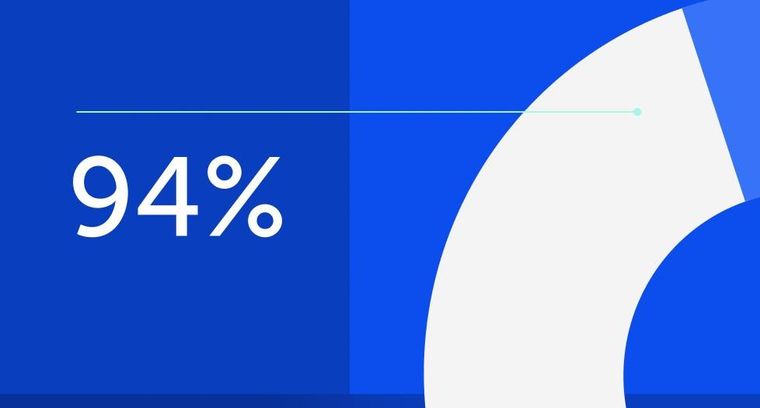
94% of researchers rate our articles as excellent or good
Learn more about the work of our research integrity team to safeguard the quality of each article we publish.
Find out more
ORIGINAL RESEARCH article
Front. Endocrinol., 17 April 2023
Sec. Obesity
Volume 14 - 2023 | https://doi.org/10.3389/fendo.2023.1148954
This article is part of the Research TopicNovel Regulatory Mechanisms behind Thermogenesis of Brown and Beige AdipocytesView all 11 articles
Introduction: Naringenin, a peroxisome proliferator-activated receptor (PPAR) activator found in citrus fruits, upregulates markers of thermogenesis and insulin sensitivity in human adipose tissue. Our pharmacokinetics clinical trial demonstrated that naringenin is safe and bioavailable, and our case report showed that naringenin causes weight loss and improves insulin sensitivity. PPARs form heterodimers with retinoic-X-receptors (RXRs) at promoter elements of target genes. Retinoic acid is an RXR ligand metabolized from dietary carotenoids. The carotenoid β-carotene reduces adiposity and insulin resistance in clinical trials. Our goal was to examine if carotenoids strengthen the beneficial effects of naringenin on human adipocyte metabolism.
Methods: Human preadipocytes from donors with obesity were differentiated in culture and treated with 8µM naringenin + 2µM β-carotene (NRBC) for seven days. Candidate genes involved in thermogenesis and glucose metabolism were measured as well as hormone-stimulated lipolysis.
Results: We found that β-carotene acts synergistically with naringenin to boost UCP1 and glucose metabolism genes including GLUT4 and adiponectin, compared to naringenin alone. Protein levels of PPARα, PPARγ and PPARγ-coactivator-1α, key modulators of thermogenesis and insulin sensitivity, were also upregulated after treatment with NRBC. Transcriptome sequencing was conducted and the bioinformatics analyses of the data revealed that NRBC induced enzymes for several non-UCP1 pathways for energy expenditure including triglyceride cycling, creatine kinases, and Peptidase M20 Domain Containing 1 (PM20D1). A comprehensive analysis of changes in receptor expression showed that NRBC upregulated eight receptors that have been linked to lipolysis or thermogenesis including the β1-adrenergic receptor and the parathyroid hormone receptor. NRBC increased levels of triglyceride lipases and agonist-stimulated lipolysis in adipocytes. We observed that expression of RXRγ, an isoform of unknown function, was induced ten-fold after treatment with NRBC. We show that RXRγ is a coactivator bound to the immunoprecipitated PPARγ protein complex from white and beige human adipocytes.
Discussion: There is a need for obesity treatments that can be administered long-term without side effects. NRBC increases the abundance and lipolytic response of multiple receptors for hormones released after exercise and cold exposure. Lipolysis provides the fuel for thermogenesis, and these observations suggest that NRBC has therapeutic potential.
Adipose tissue is a complex, adaptable organ composed of multiple types of adipocytes which vary in function (1). White adipocytes store triglycerides and expand in number and size under conditions of excess energy intake. Brown and beige adipocytes abound in mitochondria and express uncoupling protein 1 (UCP1), a protein that shifts mitochondrial fat oxidation away from ATP production and towards thermogenesis. Increased density of beige adipocytes and UCP1 in fat depots is associated with elevated energy expenditure and resistance to weight gain and type 2 diabetes (2–4). In rodents, white adipose tissues can adapt to a chronic environmental stimulus such as cold exposure by producing beige adipocytes from precursor cells and by converting white adipocytes into beige cells (5). The adaptive response to cold exposure involves release of norepinephrine by sympathetic nerve fibers to activate β3-adrenergic receptors (β3AR) abundantly expressed in adipocytes (6).
In contrast to rodents, human adipocytes express extremely low levels of the βARs. Humans lack a robust response to systemic infusion of β-adrenergic agonists even when combined with cold exposure (7–9). However, synthetic peroxisome proliferator activator receptor (PPAR)α and PPARƴ ligands have been shown to have potent activity in the conversion of primary human white adipocytes to beige UCP1-expressing cells in vitro (10, 11). PPARs are ligand-activated nuclear receptors enriched in metabolic tissues, and they regulate UCP1 and many genes controlling fat oxidation and insulin sensitivity by binding to upstream PPAR responsive elements (PPREs). PPARγ is subject to complex cell-specific post-translational regulatory mechanisms and has multiple ligand binding domains (12). Depending on the binding characteristics of a particular ligand, PPARγ can stimulate adipogenesis, insulin sensitivity, fat oxidation or thermogenesis in adipose tissues (13).
Thiazolidinediones (TZDs) are a class of potent PPARγ agonists that have been approved by the United States Food and Drug Administration (FDA) for the treatment of type 2 diabetes. However, TZDs have multiple adverse effects including weight gain, heart failure, and risk for bladder cancer (14, 15). PPARα agonists primarily upregulate genes for lipolysis and mitochondrial β-oxidation of fatty acids in primary human adipocytes (16). A synthetic PPARα activator, fenofibrate, has been approved for treatment of dyslipidemia (17, 18). At this time, there are no FDA-approved PPARγ or PPARα activators for treatment of obesity (19–21).
Despite the complex regulation of ligand binding to PPARγ, evidence is growing that selective modulators and partial agonists can direct activity toward thermogenesis and away from adipogenesis (12, 22, 23). Naringenin (NR), a polyphenol found in citrus fruit, activates transcriptional activity of PPARγ and PPARα in an expression system with a PPRE linked to a reporter gene (24). In a previous study, we treated human subcutaneous adipocytes with NR and saw induction of UCP1 mRNA as well as increases in basal and maximal oxygen consumption rate (OCR) (25). We used selective inhibitors to demonstrate that upregulation of thermogenesis genes by NR requires activation of both PPARγ and PPARα in adipocytes (26). Data from our clinical studies suggest that naringenin is bioavailable and has potential as a treatment for obesity and type 2 diabetes. Our pharmacokinetic clinical trial showed that naringenin is safe and well-tolerated at doses ranging from 150 mg to 900 mg (27). In a case study of an individual with obesity and untreated type 2 diabetes, we found that body weight and fasting insulin concentrations decreased, and there was a measurable increase in energy expenditure after ingestion of NR for eight weeks (26).
Carotenoids are vitamin A precursors found in fruits and vegetables, and their consumption has been shown to reduce fat mass and insulin resistance in children. Baseline blood concentrations of β-carotene (BC) are inversely correlated with fat mass (28, 29). Most carotenoids are metabolized into retinoid ligands for retinoic acid receptors (RAR) and retinoic-X-receptors (RXR), which are transcriptional coactivators for PPARs. The objective of this study was to determine whether carotenoids could amplify the effects of NR on gene expression and function to convert primary human adipocytes to a beige phenotype. We observed synergistic increases in a subset of mRNAs including UCP1, GLUT4, ATGL and adiponectin with the combination of NR and BC (NRBC). Protein levels of PPARα, PPARγ, PGC-1α and NAMPT were selectively upregulated without increases in mRNA levels. Whole transcriptome sequencing was conducted, and the results showed that NRBC induced genes for multiple non-UCP1 energy-dissipating futile cycles, beneficial secreted peptides, and adipokines. Importantly, NRBC increased levels of multiple thermogenesis and lipolysis-linked receptors including the β1- adrenergic receptor (β1AR), parathyroid hormone receptor (PTHR), and the stimulatory ratio of natriuretic peptide receptors (NPR1/NPR3). A comprehensive analysis of lipolysis was conducted, and the results showed that the capacity for PTHR and βAR agonist-stimulated lipolysis was substantially higher in NRBC-treated adipocytes compared to untreated cells. Expression of RXRγ, a unique isoform associated with brown adipogenesis, was upregulated ten-fold after treatment. We observed that RXRγ was bound in the PPARγ transcriptional complex immunoprecipitated from human adipocytes. These results suggest that NRBC has potential as a treatment for obesity and type 2 diabetes and is safe primarily because it acts on peripheral tissues, unlike most obesity medications that act on the central nervous system (CNS) (30).
Naringenin extract (NR) from whole citrus sinensis oranges (purity ≥ 30%) was purchased from GE Nutrients, Inc. (Gencor, Irvine, CA). BC, lycopene, and lutein were from Cayman Chemical Co. Protease and phosphatase inhibitors were purchased from Cell Signaling Technology (Danvers, MA), TGX protein gels from BIO-RAD (Hercules, CA). Type 1 collagenase, glycerol standard solution, adenosine, estradiol, human pituitary growth hormone, dobutamine hydrochloride, human atrial natriuretic peptide, ACTH, menthol, 8-CPT-cAMP were purchased from Sigma-Aldrich. Isoproterenol, human parathyroid hormone (1–34), CDCA, were purchased from Cayman Chemicals. Glycerol reagent A was from ZenBio (Durham, NC). All other chemicals were purchased from Sigma (St. Louis, Mo) unless otherwise indicated.
Primary antibodies used were UCP1 (#MAB6158, R&D Systems), GLUT4 (Ab654 Abcam), PGC-1α (ST1202, Sigma) and β-Actin (A5316, Sigma), or monoclonals from Santa Cruz against ATGL (sc-365278), adiponectin (sc-136131), PPARα (398394), PPARγ (sc-7273), NAMPT (sc-393444), RXRγ (sc-514134), RXRα (sc-515929). HRP-linked anti-rabbit (12-348, Sigma), anti-mouse (AP130P, Sigma) and anti-IgG kappa light chain (sc-516102, Santa Cruz) were used to detect specific antibody-antigen complexes. Western Lightning Plus-ECL was from PerkinElmer (Waltham, MA).
Human adipose-derived stem cells from overweight and obese female donors were purchased from LaCell, LLC (New Orleans, Louisiana) or isolated from lipoaspirate waste donated post-surgery from women with obesity using methods as previously described (31). Cells were seeded, maintained until two days after becoming confluent, and differentiated into adipocytes in the presence of rosiglitazone and isobutylmethylxanthine for five days as previously described (25). Treatments of adipocytes with 8µM NR and 2µM carotenoid, dissolved in DMSO at 1000X, started 5 days after the differentiation period and lasted for seven days in adipocyte maintenance medium with heat inactivated serum, before RNA and protein were isolated from adipocyte cultures.
