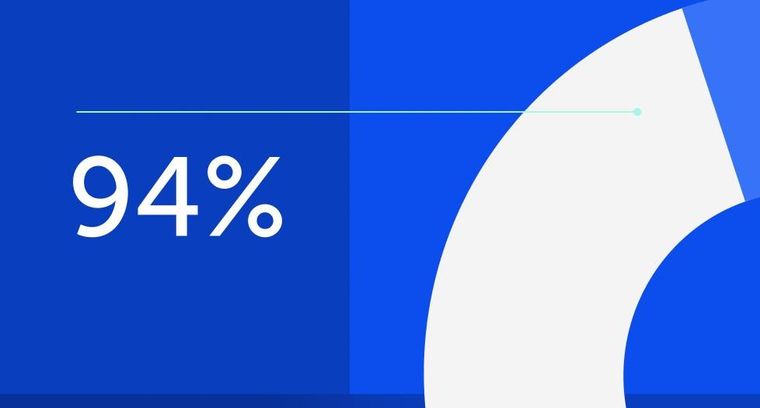
94% of researchers rate our articles as excellent or good
Learn more about the work of our research integrity team to safeguard the quality of each article we publish.
Find out more
REVIEW article
Front. Endocrinol., 20 March 2023
Sec. Cellular Endocrinology
Volume 14 - 2023 | https://doi.org/10.3389/fendo.2023.1150059
This article is part of the Research TopicAnti-obesity: Targeting Brown and Beige Adipocytes, Volume IIView all 5 articles
The ability to generate thermogenic fat could be a targeted therapy to thwart obesity and improve metabolic health. Brown and beige adipocytes are two types of thermogenic fat cells that regulate energy balance. Both adipocytes share common morphological, biochemical, and thermogenic properties. Yet, recent evidence suggests unique features exist between brown and beige adipocytes, such as their cellular origin and thermogenic regulatory processes. Beige adipocytes also appear highly plastic, responding to environmental stimuli and interconverting between beige and white adipocyte states. Additionally, beige adipocytes appear to be metabolically heterogenic and have substrate specificity. Nevertheless, obese and aged individuals cannot develop beige adipocytes in response to thermogenic fat-inducers, creating a key clinical hurdle to their therapeutic promise. Thus, elucidating the underlying developmental, molecular, and functional mechanisms that govern thermogenic fat cells will improve our understanding of systemic energy regulation and strive for new targeted therapies to generate thermogenic fat. This review will examine the recent advances in thermogenic fat biogenesis, molecular regulation, and the potential mechanisms for their failure.
Mammalian survival depends upon metabolic plasticity by responding to variations in food availability and environmental signals (1). These dynamic changes often trigger cellular adaptation, metabolic reprogramming, and altered energy homeostasis. For example, white adipose tissue can rapidly expand in response to a positive energy balance (2). This expansion relies on storing excess nutrients as triglycerides within lipid droplets of existing white adipocytes (hypertrophy). Alternatively, new white adipocytes can be recruited from adipocyte progenitor cell (APC) pools located within adipose depots (hyperplasia) (3). Opposing white adipose tissue accumulation and expansion are thermogenic fat cells—brown and beige adipocytes (4). Thermogenic fat cells can be recruited and activated in response to sympathetic nervous system (SNS) activation, such as cold temperatures (5, 6). Once activated, these cells utilize and combust glucose and free fatty acids to drive thermogenesis rather than cellular energy production (7). These observations highlight the importance of adipose tissue as a highly dynamic organ responding to nutritional cues and environmental stimuli to coordinate systemic metabolism. Thus, caloric excess and reduced variation in temperature challenges could support uncontrolled expansion and accumulation of white adipose tissue, potentiating metabolic dysregulation.
Indeed, the maladaptation of adipose tissue metabolic flexibility is strongly associated with obesity and chronic metabolic disease (8). For instance, sustained obesogenic signals foster white adipose tissue dysfunction, increasing the risk of developing type 2 diabetes, arteriosclerosis, hypertension, dyslipidemia, fatty liver disease, and premature death (9). Further, chronic overnutrition also augments adipose tissue inflammation, which modifies adipocyte function and insulin responses and, consequently, leads to adipocyte cell death (10). Adipose tissue dysregulation originates from various changes in mitochondria function and biogenesis, extracellular matrix accumulation, adipokine secretion profiles, lipid composition, and thermogenic fat cell development. In particular, the inability to generate thermogenic fat cells in aged and obese humans represents a significant clinical challenge to counteract white adipose tissue accumulation and expansion (11). Thus, strategies devised at increasing or rejuvenating thermogenic fat biogenesis and activation could be complementary treatments for obesity and its associated metabolic diseases (Figure 1). Here, we review the current understanding of thermogenic fat formation and decline and its benefit and discuss strategies to restore thermogenic fat in aged and obese humans.
Figure 1 Metabolic hallmarks of thermogenic fat. Chronic overnutrition promotes adipose tissue malfunction, increasing the risk for developing metabolic diseases, such as type II diabetes, hypertension, fatty liver disease, and cardiometabolic disease, and premature death. Thermogenic fat (brown and beige) biogenesis and activation offers promising potential to improve systemic energy expenditure and ameliorate obesity and its associated comorbidities. Illustration created with BioRender.com.
Three types of mammalian adipocytes exist—white, beige, and brown—spatiotemporally organized throughout the body (12–14). White adipocytes are specialized for energy storage but regulate various systemic physiologic and metabolic responses such as appetite, reproduction, and glucose and lipid metabolism (15). In contrast to the energy storage function of white adipocytes, brown and beige fat cells perform thermogenesis to increase energy expenditure. In 1551, Conrad Gesner first described and harbingered brown adipose tissue (BAT) in marmots as a mammalian hibernating gland (16). Yet, somewhat surprisingly, its function remained a mystery for over four centuries. In the early 1900s, preliminary studies used in vitro culturing and organotypic slices to reveal that BAT respired more than white fat or other non-adipose organs (16–18). Strikingly, the effect on respiration was heightened in response to hibernation and cold exposure, suggesting that BAT is a metabolically active organ required for temperature defense (19). Indeed, cold-adapted mammals utilize non-shivering thermogenesis to achieve temperature defense and stimulate BAT remodeling by increasing sympathetic innervation and vascularization (16). For instance, Hausberger and Widelitz showed that the vascularity of BAT within rats was four to six times higher than white adipose tissue but appeared comparable to resting skeletal muscle (20). The abundance of vascularity coupled with the thermogenic action of BAT provided an opportunity to establish the biothermic flow of blood and the vascular configuration within BAT lobes (16). These formative studies on the BAT vascular structure revealed the thermal conduction, exchanges, and organ series required for BAT to maintain homeothermy.
Differentiated brown adipocytes reside within BAT depots that are visually distinct compared to white fat cells. For instance, white adipocytes are circular, lack cytoplasmic volume, and contain a single large lipid inclusion (21). Conversely, brown adipocytes appear smaller, are polygonal, have more cytoplasmic volume, and have a dispersion of many tiny lipid droplets (multilocular) (21, 22). The multilocular lipid droplet organization of brown adipocytes is thought to assist in improving thermogenic substrate utilization by enhancing the lipid droplet surface area-to-volume ratio (23). Lipid droplet-associated proteins are thought to inhibit droplet coalescence, maintaining the multilocular phenotype of brown adipocytes (24). A recent study by Tontonoz and colleagues revealed that calsyntenin-3β (CLSTN3β)—a member of a family of proteins that regulate intracellular trafficking, synaptic function, and neuronal communication (25)—acts to confine lipid droplet-associated proteins averting droplet fusion to maintain the multilocular lipid droplet morphology (26).
Brown adipocytes contain numerous mitochondria that are large, closely packed, and contain laminar cristae compared to white adipocyte mitochondria, which appear small, elongated, and contain randomly oriented cristae (16). Differences in mitochondria number and structure correspond to the functional roles between energy utilization versus storage. In agreement, brown adipocytes express restricted transcription factors and co-factors that cooperate to induce mitochondria biogenesis and facilitate fatty acid oxidation. For example, peroxisome proliferator-activated receptor-γ (PPARγ) coactivator-1α (Pgc1α) is a transcriptional co−activator that unequivocally controls mitochondria biogenesis within brown adipocytes (27). While Pgc1α is noncompulsory for BAT development, it is required for cold temperature- and β3-adrenergic receptor (Adrb3)-agonist-induced brown adipocyte activation and thermogenesis. Consistent with this notion, Pgc1α is highly induced in response to cold exposure, allowing Pgc1α to interact with various transcriptional regulators (28). Thus, the discovery of BAT and brown adipocytes signified a mammalian adaptation designed to protect homeothermy.
BAT relies on a dense network of sympathetic neurons innervating the tissue to activate thermogenesis. Indeed, denervation studies have demonstrated the importance of sympathetic neurite dispersion throughout BAT to activate thermogenesis (5, 29, 30). To engage the SNS, thermoreceptors within the skin detect subtle cooling in the ambient temperature, which transmits these sensations along afferent neurons to the hypothalamus (31–33). The hypothalamus engages the postganglionic sympathetic nerve termini to release catecholamines (norepinephrine) into the BAT milieu to activate Adrb3 on brown adipocytes. Specifically, Adrb3 is a G protein-coupled receptor that engages adenylate cyclase activation in response to norepinephrine binding (34). Unlike other Adrbs, Adrb3 is primarily expressed in adipose tissue to initiate lipolysis and thermogenesis (35). Upon norepinephrine binding, Adrb3 is stimulated, initiating the downstream phosphorylation and activation of protein kinase A (PKA) and cyclic AMP (cAMP), facilitating lipolysis, beta-oxidation, and the transcriptional upregulation of thermogenic and mitochondrial biogenic proteins (36–38). Catecholamine release also stimulates brown adipocyte progenitors within the BAT stromal vascular fraction (SVF) to differentiate (29, 39–41). Moreover, brown fat sympathetic innervation may also serve as an interorgan communication network, specifically for those tissues with diffuse sympathetic innervation, such as white fat (42–44). Interestingly, the cholinergic receptor (CHRNA2), a nicotinic acetylcholine receptor, has also been shown to stimulate thermogenic fat development by activating PKA and cAMP signaling, independent of Adrb3 activation (45). What’s more is that immune cells located within subcutaneous fat appear to produce acetylcholine potentiating CHRNA2 activation (46). Regardless of activation, cAMP signaling culminates in the transcriptional upregulation of uncoupling protein 1 (Ucp1). Ucp1 is a mitochondrial inner membrane protein that reduces the proton potential between the mitochondrial intermembrane space and matrix, collapsing the electron transport chain and preventing ATP production (47). The resulting changes in the ATP/ADP ratio elevate the rate of substrate oxidization, such as lipids, continuing the uncoupling process of the electron transport change and the generation of heat (48).
While Ucp1 activity and regulation have been well-characterized in energy uncoupling, several studies have revisited the potential for Ucp1-independent thermogenic mechanisms. These studies originated from observations related to Ucp1 genetic modeling and the low expression level of Ucp1 in beige fat relative to brown adipocytes (49, 50). Consistent with Ucp1-independent thermogenic pathways, Ucp1-null mice show varied effects in response to cold exposure and diet-induced obesity, which appear to relate to vivarium temperature conditions and genetics (51). Yet, adipocyte-specific overexpression of Ucp1 prevents genetic obesity (52). Nevertheless, emerging adipocyte metabolic studies have focused on cellular redox status, purine nucleotide pool size, calcium signaling, succinate bioavailability, and creatine cycling as Ucp1-dependent and independent thermogenic mediators (reviewed in (53, 54)). Modulating several of these pathways in Ucp1-deficient mice, has revealed adaptations in macronutrient utilization and protection from diet-induced obesity, showcasing Ucp1-independent thermogenic pathways (54). Adding further to this notion is the identification that brown adipocytes appear to differ in Ucp1 status (low vs high) (55). Using the AdipoChaser mouse models combined with single-cell RNA sequencing and 3D tissue profiling, Song et al. (2020) found a coexistence of two brown adipocyte subpopulations—brown adipocytes that highly express Ucp1 and brown adipocytes that lowly express Ucp1. For instance, low Ucp1 expressing brown adipocytes were associated with lower adiponectin gene expression, larger lipid droplets, and lower mitochondria content (55). Even though Ucp1 expression is lower, these brown adipocytes appear to be specialized in fatty acid uptake. Yet, upon cold stimulus, low Ucp1 expressing brown adipocytes interconverted into high Ucp1 expressing brown adipocytes and vice versa in response to thermoneutral temperatures (55). These findings indicate that brown adipocytes are highly heterogenetic tissue and may be specialized in metabolic responses. Further, these findings suggest that some thermogenic fat cells are poised to become activated or deactivated in response to temperature changes. Overall, thermogenic fat cells appear highly dynamic and utilize various substrates to modulate energy uncoupling and function in Ucp1- dependent and independent mechanisms. The potential employment of these pathways may reflect substrate bioavailability, Ucp1 post-translational modification, and thermogenic fat stimuli (56). Moreover, the biological significance of Ucp1-independent thermogenic pathways has yet to be demonstrated in humans (57).
White and brown adipose depots are speculated to develop from a mesodermal cellular lineage, sharing a commonality with skeletal muscle, bone, and connective tissue (58). Like other mesodermal tissues, brown adipocytes are specified during embryogenesis and develop into distinct depots throughout the body. Indeed, mouse interscapular brown fat can be histologically observed as early as embryonic day 15.5 (E15.5) (59, 60). Yet, advances in cellular lineage tracing using the adiponectin promoter (AdipoChaser (61)) suggest that brown adipocytes can develop as early as E10 (55). These primitive BAT depots seem to be generated by hyperproliferative fibroblast-like cells that remain lipid depleted until the peripartum period (62, 63). To gain insight into the cellular ontology, early fate-mapping studies of embryonic precursors showed that brown adipocytes derived from the paraxial mesoderm of early somites within the dermomyotome (Figure 2). For example, using a marker of the central dermomyotome, engrail-1—a transcription factor involved in embryonic development and patterning (64, 65)—revealed that brown fat and skeletal muscle shared a common cellular history (66). In agreement, myogenic factor five (Myf5), an early myogenic transcription factor, lineage reporting studies demonstrated the shared cellular lineage between skeletal muscle and brown fat (67–69). Consistently utilizing two skeletal muscle transcription factor driven genetic tools, Pax3 and Pax7 (70, 71), to mark the developing muscle lineage, again showed embryonic muscle progenitors’ ability to develop into brown fat cells (71). While developmental muscle progenitors appear to be multipotent, myoblasts, a muscle lineage-committed progenitor, cannot generate brown adipocytes, suggesting lineage restriction after skeletal muscle commitment (72–74). Overall, genetic tools marking early embryonic muscle progenitors show the capacity to generate skeletal muscle and brown adipocytes.
Figure 2 Cellular ontology of thermogenic fat biogenesis. Multipotent progenitor cells located within the paraxial mesoderm of the dermomyotome can give rise to brown fat precursors that express myogenic markers. Though brown fat and skeletal muscle may share similar cellular ancestry, specific transcriptional factors regulate and facilitate brown adipocyte lineage specification and determination. Contrastingly, white and beige adipocytes arise from a Myf5- mesodermal origin, resemble mural cells, and reside in a perivascular niche. Upon cold exposure, these cells invoke a thermogenic transcriptional program and differentiate into beige adipocytes. Illustration created with BioRender.com.
Broadly, the growing lineage tracing evidence suggested that brown and white adipocytes arise from distinct embryonic progenitors. However, the recent re-examination of the Myf5 lineage identified that specific white adipocytes within restricted adipose depots are generated from a myogenic lineage (68, 69). Thus, revealing the mosaic contribution of the Myf5 lineage to white fat development. Consistent with this notion, Rodeheffer and colleagues, using the somite-specific Meox-1-Cre, showed that both dorso-axial white and brown adipocytes derive from the somitic mesoderm (75). In contrast to previous lineage data, only a subset of interscapular brown and white adipocytes originate from a Pax7+ lineage. These data favor an epaxial, not central, dermomyotome lineage for interscapular fat and the selective co-development of white and brown adipocyte depots (75). However, differences in lineage analysis studies could be attributed to Cre driver development (transgenic or knockin), the mouse strain genetic background, sex, reporter recombination efficiency, and spontaneous recombination and marking, which could influence cellular contribution and specification (76–79).
