At the same time, the YAP signaling pathway, Piezo signaling pathway and SDF-1/CXCR4 signaling pathway are also involved (Figure 2) (12, 26, 27, 31, 34, 37, 41, 43, 50, 53, 59, 67, 70–80). These cellular signaling pathways are thought to be involved in the effects of LIPUS on tissue resident stem cells, but the exact mechanisms by which LIPUS activates these pathways are not fully understood. Further research is needed to fully elucidate the cellular and molecular mechanisms underlying the effects of LIPUS on tissue resident stem cells, and to optimize the use of LIPUS for therapeutic applications in ophthalmology.
4 Potential applications of LIPUS therapy in ophthalmic diseases
LIPUS has shown great potential for stem cell therapy in various ophthalmic diseases, including 1). Age-related macular degeneration (AMD): AMD is a leading cause of vision loss in older adults. LIPUS can stimulate the proliferation and differentiation of RPE cells, which play a critical role in maintaining the health of the retina. 2). Glaucoma: Glaucoma is a group of eye conditions that can lead to irreversible vision loss. LIPUS can stimulate the differentiation of stem cells into RGCs, which are the cells that are damaged in glaucoma. 3). Corneal injuries and diseases: the cornea is a transparent layer at the front of the eye that can be damaged by injury or disease. LIPUS can stimulate the proliferation and differentiation of corneal epithelial stem cells. 4). Retinal diseases: LIPUS can stimulate the differentiation of stem cells into retinal cells to replace the damaged or destroyed cells. 5). Optic nerve injuries: Optic nerve injuries can result in permanent vision loss. LIPUS can stimulate the differentiation of stem cells into RGCs, which are the cells that are damaged in optic nerve injuries.
5 Conclusions
Stem cell research is a highly discussed topic in the field of biological science. Over the years, a growing body of evidence has shown that tissue resident stem cells represent promising targets for regenerative medicine. Sources of stem cells commonly used for retinal regeneration today include endogenous retinal stem cells (such as neural stem cells, Muller cells, and retinal stem cells from the ciliary marginal region) and exogenous stem cells (such as bone mesenchymal stem cells, adipose-derived stem cells, embryonic stem cells, and induced pluripotent stem cells) (81). Leveraging the characteristics of TRSCs, their survival, migration, differentiation and integration in the retina can be utilized to try to restore vision in patients with retinal diseases (82). LIPUS, a non-invasive therapeutic method, has been demonstrated to effectively modulate RGCs (12). However, the system specifications of LIPUS for stem cells must be carefully optimized to ensure that the desired effects are achieved without causing any harm to the tissue or the stem cells themselves. In the future, LIPUS is anticipated to be applied to treat glaucoma, retinal degeneration, and other optic nerve injury diseases. The focus of future LIPUS research in the field of ophthalmology will be on how to enhance its efficiency and accuracy, as well as understanding the underlying biological mechanisms involved.
Author contributions
AD and ZL contributed to conception and design of the study. ZL wrote the first draft of the manuscript. NH, LG and XZ wrote sections of the manuscript. YZ and ZC participated in data analysis and interpretation. All authors contributed to the article and approved the submitted version.
Funding
Funding from Shandong Provincial Natural Science Foundation, China (No: ZR2020MH173).
Acknowledgments
The authors are grateful to the Weifang Medical University and Affiliated Hospital of Weifang Medical University for the encouragement and support for this work. The authors have no other relevant affiliations or financial involvement with any organization or entity with a financial interest in or financial conflict with the subject matter or materials discussed in the manuscript apart from those disclosed.
Conflict of interest
The authors declare that the research was conducted in the absence of any commercial or financial relationships that could be construed as a potential conflict of interest.
Publisher’s note
All claims expressed in this article are solely those of the authors and do not necessarily represent those of their affiliated organizations, or those of the publisher, the editors and the reviewers. Any product that may be evaluated in this article, or claim that may be made by its manufacturer, is not guaranteed or endorsed by the publisher.
