- 1Department of Obstetrics and Gynecology, Peking University First Hospital, Beijing, China
- 2Laboratory Animal Center, Peking University First Hospital, Beijing, China
- 3Beijing Key Laboratory of Maternal Fetal Medicine of Gestational Diabetes Mellitus, Beijing, China
Placenta accreta spectrum disorder (PAS) is a kind of disease of placentation defined as abnormal trophoblast invasion of part or all of the placenta into the myometrium, even penetrating the uterus. Decidual deficiency, abnormal vascular remodeling in the maternal–fetal interface, and excessive invasion by extravillous trophoblast (EVT) cells contribute to its onset. However, the mechanisms and signaling pathways underlying such phenotypes are not fully understood, partly due to the lack of suitable experimental animal models. Appropriate animal models will facilitate the comprehensive and systematic elucidation of the pathogenesis of PAS. Due to the remarkably similar functional placental villous units and hemochorial placentation to humans, the current animal models of PAS are based on mice. There are various mouse models induced by uterine surgery to simulate different phenotypes of PAS, such as excessive invasion of EVT or immune disturbance at the maternal–fetal interface, which could define the pathological mechanism of PAS from the perspective of the “soil.” Additionally, genetically modified mouse models could be used to study PAS, which is helpful to exploring the pathogenesis of PAS from the perspectives of both “soil” and “seed,” respectively. This review details early placental development in mice, with a focus on the approaches of PAS modeling. Additionally, the strengths, limitations and the applicability of each strategy and further perspectives are summarized to provide the theoretical foundation for researchers to select appropriate animal models for various research purposes. This will help better determine the pathogenesis of PAS and even promote possible therapy.
1 Introduction
Placenta accreta spectrum disorders (PAS) refer to a group of diseases in which abnormal trophoblasts invade part or all of the placenta into the myometrium of the uterine wall (1). Although the first case series of placenta accreta (PA) was published in 1937 by Irving and Hertig (2), PAS was a relatively newly defined disorder of placentation until 2018, when the International Federation of Gynecology and Obstetrics (FIGO) named the morbidly adherent placenta, abnormal invasive placenta, adhesive placenta, and other related series of placenta accreta disease as PAS (3). Three subtypes were differentiated from PAS according to the depth of trophoblast invasion into the myometrium (1): placenta accreta, where the villi attach directly to the surface of myometrium without intervening decidua (2); placenta increta, where the invasion of the trophoblast has been into the myometrium over the 1/3; and (3) placenta percreta, where the invasion of villous reaches and penetrates through the myometrium, serosa, and surrounding structures, such as bladder (1, 4). Noticeably, there are often multiple degrees of EVT invasion into the myometrium in one patient, such as the presence of both accreta and percreta in one patient (1). Histopathological examination, the gold standard for the diagnosis of PAS, is very important to detect the occurrence and depth of abnormal EVT invasion (5).
The prevalence of PAS ranged from 0.01% to 1.1% from 1981 to 2012, and continues to increase, especially in countries with high caesarean section (CS) rates (6, 7). Compared with other pregnancy-related diseases, such as gestational diabetes mellitus (GDM) or preeclampsia (PE), the incidence of PAS is relatively lower (8). However, maternal mortality in PAS patients is higher than that in other obstetric diseases due to severe postpartum hemorrhage (4, 9–11). Therefore, it is urgent to clarify the mechanisms of PAS, which will definitely help to improve pregnancy outcomes for patients.
At present, little is known about the pathogenesis of PAS. It is generally accepted that incomplete or absent decidua in pregnancy is the main cause (12). Normal decidualization of the endometrium can prevent excessive invasion of trophoblast cells and suppress the maternal immune response to maintain implantation and decidualization (13). When the structural integrity of the endometrium or myometrium is impaired, scars form locally and the myometrium around the scar often shows degeneration and hyalinosis, accompanied by fibrous tissue hyperplasia and inflammatory cell infiltration, which is not conducive to embryo implantation and placental development (14, 15). When the placenta is attached to the scar site, the villi directly contact the myometrium, which is one of the pathogenic mechanisms of PAS (16). Recently, researchers have suggested that the distorted uteroplacental interface caused by dense fibrous deposition is an essential factor contributing to abnormal placental attachment (2, 17, 18). This challenged the classical concept that placenta accreta is simply due to villous tissue sitting atop the superficial myometrium without interposed decidua.
Elucidating its pathogenesis is the key foundation for improving the pregnancy outcome of PAS patients clinically. Appropriate animal models could facilitate comprehensive and systematic research on the pathogenesis of PAS. Similarities in genetic information between mice and humans have been reported (19). Although the structures of the mouse placenta terminology are highlighted dissimilarity with humans, mouse placentas are highly functionally conserved and the functional placental villous units are remarkably similar in mice and humans (Figure 1) (21, 22). In addition, both mouse and human placentas possess similar hemochorial blood flow (20, 23, 24) and undergo trophoblast infiltration and uterine spiral artery remodeling (20, 25–28). Regarding the time of pregnancy period, less time is required in mice, which facilitates animal experiments since it is measured in days rather than in months (27, 28).
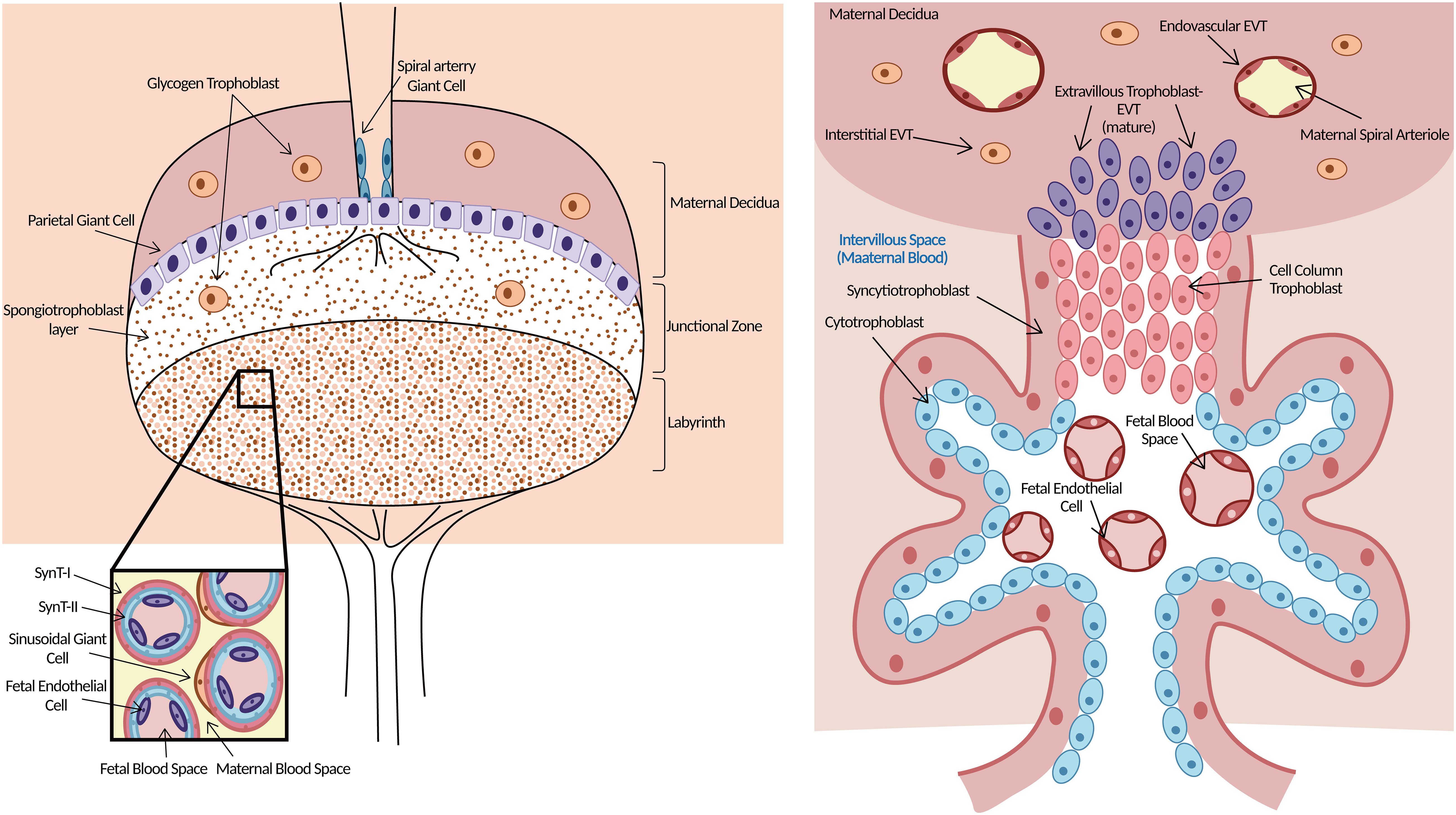
Figure 1 Morphology of mouse (left) and human (right) placenta. Left: Three layers clearly in the mouse placenta. The upper is maternal decidua. The middle layer of the supportive JZ contains spongiotrophoblasts, which give rise to TGCs and glycogen trophoblast cells. Both TGCs and glycogen trophoblast cells are invasive. The labyrinth is the functional structure of the mouse placenta where gas/nutrient exchange occurs. Maternal blood is separated from fetal blood by SynT-I, SynT-II, and sinusoidal giant cells. Right: The functional structure for gas/nutrient exchange is the chorionic villus in the human placenta. EVTs arise from the trophoblast cell column and are highly invasive, penetrating up to one-third of the thickness of the uterus. Endovascular EVTs extensively remodel maternal spiral arterioles to ensure correct blood supply to the growing embryo. Trophoblast giant cells (TGCs), junctional zone (JZ), extravillous trophoblast cells (EVTs), syncytiotrophoblast-I (SynT-I), syncytiotrophoblast- II (SynT-II). Modified from (20).