Because skeletal muscle and brown adipocytes share a common developmental cellular origin, decisive transcriptional machinery must influence cellular fate. Indeed, PR domain zinc-finger protein 16 (PRDM16) acts as a transcription factor molecular controller, favoring genes involved in brown fat cell formation over myogenesis (67). Even though Prdm16 is not required for brown adipocyte embryonic development, modulating Prdm16 can influence cell fate. For instance, in vitro overexpression of Prdm16 in a myoblast cell line—C2C12—induced brown adipocyte differentiation and induction of a thermogenic program. Conversely, downregulating Prdm16 in brown preadipocytes stimulates myotube formation (67). While Prdm16 is important in controlling brown adipocyte identity, the regulation and protein stability of Prdm16 has been undefine (80). Recent inroads into Prdm16 protein stability revealed a novel ubiquitin E3 ligase complex that catalyzes the polyubiquitination and degradation of the Prdm16 protein. Metabolically, when Prdm16 protein stability was extended by ablating the ubiquitin E3 ligase complex, mice resisted diet-induced obesity (81).
In addition to Prdm16, Early B Cell Factor-2 (Ebf2), an early developmental transcription factor, has been shown to specifically mark embryonic brown fat progenitors (63). In agreement, Ebf2 expression seems restricted to the brown fat somitic mesoderm lineage and is undetected within other lineages, including muscle. Consistent with this notion that Ebf2 is an initial driver of brown adipocyte lineage commitment and Ebf2 can cooperate with chromatin remodeling machinery to alter transcription factor accessibility at adipogenic genes such as the nuclear hormone receptor, peroxisome proliferator activated receptor gamma (Pparγ) (82). Notably, Pparγ is necessary and sufficient for adipogenesis and adipocyte regulatory function (83–86). Further studies examining inductive cues initiating Ebf2 expression and adipogenic commitment will provide insight into cellular fate and brown adipocyte lineage commitment. Beyond Prdm16 and Efb2, several other factors such as BMP7, C/EBPβ, EHMT1, EWS, and ZFP516 have been shown to facilitate brown fat adipocyte lineage specification and determination (87–90). These studies suggest multiple factors act as lineage switches and rheostats between skeletal muscle and brown fat cell types (Figure 2). In addition, these factors also appear to control brown adipocyte identity and thermogenic function.
While the developmental lineage progression of BAT has been recognized, less is known about adult BAT progenitor identity, induction, and regulation. Cold stimulus and diet are strong regulators of adult BAT homeostasis and growth. For example, thermoneutral conditions, an ambient temperature where the heat produced and heat loss are at equilibrium, diminish sympathetic tone, decreasing the requirement of the thermogenic program (50, 91). A reduction in thermogenic cues also supports brown adipocyte lipid accumulation and the acquisition of a unilocular white adipocyte-like appearance—hypertrophy. In contrast, cold exposure increases BAT mass via new brown adipocyte recruitment from a progenitor pool—hyperplasia. What might comprise the brown adipocyte progenitor pool? Genetic fate mapping, using platelet-derived growth factor receptor alpha (Pdgfrα) driven inducible Cre-reporter system, established that perivascular adventitial cells could generate brown fat cells (92). Notably, newly generated brown adipocytes from Pdgfrα+ cells predominantly occurred along the BAT dorsal edge. Additionally, Pdgfrα+ cells had the propensity to divide prior to adipogenesis (92). However, a potential confound is that Pdgfrα, a receptor tyrosine kinase involved initiating cellular signaling cascades that regulate cell growth and differentiation, is involved in the development and maintenance of numerous tissues, including the vascular system, the nervous system, organs, and connective tissues (93–96). In addition to Pdgfrα+ cells, single-cell RNA sequencing (scRNA-seq) technology identified a population of vascular smooth muscle cells expressing the transient receptor potential cation channel subfamily V member 1 (TRPV1), a membrane-bound calcium permeable channel that is part of the somatosensory system, which can be activated by temperature and pungent compounds (97, 98). Yet, overall there appears to be a lack of information on the adult brown adipocyte lineage and further investigation into the implications of the presumptive adult brown adipocyte precursor cell and their utility awaits.
In the early 1980s, researchers were cold acclimating female mice, they observed patches of brown-like adipocytes within perigonadal depots of white adipose tissues (14). Subsequently, similar studies using the selective Adrb3 agonist, CL316,243, revealed the occurrence of brown-like adipocytes within white adipose tissues, underscoring the SNS as a regulator of this “browning” process (99). Initially, these mitochondria-rich brown-like cells were thought to be dormant brown adipocytes but were activated by cold temperatures and norepinephrine signaling. However, the next four decades of research revealed a distinctive third type of adipocyte, dubbed beige/brite adipocyte (brown in white adipose tissue). Specifically, beige adipocytes are mitochondria enriched, multilocular in appearance, Ucp1 positive, and thermogenically competent. Initial studies using morphological characteristics and electron microscopy concluded that beige adipocytes derive from the trans-differentiation of mature white adipocytes. In support, Ucp1+ beige adipocyte fate mapping studies showed that thermogenic adipocytes do not vanish after cold temperature elimination; instead, they masquerade as white adipocytes (100). Interestingly, these cells retain a beige fat cell epigenetic memory and await cold re-exposure (101). Adding further to the fray is the possibility that mature Ucp1+ beige adipocytes can self-renewal and propagate in response to Adrb3 agonist administration. Additionally, uncoupling the cell cycle by p16Ink4a deletion facilitated the expansion of beige fat cells independent of stimuli, suggesting that beige adipocytes can perdure in the absence of stimuli and interconversion can be blocked (102). However, the utility of these cells and their contribution to metabolic homeostasis have not been fully elucidated. While these studies suggest that beige fat cells can interconvert from white adipocytes, several interesting questions remain. For instance, do beige-white adipocytes continue to confer metabolic benefits or the number of interconversions and the ability of cells to retain the beige epigenetic memory?
Understanding the cellular origins of a given tissue is a complex endeavor but delivers novel insight into tissue regulation and regenerative potential (58, 103). In opposition to the trans-differentiation hypothesis, an adipocyte-specific cell marking study using AdipoChaser suggested that the majority of beige adipocytes were generated from a non-adipocyte cellular pool (61). A follow-up study showed that initial cold exposure requires de novo beige adipogenesis. However, interconverting adipocytes were the primary source for the second bout of cold exposure (104). Lineage research then shifted from pre-existing adipocytes to test whether white adipocyte precursors could serve as white and beige fat cell progenitors. For instance, Pdgfrα+ adventitia fibroblasts appear bipotential, emerging into white and beige adipocytes, depending on the stimuli (105). Regarding beige adipogenic potential, Pdgfrα+ cells may only be engaged in response to the Adrb3 specific agonist, CL316,243, and not cold exposure (92, 96, 106). However, the cold temperature results seem mixed with some reports showing a 1-2% contribution to upwards of 20-30% new beige adipocytes emanating from Pdgfrα+ cells (96, 107). These variations in lineage contribution could be related to vivarium temperature conditions, different Cre-driven-reporter recombination efficiency, and the genetic background of the mice. On the other hand, using selective genetic lineage tools against smooth muscle cell markers such as smooth muscle actin (Sma; Acta2), myosin heavy chain 11 (Myh11), and Pdgfrβ, show that a majority of cold-induced beige adipocytes derived from a perivascular cellular source (106, 108, 109). Sma genetic fate mapping assessments demonstrate that Sma+ perivascular cells can generate ~50% of new beige adipocytes after one week of cold exposure (106). In contrast, smooth muscle cells marked by Myh11 and Pdgfrβ only generate beige adipocytes after two weeks of cold exposure (108, 109). Still, the extensive detail of when and where smooth muscle cells form beige fat across white adipose depots remains to be entirely determined. Further, single-cell RNA sequencing identified a CD81 marked perivascular cell population that could generate Ucp1+ beige adipocytes (110). Additionally, CD81+ cells also express Sma and other beige APC markers suggesting these cells may be bona fide beige progenitors. Beyond beige progenitor marking, CD81 also appears to control integrin-FAK signaling to initiate beige APC proliferation (110). In addition to mural progenitors, and reasonably so, several studies evaluated if beige adipocytes develop from the dermomyotome lineage, but these studies did not appear to overly support a myogenic developmental lineage (69, 106). However, recent evidence shows that some beige adipocytes are generated from a myogenic-MyoD+ precursor within white adipose tissues (111). Once generated, these MyoD-lineage-positive beige fat cells can catabolize glucose rather than free fatty acids (111). Collectively, multiple cellular pools can be employed to generate beige adipocytes at discrete anatomical locations within the adipose depots. Nevertheless, our understanding of why and when various cell types contribute to beige adipogenesis is underappreciated. Moreover, fate mapping data suggest that white and beige adipocytes may emanate from similar cellular APC pools (85, 106); however, are these pools discrete or overlapping? If overlapping, what might be the difference in cellular signals deciding their fate? The utility of single-cell sequencing coupled with lineage tracing and spatiotemporal niche labeling will help identify potential similarities and differences in progenitor populations and lineage trajectory.
Cold temperatures and Adrb3-agonism utilize aspects of the SNS but may differ in beige fat development and metabolic function. For example, within 24 hours of Adrb3 agonist administration, beige adipocytes can be observed, whereas cold-induced beige fat biogenesis occurs after several days (96). On the other hand, mechanistic studies have implied brown adipocyte activation and beige adipocyte recruitment are similarly activated through Adrb3. Yet, Adrb3 expression appears restricted to mature adipocytes and is undetected within the APC stromal vascular compartment (106, 112). Moreover, Adrb3-induced beige fat development appears to be independent of significant changes in adipocyte proliferation or the amount of DNA content (99, 113). Thus, do Adrb3 agonists stimulate beige APCs to produce beige fat cells? Using fate mapping analysis, neither Sma- nor Myh11-labeled beige APCs generated Adrb3-agonist-induced beige adipocytes (96, 106). For that matter, Pdgfrα+ cells generate roughly ~5-10% of all Ucp1+ Adrb3-agonist-induced beige adipocytes and even less (1-2%) in response to cold temperatures (92, 96, 105). Adipocyte-specific cell marking strategies found that most Adrb3-agonist-induced beige adipocytes emanated from pre-existing adipocytes (96, 104). In further support, Adrb3 genetic necessity tests showed that Adrb3 is dispensable for cold-induced beige fat formation (114). Moreover, mice lacking all three Adrbs (β-less mice) have repressed BAT function and defective beige adipogenic potential (115). Interestingly, if β-less mice slowly acclimate to cold temperatures over a 30-day period, cold tolerance is achieved. These observations suggest the existence of alternative pathways to induce and activate thermogenic fat but may depend on the beiging stimulus.
In contrast to Adrb3, Adrb1, a more ubiquitously expressed adrenergic receptor, is located within the white adipose SVF, not in mature adipocytes (96). Previous reports have posited that Adrb1 may mediate the proliferation and differentiation of classical brown adipocyte progenitors (116). Remarkably, Adrb1 null mice cannot defend their body temperature in response to cold temperature exposure (117). Yet, strikingly, Adrb1 transgenic overexpression invoked robust cold-induced beige fat formation (118). Moreover, treating mice with an Adrb1 inhibitor prevented cold-induced beige fat formation (96). While cold temperatures and Adrb3-agonism result in cAMP signaling, they may act differently on various adipose tissue cells to promote beige fat biogenesis (106). But what might be the advantage of multiple cellular pools for generating beige adipocytes? Do beige adipocytes have different metabolic functions depending on the cellular origin? Future research aimed at teasing apart beige fat development and cellular sources could inform on best practices for inducing beige fat under various conditions such as obesity, aging, or environmental temperatures.
To gain glimpses into metabolic heterogeneity between cold- and Adrb3-induced beige fat biogenesis, Soloway and colleagues used single-nucleus assay for transposase-accessible chromatin sequencing (snATAC-seq) to examine potential differences. Consistently, both stimuli increased accessibility at genes involved in thermogenesis, lipogenesis, and beige adipocyte development; however, the cellular kinetics and magnitudes appeared distinct (119). Moreover, changes in lipogenic genes, for example, were linked to changes in the lipid composition of the adipose tissue. Interestingly, both stimuli decreased the proportion of palmitic acid, a saturated fatty acid, whereas Adrb3 activation favored an increase in monounsaturated fatty acids. In contrast, cold exposure increased the proportion of polyunsaturated fatty acids (119). Consistent with this notion, Adrb3-agonist-induced beige adipocytes express higher levels of medium chain acyl-CoA dehydrogenase (MCAD) protein, an essential mitochondrial enzyme required for lipid oxidation (120). While these findings reveal common and distinct mechanisms of cold and Adrb3-induced beiging, there is a paucity of information regarding the functionality and metabolic consequences of the beige stimuli. For example, might these identified lipids have biological activity that can alter metabolic or thermogenic processes? Indeed, 12,13 diHOME, a fatty acid metabolite, identified as a cold-induced lipokine, can enhance fatty acid utilization and promotes fatty acid uptake and oxidation in myocytes (121). In the same vein, the generation of highly glycolytic beige adipocytes, from MyoD+ APCs, also reveals metabolic substrate specificity (111). Interestingly, the snATAC data (from above) also demonstrated that cold-induced beige adipocytes had increased accessibility at glycolytic processing genes, whereas Adrb3 activation increased cAMP responses. In agreement, glycolytic beige adipocytes are enriched in two enzymes that regulate glycolysis, enolase-1 and the isoenzyme of pyruvate kinase (PKM2), suggesting these cells are specialized in higher glucose utility (111). In addition to increased glucose and free fatty acid consumption, beige adipocytes enhance branch chain amino acid (BCAA) uptake and oxidation (122). This process requires SLC25A44, a BCAA mitochondria transporter. Defects in SLC25A44 impair BCAA transport and oxidation, consequentally, reducing thermogenesis and increasing susceptibility to diet-induced obesity (122). Yet might BCCA uptake and processing differ between beiging stimuli and temperature conditions? Overall, different thermogenic inducers may invoke similar but distinct metabolic programs which may derive from their cellular origin. Together, these studies suggest that thermogenic fat cells have an array of metabolic substrates, but their collective contribution to human thermogenesis and metabolism is unclear.
Human brown adipocytes develop in infants and are retained in young children, which is thought to protect newborns from hypothermia (123, 124). But beyond the postnatal years, adult humans were thought to have limited BAT deposits, with only several reports showing detectable human BAT under unique conditions (125). For instance, cold-acclimated outdoor workers in Finland were reported to have BAT deposits located in strategic sites within the body (126). Additional BAT observations were limited to postmortem dissection and autopsies reports (127). In the late 2000s, 18F-fluorodeoxyglucose positron emission tomography (FDG/PET) to trace tumor metastasis revealed a compilation of scans showing the appearance of active glucose uptake in human adipose depots (128–131). In some incidences, more glucose uptake could be observed in FDG/PET scans of adipose depots from patients that perceived to be or felt cold (132). Moreover, apparent hypermetabolic human BAT could even be reversed in patients with a warming protocol, suggesting the possibility of cold temperature-induced human thermogenic fat cells (133). These findings were solidified in a series of FDG/PET-scans, immunohistochemical, and genetic studies identifying, unequivocally, the presence of adult human thermogenic fat cells at distinct anatomical positions (cervical, supraclavicular, axillary, mediastinal, paraspinal, and abdominal) (128–131). Interestingly, a study using single-photon emission computed tomography (SPECT/CT) coupled with lipid tracers identified new mouse BAT depots having a similar topology to human adipose deposits (134). Further histological and molecular characterization of these putative human BAT depots will aid in defining their thermogenic competencies and metabolic capacity.
