- 1Department of Biological Sciences, University of Cincinnati, Cincinnati, OH, United States
- 2School of Medicine and Health Sciences TecSalud Instituto Tecnológico y de Estudios Superiores de Monterrey (ITESM), Monterrey, Nuevo Leon, Mexico
Corticosteroids are critical for development and for mediating stress responses across diverse vertebrate taxa. Study of frog metamorphosis has made significant breakthroughs in our understanding of corticosteroid signaling during development in non-mammalian vertebrate species. However, lack of adequate corticosterone (CORT) response genes in tadpoles make identification and quantification of CORT responses challenging. Here, we characterized a CORT-response gene frzb (frizzled related protein) previously identified in Xenopus tropicalis tadpole tail skin by an RNA-seq study. We validated the RNA-seq results that CORT and not thyroid hormone induces frzb in the tails using quantitative PCR. Further, maximum frzb expression was achieved by 100-250 nM CORT within 12-24 hours. frzb is not significantly induced in the liver and brain in response to 100 nM CORT. We also found no change in frzb expression across natural metamorphosis when endogenous CORT levels peak. Surprisingly, frzb is only induced by CORT in X. tropicalis tails and not in Xenopus laevis tails. The exact downstream function of increased frzb expression in tails in response to CORT is not known, but the specificity of hormone response and its high mRNA expression levels in the tail render frzb a useful marker of exogenous CORT-response independent of thyroid hormone for exogenous hormone treatments and in-vivo endocrine disruption studies.
1 Introduction
Glucocorticoids (also known as stress hormones in vertebrates) dictate multiple events in vertebrate development including organ maturation and time of birth (1, 2). Lack of glucocorticoids during development can cause death at birth while excessive fetal exposure to glucocorticoids (from exogenous replacements or endogenous stress) can cause debilitating metabolic and neurological disorders later in adulthood (3–5). Glucocorticoids affect development directly and also indirectly by interacting with other hormones necessary for normal fetal development, such as thyroid hormone, insulin, and leptin (6–8). Although the indispensable role of glucocorticoids in vertebrate development has been known for decades, the molecular mechanisms underlying tissue-specific signaling pathways during direct and indirect actions of glucocorticoids on development are not well understood in vertebrates.
Amphibian metamorphosis is an exemplary model to investigate hormonal control of development in vertebrates due to conservation of thyroid and stress hormone signaling pathways between humans and frogs and due to their extreme dependence on both hormones for survival through metamorphosis (9–12). While much information about CORT (corticosterone, the main glucocorticoid in amphibians) signaling during frog metamorphosis has been obtained from gene knockout models, one of the main challenges of isolating and analyzing CORT responses in tadpoles is the lack of adequate and appropriate CORT response genes (13–15). The process of measuring CORT levels in the tissue or plasma is cumbersome and does not show whether altered CORT levels are having a physiological effect on tissues. Quantifying altered expression of a CORT-response gene provides an efficient and convenient way to assess CORT responsivity in tissues.
Previous studies have examined several CORT-response genes in tadpoles (16, 17). klf9 is a well-established CORT-response gene, but it is also independently regulated by thyroid hormone (TH) (18, 19). Six CORT-response genes selected from a microarray study were used to establish a screening method for glucocorticoid endocrine disruption, but induction by thyroid hormone (TH) was not evaluated (20, 21). Corticotropin releasing hormone (CRH) is a common secretagogue for both CORT and TH in tadpoles, hence both CORT and TH levels increase during stress responses and during natural metamorphosis, such that a measure of increased klf9 does not distinguish between a response due to CORT, TH, or both (22, 23). ush1g was identified in a microarray study as responsive to CORT, and its expression was subsequently characterized in tadpoles during development (17, 21). ush1g is induced by CORT and not TH and was thus considered to be a “CORT-only” gene, making it the only known specific marker of CORT action in tadpoles. However, although ush1g has a high fold-induction in response to exogenous CORT, change in ush1g expression is inconsistent among experiments during natural metamorphosis, which makes it an unreliable indicator of endogenous CORT responsiveness in tissues (13, 15).
In the current study, we have characterized frizzled related protein (frzb), which was identified as a CORT-only response gene in a global gene expression study where tadpole tails were treated in vivo and in vitro with vehicle, CORT, TH, and CORT+TH for 24 hours (24). Using Xenopus tropicalis and X. laevis, we validated the hormonal regulation of frzb by CORT and TH, quantified frzb expression in multiple tissues, identified the dose of CORT and duration of CORT treatment required for optimal frzb expression in tails, and measured it during natural metamorphosis. We also identified which corticosteroid receptor (glucocorticoid receptor, GR, and/or mineralocorticoid receptor, MR) is required for frzb expression.
2 Materials and methods
2.1 Animal husbandry
Lab reared, glucocorticoid receptor knockout (GRKO) X. tropicalis heterozygous mutants and wild-type Xenopus tropicalis adult male and female frogs were primed with 20IU of human chorionic gonadotropin (Sigma-Aldrich) in the evening and boosted with 200IU the following morning for breeding. Wild-type Xenopus laevis adult male and female frogs were injected with human chorionic gonadotropin (Sigma-Aldrich) at doses of 200IU and 600IU, respectively in the evening. Resulting tadpoles were reared at 26 degrees Celsius in reconstituted reverse osmosis water with water changes every 3 days, and tadpoles were fed powdered fry food (Sera Micron Nature) twice daily. Tadpoles from adult GR heterozygous crosses were genotyped into wild-type and GRKO homozygous mutants using a previously established heteroduplex mobility assay (13, 25). The use of animals in experiments was approved by the University of Cincinnati Institutional Animal Care and Use Committee (IACUC protocol # 21-06-21-01).
2.2 Selection of CORT-response genes
Buisine et al. (24) conducted global RNA-seq on tailfin skin of premetamorphic Xenopus tropicalis tadpoles treated with vehicle, 100 nM CORT, 10 nM triiodothyronine (T3), and CORT+T3. From their supplementary data, we selected genes that were significantly upregulated by CORT and CORT+T3 by 2-fold but were not induced by T3, resulting in identification of 7 genes that fit these criteria.
2.3 Hormone treatments and tissue harvest for gene expression
To identify gene(s) (out of 7 selected) with the highest fold change in response to CORT, we treated wild-type X. tropicalis premetamorphic tadpoles at Nieuwkoop and Faber (NF) 54 with 100 nM CORT and vehicle (ethanol) for 24 hours (26). To verify specificity of hormonal regulation by CORT and TH, wild-type X. tropicalis premetamorphic tadpoles (NF54) (n=10) were treated with vehicle (ethanol), 100 nM CORT, 10 nM T3, and 100 nM CORT plus 10 nM T3 by addition into the aquarium water. For the dose response experiment, NF54 WT X. tropicalis tadpoles were treated with vehicle, 50, 100, 250, and 500 nM CORT for 24 hours. For the time course, NF54 wild-type X. tropicalis tadpoles (n=10) were treated with 100 nM CORT for 0, 3, 6, 12, 24, and 48 hours. Water changes and hormone replacements were conducted daily. To investigate if GR is necessary for gene induction, we treated wild-type and GRKO NF54 X. tropicalis tadpoles with vehicle or 100 nM CORT. Control groups were treated with ethanol because most steroid hormones (including CORT) are dissolved in 100% ethanol. Hence, treating the control group with ethanol would exhibit possible effects of ethanol (if any) on gene expression in the tadpoles across all treatments. The treatment/hormone groups would then only demonstrate effect of CORT/TH treatment on gene expression. Tails (n = 10 per treatment) were harvested from MS-222-anesthetized tadpoles, snap frozen, and stored at -80 degrees Celsius until RNA isolation. To compare responsivity among tissues, brains (fore- plus midbrain portion including pituitary) and livers (n=10) from vehicle and CORT (100 nM) treated tadpoles were dissected (27) and stored as above. To determine gene expression during natural metamorphosis, tails (n=10) were harvested from tadpoles at premetamorphosis (NF54), pro-metamorphosis (NF58) and metamorphic climax (NF62) and stored as above.