References
1. Blau HM, Daley GQ. Stem cells in the treatment of disease. N Engl J Med (2019) 380:1748–60. doi: 10.1056/NEJMra1716145
2. Sender R, Milo R. The distribution of cellular turnover in the human body. Nat Med (2021) 27:45–8. doi: 10.1038/s41591-020-01182-9
3. Bhartiya D. Adult tissue-resident stem cells-fact or fiction? Stem Cell Res Ther (2021) 12:73. doi: 10.1186/s13287-021-02142-x
4. Mimeault M, Hauke R, Batra SK. Stem cells: a revolution in therapeutics-recent advances in stem cell biology and their therapeutic applications in regenerative medicine and cancer therapies. Clin Pharmacol Ther (2007) 82:252–64. doi: 10.1038/sj.clpt.6100301
5. Mimeault M, Batra SK. Recent progress on tissue-resident adult stem cell biology and their therapeutic implications. Stem Cell Rev (2008) 4:27–49. doi: 10.1007/s12015-008-9008-2
6. Behr B, Ko SH, Wong VW, Gurtner GC, Longaker MT. Stem cells. Plast Reconstr Surg (2010) 126:1163–71. doi: 10.1097/PRS.0b013e3181ea42bb
7. Mason TJ. Therapeutic ultrasound an overview. Ultrason Sonochem (2011) 18:847–52. doi: 10.1016/j.ultsonch.2011.01.004
8. Coiado OC, Lowe J, O'Brien WD Jr. Therapeutic ultrasound in cardiovascular medicine. J Ultrasound Med (2020) 40:1061–76. doi: 10.1002/jum.15493
9. Miller DL, Smith NB, Bailey MR, Czarnota GJ, Hynynen K, Makin IR. Bioeffects committee of the American institute of ultrasound in, overview of therapeutic ultrasound applications and safety considerations. J Ultrasound Med (2012) 31:623–34. doi: 10.7863/jum.2012.31.4.623
10. Jiang X, Savchenko O, Li Y, Qi S, Yang T, Zhang W, et al. A review of low-intensity pulsed ultrasound for therapeutic applications. IEEE Trans BioMed Eng (2019) 66:2704–18. doi: 10.1109/TBME.2018.2889669
11. Peng DY, Reed-Maldonado AB, Lin GT, Xia SJ, Lue TF. Low-intensity pulsed ultrasound for regenerating peripheral nerves: potential for penile nerve. Asian J Androl (2020) 22:335–41. doi: 10.4103/aja.aja_95_19
12. Zhou JX, Liu YJ, Chen X, Zhang X, Xu J, Yang K, et al. Low-intensity pulsed ultrasound protects retinal ganglion cell from optic nerve injury induced apoptosis via yes associated protein. Front Cell Neurosci (2018) 12:160. doi: 10.3389/fncel.2018.00160
13. Amini A, Chien S, Bayat M. Impact of ultrasound therapy on stem cell differentiation - a systematic review. Curr Stem Cell Res Ther (2020) 15:462–72. doi: 10.2174/1574888X15666200225124934
14. Harrison A, Lin S, Pounder N, Mikuni-Takagaki Y. Mode & mechanism of low intensity pulsed ultrasound (LIPUS) in fracture repair. Ultrasonics (2016) 70:45–52. doi: 10.1016/j.ultras.2016.03.016
15. Pearson C, Martin K. Stem cell approaches to glaucoma: from aqueous outflow modulation to retinal neuroprotection. Prog Brain Res (2015) 220:241–56. doi: 10.1016/bs.pbr.2015.04.005
16. Altshuler A, Amitai-Lange A, Tarazi N, Dey S, Strinkovsky L, Hadad-Porat S, et al. Discrete limbal epithelial stem cell populations mediate corneal homeostasis and wound healing. Cell Stem Cell (2021) 28:1248–1261 e8. doi: 10.1016/j.stem.2021.04.003
17. Braunger BM, Ademoglu B, Koschade SE, Fuchshofer R, Gabelt BT, Kiland JA, et al. Identification of adult stem cells in schwalbe's line region of the primate eye. Invest Ophthalmol Vis Sci (2014) 55:7499–507. doi: 10.1167/iovs.14-14872
