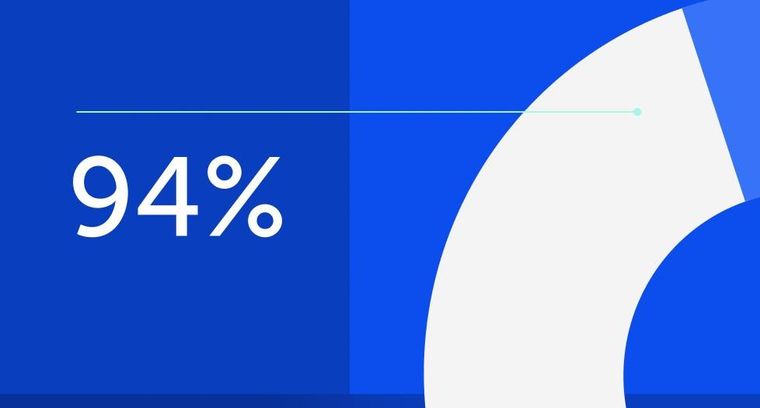
94% of researchers rate our articles as excellent or good
Learn more about the work of our research integrity team to safeguard the quality of each article we publish.
Find out more
REVIEW article
Front. Endocrinol., 20 March 2023
Sec. Cardiovascular Endocrinology
Volume 14 - 2023 | https://doi.org/10.3389/fendo.2023.1124353
This article is part of the Research TopicCircadian Rhythm in Cellular EndocrinologyView all 5 articles
Cardiovascular complications are a common death cause in type 2 diabetes patients, as they are often combined. Plasminogen-activator Inhibitor 1 (PAI-1) participates in the development and progression of cardiovascular complications in diabetes. Insulin resistance increases PAI-1 production, and high PAI-1 levels lead to an environment conducive to thrombosis and earlier and more severe vascular disease. Current evidence also suggests that PAI-1 has a rhythmic profile of circadian fluctuations and acrophase in the morning within a single day, which might explain the high morning incidence of cardiovascular events. Thus, PAI-1 is a possible drug target. Although several PAI-1 inhibitors have been developed, none have yet been allowed for clinical use. Research on rhythm has also led to the concept of “chronotherapy”, a rhythm-based drug regimen expected to improve the treatment of cardiovascular complications in diabetic patients. Herein, we searched several databases and reviewed relevant articles to describe the circadian rhythm characteristics and endogenous molecular mechanisms of PAI-1, its relationship with insulin resistance, the causes of cardiovascular complications caused by PAI-1, and the current development of PAI-1 inhibitors. We also summarized the possibility of using the circadian rhythm of PAI-1 to treat cardiovascular complications in diabetic patients.
Type 2 diabetes patients are often accompanied by cardiovascular disease, including atherosclerotic cardiovascular diseases (ASCVD) and heart failure (1, 2). Meanwhile, diabetes is an independent risk factor for cardiovascular disease (3–5). The risk of cardiovascular disease in diabetic patients is 2-3 times higher than that in healthy people (2, 4, 6), which is related to the thrombogenic environment and the earlier and more severe vascular pathological change (7, 8). Besides, hypofibrinolysis is a fundamental abnormality in the thrombogenic environment.
Plasminogen-activator Inhibitor 1 (PAI-1) participates in the occurrence and development of this state and is elevated in patients with type 2 diabetes, impaired glucose tolerance, and obesity. Insulin resistance and hyperglycemia, which often occur in these three states, are the main causes of elevated PAI-1 (9–11).
PAI-1 is a Serine Protease Inhibitor (SERPIN) superfamily member and shares a highly ordered structure with other members (12). PAI-1 can be produced by various cells, such as platelets, adipocytes, vascular endothelial cells, endometrial cells, and liver cells. After synthesis, PAI-1 is primarily stored in platelets, while circulating PAI-1 is mainly bound to its cofactor vitronectin, tissue-type Plasminogen Activator (t-PA), or urokinase-type Plasminogen Activator (u-PA) (13). Vitronectin is a heat-stable glycoprotein that binds to PAI-1 to stabilize its molecular conformation and can convert it to the activated form (14). PAI-1 has three molecular conformations corresponding to different functional states: active, latent, and cleaved (9). Active PAI-1 is mainly stored in platelets and is released when platelets are stimulated by thrombin, then PAI-1 rapidly binds to t-PA or u-PA, forming a stable 1:1 complex that inhibits the latter two (15). At the same time, PAI-1 can bind to fibrin and maintain inhibitory activity, contributing to clot stabilization and extension in thrombosis (16, 17). Additionally, PAI-1 is considered one of the most effective fibrinolytic modulators (18).
A series of studies have found that PAI-1 has a circadian rhythm and acrophase at around 6:30 am (Circadian rhythms were developed in evolution and fit with external time, so the time here is the geophysical time of the location where the study was conducted, and the times mentioned below follow the same) (19). Also, epidemiological evidence suggests that cardiovascular events such as myocardial infarction and aortic dissection have a morning peak (20–22). Thus, the PAI-1 rhythm might partially explain the high rate of cardiovascular events in the morning (19, 23–25), making it a possible therapeutic target (25).
The term circadian originates from the Latin circa diem. Circadian rhythms are present in almost all forms of life (26). In higher organisms such as humans, circadian rhythms are a complex physiological and molecular system, with the most intuitive sleep-wake cycles, feeding cycles, reward pathways, mood, and cardiovascular indicators discussed here all having circadian manifestations (27). The biological clock is a complex, genetically controlled system of molecules that controls these rhythms (28, 29). This oscillator is present in almost every organism cell, involving a transcriptional-translational feedback network and transcription factors and regulators that will be discussed in detail below.
The global incidence and prevalence of diabetes continue to increase (30), and effective and early control of cardiovascular complications, an important death cause in diabetic patients, is necessary (31). PAI-1 is a possible therapeutic target, but the research on the relationship between its circadian rhythm and diabetic cardiovascular disease is unclear, and related drugs, such as PAI-1 inhibitors, are still being developed. Therefore, based on the analysis of the characteristics and mechanisms of PAI-1 circadian rhythm generation, the elaboration of the relationship between PAI-1 and diabetic cardiovascular disease, and the assessment of the therapeutic prospects of related drugs for diabetic cardiovascular complications, we summarized the possibility of using the circadian rhythm of PAI-1 to treat the cardiovascular complications of diabetes using “chronotherapy”.
To accomplish this goal, we performed an electronic search in PubMed, Google Scholar, and SCOPUS databases to identify so far published studies using different combinations of the following keywords: plasminogen activator inhibitor-1, circadian rhythm, atherosclerotic cardiovascular diseases, cardiovascular complications, chronotherapy, drug development, type 2 diabetes mellitus. Only publications in English were considered.
Almost all variables related to the cardiovascular system have a day/night pattern or circadian rhythms (32, 33), including the heart rate (34), blood pressure (34, 35), circulating catecholamines (36, 37), and vascular endothelial function (38). Fibrinolytic markers such as PAI-1 and tissue plasminogen activator (t-PA) also fluctuate in diurnal levels in rodents and humans (39).