2.4 Gene expression
RNA was extracted from frozen tissues using TRI REAGENT RT (Molecular Research Center, Inc., Cincinnati, OH) according to manufacturer’s instructions. Complementary DNA (cDNA) for each sample was synthesized from 1000 ng total RNA using the High- Capacity cDNA reverse transcription kit (Applied Biosystems). Quantitative PCR (qPCR) using 5uL of diluted cDNA was carried out using SYBR green master mix on a 7300 Real Time PCR System (Applied Biosystems) with gene-specific primers for camta1, frzb, grpel1, klf9, musk, rpl8, sds, sult6b1, ush1g, and usp2 (Table 1). The reference gene ribosomal protein L8 (rpl8) was used and showed no significant differences among genotypes or treatments (data not shown). The relative quantification method ΔΔCt was used to compare expression levels of target genes normalized to the reference gene ribosomal protein L8 (rpl8) (28, 29).
2.5 Statistical analysis
Data were checked for normal distribution using Shapiro Wilk test of normality. For normally distributed data, unpaired Student’s t-tests, and full-factorial ANOVA were performed with base R (30). For data which did not follow normal distribution, non-parametric Kruskal-Wallis tests were conducted in R followed by pairwise comparisons using Wilcoxon rank sum exact tests. A p-value less than 0.05 was considered statistically significant.
3 Results
3.1 Selection of CORT-only genes and hormone specificity
We selected 7 genes induced by CORT and not by thyroid hormone (TH), i.e., “CORT-only” genes, from a previous RNA-seq study on premetamorphic tadpole tailfin skin (see Methods) (24), namely calmodulin binding transcription activator 1 (camta1), frizzled related protein (frzb), GrpE like 1, mitochondrial (grpel1), muscle associated receptor tyrosine kinase (musk), serine dehydratase (sds), sulfotransferase family 6B member 1 (sult6b1), and ubiquitin specific peptidase 2 (usp2), and retested their induction by CORT using quantitative PCR. Six of the seven genes, camta1, frzb, grpel1, musk, sds, and usp2, exhibited significantly induced mRNA levels in response to 100 nM CORT in the tails (Figure 1). We chose frzb for further validation and characterization due to high fold change (~8 fold) as compared to camta1 (~2.3 fold), grpel1 (~3 fold), musk (~2.5 fold), sds (~2 fold) and usp2 (~1.6 fold) (Figure 1). To validate frzb as a CORT-only gene, we measured mRNA expression levels in response to thyroid hormone (TH). frzb was significantly induced by CORT and by CORT+TH but not by TH alone (Figure 2).
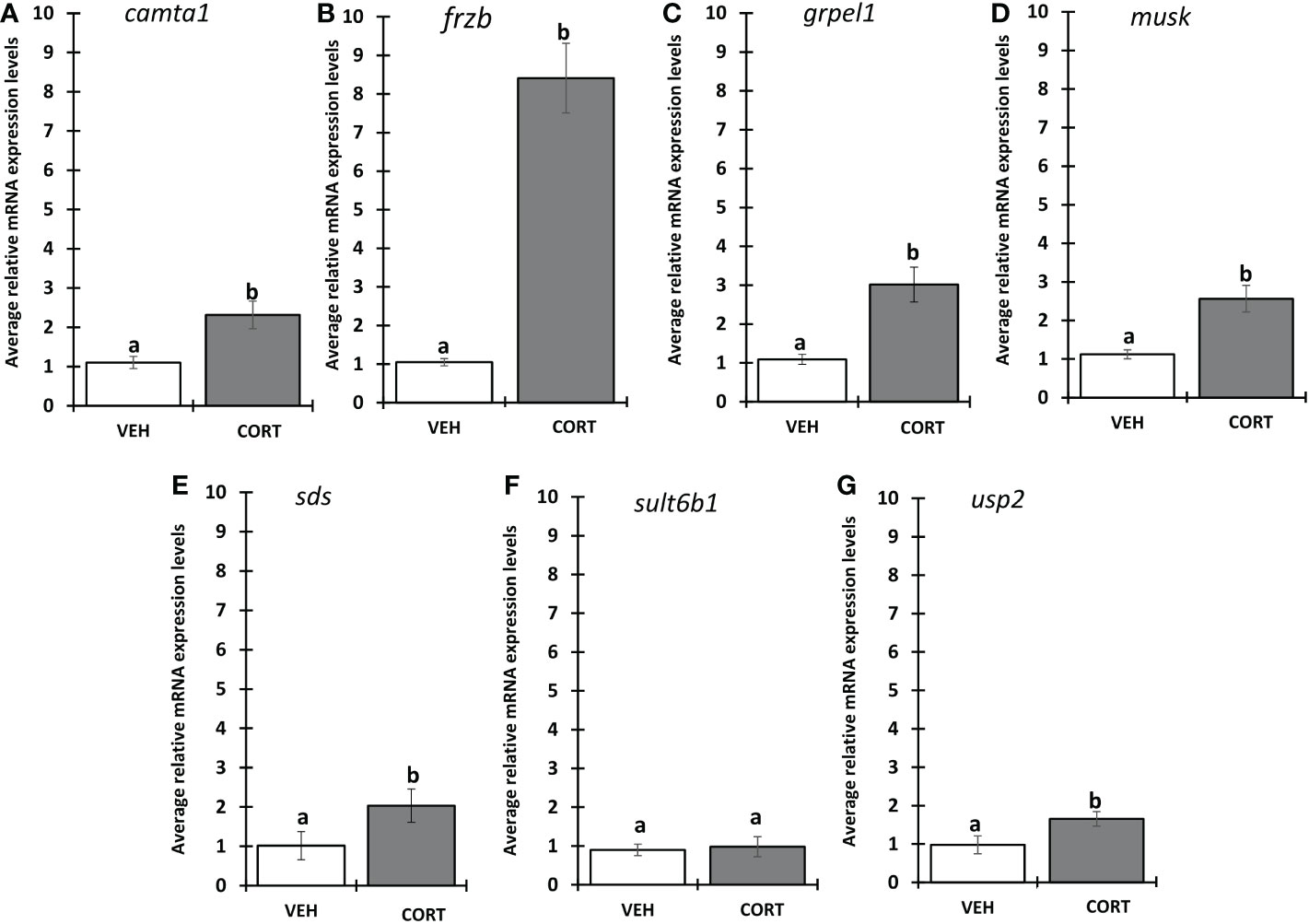
Figure 1 Evaluation of CORT response in selected genes. Premetamorphic tadpoles at Nieuwkoop and Faber stage 54 (NF54) were treated with vehicle control or 100 nM CORT (corticosterone) for 24 hours followed by tail RNA extraction and quantitative PCR to quantify mRNA expression using gene specific primers for (A) calmodulin binding transcription activator 1 (camta1), (B) frizzled related protein (frzb), (C) GrpE like 1, mitochondrial (grpel1) (D) muscle associated receptor tyrosine kinase (musk), (E) serine dehydratase (sds), (F) sulfotransferase family 6B member 1 (sult6b1), and (G) ubiquitin specific peptidase 2 (usp2). Bars represent mean mRNA levels relative to the housekeeping gene rpl8 and normalized to a vehicle control sample. n = 10 tail samples per treatment. Error bars represent SE. Letters indicate significant groups, p < 0.05.