Currently, uterine surgeries to simulate the risk factors for PAS are the main approaches to establish disease models. Uterine surgery can damage the endometrium or myometrium, which is helpful to exploring the pathogenesis of PAS from the perspective of “soil” abnormalities. The genetically modified strategy could be another option, which targets the editing of cell invasion or metastasis-related genes; it can not only mimic the abnormal decidua but also simulate the excessive invasion of trophoblast cells or the placenta in PAS, which is helpful for exploring the pathogenesis of PAS from the perspectives of both the “soil” and the “seed” respectively.
This review details early placenta development in mice, with a focus on the approaches of PAS modeling. Additionally, the strengths and limitations of each strategy and further perspectives are summarized to provide the theoretical foundation for researchers to select appropriate animal models for different research purposes. This will help better determine the pathogenesis of PAS and even promote possible therapy.
2 Placental development in mice
The oocytes and sperm at fertilization to form totipotent zygote are the beginning of embryogenesis in both humans and mice, which occurs at embryonic day (E) 2 in mice. Two distinct populations of cells, the inner cell mass (ICM) and trophoblast ectoderm (TE), emerge through the establishment of cell polarity and several rounds of cell division, respectively, which occurs at E3.5 in mice and approximately day 5 postcoitum in humans to form the blastocyst (Figure 2) (19). Blastocyst implantation occurs at E4.5 in mice and days 7–8 postcoitum in humans, when maternal endometrial stromal cells undergo a specific reaction called decidualization under the influence of a variety of hormones, which is widespread in both mice and humans (29). During decidualization, the accurate and ongoing crosstalk between embryonic-derived trophoblast cells and the decidua is an essential “warrantor” for further successful placentation and pregnancy (30). Any factors that lead to impaired decidualization, such as endometriosis or uterine scarring, exert a higher risk of infertility and abnormal placentation (31, 32).
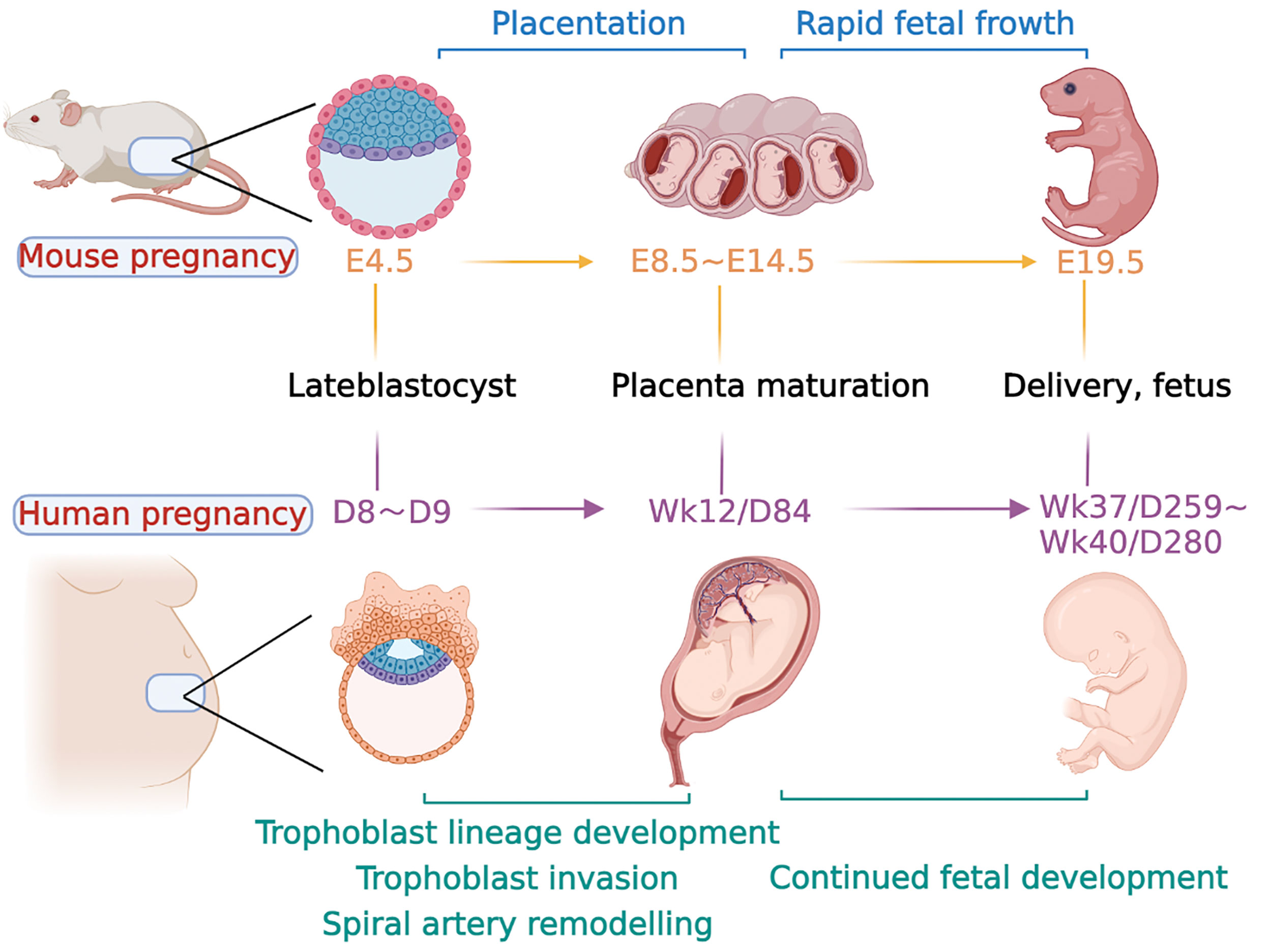
Figure 2 Major event time points in mouse and human pregnancy. The timeline for both species is calculated from conception. Days of development are denoted embryonic (E) in the mouse and week (Wk) or day (D) of development in the human. Late blastocyst implantation in mice occurred at approximately E4.5, followed by endometrial decidualization. The formation of the labyrinth at approximately E8.5, with branching morphogenesis complete by E10.5. At E10.5, the junctional zone and TGCs are also fully formed, and glycogen trophoblast cells continue to differentiate and invade the uterus along with specific TGC subtypes and form a fully functional placenta. At the same time, the embryo developed rapidly at the later stage until delivery at approximately E19.5. In humans, after a series of processes, such as trophoblast cell invasion and uterine spiral artery remodeling, a mature placenta is formed at approximately 12 weeks of gestation. The fetus develops rapidly in the later stage and is normally delivered at approximately 37–40 weeks.
The TE cells in mouse blastocysts continue to proliferate after implantation to form embryonic ectoderm (ExE) and the ectoplacental cone (EPC). At approximately E6.5, the extraembryonic mesoderm lineage is generated, which further forms the allantois and extraembryonic mesodermal layers of the amnion and chorion (19). At approximately E8.5, the fusion of the chorion and allantois is the essential step in the process of forming a mature placenta. Chorion and allantois fusion allows the endoderm-derived blood vessels of the embryo to enter the chorionic trophoblast layer (33). This interfinger differentiation between fetal blood vessels and trophoblast-lined maternal sinuses forms the labyrinth, the basic structure of the mouse placenta (34). After that, the multipotent progenitor cells in the mouse placenta facilitate the expansion of the labyrinth, as well as the differentiation into syncytial trophoblast cells (STB) and sinus trophoblast giant cells (TGCs) (35, 36).
At approximately E10.5, three layers clearly developed in the mouse placenta: the decidua, the junctional zone (JZ), and the labyrinth (20, 37). The labyrinth, which is near the fetal side, is a functional structure where gas–nutrient exchange occurs (38). Maternal blood is separated from fetal blood by three layers of trophoblasts in this layer (39). In the human placenta, there are initially two layers of chorionic villi, and later in pregnancy, there is one functional trophoblast layer that separates maternal and fetal blood (20). The function of the labyrinth is similar to that of the human chorionic villus. The JZ is next to the labyrinth and lies directly beneath the decidua, which consists of parietal trophoblast giant cells and spongiotrophoblasts. Spongiotrophoblasts give rise to TGCs and glycogen trophoblast cells that largely function in hormone secretion and metabolism (22, 40). More importantly, both TGCs and glycogen trophoblast cells are functionally invasive, similar to human EVTs (41), although the two kinds of trophoblast cells anchoring the placenta to the uterine wall in the mouse are not nearly as invasive as the equivalent EVTs in humans, where these cells invade up to one-third of the thickness of the uterine wall, including the maternal arterioles (20). Some glycogen trophoblast cells migrate deep into the maternal decidua, where they are thought to help enhance maternal blood flow (20). All of these properties lie down the foundation for exploring human EVT-related diseases through a focus on TGCs and glycogen trophoblast cells in the mouse placenta.