Circadian fluctuations of PAI-1 have a clear correlation with activity in manifestation. Rodents-based experiments found that PAI-1 levels increased at night, the active period in rodents, and reached a minimum during the day, their resting period (40, 41). In humans, Scheer et al. conducted continuous blood sampling at 1-h intervals within one day in 12 normal people and found that PAI-1 has large amplitude (19). The acrophase was about 6:30 am, and the trough occurred around 3:30 pm. Similar rhythms were found in another trial involving 66 healthy volunteers, but the acrophase was around 8:00 am, and the nadir was 2:00 pm (24). The small number of samples in a day might limit this.
Although the characteristics of PAI-1 circadian fluctuations are related to activity and rest, the current study found that the biological clock determines PAI-1 changes during the day. Furthermore, Scheer et al. found that a mild stress test (i.e., “activity”) did not affect levels of PAI-1 (19). In addition, reversing the feeding cycle did not affect the circadian phase of circulating PAI-1 levels in mice, suggesting that the feeding cycle does not affect the circadian rhythm of PAI-1 (42). The above findings suggest that PAI-1 may have an endogenous rhythm, and feeding and activity cycles are not zeitgebers.
The mechanisms of the endogenous rhythm have been previously explored. The circadian rhythms in humans and many mammals are primarily controlled by the suprachiasmatic nucleus (SCN) pacemaker neurons in the hypothalamus (43). They are mainly linked to the retina through the retinohypothalamic tract (RHT) to synchronize with ambient light rhythms (44). Pacemaker neurons isolated and cultured in vitro can also maintain rhythm autonomy (45).
Also, this link can still work in blinded patients. In 11 blind patients with no conscious perception of light, Czeisler et al. found that the circadian rhythm of melatonin could still be regulated by light in some patients, suggesting that the external light rhythm is an important zeitgeber (46). Furthermore, in conjunction with other studies showing that the mammalian eye is connected to at least two photosystems: the occipital cortex, which mediates the conscious perception of light and the recognition of images, and a subcortical system that mediates light-sensitive synchronization of the circadian pacemaker (46, 47).
At the cellular level, the rhythm is expressed as a diurnal variation in the spontaneous firing rate of pacemaker neurons, with a high frequency of activity during the day and a low frequency at night (48). Also, pacemaker neurons exhibit daily changes in membrane properties such as input resistance (Rinput) and resting membrane potential. This is attributed to the diurnal fluctuation of mRNA and protein levels, such as ion channels (46). Therefore, in the daytime, increased persistent sodium current (47), sodium leak current, and L-type calcium current increase excitability (49, 50). In the dark state at night, the increased potassium current makes the resting membrane potential hyperpolarize to the potassium balance potential, ensuring that neurons are not easily driven to the action potential (50, 51).
At the molecular level, the rhythms are formed by autoregulatory transcriptional feedback loops of multiple genes and transcription factors (52). The central loop is mainly composed of the circadian clock genes Clock, Bmal1, Period (Per1, Per2), Cryptochrome (Cry1, Cry2), and their transcription products CLOCK : BMAL1 heterodimer and repressor proteins PER1-2 and CRY1-2. The transcription and translation products of Clock and Bmal1 genes combine to form the CLOCK : BMAL1 heterodimer, which binds to the E-box of Per and Cry genes, and positively regulates the expression of the repressor proteins PER1-2 and CRY1-2. PER and CRY repressor proteins combine to translocate into the nucleus to repress their transcription (53). This feedback process lasts about 24 h and is similar to the external circadian rhythm. At the same time, CLOCK : BMAL1 regulates many downstream target genes, and most of these clock control genes (CCGs) products have diurnal fluctuations. At the post-transcriptional level, competing E3 ubiquitin ligases can degrade PER and CRY proteins, affecting their turnover rate, which can control circadian rhythms (54). Wan et al. also found that the Pai-1 gene might have an epigenetic pattern. SIRT1, a class III histone deacetylase, can bind to the Pai-1 promoter, resulting in decreased acetylation of histone H4 lysine16 (H4K16) on this region, promoting heterochromatin formation and Pai-1 silencing. In cell models, SIRT1 can improve vascular endothelial cell function and arterial stiffness in vivo and exerts a protective effect in vascular endothelial senescence (55) (Figure 1).
Figure 1 Molecular mechanism of the endogenous rhythm. The central loop is mainly composed of circadian clock genes Clock, Bmal1, Period (Per1, Per2), Cryptochrome (Cry1, Cry2), and their transcription products CLOCK : BMAL1 heterodimer and repressor proteins PER1-2 and CRY1-2. Regulation of clock control genes (CCGs) by CLOCK : BMAL1 heterodimers results in expression products with rhythmic properties, such as PAI-1. At the post-transcriptional level, PER and CRY proteins are phosphorylated, with casein kinase 1ϵ/δ (CK1ϵ/δ) and AMP kinase (AMPK) phosphorylating PER and CRY proteins, respectively, thereby enabling them to function. The CLOCK : BMAL1 heterodimer is also regulated by other feedback loops. For example, regulation by Rev-erbα (Nr1d1) and Rorα, with the former repressing Bmal1 transcription and the latter acting as a facilitator.(“+” represents facilitation, while “-” represents inhibition.).
Meanwhile, SCN controls peripheral oscillators via nerves and hormones, and common pathways include sympathetic, parasympathetic (56), and glucocorticoids (57). Current research has demonstrated that the Pai-1 is a CCG (39). The Clock gene binds directly to the promoter of PAI-1 and positively regulates its expression, which explains its circadian fluctuations (58). Using mice deficient in Bmal1 (Bmal1−/−), Somanath et al. found that knockout mice lost the circadian rhythm of PAI-1 compared to controls, shortened tail bleeding clotting time, and upregulated vWF at mRNA and protein levels (41).
Overall, the endogenous rhythm of PAI-1 is autonomous and dual. Scheer et al. suggested that this rhythm arises from evolutionary pressure (19). High PAI-1 levels at night can promote blood coagulation after injury, favoring early humans to respond to nocturnal predators. However, this rhythm increases the risk of cardiovascular events in the morning for people susceptible to cardiovascular diseases in modern society, such as type 2 diabetes patients.
PAI-1 levels are elevated in type 2 diabetes and obesity patients but decreased in the type 1 diabetic population. Regarding this phenomenon, Agren et al. found that insulin resistance is the leading cause of elevated PAI-1 levels (10). The combination of obesity leads to a further increase in PAI-1 levels. Thus, obese patients with type 2 diabetes show a further decrease in fibrinolytic activity (59). High blood glucose and insulin precursor molecules can also elevate PAI-1 levels (11, 60, 61). These studies suggested that type 2 diabetes can promote PAI-1 synthesis and secretion through multiple pathways.
Under normal conditions, insulin exerts its physiological effects through Protein Kinase B (PKB) activation via the Phosphoinositide 3-Kinase (PI3K) pathway (62). In contrast, the above signaling pathway is blocked in the insulin-resistant state. Compensatory hyperinsulinemia activates the Mitogen-Activated Protein Kinase/Extracellular signal-Regulated Kinase (MAPK/ERK) pathway (63), increasing the secretion of inflammatory markers such as Intercellular Adhesion Molecule-1 (ICAM-1), Vascular Cell Adhesion Molecule-1 (VCAM-1), and PAI-1 (64). Additionally, a study by Chen et al. on rat aortic smooth muscle revealed the presence of a cis-carbohydrate response element in the promoter region of the Pai-1 gene and that glucose induces PAI-1 expression through two Sp1 sites in its promoter region (11). Therefore, alleviating insulin resistance and lowering blood glucose might help reduce Pai-1 gene expression and improve hypofibrinolysis.