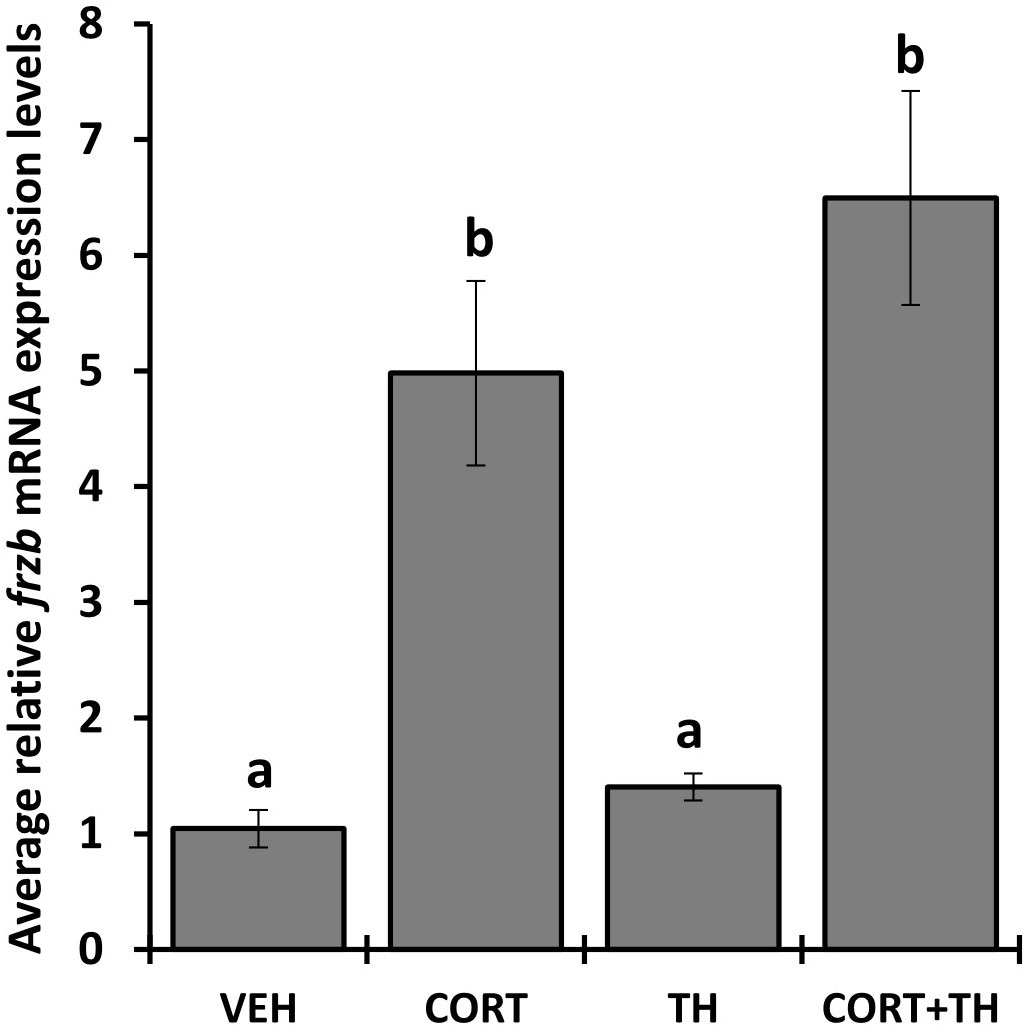
Figure 2 Hormone induction specificity of frzb in tadpole tails. Premetamorphic tadpoles (NF 54) were treated with vehicle, 100 nM CORT (corticosterone), 10 nM T3 (triiodothyronine), and CORT+T3 for 24 h. Tails were dissected, followed by RNA extraction and quantitative PCR for frzb and the housekeeping gene rpl8. Bars represent mean mRNA levels relative to rpl8 and normalized to a vehicle control sample. n = 10 tail samples per treatment. Error bars represent SE. Letters indicate significant groups, p < 0.05.
3.2 Tissue distribution, developmental profile, and receptor specific expression of frzb
To assess the ability to use frzb to quantify tissue-specific CORT responses in tadpoles, we measured frzb induction after CORT treatment among tissues. When NF54 tadpoles were treated with 100 nM CORT for 24 hours, only tails and not livers and brains demonstrated a significant increase in frzb expression levels (Figures 3A–C). We then measured frzb expression levels just in tails during natural metamorphosis where endogenous CORT levels peak at the climax of metamorphosis. Even though klf9 was highly induced at NF 62 (Figure 4A), we found that frzb expression did not change significantly during natural metamorphosis (Figure 4B). The only previously known CORT-only gene ush1g also was not induced during natural metamorphosis (Figure 4C). Receptor specificity of CORT-response genes is important for many types of studies, and so far, all known CORT-response genes are induced by GR only (i.e., not by mineralocorticoid receptor). Here, we determined if GR is required for frzb expression. Upon CORT treatment, tails from wild-type tadpoles exhibited significantly higher frzb expression, but tails from CORT-treated GR knockout tadpoles showed that frzb expression was not significantly different from vehicle-treated wild-type or GR knockout tails (Figure 5).
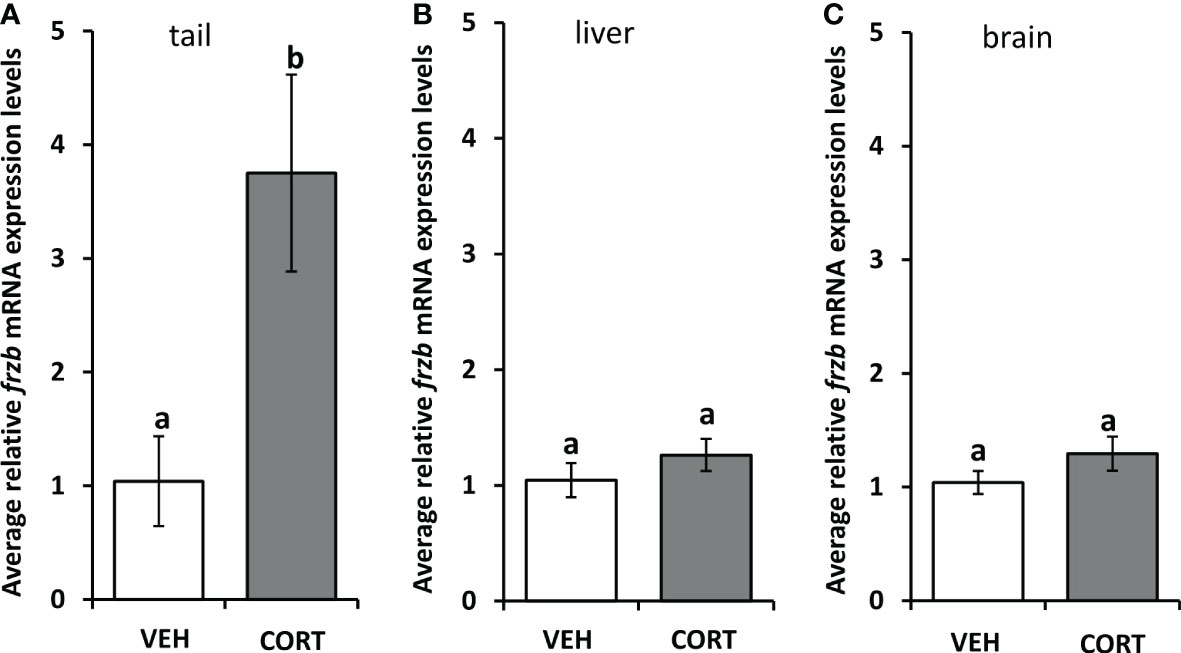
Figure 3 Tissue expression profile of frzb in tadpoles. Premetamorphic tadpoles (NF 54) were treated with vehicle and 100 nM CORT (corticosterone) for 24 hours. Tails (A), livers (B), and brains (C) were dissected, followed by RNA extraction and quantitative PCR to measure mRNA expression of frzb and the housekeeping gene rpl8. Bars represent mean mRNA levels relative to rpl8 and normalized to a vehicle control sample. n = 10 tail samples per treatment. Error bars represent SE. Letters indicate significant groups, p < 0.05.