Although there are some discrepancies in the morphology and terminology of the mouse placenta from the human placenta, the two species appear more equivalent at the functional placental villous units and the cellular composition level. Therefore, the mouse placenta is an appropriate tool for research on human placenta-related diseases.
3 Approaches to establish the PAS model
3.1 Surgery-induced models
Prenatal prediction of PAS is important to determine the appropriate delivery time and multidisciplinary planning for operative management (42), which is mostly based on ultrasound signs and risk factors (12). The main risk factors for PAS include cesarean section, placenta previa, in vitro fertilization and embryo transplantation, and uterine surgery history, such as hysteroscopic operation and endometrial curettage (43–45).
Most of the existing PAS animal models simulate PAS by uterine surgery, such as uterine incision, endometrial curettage, and hysterotomy, and check the pathological phenotypes of placentas in the subsequent pregnancy. Any surgery that damages the endometrium or myometrium may affect the regeneration and repair of endometrial epithelial cells, further resulting in the loss of decidua and the excessive invasion of EVT in the subsequent pregnancy, leading to possible placental abnormalities (46, 47). The PAS models established by different uterine surgeries are described in the following.
3.1.1 Uterine incision
The morphological changes and healing process of the mouse uterine incision within 2 weeks after surgery are similar to humans (48). In terms of the healing process, the epithelium of the lesion of the mouse uterus began to repair on the second day after the operation, and on the fourth day, the wound was completely filled with endometrial tissue on the uterine cavity side and covered by adipose tissue attached to the mesentery on the abdominal side (48). Moreover, the process of healing of the postoperative incision is associated with the estrus cycle of mice, and it is also similar to humans in that the process of healing is associated with changes in hormones (49).
It has been reported that making an incision (0.5–0.8 cm) on one side of the uterine horn of C57BL/6J mice simulates uterine damage, and the placenta showed pathological changes similar to PAS in the subsequent pregnancy (50). Specifically, although the areas of the placental JZ and labyrinth were not significantly different between the model and control groups, nor was the ratio of the placental JZ to labyrinth different, the model group showed a larger area of invasive trophoblast cells in the decidua than that in the control group. This corresponds to the extensively altered expression of genes involved in cellular invasion at the placental interface, such as Mmp2, Mmp9, Mmp3, and Dock4 (50–52). Meanwhile, the number of blood vessels in the placenta increased significantly in this model, which is similar to the hypervascularity in the placenta of PAS patients (53, 54). In accordance with the hypervascularity in the maternal–fetal interface, the serum levels of the angiogenic factors epidermal growth factor (EGF) and vascular endothelial growth factor (VEGF) tended to increase, while the levels of the antiangiogenic factors soluble fms-like tyrosine kinase 1 (sFlt) and endoglin (ENG) decreased in this model (50). Regarding the immune environment in the decidua and placenta, the proportions of T and natural killer cells (NK) in the decidua diminished significantly at the maternal–fetal interface in this model, and the number of NK cells and M2 macrophages showed a great decline, especially with respect to decidual NK (dNK) cells (50). In terms of inflammatory conditions, the expression of TNF-α and IL-4 in the decidua was upregulated but the expression of IFN-γ and IL-10 was downregulated in this model. These phenotypes are similar to the altered immune environment at the maternal–fetal interface in PAS patients, which is often accompanied by abnormal polarization of macrophages (55) and immune cell infiltrates (56).
In this PAS model, the increased IL-4 may function as a “shooter” to the overactive immune environment at the maternal–fetal interface, which further induces M2-like THP-1 macrophages to increase the expression of granulocyte colony-stimulating factor (G-CSF), prompting the proliferation, invasion, and adhesion of trophoblast cells (55). These alterations are different from PE, where decreased IL-4 in return activates B cells, prompting autoantibody production and eventually leading to endothelial dysfunction and hypertension (57, 58). Compared to the increased IL-4 in this PAS model, the decreased IL-4 in PE is consistent with the notion that shallow cytotrophoblast invasion is implicated in the pathogenesis of PE (58–61). These findings indicate that the immunomodulation in PE and PAS may be totally different, although both are diseases caused by abnormal placental development. The in-depth immune regulation mechanism could also be one of the directions for future research in both PE and PAS.
The uterine incision in the mouse led to an expansive invasion of trophoblast cells, combined with alterations in the immune microenvironment and cytokine levels in the decidua, which recapitulates the classical phenotypes of PAS in the clinic. Therefore, the model can be applied to investigate the abnormal placentation induced by uterine damage, especially to study immune disorders and inflammation regulation at the maternal–fetal interface in PAS, which are currently few studies. In addition to placental abnormalities, changes in serum sFlt and VEGF were found in this model, which suggested that it could be used for the study of serological changes in PAS, further prompting the development of clinical diagnostic markers and prediction models for PAS.
3.1.2 Caesarean section
Caesarean section (CS) is an independent risk factor for PAS (62). It is essential to note that the increase in the incidence of PAS paralleled the promotion of CS (5, 12). The relative risk of PAS was 8.8 (95% CI: 6.1–12.6) for previous CS, 6.6 (95% CI: 4.4–9.8) for a single CS, 7.4 (95% CI: 4.4–9.8) for two CS, and 55.9 (95% CI: 25.0–110.3) for a history of three or more CS (45). The process of uterine tissue repair after CS is often accompanied by decreasing endometrial glands, inhibition of myometrial smooth muscle cell growth, excessive collagen fiber deposition, and massive leukocyte infiltration, which leads to long-term and chronic inflammation and scarring (63). There appears to be preferential attachment of the blastocyst to the scar site, which may be associated with defective decidua in that region, resulting in abnormal implantation (64). In turn, disorientation of the implanted embryo and restricted stromal cell proliferation could also lead to defective decidualization and abnormal placental development, including excessive invasion of trophoblasts into the decidua and insufficient branching of fetal blood vessels (65, 66). Clinically, single-cell RNA sequencing of the PAS placenta of humans shows that in the absence of the decidua, invasive trophoblasts of various differentiation states interact strongly with maternal stromal cells, which might allow the placental villi to migrate to the serosal surface of the uterus and be associated with hypervascularity in PAS patients (67). All of these results indicate that incomplete or absent decidua is one of the key drivers of PAS.
S. D. Burke et al. simulated CS in peripartum mice by hysterotomy in experimental CD-1 mice. Concretely, an incision was made along the entire length of the experimental uterine horn, alongside the mesometrial insertion of blood vessels within 24 h of parturition, which destroyed the integrity of the myometrium and formed the uterine scar locally (68). When the recovered mice were pregnant, an increased depth of trophoblast invasion in the unmanipulated uterine horn was observed, which was assessed by measuring the cytokeratin-positive area extending beyond the placenta proper. This finding indicated that placental paracrine or endocrine signaling may induce a similar placenta accreta phenotype in the unmanipulated horn. In addition, when the researchers examined the disruption or dysplasia of the uterine smooth muscle by HE staining, they found that in this peripartum scar model, the myometrial integrity was significantly disrupted in both the manipulated and unmanipulated uterine horns (68).
This model simulates the characteristics of myometrium damage after clinical CS through hysterectomy in peripartum mice, and the impaired integrity of the myometrium is an important cause of excessive EVT invasion. However, the mechanism and signaling pathway of how myometrial injury affects EVT invasion and placental development is rarely reported. Only a few studies have reported that EVT migration and invasion into the myometrium may be related to the downregulation of functional E-cadherin in the myometrium of uterine scars (64). This model is very suitable for research on the pathomechanism of PAS in the aspect of impaired myometrium. Moreover, it is worth noting that an increase in the depth of trophoblast invasion in the unmanipulated uterine horn was observed in this model, while how the damaged uterus may induce PAS phenotypes in the unmanipulated uterine horn through paracrine or endocrine signaling is also an interesting direction for further investigation. This will help to understand the clinical pathological mechanism of placenta accreta in non-uterine scar sites.
3.1.3 Dilation & curettage
Dilation & curettage (DC) is a surgery generally considered to be relatively safe, but it is still associated with some long-term complications. The most well-known complication is intrauterine adhesions, also known as Asherman syndrome, which may lead to menstrual disorders and fertility problems (69). Previous studies have shown that the uterus of Sprague Dawley (SD) rats presents a series of abnormal changes when intrauterine adhesions occur, such as a decreased number of endometrial glands, increased deposition of collagen and fiber deposition, and disorganized morphology and structure of the myometrium (70, 71). A history of D&C is associated with the occurrence of PAS (OR 2.8; 95% CI 1.7–4.6) (62, 72). However, published studies mainly focus on the assessment of risk factors and the diagnostic utility of D&C in PAS; there is a lack of studies on the mechanism by which D&C affects blastocyst implantation and subsequent placental development.
In this kind of PAS model induced by D&C, three rounds of oblique angle curetting of the endometrium were used to simulate the history of D&C in CD-1 mice. When the recovered mice were pregnant, researchers found that the trophoblast invasion areas were deeper in the experimental uterine horn combined with significant myometrium impairment (68). This strategy to construct the PAS model indicates that D&C can affect the repair of damaged myometrium and subsequent pregnancy outcome. Further studies are clearly needed to address the poorly understood physiology of involution and regeneration of the myometrium after surgery and may help to elucidate the pathogenesis of PAS from the aspect of damaged myometrium.