18. Sun G, Asami M, Ohta H, Kosaka J, Kosaka M. Retinal stem/progenitor properties of iris pigment epithelial cells. Dev Biol (2006) 289:243–52. doi: 10.1016/j.ydbio.2005.10.035
19. Wohl SG, Schmeer CW, Isenmann S. Neurogenic potential of stem/progenitor-like cells in the adult mammalian eye. Prog Retin Eye Res (2012) 31:213–42. doi: 10.1016/j.preteyeres.2012.02.001
20. Du Y, Roh DS, Mann MM, Funderburgh ML, Funderburgh JL, Schuman JS. Multipotent stem cells from trabecular meshwork become phagocytic TM cells. Invest Ophthalmol Vis Sci (2012) 53:1566–75. doi: 10.1167/iovs.11-9134
21. Stern JH, Tian Y, Funderburgh J, Pellegrini G, Zhang K, Goldberg JL, et al. Regenerating eye tissues to preserve and restore vision. Cell Stem Cell (2018) 22:834–49. doi: 10.1016/j.stem.2018.05.013
22. Wang Y, Peng W, Liu X, Zhu M, Sun T, Peng Q, et al. Study of bilineage differentiation of human-bone-marrow-derived mesenchymal stem cells in oxidized sodium alginate/N-succinyl chitosan hydrogels and synergistic effects of RGD modification and low-intensity pulsed ultrasound. Acta Biomater (2014) 10:2518–28. doi: 10.1016/j.actbio.2013.12.052
23. Song S, Ma D, Xu L, Wang Q, Liu L, Tong X, et al. Low-intensity pulsed ultrasound-generated singlet oxygen induces telomere damage leading to glioma stem cell awakening from quiescence. iScience (2022) 25:103558. doi: 10.1016/j.isci.2021.103558
24. Menz MD, Oralkan O, Khuri-Yakub PT, Baccus SA. Precise neural stimulation in the retina using focused ultrasound. J Neurosci (2013) 33:4550–60. doi: 10.1523/JNEUROSCI.3521-12.2013
25. Sena K, Angle SR, Kanaji A, Aher C, Karwo DG, Sumner DR, et al. Low-intensity pulsed ultrasound (LIPUS) and cell-to-cell communication in bone marrow stromal cells. Ultrasonics (2011) 51:639–44. doi: 10.1016/j.ultras.2011.01.007
26. Tabuchi Y, Hasegawa H, Suzuki N, Furusawa Y, Hirano T, Nagaoka R, et al. Low-intensity pulsed ultrasound promotes the expression of immediate-early genes in mouse ST2 bone marrow stromal cells. J Med Ultrason (2020) 2001) 47:193–201. doi: 10.1007/s10396-020-01007-9
27. Xia P, Wang X, Qu Y, Lin Q, Cheng K, Gao M, et al. TGF-beta1-induced chondrogenesis of bone marrow mesenchymal stem cells is promoted by low-intensity pulsed ultrasound through the integrin-mTOR signaling pathway. Stem Cell Res Ther (2017) 8:281. doi: 10.1186/s13287-017-0733-9
28. An Y, Song Y, Wang Z, Wang J, Wu G, Zhu G, et al. Effect of low-intensity pulsed ultrasound on the biological behaviors of bone marrow mesenchymal stem cells on titanium with different surface topographies. Am J Transl Res (2018) 10:67–76.
29. Li F, Liu Y, Cai Y, Li X, Bai M, Sun T, et al. Ultrasound irradiation combined with hepatocyte growth factor accelerate the hepatic differentiation of human bone marrow mesenchymal stem cells. Ultrasound Med Biol (2018) 44:1044–52. doi: 10.1016/j.ultrasmedbio.2018.01.005
30. Lee ET, Lim KT, Kim JH, Im AL, Son HM, Cho CS, et al. Effects of low intensity ultrasound stimulation on the proliferation of alveolar bone marrow stem cell. Tissue Eng Regenerative Med (2008) 5:572–80.