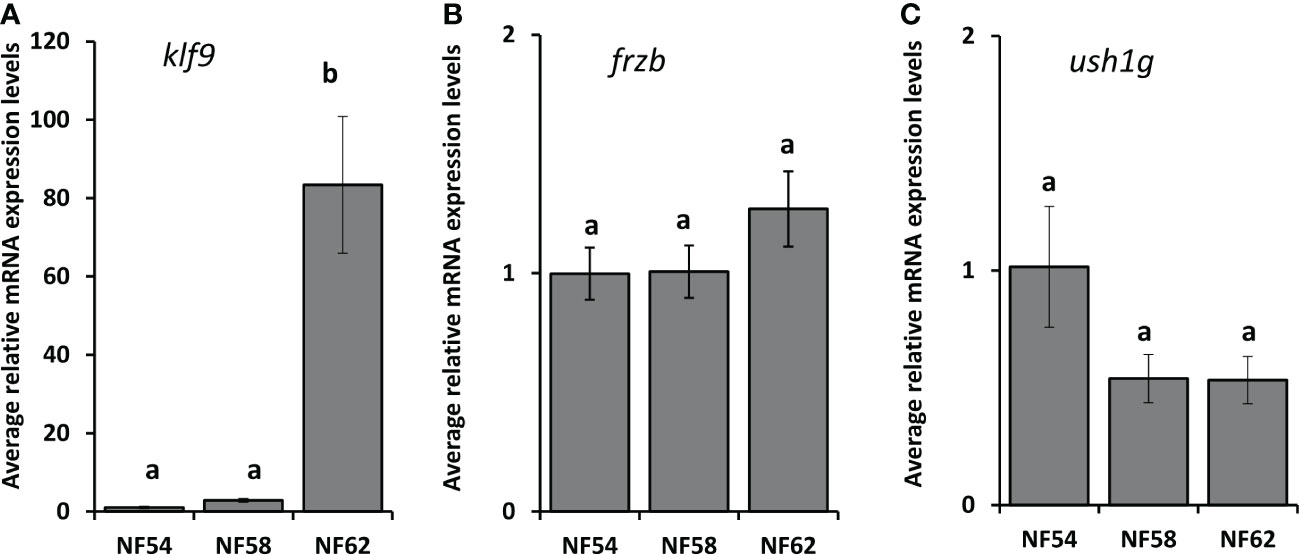
Figure 4 Developmental expression profile of klf9, frzb, and ush1g in tadpole tails throughout metamorphosis. Tails from tadpoles at the indicated NF stages were harvested, followed by tail RNA extraction and quantitative PCR to measure mRNA expression levels of klf9 (A), frzb (B), ush1g (C) and the housekeeping gene rpl8. Bars represent mean mRNA levels relative to rpl8 and normalized to a vehicle control sample. n = 10 tail samples per treatment. Error bars represent SE. Letters indicate significant groups, p < 0.05.
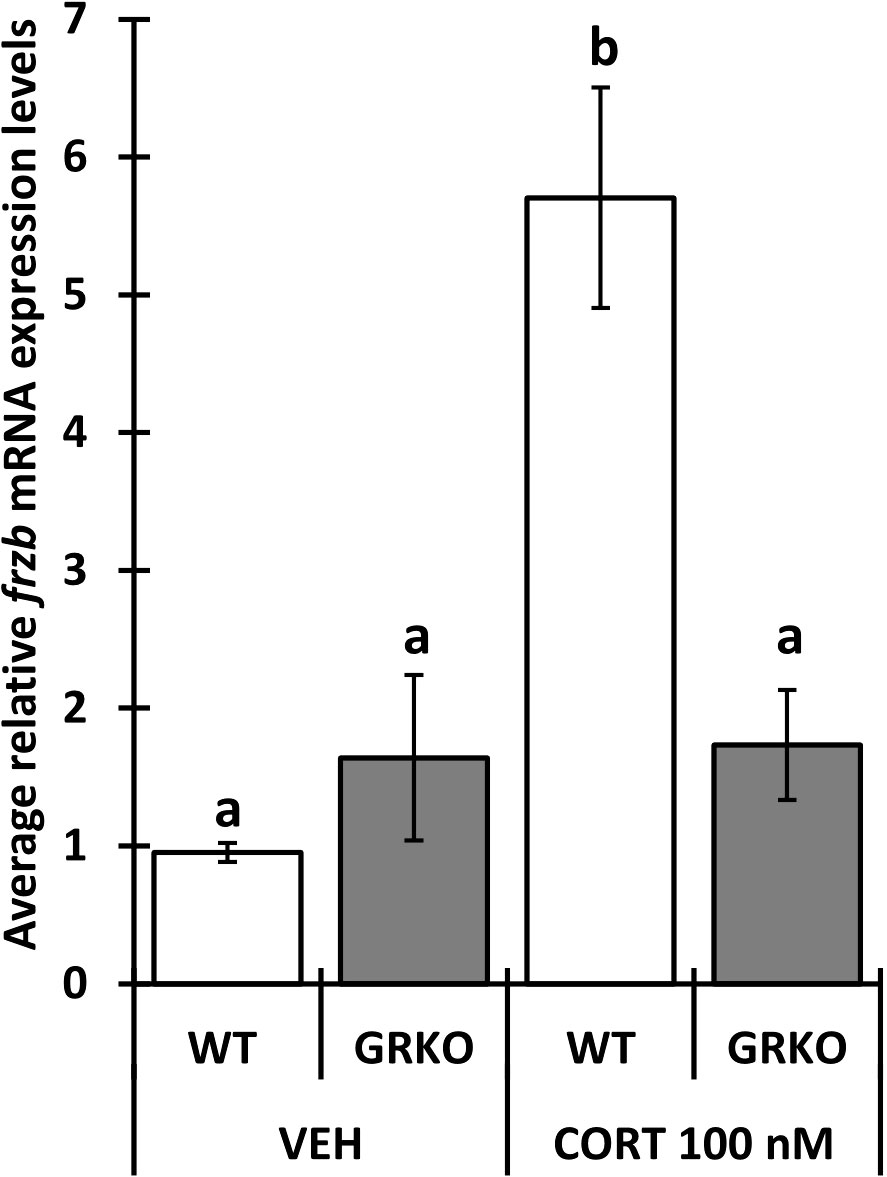
Figure 5 Impaired frzb induction in glucocorticoid receptor knockout (GRKO) tails. Premetamorphic wild-type and GRKO tadpoles (NF 54) were treated with vehicle control or 100 nM CORT (corticosterone) for 24 hours followed by tail RNA extraction and quantitative PCR to quantify mRNA expression of frzb and the housekeeping gene rpl8. Bars represent mean mRNA levels relative to rpl8 and normalized to a vehicle control sample. n = 10 tail samples per treatment. Error bars represent SE. Letters indicate significant groups, p < 0.05.