Total RNA was extracted from cells using Tri-reagent and purified with RNeasy (Qiagen) into nuclease-free water with RNAsecure Reagent (Thermo Fisher Scientific). RNA integrity was assessed using an Agilent Bioanalyzer 2100. RNA samples were diluted to 50 ng/µL and libraries were constructed using Lexogen Quant-Seq 3’ mRNA-Seq Library Prep Kit (SKU015.96) with oligo(dT) priming. Double-stranded cDNA was purified with magnetic beads, libraries were amplified using PCR, and transcripts were indexed, pooled, and forward-sequenced at 50 bp using NextSeq500 (Illumina). BlueBee software was used to analyze alignment and the DESeq2 V1.32.0 package in R V4.1.0, Rstudio V1.4.1717 and biomaRt V2.48.2 were used for differential expression analysis after estimation of possible outlier counts per gene via the Cook’s distance, and their replacement by the trimmed mean over all counts for that gene. Differential gene expression results were computed for 17525 genes of which 3881 genes were differentially regulated at an adjusted p - value¾0.05. Pathway enrichment analysis was carried out via the Gene Set Enrichment Analysis (GSEA) tool (32) by estimating enrichment on pathways present in the Kyoto Encyclopedia of Genes and Genomes (KEGG) database (33) available from the Molecular Signatures Database repository (MSigDb, http://software.broadinstitute.org/gsea/msigdb) (34), and additional custom pathways. Gene-sets with FDR ≤ 5% were considered as significantly enriched (35).
Quantitative reverse transcriptase and real-time PCR were conducted in one reaction with the gene-specific reverse PCR primer also priming the cDNA synthesis as previously described (25). Primer-probe sequences for UCP1, GLUT4, ATGL, adiponectin, PGC-1α and ribosomal RPL13A, used to adjust target gene values for total RNA in each sample, have been previously reported (25). Additional primer and probe oligonucleotide sets used in this study are shown in 5’ to 3’ orientation: PPARα Forward GTCGATTTCACAAGTGCCTTTC reverse CAGGTAAGAATTTCTGCTTTCAGTT probe AACGAATCGCGTTGTGTGACATCC; PDK4 forward CTGAGAATTATTGACCGCCTCT reverse GAAATTGGCAAGCCGTAACC probe TACATACTCCACTGCACCAACGCC; PPARγ forward CCCAAGTTTGAGTTTGCTGTG reverse GCGGTCTCCACTGAGAATAATG probe TGGAATTAGATGACAGCGACTTGGCA; NAMPT forward TGTTCCTTCAAGTGTAGCTATGT reverse TGCTGGCGTCCTATGTAAAG probe AACGTCTTCAAGGACCCAGTTGCT; CKMT1 forward CTTGACCTGTCCATCTAACCTG reverse ACTCCTCCAGTACCACGTT probe AGATAGCCGCTTCCCAAAGATCCTG. Predesigned human primer and probe sets from Thermofisher Scientific are PM20D1 Hs00399438_m1; ANGPTL4 Hs00211522_m1; GDF11 Hs00195156_m1; S100B Hs00902901_m1.
Whole cell protein was isolated from differentiated cell cultures after treatment for seven days, lysed with RIPA buffer containing protease and phosphatase inhibitors, and pushed through a 20-gauge needle four times to disrupt organelles. Fifty micrograms of total cell protein were loaded per lane and resolved in 7.5% SDS-PAGE gels, transferred to nitrocellulose membranes, and probed overnight at 4°C with specific primary antibodies. Anti-rabbit or -mouse secondary antibodies conjugated to horseradish peroxidase were used for detection of target proteins. Image J was used to quantify protein bands on Western blots. To adjust for variations in total protein loaded in each lane, β-actin was used.
PPARγ was immunoprecipitated from whole cell lysates with SC-7273 antibody (Santa Cruz) using the Pierce MS-compatible magnetic IP kit with protein A/G beads according to kit directions (Pierce 90409, Thermofisher). Briefly, primary anti-PPARу antibody was incubated with 600 micrograms of adipocyte protein lysate for four hours at 4°C on a tube inverter. The antibody-lysate mixture was then incubated with magnetic protein A/G beads for one hour, and complexes of PPARγ protein attached to beads were isolated, washed and eluted using a magnetic tube holder. Samples were evaporated to dryness under vacuum, resuspended in RIPA buffer and subjected to Western Blot analysis. An HRP-conjugated anti- IgG kappa light chain secondary antibody was used to eliminate primary antibody heavy chain bands from interfering with detection of immunoprecipitated target proteins. Proteins were visualized by chemiluminescence using Western Lightening (Amersham).
Cells were differentiated in 96-well plates and treated with cell medium (untreated) or NRBC for seven days. On the day of the lipolysis assay, cells were exposed for four hours to buffer (KRB with 1% BSA) or receptor agonists dissolved in buffer. Agonists were used at concentrations shown to give maximum responses and were: 8-Cpt-cAMP 200µM non-hydrolyzable PKA activator of maximum stimulated lipolysis (cAMP) (36), atrial natriuretic peptide 0.1 µM for NPR1 and NPR3 (37), parathyroid hormone (amino acids 1-34) 1 µM for PTHR (38), isoproterenol 1µM all βARs and dobutamine 1µM for β1AR (39), estradiol 1µM for GPER (40), growth hormone 250ng/ml for GHR (41), adrenocorticotropin hormone 1µM for MC1R (42), bile acid chenodeoxycholic acid 30µM for TGR5 (43), adenosine 1µM for ADORA1 and ADORA2B (44), and menthol 100µM for TRPM8 (45). Supernatants were removed for measurement of glycerol released using Glycerol Reagent A, and concentrations were determined using a standard curve. Data are from four or five experiments each with cells from different donors with BMI from 27 to 36.
All statistical analyses were performed using SAS 9.4 (SAS Institute, Cary, North Carolina). The synergistic effect of the combination of NR and BC was investigated by implementing linear mixed effect models including plate as the random effect. The goal was to test if the combination (NRBC) induced a greater response compared to the sum of the individual components’ effects. Based on the mixed effect model, an F test was constructed to evaluate the null hypothesis that the additive effect of NR over control and BC over control was no different from that of NRBC over control. We also used linear mixed effect models to test the effects of: 1) NR, BC, and NRBC compared to control for the target variables of mRNA and protein; 2) Agonist stimulated lipolysis in NRBC pre-treated cells v. untreated cells. All experiments were repeated at least three times in primary adipocytes from different donors. Significance was set at p < 0.05. Data are reported as least squares means ± standard error unless otherwise specified.
Metabolites of pro-vitamin A carotenoids are ligands of the retinoid X receptor (RXR) family of nuclear receptors which can heterodimerize with PPARα and PPARγ and recruit coactivators into active transcriptional complexes (46). Our first objective was to evaluate whether treatment of adipocytes with carotenoids could elevate the levels of UCP1, a PPAR target gene known to be upregulated by NR and a marker for beige adipogenesis (25). Three of the most abundant carotenoids in foods and in human serum, BC, lycopene, and lutein were tested (47). Steady state plasma concentrations are approximately 2μM in individuals who eat diets rich in carotenoids, and we used this concentration in experiments (48). Since the mean serum concentration of NR after ingestion of 150mg is 8µM (27), we used this concentration in all assays. Treatment of primary human adipocyte cultures with BC, lutein or lycopene individually for seven days had no effect on gene expression (Figure 1). When BC or lutein were combined with NR for treatment, the increase in UCP1 gene expression was synergistic, greater than the sum of the individual effects of each compound (P<0.001). In contrast, lycopene did not alter NR-stimulated UCP1 levels. Unlike lycopene, BC and lutein are pro-vitamin A carotenoids that are metabolized into retinoic acid (49). These data are consistent with a hypothesis that RXR ligands act synergistically with NR to boost thermogenesis gene expression.
Figure 1 Pro-vitamin A carotenoids and NR act synergistically to elevate levels of UCP1 mRNAs Adipocytes from 4 donors with obesity were treated for seven days with vehicle (control) or 8µM NR and 2µM carotenoids. (A) NR+BC (B) NR+Lutein (C) NR+Lycopene mRNA levels were measured using quantitative RTPCR. Data is expressed in least squares means ± standard error. Synergy for mRNA was calculated as: sum of differences ((NR- Control) + (BC - Control)) vs (NRBC - Control). *p < 0.001 for synergy, sum of differences versus NRBC. NR, naringenin; BC, beta carotene.
We next evaluated the effects of NR, BC and NRBC on expression of additional genes. BC was chosen for the rest of the study because it is safe, stable, bioavailable and has a long half-life in circulation (50). Adipocytes from five donors with body mass index (BMI) ranging from 27 to 36kg/m2 were treated with NRBC for seven days. NRBC synergistically boosted mRNA levels for UCP1 (p<0.005), GLUT4 (p<0.02), ATGL (p<0.04) and adiponectin (p<0.05) compared to NR or BC alone (Figure 2A). Protein levels showed a similar trend (Figures 2B, C). Uncropped Western blots are shown in Supplemental Figure 1. ATGL is the rate-limiting lipase for hydrolysis of fatty acids from triglycerides (TGs) (51). GLUT4 is a transporter for glucose uptake, and its upregulation in adipocytes stimulates a cascade of events that reduce insulin resistance (52, 53). Adiponectin is a key circulating factor that acts on muscle and other tissues and improves whole body glucose homeostasis (54). In a previous study we reported induction of CPT1β mRNA in adipocytes after NR treatment (25). We did not see an increase in CPT1β levels with NRBC treatment in comparison to levels induced by NR alone.
Figure 2 NR and BC synergistically induce metabolism genes (A) mRNA levels (B) Western Blots of Protein levels (C) Protein levels were measured with β-actin as loading control. Adipocytes from three or more donors with obesity were treated for seven days. mRNA data are expressed as least squares means ± standard error. Synergy for mRNA was calculated as: sum of differences ((NR- Control) + (BC - Control)) vs (NRBC - Control). *p < 0.05 for synergy. NR, Naringenin; BC, beta carotene; NRBC, naringenin and beta carotene.
NRBC upregulated protein levels of PPARα, PPARγ, PGC-1α, and NAMPT three to six-fold (Figure 3, p<0.001) without comparable increases in mRNA levels. Uncropped Western blots are shown in Supplemental Figure 2. PPARα, PPARγ, and PGC-1α proteins have a short half-life and are rapidly degraded by ubiquitin-proteosome systems (55–57). Inhibition of degradation increases protein levels and target gene expression (58). The binding of ligands and coactivators can influence the turnover rates of PPARα and PPARγ (59, 60). Treatment with NR alone did not upregulate protein levels, indicating that RXR ligand is required in addition to PPAR ligand for protein upregulation. The increase in these proteins in the absence of concurrent upregulation of their transcripts suggests that NR and BC act together to stabilize protein levels through post-translational mechanisms.