The above methods to establish PAS models by uterine surgery damaged the normal structure of the endometrium or myometrium of mice, which further facilitated EVT invasion. Moreover, uterine incision surgery can lead to immune imbalance and inflammatory infiltration in the uterine environment, causing local tissue homeostasis imbalance in the uterus, which in turn leads to PAS through multiple immune and inflammation-related signaling pathways (56, 73), which also highlights that PAS is a multifactorial disease. The strengths, limitations, and applicability of each uterine surgery model are shown in Table 1. In summary, these approaches comprehensively reflect the pathological phenotype of PAS and are appropriate to explore the pathogenesis of PAS from the perspective of “soil” abnormalities, either the damaged endometrium or myometrium. However, there is a lack of published research to explore the possibility that IVF could be accessible to establish the PAS model, so it is an important direction worth exploring to establish a new model.
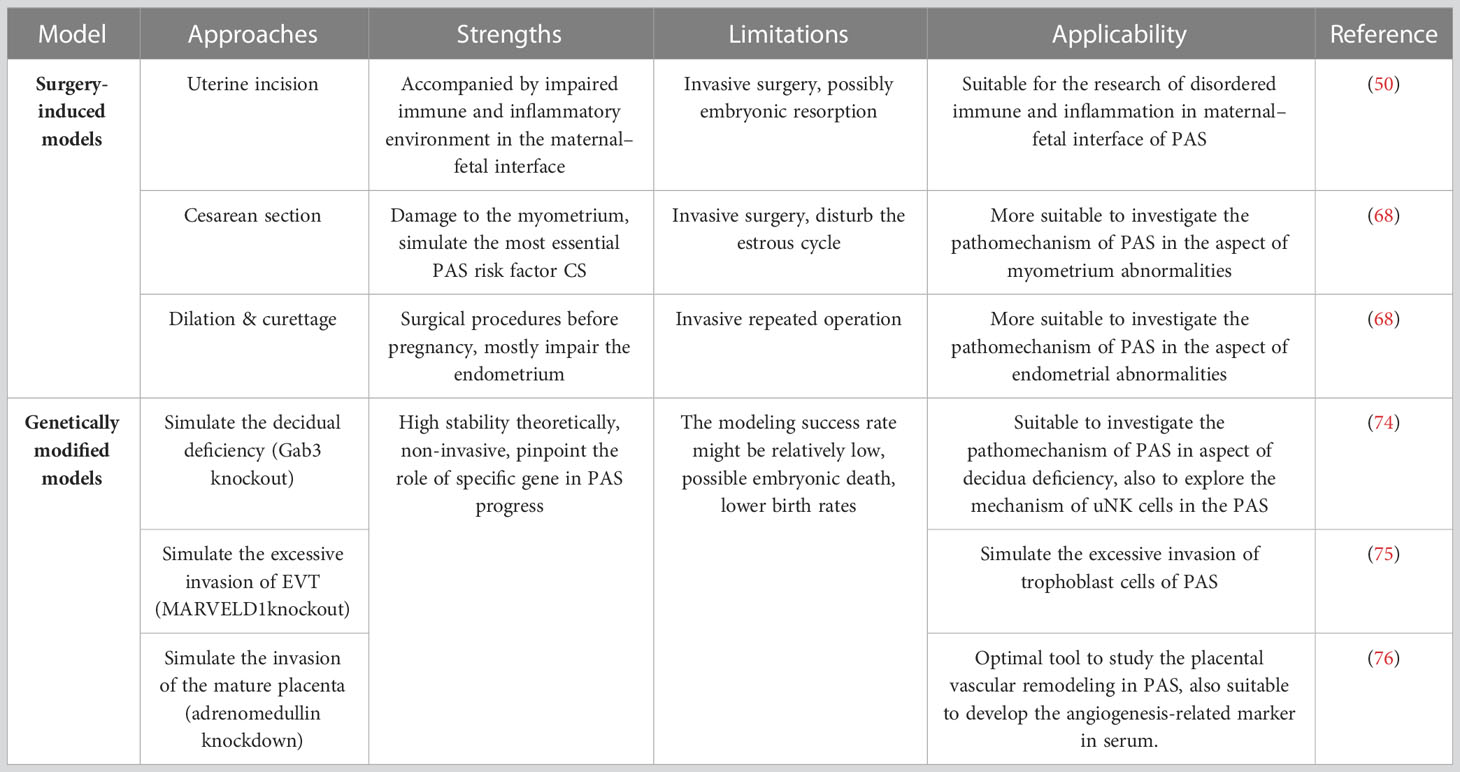
Table 1 Summary of modeling methods, strengths and limitations, and applicability of the PAS mouse models.
3.2 Genetically modified models
With the development of the clustered regularly interspaced short palindromic repeat-associated nuclease 9 (CRISPR−Cas9) and the cyclization recombinase locus of X (cross)-over in P1(Cre-loxP) target genome editing systems, genetically modified mice have been widely used and are considered to be an ideal tool to study molecular and genetic pathways in multiple diseases, especially cancers and some monogenic diseases (77). In addition, genetically modified animal models are also researched in other pregnancy-related diseases, such as GDM, PE and PAS.
Current genetically modified mouse models have been established to mimic the aberrant function of both “soil” and “seed.” Specifically, editing of some targeted genes could result in the abnormality of decidua, and editing of other targeted genes could result in excessive invasion of EVT or the mature placenta. The pathological phenotype and pregnancy outcome of each genetically modified model are depicted in the following sections.
3.2.1 Decidual deficiency
Normal decidua can prevent excessive invasion of trophoblast cells and suppress the maternal immune response to maintain implantation and decidualization (13). Incomplete or absent decidua may be involved in the pathogenesis of PAS (78).
Recently, a GRB2-associated binding protein 3 knockout (Gab3-/-) mouse model showed reduced decidual depth in mouse placentas and the spiral artery walls appeared to be heavily invaded by TGCs at approximately E12.5 (74). On E18.5, Gab3-/- mice showed a significant expansion of trophoblasts in the labyrinth and JZ in placentas, leading to an overall increase in the depth of the labyrinth, JZ, and uterine wall (74). GRB2-associated binding (Gab) family proteins, including Gab1–3, are involved in the assembly of intracellular activation signaling complexes by acting as scaffolds to perform docking functions (79, 80). As a regulator, Gab3 is essential for NK cells to perform their functions, including uterine NK (uNK) cells (74). uNK cells are predominantly located in the decidua and are required for the early development of the decidua base and vascular remodeling during pregnancy (81–87). Many studies have shown that uNK cell deficiency facilitates impaired trophoblast invasion in the mouse placenta (88–90). Gab3-/- mice exhibited impaired uNK cell expansion associated with abnormal spiral artery remodeling and increased trophoblast invasion in the decidua basalis (74). After Gab3 knockout, the average minimal distance between trophoblasts and the uterine wall was ~130 mm, whereas the average minimal distance from the wild-type mouse placenta was ~490 mm, which further indicated that Gab3 is a key component required for cytokine-mediated NK-cell priming and expansion that is essential for limiting trophoblast cell invasion during pregnancy (74).
3.2.2 Excessive invasion of EVT
As mentioned earlier, excessive invasion by EVT cells contributes to PAS onset (91). In addition to “soil” abnormalities, EVT invasion can be facilitated. Some genetic mutations related to cell-cycle activity can also lead to changes in the cell’s own ability to invade, which means abnormalities in “seed.” There are some animal models made by editing the genes that regulate the proliferation and invasion of cells to simulate this pathological phenotype of PAS.
In 2018, a genetically modified mouse model of PAS was developed by conditional knockout of MARVEL domain containing 1 (MARVELD1−/−), a tumor-suppressor gene located on human chromosome 10q24.2 (92). MARVELD1 has important biological functions in DNA damage repair, cell proliferation, metastasis, and invasion (93). It has been reported that the expression of MARVELD1 is ubiquitous in the endometrium, ovary, and other normal human tissues but is downregulated by promoter methylation in multiple primary tumors derived from the ovary, uterus, breast, testis, and so on (94–97). Yue Chen et al. reported that MARVELD1 was highly expressed in the wild-type mouse placenta, and its expression was increased in the placentas from E10.5 to E18.5, which paralleled ongoing trophoblast cell invasion. On E18.5, MARVELD1 was highly expressed in trophoblast cells that tended to migrate into the JZ (75). For the molecular mechanism, MARVELD1 binds to the integrin β4 promoter to activate its transcription, and active integrin β4 facilitates cell adhesion and suppresses cell migration. In the MARVELD1−/− mouse placenta, the boundary of the JZ was indistinct and was highly occupied by trophoblast cells, combined with the abnormal adhesion of the placenta to the myometrium, which is a placenta accreta phenotype exactly (75). On E18.5, placenta attached to the maternal uterus and the trophoblast cell invasion area into the maternal decidua was increased 3.83 times in MARVELD1−/− mouse placenta compared to the wild type, which was observed by cytokeratin 18 staining (75). All of these results could be explained by the signaling that the deletion of MARVELD1 resulted in decreased expression of integrin β4 in labyrinth layer trophoblast cells, and the adhesive ability of cells was suppressed, which boosted cell migration and invasion, leading to the excessive invasion of trophoblast cells and the placenta accreta phenotype in this model (75).