3.3 Dose response and time course of frzb induction in tails
To determine the dose of CORT required for maximum induction of frzb, we measured frzb mRNA expression in NF54 tadpole tails after treating with vehicle (ethanol), 50, 100, 250 and 500 nM CORT for 24 hours (Figures 6A–C). frzb was significantly induced at 50 nM CORT, but the highest fold change (~7 times) occurred in the 100 nM CORT treatment (Figure 6B). The induction of frzb by higher doses of CORT showed decreasing levels with increasing doses of treatment indicating an inverted “U-shaped” dose response curve (Figure 6B). The other two CORT-response genes klf9 and ush1g showed similar dose response curves (Figures 6A, C). To determine the duration of CORT treatment which results in highest mRNA expression levels of frzb, we measured frzb expression in NF54 tadpole tails after treating with 100 nM CORT for 0, 3, 6, 12, 24, and 48 hours (Figures 6D–F). frzb was significantly induced at 6 hrs. after CORT treatment, but the highest expression level occurred at 12 hrs. and stayed significantly higher at 24 hrs., after which it was significantly lower at 48 hrs compared to peak expression (Figure 6E). We compared time course of frzb induction to klf9 and ush1g and found that klf9 showed the highest induction at 3 hours and stayed significantly high at 48 hours. Ush1g induction began at 3 hours and kept increasing throughout 24 hrs (Figures 6D, F).
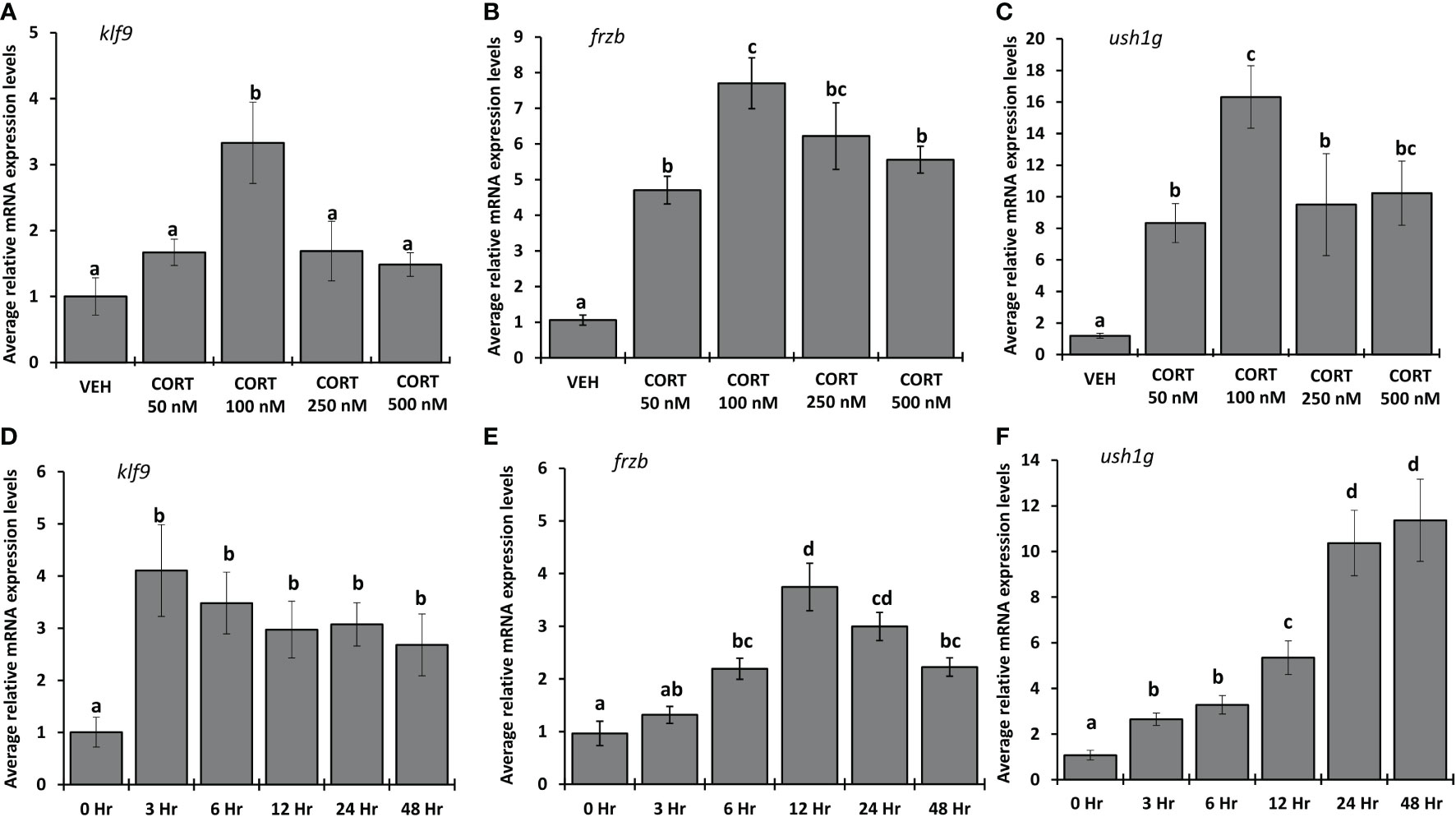
Figure 6 Dose response and time course for CORT induction of frzb, ush1g, and klf9 in tadpole tails. (A-C) Premetamorphic tadpoles (NF 54) were treated with vehicle and 50, 100, 250, and 500 nM of CORT (corticosterone) for 24 hours. (D-F) Premetamorphic tadpoles (NF 54) were treated with 100 nM CORT for 0, 3, 6, 12, 24, and 48 hours. Tails were dissected from tadpoles followed by RNA extraction and quantitative PCR to measure mRNA expression of frzb, ush1g, and klf9 and the housekeeping gene rpl8. Bars represent mean mRNA levels relative to rpl8 and normalized to a vehicle control sample. n = 10 tail samples per treatment. Error bars represent SE. Letters indicate significant groups, p < 0.05.
3.4 Frzb induction in X. laevis tadpoles
Because X. tropicalis and X. laevis are often interchangeably used for studying thyroid and stress hormone signaling, we wanted to know if frzb is induced in X. laevis as well (Figures 7A–C). Surprisingly, contrary to X. tropicalis tails, 100 nM CORT did not induce frzb nor ush1g in NF54 X. laevis tails (Figures 7B, C), even though we found that klf9 was induced (Figure 7A). We tried primers sets for both chromosomes, i.e., two primer sets for frzb.L and one for frzb.S, all with similar results (data not shown).