31. Xie S, Jiang X, Wang R, Xie S, Hua Y, Zhou S, et al. Low-intensity pulsed ultrasound promotes the proliferation of human bone mesenchymal stem cells by activating PI3K/AKt signaling pathways. J Cell Biochem (2019) 120:15823–33. doi: 10.1002/jcb.28853
32. Yang X, Wu Y, Li J, Yin W, An Y, Wang Y, et al. A pilot study of parameter-optimized low-intensity pulsed ultrasound stimulation for the bone marrow mesenchymal stem cells viability improvement. Comput Math Methods Med (2019) 2019:8386024. doi: 10.1155/2019/8386024
33. Ning GZ, Song WY, Xu H, Zhu RS, Wu QL, Wu Y, et al. Bone marrow mesenchymal stem cells stimulated with low-intensity pulsed ultrasound: Better choice of transplantation treatment for spinal cord injury treatment for SCI by LIPUS-BMSCs transplantation. CNS Neurosci Ther (2019) 25:496–508. doi: 10.1111/cns.13071
34. Chen J, Jiang J, Wang W, Qin J, Chen J, Chen W, et al. Low intensity pulsed ultrasound promotes the migration of bone marrow- derived mesenchymal stem cells via activating FAK-ERK1/2 signalling pathway. Artif Cells Nanomed Biotechnol (2019) 47:3603–13. doi: 10.1080/21691401.2019.1657878
35. He RX, Chen JL, Jiang JW, Liu BR, Liang DD, Zhou WC, et al. Synergies of accelerating differentiation of bone marrow mesenchymal stern cells induce by low intensity pulsed ultrasound, osteogenic and endothelial inductive agent. Artif Cell Nanomed B (2019) 47:674–84. doi: 10.1080/21691401.2019.1576704
36. Huang D, Gao Y, Wang S, Zhang W, Cao H, Zheng L, et al. Impact of low-intensity pulsed ultrasound on transcription and metabolite compositions in proliferation and functionalization of human adipose-derived mesenchymal stromal cells. Sci Rep (2020) 10:13690. doi: 10.1038/s41598-020-69430-z
37. Wang Y, Jiang L, Xu T, Su Z, Guo X, Tu J, et al. p38 MAPK signaling is a key mediator for low-intensity pulsed ultrasound (LIPUS) in cultured human omental adipose-derived mesenchymal stem cells. Am J Transl Res (2019) 11:418–29.
38. Jiang T, Xu T, Guo FJ, Chen AM, Xiao ZZ, Zhang D. Osteogenic effect of low intensity pulsed ultrasound on rat adipose-derived stem cells in vitro. J Huazhong U Sci-Med (2012) 32:75–81. doi: 10.1007/s11596-012-0013-y
39. Yue Y, Yang X, Wei X, Chen J, Fu N, Fu Y, et al. Osteogenic differentiation of adipose-derived stem cells prompted by low-intensity pulsed ultrasound. Cell Proliferat (2013) 46:320–7. doi: 10.1111/cpr.12035
40. Uddin SMZ, Qin YX. Enhancement of osteogenic differentiation and proliferation in human mesenchymal stem cells by a modified low intensity ultrasound stimulation under simulated microgravity. PloS One (2013) 8:e73914. doi: 10.1371/journal.pone.0073914
41. Zhang Z, Ma Y, Guo S, He Y, Bai G, Zhang W. Low-intensity pulsed ultrasound stimulation facilitates in vitro osteogenic differentiation of human adipose-derived stem cells via up-regulation of heat shock protein (HSP)70, HSP90, and bone morphogenetic protein (BMP) signaling pathway. Biosci Rep (2018) 38:BSR20180087. doi: 10.1042/BSR20180087
42. Fu N, Yang X, Ba K, Fu Y, Wei X, Yue Y, et al. Low-intensity pulsed ultrasound induced enhanced adipogenesis of adipose-derived stem cells. Cell Proliferat (2013) 46:312–9. doi: 10.1111/cpr.12031
43. Nishida T, Nagao Y, Hashitani S, Yamanaka N, Takigawa M, Kubota S. Suppression of adipocyte differentiation by low-intensity pulsed ultrasound via inhibition of insulin signaling and promotion of CCN family protein 2. J Cell Biochem (2020) 121:4724–40. doi: 10.1002/jcb.29680
44. Nagasaki R, Mukudai Y, Yoshizawa Y, Nagasaki M, Shiogama S, Suzuki M, et al. A combination of low-intensity pulsed ultrasound and nanohydroxyapatite concordantly enhances osteogenesis of adipose-derived stem cells from buccal fat pad. Cell Med (2015) 7:123–31. doi: 10.3727/215517915X688057