Insulin resistance, obesity, and lipid metabolism disorders are closely related, and the deposition of intracellular lipids can disrupt insulin signaling pathways and abnormal glucose transport and cause insulin resistance (65, 66). At the same time, insulin resistance can cause lipid disorders (67). Some studies have also found that high levels of triglyceride (TG), fatty acids, and very low-density lipoprotein (VLDL) can increase PAI-1 levels (68, 69).
Besides the already discussed reduction of fibrinolysis by PAI-1, it might also promote the formation of intimal plaques and accelerate the progression of atherosclerosis (39, 58). Thus, high PAI-1 levels have been associated with various thrombotic disorders. An intuitive manifestation of this is the higher PAI-1 levels in coronary atherosclerotic plaques in type 2 diabetic patients compared to non-diabetic patients (70), increasing the risk of disease in patients.
Furthermore, regarding the changes in the pattern of increased PAI-1 levels, Pavlov et al. analyzed the circadian rhythm of PAI-1 in acute ST-segment elevation myocardial infarction (STEMI) patients. They found that PAI-1 levels were higher than normal at all time points during the day (25). Also, Fuchs et al. found that PAI-1 levels were higher in obese patients than in healthy controls at all times and were further elevated in the presence of combined nonalcoholic fatty liver disease (71). High levels of fluctuating PAI-1 and other diurnal cardiovascular markers, combined with external stressors, increase the risk of cardiovascular events, which is mild and moderate in normal populations. However, in susceptible populations, the elevated risk might exceed the “risk threshold,” and cardiovascular events can occur (72).
In summary, in type 2 diabetes and obesity patients, multiple factors associated with insulin resistance increase PAI-1, inhibiting fibrinolysis and creating an environment of low fibrinolysis in vivo. High PAI-1 levels are considered an independent risk factor for thrombo-occlusive disease (73, 74). Thus, PAI-1 is involved in the development and progression of cardiovascular complications in diabetes and might explain the early and frequent onset of cardiovascular disease in type 2 diabetic patients.
PAI-1 plays an essential role in the fibrinolytic pathway and is also involved in other pathophysiological processes, including cancer (75), aging (76), cardiac fibrosis (77), and wound healing (78), making PAI-1 a highly promising therapeutic target. No PAI-1-related drugs have been allowed to be used clinically, although numerous animal studies have yielded promising results.
After discovering the pharmacological potential of PAI-1, many research groups and pharmaceutical companies have been developing PAI-1 inhibitors. The drugs developed cover various types, such as monoclonal antibodies, small molecule substances, and peptides, and several review articles have been published to summarize them (9, 79, 80). Although there is a wide variety of drugs, the directions of PAI-1 inhibitors are two main categories: directly inhibit PAI-1 production and interfere with its physiological effects.
Inhibition of PAI-1 production is logically the most straightforward approach, as reduced levels represent enhanced fibrinolysis and reduced risk of thrombosis. However, reducing PAI-1 levels requires fine-tuning, and fully inhibited, or reduced levels can lead to varying degrees of bleeding (81). Various drugs (e.g., T686, T2639) have been developed to reduce PAI-1 mRNA levels, but they all exert pharmacological effects in experimental animal models (82, 83), and no clinical trial data are available. Moreover, the ideal state is to suppress the elevated PAI-1 to normal levels. Thus, the dose of the drug is difficult to control, making its clinical future relatively ambiguous.
The other mode of action, interfering with the physiological functions of PAI-1, is relatively safe and mild. For example, the mouse monoclonal anti-human PAI-1 antibody CLB-2C8 cleaves and inactivates PAI-1 and significantly enhances endogenous thrombus lysis and inhibits thrombus development in a rabbit jugular vein thrombosis model but has not been further studied in humans (84). Another antibody, MEDI-579, has high affinity and specificity for PAI-1 and modulates the interaction between PAI-1 and t-PA and u-PA (85). In the small molecule class, classical ones are PAI-039 and tiplaxtinin, and many subsequent drugs are based on improving their efficacy. PAI-039 was patented in 2004 and has shown promising results in various animal models (86). However, PAI-039 does not inhibit PAI-1 bound to vitronectin (87), which requires the development of drugs that can resist vitronectin-bound PAI-1. Although various compounds that could act on vitronectin-bound PAI-1 were subsequently developed, complete inhibition was never achieved (79), which might be related to the multiple molecular structures of PAI-1 mentioned above (Table 1).
Moreover, current research has found that drugs used to treat type 2 diabetes reduce PAI-1 levels. Recalling the role of insulin precursors mentioned previously, treatment with exogenous insulin can reduce PAI-1 levels mainly by inhibiting the secretion of insulin precursors from pancreatic β-cells (91). Insulin sensitizers such as thiazolidinediones (92) and metformin (93) can positively affect the fibrinolytic system, partly mediated by insulin resistance alleviation and PAI-1 reduction. Liu et al. found that Glucagon-like Peptide-1 receptor agonists (GLP-1RA) can inhibit PAI-1 production in human vascular endothelial cells, which might improve endothelial cell dysfunction and premature atherosclerosis in type 2 diabetes patients (94). Meanwhile, hyperglycemia can act on two Sp1 sites to promote PAI-1 production, suggesting that improved blood glucose levels are conducive to lower PAI-1 levels (11).
Additionally, various drugs for cardiovascular disease can interfere with PAI-1 levels. For example, Brown et al. evaluated the Renin-Angiotensin-Aldosterone System (RAAS) and PAI-1 and suggested that Angiotensin Converting Enzyme Inhibitors (ACEI) might also reduce PAI-1 levels (95). The TG and VLDL mentioned above can increase PAI-1 expression (68, 69), while some studies have also found that statins and Phenoxyaromatic acid lipid-regulating drugs can modulate the coagulation system and reduce PAI-1 production (96). However, some studies have also presented different views about the effects of ACEI and lipid-regulating drugs, suggesting that their effects are limited (9, 97), which might be related to the complex molecular structure of PAI-1 and its different functional states (9).
Furthermore, the thrombotic complications of severe COVID-19 disease might be directly attributable to elevated PAI-1 levels. Whyte et al. suggested that PAI-1 might serve as a significant prognostic predictor and existing drugs (e.g., tenecteplase) might be used to treat COVID-19 and other respiratory diseases (98). Also, a PAI-1 inhibitor (TM5614) has been investigated for treating patients with severe COVID-19 (Study To antagOnize Plasminogen Activator Inhibitor-1 in Severe COVID-19: Trial identifier NCT04634799, https://clinicaltrials.gov/ct2/show/NCT04634799?term=PAI-1$+$inhibitor&draw=2&rank=1). Another drug (ACT001), still in phase 1 clinical trials, is expected to inhibit the proliferation, invasion, and migration of glioblastoma in combination with cisplatin and exert anti-tumor effects (99).
In conclusion, PAI-1 is a promising therapeutic target, and multiple PAI-1 inhibitors have shown promising applications. Drugs commonly used clinically for treating type 2 diabetes can also reduce PAI-1, a phenomenon primarily associated with lower insulin precursors and improved insulin resistance, expanding the evidence for the clinical use of these drugs.