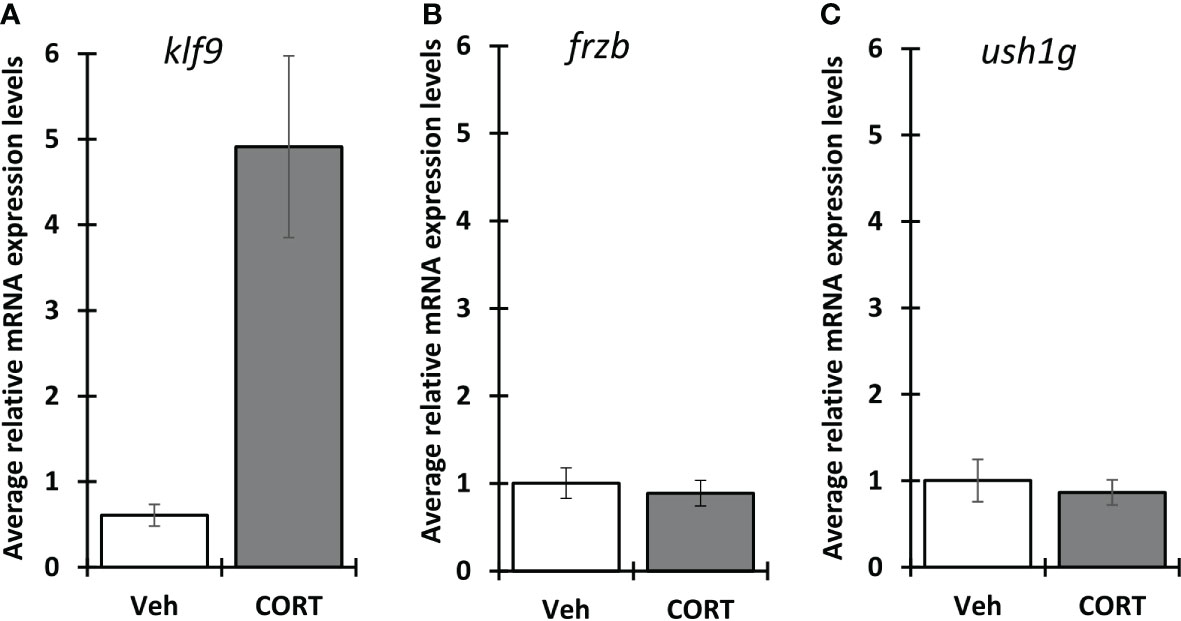
Figure 7 Induction of klf9, frzb and ush1g in X. laevis. Premetamorphic (NF 54) X. laevis tadpoles were treated with vehicle control or 100 nM CORT (corticosterone) for 24 hours followed by tail RNA extraction and quantitative PCR to quantify mRNA expression of klf9 (A) , frzb (B), and ush1g (C). Bars represent mean mRNA levels relative to the housekeeping gene rpl8 and normalized to a vehicle control sample. n = 10 tail samples per treatment. Error bars represent SE. Letters indicate significant groups, p < 0.05.
4 Discussion
Corticosteroids have pleiotropic effects on vertebrate development directly and/or indirectly through interaction with other key hormones, mainly thyroid hormone, insulin, and leptin (31–34). While much has been known about corticosteroid signaling from mammalian models, questions remain about how corticosteroid deficiency and excess in early development increase the risk of metabolic and neurological diseases in adults (4, 35). Lower vertebrates such as fish and frogs have been instrumental in unfolding some of the key corticosteroid signaling mechanisms, especially through the use of knockout models (13–15, 36, 37). Investigations on CORT signaling in tadpoles have also informed us a great deal about the impact that adaptation to stressful stimuli can have on the evolutionary ecology of the species (38). However, lack of adequate CORT response genes makes it challenging to detect and quantify endogenous and exogenous CORT response without going through the expensive and labor-intensive process of quantifying steroid hormone in the tissue and/or plasma. In this study, we characterized frzb (frizzled related protein), which along with 6 other genes (musk, camta1, sult6b1, sds, and grpel1) were found to be induced by only CORT and not TH in a previous global RNA-seq study on the X. tropicalis tail skin. This unique pattern of hormone induction drove our interest in these CORT-only genes. Previously, klf9 and ush1g had been the only 2 CORT-response genes reported in tadpoles (16–18). However, klf9 is also induced independently by TH and synergistically by both CORT and TH (19). ush1g is a CORT-only gene, however, ush1g expression during natural metamorphosis is inconsistent between clutches and thus unreliable (13, 15).
We further verified frzb to be induced only by CORT independent of TH with a fold change of around 8, which was higher than the remaining 5 genes, which had a fold change up to 2.5. Due to higher fold change, we used frzb for further characterization of a CORT-only response gene. We found that frzb expression was highest in the tails and no change in frzb expression was observed in the brain and liver. Frzb expression patterns in the brain and liver are similar to our previous study reporting ush1g expression in the brain and liver. No change in brain ush1g expression was observed and there was small increase in liver ush1g expression in response to CORT treatment (17). Klf9 levels were previously found to be induced in the brain by CORT treatment (16). Highest frzb expression in the tails took place at 12-24 hours when treated with 100-250 nM CORT. Similar to frzb, both klf9 and ush1g expression levels peak at 100 nM in the dose response experiment in the current study. In the time course experiment, similar to frzb, ush1g peaks at 24 hours. However, klf9 expression levels peak at 3 hours in the current study and at 2 hours in a previous study probably because klf9 is a direct CORT response gene (16).
In embryos, frzb is expressed in the anterior endoderm or in the prechordal mesoderm and plays a crucial role in anteroposterior patterning in Xenopus laevis by binding and inactivating Xwnt-8 during gastrulation (39). Inhibition of Wnt-8 signaling is necessary to prevent excessive ventralization and posteriorization by Wnt/β-catenin signaling in order to promote dorsoanterior development, specifically head formation (40, 41). frzb is also known to promote cartilage and long bone development in chick embryos (42, 43). However, there is no evidence of frzb affecting development in tadpoles through alteration of corticosteroid signaling. We showed frzb induction by CORT requires GR, and lack of GR in GR knockout tadpoles, which exhibit altered developmental rate and death at metamorphosis, had no apparent effect on head formation. Future studies using frzb knockout tadpoles should be conducted to identify an effect of frzb in CORT-induced tadpole tails.
Two surprising results from characterizing frzb were no increase in expression levels during natural metamorphosis and no induction by exogenous CORT in X. laevis despite high level of induction in X. tropicalis. An explanation could be that the measurable increases in CORT levels during metamorphic climax might be enough to induce klf9 (an early and direct response gene), but not enough to induce ush1g and frzb (44, 45). Additionally, TH levels also increase during metamorphic climax, hence, a combination of both CORT and TH might be enough to increase expression levels of the CORT and TH response gene klf9 but not CORT-only genes frzb or ush1g (23). frzb is expressed in both X. tropicalis and X. laevis, although due to allotetraploidy, X. laevis also has two copies (each on L and S chromosomes) of frzb (46). Primers quantifying expression levels of frzb in this study were designed to target L and S chromosomes which should have captured change in expression levels induced by one and/or the other allele of frzb. However, despite strong conservation in gene expression between X. laevis and X. tropicalis, significant differences have been observed in gene expression patterns between the two related species (47–49). Additionally, the role of frzb in anteroposterior patterning in Xenopus has mostly been studied in X. laevis tadpoles and not X. tropicalis, and no previous information exists regarding whether frzb is regulated by CORT in either of the two related species (39, 50–52). Further investigation is required surrounding the role of frzb in CORT signaling and causes of such species-specific divergence.
5 Conclusion
In this study, we have reported a glucocorticoid response gene, frizzled related protein (frzb) in X. tropicalis (but not X. laevis) tadpoles, which exhibit high mRNA induction in response to exogenous CORT but not TH in tails, and hence can be used to detect and quantify CORT responsivity independent of TH. Unfortunately, frzb cannot be used to study changes in CORT levels during natural metamorphosis or to study CORT response in the liver or brain. frzb is induced through GR and not MR and thus could be used to evaluate potential endocrine disrupting chemicals targeting GR. Any potential effects of frzb in CORT signaling await further study.
Data availability statement
The raw data supporting the conclusions of this article will be made available by the authors, without undue reservation.
Ethics statement
The animal study was reviewed and approved by University of Cincinnati Institutional Animal Care and Use.
Author contributions
BP: Conceptualization, Funding acquisition, Investigation, Methodology, Writing – original draft, Writing – review and editing. RD: Sample collection, RNA extraction and quantitative PCR. VV: Sample collection, RNA extraction and quantitative PCR. DB: Conceptualization, Funding acquisition, Project administration, Supervision, Writing – review and editing. All authors contributed to the article and approved the submitted version.