Figure 3 NRBC upregulates a subset of key regulatory proteins without mRNA increases (A) PPARα; (B) PPARγ; (C) PGC-1α; and (D) NAMPT. Adipocytes from three to five donors with obesity were treated for seven days. Protein was measured by Western Blotting with β-actin used to adjust for loading. mRNA was quantified by real-time PCR. Data are expressed as least squares means ± standard error, *p < 0.001 NR, Naringenin; BC, beta carotene; NRBC, naringenin and beta carotene.
To expand our understanding of human adipocyte reprogramming by NRBC, we conducted whole transcriptome sequencing. Adipocyte cultures from two female donors with overweight and obesity were treated with cell medium (vehicle control) or NRBC for seven days and RNA samples were processed for library construction. Differential gene expression results were computed for 17525 genes of which 3881 genes were significantly regulated at an adjusted p-value <0.05. Pathway enrichment analysis identified PPAR signaling, adipocyte signaling and insulin signaling pathways to be the most significantly increased after treatment (Figure 4). In addition, the data analysis showed increases in genes for metabolism of pyruvate, fatty acids, glucose, and amino acids. These changes in mRNA levels were validated for selected genes by qRT-PCR (Figure 5, p<0001).
Figure 4 Whole transcriptome sequencing analysis of pathways stimulated by naringenin and β-carotene (NRBC) Adipocytes from two donors with BMI of 27 and 36 kg/m2 were treated with cell medium (vehicle control) or NRBC for seven days. cDNA libraries from expressed transcripts were constructed, sequenced and differential gene expression was analyzed. Gene-sets with false discovery rate (FDR) ≤ 5% were considered as significantly enriched, and the top pathways are shown.
Figure 5 RT-PCR validation of genes upregulated by naringenin and beta carotene (NRBC) in RNA sequencing analysis. Adipocytes from three donors with obesity were treated for seven days. mRNA levels were measured using quantitative RT-PCR. Data are expressed as mean ± standard error, *p < 0.001.
NRBC robustly upregulated a number of classical brown and beige genes that have previously been identified as targets of synthetic PPARα and PPARу ligands (10) including CIDEA, CITED, perilipins 2, -4, -5, PDK4, GK, AQP7, fatty acid elongases ELOVL 3, -5, -6, ACSL5 and the fatty acid binding proteins FABP3, FABP4 and FABP7 (Table 1). In addition, FABP5, which delivers retinoic acid to nuclear receptors was significantly upregulated.
Table 1 NRBC-induced genes associated with brown/beige phenotype previously shown as upregulated by synthetic PPARγ and PPARα activators.
Adipose tissue is an endocrine organ that secretes proteins, hormones, and bioactive lipids with beneficial paracrine effects on whole-body fat and glucose metabolism. NRBC treatment significantly upregulated the expression of a number of these genes including ANGPTL4, FNDC4 and GDF11 (Table 2). ANGPTL4 is produced by adipocytes and promotes lipolysis (Table 2) (61). Both the full-length protein and a truncated form of ANGPTL4 are secreted, and their overexpression in mice stimulates energy expenditure, lowers adiposity, and converts white fat to the beige phenotype (62). FNDC4 induces UCP1 and beige genes and promotes insulin sensitivity in adipocytes (63, 64). GDF11, a circulating cytokine in the transforming growth factor β superfamily, declines with age and has been under investigation as an anti- aging therapeutic (65). Restoration of circulating levels in aged mice promotes adiponectin secretion by fat tissues and reduces adiposity (66).
Hydroxyeicosatetraenoic acids (HETEs) and dihydroxyoctadecanoic acids (diHOMEs) are secreted bioactive lipids that are produced from cytochrome P450 metabolites in brown adipocytes after cold exposure and exercise (67, 68). CYP4F11, a cytochrome that produces 20-HETE from arachidonic acid (69), was abundantly increased by NRBC. Circulating levels of 20-HETE correlate with elevated energy expenditure after cold exposure in people with detectable levels of BAT (70), and it is a PPARα activator (71). In addition, NRBC induced epoxide hydrolases EPHX1 and EPHX2, enzymes that utilize HETEs to produce 12,13-diHOME, a lipokine associated with improved insulin sensitivity and reduced triglycerides after exercise (72, 73).
Several circulating CNS-acting proteins were increased by NRBC. Neuromedin B is a peptide that acts on hypothalamic neurons to promote satiety, and a missense mutation is linked to hyperphagia and obesity in genetic studies (74, 75). Pro-opiomelanocortin (POMC), a peptide prohormone that is cleaved by PCSK1 into the hormone α-MSH, is involved in the suppression of food intake (76). POMC and PCSK1 were both elevated by NRBC in adipocytes. The important role of POMC is shown in studies linking severe obesity in humans to rare genetic mutations in POMC or PCSK1 (77). S100b, which has been characterized in brown adipocytes of mice, stimulates neurite outgrowth of sympathetic terminals in adipose tissue following cold exposure (78). The upregulation of genes for CNS-acting circulating factors suggests that appetite reduction could play a role in the physiological response to NRBC.
Several mechanisms other than uncoupling of mitochondria by UCP1 can yield thermogenic energy expenditure. These mechanisms were originally identified in studies of brown adipose tissue (BAT) from cold exposed Ucp1-knockout mice that are able to maintain their body temperature (79). We found that NRBC induced significant increases in a number of novel thermogenesis genes in human adipocytes (Table 3). RXRγ, a retinoic acid receptor isoform of unknown function, was highly upregulated. This isoform is localized in UCP1 positive cells in human adipose tissues and is expressed at elevated basal levels in adipose-derived stem cells that subsequently differentiate into UCP1-expressing brown adipocytes (80). These observations suggest that RXRγ may be a key transcription factor in the differentiation of human brown and beige adipocytes.
PM20D1 levels were upregulated approximately 15-fold in adipocytes treated with NRBC, and human genetic studies have shown that the PM20D1 gene promoter has a PPRE (81). PM20D1 is a secreted enzyme that regulates synthesis and degradation of N-acyl amino acids (NAAs), molecules that directly uncouple mitochondria and increase energy expenditure (82). High PM20D1 levels in the white adipose tissue of mice correlates with increased respiration, reversal of high fat diet-induced obesity, and reductions in blood glucose (82–84). Creatine phosphate cycling is an enzymatic pathway that contributes to thermogenesis in Ucp1-knockout mice (85). The synthesis and breakdown of creatine phosphate by the mitochondrial creatine kinases CKMT1A, CKMT1B and CKMT2 releases heat and consumes ATP. We found strong upregulation of the genes for all three CKMT isozymes after NRBC treatment of white adipocytes. In humans, CKMT proteins have previously only been detected in primary brown adipocytes (86).
Beige cells favor utilization of fatty acids rather than glucose to fuel thermogenesis and have high lipase activity (87). In addition to ATGL, hormone sensitive lipase (HSL) and protein kinase A regulatory subunit 2A (PRKAR2B) were upregulated by NRBC treatment (Table 3). PRKAR2B is the key PKA subunit regulating activation of lipolysis subsequent to ligand binding of Gs-coupled receptors (88). NRBC significantly upregulated genes for futile cycling of triglycerides, PDK4, GPD1, GK and PCK1. The activities of these enzymes result in shuttling of glycolytic intermediates into pyruvate for the synthesis of glycerol and glycerol-3-phosphate, the precursors for re-esterification of fatty acids during TG synthesis (10). NRBC also stimulated increases in AIFM2, an NADH oxidase (AIFM2) that supports glucose metabolism during thermogenesis in brown adipocytes (89), and UCP2. Elevated UCP2 levels are associated with cells that have high fatty acid oxidation rates, and evidence suggests that UCP2 facilitates fatty acid oxidation to fuel mitochondrial thermogenesis (90–92).
Nicotinamide phosphoribosyltransferase (NAMPT) and nicotinic acid phosphoribosyltransferase (NAPRT) encode enzymes that produce nicotine adenine dinucleotide (NAD) and are upregulated over two-fold. NAD is a key cofactor for cellular metabolism enzymes. Impaired NAD synthesis in adipocytes causes systemic insulin resistance and suppresses lipolysis and thermogenesis in mice (93, 94). In human adipocytes, increasing intracellular NAD induces UCP1 and mitochondrial biogenesis, which are markers of beige cells (95). In summary, these changes in thermogenesis genes suggest that NRBC-treated adipocytes have a higher capacity for uncoupled respiration and thermogenic futile cycling pathways.
There is little comprehensive data available on relative receptor levels or hormone-stimulated lipolysis in human adipocytes. Based on our RNA sequencing data, receptor abundance was estimated from transcript reads per total number of kilobases sequenced (Figures 6A, B). NRBC treatment significantly increased expression of eight receptors potentially capable of driving lipolysis or thermogenesis through various mechanisms including the β1AR, bile acid receptor TGR5, cold receptor TRPM8, adenosine receptor ADORA1, NPR1, G-protein coupled estrogen receptor-1 (GPER1), growth hormone receptor (GHR) and PTHR1 (Padj<0.002). In addition, there were increases in β2AR, β3AR, and melanocortin-1 receptor (MC1R) that did not achieve statistical significance due to variability in the response. The β2AR and β3AR had the lowest expression levels compared to all other receptors in white adipocytes. The β1AR was approximately four times more abundant than the β2AR and β3AR and increased another three-fold after NRBC exposure (Figure 6A). TGR5, TRPM8 and ADORA1 and ADORA2 were also expressed at low levels in untreated white adipocytes.
Figure 6 Relative receptor levels in white adipocytes (untreated) and NRBC-treated cells. (A) Low abundance (B) High abundance. Adipocytes from two donors with obesity were treated with cell medium (Control) or NRBC for seven days and transcript sequencing analysis was conducted. Data are expressed as mean normalized transcript counts (DEseq2). * indicates Padj< 0.002 for Control vs NRBC. β-adrenergic receptors (β1AR, β2AR, β3AR), G-protein coupled bile acid receptor (TGR5), Transient receptor potential cation channel subfamily M member 8 (TRPM8), Melanocortin-1 receptor (MC1R), Adenosine receptors A1 and A2B (ADORA1, ADORA2B), Natriuretic peptide receptors (NPR1, NPR3), G-protein coupled estrogen receptor 1 (GPER1), Parathyroid hormone receptor 1 (PTHR1), Growth hormone receptor (GHR).
The ratio of stimulatory NPR1 to the clearance receptor NPR3 determines the magnitude of the response to natriuretic peptides. NRBC treatment increased the NPR1/NPR3 ratio four-fold in NRBC- treated adipocytes, suggesting that adipocytes would be more responsive to natriuretic peptides (Figure 6B). PTHR levels were upregulated over fifty percent by NRBC (Figure 6B). GHR was the most abundant of all receptors upregulated by NRBC, and studies suggest that GH triggers lipolysis by unique mechanisms that occur downstream of receptor signaling in human adipocytes (41, 96). GPER1 is abundantly expressed in adipocytes and estradiol is the endogenous ligand. Little is known about the role of GPER1 in white adipocytes (97). A selective synthetic agonist for GPER stimulates weight loss and energy expenditure in mice (98).