In regard to cancer and PAS, there are definitely some interesting parallels (98). For example, EVT cells and cancer cells both possess considerable invasion ability and epithelial–mesenchymal transformation (EMT) in the process of migration and invasion (99, 100). Therefore, some cell invasion, metastasis, angiogenesis, and immune-associated genes that have been elucidated in cancer development may be important regulators involved in PAS progression, and it could be one of the directions for research in the future.
3.2.3 Invasion of the placenta
Invasion of fetal placental tissue beyond the maternal decidua causes abnormal anchoring of the placenta to the uterine myometrium, which is the typical ultrasonographic manifestation of PAS patients (101, 102). Invasion of the placenta into the uterus is associated with significant maternal morbidity and mortality due to the possibility of hemorrhage during delivery (103). It has been reported that in adrenomedullin knockout heterozygotes (AM+/-), the fetal placental tissue invaded into the adjacent maternal–fetal interface, hypertrophied, and extended beyond the confines of the maternal decidua. H&E staining showed the abnormal invasion of TGCs, a small area of labyrinth layer, a significantly reduced spongiotrophoblast layer, and almost no maternal–fetal interface (76). In other words, the AM+/- intercross placenta completely lacked the normal histological characteristics of a wild-type placenta. All of these characteristics resemble the abnormal invasion of placental tissue into uterine tissue (76). In addition to morphological changes in the placenta, overcrowded conceptuses, a high incidence of fetal growth restriction (FGR) in AM+/– intercrosses, and embryonic loss occurring between E9.5 and E12.5 are the main reasons for the reduced fertility observed in female AM+/– mice (76). This is consistent with the circumstance that except for hemorrhage or other adverse maternal pregnancy outcomes, clinical PAS patients possibly have FGR in some cases (104–106).
Adrenomedullin (AM) is located on human chromosome 11p15.4. The protein encoded by this gene is a preprohormone that is cleaved to form two biologically active peptides, adrenomedullin and proadrenomedullin N-terminal 20 peptide. Adrenomedullin is a bioactive peptide consisting of 52 amino acids (107) that possesses a variety of biological functions, including vasodilation, regulation of hormone secretion, promotion of angiogenesis, and antimicrobial activity (108–111). Previous studies have shown that AM and its receptors are highly expressed in the uterus and placenta, especially in the fetal membranes and umbilical cord blood vessels, which could relax the placental blood vessels in a dose-dependent manner through autocrine or paracrine forms and reduce the resistance of placental blood vessels (112, 113). Abnormal AM levels are related to some pregnancy-related diseases, such as GDM and abortion (114, 115). All of these studies confirmed that adrenomedullin possesses multiple functions in both human pregnancy and animal models (116–118).
In regard to the regulation of placental vascular remodeling and fetal perfusion, in addition to the AM, many molecules have been elucidated to be clearly involved, including VEGF and placental growth factor (PlGF). Correspondingly, there are studies reporting that dysregulating placental vascular remodeling is clearly evident in the majority of PAS cases, which are combined with the alteration of VEGF, PlGF, and their receptors (VEGFR) in both the placenta and serum (119, 120). Therefore, genetically modified animal models based on the current understanding of molecular biological processes involved in the abnormal vascular remodeling of PAS will also become one of the paths to establish new PAS. For example, VEGF-targeted gene editing has been found to be an essential regulator in the placental vascular abnormalities of PAS (73, 120, 121).
Taken together, target gene editing is a non-invasive strategy with good theoretical stability to pinpoint the role of specific genes in disease onset and progression (122). Targeted gene editing in mice leads to abnormal decidua and excessive invasion of trophoblast cells or placenta, which is helpful to exploring the pathogenesis of PAS from both the perspective of “soil” and “seed” abnormalities. However, it should be emphasized that PAS is not a unifactorial disease caused by either uterine or placental abnormalities but is a multifactorial disease. The interactions of “soil” and “seed” lead to PAS. Therefore, when using genetically modified animals, one should not only observe one of the phenotypes but should be explored comprehensively. Additionally, there are still some limitations to genetically modified animals, complete gene knockout mice will lead to embryonic death or birth defects, while the construction cycle of the conditional knockout mouse model is relatively long (123). In addition, the incidence of PAS in targeted gene-edited mice might be relatively low. For instance, 45.95% of MARVELD1−/− mice exhibit the placenta accreta phenotype (75). Researchers should select appropriate target genes and gene editing methods according to the experimental design and objective.
4 Summary and prospects
The existing animal models mostly simulate the risk factors or pathological phenotypes by uterine surgeries or targeted gene editing in mice. Various surgeries, whether uterine incision, CS, or D&C, can damage the endometrium or myometrium, which could define the pathological mechanism of PAS from the perspective of “soil.” Genetically modified animal models can not only mimic the abnormal decidua but also simulate the excessive invasion of trophoblast cells or the placenta in PAS, which is helpful for exploring the pathogenesis of PAS from the perspectives of both the “soil” and the “seed” respectively.
Notably, since PAS is a multifactorial disease, it is caused by the interaction between the uterus and placenta. Therefore, the criterion for animal models should not be solely limited to the excessive invasiveness of EVT or the damaged uterus locally. In future studies of animal models, more systematic model evaluation criteria should be established to comprehensively and accurately simulate the clinical manifestations of PAS patients. In addition, when using animal models for research, attention should be given to the difference in the physiological structure of the experimental animal uterus and humans; for example, mice have a bichorned uterus, while surgical modeling is usually performed only on one side, and the unmanipulated uterine horn may be affected by the surgical side through paracrine or endocrine mechanisms. Except for existing genetically modified animal models, other genetically modified animal models based on the current understanding of molecular biological processes associated with PAS will also become one of the paths in the field of PAS animal model development. Undoubtedly, although little is known about the pathogenesis of PAS, the development and application of animal models will help advance the research of PAS to elucidate its pathological mechanism and possible treatment and prevention.
Author contributions
JM offered the main idea and significant guidance of this manuscript, YM and JM drafted the manuscript, and YH reviewed and revised the manuscript. All listed authors have agreed to the final submitted version.
Funding
This study was supported by the Ferring Institute of Reproductive Medicine (FIRM) Project fund (FIRMA181104) and the National Key Technologies R&D program of China (2016YFC1000303) to JM.
Acknowledgments
Part of the figures of this manuscript were created with BioRender.com. I would like to express my gratitude to Prof. Mana Parast for her support in drawing.
Conflict of interest
The authors declare that the research was conducted in the absence of any commercial or financial relationships that could be construed as a potential conflict of interest.
Publisher’s note
All claims expressed in this article are solely those of the authors and do not necessarily represent those of their affiliated organizations, or those of the publisher, the editors and the reviewers. Any product that may be evaluated in this article, or claim that may be made by its manufacturer, is not guaranteed or endorsed by the publisher.