Funding
This work was supported by a Graduate Student Government (GSG) Research Fellowship and a Weiman Wendel Benedict grant from the Department of Biological Sciences, University of Cincinnati awarded to BP and by NSF IOS 2035732 to DB.
Conflict of interest
The authors declare that the research was conducted in the absence of any commercial or financial relationships that could be construed as a potential conflict of interest.
Publisher’s note
All claims expressed in this article are solely those of the authors and do not necessarily represent those of their affiliated organizations, or those of the publisher, the editors and the reviewers. Any product that may be evaluated in this article, or claim that may be made by its manufacturer, is not guaranteed or endorsed by the publisher.
References
1. Moisiadis VG, Matthews SG. Glucocorticoids and fetal programming part 2: Mechanisms. Nat Rev Endocrinology. (2014) 10(7):403–11. doi: 10.1038/nrendo.2014.74
2. Zambrano E, Reyes-Castro LA, Nathanielsz PW. Aging, glucocorticoids and developmental programming. AGE. (2015) 37(3):52. doi: 10.1007/s11357-015-9774-0
3. Tegethoff M, Pryce C, Meinlschmidt G. Effects of intrauterine exposure to synthetic glucocorticoids on fetal, newborn, and infant hypothalamic-Pituitary-Adrenal axis function in humans: A systematic review. Endocrine Rev (2009) 30(7):753–89. doi: 10.1210/er.2008-0014
4. Khulan B, Drake AJ. Glucocorticoids as mediators of developmental programming effects. Best Pract Res Clin Endocrinol Metab (2012) 26(5):689–700. doi: 10.1016/j.beem.2012.03.007
5. White PC, Speiser PW. Congenital adrenal hyperplasia due to 21-hydroxylase deficiency 1. Endocrine Rev (2000) 21(3):245–91. doi: 10.1210/edrv.21.3.0398,9
6. Espina JEC, Bagamasbad PD. Chapter two - synergistic gene regulation by thyroid hormone and glucocorticoid in the hippocampus. In: Litwack GBTV, editor. Hormones, regulators and hippocampus. National Institute of Molecular Biology and Biotechnology, University of the Philippines Diliman, Quezon City, Philippines:Academic Press (2022). p. 35–81. Available at: https://www.sciencedirect.com/science/article/pii/S0083672921000698.
7. Li JX, Cummins CL. Fresh insights into glucocorticoid-induced diabetes mellitus and new therapeutic directions. Nat Rev Endocrinology. (2022) 18(9):540–57. doi: 10.1038/s41574-022-00683-6
8. Malcher-Lopes R, Di S, Marcheselli VS, Weng FJ, Stuart CT, Bazan NG, et al. Opposing crosstalk between leptin and glucocorticoids rapidly modulates synaptic excitation via endocannabinoid release. J Neurosci (2006) 26(24):6643–50. doi: 10.1523/JNEUROSCI.5126-05.2006
9. Sachs LM, Buchholz DR. Frogs model man: In vivo thyroid hormone signaling during development. Genesis. (2017) 55(1–2):1–10. doi: 10.1002/dvg.23000
10. Buchholz DR. More similar than you think: Frog metamorphosis as a model of human perinatal endocrinology. Dev Biol (2015) 408(2):188–95. doi: 10.1016/j.ydbio.2015.02.018
11. Paul B, Sterner ZR, Buchholz DR, Shi YB, Sachs LM. Thyroid and corticosteroid signaling in amphibian metamorphosis. Cells. (2022) 11(10):1595. doi: 10.3390/cells11101595
12. Choi J, Suzuki K ichi T, Sakuma T, Shewade L, Yamamoto T, Buchholz DR. Unliganded thyroid hormone receptor α regulates developmental timing via gene repression in Xenopus tropicalis. Endocrinology. (2015) 156(2):735–44. doi: 10.1210/en.2014-1554
13. Sterner ZR, Shewade LH, Mertz KM, Sturgeon SM, Buchholz DR. Glucocorticoid receptor is required for survival through metamorphosis in the frog xenopus tropicalis. Gen Comp Endocrinology. (2020) 291:113419. doi: 10.1016/j.ygcen.2020.113419
14. Shewade LH, Schoephoerster JA, Patmann MD, Kulkarni SS, Buchholz DR. Corticosterone is essential for survival through frog metamorphosis. Endocrinology (2020) 161(12). doi: 10.1210/endocr/bqaa193/5938994
15. Paul B, Shewade LH, Buchholz DR. cyp21a2 knockout tadpoles survive metamorphosis despite low corticosterone. Endocrinology. (2022) 27:bqac182. doi: 10.1210/endocr/bqac182
16. Shewade LH, Schneider KA, Brown AC, Buchholz DR. In-vivo regulation of krüppel-like factor 9 by corticosteroids and their receptors across tissues in tadpoles of xenopus tropicalis. Gen Comp Endocrinology. (2017) 248:79–86. doi: 10.1016/j.ygcen.2017.02.007
17. Schneider KA, Shewade LH, Buisine N, Sachs LM, Buchholz DR. A novel stress hormone response gene in tadpoles of xenopus tropicalis. Gen Comp Endocrinology. (2018) 260:107–14. doi: 10.1016/j.ygcen.2018.01.006
18. Bonett RM, Hoopfer ED, Denver RJ. Molecular mechanisms of corticosteroid synergy with thyroid hormone during tadpole metamorphosis. Gen Comp Endocrinology. (2010) 168(2):209–19. doi: 10.1016/j.ygcen.2010.03.014
19. Sterner ZR, Buchholz DR. Glucocorticoid receptor mediates corticosterone-thyroid hormone synergy essential for metamorphosis in xenopus tropicalis tadpoles. Gen Comp endocrinology. (2022) 315:113942. doi: 10.1016/j.ygcen.2021.113942
20. Chen X, Li Y, Zhu M, Qin Z. An ex vivo assay for screening glucocorticoid signaling disruption based on glucocorticoid-response gene transcription in xenopus tails. J Environ Sci (2018) 66:104–12. doi: 10.1016/j.jes.2017.05.017
21. Kulkarni SS, Buchholz DR. Beyond synergy: Corticosterone and thyroid hormone have numerous interaction effects on gene regulation in xenopus tropicalis tadpoles. Endocrinology. (2012) 153(11):5309–24. doi: 10.1210/en.2012-1432
22. Denver RJ. Evolution of the corticotropin-releasing hormone signaling system and its role in stress-induced phenotypic plasticity. Ann New York Acad Sci (1999) 897:46–53. doi: 10.1111/j.1749-6632.1999.tb07877.x
23. Denver RJ. Acceleration of anuran amphibian metamorphosis by corticotropin-releasing hormone-like peptides. Gen Comp Endocrinology. (1993) 91(1):38–51. doi: 10.1006/gcen.1993.1102
24. Buisine N, Grimaldi A, Jonchere V, Rigolet M, Blugeon C, Hamroune J, et al. Transcriptome and methylome analysis reveal complex cross-talks between thyroid hormone and glucocorticoid signaling at xenopus metamorphosis. Cells. (2021) 10(9):2375. doi: 10.3390/cells10092375
25. Foster SD, Glover SR, Turner AN, Chatti K, Challa AK. A mixing heteroduplex mobility assay (mHMA) to genotype homozygous mutants with small indels generated by CRISPR-Cas9 nucleases. MethodsX. (2019) 6:1–5. doi: 10.1016/j.mex.2018.11.017
26. Nieuwkoop DP. Normal table of xenopus laevis (Daudin). Normal table Xenopus Laevis (Daudin). (1956), 162–203.
27. Patmann MD, Shewade LH, Schneider KA, Buchholz DR. Xenopus tadpole tissue harvest. Cold Spring Harbor Protoc (2017) 11). doi: 10.1101/pdb.prot097675
28. Dhorne-Pollet S, Thélie A, Pollet N. Validation of novel reference genes for RT-qPCR studies of gene expression in xenopus tropicalis during embryonic and post-embryonic development. Dev Dynamics. (2013) 242(6):709–17. doi: 10.1002/dvdy.23972
29. Livak KJ, Schmittgen TD. Analysis of relative gene expression data using real-time quantitative PCR and the 2-ΔΔCT method. Methods. (2001) 25(4):402–8. doi: 10.1006/meth.2001.1262
30. Team RC. R: A language and environment for statistical computing (Version 3.5. 2;)[Computer software]. Vienna: R foundation for statistical computing. (2018).