Thermogenesis is fueled by lipolysis, so we determined whether agonist-stimulated glycerol release, a measure of lipolysis, reflected the increases in receptors and lipolysis machinery in NRBC-treated adipocytes. First, adipocyte cultures from four donors who had obesity were treated with either cell medium (untreated control cells) or NRBC for seven days. On the day of the acute lipolysis assay, cells were exposed to the individual receptor agonists for four hours in buffer and glycerol released into the cell supernatant was measured. Non-hydrolyzable 8-cpt-cAMP, a potent activator of PKA signaling, was also evaluated in each experiment as a positive control since it bypasses individual receptors and measures the maximum capacity for lipolysis (36). The βARs, PTHR, MC1R, GPER and ADORA2B are all stimulatory G-protein (Gs) coupled receptors and signal through the cAMP-PKA signal transduction mechanism. NPR1 is not a Gs-coupled receptor and signals through an alternate mechanism of guanylate cyclase-cGMP-protein kinase G (PKG).
Of all the hormones tested, PTH- stimulated lipolysis was the highest in control white adipocytes and increased by the greatest magnitude in cultures exposed to NRBC (Figure 7). PTH treatment of human adipocytes was previously shown to stimulate UCP1 expression and oxygen consumption (99). We tested atrial natriuretic peptide (ANP), one of the key endogenous ligands for NPR1 released from atrial myocytes in response to increases in blood pressure and environmental stimuli such as exercise and cold exposure (100, 101). ANP-stimulated lipolysis increased in NRBC-treated cells, but the overall effect was not statistically significant in adipocytes from four donors.
Figure 7 Hormone-stimulated lipolysis in white adipocytes (untreated) and NRBC-pretreated cells. After 7d pretreatment with vehicle (untreated) or NRBC, adipocytes were exposed for 4 hours to receptor agonists in KRB buffer. Supernatants were removed for measurement of glycerol. Data are presented as least squares mean ± standard error from experiments using cells from four different donors with BMIs ranging from 27 to 36, each with at least 6 replicates. (*indicates a difference between untreated white adipocytes and NRBC-treated adipocytes p≤ 0.02) cAMP 8-Cpt-cAMP 200µM, ANP atrial natriuretic peptide 0.1 µM, PTH parathyroid hormone (1-34) 1 µM, isoprot isoproterenol 1µM, dobutam dobutamine 1µM, estradiol 1µM, GH growth hormone 250ng/ml, ACTH adrenocorticotropin hormone 1µM, CDCA chenodeoxycholic acid 30µM, adenosine 1µM, menthol 100µM.
The collective activity of the βARs was measured using isoproterenol, a non-selective agonist of all three βARs, to evaluate the overall potential for increased sensitivity to sympathetic stimulation. Since the β1AR was 5-fold more abundant than the other βARs, the β1AR-selective ligand dobutamine was also tested. Isoproterenol- and dobutamine- stimulated lipolysis were significantly boosted in NRBC-treated cells, and dobutamine activity was comparable with isoproterenol activity (Figure 7). In NRBC-treated adipocytes, lipolysis stimulated by 8-cpt-cAMP was significantly higher than levels in control cells, showing that maximum capacity for lipolysis was elevated in parallel with the upregulation of the PKA regulatory subunit and lipases.
Agonists for the receptors GHR, MC1R, ADORA1/ADORA2B, TGR5 and TRPM8 did not stimulate detectable increases in lipolysis. In murine white and brown adipocytes adrenocorticotropic hormone (ACTH) and adenosine, endogenous ligands for melanocortin receptors and ADORA2B respectively, stimulate lipolysis and OCR (42, 44, 102). Bile acids activate TGR5 in human brown adipocytes and increase OCR (43). Although GPER activation by estrogen stimulates cAMP production in cancer cells (36), our data showed a small reduction in lipolysis.
NRBC induced a strong increase in RXRγ mRNA after treatment and RXRα was unchanged. RXRγ is a unique isoform associated with differentiation of brown adipocytes in human adipose tissue (80). RXRs can form homodimers or heterodimers with multiple nuclear receptors other than PPARγ, so we evaluated whether RXRγ was bound to PPARγ after NRBC treatment. PPARγ was immunoprecipitated from protein lysates of untreated and NRBC- treated adipocytes. The protein complexes pulled down with PPARγ were analyzed on Western Blots (Figure 8). RXRγ protein expression was too low for detection on regular Western Blots of whole cell protein, consistent with our transcriptome sequencing data indicating that it has a low copy number. However, we observed that RXRγ was visible in the immunoprecipitated PPARγ protein complex in control cells (white adipocytes) and in NRBC treated beige adipocytes (Figure 8). RXRα is more abundantly expressed and was readily detected in Western Blots and in PPARγ immunoprecipitates. These results suggest that both isoforms are bound constitutively and that binding of RXRу is independent of the addition of exogenous ligands. RXR isoforms stabilize PPARγ complexes at PPRE promoter elements and direct expression of specific target genes subsequent to ligand binding (46). Therefore, elevated levels of RXRγ may direct expression towards beige genes after the addition of BC.
Figure 8 Analysis of RXR isoforms bound to immunoprecipitated PPARγ complexes. Adipocytes were treated with vehicle or NRBC for seven days. RXRγ, RXRα and PPARγ protein levels were analyzed by Western blotting. For analysis of whole cell protein levels (input), 50 μg of protein lysate was loaded in each lane. PPARγ was immunoprecipitated from 600 μg of the same whole cell lysate for analysis of PPARγ cofactor binding. An anti-IgGκ light chain secondary antibody was used for detection. This experiment was repeated three times with adipocytes from obese donors.
Thermogenesis is a fundamental component of energy balance. A number of studies have demonstrated that human adipocytes have the functional plasticity to be transformed into thermogenic cells by non-adrenergic stimuli, making adipose tissue a relevant peripheral target tissue for obesity drugs (10, 37, 45) (99). In this report we investigated the potential of carotenoids to intensify the thermogenic response of human adipocytes to NR, a natural activator of PPARα and PPARγ. Pro-vitamin A carotenoids are converted into ligands for RXR nuclear receptors that form heterodimers with PPARs and coordinate expression of metabolism genes. We evaluated adipocytes treated for seven days with NR and carotenoids at concentrations that are reached in serum after oral administration to humans. The two pro-vitamin A carotenoids, BC and lutein, synergistically enhanced levels of UCP1 compared to NR alone. Lycopene, the third carotenoid that we investigated is not converted into an RXR ligand and did not alter the NR response. We used BC for additional experiments and found that NRBC also enhanced expression of ATGL, GLUT4, and adiponectin, which are drivers of lipolysis and insulin sensitivity. The effect of BC was selective. Expression of the PPARα target gene CPT1β, and PM20D1, a PPARγ regulated gene, were not elevated compared to treatment with NR alone.
NRBC upregulated protein levels of PGC-1α, PPARγ, and PPARα without comparable increases in mRNA levels, suggesting that the mechanism does not involve enhanced translation. Pgc-1α is a cold- induced coactivator for PPARγ and PPARα with a short protein half-life, and its transcriptional activity is upregulated in mice by mechanisms that slow degradation (58). When its protein levels increase, Pgc-1α protein associates with the PPARγ/RXR complex and selectively promotes expression of UCP1 and mitochondrial proteins (103). Elevated PGC-1α protein drives the PPARα/RXR complex towards GK expression and TG cycling activity in human adipocytes (104). The half-life of PPARγ and PPARα proteins is regulated by control of degradation rate after binding of ligands and cofactors (56, 57, 59). Since treatment with NR alone did not upregulate protein levels, our data suggest that NR and BC are both required to stabilize PPAR protein levels.
Brown and beige adipocytes release specialized bioactive lipokines and proteins into circulation that activate whole body insulin sensitivity and fatty acid uptake. Transcriptome sequencing showed that NRBC treatment substantially upregulated Cyp4f11, EPDX1 and EPDX2, enzymes that produce HETES and di-HOMEs (69, 72). Secreted HETES and di-HOMEs can signal tissues to increase uptake of fatty acids, and these actions have beneficial effects on lowering blood lipids (73). HETEs are strong PPAR activators (71), and when combined with the increased levels of PPAR proteins observed after NRBC treatment could amplify PPAR target gene expression. NRBC also induced ANGPTL4, a secreted protein that stimulates adipocyte lipolysis, and the insulin sensitizers adiponectin, FNDC4, and GDF11.
ATGL is the rate-limiting lipase for release of fatty acids from TGs, and fatty acids are natural ligands of PPARα and PPARу. In metabolically active beige cells, fatty acids are shuttled into mitochondria to fuel thermogenesis (87). In an energy-wasting futile cycle, fatty acids are also re-esterified onto the glycerol backbone of TGs. Whole transcriptome sequencing showed that NRBC stimulated a number of genes for glyceroneogenesis and TG synthesis, including PDK4, PCK1, GK, and GPD1. When thermogenesis is activated in adipocytes, PDK4 directs the flow of pyruvate generated by glycolysis into glycerol production (105). The PCK1 gene encodes PEPCK, the rate-limiting enzyme for glycerol synthesis from pyruvate. Studies using radio-labelled pyruvate in human adipocytes showed incorporation of the label into the glycerol backbone and into TGs after induction of PCK1 or PDK4 in human adipocytes (10, 106). In addition, GK and GPD1 encode enzymes that produce glycerol-3-phosphate, the substrate for fatty acid esterification (107, 108). The net result of TG cycling is elevated energy expenditure and a decrease of free fatty acids released into circulation (105).
In addition to UCP1 and TG cycling, we found that NRBC stimulated multiple other uncoupling and ATP-consuming enzymatic pathways. The most highly upregulated gene was PM20D1, encoding an enzyme that reversibly synthesizes NAAs that have potent mitochondrial uncoupling activity (109). In humans, the functional phenotype of PM20D1 is not well defined. In a clinical trial, serum concentrations of PM20D1 were positively correlated with adiposity and biomarkers of glucose metabolism such as glycated hemoglobin and fasting blood glucose (110). Both PM20D1 and NAAs are bound to serum proteins and regulation of circulating levels of NAAs in humans is complex (111). The relationship between adipose tissue PM20D1 and body weight in humans is unclear. Mutations in upstream PPARγ-binding regulatory elements of the PM20D1 gene cause a wide variation in basal expression levels in adipocytes. However, basal levels of PM20D1 in human adipose tissue do not correlate with circulating NAA levels (81). Presently, no clinical studies have been conducted to determine whether a substantial increase PM20D1 expression in thermogenic beige adipose tissue is sufficient to cause an increase in circulating NAA levels and contribute to weight loss.