The discovery of human BAT spurred multiple studies examining the role of thermogenic fat in human health, which showed a negative association with body mass index and adiposity, heralding thermogenic fat as an anti-obesity target (135). The presence of thermogenic fat is also positively correlated with insulin sensitivity (136). Moreover, higher human BAT prevalence is associated with a reduction in cardiometabolic diseases such as hypertension, coronary artery disease, and congestive heart failure (137). Human BAT presence is also correlated with body fat redistribution, protecting metabolically healthy subcutaneous fat while reducing visceral adiposity. These changes were also associated with fewer incidents of type 2 diabetes and fatty liver disease (138). Metabolically, PET scanning, using both glucose and lipid tracers, has successfully demonstrated the capacity of BAT to consume substrates and create a metabolic sink (139). For example, using the fatty acid tracer, 18F-fluoro- thiaheptadecanoic acid (18FTHA) to quantify BAT oxidation in cold-exposed healthy males, showed a substantial increase in nonesterified free fatty acid uptake (140). Correspondingly, BAT radio-density increased after cold exposure suggesting that brown adipocytes were actively lipolytic. Indeed, BAT activation in humans is associated with accelerated lipid metabolism and a 45-fold change in BAT mitochondrial thermogenic potential (139). Furthermore, BAT-induced glucose and free fatty acid uptake are inversely correlated with shivering and associated with a 1.8-fold increase in total energy expenditure in cold-exposed humans (140). Interestingly, in mice lacking Ucp1, FDG/PET scans reveal similar glucose uptake, suggesting fuel uptake can be separated from its oxidation (141). Therefore, uptake and oxidation must be consideration when probing human thermogenic fat function.
How much thermogenic fat do humans have? This question is challenging to answer due to whole-body imaging techniques—PET/MRI—to identify and define anatomical BAT locations (142). A further confounder is that BAT amount and presence vary by age and sex (143). More troubling is that BAT activity and amount are inversely associated with body mass index, with obese patients tending to have low to undetectable levels of BAT. In addition, the amount of BAT present in lean young adults’ ranges from 0.1 to 0.5% of total body mass (144). What’s more, humans, proportionally, have significantly less BAT than smaller mammals, which appears insufficient to support metabolic demand. In support, Wolfrum and colleagues used single nuclei RNA sequencing to profile mouse and human adipocyte heterogeneity to identify a subpopulation of high temperature (warming) induced adipocytes (145). This subpopulation appears to negatively influence the adipose tissue niche by modulating acetate bioavailability, stifling the number of thermogenic fat cells and activation. Human biopsies revealed an abundance of these anti-thermogenic adipocytes, which may explain why human fat has a lower thermogenic potential (145). Nevertheless, it remains to be determined if or how these cells function to control thermogenic fat bioavailability and their potential role in systemic metabolism and obesogenic conditions. While humans have metabolically activated BAT, which could influence energy expenditure, the critical question is: can humans recruit enough BAT to offset obesity and metabolic disease? Enthusiastically, both retrospective and prospective studies show that even limited thermogenic tissue can be inversely correlated with body mass, suggesting metabolic utility (146, 147).
At the cellular level, brown and beige adipocytes appear morphologically similar. Moreover, isolated mouse brown and beige adipocytes have the equivalent thermogenic capacity and Ucp1 induction after norepinephrine administration (148). Additionally, a combination of MRI scans and molecular analysis revealed that human infant interscapular BAT resembles classical mouse interscapular BAT. In contrast, human supraclavicular and retroperitoneal depots genetically mirrored rodent beige adipose tissue (149). To test if there are unique differences between beige and brown adipocytes, Wu and colleagues evaluated global gene expression profiles and identified common thermogenic genes but also found divergent genes within beige adipocytes, such as Tmem26 and CD137. Moreover, human thermogenic fat has a genetic signature resembling rodent beige adipocytes rather than classical brown adipocytes (150–152). Yet this comparison could be masked due to the physiological state of the mice. For example, standard mouse procedures are performed at room temperature (~20-23°C); however, this is considered a thermal stress for mice, resulting in metabolic rate doubling (91). This would be equivalent to constant exposure of an unclothed human at 10°C (153). Humans have near domination over their thermoneutrality by modifying their environment and mitigating temperature fluctuations (i.e., clothing). Thus, is the correct comparison between thermoneutral humans and thermal stressed mice or thermoneutral humans and humanized mice? This debate is ongoing, and future research to elucidate the cellular identity of human thermogenic fat will be critical for understanding the molecular underpinning and focusing therapies (154).
While multiple studies have shown that cold exposure can stimulate human thermogenic fat, can pharmacological approaches be considered? Targeting Adrbs in most circumstances appears contraindicative for thermogenic intervention due to adverse cardiac events. However, a specific Adrb3-agonist, Mirabegron, has been designed and food and drug administration (FDA)-approved to treat patients with overactive bladder (155). Using a single dose (50 mg), Mirabegron increased glucose uptake and energy expenditure in healthy adult humans (156). Unfortunately, but as possibly anticipated, adverse cardiac-associated events (i.e., increased blood pressure) were observed in Mirabegron-treated patients (156, 157). Yet, treating obese subjects with mirabegron activated thermogenic fat tissue and increased glucose uptake and was associated with few cardiovascular events (158). While cold exposure and Adrb3-agonist stimulate thermogenesis, these methods are inconvenient, cumbersome, and elevate heart rate and blood pressure. Going forward, developing pharmaceuticals diverging from Adrb activators and focusing on alternative thermogenic pathways or stimulating fat cell progenitors will be critical. However, these endeavors may prove challenging as thermogenic targets could have opposing effects on systemic physiology.
Thermogenic fat has clinical utility to counter metabolic imbalance and reduce adiposity; however, a key challenge has been the effectiveness of inducing beige fat in aging and obese humans. While short-term cold exposure increases insulin sensitivity and glucose uptake in healthy adults with active thermogenic fat (136), this effect is significantly blunted in obese subjects compared to normal-weight individuals (159). For reasons not entirely understood, the ability to generate cold-induced beige fat declines with increased adiposity and age (Figure 3). For example, Yoneshiro et al., showed that less than 10% of BAT activity could be detected in 50- and 60-year-old men and women, whereas young adults (~20s) showed >50% of BAT activity after 2 hours of cold exposure (160, 161). Similarly, in mice, aging causes a programmed decline in beige fat development within subcutaneous adipose tissue (162). These observations could, in part, contribute to the age-dependent accumulation of fat mass and the so-called “slowing” of metabolism in aging mammals. What is clear is that aging is associated with changes in visceral adiposity, reduced exercise capacity, impaired muscle maintenance and growth, increased peripheral nerve damage (neuropathy), the inability to regulate body temperature, and the constant feeling of cold (163, 164). Consistent with dysregulated homothermic control is the decrease in sympathetic innervation in aging humans (165). In agreement, while basal adipocyte lipolytic rates remain similar between young and aged human subcutaneous fat depots, catecholamine-stimulated lipolysis is 50% less (166). The disruption to transmit catecholamine signaling appeared to depend on hormone sensitive lipase activation and not necessarily, Adrb expression (166). In contrast, rodent studies suggest that sympathetic tone is elevated in aged mice demonstrating, more sympathetic stimulation (167). However, aging does not affect Adrb3 density on brown adipocyte membranes, suggesting that stimulation is preserved but not activated (168). Notably, few studies have examined the relationship between BAT activity and human genetic variation. For instance, single nucleotide polymorphisms found in the human UCP1 and ADRB3 genes are correlated with the age-dependent decline in thermogenic fat and augmentation of adiposity in healthy Japanese adults (169, 170). Nevertheless, what might account for the overall decline in thermogenic fat biogenesis and activation in the aging population?
Figure 3 The decline in thermogenic fat biogenesis. Environmental, biochemical, and dietary activators can induce beige adipocyte formation in humans. In lean and young individuals, healthy white adipose tissue is freely able to perform beige-white adipocyte conversion depending on stimuli. In obese and aged settings, beige APCs become disrupted and display a senescent signature, becoming unresponsive to beige adipogenic stimuli. Moreover, changes in beige APCs facilitate fibrotic tissue replacement and inflammation. Additionally, aging is associated with changes in mitochondria function and thyroid hormone sensitivity resulting dysregulated white-to-beige interconversion. Together, age- and obese-associated changes in adipose tissue homeostasis stifle thermogenic fat biogenesis and metabolic protection. Illustration created with BioRender.com.
Age-associated changes in beige fat biogenesis also appear to be driven, in part, by beige progenitor dysfunction, ultimately becoming unresponsive to cold signals. The reason(s) why these beige APCs become dysfunctional is vague, but it may emanate from a cellular aging-senescent-like phenotype. That is, with age, beige APCs begin to express a bevy of senescent markers (p16Ink4a and p21), senescence activated β-galactosidase, and the senescence-associated secretory phenotype (SASP) (171). Age-associated changes in SASP signals, such as Tnfα and IL6, can further disrupt the tissue microenvironment facilitating inflammatory, necrotic, and senescent signals onto neighboring cells (172, 173). Consistent with the idea that senescent cells can be detrimental to tissue function, elegant studies designed to remove senescent cells—genetically or pharmacologically—from adipose tissue have demonstrated significant improvement in adipocyte tissue function and health (174). For example, removing white adipose tissue resident p21high cells prevents and alleviates insulin resistance in obese mice (175). In agreement with these findings, deleting senescence inducers reinstates beige APC adipogenic potential and some metabolic benefits in aged mice. Strikingly, these age-dependent changes in senescence machinery and beige adipogenic failure were confirmed in white adipose tissue stromal cells from adolescent and adult humans (171). Mechanistically, cellular aging signals involve the activation of the p38/MAPK pathway, acting as a central node in regulating cellular senescence and aging genes. In support, upregulating the p38/MAPK senescence pathway within beige APCs prematurely blocks beige adipocyte development in young mice. Alternatively, pharmacologically blocking p38/MAPK in aged beige APCs rejuvenated beige fat development and improved aspects of systemic metabolism (171). Furthermore, p53, acting downstream of p38/MAPK signaling, is upregulated in aged white adipose tissue, and genetic and pharmacological blockade of p53 restores beige adipogenesis, potentially via mitophagy (176) (Figure 3). Yet, several important questions remain regarding the cellular aging processes; for example, what are the upstream signaling events that promote p38/MAPK hyperphosphorylation? For that matter, how does white adipose tissue aging affect the beige APC niche and vice versa? Answers to these questions might provide preventive clues for new senolytic-like compounds to restore beige fat in aged humans to boost metabolism and prevent middle-aged waist expansion (174, 177, 178).
While cellular aging appears to contribute to age-associated beige adipogenic failure, other cellular aging mechanisms that facilitate thermogenic fat decline may exist. For instance, Seale and colleagues demonstrated that cold exposure prevented beige APCs from adopting a fibrogenic phenotype (Figure 3) (179). In contrast, aging appeared to promote the fibrogenic phenotype, which correlated with the loss of Prdm16. Indeed, restoring Prdm16 levels in aged mice resulted in the rejuvenation of beige adipogenesis. This Prdm16-mediated process appears to involve β-hydroxybutyrate, a ketone body, which may reflect why ketogenic diets may successfully reduce adiposity and improve systemic metabolism.
Age-associated changes in mitochondria biogenesis and function may also facilitate the decline in thermogenic fat development. Mitochondria dysfunction is characterized by increased DNA damage, elevated reactive oxygen species, and decreased mitochondria biogenesis and oxidative phosphorylation (180). Thermogenic fat cells represent a unique cell type to examine mitochondrial biogenesis and clearance due to increased mitochondria number and activity. Damaged mitochondria are removed by a process known as mitophagy, an autophagic process targeting mitochondria (181). Because thermogenic fat cells can interconvert into white adipocytes, mitophagy is engaged to clear mitochondria, expediting the conversion. For instance, Kajimura and colleagues demonstrated that mitophagy removes the excess mitochondria to adopt a white fat–like phenotype after the withdrawal of cold-stimulus or Adrb3-agonist (182). Pharmacologically blocking or deleting genes associated with autophagy-mediated mitochondrial clearance preserves the beige adipocyte phenotype and function. Moreover, blocking mitophagy promotes the perdurance of beige fat and prevents obesity and glucose intolerance (183). Further investigation into age-associated decline in mitophagy could trigger the precipitous decline in thermogenic fat. For instance, aging may be associated with changes in autophagy-related genes, such as ATG5, ATG12 or PARK2, rapidly converting thermogenic fat cells into white adipocytes (182, 183). Consistent with this notion, the age-related downregulation of the epigenetic eraser, Lsd1, a genetic regulator of the mitophagy machinery, leads to the inability to induce thermogenic fat. Alternatively, overexpressing Lsd1 promotes a thermogenic fat cell phenotype (184, 185). Moreover, a recent report indicated that brown adipocytes release extracellular vesicles that contain oxidatively damaged mitochondrial parts to avoid thermogenic failure. BAT resident macrophages remove these extracellular vesicles; however, thermogenesis is dampened if macrophage function or accrual is disrupted (186). Further investigation into mitophagy and mitochondrial regulatory processes could provide new insight into the regulation of thermogenic fat cell viability and longevity, especially in the aged setting.
Thyroid hormones, triiodothyronine (T3) and thyroxine (T4), which can activate the thyroid hormone receptor to control gene transcription, can regulate BAT activation and differentiation thereby governing energy expenditure (187–190). While hyperthyroidism causes hyperthermia, the mechanisms remain controversial but may be partly mediated by skeletal muscle and by elevating the body’s temperature setpoint (191). This effect is exemplified in rodents demonstrating that T3 augments heat loss via the tail vein (192). Yet, T3 can also directly stimulate hypothalamic neurons to engage BAT thermogenesis (193, 194). While T3 and T4 can induce Ucp1 expression and thermogenic fat formation, aging is associated with a decrease in serum T3 and a reduced conversion of T4 to T3 (195). Correspondingly, human association studies suggest that UCP1 expression corresponds with circulating thyroxine serum levels, suggesting that lower thyroid hormone status could indicate reduced thermogenic potential, particularly with advanced aging (190). Yet, thyroid hormone-induced Ucp1-positive beige adipocytes may be decoys. Intriguingly, while these cells resemble beige adipocytes and express Ucp1, they appear metabolically inactive without adrenergic stimulation (196). Moreover, selective hyperthyroidism in Ucp1-null mice maintains metabolic and thermogenic responses (197). Suggestively, thyroid-induced hyperthermia is independent of brown and beige adipocytes and may involve Ucp1-independent thermogenic thyroid hormone mechanisms (196). A longitudinal study examining thyroid carcinoma patients undergoing thyroidectomy that receive the thyroid hormone (T4) replacement analog, levothyroxine, displays a boost in energy expenditure and glucose uptake into thermogenic fat depots (198). Consistently, a T3 analog, liothyronine, significantly decreased insulin resistance in patients with an insulin receptor mutation (199). While the data suggest that thyroid hormone and its analogs can stimulate hyperthermia, it may be unlikely that these effects are solely thermogenic fat dependent. Moreover, it is unclear how thyroid levels and thyroid receptor signaling mediate thermogenic fat biogenesis in aged mammals. Further, thyroid analogs show promise in thyroid cancer clinical trials, yet can these thyroid mimetics stimulate active thermogenic fat within the obese and aging populations to augment energy expenditure, remains to be clarified.
Obesity and aging are associated with increased pro-inflammatory cytokine production in white and brown adipose tissue (200). These changes in cytokine composition further recruit and activate M1 macrophages and several other immune cell populations that facilitate tissue disruption and chronic low-grade inflammation (Figure 3). Moreover, pro-inflammatory cytokines disrupt cold-induced thermogenesis by directly suppressing thermogenic gene expression and dampening metabolic fluxes. Cold temperature exposure can remodel white adipose tissue immune cell composition, including M2 macrophages, mast cells, eosinophils, and type 2 innate lymphoid cells (ILC2s). For example, while ILC2s can support tissue homeostasis, they appear necessary for mediating energy balance in response to changes in environmental temperatures (201, 202). ILC2s are recruited and activated by the cytokine, interleukin-33 (IL-33), and once activated, produce an opioid-like peptide, methionine-enkephalin (MetEnk) peptide, that can stimulate beige fat development. It is unspecified if MetEnk mediates de novo beige adipogenesis or white-to-beige interconversion or its function mechanism (201). Alternatively, ILC2 and eosinophil byproducts, such as IL-13 and IL-4, have been shown to directly act on beige adipocyte progenitors to stimulate proliferation and differentiation (202, 203). Likewise, beige APCs also have been shown to produce and secrete IL-33 to facilitate a positive-beige-ILC2 adipogenic feedback circuit (204, 205). Nevertheless, this circuit may deteriorate with advanced aging and obesogenic signals, causing changes to beige fat generation, yet the exact mechanisms remain unknown (206). Moreover, a recent study showed the emergence of an age-dependent regulatory cell (ARC) population within white adipose tissue. ARCs resemble APCs but lack adipogenic capacity; instead, these cells secrete high levels of pro-inflammatory chemokines, including Ccl6, to inhibit the proliferation and differentiation of neighboring adipose precursors (207). Yet, the involvement of ARCs in beige fat biogenesis is unknown. Consistent with changes in pro-inflammatory cytokines, suppressing interleukin-10 (IL-10) induces thermogenic gene expression, whereas deleting the IL-10 receptor enhances beige fat formation and blunts obesity (208). More broadly, using single-cell RNA sequencing, Farmer and colleagues developed a comprehensive atlas of the cellular and transcriptional changes varying between cold temperature exposure and Adrb3-agonism. The analysis revealed that Adrb3 stimulated the interferon/Stat1 pathways, favoring myeloid immune cell accrual, whereas cold promoted lymphoid immune cell recruitment (209). Yet, the physiological function and molecular mechanisms of why certain beige stimuli favor specific immune populations remain to be fully understood.