45. Kang PL, Huang HH, Chen T, Ju KC, Kuo SM. Angiogenesis-promoting effect of LIPUS on hADSCs and HUVECs cultured on collagen/hyaluronan scaffolds. Mater Sci Eng C Mater Biol Appl (2019) 102:22–33. doi: 10.1016/j.msec.2019.04.045
46. El-Bialy T, Alhadlaq A, Lam B. Effect of therapeutic ultrasound on human periodontal ligament cells for dental and periodontal tissue engineering. Open Dent J (2012) 6:235–9. doi: 10.2174/1874210601206010235
47. Hu B, Zhang YY, Zhou J, Li J, Deng F, Wang ZB, et al. Low-intensity pulsed ultrasound stimulation facilitates osteogenic differentiation of human periodontal ligament cells. PloS One (2014) 9:e95168. doi: 10.1371/journal.pone.0095168
48. Li H, Deng Y, Tan M, Feng G, Kuang Y, Li J, et al. Low-intensity pulsed ultrasound upregulates osteogenesis under inflammatory conditions in periodontal ligament stem cells through unfolded protein response. Stem Cell Res Ther (2020) 11:215. doi: 10.1186/s13287-020-01732-5
49. Kusuyama J, Nakamura T, Ohnishi T, Eiraku N, Noguchi K, Matsuguchi T. Low-intensity pulsed ultrasound (LIPUS) promotes BMP9-induced osteogenesis and suppresses inflammatory responses in human periodontal ligament-derived stem cells. J Orthop Trauma (2017) 31:S4. doi: 10.1097/01.bot.0000520897.92470.70
50. Gao QH, Walmsley AD, Cooper PR, Scheven BA. Ultrasound stimulation of different dental stem cell populations: Role of mitogen-activated protein kinase signaling. J Endodont (2016) 42:425–31. doi: 10.1016/j.joen.2015.12.019
51. El-Bialy T, Alhadlaq A, Wong B, Kucharski C. Ultrasound effect on neural differentiation of gingival Stem/Progenitor cells. Ann Biomed Eng (2014) 42:1406–12. doi: 10.1007/s10439-014-1013-9
52. Lim K, Kim J, Seonwoo H, Park SH, Choung PH, Chung JH. In vitro effects of low-intensity pulsed ultrasound stimulation on the osteogenic differentiation of human alveolar bone-derived mesenchymal stem cells for tooth tissue engineering. BioMed Res Int (2013) 2013:269724. doi: 10.1155/2013/269724
53. Wu Y, Gao Q, Zhu S, Wu Q, Zhu R, Zhong H, et al. Low-intensity pulsed ultrasound regulates proliferation and differentiation of neural stem cells through notch signaling pathway. Biochem Biophys Res Commun (2020) 526:793–8. doi: 10.1016/j.bbrc.2020.03.142
54. Lv YG, Zhao PC, Chen GB, Sha YQ, Yang L. Effects of low-intensity pulsed ultrasound on cell viability, proliferation and neural differentiation of induced pluripotent stem cells-derived neural crest stem cells. Biotechnol Lett (2013) 35:2201–12. doi: 10.1007/s10529-013-1313-4
55. Xia B, Zou Y, Xu Z, Lv Y. Gene expression profiling analysis of the effects of low-intensity pulsed ultrasound on induced pluripotent stem cell-derived neural crest stem cells. Biotechnol Appl Biochem (2017) 64:927–37. doi: 10.1002/bab.1554
56. Lv YG, Nan PP, Chen GB, Sha YQ, Xia B, Yang L. In vivo repair of rat transected sciatic nerve by low-intensity pulsed ultrasound and induced pluripotent stem cells-derived neural crest stem cells. Biotechnol Lett (2015) 37:2497–506. doi: 10.1007/s10529-015-1939-5
57. Xu P, Gul-Uludag H, Ang WT, Yang XY, Huang M, Marquez-Curtis L, et al. Low-intensity pulsed ultrasound-mediated stimulation of hematopoietic stem/progenitor cell viability, proliferation and differentiation in vitro. Biotechnol Lett (2012) 34:1965–73. doi: 10.1007/s10529-012-0984-6
58. Liu BR, Luo YP, Luo D, Zhou WC, Zhang Y, He RX, et al. Treatment effect of low intensity pulsed ultrasound on leukopenia induced by cyclophosphamide in rabbits. Am J Trans Res (2017) 9:3315–25.