The mitochondrial creatine kinases CKMT1A, CKMT1B and CKMT2 were highly induced by NRBC. Functional studies in mice showed that creatine kinases are upregulated in brown adipocytes after cold exposure or in the absence of Ucp1 and contribute to whole body energy expenditure (85). Interestingly, ablation of creatine metabolism in white adipose tissues inhibits thermogenesis and drives obesity in mice (112). In primary human brown adipocytes, proteomic analysis of thermogenesis pathways showed that ATP-coupled respiration is stimulated in parallel to uncoupled respiration and contributes half of the total oxygen consumed (86). Cycling of creatine phosphate supports ATP-coupled mitochondrial respiration by increasing the availability of ADP and phosphate to ATP-synthase. The strong induction of creatine kinases by NRBC suggests that ATP-coupled respiration and creatine phosphate cycling could contribute to thermogenesis in human beige adipocytes.
We showed that the enzymes involved in maintaining intracellular NAD levels, including AIFM2, NAMPT and NAPRT, increased after NRBC exposure. AIFM2 converts NADH to NAD to support the high levels required for glycolysis (89). NAMPT and NAPRT both synthesize NAD from intracellular precursors to support cellular needs during conditions of increased metabolic rate and oxidative stress (113). In addition, NAD is a cofactor for the sirtuin enzymes (SIRTs), which are protein deacetylases that regulate the activity of PPARγ, PGC-1α and other transcriptional activators of mitochondrial biogenesis and metabolism genes (114). Increasing NAD levels in human adipocytes can shift the phenotype from white to beige (95).
Our quantitative RNAseq analysis showed that the RXRα transcript is 300-fold more abundant than RXRγ in human white adipocytes, however, only the RXRγ isoform was robustly upregulated by NRBC treatment. We found that both RXRγ and RXRα proteins were bound in immunoprecipitated PPARγ complexes from untreated white adipocytes and in NRBC-treated beige cells. These results indicate that binding of both isoforms to the PPARγ complex is constitutive and ligand independent. In human perirenal adipose tissue, RXRγ expression is enriched in brown adipocyte progenitors and is induced in parallel with UCP1 during conversion of white to beige adipocytes (80). Possible mechanisms for targeting of beige genes could be recruitment of specific coactivators by RXRγ or conformational changes stimulated by BC binding that facilitate and stabilize interaction of this isoform with specific promoter elements (115) (46). In addition, the increase in RXRγ, PGC1α, PPARα and PPARγ proteins after NRBC treatment suggests the existence of a positive feedback loop that upregulates the genes identified in this study.
We used transcriptome sequencing data to estimate relative expression levels of receptors known to activate lipolysis or thermogenesis in adipocytes. In cells treated with NRBC, eight receptors were upregulated and several others trended higher. We evaluated stimulation of lipolysis with agonists for all receptors altered by NRBC and found that only a small subset were lipolytic. ACTH and adenosine stimulate lipolysis in murine white adipocytes and we saw no effect with either (44, 102). TGR5 and TRPM8 agonists induce thermogenesis genes in human adipocytes but did not stimulate lipolysis in our assay. We and others have shown that mild cold exposure of subcutaneous human adipocytes activates UCP1 expression by TRPM8, so the large increase in receptor levels after NRBC treatment has potential to act locally to sensitize adipocytes to cold and stimulate thermogenesis (45, 116). GHR and GPER1 were induced to the highest levels of all receptors after NRBC exposure, but neither growth hormone nor estrogen stimulated lipolysis.
NRBC upregulated PTHR levels. Moreover, PTH stimulated the greatest magnitude of lipolysis of all hormones tested in NRBC pretreated cells. PTH is released from parathyroid glands for regulation of systemic calcium homeostasis and all tissues express the PTHR (117). Evidence is growing that PTH plays an important role in adipose tissue metabolism. PTH stimulates lipolysis in mouse adipocytes and thermogenic gene expression in human adipocytes (99, 118). Cold-induced increases in circulating PTH shift whole-body metabolism toward lipid utilization to fuel energy expenditure in swimmers (119).
NRBC increased the stimulatory ratio of NPR1/NPR3 receptors. ANP is released after cold exposure and exercise and acts additively with adrenergic agonists to stimulate lipolysis and brown adipocyte characteristics in human white adipocytes (37). Although the increase was not statistically significant, our data showed a trend toward increased ANP-activated lipolysis in NRBC-treated cells.
We observed that the β1AR expression level in human adipocytes was substantially higher than the other βARs, and the β1AR was induced an additional three-fold by NRBC. We tested glycerol release with the pan-βAR agonist isoproterenol and the β1AR-selective agonist dobutamine and found that both stimulated a similar increase in NRBC-exposed cells compared to untreated. These results are in line with other studies showing that the β1AR is the dominant subtype in human white and brown adipocytes (39, 120).
NRBC significantly increased expression of PRKAR2B, the key PKA regulatory subunit linked to insulin sensitivity and resistance to weight gain in humans (88). We used 8-cpt-cAMP to activate PKA downstream of receptors and observed a considerable increase in the maximum capacity for lipolysis in adipocytes pretreated with NRBC. Impaired cAMP-stimulated TG lipolysis in subcutaneous adipose tissue is a characteristic of obesity and insulin resistance (121). A comprehensive analysis of two female cohorts with a ten-year follow-up was conducted to determine adipose tissue characteristics that predict weight gain. Low levels of stimulated lipolysis and PRKAR2B expression predicted weight gain and impaired glucose metabolism (122). Interestingly, there was no correlation with basal lipolysis or fat oxidation.
The human response to cold exposure and exercise involves transient release of ANP and PTH into circulation and sympathetic release of catecholamines in adipose tissues (100, 119) (37, 39, 99). Our data suggest that adipose tissue in an individual consuming NRBC would be more responsive to circulating hormones released after these stimuli. We demonstrate in this report that NRBC reprograms adipocytes by upregulating multiple thermogenic pathways, beneficial secreted factors, receptors and lipolysis, summarized in Figure 9. NR and BC have a good safety profiles and have the potential to be administered long-term without adverse effects (27, 29). A randomized, double blinded placebo-controlled clinical trial will be needed to determine whether the effects of NRBC on adipocytes will translate into weight loss and improvements in insulin sensitivity.
Figure 9 Proposed paradigm for remodeling of white adipocytes by NRBC. NR and BC bind nuclear receptors and activate gene expression at PPRE motifs. UCP1 and other uncoupling compounds and mitochondrial proteins mediate multiple energy-wasting enzymatic cycles that generate heat. PM20D1 regulates synthesis and degradation of N-acyl amino acids (NAA), molecules that directly uncouple mitochondria and increase energy expenditure. The synthesis and breakdown of creatine phosphate by the mitochondrial creatine kinases CKMT1A, CKMT1B and CKMT2 facilitates ATP-coupled respiration and enhances oxygen consumption. PDK4 directs pyruvate into synthesis of glycerol, fatty acids, and TGs to promote futile TG recycling. Lipolytic receptors and PKA are upregulated, increasing responsiveness to hormones and lipolysis. Fatty acids are transferred into mitochondria to fuel thermogenesis. Genes are turned on for production of bioactive peptides and lipokines which have autocrine insulin sensitizing effects and are secreted into circulation. NR (Naringenin), BC (β-carotene) Created with Biorender.com.
The datasets presented in this study can be found in online repositories. The names of the repository/repositories and accession number(s) can be found below: https://www.ncbi.nlm.nih.gov/geo/, GSE223313.
Conceptualization, FG, AC and CR. Methodology: FG, AC, LC and CR. Formal analysis: AC, DZ, SG. Conducted research: AC, CC, SJ, CR, SG. Writing—original draft preparation: AC. Writing—review and editing: CR, YH, FG, CC. All authors contributed to the article and approved the submitted version.
This work was supported in part by grants from the Brown Foundation of Louisiana, the National Institute on Aging of the National Institutes of Health (R00AG065419), and in part in part by U54 GM104940 from the National Institute of General Medical Sciences of the National Institutes of Health, which funds the Louisiana Clinical and Translational Science Center. The content is solely the responsibility of the authors and does not necessarily represent the official views of the Brown Foundation or the National Institutes of Health.
The authors declare that the research was conducted in the absence of any commercial or financial relationships that could be construed as a potential conflict of interest.
All claims expressed in this article are solely those of the authors and do not necessarily represent those of their affiliated organizations, or those of the publisher, the editors and the reviewers. Any product that may be evaluated in this article, or claim that may be made by its manufacturer, is not guaranteed or endorsed by the publisher.
The Supplementary Material for this article can be found online at: https://www.frontiersin.org/articles/10.3389/fendo.2023.1148954/full#supplementary-material
1. Kajimura S, Spiegelman BM, Seale P. Brown and beige fat: Physiological roles beyond heat generation. Cell Metab (2015) 22(4):546–59. doi: 10.1016/j.cmet.2015.09.007
2. Guerra C, Koza RA, Yamashita H, Walsh K, Kozak LP. Emergence of brown adipocytes in white fat in mice is under genetic control. effects on body weight and adiposity. J Clin Invest (1998) 102(2):412–20.
3. Kopecky J, Clarke G, Enerback S, Spiegelman B, Kozak LP. Expression of the mitochondrial uncoupling protein gene from the aP2 gene promoter prevents genetic obesity. J Clin Invest (1995) 96(6):2914–23. doi: 10.1172/JCI118363
4. Vallerand AL, Perusse F, Bukowiecki LJ. Stimulatory effects of cold exposure and cold acclimation on glucose uptake in rat peripheral tissues. Am J Physiol (1990) 259(5 Pt 2):R1043–9. doi: 10.1152/ajpregu.1990.259.5.R1043
5. Barbatelli G, Murano I, Madsen L, Hao Q, Jimenez M, Kristiansen K, et al. The emergence of cold-induced brown adipocytes in mouse white fat depots is determined predominantly by white to brown adipocyte transdifferentiation. Am J Physiol Endocrinol Metab (2010) 298(6):E1244–53. doi: 10.1152/ajpendo.00600.2009
6. Himms-Hagen J, Cui J, Danforth E Jr., Taatjes DJ, Lang SS, Waters BL, et al. Effect of CL-316,243, a thermogenic beta 3-agonist, on energy balance and brown and white adipose tissues in rats. Am J Physiol (1994) 266(4 Pt 2):R1371–82. doi: 10.1152/ajpregu.1994.266.4.R1371
7. Cypess AM, Chen YC, Sze C, Wang K, English J, Chan O, et al. Cold but not sympathomimetics activates human brown adipose tissue in vivo. Proc Natl Acad Sci U.S.A. (2012) 109(25):10001–5. doi: 10.1073/pnas.1207911109
8. Finlin BS, Memetimin H, Zhu B, Confides AL, Vekaria HJ, El Khouli RH, et al. The beta3-adrenergic receptor agonist mirabegron improves glucose homeostasis in obese humans. J Clin Invest (2020) 130(5):2319–31. doi: 10.1172/JCI134892
9. Vosselman MJ, van der Lans AA, Brans B, Wierts R, van Baak MA, Schrauwen P, et al. Systemic beta-adrenergic stimulation of thermogenesis is not accompanied by brown adipose tissue activity in humans. Diabetes (2012) 61(12):3106–13. doi: 10.2337/db12-0288
10. Barquissau V, Beuzelin D, Pisani DF, Beranger GE, Mairal A, Montagner A, et al. White-to-brite conversion in human adipocytes promotes metabolic reprogramming towards fatty acid anabolic and catabolic pathways. Mol Metab (2016) 5(5):352–65. doi: 10.1016/j.molmet.2016.03.002
11. Loft A, Forss I, Siersbaek MS, Schmidt SF, Larsen AS, Madsen JG, et al. Browning of human adipocytes requires KLF11 and reprogramming of PPARgamma superenhancers. Genes Dev (2015) 29(1):7–22. doi: 10.1101/gad.250829.114
12. Kaupang A, Hansen TV. The PPAR omega pocket: Renewed opportunities for drug development. PPAR Res (2020) 2020:9657380. doi: 10.1155/2020/9657380
13. Tan Y, Muise ES, Dai H, Raubertas R, Wong KK, Thompson GM, et al. Novel transcriptome profiling analyses demonstrate that selective peroxisome proliferator-activated receptor gamma (PPARgamma) modulators display attenuated and selective gene regulatory activity in comparison with PPARgamma full agonists. Mol Pharmacol (2012) 82(1):68–79. doi: 10.1124/mol.111.076679
14. Benvenuti S, Cellai I, Luciani P, Deledda C, Baglioni S, Giuliani C, et al. Rosiglitazone stimulates adipogenesis and decreases osteoblastogenesis in human mesenchymal stem cells. J Endocrinol Invest (2007) 30(9):RC26–30. doi: 10.1007/BF03350807
15. Soccio RE, Chen ER, Lazar MA. Thiazolidinediones and the promise of insulin sensitization in type 2 diabetes. Cell Metab (2014) 20(4):573–91. doi: 10.1016/j.cmet.2014.08.005
16. Ribet C, Montastier E, Valle C, Bezaire V, Mazzucotelli A, Mairal A, et al. Peroxisome proliferator-activated receptor-alpha control of lipid and glucose metabolism in human white adipocytes. Endocrinology (2010) 151(1):123–33.