While the beneficial effects of exercise on human health are well appreciated, the ability of exercise to induce thermogenic fat biogenesis and activation appears mixed and may be species-specific (reviewed in (210)). For instance, a bout of exercise in rodents can generate thermogenic fat cells and upregulate the expression of thermogenic genes such as Prdm16 and Ucp1 in white adipose tissue (211, 212). Yet, in humans, there is a lack of association between exercise and thermogenic fat production in white fat depots (213–216). For example, lean and obese individuals that underwent endurance exercise training for 10-16 weeks did not elevate thermogenic gene expression in subcutaneous adipose tissue (217). Additionally, no changes in Ucp1 expression have been observed in human populations with active lifestyles compared to sedentary populations (218, 219). It is still being determined why these differences between humans and rodents exist. As discussed above, a potential confound could be the differences in ambient temperatures in which the experiments were conducted. These findings also pose an interesting physiological question, why would exercise generate thermogenic fat cells? The rodent exercise-induced beiging appears counterintuitive to the actual heat dissipation function of thermogenic fat cells (220, 221). A possible explanation is that exercise could diminish BAT function to maintain homeostatic temperature. This is conceivable because both thermogenic fat and exercise increase energy expenditure and thermogenesis (220, 221). Simplistically, exercise can also stimulate sympathetic activity, thus, by default, enhancing thermogenic fat biogenesis (222). On the other hand, exercise can stimulate the release of muscle- and adipocyte-derived secretory hormones (myokines and adipokines) that can facilitate thermogenic fat development. For example, irisin (223), myostatin (224), meteorin-like1 (Metrnl) (225), lactate (226), and b-aminoisobutyric acid (BAIBA) (227) can be released from muscle during exercise to stimulate thermogenic fat development. Even less convincing is the effect of exercise on classical BAT. For example, several studies have reported no effect or a decrease in BAT activity in response to exercise (212, 228). However, cold water swim tests suggest cooperation between cold temperature exposure and exercise to increase BAT mass. Nevertheless, human BAT exercise studies are lacking, which could be attributed to the decline in thermogenic fat tissue in aging humans. Additional exploration into the physiology and molecular mechanisms underlining the effects of exercise on thermogenic fat adaptation and decline remains to be fully elucidated.
Most individuals do not prefer to spend several hours per day in a cold chamber activating thermogenic fat. Thus, developing and implementing more tolerable and potentially desirable methods should be employed to promote thermogenic fat biogenesis. For thousands of years, diet, nutritional ingredients, and natural compounds have provided therapeutic and medicinal effects on human metabolism and whole-body physiology. These alternative approaches could harbor tactics to avoid cold exposure or synthetic compounds altogether. For instance, besides cold exposure, the SNS can also be engaged by stress, inflammation, and diet. Diet is particularly interesting because it can be a modifiable and controllable aspect of human life. Furthermore, diet appears to induce obligatory and facultative thermogenesis. Obligatory thermogenesis entails heat generation during digestion, absorption, and processing of dietary components, often referred to as the thermogenic effect of food. Facultative thermogenesis is the breakdown of macronutrients and the activation of classical BAT thermogenesis, resulting in heat dispersion from food energy. Yet, the role of diet in controlling thermogenesis has not been largely undefined; however, studies using specific diets, such as the ketogenic diet, suggest that certain food may potentiate or even block thermogenic fat activity (229). Moreover, single-meal studies suggest that food can alter BAT activity immediately. For instance, FDG PET scanning revealed less glucose uptake into BAT depots in healthy human participants 90 minutes after a meal (230, 231). These results suggest that diet may negatively impact BAT activity, yet a possible explanation could involve insulin mediated FDG uptake into muscle, thereby limiting the free FDG for BAT utilization. This explanation could justify the underestimate in BAT glucose uptake—alternative measurements, such as oxygen uptake, may be more applicable for measuring mitochondrial function and BAT activity (232). Indeed, a recent report demonstrated that BAT oxygen and blood flow rose immediately after a single meal. In further support, participants with more BAT had higher total energy expenditure after meals than individuals with lower BAT activity (233). These studies suggest that diet can affect BAT activity; however, more investigation is needed to elucidate how cold exposure and diet could be coupled to control BAT activity.
In addition to diet and meals, nutrient composition of food may also affect thermogenic fat biogenesis and activity. For example, capsaicin is a pungent compound found in hot peppers that can induce hypermetabolism and sweating upon consumption (234). Multiple studies using rodents and humans have shown the beneficial effects of acute and chronic administration of capsaicin on total energy expenditure and fatty acid oxidation (234–238). Furthermore, overweight or obese subjects that ingested red peppers with meals had reduced adiposity, increased fatty acid oxidation, and enhanced whole-body energy expenditure (239). Nevertheless, due to its spiciness, capsaicin is less palatable, preventing ingestion of large quantities, thus, making capsaicin less desirable and to a lesser extent, a convincing beige fat therapy (240). However, capsinoids, analogs of capsaicin but without the pungency, can be as potent as capsaicin in boosting energy expenditure in rodents and humans (241). For example, after two hours of cold exposure (19°C), test subjects that had received capsinoids (9 mg) increased FDG uptake into adipose depots within the supraclavicular and paraspinal regions. Moreover, energy expenditure increased three-fold in response to cold exposure after oral ingestion of capsinoids but not under warm temperature conditions (242). Mechanistically, capsinoids are a class of vanilloid compounds that directly bind to the TRPV1 channel to increase intracellular calcium signaling, thereby increasing thermogenic action, potentially via Ucp1 and mitochondrial biogenesis (98, 243). Notably, it appears that two capsinoid molecules may be needed to activate the channel (244). In addition, mice lacking TRPV1 are completely blocked from capsaicin’s effect on energetics and beige fat formation (245). Like capsinoids, other naturally occurring compounds such as allicin and alliin from garlic and onion and ginger-derived compounds can activate TRPV1, suggesting a broad spectrum of nutritional compounds that could elicit thermogenic responses (246, 247). Thus, while capsinoids provide unique insight into thermogenic activation via non-adrenergic methods, other potential pungent and non-pungent compounds could be exploited as thermogenic ingredients (248). For example, menthol, a compound found in mint, has been shown to stimulate thermogenesis and increase energy expenditure in rodents via TRP channel activation (249). In addition, other food molecules and nutrients, such as retinoic acid, resveratrol, and fish oils, have been shown to stimulate thermogenic responses to improve metabolic fitness (250–252). For example, treating obese mice with slow-release retinoic acid pellets reduced body weight and fat mass, improved glucose clearance, and upregulated a thermogenic program via two nuclear hormone receptors—retinoic acid receptor (RAR) and Pparβ/δ[252]. However, the functional utility of retinoic acid and other natural compounds in humans has yet to be fully determined (252, 253). Additionally, future investigation into relevant mechanisms of nutritional components will highlight the efficacy of these natural compounds in augmenting thermogenic fat formation. Moreover, might these natural ingredients have the potential to avoid age and obesity-induced effects on thermogenic function? Promisingly, the convenience of natural ingredients/compounds could be a strategy for obesity prevention by combining them with dietary foods, such as the marketing success of spicy chocolate.
In the United States, the adult obesity prevalence is 41.9%, but even more staggering is the 19.1% obesity occurrence among children (2-19 yrs.) (254). The defining feature of obesity is excess white fat mass, increasing the risk for metabolic disorders and premature death (255). Thus, identifying potential and tangible therapies is rapidly needed to counteract this public health problem (254). The ability to generate thermogenic fat is a feasible and highly desirable anti-obesity target due to its ability to increase energy expenditure and futilely burn substrates (Figure 1) (4, 125, 255). Unequivocally, the generation of thermogenic fat in rodents and healthy humans suggests metabolic potential (Figure 1) (256–258). Skeptically, it could and has been argued that the amount of thermogenic fat needed to alter whole-body human metabolism is more than what can be generated. Still, the human thermogenic fat field is nascent and has only begun detecting human thermogenic fat with the possibility of new depots being discovered. The development and advancement of imaging and the need for substrate tracer technology are considered critical for defining and characterizing thermogenic fat deposits and metabolic sink potential. Moreover, studies involving obese subjects will be required to understand human thermogenic fat-induced metabolic reprogramming. Additionally, cold exposure may provide its own therapeutic effect, independent of thermogenic fat development, which may boost metabolism, lower adiposity, and reduce inflammation.
Several rodent therapeutic strategies have successfully targeted thermogenic fat; however, these tools may not apply to human adipocytes. For instance, a recent study showed that Adrb2 drives human thermogenic fat biogenesis, not Adrb3 (259). But another study suggests that Adrb3 does mediate human thermogenic fat biogenesis (260). Nevertheless, as observed from Adrb agonists, such as Mirabegron, targeting Adrbs can alter energy homeostasis but may be contraindicative in the larger population. Therefore, the development of targeted therapies, such as gene-based or tissue-specific pharmaceuticals, is ideal, providing precision in thermogenic fat biogenesis and limiting off-target side effects (261). Furthermore, thermogenic adipocytes appear heterogenic and are derived from various cellular sources. Hence, a continued examination of cell ontology, lineage tracing, and genetic profiling will help unearth the complexity and lineage relationships. For example, while single-cell transcriptomics provides resolution on cellular populations and their trajectories, incorporating lineage tracing and spatial-niche reconstruction technology such as RNA-fluorescence in situ hybridization (FISH) will provide information on cellular utility, tissue microenvironment, and adipogenic competency (262, 263). Previous studies have posited that adipose tissues are homogenous; however, identifying brown and beige adipocyte subpopulations provides new insights into how adipocytes are potentially formed, activated, and metabolically induced.
A potential, but unexplored node of nutritional research, is the advancement of dietary thermogenic inducers (248). Critically, mechanistic insights into nutritional thermogenic regulators still need to be improved, which would be necessary to provide tailored thermogenic fat development. For instance, do capsaicinoids stimulate thermogenic progenitors or white-beige interconversion, and what is the metabolic substrate preference of capsaicinoid-induced thermogenic fat? While these questions may appear nuanced, they could direct whether capsaicinoid supplements or other nutrient thermogenic activators will be effective depending on the patient’s metabolic phenotype. Moreover, these new thermogenic regulators could offer a vast pharmacopeia of agents to ignite thermogenesis, hoping to circumvent pesky cold exposure altogether. However, these efforts may all be in vain, especially if thermogenic fat fails with age, beginning in our mid-30s (160). For that matter, increased adiposity also slows the development of thermogenic fat biogenesis. Thus, how can thermogenic fat be renewed in the aging-obese population? Studies targeting the hallmarks of age-dependent thermogenic fat failure will be vital for thermogenic fat renewal. Moreover, aging is a process throughout life, not a terminal age, which may be a critical point for intercepting its decline (264). Nevertheless, there may be hope with the development of senolytics and other pharmaceuticals that have shown promise in restoring thermogenic fat in obese or aged subjects. In conclusion, the advancement in thermogenic fat formation over the past decade has been substantial. Yet, developing new biomedical technologies coupled with basic cellular and molecular biological research will foster new developments into the full potential of thermogenic fat as a possible therapy to thwart obesity and metabolic disease.
SX wrote the draft and revised the review. SX, DL, and DB revised and wrote the final version. All authors contributed to the article and approved the submitted version.
This work is supported by the NIDDK awards R01 DK132264 to DB.
The authors declare that the research was conducted in the absence of any commercial or financial relationships that could be construed as a potential conflict of interest.
All claims expressed in this article are solely those of the authors and do not necessarily represent those of their affiliated organizations, or those of the publisher, the editors and the reviewers. Any product that may be evaluated in this article, or claim that may be made by its manufacturer, is not guaranteed or endorsed by the publisher.
1. Anderson RM, Weindruch R. Metabolic reprogramming, caloric restriction and aging. Trends Endocrinol Metab (2010) 21:134–41. doi: 10.1016/j.tem.2009.11.005
2. Li Q, Spalding KL. The regulation of adipocyte growth in white adipose tissue. Front Cell Dev Biol (2022) 10:1003219. doi: 10.3389/fcell.2022.1003219
3. Vishvanath L, Gupta RK. Contribution of adipogenesis to healthy adipose tissue expansion in obesity. J Clin Invest (2019) 129:4022–31. doi: 10.1172/JCI129191
4. Harms M, Seale P. Brown and beige fat: development, function and therapeutic potential. Nat Med (2013) 19:1252–63. doi: 10.1038/nm.3361
5. Bartness TJ, Shrestha YB, Vaughan CH, Schwartz GJ, Song CK. Sensory and sympathetic nervous system control of white adipose tissue lipolysis. Mol Cell Endocrinol (2010) 318:34–43. doi: 10.1016/j.mce.2009.08.031
6. Bartness TJ, Vaughan CH, Song CK. Sympathetic and sensory innervation of brown adipose tissue. Int J Obes (Lond) (2010) 34 Suppl 1:S36–42. doi: 10.1038/ijo.2010.182
7. Schonbaum E, Johnson GE, Sellers EA, Gill MJ. Adrenergic beta-receptors and non-shivering thermogenesis. Nature (1966) 210:426. doi: 10.1038/210426a0
8. Spiegelman BM, Flier JS. Obesity and the regulation of energy balance. Cell (2001) 104:531–43. doi: 10.1016/S0092-8674(01)00240-9
9. Rosen ED, Spiegelman BM. What we talk about when we talk about fat. Cell (2014) 156:20–44. doi: 10.1016/j.cell.2013.12.012
10. Crewe C, An YA, Scherer PE. The ominous triad of adipose tissue dysfunction: inflammation, fibrosis, and impaired angiogenesis. J Clin Invest (2017) 127:74–82. doi: 10.1172/JCI88883
11. Zoico E, Rubele S, De Caro A, Nori N, Mazzali G, Fantin F, et al. Brown and beige adipose tissue and aging. Front Endocrinol (Lausanne) (2019) 10:368. doi: 10.3389/fendo.2019.00368
12. Cousin B, Cinti S, Morroni M, Raimbault S, Ricquier D, Penicaud L, et al. Occurrence of brown adipocytes in rat white adipose tissue: molecular and morphological characterization. J Cell Sci (1992) 103(Pt 4):931–42. doi: 10.1242/jcs.103.4.931
13. Smorlesi A, Frontini A, Giordano A, Cinti S. The adipose organ: white-brown adipocyte plasticity and metabolic inflammation. Obes Rev (2012) 13 Suppl 2:83–96. doi: 10.1111/j.1467-789X.2012.01039.x
14. Young P, Arch JR, Ashwell M. Brown adipose tissue in the parametrial fat pad of the mouse. FEBS Lett (1984) 167:10–4. doi: 10.1016/0014-5793(84)80822-4
15. Sakers A, De Siqueira MK, Seale P, Villanueva CJ. Adipose-tissue plasticity in health and disease. Cell (2022) 185:419–46. doi: 10.1016/j.cell.2021.12.016
16. Smith RE, Horwitz BA. Brown fat and thermogenesis. Physiol Rev (1969) 49:330–425. doi: 10.1152/physrev.1969.49.2.330
17. FELIX K, EGER. W. Die bildung von fett aus kohlehydrat in dem fettorganen. Deut. Arch KZin. Med (1938) 182:623–34.
18. FLEISCHMANN W. Beitrage zur physiologie der gewebsatmung nach untersuchungen an winterschlffern. Arch Ges. Physiol (1929) 222:541–7.