59. Ling L, Wei T, He L, Wang Y, Wang Y, Feng X, et al. Low-intensity pulsed ultrasound activates ERK1/2 and PI3K-akt signalling pathways and promotes the proliferation of human amnion-derived mesenchymal stem cells. Cell Prolif (2017) 50:e12383. doi: 10.1111/cpr.12383
60. Ling L, Feng X, Wei T, Wang Y, Wang Y, Zhang W, et al. Effects of low-intensity pulsed ultrasound (LIPUS)-pretreated human amnion-derived mesenchymal stem cell (hAD-MSC) transplantation on primary ovarian insufficiency in rats. Stem Cell Res Ther (2017) 8:283. doi: 10.1186/s13287-017-0739-3
61. Mohaqiq M, Movahedin M, Mokhtari Dizaji M, Mazaheri Z. Upregulation of integrin-alpha6 and integrin-beta1 gene expressions in mouse spermatogonial stem cells after continues and pulsed low intensity ultrasound stimulation. Cell J (2018) 19:634–9. doi: 10.22074/cellj.2018.4286
62. Omes C, Fassina L, Magenes G, Ogliari D, Tinelli C, Riva F. Biological effects of ultrasound stimulus on cells derived from human ovarian follicular liquid. Annu Int Conf IEEE Eng Med Biol Soc (2013) 2013:850–3. doi: 10.1109/EMBC.2013.6609634
63. de Lucas B, Perez LM, Bernal A, Galvez BG. Application of low-intensity pulsed therapeutic ultrasound on mesenchymal precursors does not affect their cell properties. PloS One (2021) 16:e0246261. doi: 10.1371/journal.pone.0246261
64. Crossman J, Alzaheri N, Abdallah MN, Tamimi F, Flood P, Alhadainy H, et al. Low intensity pulsed ultrasound increases mandibular height and col-II and VEGF expression in arthritic mice. Arch Oral Biol (2019) 104:112–8. doi: 10.1016/j.archoralbio.2019.05.032
65. Lei H, Xin H, Guan R, Xu Y, Li H, Tian W, et al. Low-intensity pulsed ultrasound improves erectile function in streptozotocin-induced type I diabetic rats. Urology (2015) 86:1241 e11–8. doi: 10.1016/j.urology.2015.07.026
66. He D, Wang J, Li Y, Wu G, Zhu G, Chen L. Low-intensity pulsed ultrasound promotes aggrecan expression via ZNT-9 in temporomandibular joint chondrocytes. Gene (2021) 768:145318. doi: 10.1016/j.gene.2020.145318
67. Zhou S, Schmelz A, Seufferlein T, Li Y, Zhao J, Bachem MG. Molecular mechanisms of low intensity pulsed ultrasound in human skin fibroblasts. J Biol Chem (2004) 279:54463–9. doi: 10.1074/jbc.M404786200
68. Liao B, Guan M, Tan Q, Wang G, Zhang R, Huang J, et al. Low-intensity pulsed ultrasound inhibits fibroblast-like synoviocyte proliferation and reduces synovial fibrosis by regulating wnt/beta-catenin signaling. J Orthop Translat (2021) 30:41–50. doi: 10.1016/j.jot.2021.08.002
69. Hua Z, Li S, Liu Q, Yu M, Liao M, Zhang H, et al. Low-intensity pulsed ultrasound promotes osteogenic potential of iPSC-derived MSCs but fails to simplify the iPSC-EB-MSC differentiation process. Front Bioeng Biotechnol (2022) 10:841778. doi: 10.3389/fbioe.2022.841778
70. Kusuyama J, Bandow K, Shamoto M, Kakimoto K, Ohnishi T, Matsuguchi T. Low intensity pulsed ultrasound (LIPUS) influences the multilineage differentiation of mesenchymal stem and progenitor cell lines through ROCK-Cot/Tpl2-MEK-ERK signaling pathway. J Biol Chem (2014) 289:10330–44. doi: 10.1074/jbc.M113.546382
71. Xia B, Chen G, Zou Y, Yang L, Pan J, Lv Y. Low-intensity pulsed ultrasound combination with induced pluripotent stem cells-derived neural crest stem cells and growth differentiation factor 5 promotes sciatic nerve regeneration and functional recovery. J Tissue Eng Regener Med (2019) 13:625–36. doi: 10.1002/term.2823