In modern life, circadian rhythm disorders caused by unhealthy habits such as staying up late, artificial light, and night shift work are quite common. Current research has demonstrated that circadian rhythm disturbances are associated with myocardial infarction, cancer, and neurodegenerative diseases (21). Hence, circadian rhythm disorders can lead to a wide range of conditions.
From another point of view, cardiovascular events and cardiovascular indicators are affected by time, and the therapeutic effects might be most effective if the drug is administered following these rhythms. Additionally, the metabolism of drugs is also regulated by circadian rhythms (100, 101), which led to the proposition of the “chronotherapy” concept (102).
In fact, “chronotherapy” has been studied to treat cardiovascular disease. As early as 1985, Muller et al. found that beta-adrenergic receptor blockers reduced the incidence of adverse cardiovascular events and controlled their morning peak (20). Sica et al. specifically analyzed the pharmacokinetic profile of a propranolol extended-release agent and concluded that nighttime dosing is recommended to ensure morning drug concentrations (103). Besides, a prospective study followed 448 type 2 diabetic patients with comorbid hypertension for a median duration of 5.4 years and found that cardiovascular risk was significantly lower in patients who took one or more hypertensive drugs at bedtime than in subjects who took them after rising in the morning (104). These studies support “chronotherapy” effectiveness.
Therefore, PAI-1-related drugs are expected to reduce the risk of cardiovascular events if their mode of administration can be adjusted to maintain good blood concentrations at the acrophase of PAI-1 levels. However, there are no reports of PAI-1-related drugs incorporating circadian rhythms. Nevertheless, the use of chronotherapy faces some practical problems. The first obstacle is the difficulty of detecting and diagnosing circadian rhythm disorders. Detecting a complete rhythm requires continuous sampling, which must be done partly at night, making it difficult to complete in an outpatient setting and often difficult for patients to accept (33). Second, the presence of comorbidities can also affect chronotherapy effectiveness. For example, in people with comorbid obstructive sleep apnea syndrome, the peak of cardiovascular events occurs earlier in the night rather than in the morning (105). Additionally, the intersection of multiple factors makes chronotherapy limited. For example, using beta-adrenergic receptor blockers at night can reduce the risk of cardiovascular events as described above but can impair sleep by inhibiting melatonin release (106, 107).
Based on the above studies, PAI-1 has a rhythm controlled by genes, and its morning acrophase might explain the high incidence of cardiovascular events in the morning. Insulin resistance in patients with type 2 diabetes or obesity leads to an increase in PAI-1 and high levels throughout the day compared to normal individuals, creating an environment of reduced fibrinolysis that promotes the development of cardiovascular complications. Concurrently high PAI-1 levels, combined with individual susceptibility and external stressors, cardiovascular events might occur when the risk exceeds the “risk threshold” described above.
However, our current study also has some limitations. Many of the above cardiovascular indicators (e.g., heart rate, blood pressure, circulating catecholamines) have circadian rhythms, and their patterns are also associated with cardiovascular events. Some of these indicators (e.g., blood pressure) can affect PAI-1 levels (108), which makes the role of PAI-1 in this not very conclusive. Hence, further studies are needed to clarify it.
The role of PAI-1 in cardiovascular complications of diabetes has been widely studied. Although no PAI-1 inhibitors have been approved for human treatment, there is a genuine clinical need for safe and effective PAI-1-related drugs. At the same time, the discovery of the circadian clock has deepened our understanding of circadian rhythms, but it is still challenging to transpose these discoveries into clinical practice (33).
In summary, we expect a safe and effective PAI-1-associated drug with a coordinated drug use pattern that maintains good drug concentrations when PAI-1 is expected to reach its acrophase, which might reduce the risk of cardiovascular events. Finally, considering the poor prognosis of cardiovascular events, prevention, such as regular follow-up in a plain, healthy lifestyle and good glycemic control, should also be emphasized.
YY contributed to the conception and the writing of the article. WL performed the framework. LX gave the constructive discussions to the article. YW revised important intellectual content critically for important intellectual content. All authors contributed to the article and approved the submitted version.
The authors declare that the research was conducted in the absence of any commercial or financial relationships that could be construed as a potential conflict of interest.
All claims expressed in this article are solely those of the authors and do not necessarily represent those of their affiliated organizations, or those of the publisher, the editors and the reviewers. Any product that may be evaluated in this article, or claim that may be made by its manufacturer, is not guaranteed or endorsed by the publisher.
1. Shah AD, Langenberg C, Rapsomaniki E, Denaxas S, Pujades-Rodriguez M, Gale CP, et al. Type 2 diabetes and incidence of cardiovascular diseases: A cohort study in 1·9 million people. Lancet Diabetes Endocrinol (2015) 3(2):105–13. doi: 10.1016/S2213-8587(14)70219-0
2. Cavallari I, Bhatt DL, Steg PG, Leiter LA, McGuire DK, Mosenzon O, et al. Causes and risk factors for death in diabetes: A competing-risk analysis from the SAVOR-TIMI 53 trial. J Am Coll Cardiol (2021) 77(14):1837–40. doi: 10.1016/j.jacc.2021.02.030
3. Kannel WB, D'Agostino RB, Wilson PW, Belanger AJ, Gagnon DR. Diabetes, fibrinogen, and risk of cardiovascular disease: The framingham experience. Am Heart J (1990) 120(3):672–6. doi: 10.1016/0002-8703(90)90026-T