18. FDA. Tricor fenofibrate. Available at: https://www.accessdata.fda.gov/drugsatfda_docs/label/2010/021656s019lbl.pdf.
19. Cheng HS, Tan WR, Low ZS, Marvalim C, Lee JYH, Tan NS. Exploration and development of PPAR modulators in health and disease: An update of clinical evidence. Int J Mol Sci (2019) 20(20).
20. Boeckmans J, Natale A, Rombaut M, Buyl K, Rogiers V, De Kock J, et al. Anti-NASH drug development hitches a lift on PPAR agonism. Cells (2019) 9(1).
21. Steele H, Gomez-Duran A, Pyle A, Hopton S, Newman J, Stefanetti RJ, et al. Metabolic effects of bezafibrate in mitochondrial disease. EMBO Mol Med (2020) 12(3):e11589.
22. Choi JH, Banks AS, Estall JL, Kajimura S, Bostrom P, Laznik D, et al. Anti-diabetic drugs inhibit obesity-linked phosphorylation of PPARgamma by Cdk5. Nature (2010) 466(7305):451–6. doi: 10.1038/nature09291
23. Zhang F, Lavan BE, Gregoire FM. Selective modulators of PPAR-gamma activity: Molecular aspects related to obesity and side-effects. PPAR Res (2007) 2007:32696. doi: 10.1155/2007/32696
24. Goldwasser J, Cohen PY, Yang E, Balaguer P, Yarmush ML, Nahmias Y. Transcriptional regulation of human and rat hepatic lipid metabolism by the grapefruit flavonoid naringenin: role of PPARalpha, PPARgamma and LXRalpha. PloS One (2010) 5(8):e12399. doi: 10.1371/journal.pone.0012399
25. Rebello CJ, Greenway FL, Lau FH, Lin Y, Stephens JM, Johnson WD, et al. Naringenin promotes thermogenic gene expression in human white adipose tissue. Obes (Silver Spring) (2019) 27(1):103–11. doi: 10.1002/oby.22352
26. Murugesan N, Woodard K, Ramaraju R, Greenway FL, Coulter AA, Rebello CJ. Naringenin increases insulin sensitivity and metabolic rate: A case study. J Med Food (2020) 23(3):343–8. doi: 10.1089/jmf.2019.0216
27. Rebello CJ, Beyl RA, Lertora JJL, Greenway FL, Ravussin E, Ribnicky DM, et al. Safety and pharmacokinetics of naringenin: A randomized, controlled, single-ascending-dose clinical trial. Diabetes Obes Metab (2020) 22(1):91–8. doi: 10.1111/dom.13868
28. Canas JA. Mixed carotenoid supplementation and dysmetabolic obesity: gaps in knowledge. Int J Food Sci Nutr (2021) 72(5):653–9. doi: 10.1080/09637486.2020.1852193
29. Canas JA, Lochrie A, McGowan AG, Hossain J, Schettino C, Balagopal PB. Effects of mixed carotenoids on adipokines and abdominal adiposity in children: A pilot study. J Clin Endocrinol Metab (2017) 102(6):1983–90. doi: 10.1210/jc.2017-00185
30. Coulter AA, Rebello CJ, Greenway FL. Centrally acting agents for obesity: Past, present, and future. Drugs (2018) 78(11):1113–32. doi: 10.1007/s40265-018-0946-y
31. Li J, Curley JL, Floyd ZE, Wu X, Halvorsen YDC, Gimble JM. Isolation of human adipose-derived stem cells from lipoaspirates. Methods Mol Biol (2018) 1773:155–65. doi: 10.1007/978-1-4939-7799-4_13
32. Subramanian A, Tamayo P, Mootha VK, Mukherjee S, Ebert BL, Gillette MA, et al. Gene set enrichment analysis: a knowledge-based approach for interpreting genome-wide expression profiles. Proc Natl Acad Sci U.S.A. (2005) 102(43):15545–50. doi: 10.1073/pnas.0506580102
33. Ogata H, Goto S, Sato K, Fujibuchi W, Bono H, Kanehisa M. KEGG: Kyoto encyclopedia of genes and genomes. Nucleic Acids Res (1999) 27(1):29–34. doi: 10.1093/nar/27.1.29
34. Liberzon A, Birger C, Thorvaldsdottir H, Ghandi M, Mesirov JP, Tamayo P. The molecular signatures database (MSigDB) hallmark gene set collection. Cell Syst (2015) 1(6):417–25. doi: 10.1016/j.cels.2015.12.004
35. Reiner A, Yekutieli D, Benjamini Y. Identifying differentially expressed genes using false discovery rate controlling procedures. Bioinformatics (2003) 19(3):368–75. doi: 10.1093/bioinformatics/btf877
36. Gettys TW, Blackmore PF, Corbin JD. An assessment of phosphodiesterase activity in situ after treatment of hepatocytes with hormones. Am J Physiol (1988) 254(4 Pt 1):E449–53. doi: 10.1152/ajpendo.1988.254.4.E449
37. Bordicchia M, Liu D, Amri EZ, Ailhaud G, Dessi-Fulgheri P, Zhang C, et al. Cardiac natriuretic peptides act via p38 MAPK to induce the brown fat thermogenic program in mouse and human adipocytes. J Clin Invest (2012) 122(3):1022–36. doi: 10.1172/JCI59701
38. Hoare SR, Gardella TJ, Usdin TB. Evaluating the signal transduction mechanism of the parathyroid hormone 1 receptor. effect of receptor-g-protein interaction on the ligand binding mechanism and receptor conformation. J Biol Chem (2001) 276(11):7741–53. doi: 10.1074/jbc.M009395200
39. Riis-Vestergaard MJ, Richelsen B, Bruun JM, Li W, Hansen JB, Pedersen SB. Beta-1 and not beta-3 adrenergic receptors may be the primary regulator of human brown adipocyte metabolism. J Clin Endocrinol Metab (2020) 105(4):e994–1005. doi: 10.1210/clinem/dgz298
40. Filardo EJ, Quinn JA, Frackelton AR Jr., Bland KI. Estrogen action via the G protein-coupled receptor, GPR30: stimulation of adenylyl cyclase and cAMP-mediated attenuation of the epidermal growth factor receptor-to-MAPK signaling axis. Mol Endocrinol (2002) 16(1):70–84. doi: 10.1210/mend.16.1.0758
41. Sharma VM, Vestergaard ET, Jessen N, Kolind-Thomsen P, Nellemann B, Nielsen TS, et al. Growth hormone acts along the PPARgamma-FSP27 axis to stimulate lipolysis in human adipocytes. Am J Physiol Endocrinol Metab (2019) 316(1):E34–42. doi: 10.1152/ajpendo.00129.2018
42. Schnabl K, Westermeier J, Li Y, Klingenspor M. Opposing actions of adrenocorticotropic hormone and glucocorticoids on UCP1-mediated respiration in brown adipocytes. Front Physiol (2018) 9:1931. doi: 10.3389/fphys.2018.01931
43. Broeders EP, Nascimento EB, Havekes B, Brans B, Roumans KH, Tailleux A, et al. The bile acid chenodeoxycholic acid increases human brown adipose tissue activity. Cell Metab (2015) 22(3):418–26. doi: 10.1016/j.cmet.2015.07.002
44. Gnad T, Scheibler S, von Kugelgen I, Scheele C, Kilic A, Glode A, et al. Adenosine activates brown adipose tissue and recruits beige adipocytes via A2A receptors. Nature (2014) 516(7531):395–9. doi: 10.1038/nature13816
45. Rossato M, Granzotto M, Macchi V, Porzionato A, Petrelli L, Calcagno A, et al. Human white adipocytes express the cold receptor TRPM8 which activation induces UCP1 expression, mitochondrial activation and heat production. Mol Cell Endocrinol (2014) 383(1-2):137–46. doi: 10.1016/j.mce.2013.12.005
46. IJpenberg A, Tan NS, Gelman L, Kersten S, Seydoux J, Xu J, et al. In vivo activation of PPAR target genes by RXR homodimers. EMBO J (2004) 23(10):2083–91.
47. Rao AV, Rao LG. Carotenoids and human health. Pharmacol Res (2007) 55(3):207–16. doi: 10.1016/j.phrs.2007.01.012
48. Pierce JP, Natarajan L, Sun S, Al-Delaimy W, Flatt SW, Kealey S, et al. Increases in plasma carotenoid concentrations in response to a major dietary change in the women's healthy eating and living study. Cancer Epidemiol Biomarkers Prev (2006) 15(10):1886–92. doi: 10.1158/1055-9965.EPI-05-0928
49. Arballo J, Amengual J, Erdman JW Jr. Lycopene: A critical review of digestion, absorption, metabolism, and excretion. Antioxidants (Basel) (2021) 10(3). doi: 10.3390/antiox10030342
50. Unlu NZ, Bohn T, Francis D, Clinton SK, Schwartz SJ. Carotenoid absorption in humans consuming tomato sauces obtained from tangerine or high-beta-carotene varieties of tomatoes. J Agric Food Chem (2007) 55(4):1597–603. doi: 10.1021/jf062337b
51. Bezaire V, Mairal A, Ribet C, Lefort C, Girousse A, Jocken J, et al. Contribution of adipose triglyceride lipase and hormone-sensitive lipase to lipolysis in hMADS adipocytes. J Biol Chem (2009) 284(27):18282–91. doi: 10.1074/jbc.M109.008631
52. Moraes-Vieira PM, Saghatelian A, Kahn BB. GLUT4 expression in adipocytes regulates De novo lipogenesis and levels of a novel class of lipids with antidiabetic and anti-inflammatory effects. Diabetes (2016) 65(7):1808–15.