References
1. Society of Gynecologic, American College of Obstetricians and Gynecologists and the Society for Maternal–Fetal Medicine, Cahill AG, Beigi R, Heine RP, Silver RM, Wax JR, et al. Placenta accreta spectrum. Am J Obstet Gynecol (2018) 219(6):B2–B16. doi: 10.1016/j.ajog.2018.09.042
2. Jauniaux E, Jurkovic D, Hussein AM, Burton GJ. New insights into the etiopathology of placenta accreta spectrum. Am J Obstetrics Gynecol (2022) 227(3):384–91. doi: 10.1016/j.ajog.2022.02.038
3. Jauniaux E, Ayres-de-Campos D. FIGO consensus guidelines on placenta accreta spectrum disorders: Introduction. Int J Gynaecol Obstet (2018) 140(3):261–4. doi: 10.1002/ijgo.12406
4. Silver RM, Branch DW. Placenta accreta spectrum. N Engl J Med (2018) 378(16):1529–36. doi: 10.1056/NEJMcp1709324
5. Jauniaux E, Chantraine F, Silver RM, Langhoff-Roos J. FIGO consensus guidelines on placenta accreta spectrum disorders: Epidemiology. Int J Gynaecol Obstet (2018) 140(3):265–73. doi: 10.1002/ijgo.12407
6. Jauniaux E, Bunce C, Grønbeck L, Langhoff-Roos J. Prevalence and main outcomes of placenta accreta spectrum: a systematic review and meta-analysis. Am J Obstet Gynecol (2019) 221(3):208–18. doi: 10.1016/j.ajog.2019.01.233
7. Einerson BD, Weiniger CF. Placenta accreta spectrum disorder: updates on anesthetic and surgical management strategies. Int J Obstet Anesth (2021) 46:102975. doi: 10.1016/j.ijoa.2021.102975
8. Laredo-Aguilera JA, Gallardo-Bravo M, Rabanales-Sotos JA, Cobo-Cuenca AI, Carmona-Torres JM. Physical activity programs during pregnancy are effective for the control of gestational diabetes mellitus. Int J Environ Res Public Health (2020) 17(17):6151. doi: 10.3390/ijerph17176151
9. Jauniaux E, Collins S, Burton GJ. Placenta accreta spectrum: pathophysiology and evidence-based anatomy for prenatal ultrasound imaging. Am J Obstet Gynecol (2018) 218(1):75–87. doi: 10.1016/j.ajog.2017.05.067
10. Usta IM, Hobeika EM, Musa AA, Gabriel GE, Nassar AH. Placenta previa-accreta: risk factors and complications. Am J Obstet Gynecol (2005) 193(3 Pt 2):1045–9. doi: 10.1016/j.ajog.2005.06.037
11. Shellhaas CS, Gilbert S, Landon MB, Varner MW, Leveno KJ, Hauth JC, et al. The frequency and complication rates of hysterectomy accompanying cesarean delivery. Obstet Gynecol (2009) 114(2 Pt 1):224–9. doi: 10.1097/AOG.0b013e3181ad9442
12. Jauniaux E, Bhide A, Kennedy A, Woodward P, Hubinont C, Collins S, et al. FIGO consensus guidelines on placenta accreta spectrum disorders: Prenatal diagnosis and screening. Int J Gynaecol Obstet (2018) 140(3):274–80. doi: 10.1002/ijgo.12408
13. Siriwardena D, Boroviak TE. Evolutionary divergence of embryo implantation in primates. Philos Trans R Soc Lond B Biol Sci (2022) 377(1865):20210256. doi: 10.1098/rstb.2021.0256
14. Murata H, Tanaka S, Okada H. The regulators of human endometrial stromal cell decidualization. Biomolecules (2022) 12(9). doi: 10.3390/biom12091275
15. Timor-Tritsch IE, Monteagudo A, Calì G, D'Antonio F, Kaelin Agten A. Cesarean scar pregnancy: Diagnosis and pathogenesis. Obstet Gynecol Clin North Am (2019) 46(4):797–811. doi: 10.1016/j.ogc.2019.07.009
16. Ben-Nagi J, Walker A, Jurkovic D, Yazbek J, Aplin JD. Effect of cesarean delivery on the endometrium. Int J Gynaecol Obstet (2009) 106(1):30–4. doi: 10.1016/j.ijgo.2009.02.019
17. Jauniaux E, Hussein AM, Elbarmelgy RM, Elbarmelgy RA, Burton GJ. Failure of placental detachment in accreta placentation is associated with excessive fibrinoid deposition at the utero-placental interface. Am J Obstet Gynecol (2022) 226(2):243 e1–243.e10. doi: 10.1016/j.ajog.2021.08.02
18. Jauniaux E, Hussein AM, Einerson BD, Silver RM. Debunking 20(th) century myths and legends about the diagnosis of placenta accreta spectrum. Ultrasound Obstet Gynecol (2022) 59(4):417–23. doi: 10.1002/uog.24890
19. Hemberger M, Hanna CW, Dean W. Mechanisms of early placental development in mouse and humans. Nat Rev Genet (2020) 21(1):27–43. doi: 10.1038/s41576-019-0169-4
20. Soncin F, Natale D, Parast MM. Signaling pathways in mouse and human trophoblast differentiation: a comparative review. Cell Mol Life Sci (2015) 72(7):1291–302. doi: 10.1007/s00018-014-1794-x
21. Rossant J, Cross JC. Placental development: lessons from mouse mutants. Nat Rev Genet (2001) 2(7):538–48. doi: 10.1038/35080570
22. Woods L, Perez-Garcia V, Hemberger M. Regulation of placental development and its impact on fetal growth-new insights from mouse models. Front Endocrinol (Lausanne) (2018) 9:570. doi: 10.3389/fendo.2018.00570
23. Maltepe E, Fisher SJ. Placenta: the forgotten organ. Annu Rev Cell Dev Biol (2015) 31:523–52. doi: 10.1146/annurev-cellbio-100814-125620
24. Soares MJ, Varberg KM, Iqbal K. Hemochorial placentation: development, function, and adaptations. Biol Reprod (2018) 99(1):196–211. doi: 10.1093/biolre/ioy049
25. Silva JF, Serakides R. Intrauterine trophoblast migration: A comparative view of humans and rodents. Cell Adh Migr (2016) 10(1-2):88–110. doi: 10.1080/19336918.2015.1120397
26. Cross JC, Baczyk D, Dobric N, Hemberger M, Hughes M, Simmons DG, et al. Genes, development and evolution of the placenta. Placenta (2003) 24(2-3):123–30. doi: 10.1053/plac.2002.0887
27. Sones JL, Davisson RL. Preeclampsia, of mice and women. Physiol Genomics (2016) 48(8):565–72. doi: 10.1152/physiolgenomics.00125.2015
28. Blum JL, Chen LC, Zelikoff JT. Exposure to ambient particulate matter during specific gestational periods produces adverse obstetric consequences in mice. Environ Health Perspect (2017) 125(7):077020. doi: 10.1289/EHP1029
29. Ramathal CY, Bagchi IC, Taylor RN, Bagchi MK. Endometrial decidualization: of mice and men. Semin Reprod Med (2010) 28(1):17–26. doi: 10.1055/s-0029-1242989
30. Woods L, Perez-Garcia V, Kieckbusch J, Wang X, DeMayo F, Colucci F, et al. Decidualisation and placentation defects are a major cause of age-related reproductive decline. Nat Commun (2017) 8(1):352. doi: 10.1038/s41467-017-00308-x
31. Brosens I, Puttemans P, Benagiano G. Placental bed research: I. the placental bed: from spiral arteries remodeling to the great obstetrical syndromes. Am J Obstet Gynecol (2019) 221(5):437–56.
32. Ng SW, Norwitz GA, Pavlicev M, Tilburgs T, Simón C, Norwitz ER, et al. Endometrial decidualization: The primary driver of pregnancy health. Int J Mol Sci (2020) 21(11). doi: 10.3390/ijms21114092
33. Perez-Garcia V, Fineberg E, Wilson R, Murray A, Mazzeo CI, Tudor C, et al. Placentation defects are highly prevalent in embryonic lethal mouse mutants. Nature (2018) 555(7697):463–8. doi: 10.1038/nature26002
34. Carter AM. Animal models of human pregnancy and placentation: alternatives to the mouse. Reproduction (2020) 160(6):R129–43. doi: 10.1530/REP-20-0354
35. Ueno M, Lee LK, Chhabra A, Kim YJ, Sasidharan R, Van Handel B, et al. C-met-dependent multipotent labyrinth trophoblast progenitors establish placental exchange interface. Dev Cell (2013) 27(4):373–86. doi: 10.1016/j.devcel.2013.10.019
36. Natale BV, Schweitzer C, Hughes M, Globisch MA, Kotadia R, Tremblay E, et al. Sca-1 identifies a trophoblast population with multipotent potential in the mid-gestation mouse placenta. Sci Rep (2017) 7(1):5575. doi: 10.1038/s41598-017-06008-2
37. Shiura H, Ono R, Tachibana S, Kohda T, Kaneko-Ishino T, Ishino F, et al. PEG10 viral aspartic protease domain is essential for the maintenance of fetal capillary structure in the mouse placenta. Development (2021) 148(19). doi: 10.1101/2021.03.02.433660
38. Elmore SA, Cochran RZ, Bolon B, Lubeck B, Mahler B, Sabio D, et al. Histology atlas of the developing mouse placenta. Toxicol Pathol (2022) 50(1):60–117. doi: 10.1177/01926233211042270
39. De Clercq K, Lopez-Tello J, Vriens J, Sferruzzi-Perri AN. Double-label immunohistochemistry to assess labyrinth structure of the mouse placenta with stereology. Placenta (2020) 94:44–7. doi: 10.1016/j.placenta.2020.03.014
40. Simmons DG, Fortier AL, Cross JC. Diverse subtypes and developmental origins of trophoblast giant cells in the mouse placenta. Dev Biol (2007) 304(2):567–78. doi: 10.1016/j.ydbio.2007.01.009
41. Fan X, Muruganandan S, Shallie PD, Dhal S, Petitt M, Nayak NR. VEGF maintains maternal vascular space homeostasis in the mouse placenta through modulation of trophoblast giant cell functions. Biomolecules (2021) 11(7). doi: 10.3390/biom11071062
42. Singh S, Carusi DA, Wang P, Reitman-Ivashkov E, Landau R, Fields KG, et al. External validation of a multivariable prediction model for placenta accreta spectrum. Anesth Analg (2022). doi: 10.1213/ANE.0000000000006222
43. Hobson SR, Kingdom JC, Murji A, Windrim RC, Carvalho JCA, Singh SS, et al. No. 383-screening, diagnosis, and management of placenta accreta spectrum disorders. J Obstet Gynaecol Can (2019) 41(7):1035–49.