4. Kannel WB, McGee DL. Diabetes and glucose tolerance as risk factors for cardiovascular disease: The framingham study. Diabetes Care (1979) 2(2):120–6. doi: 10.2337/diacare.2.2.120
5. Ji L, Hu D, Pan C, Weng J, Huo Y, Ma C, et al. Primacy of the 3B approach to control risk factors for cardiovascular disease in type 2 diabetes patients. Am J Med (2013) 126(10):925.e11–22. doi: 10.1016/j.amjmed.2013.02.035
6. Sarwar N, Gao P, Seshasai SR, Gobin R, Kaptoge S, Di Angelantonio E, et al. Diabetes mellitus, fasting blood glucose concentration, and risk of vascular disease: A collaborative meta-analysis of 102 prospective studies. Lancet (2010) 375(9733):2215–22. doi: 10.1016/S0140-6736(10)60484-9
7. Carrizzo A, Izzo C, Oliveti M, Alfano A, Virtuoso N, Capunzo M, et al. The main determinants of diabetes mellitus vascular complications: Endothelial dysfunction and platelet hyperaggregation. Int J Mol Sci (2018) 19(10). doi: 10.3390/ijms19102968
8. Ajjan RA, Kietsiriroje N, Badimon L, Vilahur G, Gorog DA, Angiolillo DJ, et al. Antithrombotic therapy in diabetes: Which, when, and for how long? Eur Heart J (2021) 42(23):2235–59. doi: 10.1093/eurheartj/ehab128
9. Altalhi R, Pechlivani N, Ajjan RA. PAI-1 in diabetes: Pathophysiology and role as a therapeutic target. Int J Mol Sci (2021) 22(6). doi: 10.3390/ijms22063170
10. Agren A, Jörneskog G, Elgue G, Henriksson P, Wallen H, Wiman B. Increased incorporation of antiplasmin into the fibrin network in patients with type 1 diabetes. Diabetes Care (2014) 37(7):2007–14. doi: 10.2337/dc13-1776
11. Chen YQ, Su M, Walia RR, Hao Q, Covington JW, Vaughan DE. Sp1 sites mediate activation of the plasminogen activator inhibitor-1 promoter by glucose in vascular smooth muscle cells. J Biol Chem (1998) 273(14):8225–31. doi: 10.1074/jbc.273.14.8225
12. Gettins PG, Olson ST. Inhibitory serpins. new insights into their folding, polymerization, regulation and clearance. Biochem J (2016) 473(15):2273–93. doi: 10.1042/BCJ20160014
13. Cesari M, Pahor M, Incalzi RA. Plasminogen activator inhibitor-1 (PAI-1): A key factor linking fibrinolysis and age-related subclinical and clinical conditions. Cardiovasc Ther (2010) 28(5):e72–91. doi: 10.1111/j.1755-5922.2010.00171.x
14. Schroeck F, Arroyo de Prada N, Sperl S, Schmitt M, Viktor M. Interaction of plasminogen activator inhibitor type-1 (PAI-1) with vitronectin (Vn): Mapping the binding sites on PAI-1 and vn. Biol Chem (2002) 383(7-8):1143–9. doi: 10.1515/BC.2002.125
15. Lindahl TL, Ohlsson PI, Wiman B. The mechanism of the reaction between human plasminogen-activator inhibitor 1 and tissue plasminogen activator. Biochem J (1990) 265(1):109–13. doi: 10.1042/bj2650109
16. Wagner OF, de Vries C, Hohmann C, Veerman H, Pannekoek H. Interaction between plasminogen activator inhibitor type 1 (PAI-1) bound to fibrin and either tissue-type plasminogen activator (t-PA) or urokinase-type plasminogen activator (u-PA). binding of t-PA/PAI-1 complexes to fibrin mediated by both the finger and the kringle-2 domain of t-PA. J Clin Invest (1989) 84(2):647–55. doi: 10.1172/JCI114211
17. Levi M, Biemond BJ, van Zonneveld AJ, ten Cate JW, Pannekoek H. Inhibition of plasminogen activator inhibitor-1 activity results in promotion of endogenous thrombolysis and inhibition of thrombus extension in models of experimental thrombosis. Circulation (1992) 85(1):305–12. doi: 10.1161/01.CIR.85.1.305
18. Mertens I, Van Gaal LF. Obesity, haemostasis and the fibrinolytic system. Obes Rev (2002) 3(2):85–101. doi: 10.1046/j.1467-789X.2002.00056.x
19. Scheer FA, Shea SA. Human circadian system causes a morning peak in prothrombotic plasminogen activator inhibitor-1 (PAI-1) independent of the sleep/wake cycle. Blood (2014) 123(4):590–3. doi: 10.1182/blood-2013-07-517060
20. Muller JE, Stone PH, Turi ZG, Rutherford JD, Czeisler CA, Parker C, et al. Circadian variation in the frequency of onset of acute myocardial infarction. N Engl J Med (1985) 313(21):1315–22. doi: 10.1056/NEJM198511213132103
21. Mohammad MA, Karlsson S, Haddad J, Cederberg B, Jernberg T, Lindahl B, et al. Christmas, National holidays, sport events, and time factors as triggers of acute myocardial infarction: SWEDEHEART observational study 1998-2013. Bmj (2018) 363:k4811. doi: 10.1136/bmj.k4811
22. Vitale J, Manfredini R, Gallerani M, Mumoli N, Eagle KA, Ageno W, et al. Chronobiology of acute aortic rupture or dissection: A systematic review and a meta-analysis of the literature. Chronobiol Int (2015) 32(3):385–94. doi: 10.3109/07420528.2014.983604
23. Angleton P, Chandler WL, Schmer G. Diurnal variation of tissue-type plasminogen activator and its rapid inhibitor (PAI-1). Circulation (1989) 79(1):101–6. doi: 10.1161/01.CIR.79.1.101
24. Budkowska M, Lebiecka A, Marcinowska Z, Woźniak J, Jastrzębska M, Dołęgowska B. The circadian rhythm of selected parameters of the hemostasis system in healthy people. Thromb Res (2019) 182:79–88. doi: 10.1016/j.thromres.2019.08.015
25. Pavlov M, Ćelap I. Plasminogen activator inhibitor 1 in acute coronary syndromes. Clin Chim Acta (2019) 491:52–8. doi: 10.1016/j.cca.2019.01.013
26. Gehring W, Rosbash M. The coevolution of blue-light photoreception and circadian rhythms. J Mol Evol (2003) 57(1):S286–S9. doi: 10.1007/s00239-003-0038-8
27. Asher G, Sassone-Corsi P. Time for food: The intimate interplay between nutrition, metabolism, and the circadian clock. Cell (2015) 161(1):84–92. doi: 10.1016/j.cell.2015.03.015
28. Eckel-Mahan K, Sassone-Corsi P. Metabolism and the circadian clock converge. Physiol Rev (2013) 93(1):107–35. doi: 10.1152/physrev.00016.2012
29. Zhang EE, Kay SA. Clocks not winding down: Unravelling circadian networks. Nat Rev Mol Cell Biol (2010) 11(11):764–76. doi: 10.1038/nrm2995
30. Sun H, Saeedi P, Karuranga S, Pinkepank M, Ogurtsova K, Duncan BB, et al. IDF diabetes atlas: Global, regional and country-level diabetes prevalence estimates for 2021 and projections for 2045. Diabetes Res Clin Pract (2022) 183:109119. doi: 10.1016/j.diabres.2021.109119
31. Wu H, Lau ESH, Yang A, Zhang X, Fan B, Ma RCW, et al. Age-specific population attributable risk factors for all-cause and cause-specific mortality in type 2 diabetes: An analysis of a 6-year prospective cohort study of over 360,000 people in Hong Kong. PloS Med (2023) 20(1):e1004173. doi: 10.1371/journal.pmed.1004173