53. Carvalho E, Kotani K, Peroni OD, Kahn BB. Adipose-specific overexpression of GLUT4 reverses insulin resistance and diabetes in mice lacking GLUT4 selectively in muscle. Am J Physiol Endocrinol Metab (2005) 289(4):E551–61.
54. Achari AE, Jain SK. Adiponectin, a therapeutic target for obesity, diabetes, and endothelial dysfunction. Int J Mol Sci (2017) 18(6).
55. Miller KN, Clark JP, Anderson RM. Mitochondrial regulator PGC-1a-Modulating the modulator. Curr Opin Endocr Metab Res (2019) 5:37–44.
56. Kilroy GE, Zhang X, Floyd ZE. PPAR-gamma AF-2 domain functions as a component of a ubiquitin-dependent degradation signal. Obes (Silver Spring) (2009) 17(4):665–73.
57. Blanquart C, Barbier O, Fruchart JC, Staels B, Glineur C. Peroxisome proliferator-activated receptor alpha (PPARalpha ) turnover by the ubiquitin-proteasome system controls the ligand-induced expression level of its target genes. J Biol Chem (2002) 277(40):37254–9. doi: 10.1074/jbc.M110598200
58. Wei P, Pan D, Mao C, Wang YX. RNF34 is a cold-regulated E3 ubiquitin ligase for PGC-1alpha and modulates brown fat cell metabolism. Mol Cell Biol (2012) 32(2):266–75. doi: 10.1128/MCB.05674-11
59. Blanquart C, Mansouri R, Fruchart JC, Staels B, Glineur C. Different ways to regulate the PPARalpha stability. Biochem Biophys Res Commun (2004) 319(2):663–70. doi: 10.1016/j.bbrc.2004.05.035
60. Hauser S, Adelmant G, Sarraf P, Wright HM, Mueller E, Spiegelman BM. Degradation of the peroxisome proliferator-activated receptor gamma is linked to ligand-dependent activation. J Biol Chem (2000) 275(24):18527–33. doi: 10.1074/jbc.M001297200
61. Gray NE, Lam LN, Yang K, Zhou AY, Koliwad S, Wang JC. Angiopoietin-like 4 (Angptl4) protein is a physiological mediator of intracellular lipolysis in murine adipocytes. J Biol Chem (2017) 292(39):16135. doi: 10.1074/jbc.A111.294124
62. McQueen AE, Kanamaluru D, Yan K, Gray NE, Wu L, Li ML, et al. The c-terminal fibrinogen-like domain of angiopoietin-like 4 stimulates adipose tissue lipolysis and promotes energy expenditure. J Biol Chem (2017) 292(39):16122–34. doi: 10.1074/jbc.M117.803973
63. Fruhbeck G, Fernandez-Quintana B, Paniagua M, Hernandez-Pardos AW, Valenti V, Moncada R, et al. FNDC4, a novel adipokine that reduces lipogenesis and promotes fat browning in human visceral adipocytes. Metabolism (2020) 108:154261. doi: 10.1016/j.metabol.2020.154261
64. Georgiadi A, Lopez-Salazar V, Merahbi RE, Karikari RA, Ma X, Mourao A, et al. Orphan GPR116 mediates the insulin sensitizing effects of the hepatokine FNDC4 in adipose tissue. Nat Commun (2021) 12(1):2999. doi: 10.1038/s41467-021-22579-1
65. Fadini GP, Menegazzo L, Bonora BM, Mazzucato M, Persano S, Vigili de Kreutzenberg S, et al. Effects of age, diabetes, and vascular disease on growth differentiation factor 11: First-in-Human study. Diabetes Care (2015) 38(8):e118–9. doi: 10.2337/dc15-0868
66. Katsimpardi L, Kuperwasser N, Camus C, Moigneu C, Chiche A, Tolle V, et al. Systemic GDF11 stimulates the secretion of adiponectin and induces a calorie restriction-like phenotype in aged mice. Aging Cell (2020) 19(1):e13038. doi: 10.1111/acel.13038
67. Lynes MD, Leiria LO, Lundh M, Bartelt A, Shamsi F, Huang TL, et al. The cold-induced lipokine 12,13-diHOME promotes fatty acid transport into brown adipose tissue. Nat Med (2017) 23(5):631–7. doi: 10.1038/nm.4297
68. Gollasch B, Dogan I, Rothe M, Gollasch M, Luft FC. Maximal exercise and plasma cytochrome P450 and lipoxygenase mediators: a lipidomics study. Physiol Rep (2019) 7(13):e14165. doi: 10.14814/phy2.14165
69. Yi M, Cho SA, Min J, Kim DH, Shin JG, Lee SJ. Functional characterization of a common CYP4F11 genetic variant and identification of functionally defective CYP4F11 variants in erythromycin metabolism and 20-HETE synthesis. Arch Biochem Biophys (2017) 620:43–51. doi: 10.1016/j.abb.2017.03.010
70. Kulterer OC, Niederstaetter L, Herz CT, Haug AR, Bileck A, Pils D, et al. The presence of active brown adipose tissue determines cold-induced energy expenditure and oxylipin profiles in humans. J Clin Endocrinol Metab (2020) 105(7):2203–16. doi: 10.1210/clinem/dgaa183
71. Ng VY, Huang Y, Reddy LM, Falck JR, Lin ET, Kroetz DL. Cytochrome P450 eicosanoids are activators of peroxisome proliferator-activated receptor alpha. Drug Metab Dispos (2007) 35(7):1126–34. doi: 10.1124/dmd.106.013839
72. Stanford KI, Lynes MD, Takahashi H, Baer LA, Arts PJ, May FJ, et al. 12,13-diHOME: An exercise-induced lipokine that increases skeletal muscle fatty acid uptake. Cell Metab (2018) 27(6):1357.
73. Vasan SK, Noordam R, Gowri MS, Neville MJ, Karpe F, Christodoulides C. The proposed systemic thermogenic metabolites succinate and 12,13-diHOME are inversely associated with adiposity and related metabolic traits: evidence from a large human cross-sectional study. Diabetologia (2019) 62(11):2079–87. doi: 10.1007/s00125-019-4947-5
74. Bouchard L, Drapeau V, Provencher V, Lemieux S, Chagnon Y, Rice T, et al. Neuromedin beta: a strong candidate gene linking eating behaviors and susceptibility to obesity. Am J Clin Nutr (2004) 80(6):1478–86. doi: 10.1093/ajcn/80.6.1478
75. Blanchet R, Lemieux S, Couture P, Bouchard L, Vohl MC, Perusse L. Effects of neuromedin-beta on caloric compensation, eating behaviours and habitual food intake. Appetite (2011) 57(1):21–7. doi: 10.1016/j.appet.2011.04.003
76. Schwartz MW, Woods SC, Porte D Jr., Seeley RJ, Baskin DG. Central nervous system control of food intake. Nature (2000) 404(6778):661–71. doi: 10.1038/35007534
77. Wabitsch M, Farooqi S, Fluck CE, Bratina N, Mallya UG, Stewart M, et al. Natural history of obesity due to POMC, PCSK1, and LEPR deficiency and the impact of setmelanotide. J Endocr Soc (2022) 6(6):bvac057. doi: 10.1210/jendso/bvac057
78. Zeng X, Ye M, Resch JM, Jedrychowski MP, Hu B, Lowell BB, et al. Innervation of thermogenic adipose tissue via a calsyntenin 3beta-S100b axis. Nature (2019) 569(7755):229–35. doi: 10.1038/s41586-019-1156-9
79. Chang SH, Song NJ, Choi JH, Yun UJ, Park KW. Mechanisms underlying UCP1 dependent and independent adipocyte thermogenesis. Obes Rev (2019) 20(2):241–51. doi: 10.1111/obr.12796
80. Svensson PA, Lindberg K, Hoffmann JM, Taube M, Pereira MJ, Mohsen-Kanson T, et al. Characterization of brown adipose tissue in the human perirenal depot. Obes (Silver Spring) (2014) 22(8):1830–7. doi: 10.1002/oby.20765
81. Benson KK, Hu W, Weller AH, Bennett AH, Chen ER, Khetarpal SA, et al. Natural human genetic variation determines basal and inducible expression of PM20D1, an obesity-associated gene. Proc Natl Acad Sci USA (2019) 116(46):23232–42.
82. Long JZ, Svensson KJ, Bateman LA, Lin H, Kamenecka T, Lokurkar IA, et al. The secreted enzyme PM20D1 regulates lipidated amino acid uncouplers of mitochondria. Cell (2016) 166(2):424–35. doi: 10.1016/j.cell.2016.05.071
83. Long JZ, Roche AM, Berdan CA, Louie SM, Roberts AJ, Svensson KJ, et al. Ablation of PM20D1 reveals n-acyl amino acid control of metabolism and nociception. Proc Natl Acad Sci U.S.A. (2018) 115(29):E6937–E45. doi: 10.1073/pnas.1803389115
84. Li D, Liu Y, Gao W, Han J, Yuan R, Zhang M, et al. Inhibition of miR-324-5p increases PM20D1-mediated white and brown adipose loss and reduces body weight in juvenile mice. Eur J Pharmacol (2019) 863:172708. doi: 10.1016/j.ejphar.2019.172708
85. Kazak L, Chouchani ET, Jedrychowski MP, Erickson BK, Shinoda K, Cohen P, et al. A creatine-driven substrate cycle enhances energy expenditure and thermogenesis in beige fat. Cell (2015) 163(3):643–55. doi: 10.1016/j.cell.2015.09.035
86. Muller S, Balaz M, Stefanicka P, Varga L, Amri EZ, Ukropec J, et al. Proteomic analysis of human brown adipose tissue reveals utilization of coupled and uncoupled energy expenditure pathways. Sci Rep (2016) 6:30030. doi: 10.1038/srep30030
87. Braun K, Oeckl J, Westermeier J, Li Y, Klingenspor M. Non-adrenergic control of lipolysis and thermogenesis in adipose tissues. J Exp Biol (2018) 221(Pt Suppl 1). doi: 10.1242/jeb.165381
88. Mantovani G, Bondioni S, Alberti L, Gilardini L, Invitti C, Corbetta S, et al. Protein kinase a regulatory subunits in human adipose tissue: decreased R2B expression and activity in adipocytes from obese subjects. Diabetes (2009) 58(3):620–6. doi: 10.2337/db08-0585
89. Nguyen HP, Yi D, Lin F, Viscarra JA, Tabuchi C, Ngo K, et al. Aifm2, a NADH oxidase, supports robust glycolysis and is required for cold- and diet-induced thermogenesis. Mol Cell (2020) 77(3):600–17.e4. doi: 10.1016/j.molcel.2019.12.002
90. Caron A, Labbe SM, Carter S, Roy MC, Lecomte R, Ricquier D, et al. Loss of UCP2 impairs cold-induced non-shivering thermogenesis by promoting a shift toward glucose utilization in brown adipose tissue. Biochimie (2017) 134:118–26. doi: 10.1016/j.biochi.2017.01.006
91. Bouillaud F. UCP2, not a physiologically relevant uncoupler but a glucose sparing switch impacting ROS production and glucose sensing. Biochim Biophys Acta (2009) 1787(5):377–83. doi: 10.1016/j.bbabio.2009.01.003
92. Jahansouz C, Xu H, Hertzel AV, Kizy S, Steen KA, Foncea R, et al. Partitioning of adipose lipid metabolism by altered expression and function of PPAR isoforms after bariatric surgery. Int J Obes (Lond) (2018) 42(2):139–46. doi: 10.1038/ijo.2017.197
93. Yamaguchi S, Franczyk MP, Chondronikola M, Qi N, Gunawardana SC, Stromsdorfer KL, et al. Adipose tissue NAD(+) biosynthesis is required for regulating adaptive thermogenesis and whole-body energy homeostasis in mice. Proc Natl Acad Sci U.S.A. (2019) 116(47):23822–8.