72. Bernal A, Perez LM, De Lucas B, Martin NS, Kadow-Romacker A, Plaza G, et al. Low-intensity pulsed ultrasound improves the functional properties of cardiac mesoangioblasts. Stem Cell Rev Rep (2015) 11:852–65. doi: 10.1007/s12015-015-9608-6
73. Maung WM, Nakata H, Miura M, Miyasaka M, Kim YK, Kasugai S, et al. Low-intensity pulsed ultrasound stimulates osteogenic differentiation of periosteal cells in vitro. Tissue Eng Part A (2021) 27:63–73. doi: 10.1089/ten.tea.2019.0331
74. Reddy P, Deguchi M, Cheng Y, Hsueh AJ. Actin cytoskeleton regulates hippo signaling. PloS One (2013) 8:e73763. doi: 10.1371/journal.pone.0073763
75. Wei FY, Leung KS, Li G, Qin JH, Chow SKH, Huang S, et al. Low intensity pulsed ultrasound enhanced mesenchymal stem cell recruitment through stromal derived factor-1 signaling in fracture healing. PloS One (2014) 9:e106722. doi: 10.1371/journal.pone.0106722
76. Gao Q, Cooper PR, Walmsley AD, Scheven BA. Role of piezo channels in ultrasound-stimulated dental stem cells. J Endod (2017) 43:1130–6. doi: 10.1016/j.joen.2017.02.022
77. Budhiraja G, Sahu N, Subramanian A. Low-intensity ultrasound upregulates the expression of cyclin-D1 and promotes cellular proliferation in human mesenchymal stem cells. Biotechnol J (2018) 13:e1700382. doi: 10.1002/biot.201700382
78. Wang YJ, Li J, Qiu Y, Hu B, Chen J, Fu TW, et al. Low-intensity pulsed ultrasound promotes periodontal ligament stem cell migration through TWIST1-mediated SDF-1 expression. Int J Mol Med (2018) 42:322–30. doi: 10.3892/ijmm.2018.3592
79. Su Z, Xu T, Wang Y, Guo X, Tu J, Zhang D, et al. Lowintensity pulsed ultrasound promotes apoptosis and inhibits angiogenesis via p38 signalingmediated endoplasmic reticulum stress in human endothelial cells. Mol Med Rep (2019) 19:4645–54. doi: 10.3892/mmr.2019.10136
80. Tan Y, Guo Y, Reed-Maldonado AB, Li Z, Lin G, Xia SJ, et al. Low-intensity pulsed ultrasound stimulates proliferation of stem/progenitor cells: what we need to know to translate basic science research into clinical applications. Asian J Androl (2021) 23:602–10. doi: 10.4103/aja.aja_25_21
81. Lin TC, Hsu CC, Chien KH, Hung KH, Peng CH, Chen SJ. Retinal stem cells and potential cell transplantation treatments. J Chin Med Assoc (2014) 77:556–61. doi: 10.1016/j.jcma.2014.08.001
Keywords: low-intensity pulsed ultrasound (LIPUS), stem cells, tissue endogenous stem cells, ophthalmic diseases, clinical application, mechanism, treatment
Citation: Lin Z, Gao L, Hou N, Zhi X, Zhang Y, Che Z and Deng A (2023) Application of low-intensity pulsed ultrasound on tissue resident stem cells: Potential for ophthalmic diseases. Front. Endocrinol. 14:1153793. doi: 10.3389/fendo.2023.1153793
Received: 30 January 2023; Accepted: 07 March 2023;
Published: 17 March 2023.
Edited by:
Yan Wang, Chongqing Medical University, ChinaReviewed by:
Chunhui Li, University of Dundee, United KingdomQingchun Mu, Hainan General Hospital, China
Copyright © 2023 Lin, Gao, Hou, Zhi, Zhang, Che and Deng. This is an open-access article distributed under the terms of the Creative Commons Attribution License (CC BY). The use, distribution or reproduction in other forums is permitted, provided the original author(s) and the copyright owner(s) are credited and that the original publication in this journal is cited, in accordance with accepted academic practice. No use, distribution or reproduction is permitted which does not comply with these terms.
*Correspondence: Aijun Deng, ZGVuZ2FpanVuQGhvdG1haWwuY29t