32. Zhang J, Chatham JC, Young ME. Circadian regulation of cardiac physiology: Rhythms that keep the heart beating. Annu Rev Physiol (2020) 82:79–101. doi: 10.1146/annurev-physiol-020518-114349
33. Allada R, Bass J. Circadian mechanisms in medicine. New Engl J Med (2021) 384(6):550–61. doi: 10.1056/NEJMra1802337
34. Degaute JP, van de Borne P, Linkowski P, Van Cauter E. Quantitative analysis of the 24-hour blood pressure and heart rate patterns in young men. Hypertension (1991) 18(2):199–210. doi: 10.1161/01.HYP.18.2.199
35. Smolensky MH, Hermida RC, Portaluppi F. Circadian mechanisms of 24-hour blood pressure regulation and patterning. Sleep Med Rev (2017) 33:4–16. doi: 10.1016/j.smrv.2016.02.003
36. Dodt C, Breckling U, Derad I, Fehm HL, Born J. Plasma epinephrine and norepinephrine concentrations of healthy humans associated with nighttime sleep and morning arousal. Hypertension (1997) 30(1 Pt 1):71–6. doi: 10.1161/01.HYP.30.1.71
37. Rasch B, Dodt C, Mölle M, Born J. Sleep-stage-specific regulation of plasma catecholamine concentration. Psychoneuroendocrinology (2007) 32(8-10):884–91. doi: 10.1016/j.psyneuen.2007.06.007
38. Otto ME, Svatikova A, Barretto RB, Santos S, Hoffmann M, Khandheria B, et al. Early morning attenuation of endothelial function in healthy humans. Circulation (2004) 109(21):2507–10. doi: 10.1161/01.CIR.0000128207.26863.C4
39. Carmona P, Mendez N, Ili CG, Brebi P. The role of clock genes in fibrinolysis regulation: Circadian disturbance and its effect on fibrinolytic activity. Front Physiol (2020) 11:129. doi: 10.3389/fphys.2020.00129
40. Ohkura N, Oishi K, Sakata T, Kadota K, Kasamatsu M, Fukushima N, et al. Circadian variations in coagulation and fibrinolytic factors among four different strains of mice. Chronobiol Int (2007) 24(4):651–69. doi: 10.1080/07420520701534673
41. Somanath PR, Podrez EA, Chen J, Ma Y, Marchant K, Antoch M, et al. Deficiency in core circadian protein Bmal1 is associated with a prothrombotic and vascular phenotype. J Cell Physiol (2011) 226(1):132–40. doi: 10.1002/jcp.22314
42. Oishi K, Ohkura N, Yasumoto Y, Yamamoto S. Circadian fluctuations in circulating plasminogen activator inhibitor-1 are independent of feeding cycles in mice. Chronobiol Int (2017) 34(2):254–9. doi: 10.1080/07420528.2016.1256299
43. Ralph MR, Foster RG, Davis FC, Menaker M. Transplanted suprachiasmatic nucleus determines circadian period. Science (1990) 247(4945):975–8. doi: 10.1126/science.2305266
44. Gooley JJ, Lu J, Chou TC, Scammell TE, Saper CB. Melanopsin in cells of origin of the retinohypothalamic tract. Nat Neurosci (2001) 4(12):1165. doi: 10.1038/nn768
45. Welsh DK, Takahashi JS, Kay SA. Suprachiasmatic nucleus: cell autonomy and network properties. Annu Rev Physiol (2010) 72:551–77. doi: 10.1146/annurev-physiol-021909-135919
46. Allen CN, Nitabach MN, Colwell CS. Membrane currents, gene expression, and circadian clocks. Cold Spring Harb Perspect Biol (2017) 9(5). doi: 10.1101/cshperspect.a027714
47. Paul JR, DeWoskin D, McMeekin LJ, Cowell RM, Forger DB, Gamble KL. Regulation of persistent sodium currents by glycogen synthase kinase 3 encodes daily rhythms of neuronal excitability. Nat Commun (2016) 7:13470. doi: 10.1038/ncomms13470
48. Inouye ST, Kawamura H. Persistence of circadian rhythmicity in a mammalian hypothalamic "island" containing the suprachiasmatic nucleus. Proc Natl Acad Sci USA (1979) 76(11):5962–6. doi: 10.1073/pnas.76.11.5962
49. Paul JR, Davis JA, Goode LK, Becker BK, Fusilier A, Meador-Woodruff A, et al. Circadian regulation of membrane physiology in neural oscillators throughout the brain. Eur J Neurosci (2020) 51(1):109–38. doi: 10.1111/ejn.14343
50. Flourakis M, Kula-Eversole E, Hutchison AL, Han TH, Aranda K, Moose DL, et al. A conserved bicycle model for circadian clock control of membrane excitability. Cell (2015) 162(4):836–48. doi: 10.1016/j.cell.2015.07.036
51. Kuhlman SJ, McMahon DG. Rhythmic regulation of membrane potential and potassium current persists in SCN neurons in the absence of environmental input. Eur J Neurosci (2004) 20(4):1113–7. doi: 10.1111/j.1460-9568.2004.03555.x
52. Takahashi JS. Molecular architecture of the circadian clock in mammals. In: Sassone-Corsi P, Christen Y, editors. A time for metabolism and hormones. Cham (CH: Springer (2016). p. 13–24.
53. Lowrey PL, Takahashi JS. Genetics of the mammalian circadian system: Photic entrainment, circadian pacemaker mechanisms, and posttranslational regulation. Annu Rev Genet (2000) 34:533–62. doi: 10.1146/annurev.genet.34.1.533
54. Yoo SH, Mohawk JA, Siepka SM, Shan Y, Huh SK, Hong HK, et al. Competing E3 ubiquitin ligases govern circadian periodicity by degradation of CRY in nucleus and cytoplasm. Cell (2013) 152(5):1091–105. doi: 10.1016/j.cell.2013.01.055
55. Wan YZ, Gao P, Zhou S, Zhang ZQ, Hao DL, Lian LS, et al. SIRT1-mediated epigenetic downregulation of plasminogen activator inhibitor-1 prevents vascular endothelial replicative senescence. Aging Cell (2014) 13(5):890–9. doi: 10.1111/acel.12247
56. Kalsbeek A, Bruinstroop E, Yi CX, Klieverik LP, La Fleur SE, Fliers E. Hypothalamic control of energy metabolism via the autonomic nervous system. Ann N Y Acad Sci (2010) 1212:114–29. doi: 10.1111/j.1749-6632.2010.05800.x
57. Mohawk JA, Green CB, Takahashi JS. Central and peripheral circadian clocks in mammals. Annu Rev Neurosci (2012) 35:445–62. doi: 10.1146/annurev-neuro-060909-153128
58. Jiang Q, Liu H, Wang S, Wang J, Tang Y, He Z, et al. Circadian locomotor output cycles kaput accelerates atherosclerotic plaque formation by upregulating plasminogen activator inhibitor-1 expression. Acta Biochim Biophys Sinica (2018) 50(9):869–79. doi: 10.1093/abbs/gmy087
59. Aso Y, Matsumoto S, Fujiwara Y, Tayama K, Inukai T, Takemura Y. Impaired fibrinolytic compensation for hypercoagulability in obese patients with type 2 diabetes: Association with increased plasminogen activator inhibitor-1. Metabolism (2002) 51(4):471–6. doi: 10.1053/meta.2002.31334
60. Pandolfi A, Iacoviello L, Capani F, Vitacolonna E, Donati MB, Consoli A. Glucose and insulin independently reduce the fibrinolytic potential of human vascular smooth muscle cells in culture. Diabetologia (1996) 39(12):1425–31. doi: 10.1007/s001250050594
61. Schneider DJ, Nordt TK, Sobel BE. Stimulation by proinsulin of expression of plasminogen activator inhibitor type-I in endothelial cells. Diabetes (1992) 41(7):890–5. doi: 10.2337/diab.41.7.890