31. Fowden AL, Li J, Forhead AJ. Glucocorticoids and the preparation for life after birth: are there long-term consequences of the life insurance? Proc Nutr Society. (1998) 57(1):113–22. doi: 10.1079/pns19980017
32. Sachs LM, Buchholz DR. Insufficiency of thyroid hormone in frog metamorphosis and the role of glucocorticoids. Front Endocrinology. (2019) 10. doi: 10.3389/fendo.2019.00287
33. Fowden AL, Forhead AJ. Endocrine regulation of feto-placental growth. Hormone Res (2009) 72(5):257–65. doi: 10.1159/000245927
34. Freeman BM, Vince MA. Freeman BM, Vince MA, Hormones in Development. editors, vol. p . Dordrecht: Springer Netherlands (1974). p. 208–36. doi: 10.1007/978-94-009-5710-7_13
35. Wyrwoll CS, Holmes MC. Prenatal excess glucocorticoid exposure and adult affective disorders: A role for serotonergic and catecholamine pathways. Neuroendocrinology. (2012) 95(1):47–55. doi: 10.1159/000331345
36. Eachus H, Zaucker A, Oakes JA, Griffin A, Weger M, Güran T, et al. Genetic disruption of 21-hydroxylase in zebrafish causes interrenal hyperplasia. Endocrinology. (2017) 158(12):4165–73. doi: 10.1210/en.2017-00549
37. Paul B, Sterner ZR, Bhawal R, Anderson ET, Zhang S, Buchholz DR. Impaired negative feedback and death following acute stress in glucocorticoid receptor knockout xenopus tropicalis tadpoles. Gen Comp endocrinology. (2022) 326:114072. doi: 10.1016/j.ygcen.2022.114072
38. Denver RJ. Stress hormones mediate developmental plasticity in vertebrates with complex life cycles. Neurobiol Stress. (2021) 14:100301. doi: 10.1016/j.ynstr.2021.100301
39. Wang S, Krinks M, Lin K, Luyten FP, Moos M. Frzb, a secreted protein expressed in the spemann organizer, binds and inhibits wnt-8. Cell. (1997) 88(6):757–66. doi: 10.1016/S0092-8674(00)81922-4
40. Lin K, Wang S, Julius MA, Kitajewski J, Moos M, Luyten FP. The cysteine-rich frizzled domain of frzb-1 is required and sufficient for modulation of wnt signaling. Proc Natl Acad Sci (1997) 94(21):11196–200. doi: 10.1073/pnas.94.21.11196
41. Carron C, Shi DL. Specification of anteroposterior axis by combinatorial signaling during xenopus development. Wiley Interdiscip Reviews: Dev Biol (2016) 5(2):150–68. doi: 10.1002/wdev.217
42. Enomoto-Iwamoto M, Kitagaki J, Koyama E, Tamamura Y, Wu C, Kanatani N, et al. The wnt antagonist frzb-1 regulates chondrocyte maturation and long bone development during limb skeletogenesis. Dev Biol (2002) 251(1):142–56. doi: 10.1006/dbio.2002.0802
43. Enomoto-Iwamoto M, Kitagaki J, Koyama E, Tamamura Y, Kanatani N, Komori T, et al. Dual roles of the wnt antagonist, frzb-1 in cartilage development. Biomed AND Health RESEARCH-COMMISSION OF THE Eur COMMUNITIES THEN IOS Press (2002) 54:235–44.
44. Glennemeier KA, Denver RJ. Developmental changes in interrenal responsiveness in anuran amphibians. Integr Comp Biol (2002) 42(3):565–73. doi: 10.1093/icb/42.3.565
45. Jolivet G, Hatey JL. Variations in aldosterone and corticosterone plasma levels during metamorphosis in xenopus laevis tadpoles. Gen AND Comp ENDOCRINOLOGY. (1984) 56:59–65. doi: 10.1016/0016-6480(84)90061-3
46. Mii Y, Taira M. Secreted wnt “inhibitors” are not just inhibitors: Regulation of extracellular wnt by secreted frizzled-related proteins. Development Growth Differentiation. (2011) 53(8):911–23. doi: 10.1111/j.1440-169X.2011.01299.x
47. Watanabe M, Yasuoka Y, Mawaribuchi S, Kuretani A, Ito M, Kondo M, et al. Conservatism and variability of gene expression profiles among homeologous transcription factors in xenopus laevis. Dev Biol (2017) 426(2):301–24. doi: 10.1016/j.ydbio.2016.09.017
48. Nieber F, Pieler T, Henningfeld KA. Comparative expression analysis of the neurogenins in xenopus tropicalis and xenopus laevis. Dev Dynamics. (2009) 238(2):451–8. doi: 10.1002/dvdy.21845
49. Yanai I, Peshkin L, Jorgensen P, Kirschner MW. Mapping gene expression in two xenopus species: Evolutionary constraints and developmental flexibility. Dev Cell (2011) 20(4):483–96. doi: 10.1016/j.devcel.2011.03.015
50. Leyns L, Bouwmeester T, Kim SH, Piccolo S, De Robertis EM. Frzb-1 is a secreted antagonist of wnt signaling expressed in the spemann organizer. Cell. (1997) 88(6):747–56. doi: 10.1016/S0092-8674(00)81921-2
51. Bu Q, Li Z, Zhang J, Xu F, Liu J, Liu H. The crystal structure of full-length sizzled from xenopus laevis yields insights into wnt-antagonistic function of secreted frizzled-related proteins. J Biol Chem (2017) 292(39):16055–69. doi: 10.1074/jbc.M117.791756
Keywords: glucocorticoids, stress hormone, gene expression, Xenopus tropicalis, metamorphosis
Citation: Paul B, Dockery R, Valverde VM and Buchholz DR (2023) Characterization of a novel corticosterone response gene in Xenopus tropicalis tadpole tails. Front. Endocrinol. 14:1121002. doi: 10.3389/fendo.2023.1121002
Received: 10 December 2022; Accepted: 11 January 2023;
Published: 26 January 2023.
Edited by:
Wei Zhu, Chengdu Institute of Biology (CAS), ChinaReviewed by:
Lusha Liu, Huazhong Agricultural University, ChinaShouhong Wang, National Institutes of Health (NIH), United States
Copyright © 2023 Paul, Dockery, Valverde and Buchholz. This is an open-access article distributed under the terms of the Creative Commons Attribution License (CC BY). The use, distribution or reproduction in other forums is permitted, provided the original author(s) and the copyright owner(s) are credited and that the original publication in this journal is cited, in accordance with accepted academic practice. No use, distribution or reproduction is permitted which does not comply with these terms.
*Correspondence: Daniel R. Buchholz, YnVjaGhvZHJAdWNtYWlsLnVjLmVkdQ==
†ORCID: Daniel R. Buchholz, orcid.org/0000-0003-2070-5684