19. Hook WE, Barron ESG. The respiration of brown adipose tissue and kidney of the hibernating and non-hibernating ground squirrel. Am J Physiol (1941) 133:56–63. doi: 10.1152/ajplegacy.1941.133.1.56
20. Hausberger FX, Widelitz MM. Distribution of labeled erythrocytes in adipose tissue and muscle in the rat. Am J Physiol (1963) 204:649–52. doi: 10.1152/ajplegacy.1963.204.4.649
21. Napolitano LM. Observations on the fine structure of adipose cells. Ann N Y Acad Sci (1965) 131:34–42. doi: 10.1111/j.1749-6632.1965.tb34777.x
22. Lever JD. The fine structure of brown adipose tissue in the rat with observations on the cytological changes following starvation and adrenalectomy. Anat Rec (1957) 128:361–77. doi: 10.1002/ar.1091280302
23. Barneda D, Planas-Iglesias J, Gaspar ML, Mohammadyani D, Prasannan S, Dormann D, et al. The brown adipocyte protein CIDEA promotes lipid droplet fusion via a phosphatidic acid-binding amphipathic helix. Elife (2015) 4:e07485. doi: 10.7554/eLife.07485.020
24. Puri V, Ranjit S, Konda S, Nicoloro SM, Straubhaar J, Chawla A, et al. Cidea is associated with lipid droplets and insulin sensitivity in humans. Proc Natl Acad Sci U.S.A. (2008) 105:7833–8. doi: 10.1073/pnas.0802063105
25. Hintsch G, Zurlinden A, Meskenaite V, Steuble M, Fink-Widmer K, Kinter J, et al. The calsyntenins–a family of postsynaptic membrane proteins with distinct neuronal expression patterns. Mol Cell Neurosci (2002) 21:393–409. doi: 10.1006/mcne.2002.1181
26. Qian K, Tol MJ, Wu J, Uchiyama LF, Xiao X, Cui L, et al. CLSTN3beta enforces adipocyte multilocularity to facilitate lipid utilization. Nature (2023) 613:160–8. doi: 10.1038/s41586-022-05507-1
27. Puigserver P, Wu Z, Park CW, Graves R, Wright M, Spiegelman BM. A cold-inducible coactivator of nuclear receptors linked to adaptive thermogenesis. Cell (1998) 92:829–39. doi: 10.1016/S0092-8674(00)81410-5
28. Puigserver P, Spiegelman BM. Peroxisome proliferator-activated receptor-gamma coactivator 1 alpha (PGC-1 alpha): transcriptional coactivator and metabolic regulator. Endocr Rev (2003) 24:78–90. doi: 10.1210/er.2002-0012
29. Rothwell NJ, Stock MJ. Effects of denervating brown adipose tissue on the responses to cold, hyperphagia and noradrenaline treatment in the rat. J Physiol (1984) 355:457–63. doi: 10.1113/jphysiol.1984.sp015431
30. Youngstrom TG, Bartness TJ. White adipose tissue sympathetic nervous system denervation increases fat pad mass and fat cell number. Am J Physiol (1998) 275:R1488–93. doi: 10.1152/ajpregu.1998.275.5.R1488
31. Chen KY, Brychta RJ, Linderman JD, Smith S, Courville A, Dieckmann W, et al. Brown fat activation mediates cold-induced thermogenesis in adult humans in response to a mild decrease in ambient temperature. J Clin Endocrinol Metab (2013) 98:E1218–23. doi: 10.1210/jc.2012-4213
32. Nakamura K, Morrison SF. Central efferent pathways mediating skin cooling-evoked sympathetic thermogenesis in brown adipose tissue. Am J Physiol Regul Integr Comp Physiol (2007) 292:R127–36. doi: 10.1152/ajpregu.00427.2006
33. Tajino K, Hosokawa H, Maegawa S, Matsumura K, Dhaka A, Kobayashi S. Cooling-sensitive TRPM8 is thermostat of skin temperature against cooling. PloS One (2011) 6:e17504. doi: 10.1371/journal.pone.0017504
34. Emorine LJ, Marullo S, Briend-Sutren MM, Patey G, Tate K, Delavier-Klutchko C, et al. Molecular characterization of the human beta 3-adrenergic receptor. Science (1989) 245:1118–21. doi: 10.1126/science.2570461
35. Collins S. Beta-adrenergic receptors and adipose tissue metabolism: Evolution of an old story. Annu Rev Physiol (2022) 84:1–16. doi: 10.1146/annurev-physiol-060721-092939
36. Guilherme A, Yenilmez B, Bedard AH, Henriques F, Liu D, Lee A, et al. Control of adipocyte thermogenesis and lipogenesis through beta3-adrenergic and thyroid hormone signal integration. Cell Rep (2020) 31:107598. doi: 10.1016/j.celrep.2020.107598
37. Robidoux J, Cao W, Quan H, Daniel KW, Moukdar F, Bai X, et al. Selective activation of mitogen-activated protein (MAP) kinase kinase 3 and p38alpha MAP kinase is essential for cyclic AMP-dependent UCP1 expression in adipocytes. Mol Cell Biol (2005) 25:5466–79. doi: 10.1128/MCB.25.13.5466-5479.2005
38. Collins S. Beta-adrenoceptor signaling networks in adipocytes for recruiting stored fat and energy expenditure. Front Endocrinol (Lausanne) (2011) 2:102. doi: 10.3389/fendo.2011.00102
39. Labbe SM, Caron A, Lanfray D, Monge-Rofarello B, Bartness TJ, Richard D. Hypothalamic control of brown adipose tissue thermogenesis. Front Syst Neurosci (2015) 9:150. doi: 10.3389/fnsys.2015.00150
40. Silva JE, Larsen PR. Adrenergic activation of triiodothyronine production in brown adipose tissue. Nature (1983) 305:712–3. doi: 10.1038/305712a0
41. Takahashi A, Shimazu T, Maruyama Y. Importance of sympathetic nerves for the stimulatory effect of cold exposure on glucose utilization in brown adipose tissue. Jpn J Physiol (1992) 42:653–64. doi: 10.2170/jjphysiol.42.653
42. Garretson JT, Szymanski LA, Schwartz GJ, Xue B, Ryu V, Bartness TJ. Lipolysis sensation by white fat afferent nerves triggers brown fat thermogenesis. Mol Metab (2016) 5:626–34. doi: 10.1016/j.molmet.2016.06.013
43. Nguyen NLT, Xue B, Bartness TJ. Sensory denervation of inguinal white fat modifies sympathetic outflow to white and brown fat in Siberian hamsters. Physiol Behav (2018) 190:28–33. doi: 10.1016/j.physbeh.2018.02.019
44. Schulz TJ, Tseng YH. Brown adipose tissue: development, metabolism and beyond. Biochem J (2013) 453:167–78. doi: 10.1042/BJ20130457
45. Jun H, Ma Y, Chen Y, Gong J, Liu S, Wang J, et al. Adrenergic-independent signaling via CHRNA2 regulates beige fat activation. Dev Cell (2020) 54:106–116 e5. doi: 10.1016/j.devcel.2020.05.017
46. Jun H, Yu H, Gong J, Jiang J, Qiao X, Perkey E, et al. An immune-beige adipocyte communication via nicotinic acetylcholine receptor signaling. Nat Med (2018) 24:814–22. doi: 10.1038/s41591-018-0032-8
47. Nedergaard J, Golozoubova V, Matthias A, Asadi A, Jacobsson A, Cannon B. UCP1: the only protein able to mediate adaptive non-shivering thermogenesis and metabolic inefficiency. Biochim Biophys Acta (2001) 1504:82–106. doi: 10.1016/S0005-2728(00)00247-4
48. Fedorenko A, Lishko PV, Kirichok Y. Mechanism of fatty-acid-dependent UCP1 uncoupling in brown fat mitochondria. Cell (2012) 151:400–13. doi: 10.1016/j.cell.2012.09.010
49. Nedergaard J, Cannon B. UCP1 mRNA does not produce heat. Biochim Biophys Acta (2013) 1831:943–9. doi: 10.1016/j.bbalip.2013.01.009
50. Nedergaard J, Cannon B. The browning of white adipose tissue: some burning issues. Cell Metab (2014) 20:396–407. doi: 10.1016/j.cmet.2014.07.005
51. Enerback S, Jacobsson A, Simpson EM, Guerra C, Yamashita H, Harper ME, et al. Mice lacking mitochondrial uncoupling protein are cold-sensitive but not obese. Nature (1997) 387:90–4. doi: 10.1038/387090a0
52. Kopecky J, Clarke G, Enerback S, Spiegelman B, Kozak LP. Expression of the mitochondrial uncoupling protein gene from the aP2 gene promoter prevents genetic obesity. J Clin Invest (1995) 96:2914–23. doi: 10.1172/JCI118363
53. Chouchani ET, Kajimura S. Metabolic adaptation and maladaptation in adipose tissue. Nat Metab (2019) 1:189–200. doi: 10.1038/s42255-018-0021-8
54. Cohen P, Kajimura S. The cellular and functional complexity of thermogenic fat. Nat Rev Mol Cell Biol (2021) 22:393–409. doi: 10.1038/s41580-021-00350-0
55. Song A, Dai W, Jang MJ, Medrano L, Li Z, Zhao H, et al. Low- and high-thermogenic brown adipocyte subpopulations coexist in murine adipose tissue. J Clin Invest (2020) 130:247–57. doi: 10.1172/JCI129167
56. Chouchani ET, Kazak L, Spiegelman BM. New advances in adaptive thermogenesis: UCP1 and beyond. Cell Metab (2019) 29:27–37. doi: 10.1016/j.cmet.2018.11.002
57. Nicholls DG, Brand MD. A critical assessment of the role of creatine in brown adipose tissue thermogenesis. Nat Metab (2023) 5(1):21–8. doi: 10.1038/s42255-022-00718-2
58. Berry DC, Stenesen D, Zeve D, Graff JM. The developmental origins of adipose tissue. Development (2013) 140:3939–49. doi: 10.1242/dev.080549
59. Giralt M, Martin I, Iglesias R, Vinas O, Villarroya F, Mampel T. Ontogeny and perinatal modulation of gene expression in rat brown adipose tissue. unaltered iodothyronine 5'-deiodinase activity is necessary for the response to environmental temperature at birth. Eur J Biochem (1990) 193:297–302. doi: 10.1111/j.1432-1033.1990.tb19336.x
60. Houstek J, Kopecky J, Rychter Z, Soukup T. Uncoupling protein in embryonic brown adipose tissue–existence of nonthermogenic and thermogenic mitochondria. Biochim Biophys Acta (1988) 935:19–25. doi: 10.1016/0005-2728(88)90103-X
61. Wang QA, Tao C, Gupta RK, Scherer PE. Tracking adipogenesis during white adipose tissue development, expansion and regeneration. Nat Med (2013) 19:1338–44. doi: 10.1038/nm.3324
62. Wang W, Seale P. Control of brown and beige fat development. Nat Rev Mol Cell Biol (2016) 17:691–702. doi: 10.1038/nrm.2016.96
63. Wang W, Kissig M, Rajakumari S, Huang L, Lim HW, Won KJ, et al. Ebf2 is a selective marker of brown and beige adipogenic precursor cells. Proc Natl Acad Sci U.S.A. (2014) 111:14466–71. doi: 10.1073/pnas.1412685111
64. Logan C, Hanks MC, Noble-Topham S, Nallainathan D, Provart NJ, Joyner AL. Cloning and sequence comparison of the mouse, human, and chicken engrailed genes reveal potential functional domains and regulatory regions. Dev Genet (1992) 13:345–58. doi: 10.1002/dvg.1020130505
65. Loomis CA, Harris E, Michaud J, Wurst W, Hanks M, Joyner AL. The mouse engrailed-1 gene and ventral limb patterning. Nature (1996) 382:360–3. doi: 10.1038/382360a0
66. Atit R, Sgaier SK, Mohamed OA, Taketo MM, Dufort D, Joyner AL, et al. Beta-catenin activation is necessary and sufficient to specify the dorsal dermal fate in the mouse. Dev Biol (2006) 296:164–76. doi: 10.1016/j.ydbio.2006.04.449
67. Seale P, Bjork B, Yang W, Kajimura S, Chin S, Kuang S, et al. PRDM16 controls a brown fat/skeletal muscle switch. Nature (2008) 454:961–7. doi: 10.1038/nature07182
68. Sanchez-Gurmaches J, Guertin DA. Adipocytes arise from multiple lineages that are heterogeneously and dynamically distributed. Nat Commun (2014) 5:4099. doi: 10.1038/ncomms5099
69. Sanchez-Gurmaches J, Hung CM, Sparks CA, Tang Y, Li H, Guertin DA. PTEN loss in the Myf5 lineage redistributes body fat and reveals subsets of white adipocytes that arise from Myf5 precursors. Cell Metab (2012) 16:348–62. doi: 10.1016/j.cmet.2012.08.003
70. Relaix F, Montarras D, Zaffran S, Gayraud-Morel B, Rocancourt D, Tajbakhsh S, et al. Pax3 and Pax7 have distinct and overlapping functions in adult muscle progenitor cells. J Cell Biol (2006) 172:91–102. doi: 10.1083/jcb.200508044
71. Lepper C, Fan CM. Inducible lineage tracing of Pax7-descendant cells reveals embryonic origin of adult satellite cells. Genesis (2010) 48:424–36. doi: 10.1002/dvg.20630
72. Lang D, Lu MM, Huang L, Engleka KA, Zhang M, Chu EY, et al. Pax3 functions at a nodal point in melanocyte stem cell differentiation. Nature (2005) 433:884–7. doi: 10.1038/nature03292
73. Liu W, Liu Y, Lai X, Kuang S. Intramuscular adipose is derived from a non-Pax3 lineage and required for efficient regeneration of skeletal muscles. Dev Biol (2012) 361:27–38. doi: 10.1016/j.ydbio.2011.10.011
74. Timmons JA, Wennmalm K, Larsson O, Walden TB, Lassmann T, Petrovic N, et al. Myogenic gene expression signature establishes that brown and white adipocytes originate from distinct cell lineages. Proc Natl Acad Sci U.S.A. (2007) 104:4401–6. doi: 10.1073/pnas.0610615104
75. Sebo ZL, Jeffery E, Holtrup B, Rodeheffer MS. A mesodermal fate map for adipose tissue. Development (2018) 145. doi: 10.1242/dev.166801
76. Madisen L, Zwingman TA, Sunkin SM, Oh SW, Zariwala HA, Gu H, et al. A robust and high-throughput cre reporting and characterization system for the whole mouse brain. Nat Neurosci (2010) 13:133–40. doi: 10.1038/nn.2467
77. Magnuson MA, Osipovich AB. Pancreas-specific cre driver lines and considerations for their prudent use. Cell Metab (2013) 18:9–20. doi: 10.1016/j.cmet.2013.06.011
78. Steiner BM, Berry DC. The regulation of adipose tissue health by estrogens. Front Endocrinol (Lausanne) (2022) 13:889923. doi: 10.3389/fendo.2022.889923
79. Tallquist MD, Weismann KE, Hellstrom M, Soriano P. Early myotome specification regulates PDGFA expression and axial skeleton development. Development (2000) 127:5059–70. doi: 10.1242/dev.127.23.5059
80. Harms MJ, Ishibashi J, Wang W, Lim HW, Goyama S, Sato T, et al. Prdm16 is required for the maintenance of brown adipocyte identity and function in adult mice. Cell Metab (2014) 19:593–604. doi: 10.1016/j.cmet.2014.03.007
81. Wang Q, Li H, Tajima K, Verkerke ARP, Taxin ZH, Hou Z, et al. Post-translational control of beige fat biogenesis by PRDM16 stabilization. Nature (2022) 609:151–8. doi: 10.1038/s41586-022-05067-4
82. Shapira SN, Lim HW, Rajakumari S, Sakers AP, Ishibashi J, Harms MJ, et al. EBF2 transcriptionally regulates brown adipogenesis via the histone reader DPF3 and the BAF chromatin remodeling complex. Genes Dev (2017) 31:660–73. doi: 10.1101/gad.294405.116
83. Tontonoz P, Hu E, Spiegelman BM. Stimulation of adipogenesis in fibroblasts by PPAR gamma 2, a lipid-activated transcription factor. Cell (1994) 79:1147–56. doi: 10.1016/0092-8674(94)90006-X