44. Fitzpatrick KE, Sellers S, Spark P, Kurinczuk JJ, Brocklehurst P, Knight M, et al. Incidence and risk factors for placenta accreta/increta/percreta in the UK: a national case-control study. PloS One (2012) 7(12):e52893. doi: 10.1371/journal.pone.0052893
45. Thurn L, Lindqvist PG, Jakobsson M, Colmorn LB, Klungsoyr K, Bjarnadóttir RI, et al. Abnormally invasive placenta-prevalence, risk factors and antenatal suspicion: results from a large population-based pregnancy cohort study in the Nordic countries. BJOG (2016) 123(8):1348–55. doi: 10.1111/1471-0528.13547
46. De Mucio B, Serruya S, Alemán A, Castellano G, Sosa CG. A systematic review and meta-analysis of cesarean delivery and other uterine surgery as risk factors for placenta accreta. Int J Gynaecol Obstet (2019) 147(3):281–91. doi: 10.1002/ijgo.12948
47. Baldwin HJ, Patterson JA, Nippita TA, Torvaldsen S, Ibiebele I, Simpson JM. Antecedents of abnormally invasive placenta in primiparous women: Risk associated with gynecologic procedures. Obstet Gynecol (2018) 131(2):227–33. doi: 10.1097/AOG.0000000000002434
48. Tikhonova NB, Milovanov AP, Aleksankina VV, Fokina TV, Boltovskaya MN, Aleksankin AP, et al. Analysis of healing of rat uterine wall after full-thickness surgical incision. Bull Exp Biol Med (2021) 172(1):100–4. doi: 10.1007/s10517-021-05340-y
49. Lethaby A, Vollenhoven B, Sowter M. Efficacy of pre-operative gonadotrophin hormone releasing analogues for women with uterine fibroids undergoing hysterectomy or myomectomy: a systematic review. BJOG (2002) 109(10):1097–108. doi: 10.1111/j.1471-0528.2002.01225.x
50. Zhou J, Chen H, Xu X, Liu Y, Chen S, Yang S, et al. Uterine damage induces placenta accreta and immune imbalance at the maternal-fetal interface in the mouse. Placenta (2022) 119:8–16. doi: 10.1016/j.placenta.2022.01.002
51. Chen Y, Wang L, Bao J, Sha X, Cui L, Huang Q, et al. Persistent hypoxia induced autophagy leading to invasiveness of trophoblasts in placenta accreta. J Matern Fetal Neonatal Med (2021) 34(8):1297–303. doi: 10.1080/14767058.2019.1635582
52. El-Hussieny M, Mohammed EM, Zenhom NM, Refaie MM, Okasha AM, Tawab MAE. Possible role of TGF-beta1, MMP-2, e-CAD, beta-catenin and antioxidants in pathogenesis of placenta accreta. Fetal Pediatr Pathol (2021) 40(3):222–32. doi: 10.1080/15513815.2020.1843574
53. Jauniaux E, Zosmer N, De Braud LV, Ashoor G, Ross J, Jurkovic D. Development of the utero-placental circulation in cesarean scar pregnancies: a case-control study. Am J Obstet Gynecol (2022) 226(3):399.e1–399.e10. doi: 10.1016/j.ajog.2021.08.056
54. Lu R, Chu R, Wang Q, Xu Y, Zhao Y, Tao G, et al. Role of abdominal aortic balloon placement in planned conservative management of placenta previa with placenta increta or percreta. Front Med (Lausanne) (2021) 8:767748. doi: 10.3389/fmed.2021.767748
55. Che JH, Zheng ZM, Li MQ, Yao X. Macrophage polarization in placenta accreta and macrophage-trophoblast interactions. Am J Reprod Immunol (2022):e13611. doi: 10.1111/aji.13611
56. Hecht JL, Karumanchi SA, Shainker SA. Immune cell infiltrate at the utero-placental interface is altered in placenta accreta spectrum disorders. Arch Gynecol Obstet (2020) 301(2):499–507. doi: 10.1007/s00404-020-05453-1
57. Cornelius DC, Cottrell J, Amaral LM, LaMarca B. Inflammatory mediators: a causal link to hypertension during preeclampsia. Br J Pharmacol (2019) 176(12):1914–21. doi: 10.1111/bph.14466
58. Xie N, Jia Z, Li L. miR320a upregulation contributes to the development of preeclampsia by inhibiting the growth and invasion of trophoblast cells by targeting interleukin 4. Mol Med Rep (2019) 20(4):3256–64. doi: 10.3892/mmr.2019.10574
59. Ho L, van Dijk M, Chye STJ, Messerschmidt DM, Chng SC, Ong S, et al. ELABELA deficiency promotes preeclampsia and cardiovascular malformations in mice. Science (2017) 357(6352):707–13. doi: 10.1126/science.aam6607
60. Singh J, Ahmed A, Girardi G. Role of complement component C1q in the onsetof preeclampsia in mice. Hypertension (2011) 58(4):716–24. doi: 10.1161/HYPERTENSIONAHA.111.175919
61. Garrido-Gomez T, Castillo-Marco N, Cordero T, Simón C. Decidualization resistance in the origin of preeclampsia. Am J Obstet Gynecol (2022) 226(2S):S886–94. doi: 10.1016/j.ajog.2020.09.039
62. Imafuku H, Tanimura K, Shi Y, Uchida A, Deguchi M, Terai Y, et al. Clinical factors associated with a placenta accreta spectrum. Placenta (2021) 112:180–4. doi: 10.1016/j.placenta.2021.08.001
63. Zhang NN, Wang GW, Zuo N, Yang Q. Novel laparoscopic surgery for the repair of cesarean scar defect without processing scar resection. BMC Pregnancy Childbirth (2021) 21(1):815. doi: 10.1186/s12884-021-04281-8
64. Gao L, Chen H, Liu J, Wang M, Lin F, Yang G, et al. Extravillous trophoblast invasion and decidualization in cesarean scar pregnancies. Acta Obstet Gynecol Scand (2022) 101(10):1120–8. doi: 10.1111/aogs.14435
65. Berkley EM, Abuhamad A. Imaging of placenta accreta spectrum. Clin Obstet Gynecol (2018) 61(4):755–65. doi: 10.1097/GRF.0000000000000407
66. Li Z, Li Z, Bian X, Ma Y, Yang Q, Jia W, et al. Uterine scarring leads to adverse pregnant consequences by impairing the endometrium response to steroids. Endocrinology (2020) 161(11). doi: 10.1210/endocr/bqaa174
67. Ma J, Liu Y, Guo Z, Sun R, Yang X, Zheng W, et al. The diversity of trophoblast cells and niches of placenta accreta spectrum disorders revealed by single-cell RNA sequencing. Front Cell Dev Biol (2022) 10:1044198. doi: 10.3389/fcell.2022.1044198
68. Burke SD, Zsengellér ZK, Karumanchi SA, Shainker SA. A mouse model of placenta accreta spectrum. Placenta (2020) 99:8–15. doi: 10.1016/j.placenta.2020.06.006
69. Weng XL, Xie X, Liu CB, Yi JS. Postoperative reproductive results of infertile patients with intrauterine adhesions: A retrospective analysis. J Int Med Res (2022) 50(9):3000605221119664. doi: 10.1177/03000605221119664
70. Liu F, Hu S, Yang H, Li Z, Huang K, Su T, et al. Hyaluronic acid hydrogel integrated with mesenchymal stem cell-secretome to treat endometrial injury in a rat model of asherman's syndrome. Adv Healthc Mater (2019) 8(14):e1900411. doi: 10.1002/adhm.201900411
71. Ji W, Wen J, Lin W, He P, Hou B, Quan S, et al. Comparing the characteristics of amniotic membrane-, endometrium-, and urinary-derived ECMs and their effects on endometrial regeneration in a rat uterine injury model. Front Bioeng Biotechnol (2022) 10:861496. doi: 10.3389/fbioe.2022.861496
72. Ali H, Chandraharan E. Etiopathogenesis and risk factors for placental accreta spectrum disorders. Best Pract Res Clin Obstet Gynaecol (2021) 72:4–12. doi: 10.1016/j.bpobgyn.2020.07.006
73. Shainker SA, Silver RM, Modest AM, Hacker MR, Hecht JL, Salahuddin S, et al. Placenta accreta spectrum: biomarker discovery using plasma proteomics. Am J Obstet Gynecol (2020) 223(3):433.e1–433.e14. doi: 10.1016/j.ajog.2020.03.019
74. Sliz A, Locker KCS, Lampe K, Godarova A, Plas DR, Janssen EM, et al. Gab3 is required for IL-2- and IL-15-induced NK cell expansion and limits trophoblast invasion during pregnancy. Sci Immunol (2019) 4(38). doi: 10.1126/sciimmunol.aav3866
75. Chen Y, Zhang H, Han F, Yue L, Qiao C, Zhang Y, et al. The depletion of MARVELD1 leads to murine placenta accreta via integrin beta4-dependent trophoblast cell invasion. J Cell Physiol (2018) 233(3):2257–69. doi: 10.1002/jcp.26098
76. Li M, Yee D, Magnuson TR, Smithies O, Caron KM. Reduced maternal expression of adrenomedullin disrupts fertility, placentation, and fetal growth in mice. J Clin Invest (2006) 116(10):2653–62. doi: 10.1172/JCI28462
77. Wang SW, Gao C, Zheng YM, Yi L, Lu JC, Huang XY, et al. Current applications and future perspective of CRISPR/Cas9 gene editing in cancer. Mol Cancer (2022) 21(1):57. doi: 10.1186/s12943-022-01518-8
78. Kudo Y, Koh I, Yamazaki T, Oomori Y, Mukai Y, Sugimoto J, et al. Indoleamine 2,3-dioxygenase and trophoblast invasion in caesarean scar pregnancy: Implications for the aetiopathogenesis of placenta accreta spectrum. J Reprod Immunol (2020) 138:103099. doi: 10.1016/j.jri.2020.103099
79. Wohrle FU, Daly RJ, Brummer T. Function, regulation and pathological roles of the Gab/DOS docking proteins. Cell Commun Signal (2009) 7:22. doi: 10.1186/1478-811X-7-22
80. Nakaoka Y, Komuro I. Gab docking proteins in cardiovascular disease, cancer, and inflammation. Int J Inflam (2013), 141068. doi: 10.1155/2013/141068
81. Ashkar AA, Di Santo JP, Croy BA. Interferon gamma contributes to initiation of uterine vascular modification, decidual integrity, and uterine natural killer cell maturation during normal murine pregnancy. J Exp Med (2000) 192(2):259–70. doi: 10.1084/jem.192.2.259
82. Ratsep MT, Felker AM, Kay VR, Tolusso L, Hofmann AP, Croy BA, et al. Uterine natural killer cells: supervisors of vasculature construction in early decidua basalis. Reproduction (2015) 149(2):R91–102. doi: 10.1530/REP-14-0271
83. Xie M, Li Y, Meng YZ, Xu P, Yang YG, Dong S, et al. Uterine natural killer cells: A rising star in human pregnancy regulation. Front Immunol (2022) 13:918550. doi: 10.3389/fimmu.2022.918550
84. Sfakianoudis K, Rapani A, Grigoriadis S, Pantou A, Maziotis E, Kokkini G, et al. The role of uterine natural killer cells on recurrent miscarriage and recurrent implantation failure: From pathophysiology to treatment. Biomedicines (2021) 9(10). doi: 10.3390/biomedicines9101425
85. Shih AJ, Adelson RP, Vashistha H, Khalili H, Nayyar A, Puran R, et al. Single-cell analysis of menstrual endometrial tissues defines phenotypes associated with endometriosis. BMC Med (2022) 20(1):315. doi: 10.1186/s12916-022-02500-3
86. Quenby S, Nik H, Innes B, Lash G, Turner M, Drury J, et al. Uterine natural killer cells and angiogenesis in recurrent reproductive failure. Hum Reprod (2009) 24(1):45–54.