62. Aquila S, Gentile M, Middea E, Catalano S, Morelli C, Pezzi V, et al. Leptin secretion by human ejaculated spermatozoa. J Clin Endocrinol Metab (2005) 90(8):4753–61. doi: 10.1210/jc.2004-2233
63. Cusi K, Maezono K, Osman A, Pendergrass M, Patti ME, Pratipanawatr T, et al. Insulin resistance differentially affects the PI 3-kinase- and MAP kinase-mediated signaling in human muscle. J Clin Invest (2000) 105(3):311–20. doi: 10.1172/JCI7535
64. Montagnani M, Golovchenko I, Kim I, Koh GY, Goalstone ML, Mundhekar AN, et al. Inhibition of phosphatidylinositol 3-kinase enhances mitogenic actions of insulin in endothelial cells. J Biol Chem (2002) 277(3):1794–9. doi: 10.1074/jbc.M103728200
65. Dresner A, Laurent D, Marcucci M, Griffin ME, Dufour S, Cline GW, et al. Effects of free fatty acids on glucose transport and IRS-1-associated phosphatidylinositol 3-kinase activity. J Clin Invest (1999) 103(2):253–9. doi: 10.1172/JCI5001
66. Griffin ME, Marcucci MJ, Cline GW, Bell K, Barucci N, Lee D, et al. Free fatty acid-induced insulin resistance is associated with activation of protein kinase c theta and alterations in the insulin signaling cascade. Diabetes (1999) 48(6):1270–4. doi: 10.2337/diabetes.48.6.1270
67. Shulman GI. Ectopic fat in insulin resistance, dyslipidemia, and cardiometabolic disease. New Engl J Med (2014) 371(12):1131–41. doi: 10.1056/NEJMra1011035
68. Chen Y, Billadello JJ, Schneider DJ. Identification and localization of a fatty acid response region in the human plasminogen activator inhibitor-1 gene. Arterioscler Thromb Vasc Biol (2000) 20(12):2696–701. doi: 10.1161/01.ATV.20.12.2696
69. Banfi C, Mussoni L, Risé P, Cattaneo MG, Vicentini L, Battaini F, et al. Very low density lipoprotein-mediated signal transduction and plasminogen activator inhibitor type 1 in cultured HepG2 cells. Circ Res (1999) 85(2):208–17. doi: 10.1161/01.RES.85.2.208
70. Sobel BE, Woodcock-Mitchell J, Schneider DJ, Holt RE, Marutsuka K, Gold H. Increased plasminogen activator inhibitor type 1 in coronary artery atherectomy specimens from type 2 diabetic compared with nondiabetic patients: A potential factor predisposing to thrombosis and its persistence. Circulation (1998) 97(22):2213–21. doi: 10.1161/01.CIR.97.22.2213
71. Fuchs A, Samovski D, Smith GI, Cifarelli V, Farabi SS, Yoshino J, et al. Associations among adipose tissue immunology, inflammation, exosomes and insulin sensitivity in people with obesity and nonalcoholic fatty liver disease. Gastroenterology (2021) 161(3):968–+. doi: 10.1053/j.gastro.2021.05.008
72. Thosar SS, Butler MP, Shea SA. Role of the circadian system in cardiovascular disease. J Clin Invest (2018) 128(6):2157–67. doi: 10.1172/JCI80590
73. Johansson L, Jansson JH, Boman K, Nilsson TK, Stegmayr B, Hallmans G. Tissue plasminogen activator, plasminogen activator inhibitor-1, and tissue plasminogen activator/plasminogen activator inhibitor-1 complex as risk factors for the development of a first stroke. Stroke (2000) 31(1):26–32. doi: 10.1161/01.STR.31.1.26
74. Jung RG, Simard T, Labinaz A, Ramirez FD, Di Santo P, Motazedian P, et al. Role of plasminogen activator inhibitor-1 in coronary pathophysiology. Thromb Res (2018) 164:54–62. doi: 10.1016/j.thromres.2018.02.135
75. Placencio VR, DeClerck YA. Plasminogen activator inhibitor-1 in cancer: Rationale and insight for future therapeutic testing. Cancer Res (2015) 75(15):2969–74. doi: 10.1158/0008-5472.CAN-15-0876
76. Vaughan DE, Rai R, Khan SS, Eren M, Ghosh AK. Plasminogen activator inhibitor-1 is a marker and a mediator of senescence. Arterioscler Thromb Vasc Biol (2017) 37(8):1446–52. doi: 10.1161/ATVBAHA.117.309451
77. Flevaris P, Vaughan D. The role of plasminogen activator inhibitor type-1 in fibrosis. Semin Thromb Hemost (2017) 43(2):169–77. doi: 10.1055/s-0036-1586228
78. Simone TM, Longmate WM, Law BK, Higgins PJ. Targeted inhibition of PAI-1 activity impairs epithelial migration and wound closure following cutaneous injury. Adv Wound Care (New Rochelle) (2015) 4(6):321–8. doi: 10.1089/wound.2014.0611
79. Fortenberry YM. Plasminogen activator inhibitor-1 inhibitors: A patent review (2006-present). Expert Opin Ther Pat (2013) 23(7):801–15. doi: 10.1517/13543776.2013.782393
80. Rouch A, Vanucci-Bacqué C, Bedos-Belval F, Baltas M. Small molecules inhibitors of plasminogen activator inhibitor-1 - an overview. Eur J Med Chem (2015) 92:619–36. doi: 10.1016/j.ejmech.2015.01.010
81. Khan SS, Shah SJ, Klyachko E, Baldridge AS, Eren M, Place AT, et al. A null mutation in SERPINE1 protects against biological aging in humans. Sci Adv (2017) 3(11):eaao1617. doi: 10.1126/sciadv.aao1617
82. Miyazaki H, Sai H, Ohmizu H, Murakami J, Ohtani A, Ogiku T. Synthesis and evaluation of 1,4-diphenylbutadiene derivatives as inhibitors of plasminogen activator inhibitor-1 (PAI-1) production. Bioorg Med Chem (2010) 18(5):1968–79. doi: 10.1016/j.bmc.2010.01.032
83. Miyazaki H, Ogiku T, Sai H, Ohmizu H, Murakami J, Ohtani A. Design, synthesis, and evaluation of orally active inhibitors of plasminogen activator inhibitor-1 (PAI-1) production. Bioorg Med Chem Lett (2008) 18(24):6419–22. doi: 10.1016/j.bmcl.2008.10.067
84. Biemond BJ, Levi M, Coronel R, Janse MJ, ten Cate JW, Pannekoek H. Thrombolysis and reocclusion in experimental jugular vein and coronary artery thrombosis. effects of a plasminogen activator inhibitor type 1-neutralizing monoclonal antibody. Circulation (1995) 91(4):1175–81. doi: 10.1161/01.CIR.91.4.1175
85. Vousden KA, Lundqvist T, Popovic B, Naiman B, Carruthers AM, Newton P, et al. Discovery and characterisation of an antibody that selectively modulates the inhibitory activity of plasminogen activator inhibitor-1. Sci Rep (2019) 9(1):1605. doi: 10.1038/s41598-019-38842-x
86. Elokdah H, Abou-Gharbia M, Hennan JK, McFarlane G, Mugford CP, Krishnamurthy G, et al. Tiplaxtinin, a novel, orally efficacious inhibitor of plasminogen activator inhibitor-1: Design, synthesis, and preclinical characterization. J Med Chem (2004) 47(14):3491–4. doi: 10.1021/jm049766q
87. Gorlatova NV, Cale JM, Elokdah H, Li D, Fan K, Warnock M, et al. Mechanism of inactivation of plasminogen activator inhibitor-1 by a small molecule inhibitor. J Biol Chem (2007) 282(12):9288–96. doi: 10.1074/jbc.M611642200