84. Chawla A, Schwarz EJ, Dimaculangan DD, Lazar MA. Peroxisome proliferator-activated receptor (PPAR) gamma: adipose-predominant expression and induction early in adipocyte differentiation. Endocrinology (1994) 135:798–800. doi: 10.1210/endo.135.2.8033830
85. Jiang Y, Berry DC, Tang W, Graff JM. Independent stem cell lineages regulate adipose organogenesis and adipose homeostasis. Cell Rep (2014) 9:1007–22. doi: 10.1016/j.celrep.2014.09.049
86. Imai T, Takakuwa R, Marchand S, Dentz E, Bornert JM, Messaddeq N, et al. Peroxisome proliferator-activated receptor gamma is required in mature white and brown adipocytes for their survival in the mouse. Proc Natl Acad Sci U.S.A. (2004) 101:4543–7. doi: 10.1073/pnas.0400356101
87. Dempersmier J, Sambeat A, Gulyaeva O, Paul SM, Hudak CS, Raposo HF, et al. Cold-inducible Zfp516 activates UCP1 transcription to promote browning of white fat and development of brown fat. Mol Cell (2015) 57:235–46. doi: 10.1016/j.molcel.2014.12.005
88. Park JH, Kang HJ, Kang SI, Lee JE, Hur J, Ge K, et al. A multifunctional protein, EWS, is essential for early brown fat lineage determination. Dev Cell (2013) 26:393–404. doi: 10.1016/j.devcel.2013.07.002
89. Kajimura S, Seale P, Kubota K, Lunsford E, Frangioni JV, Gygi SP, et al. Initiation of myoblast to brown fat switch by a PRDM16-C/EBP-beta transcriptional complex. Nature (2009) 460:1154–8. doi: 10.1038/nature08262
90. Schulz TJ, Huang P, Huang TL, Xue R, McDougall LE, Townsend KL, et al. Brown-fat paucity due to impaired BMP signalling induces compensatory browning of white fat. Nature (2013) 495:379–83. doi: 10.1038/nature11943
91. Fischer AW, Cannon B, Nedergaard J. Optimal housing temperatures for mice to mimic the thermal environment of humans: An experimental study. Mol Metab (2018) 7:161–70. doi: 10.1016/j.molmet.2017.10.009
92. Lee YH, Petkova AP, Konkar AA, Granneman JG. Cellular origins of cold-induced brown adipocytes in adult mice. FASEB J (2015) 29:286–99. doi: 10.1096/fj.14-263038
93. Tallquist M, Kazlauskas A. PDGF signaling in cells and mice. Cytokine Growth Factor Rev (2004) 15:205–13. doi: 10.1016/j.cytogfr.2004.03.003
94. Tallquist MD, French WJ, Soriano P. Additive effects of PDGF receptor beta signaling pathways in vascular smooth muscle cell development. PloS Biol (2003) 1:E52. doi: 10.1371/journal.pbio.0000052
95. Shin S, Pang Y, Park J, Liu L, Lukas BE, Kim SH, et al. Dynamic control of adipose tissue development and adult tissue homeostasis by platelet-derived growth factor receptor alpha. Elife (2020) 9. doi: 10.7554/eLife.56189
96. Jiang Y, Berry DC, Graff JM. Distinct cellular and molecular mechanisms for beta3 adrenergic receptor-induced beige adipocyte formation. Elife (2017) 6. doi: 10.7554/eLife.30329
97. Shamsi F, Piper M, Ho LL, Huang TL, Gupta A, Streets A, et al. Vascular smooth muscle-derived Trpv1(+) progenitors are a source of cold-induced thermogenic adipocytes. Nat Metab (2021) 3:485–95. doi: 10.1038/s42255-021-00373-z
98. Sun W, Luo Y, Zhang F, Tang S, Zhu T. Involvement of TRP channels in adipocyte thermogenesis: An update. Front Cell Dev Biol (2021) 9:686173. doi: 10.3389/fcell.2021.686173
99. Barbatelli G, Murano I, Madsen L, Hao Q, Jimenez M, Kristiansen K, et al. The emergence of cold-induced brown adipocytes in mouse white fat depots is determined predominantly by white to brown adipocyte transdifferentiation. Am J Physiol Endocrinol Metab (2010) 298:E1244–53. doi: 10.1152/ajpendo.00600.2009
100. Rosenwald M, Perdikari A, Rulicke T, Wolfrum C. Bi-directional interconversion of brite and white adipocytes. Nat Cell Biol (2013) 15:659–67. doi: 10.1038/ncb2740
101. Roh HC, Tsai LTY, Shao M, Tenen D, Shen Y, Kumari M, et al. Warming induces significant reprogramming of beige, but not brown, adipocyte cellular identity. Cell Metab (2018) 27:1121–1137 e5. doi: 10.1016/j.cmet.2018.03.005
102. Park J, Shin S, Liu L, Jahan I, Ong SG, Xu P, et al. Progenitor-like characteristics in a subgroup of UCP1+ cells within white adipose tissue. Dev Cell (2021) 56(7):985–99.e4. doi: 10.1016/j.devcel.2021.02.018
103. Morrison SJ, Spradling AC. Stem cells and niches: mechanisms that promote stem cell maintenance throughout life. Cell (2008) 132:598–611. doi: 10.1016/j.cell.2008.01.038
104. Shao M, Wang QA, Song A, Vishvanath L, Busbuso NC, Scherer PE, et al. Cellular origins of beige fat cells revisited. Diabetes (2019) 68:1874–85. doi: 10.2337/db19-0308
105. Lee YH, Petkova AP, Mottillo EP, Granneman JG. In vivo identification of bipotential adipocyte progenitors recruited by beta3-adrenoceptor activation and high-fat feeding. Cell Metab (2012) 15:480–91. doi: 10.1016/j.cmet.2012.03.009
106. Berry DC, Jiang Y, Graff JM. Mouse strains to study cold-inducible beige progenitors and beige adipocyte formation and function. Nat Commun (2016) 7:10184. doi: 10.1038/ncomms10184
107. Sun C, Sakashita H, Kim J, Tang Z, Upchurch GM, Yao L, et al. Mosaic mutant analysis identifies PDGFRalpha/PDGFRbeta as negative regulators of adipogenesis. Cell Stem Cell (2020) 26:707–721 e5. doi: 10.1016/j.stem.2020.03.004
108. Vishvanath L, MacPherson KA, Hepler C, Wang QA, Shao M, Spurgin SB, et al. Pdgfrbeta+ mural preadipocytes contribute to adipocyte hyperplasia induced by high-Fat-Diet feeding and prolonged cold exposure in adult mice. Cell Metab (2016) 23:350–9. doi: 10.1016/j.cmet.2015.10.018
109. Long JZ, Svensson KJ, Tsai L, Zeng X, Roh HC, Kong X, et al. A smooth muscle-like origin for beige adipocytes. Cell Metab (2014) 19(5):810–20. doi: 10.1016/j.cmet.2014.03.025
110. Oguri Y, Shinoda K, Kim H, Alba DL, Bolus WR, Wang Q, et al. CD81 controls beige fat progenitor cell growth and energy balance via FAK signaling. Cell (2020) 182:563–577 e20. doi: 10.1016/j.cell.2020.06.021
111. Chen Y, Ikeda K, Yoneshiro T, Scaramozza A, Tajima K, Wang Q, et al. Thermal stress induces glycolytic beige fat formation via a myogenic state. Nature (2019) 565:180–5. doi: 10.1038/s41586-018-0801-z
112. Collins S, Daniel KW, Rohlfs EM, Ramkumar V, Taylor IL, Gettys TW. Impaired expression and functional activity of the beta 3- and beta 1-adrenergic receptors in adipose tissue of congenitally obese (C57BL/6J ob/ob) mice. Mol Endocrinol (1994) 8:518–27. doi: 10.1210/mend.8.4.7914350
113. Himms-Hagen J, Melnyk A, Zingaretti MC, Ceresi E, Barbatelli G, Cinti S. Multilocular fat cells in WAT of CL-316243-treated rats derive directly from white adipocytes. Am J Physiol Cell Physiol (2000) 279(3):C670–81. doi: 10.1152/ajpcell.2000.279.3.C670
114. de Jong JMA, Wouters RTF, Boulet N, Cannon B, Nedergaard J, Petrovic N. The beta3-adrenergic receptor is dispensable for browning of adipose tissues. Am J Physiol Endocrinol Metab (2017) 312:E508–18. doi: 10.1152/ajpendo.00437.2016
115. Bachman ES, Dhillon H, Zhang CY, Cinti S, Bianco AC, Kobilka BK, et al. betaAR signaling required for diet-induced thermogenesis and obesity resistance. Science (2002) 297:843–5. doi: 10.1126/science.1073160
116. Bronnikov G, Houstek J, Nedergaard J. Beta-adrenergic, cAMP-mediated stimulation of proliferation of brown fat cells in primary culture. Mediation via beta 1 but not via beta 3 adrenoceptors J Biol Chem (1992) 267:2006–13.
117. Ueta CB, Fernandes GW, Capelo LP, Fonseca TL, Maculan FD, Gouveia CH, et al. beta(1) adrenergic receptor is key to cold- and diet-induced thermogenesis in mice. J Endocrinol (2012) 214:359–65. doi: 10.1530/JOE-12-0155
118. Soloveva V, Graves RA, Rasenick MM, Spiegelman BM, Ross SR. Transgenic mice overexpressing the beta 1-adrenergic receptor in adipose tissue are resistant to obesity. Mol Endocrinol (1997) 11:27–38. doi: 10.1210/mend.11.1.9870
119. Lee S, Benvie AM, Park HG, Spektor R, Harlan B, Brenna JT, et al. Remodeling of gene regulatory networks underlying thermogenic stimuli-induced adipose beiging. Commun Biol (2022) 5:584. doi: 10.1038/s42003-022-03531-5
120. Lee YH, Kim SN, Kwon HJ, Granneman JG. Metabolic heterogeneity of activated beige/brite adipocytes in inguinal adipose tissue. Sci Rep (2017) 7:39794. doi: 10.1038/srep39794
121. Lynes MI, Leiria L, Lundh M, Bartelt A, Shamsi F, Huang TL, et al. The cold-induced lipokine 12,13-diHOME promotes fatty acid transport into brown adipose tissue. Nat Med (2017) 23:1384–4:pg 631. doi: 10.1038/nm.4297
122. Yoneshiro T, Wang Q, Tajima K, Matsushita M, Maki H, Igarashi K, et al. BCAA catabolism in brown fat controls energy homeostasis through SLC25A44. Nature (2019) 572:614–9. doi: 10.1038/s41586-019-1503-x
123. Gesta S, Tseng YH, Kahn CR. Developmental origin of fat: tracking obesity to its source. Cell (2007) 131:242–56. doi: 10.1016/j.cell.2007.10.004
124. Merklin RJ. Growth and distribution of human fetal brown fat. Anat Rec (1974) 178:637–45. doi: 10.1002/ar.1091780311
125. Cannon B, Nedergaard J. Brown adipose tissue: function and physiological significance. Physiol Rev (2004) 84:277–359. doi: 10.1152/physrev.00015.2003
126. Huttunen P, Hirvonen J, Kinnula V. The occurrence of brown adipose tissue in outdoor workers. Eur J Appl Physiol Occup Physiol (1981) 46:339–45. doi: 10.1007/BF00422121
128. Cypess AM, Lehman S, Williams G, Tal I, Rodman D, Goldfine AB, et al. Identification and importance of brown adipose tissue in adult humans. N Engl J Med (2009) 360:1509–17. doi: 10.1056/NEJMoa0810780
129. Saito M, Okamatsu-Ogura Y, Matsushita M, Watanabe K, Yoneshiro T, Nio-Kobayashi J, et al. High incidence of metabolically active brown adipose tissue in healthy adult humans: effects of cold exposure and adiposity. Diabetes (2009) 58:1526–31. doi: 10.2337/db09-0530
130. van Marken Lichtenbelt WD, Vanhommerig JW, Smulders NM, Drossaerts JM, Kemerink GJ, Bouvy ND, et al. Cold-activated brown adipose tissue in healthy men. N Engl J Med (2009) 360:1500–8. doi: 10.1056/NEJMoa0808718
131. Hany TF, Gharehpapagh E, Kamel EM, Buck A, Himms-Hagen J, von Schulthess GK. Brown adipose tissue: a factor to consider in symmetrical tracer uptake in the neck and upper chest region. Eur J Nucl Med Mol Imaging (2002) 29:1393–8. doi: 10.1007/s00259-002-0902-6
132. Christensen CR, Clark PB, Morton KA. Reversal of hypermetabolic brown adipose tissue in f-18 FDG PET imaging. Clin Nucl Med (2006) 31:193–6. doi: 10.1097/01.rlu.0000204199.33136.05
133. Williams G, Kolodny GM. Method for decreasing uptake of 18F-FDG by hypermetabolic brown adipose tissue on PET. AJR Am J Roentgenol (2008) 190:1406–9. doi: 10.2214/AJR.07.3205
134. Zhang F, Hao G, Shao M, Nham K, An Y, Wang Q, et al. An adipose tissue atlas: An image-guided identification of human-like BAT and beige depots in rodents. Cell Metab (2018) 27:252–262 e3. doi: 10.1016/j.cmet.2017.12.004
135. Kajimura S, Saito M. A new era in brown adipose tissue biology: molecular control of brown fat development and energy homeostasis. Annu Rev Physiol (2014) 76:225–49. doi: 10.1146/annurev-physiol-021113-170252
136. Chondronikola M, Volpi E, Borsheim E, Porter C, Annamalai P, Enerback S, et al. Brown adipose tissue improves whole body glucose homeostasis and insulin sensitivity in humans. Diabetes (2014) 63(12):4089–99. doi: 10.2337/db14-0746
137. Becher T, Palanisamy S, Kramer DJ, Eljalby M, Marx SJ, Wibmer AG, et al. Brown adipose tissue is associated with cardiometabolic health. Nat Med (2021) 27:58–65. doi: 10.1038/s41591-020-1126-7
138. Wibmer AG, Becher T, Eljalby M, Crane A, Andrieu PC, Jiang CS, et al. Brown adipose tissue is associated with healthier body fat distribution and metabolic benefits independent of regional adiposity. Cell Rep Med (2021) 2:100332. doi: 10.1016/j.xcrm.2021.100332
139. Chondronikola M, Volpi E, Borsheim E, Porter C, Saraf MK, Annamalai P, et al. Brown adipose tissue activation is linked to distinct systemic effects on lipid metabolism in humans. Cell Metab (2016) 23:1200–6. doi: 10.1016/j.cmet.2016.04.029
140. Ouellet V, Labbe SM, Blondin DP, Phoenix S, Guerin B, Haman F, et al. Brown adipose tissue oxidative metabolism contributes to energy expenditure during acute cold exposure in humans. J Clin Invest (2012) 122:545–52. doi: 10.1172/JCI60433
141. Olsen JM, Csikasz RI, Dehvari N, Lu L, Sandstrom A, Oberg AI, et al. beta(3)-adrenergically induced glucose uptake in brown adipose tissue is independent of UCP1 presence or activity: Mediation through the mTOR pathway. Mol Metab (2017) 6:611–9. doi: 10.1016/j.molmet.2017.02.006
142. Fraum TJ, Crandall JP, Ludwig DR, Chen S, Fowler KJ, Laforest RA, et al. Repeatability of quantitative brown adipose tissue imaging metrics on positron emission tomography with (18)F-fluorodeoxyglucose in humans. Cell Metab (2019) 30:212–224 e4. doi: 10.1016/j.cmet.2019.05.019
143. Pfannenberg C, Werner MK, Ripkens S, Stef I, Deckert A, Schmadl M, et al. Impact of age on the relationships of brown adipose tissue with sex and adiposity in humans. Diabetes (2010) 59:1789–93. doi: 10.2337/db10-0004
144. Leitner BP, Huang S, Brychta RJ, Duckworth CJ, Baskin AS, McGehee S, et al. Mapping of human brown adipose tissue in lean and obese young men. Proc Natl Acad Sci U.S.A. (2017) 114:8649–54. doi: 10.1073/pnas.1705287114
145. Sun W, Dong H, Balaz M, Slyper M, Drokhlyansky E, Colleluori G, et al. snRNA-seq reveals a subpopulation of adipocytes that regulates thermogenesis. Nature (2020) 587:98–102. doi: 10.1038/s41586-020-2856-x
146. Ouellet V, Routhier-Labadie A, Bellemare W, Lakhal-Chaieb L, Turcotte E, Carpentier AC, et al. Age, sex, body mass index, and diabetic status determine the prevalence, mass, and glucose-uptake activity of 18F-FDG-detected BAT in humans. J Clin Endocrinol Metab (2011) 96:192–9. doi: 10.1210/jc.2010-0989