94. Yamaguchi S, Yoshino J. Adipose tissue NAD(+) biology in obesity and insulin resistance: From mechanism to therapy. Bioessays (2017) 39(5).
95. Nagy L, Rauch B, Szerafin T, Uray K, Toth A, Bai P. Nicotinamide-riboside shifts the differentiation of human primary white adipocytes to beige adipocytes impacting substrate preference and uncoupling respiration through SIRT1 activation and mitochondria-derived reactive species production. Front Cell Dev Biol (2022) 10:979330.
96. Balaz M, Ukropcova B, Kurdiova T, Vlcek M, Surova M, Krumpolec P, et al. Improved adipose tissue metabolism after 5-year growth hormone replacement therapy in growth hormone deficient adults: The role of zinc-alpha2-glycoprotein. Adipocyte (2015) 4(2):113–22.
97. Prossnitz ER, Barton M. Estrogen biology: new insights into GPER function and clinical opportunities. Mol Cell Endocrinol (2014) 389(1-2):71–83.
98. Sharma G, Hu C, Staquicini DI, Brigman JL, Liu M, Mauvais-Jarvis F, et al. Preclinical efficacy of the GPER-selective agonist G-1 in mouse models of obesity and diabetes. Sci Transl Med (2020) 12(528). doi: 10.1126/scitranslmed.aau5956
99. Hedesan OC, Fenzl A, Digruber A, Spirk K, Baumgartner-Parzer S, Bilban M, et al. Parathyroid hormone induces a browning program in human white adipocytes. Int J Obes (Lond) (2019) 43(6):1319–24. doi: 10.1038/s41366-018-0266-z
100. Carper D, Coue M, Nascimento EBM, Barquissau V, Lagarde D, Pestourie C, et al. Atrial natriuretic peptide orchestrates a coordinated physiological response to fuel non-shivering thermogenesis. Cell Rep (2020) 32(8):108075. doi: 10.1016/j.celrep.2020.108075
101. Moro C, Crampes F, Sengenes C, De Glisezinski I, Galitzky J, Thalamas C, et al. Atrial natriuretic peptide contributes to physiological control of lipid mobilization in humans. FASEB J (2004) 18(7):908–10. doi: 10.1096/fj.03-1086fje
102. Cho KJ, Shim JH, Cho MC, Choe YK, Hong JT, Moon DC, et al. Signaling pathways implicated in alpha-melanocyte stimulating hormone-induced lipolysis in 3T3-L1 adipocytes. J Cell Biochem (2005) 96(4):869–78. doi: 10.1002/jcb.20561
103. Puigserver P, Wu Z, Park CW, Graves R, Wright M, Spiegelman BM. A cold-inducible coactivator of nuclear receptors linked to adaptive thermogenesis. Cell (1998) 92(6):829–39. doi: 10.1016/S0092-8674(00)81410-5
104. Mazzucotelli A, Viguerie N, Tiraby C, Annicotte JS, Mairal A, Klimcakova E, et al. The transcriptional coactivator peroxisome proliferator activated receptor (PPAR)gamma coactivator-1 alpha and the nuclear receptor PPAR alpha control the expression of glycerol kinase and metabolism genes independently of PPAR gamma activation in human white adipocytes. Diabetes (2007) 56(10):2467–75. doi: 10.2337/db06-1465
105. Cadoudal T, Distel E, Durant S, Fouque F, Blouin JM, Collinet M, et al. Pyruvate dehydrogenase kinase 4: regulation by thiazolidinediones and implication in glyceroneogenesis in adipose tissue. Diabetes (2008) 57(9):2272–9. doi: 10.2337/db08-0477
106. Cadoudal T, Blouin JM, Collinet M, Fouque F, Tan GD, Loizon E, et al. Acute and selective regulation of glyceroneogenesis and cytosolic phosphoenolpyruvate carboxykinase in adipose tissue by thiazolidinediones in type 2 diabetes. Diabetologia (2007) 50(3):666–75. doi: 10.1007/s00125-006-0560-5
107. Iwase M, Tokiwa S, Seno S, Mukai T, Yeh YS, Takahashi H, et al. Glycerol kinase stimulates uncoupling protein 1 expression by regulating fatty acid metabolism in beige adipocytes. J Biol Chem (2020) 295(20):7033–45. doi: 10.1074/jbc.RA119.011658
108. Ou X, Ji C, Han X, Zhao X, Li X, Mao Y, et al. Crystal structures of human glycerol 3-phosphate dehydrogenase 1 (GPD1). J Mol Biol (2006) 357(3):858–69.
109. Keipert S, Kutschke M, Ost M, Schwarzmayr T, van Schothorst EM, Lamp D, et al. Long-term cold adaptation does not require FGF21 or UCP1. Cell Metab (2017) 26(2):437–46.e5.
110. Yang R, Hu Y, Lee CH, Liu Y, Diaz-Canestro C, Fong CHY, et al. PM20D1 is a circulating biomarker closely associated with obesity, insulin resistance and metabolic syndrome. Eur J Endocrinol (2021) 186(2):151–61.
111. Kim JT, Jedrychowski MP, Wei W, Fernandez D, Fischer CR, Banik SM, et al. A plasma protein network regulates PM20D1 and n-acyl amino acid bioactivity. Cell Chem Biol (2020) 27(9):1130–9.e4.
112. Kazak L, Chouchani ET, Lu GZ, Jedrychowski MP, Bare CJ, Mina AI, et al. Genetic depletion of adipocyte creatine metabolism inhibits diet-induced thermogenesis and drives obesity. Cell Metab (2017) 26(4):660–71 e3.
113. Audrito V, Messana VG, Deaglio S. NAMPT and NAPRT: Two metabolic enzymes with key roles in inflammation. Front Oncol (2020) 10:358.
114. Qiang L, Wang L, Kon N, Zhao W, Lee S, Zhang Y, et al. Brown remodeling of white adipose tissue by SirT1-dependent deacetylation of ppargamma. Cell (2012) 150(3):620–32.
115. DiRenzo J, Soderstrom M, Kurokawa R, Ogliastro MH, Ricote M, Ingrey S, et al. Peroxisome proliferator-activated receptors and retinoic acid receptors differentially control the interactions of retinoid X receptor heterodimers with ligands, coactivators, and corepressors. Mol Cell Biol (1997) 17(4):2166–76.
116. Rebello CJ, Coulter AA, Reaume AG, Cong W, Cusimano LA, Greenway FL. MLR-1023 treatment in mice and humans induces a thermogenic program, and menthol potentiates the effect. Pharm (Basel) (2021) 14(11).
117. Wysolmerski JJ, Stewart AF. The physiology of parathyroid hormone-related protein: an emerging role as a developmental factor. Annu Rev Physiol (1998) 60:431–60.
118. Larsson S, Jones HA, Goransson O, Degerman E, Holm C. Parathyroid hormone induces adipocyte lipolysis via PKA-mediated phosphorylation of hormone-sensitive lipase. Cell Signal (2016) 28(3):204–13.
119. Kovanicova Z, Kurdiova T, Balaz M, Stefanicka P, Varga L, Kulterer OC, et al. Cold exposure distinctively modulates parathyroid and thyroid hormones in cold-acclimatized and non-acclimatized humans. Endocrinology (2020) 161(7).
120. Mattsson CL, Csikasz RI, Chernogubova E, Yamamoto DL, Hogberg HT, Amri EZ, et al. beta(1)-adrenergic receptors increase UCP1 in human MADS brown adipocytes and rescue cold-acclimated beta(3)-adrenergic receptor-knockout mice via nonshivering thermogenesis. Am J Physiol Endocrinol Metab (2011) 301(6):E1108–18.
121. Arner P, Bernard S, Salehpour M, Possnert G, Liebl J, Steier P, et al. Dynamics of human adipose lipid turnover in health and metabolic disease. Nature (2011) 478(7367):110–3.
Keywords: UCP1, naringenin, carotenoid, PPARγ, RXRγ, PPARα, adiponectin, lipolysis
Citation: Coulter AA, Greenway FL, Zhang D, Ghosh S, Coulter CR, James SL, He Y, Cusimano LA and Rebello CJ (2023) Naringenin and β-carotene convert human white adipocytes to a beige phenotype and elevate hormone- stimulated lipolysis. Front. Endocrinol. 14:1148954. doi: 10.3389/fendo.2023.1148954
Received: 20 January 2023; Accepted: 20 March 2023;
Published: 17 April 2023.
Edited by:
Rubén Cereijo, University of Barcelona, SpainReviewed by:
Alexander Bartelt, Ludwig Maximilian University of Munich, GermanyCopyright © 2023 Coulter, Greenway, Zhang, Ghosh, Coulter, James, He, Cusimano and Rebello. This is an open-access article distributed under the terms of the Creative Commons Attribution License (CC BY). The use, distribution or reproduction in other forums is permitted, provided the original author(s) and the copyright owner(s) are credited and that the original publication in this journal is cited, in accordance with accepted academic practice. No use, distribution or reproduction is permitted which does not comply with these terms.
*Correspondence: Candida J. Rebello, Y2FuZGlkYS5yZWJlbGxvQHBicmMuZWR1
†Deceased
Disclaimer: All claims expressed in this article are solely those of the authors and do not necessarily represent those of their affiliated organizations, or those of the publisher, the editors and the reviewers. Any product that may be evaluated in this article or claim that may be made by its manufacturer is not guaranteed or endorsed by the publisher.
Research integrity at Frontiers
Learn more about the work of our research integrity team to safeguard the quality of each article we publish.