88. Pawlowska Z, Chabielska E, Kobylanska A, Maciaszek A, Swiatkowska M, Buczko W, et al. Regulation of PAI-1 concentration in platelets by systemic administration of antisense oligonucleotides to rats. Thromb Haemost (2001) 85(6):1086–9. doi: 10.1055/s-0037-1615968
89. Huang WT, Vayalil PK, Miyata T, Hagood J, Liu RM. Therapeutic value of small molecule inhibitor to plasminogen activator inhibitor-1 for lung fibrosis. Am J Respir Cell Mol Biol (2012) 46(1):87–95. doi: 10.1165/rcmb.2011-0139OC
90. Cale JM, Li SH, Warnock M, Su EJ, North PR, Sanders KL, et al. Characterization of a novel class of polyphenolic inhibitors of plasminogen activator inhibitor-1. J Biol Chem (2010) 285(11):7892–902. doi: 10.1074/jbc.M109.067967
91. Jain SK, Nagi DK, Slavin BM, Lumb PJ, Yudkin JS. Insulin therapy in type 2 diabetic subjects suppresses plasminogen activator inhibitor (PAI-1) activity and proinsulin-like molecules independently of glycaemic control. Diabetes Med (1993) 10(1):27–32. doi: 10.1111/j.1464-5491.1993.tb01992.x
92. Kruszynska YT, Yu JG, Olefsky JM, Sobel BE. Effects of troglitazone on blood concentrations of plasminogen activator inhibitor 1 in patients with type 2 diabetes and in lean and obese normal subjects. Diabetes (2000) 49(4):633–9. doi: 10.2337/diabetes.49.4.633
93. Nagi DK, Ali VM, Yudkin JS. Effect of metformin on intact proinsulin and des 31,32 proinsulin concentrations in subjects with non-insulin-dependent (type 2) diabetes mellitus. Diabetes Med (1996) 13(8):753–7. doi: 10.1002/(SICI)1096-9136(199608)13:8<753::AID-DIA163>3.0.CO;2-Z
94. Liu H, Dear AE, Knudsen LB, Simpson RW. A long-acting glucagon-like peptide-1 analogue attenuates induction of plasminogen activator inhibitor type-1 and vascular adhesion molecules. J Endocrinol (2009) 201(1):59–66. doi: 10.1677/JOE-08-0468
95. Brown NJ, Agirbasli MA, Williams GH, Litchfield WR, Vaughan DE. Effect of activation and inhibition of the renin-angiotensin system on plasma PAI-1. Hypertension (1998) 32(6):965–71. doi: 10.1161/01.HYP.32.6.965
96. Zirlik A, Ernst S, Leugers A, Willecke F, Sobel BE, Bode C, et al. Inhibition by fibrates of plasminogen activator inhibitor type-1 expression in human adipocytes and preadipocytes. Thromb Haemost (2009) 101(6):1060–9.
97. Cesari M, Kritchevsky SB, Atkinson HH, Penninx BW, Di Bari M, Tracy RP, et al. Angiotensin-converting enzyme inhibition and novel cardiovascular risk biomarkers: Results from the trial of angiotensin converting enzyme inhibition and novel cardiovascular risk factors (TRAIN) study. Am Heart J (2009) 157(2):334.e1–8. doi: 10.1016/j.ahj.2008.10.026
98. Whyte CS, Simpson M, Morrow GB, Wallace CA, Mentzer AJ, Knight JC, et al. The suboptimal fibrinolytic response in COVID-19 is dictated by high PAI-1. J Thromb Haemost (2022) 20(10):2394–406. doi: 10.1111/jth.15806
99. Xi X, Liu N, Wang Q, Chu Y, Yin Z, Ding Y, et al. ACT001, a novel PAI-1 inhibitor, exerts synergistic effects in combination with cisplatin by inhibiting PI3K/AKT pathway in glioma. Cell Death Dis (2019) 10(10):757. doi: 10.1038/s41419-019-1986-2
100. Levi F, Schibler U. Circadian rhythms: Mechanisms and therapeutic implications. Annu Rev Pharmacol Toxicol (2007) 47:593–628. doi: 10.1146/annurev.pharmtox.47.120505.105208
101. Ayyar VS, Sukumaran S. Circadian rhythms: influence on physiology, pharmacology, and therapeutic interventions. J Pharmacokinet Pharmacodyn (2021) 48(3):321–38. doi: 10.1007/s10928-021-09751-2
102. Ruan W, Yuan X, Eltzschig HK. Circadian rhythm as a therapeutic target. Nat Rev Drug Discov (2021) 20(4):287–307. doi: 10.1038/s41573-020-00109-w
103. Sica D, Frishman WH, Manowitz N. Pharmacokinetics of propranolol after single and multiple dosing with sustained release propranolol or propranolol CR (innopran XL), a new chronotherapeutic formulation. Heart Dis (2003) 5(3):176–81. doi: 10.1097/01.HDX.0000074436.09658.3b
104. Hermida RC, Ayala DE, Mojón A, Fernández JR. Influence of time of day of blood pressure-lowering treatment on cardiovascular risk in hypertensive patients with type 2 diabetes. Diabetes Care (2011) 34(6):1270–6. doi: 10.2337/dc11-0297
105. Gami AS, Howard DE, Olson EJ, Somers VK. Day-night pattern of sudden death in obstructive sleep apnea. N Engl J Med (2005) 352(12):1206–14. doi: 10.1056/NEJMoa041832
106. Arendt J, Bojkowski C, Franey C, Wright J, Marks V. Immunoassay of 6-hydroxymelatonin sulfate in human plasma and urine: Abolition of the urinary 24-hour rhythm with atenolol. J Clin Endocrinol Metab (1985) 60(6):1166–73. doi: 10.1210/jcem-60-6-1166
107. Scheer FA, Stone PH, Shea SA. Decreased sleep in heart failure: Are medications to blame? Arch Intern Med (2007) 167(10):1098–9. doi: 10.1001/archinte.167.10.1098-b
Keywords: plasminogen activator inhibitor-1, circadian rhythm, cardiovascular complications, type 2 diabetes, pharmaceutical development
Citation: Yu Y, Li W, Xu L and Wang Y (2023) Circadian rhythm of plasminogen activator inhibitor-1 and cardiovascular complications in type 2 diabetes. Front. Endocrinol. 14:1124353. doi: 10.3389/fendo.2023.1124353
Received: 15 December 2022; Accepted: 27 February 2023;
Published: 20 March 2023.
Edited by:
Sarah Costantino, University of Zurich, SwitzerlandReviewed by:
Andrey Panchenko, Research Institute of Medical Primatology, RussiaCopyright © 2023 Yu, Li, Xu and Wang. This is an open-access article distributed under the terms of the Creative Commons Attribution License (CC BY). The use, distribution or reproduction in other forums is permitted, provided the original author(s) and the copyright owner(s) are credited and that the original publication in this journal is cited, in accordance with accepted academic practice. No use, distribution or reproduction is permitted which does not comply with these terms.
*Correspondence: Yangang Wang, d2FuZ3lnMTk2NkAxMjYuY29t
Disclaimer: All claims expressed in this article are solely those of the authors and do not necessarily represent those of their affiliated organizations, or those of the publisher, the editors and the reviewers. Any product that may be evaluated in this article or claim that may be made by its manufacturer is not guaranteed or endorsed by the publisher.
Research integrity at Frontiers
Learn more about the work of our research integrity team to safeguard the quality of each article we publish.