147. Lee P, Greenfield JR, Ho KK, Fulham MJ. A critical appraisal of the prevalence and metabolic significance of brown adipose tissue in adult humans. Am J Physiol Endocrinol Metab (2010) 299:E601–6. doi: 10.1152/ajpendo.00298.2010
148. Okamatsu-Ogura Y, Fukano K, Tsubota A, Uozumi A, Terao A, Kimura K, et al. Thermogenic ability of uncoupling protein 1 in beige adipocytes in mice. PloS One (2013) 8:e84229. doi: 10.1371/journal.pone.0084229
149. Lidell ME, Betz MJ, Dahlqvist Leinhard O, Heglind M, Elander L, Slawik M, et al. Evidence for two types of brown adipose tissue in humans. Nat Med (2013) 19:631–4. doi: 10.1038/nm.3017
150. Wu J, Bostrom P, Sparks LM, Ye L, Choi JH, Giang AH, et al. Beige adipocytes are a distinct type of thermogenic fat cell in mouse and human. Cell (2012) 150:366–76. doi: 10.1016/j.cell.2012.05.016
151. Shinoda K, Luijten IH, Hasegawa Y, Hong H, Sonne SB, Kim M, et al. Genetic and functional characterization of clonally derived adult human brown adipocytes. Nat Med (2015) 21:389–94. doi: 10.1038/nm.3819
152. Xue R, Lynes MD, Dreyfuss JM, Shamsi F, Schulz TJ, Zhang H, et al. Clonal analyses and gene profiling identify genetic biomarkers of the thermogenic potential of human brown and white preadipocytes. Nat Med (2015) 21:760–8. doi: 10.1038/nm.3881
153. Erikson H, Krog J, Andersen KL, Scholander PF. The critical temperature in naked man. Acta Physiol Scand (1956) 37:35–9. doi: 10.1111/j.1748-1716.1956.tb01339.x
154. de Jong JMA, Sun W, Pires ND, Frontini A, Balaz M, Jespersen NZ, et al. Human brown adipose tissue is phenocopied by classical brown adipose tissue in physiologically humanized mice. Nat Metab (2019) 1:830–43. doi: 10.1038/s42255-019-0101-4
155. Andersson KE, Martin N, Nitti V. Selective beta(3)-adrenoceptor agonists for the treatment of overactive bladder. J Urol (2013) 190:1173–80. doi: 10.1016/j.juro.2013.02.104
156. Cypess AM, Weiner LS, Roberts-Toler C, Elia EF, Kessler SH, Kahn PA, et al. Activation of human brown adipose tissue by a beta3-adrenergic receptor agonist. Cell Metab (2015) 21:33–8. doi: 10.1016/j.cmet.2014.12.009
157. Johnson JA, Liggett SB. Cardiovascular pharmacogenomics of adrenergic receptor signaling: clinical implications and future directions. Clin Pharmacol Ther (2011) 89:366–78. doi: 10.1038/clpt.2010.315
158. Finlin BS, Memetimin H, Zhu B, Confides AL, Vekaria HJ, El Khouli RH, et al. The beta3-adrenergic receptor agonist mirabegron improves glucose homeostasis in obese humans. J Clin Invest (2020) 130:2319–31. doi: 10.1172/JCI134892
159. Orava J, Nuutila P, Noponen T, Parkkola R, Viljanen T, Enerback S, et al. Blunted metabolic responses to cold and insulin stimulation in brown adipose tissue of obese humans. Obes (Silver Spring) (2013) 21:2279–87. doi: 10.1002/oby.20456
160. Yoneshiro T, Aita S, Matsushita M, Okamatsu-Ogura Y, Kameya T, Kawai Y, et al. Age-related decrease in cold-activated brown adipose tissue and accumulation of body fat in healthy humans. Obes (Silver Spring) (2011) 19:1755–60. doi: 10.1038/oby.2011.125
161. Yoneshiro T, Aita S, Matsushita M, Kameya T, Nakada K, Kawai Y, et al. Brown adipose tissue, whole-body energy expenditure, and thermogenesis in healthy adult men. Obes (Silver Spring) (2011) 19:13–6. doi: 10.1038/oby.2010.105
162. Rogers NH, Landa A, Park S, Smith RG. Aging leads to a programmed loss of brown adipocytes in murine subcutaneous white adipose tissue. Aging Cell (2012) 11:1074–83. doi: 10.1111/acel.12010
163. Niccoli T, Partridge L. Ageing as a risk factor for disease. Curr Biol (2012) 22:R741–52. doi: 10.1016/j.cub.2012.07.024
164. Tchkonia T, Morbeck DE, Von Zglinicki T, Van Deursen J, Lustgarten J, Scrable H, et al. Aging, and cellular senescence. Aging Cell (2010) 9:667–84. doi: 10.1111/j.1474-9726.2010.00608.x
165. Bahler L, Verberne HJ, Admiraal WM, Stok WJ, Soeters MR, Hoekstra JB, et al. Differences in sympathetic nervous stimulation of brown adipose tissue between the young and old, and the lean and obese. J Nucl Med (2016) 57:372–7. doi: 10.2967/jnumed.115.165829
166. Arner P, Kriegholm E, Engfeldt P, Bolinder J. Adrenergic regulation of lipolysis in situ at rest and during exercise. J Clin Invest (1990) 85:893–8. doi: 10.1172/JCI114516
167. Kawate R, Talan MI, Engel BT. Aged C57BL/6J mice respond to cold with increased sympathetic nervous activity in interscapular brown adipose tissue. J Gerontol (1993) 48:B180–3. doi: 10.1093/geronj/48.5.B180
168. Scarpace PJ, Mooradian AD, Morley JE. Age-associated decrease in beta-adrenergic receptors and adenylate cyclase activity in rat brown adipose tissue. J Gerontol (1988) 43:B65–70. doi: 10.1093/geronj/43.3.B65
169. Yoneshiro T, Ogawa T, Okamoto N, Matsushita M, Aita S, Kameya T, et al. Impact of UCP1 and beta3AR gene polymorphisms on age-related changes in brown adipose tissue and adiposity in humans. Int J Obes (Lond) (2013) 37:993–8. doi: 10.1038/ijo.2012.161
170. Nakayama K, Miyashita H, Yanagisawa Y, Iwamoto S. Seasonal effects of UCP1 gene polymorphism on visceral fat accumulation in Japanese adults. PloS One (2013) 8:e74720. doi: 10.1371/journal.pone.0074720
171. Berry DC, Jiang Y, Arpke RW, Close EL, Uchida A, Reading D, et al. Cellular aging contributes to failure of cold-induced beige adipocyte formation in old mice and humans. Cell Metab (2017) 25:166–81. doi: 10.1016/j.cmet.2016.10.023
172. Coppe JP, Desprez PY, Krtolica A, Campisi J. The senescence-associated secretory phenotype: The dark side of tumor suppression. Annu Rev Pathol-Mech (2010) 5:99–118. doi: 10.1146/annurev-pathol-121808-102144
173. Tchkonia T, Zhu Y, van Deursen J, Campisi J, Kirkland JL. Cellular senescence and the senescent secretory phenotype: therapeutic opportunities. J Clin Invest (2013) 123:966–72. doi: 10.1172/JCI64098
174. Xu M, Pirtskhalava T, Farr JN, Weigand BM, Palmer AK, Weivoda MM, et al. Senolytics improve physical function and increase lifespan in old age. Nat Med (2018) 24:1246–56. doi: 10.1038/s41591-018-0092-9
175. Wang L, Wang B, Gasek NS, Zhou Y, Cohn RL, Martin DE, et al. Targeting p21(Cip1) highly expressing cells in adipose tissue alleviates insulin resistance in obesity. Cell Metab (2022) 34:186. doi: 10.1016/j.cmet.2021.12.014
176. Fu W, Liu Y, Sun C, Yin H. Transient p53 inhibition sensitizes aged white adipose tissue for beige adipocyte recruitment by blocking mitophagy. FASEB J (2019) 33:844–56. doi: 10.1096/fj.201800577R
177. Palmer AK, Xu M, Zhu Y, Pirtskhalava T, Weivoda MM, Hachfeld CM, et al. Targeting senescent cells alleviates obesity-induced metabolic dysfunction. Aging Cell (2019) 18:e12950. doi: 10.1111/acel.12950
178. Robbins PD, Jurk D, Khosla S, Kirkland JL, LeBrasseur NK, Miller JD, et al. Senolytic drugs: Reducing senescent cell viability to extend health span. Annu Rev Pharmacol Toxicol (2021) 61:779–803. doi: 10.1146/annurev-pharmtox-050120-105018
179. Wang W, Ishibashi J, Trefely S, Shao M, Cowan AJ, Sakers A, et al. A PRDM16-driven metabolic signal from adipocytes regulates precursor cell fate. Cell Metab (2019) 30:174–189 e5. doi: 10.1016/j.cmet.2019.05.005
180. Amorim JA, Coppotelli G, Rolo AP, Palmeira CM, Ross JM, Sinclair DA. Mitochondrial and metabolic dysfunction in ageing and age-related diseases. Nat Rev Endocrinol (2022) 18:243–58. doi: 10.1038/s41574-021-00626-7
181. Chen G, Kroemer G, Kepp O. Mitophagy: An emerging role in aging and age-associated diseases. Front Cell Dev Biol (2020) 8:200. doi: 10.3389/fcell.2020.00200
182. Lu X, Altshuler-Keylin S, Wang Q, Chen Y, Henrique Sponton C, Ikeda K, et al. Mitophagy controls beige adipocyte maintenance through a parkin-dependent and UCP1-independent mechanism. Sci Signal (2018) 11. doi: 10.1126/scisignal.aap8526
183. Altshuler-Keylin S, Shinoda K, Hasegawa Y, Ikeda K, Hong H, Kang Q, et al. Beige adipocyte maintenance is regulated by autophagy-induced mitochondrial clearance. Cell Metab (2016) 24:402–19. doi: 10.1016/j.cmet.2016.08.002
184. Duteil D, Tosic M, Schule R. Lsd1, a metabolic sensor of environment requirements that prevents adipose tissue from aging. Adipocyte (2017) 6:298–303. doi: 10.1080/21623945.2017.1345831
185. Duteil D, Tosic M, Willmann D, Georgiadi A, Kanouni T, Schule R. Lsd1 prevents age-programed loss of beige adipocytes. Proc Natl Acad Sci U.S.A. (2017) 114:5265–70. doi: 10.1073/pnas.1702641114
186. Rosina M, Ceci V, Turchi R, Chuan L, Borcherding N, Sciarretta F, et al. Ejection of damaged mitochondria and their removal by macrophages ensure efficient thermogenesis in brown adipose tissue. Cell Metab (2022) 34:533–548 e12. doi: 10.1016/j.cmet.2022.02.016
187. H. al-Adsani LJ, Hoffer, Silva JE. Resting energy expenditure is sensitive to small dose changes in patients on chronic thyroid hormone replacement. J Clin Endocrinol Metab (1997) 82:1118–25. doi: 10.1210/jc.82.4.1118
188. Rabelo R, Reyes C, Schifman A, Silva JE. Interactions among receptors, thyroid hormone response elements, and ligands in the regulation of the rat uncoupling protein gene expression by thyroid hormone. Endocrinology (1996) 137:3478–87. doi: 10.1210/endo.137.8.8754777
189. Silva JE. The multiple contributions of thyroid hormone to heat production. J Clin Invest (2001) 108:35–7. doi: 10.1172/JCI200113397
190. Weiner J, Hankir M, Heiker JT, Fenske W, Krause K. Thyroid hormones and browning of adipose tissue. Mol Cell Endocrinol (2017) 458:156–9. doi: 10.1016/j.mce.2017.01.011
191. Salvatore D, Simonides WS, Dentice M, Zavacki AM, Larsen PR. Thyroid hormones and skeletal muscle–new insights and potential implications. Nat Rev Endocrinol (2014) 10:206–14. doi: 10.1038/nrendo.2013.238
192. Weiner J, Kranz M, Kloting N, Kunath A, Steinhoff K, Rijntjes E, et al. Thyroid hormone status defines brown adipose tissue activity and browning of white adipose tissues in mice. Sci Rep (2016) 6:38124. doi: 10.1038/srep38124
193. Mullur R, Liu YY, Brent GA. Thyroid hormone regulation of metabolism. Physiol Rev (2014) 94:355–82. doi: 10.1152/physrev.00030.2013
194. Zhang Z, Boelen A, Kalsbeek A, Fliers E, Neurons TRH. And thyroid hormone coordinate the hypothalamic response to cold. Eur Thyroid J (2018) 7:279–88. doi: 10.1159/000493976
195. Herrmann J, Heinen E, Kroll HJ, Rudorff KH, Kruskemper HL. Thyroid function and thyroid hormone metabolism in elderly people. low T3-syndrome in old age? Klin Wochenschr (1981) 59:315–23. doi: 10.1007/BF01525000
196. Johann K, Cremer AL, Fischer AW, Heine M, Pensado ER, Resch J, et al. Thyroid-Hormone-Induced browning of white adipose tissue does not contribute to thermogenesis and glucose consumption. Cell Rep (2019) 27:3385–3400 e3. doi: 10.1016/j.celrep.2019.05.054
197. Dittner C, Lindsund E, Cannon B, Nedergaard J. At thermoneutrality, acute thyroxine-induced thermogenesis and pyrexia are independent of UCP1. Mol Metab (2019) 25:20–34. doi: 10.1016/j.molmet.2019.05.005
198. Broeders EP, Vijgen GH, Havekes B, Bouvy ND, Mottaghy FM, Kars M, et al. Thyroid hormone activates brown adipose tissue and increases non-shivering thermogenesis–a cohort study in a group of thyroid carcinoma patients. PloS One (2016) 11:e0145049. doi: 10.1371/journal.pone.0145049
199. Kushchayeva YS, Startzell M, Cochran E, Auh S, Sekizkardes H, Soldin SJ, et al. Thyroid hormone effects on glucose disposal in patients with insulin receptor mutations. J Clin Endocrinol Metab (2020) 105:e158–71. doi: 10.1210/clinem/dgz079
200. O'Neill LA, Kishton RJ, Rathmell J. A guide to immunometabolism for immunologists. Nat Rev Immunol (2016) 16:553–65. doi: 10.1038/nri.2016.70
201. Brestoff JR, Kim BS, Saenz SA, Stine RR, Monticelli LA, Sonnenberg GF, et al. Group 2 innate lymphoid cells promote beiging of white adipose tissue and limit obesity. Nature (2015) 519:242–6. doi: 10.1038/nature14115
202. Nussbaum JC, Van Dyken SJ, von Moltke J, Cheng LE, Mohapatra A, Molofsky AB, et al. Type 2 innate lymphoid cells control eosinophil homeostasis. Nature (2013) 502:245–8. doi: 10.1038/nature12526
203. Lee MW, Odegaard JI, Mukundan L, Qiu Y, Molofsky AB, Nussbaum JC, et al. Activated type 2 innate lymphoid cells regulate beige fat biogenesis. Cell (2015) 160:74–87. doi: 10.1016/j.cell.2014.12.011
204. Griesenauer B, Paczesny S. The ST2/IL-33 axis in immune cells during inflammatory diseases. Front Immunol (2017) 8:475. doi: 10.3389/fimmu.2017.00475
205. Shan B, Shao M, Zhang Q, An YA, Vishvanath L, Gupta RK. Cold-responsive adipocyte progenitors couple adrenergic signaling to immune cell activation to promote beige adipocyte accrual. Genes Dev (2021) 35:1333–8. doi: 10.1101/gad.348762.121
206. Goldberg EL, Shchukina I, Youm YH, Ryu S, Tsusaka T, Young KC, et al. IL-33 causes thermogenic failure in aging by expanding dysfunctional adipose ILC2. Cell Metab (2021) 33:2277–2287 e5. doi: 10.1016/j.cmet.2021.08.004
207. Nguyen HP, Lin F, Yi D, Xie Y, Dinh J, Xue P, et al. Aging-dependent regulatory cells emerge in subcutaneous fat to inhibit adipogenesis. Dev Cell (2021) 56:1437–1451 e3. doi: 10.1016/j.devcel.2021.03.026
208. Rajbhandari P, Thomas BJ, Feng AC, Hong C, Wang J, Vergnes L, et al. IL-10 signaling remodels adipose chromatin architecture to limit thermogenesis and energy expenditure. Cell (2018) 172:218–233 e17. doi: 10.1016/j.cell.2017.11.019
209. Rabhi N, Belkina AC, Desevin K, Cortez BN, Farmer SR. Shifts of immune cell populations differ in response to different effectors of beige remodeling of adipose tissue. iScience (2020) 23:101765. doi: 10.1016/j.isci.2020.101765
210. Vidal P, Stanford KI. Exercise-induced adaptations to adipose tissue thermogenesis. Front Endocrinol (Lausanne) (2020) 11:270. doi: 10.3389/fendo.2020.00270
211. Stanford KI, Middelbeek RJ, Townsend KL, Lee MY, Takahashi H, So K, et al. A novel role for subcutaneous adipose tissue in exercise-induced improvements in glucose homeostasis. Diabetes (2015) 64:2002–14. doi: 10.2337/db14-0704
212. Wu MV, Bikopoulos G, Hung S, Ceddia RB. Thermogenic capacity is antagonistically regulated in classical brown and white subcutaneous fat depots by high fat diet and endurance training in rats: impact on whole-body energy expenditure. J Biol Chem (2014) 289:34129–40. doi: 10.1074/jbc.M114.591008
213. Brandao CFC, de Carvalho FG, Souza AO, Junqueira-Franco MVM, Batitucci G, Couto-Lima CA, et al. UCP1 expression, mitochondrial density, and coupling in adipose tissue from women with obesity. Scand J Med Sci Sports (2019) 29:1699–706. doi: 10.1111/sms.13514
214. Camera DM, Anderson MJ, Hawley JA, Carey AL. Short-term endurance training does not alter the oxidative capacity of human subcutaneous adipose tissue. Eur J Appl Physiol (2010) 109:307–16. doi: 10.1007/s00421-010-1356-3
215. Norheim F, Langleite TM, Hjorth M, Holen T, Kielland A, Stadheim HK, et al. The effects of acute and chronic exercise on PGC-1alpha, irisin and browning of subcutaneous adipose tissue in humans. FEBS J (2014) 281:739–49. doi: 10.1111/febs.12619
216. Tsiloulis T, Carey AL, Bayliss J, Canny B, Meex RCR, Watt MJ. No evidence of white adipocyte browning after endurance exercise training in obese men. Int J Obes (Lond) (2018) 42:721–7. doi: 10.1038/ijo.2017.295
217. Stinkens R, Brouwers B, Jocken JW, Blaak EE, Teunissen-Beekman KF, Hesselink MK, et al. Exercise training-induced effects on the abdominal subcutaneous adipose tissue phenotype in humans with obesity. J Appl Physiol (1985) 125(2018):1585–93. doi: 10.1152/japplphysiol.00496.2018
218. Pino MF, Parsons SA, Smith SR, Sparks LM. Active individuals have high mitochondrial content and oxidative markers in their abdominal subcutaneous adipose tissue. Obes (Silver Spring) (2016) 24:2467–70. doi: 10.1002/oby.21669
219. Vosselman MJ, Hoeks J, Brans B, Pallubinsky H, Nascimento EB, van der Lans AA, et al. Low brown adipose tissue activity in endurance-trained compared with lean sedentary men. Int J Obes (Lond) (2015) 39:1696–702. doi: 10.1038/ijo.2015.130
220. Lehnig AC, Stanford KI. Exercise-induced adaptations to white and brown adipose tissue. J Exp Biol 221 (2018) 221. doi: 10.1242/jeb.161570
221. Saugen E, Vollestad NK. Nonlinear relationship between heat production and force during voluntary contractions in humans. J Appl Physiol (1985) 79(1995):2043–9. doi: 10.1152/jappl.1995.79.6.2043
222. Ranallo RF, Rhodes EC. Lipid metabolism during exercise. Sports Med (1998) 26:29–42. doi: 10.2165/00007256-199826010-00003
223. Bostrom P, Wu J, Jedrychowski MP, Korde A, Ye L, Lo JC, et al. A PGC1-alpha-dependent myokine that drives brown-fat-like development of white fat and thermogenesis. Nature (2012) 481:463–8. doi: 10.1038/nature10777
224. Feldman BJ, Streeper RS, Farese RV Jr., Yamamoto KR. Myostatin modulates adipogenesis to generate adipocytes with favorable metabolic effects. Proc Natl Acad Sci U.S.A. (2006) 103:15675–80. doi: 10.1073/pnas.0607501103
225. Rao RR, Long JZ, White JP, Svensson KJ, Lou J, Lokurkar I, et al. Meteorin-like is a hormone that regulates immune-adipose interactions to increase beige fat thermogenesis. Cell (2014) 157:1279–91. doi: 10.1016/j.cell.2014.03.065
226. Carriere A, Jeanson Y, Berger-Muller S, Andre M, Chenouard V, Arnaud E, et al. Browning of white adipose cells by intermediate metabolites: an adaptive mechanism to alleviate redox pressure. Diabetes (2014) 63:3253–65. doi: 10.2337/db13-1885
227. Roberts LD, Bostrom P, O'Sullivan JF, Schinzel RT, Lewis GD, Dejam A, et al. Beta-aminoisobutyric acid induces browning of white fat and hepatic beta-oxidation and is inversely correlated with cardiometabolic risk factors. Cell Metab (2014) 19:96–108. doi: 10.1016/j.cmet.2013.12.003
228. Knuth CM, Peppler WT, Townsend LK, Miotto PM, Gudiksen A, Wright DC. Prior exercise training improves cold tolerance independent of indices associated with non-shivering thermogenesis. J Physiol (2018) 596:4375–91. doi: 10.1113/JP276228
229. Srivastava S, Baxa U, Niu G, Chen X, Veech RL. A ketogenic diet increases brown adipose tissue mitochondrial proteins and UCP1 levels in mice. IUBMB Life (2013) 65:58–66. doi: 10.1002/iub.1102
230. Vrieze A, Schopman JE, Admiraal WM, Soeters MR, Nieuwdorp M, Verberne HJ, et al. Fasting and postprandial activity of brown adipose tissue in healthy men. J Nucl Med (2012) 53:1407–10. doi: 10.2967/jnumed.111.100701
231. Vosselman MJ, Brans B, van der Lans AA, Wierts R, van Baak MA, Mottaghy FM, et al. Brown adipose tissue activity after a high-calorie meal in humans. Am J Clin Nutr (2013) 98:57–64. doi: 10.3945/ajcn.113.059022
232. Muzik O, Mangner TJ, Leonard WR, Kumar A, Janisse J, Granneman JG. 15O PET measurement of blood flow and oxygen consumption in cold-activated human brown fat. J Nucl Med (2013) 54:523–31. doi: 10.2967/jnumed.112.111336
233. Hibi M, Oishi S, Matsushita M, Yoneshiro T, Yamaguchi T, Usui C, et al. Brown adipose tissue is involved in diet-induced thermogenesis and whole-body fat utilization in healthy humans. Int J Obes (Lond) (2016) 40:1655–61. doi: 10.1038/ijo.2016.124
234. Luo XJ, Peng J, Li YJ. Recent advances in the study on capsaicinoids and capsinoids. Eur J Pharmacol (2011) 650:1–7. doi: 10.1016/j.ejphar.2010.09.074
235. Kawada T, Hagihara K, Iwai K. Effects of capsaicin on lipid metabolism in rats fed a high fat diet. J Nutr (1986) 116:1272–8. doi: 10.1093/jn/116.7.1272
236. Kawada T, Watanabe T, Takaishi T, Tanaka T, Iwai K. Capsaicin-induced beta-adrenergic action on energy metabolism in rats: influence of capsaicin on oxygen consumption, the respiratory quotient, and substrate utilization. Proc Soc Exp Biol Med (1986) 183:250–6. doi: 10.3181/00379727-183-42414
237. Lejeune MP, Kovacs EM, Westerterp-Plantenga MS. Effect of capsaicin on substrate oxidation and weight maintenance after modest body-weight loss in human subjects. Br J Nutr (2003) 90:651–59. doi: 10.1079/BJN2003938
238. Smeets AJ, Westerterp-Plantenga MS. The acute effects of a lunch containing capsaicin on energy and substrate utilisation, hormones, and satiety. Eur J Nutr (2009) 48:229–34. doi: 10.1007/s00394-009-0006-1
239. Yoshioka M, St-Pierre S, Suzuki M, Tremblay A. Effects of red pepper added to high-fat and high-carbohydrate meals on energy metabolism and substrate utilization in Japanese women. Br J Nutr (1998) 80:503–10. doi: 10.1017/S0007114598001597
240. Saito M, Matsushita M, Yoneshiro T, Okamatsu-Ogura Y. Brown adipose tissue, diet-induced thermogenesis, and thermogenic food ingredients: From mice to men. Front Endocrinol (Lausanne) (2020) 11:222. doi: 10.3389/fendo.2020.00222
241. Inoue N, Matsunaga Y, Satoh H, Takahashi M. Enhanced energy expenditure and fat oxidation in humans with high BMI scores by the ingestion of novel and non-pungent capsaicin analogues (capsinoids). Biosci Biotechnol Biochem (2007) 71:380–9. doi: 10.1271/bbb.60341
242. Yoneshiro T, Aita S, Kawai Y, Iwanaga T, Saito M. Nonpungent capsaicin analogs (capsinoids) increase energy expenditure through the activation of brown adipose tissue in humans. Am J Clin Nutr (2012) 95:845–50. doi: 10.3945/ajcn.111.018606
243. Vriens J, Nilius B, Vennekens R. Herbal compounds and toxins modulating TRP channels. Curr Neuropharmacol (2008) 6:79–96. doi: 10.2174/157015908783769644
244. Hui K, Liu B, Qin F. Capsaicin activation of the pain receptor, VR1: multiple open states from both partial and full binding. Biophys J (2003) 84:2957–68. doi: 10.1016/S0006-3495(03)70022-8
245. Baskaran P, Krishnan V, Ren J, Thyagarajan B. Capsaicin induces browning of white adipose tissue and counters obesity by activating TRPV1 channel-dependent mechanisms. Br J Pharmacol (2016) 173:2369–89. doi: 10.1111/bph.13514
246. Dedov VN, Tran VH, Duke CC, Connor M, Christie MJ, Mandadi S, et al. Gingerols: a novel class of vanilloid receptor (VR1) agonists. Br J Pharmacol (2002) 137:793–8. doi: 10.1038/sj.bjp.0704925
247. Macpherson LJ, Geierstanger BH, Viswanath V, Bandell M, Eid SR, Hwang S, et al. The pungency of garlic: activation of TRPA1 and TRPV1 in response to allicin. Curr Biol (2005) 15:929–34. doi: 10.1016/j.cub.2005.04.018
248. Broeders E, Bouvy ND, van Marken Lichtenbelt WD. Endogenous ways to stimulate brown adipose tissue in humans. Ann Med (2014) 47(2):123–32. doi: 10.3109/07853890.2013.874663
249. Sanders OD, Rajagopal JA, Rajagopal L. Menthol to induce non-shivering thermogenesis via TRPM8/PKA signaling for treatment of obesity. J Obes Metab Syndr (2021) 30:4–11. doi: 10.7570/jomes20038
250. Andrade JM, Frade AC, Guimaraes JB, Freitas KM, Lopes MT, Guimaraes AL, et al. Resveratrol increases brown adipose tissue thermogenesis markers by increasing SIRT1 and energy expenditure and decreasing fat accumulation in adipose tissue of mice fed a standard diet. Eur J Nutr (2014) 53:1503–10. doi: 10.1007/s00394-014-0655-6
251. Kim M, Goto T, Yu R, Uchida K, Tominaga M, Kano Y, et al. Fish oil intake induces UCP1 upregulation in brown and white adipose tissue via the sympathetic nervous system. Sci Rep (2015) 5:18013. doi: 10.1038/srep18013
252. Berry DC, Noy N. All-trans-retinoic acid represses obesity and insulin resistance by activating both peroxisome proliferation-activated receptor beta/delta and retinoic acid receptor. Mol Cell Biol (2009) 29:3286–96. doi: 10.1128/MCB.01742-08
253. Berry DC, DeSantis D, Soltanian H, Croniger CM, Noy N. Retinoic acid upregulates preadipocyte genes to block adipogenesis and suppress diet-induced obesity. Diabetes (2012) 61:1112–21. doi: 10.2337/db11-1620
254. Stierman B, Afful J, Carroll MD, Chen T-C, Davy O, Fink S, et al. National health and nutrition examination survey 2017–march 2020 prepandemic data files development of files and prevalence estimates for selected health outcomes. Natl Health Stat Rep (2021). doi: 10.15620/cdc:106273
255. Cypess AM. Reassessing human adipose tissue. N Engl J Med (2022) 386:768–79. doi: 10.1056/NEJMra2032804
256. Bartelt A, Heeren J. Adipose tissue browning and metabolic health. Nat Rev Endocrinol (2014) 10:24–36. doi: 10.1038/nrendo.2013.204
257. Lidell ME, Betz MJ, Enerback S. Brown adipose tissue and its therapeutic potential. J Intern Med (2014) 276(4):364–77. doi: 10.1111/joim.12255
258. Sidossis L, Kajimura S. Brown and beige fat in humans: thermogenic adipocytes that control energy and glucose homeostasis. J Clin Invest (2015) 125:478–86. doi: 10.1172/JCI78362
259. Blondin DP, Nielsen S, Kuipers EN, Severinsen MC, Jensen VH, Miard S, et al. Human brown adipocyte thermogenesis is driven by beta2-AR stimulation. Cell Metab (2020) 32:287–300 e7. doi: 10.1016/j.cmet.2020.07.005
260. Cero C, Lea HJ, Zhu KY, Shamsi F, Tseng YH, Cypess AM. beta3-adrenergic receptors regulate human brown/beige adipocyte lipolysis and thermogenesis. JCI Insight (2021) 6. doi: 10.1172/jci.insight.139160
261. Shamsi F, Wang CH, Tseng YH. The evolving view of thermogenic adipocytes - ontogeny, niche and function. Nat Rev Endocrinol (2021) 17:726–44. doi: 10.1038/s41574-021-00562-6
262. Wagner DE, Klein AM. Lineage tracing meets single-cell omics: opportunities and challenges. Nat Rev Genet (2020) 21:410–27. doi: 10.1038/s41576-020-0223-2
263. Kretzschmar K, Watt FM. Lineage tracing. Cell (2012) 148:33–45. doi: 10.1016/j.cell.2012.01.002
Keywords: beige adipocyte, brown adipocyte development, aging, obesity, metabolism & endocrinology
Citation: Xue S, Lee D and Berry DC (2023) Thermogenic adipose tissue in energy regulation and metabolic health. Front. Endocrinol. 14:1150059. doi: 10.3389/fendo.2023.1150059
Received: 23 January 2023; Accepted: 07 March 2023;
Published: 20 March 2023.
Edited by:
XueJiang Gu, First Affiliated Hospital of Wenzhou Medical University, ChinaReviewed by:
Nabil Rabhi, Boston University, United StatesCopyright © 2023 Xue, Lee and Berry. This is an open-access article distributed under the terms of the Creative Commons Attribution License (CC BY). The use, distribution or reproduction in other forums is permitted, provided the original author(s) and the copyright owner(s) are credited and that the original publication in this journal is cited, in accordance with accepted academic practice. No use, distribution or reproduction is permitted which does not comply with these terms.
*Correspondence: Daniel C. Berry, ZGNiMzdAY29ybmVsbC5lZHU=
Disclaimer: All claims expressed in this article are solely those of the authors and do not necessarily represent those of their affiliated organizations, or those of the publisher, the editors and the reviewers. Any product that may be evaluated in this article or claim that may be made by its manufacturer is not guaranteed or endorsed by the publisher.
Research integrity at Frontiers
Learn more about the work of our research integrity team to safeguard the quality of each article we publish.