87. Long W, Shi Z, Fan S, Liu L, Lu Y, Guo X, et al. Association of maternal KIR and fetal HLA-c genes with the risk of preeclampsia in the Chinese han population. Placenta (2015) 36(4):433–7. doi: 10.1016/j.placenta.2014.05.008
88. Gomaa MF, Serag Eldeen IF, Farid LA, El-Saeed MME, Abas AM, Aawd NM, et al. Uterine natural killer cells dysregulation in idiopathic human preterm birth: a pilot study. J Matern Fetal Neonatal Med (2017) 30(15):1782–6. doi: 10.1080/14767058.2016.1224840
89. Moffett A, Hiby SE, Sharkey AM. The role of the maternal immune system in the regulation of human birthweight. Philos Trans R Soc Lond B Biol Sci (2015) 370(1663):20140071. doi: 10.1098/rstb.2014.0071
90. Tan HX, Yang SL, Li MQ, Wang HY. Autophagy suppression of trophoblast cells induces pregnancy loss by activating decidual NK cytotoxicity and inhibiting trophoblast invasion. Cell Commun Signal (2020) 18(1):73. doi: 10.1186/s12964-020-00579-w
91. Duan L, Schimmelmann M, Wu Y, Reisch B, Faas M, Kimmig R, et al. CCN3 signaling is differently regulated in placental diseases preeclampsia and abnormally invasive placenta. Front Endocrinol (Lausanne) (2020) 11:597549. doi: 10.3389/fendo.2020.597549
92. Mimori K, Shiraishi T, Mashino K, Sonoda H, Yamashita K, Yoshinaga K, et al. MAL gene expression in esophageal cancer suppresses motility, invasion and tumorigenicity and enhances apoptosis through the fas pathway. Oncogene (2003) 22(22):3463–71. doi: 10.1038/sj.onc.1206378
93. Sanchez-Pulido L, Martín-Belmonte F, Valencia A, Alonso MA. MARVEL: a conserved domain involved in membrane apposition events. Trends Biochem Sci (2002) 27(12):599–601. doi: 10.1016/S0968-0004(02)02229-6
94. Zhang J, Li Q, Sun Q, Wang B, Cui Y, Lou C, et al. Epigenetic modifications inhibit the expression of MARVELD1 and in turn tumorigenesis by regulating the wnt/beta-catenin pathway in pan-cancer. J Cancer (2022) 13(1):225–42. doi: 10.7150/jca.63608
95. Liu W, Han F, Qu S, Yao Y, Zhao J, Akhtar ML, et al. MARVELD1 depletion leads to dysfunction of motor and cognition via regulating glia-dependent neuronal migration during brain development. Cell Death Dis (2018) 9(10):999. doi: 10.1038/s41419-018-1027-6
96. Fekete JT, Ősz Á, Pete I, Nagy GR, Vereczkey I, Győrffy B, et al. Predictive biomarkers of platinum and taxane resistance using the transcriptomic data of 1816 ovarian cancer patients. Gynecol Oncol (2020) 156(3):654–61. doi: 10.1016/j.ygyno.2020.01.006
97. Ma W, Shen H, Li Q, Song H, Guo Y, Li F, et al. MARVELD1 attenuates arsenic trioxide-induced apoptosis in liver cancer cells by inhibiting reactive oxygen species production. Ann Transl Med (2019) 7(9):200. doi: 10.21037/atm.2019.04.38
98. Wagner GP, Kshitiz Dighe A, Levchenko A. The coevolution of placentation and cancer. Annu Rev Anim Biosci (2022) 10:259–79. doi: 10.1146/annurev-animal-020420-031544
99. Illsley NP, DaSilva-Arnold SC, Zamudio S, Alvarez M, Al-Khan A. Trophoblast invasion: Lessons from abnormally invasive placenta (placenta accreta). Placenta (2020) 102:61–6. doi: 10.1016/j.placenta.2020.01.004
100. Sun M, Gao J, Meng T, Liu S, Chen H, Liu Q, et al. Cyclin G2 upregulation impairs migration, invasion, and network formation through RNF123/Dvl2/JNK signaling in the trophoblast cell line HTR8/SVneo, a possible role in preeclampsia. FASEB J (2021) 35(2):e21169. doi: 10.1096/fj.202001559RR
101. Kazandi M. Placenta percreta: report of two cases and review of the literature. Clin Exp Obstet Gynecol (2003) 30(1):70–2.
102. Jauniaux E, Alfirevic Z, Bhide AG, Belfort MA, Burton GJ, Collins SL, et al. Placenta praevia and placenta accreta: Diagnosis and management: Green-top guideline no. 27a. BJOG (2019) 126(1):e1–e48. doi: 10.1111/1471-0528.15306
103. Booker W, Moroz L. Abnormal placentation. Semin Perinatol (2019) 43(1):51–9. doi: 10.1053/j.semperi.2018.11.009
104. Okada M, Mitsui T, Morita T, Nomura M, Ohta T, Itakura A. A case of placenta accreta complicated by severe IUGR and maternal coagulopathy. Acta Obstet Gynecol Scand (2007) 86(6):760–2. doi: 10.1080/00016340600617312
105. Vettorazzi J, Salazar CC, Bigolin André, Raupp GdS, Cosner AM, Garcia TS, et al. Can necrosis and ovarian vein thrombus be a serious complication in morbidly adherent placenta? Open J Obstetrics Gynecol (2021) 11(11):1477–83. doi: 10.4236/ojog.2021.1111138
106. da Cunha AC, da Silveira Betat R, Dal Pai TK, Arcolini CP, Gobatto AM, de Holleben Bicca AM, et al. Prenatal diagnosis of a true umbilical cord knot in a fetus with intrauterine growth restriction and placenta accreta. Taiwan J Obstet Gynecol (2016) 55(4):616–7. doi: 10.1016/j.tjog.2015.02.007
107. Fischer JP, Els-Heindl S, Beck-Sickinger AG. Adrenomedullin - current perspective on a peptide hormone with significant therapeutic potential. Peptides (2020) 131:170347. doi: 10.1016/j.peptides.2020.170347
108. Boorsma EM, Ter Maaten JM, Damman K, van Essen BJ, Zannad F, van Veldhuisen DJ, et al. Albuminuria as a marker of systemic congestion in patients with heart failure. Eur Heart J (2022). doi: 10.1093/eurheartj/ehac528
109. Kita T, Ashizuka S, Takeda T, Matsumoto T, Ohmiya N, Nakase H, et al. Adrenomedullin for biologic-resistant crohn's disease: A randomized, double-blind, placebo-controlled phase 2a clinical trial. J Gastroenterol Hepatol (2022) 37(11):2051–9. doi: 10.1111/jgh.15945
110. Corr MP, Fairley D, McKenna JP, Shields MD, Waterfield T. Diagnostic value of mid-regional pro-adrenomedullin as a biomarker of invasive bacterial infection in children: a systematic review. BMC Pediatr (2022) 22(1):176. doi: 10.1186/s12887-022-03255-9
111. Martinez-Herrero S, Martinez A. Adrenomedullin: Not just another gastrointestinal peptide. Biomolecules (2022) 12(2). doi: 10.3390/biom12020156
112. Thota C, Gangula PR, Dong YL, Yallampalli C. Changes in the expression of calcitonin receptor-like receptor, receptor activity-modifying protein (RAMP) 1, RAMP2, and RAMP3 in rat uterus during pregnancy, labor, and by steroid hormone treatments. Biol Reprod (2003) 69(4):1432–7. doi: 10.1095/biolreprod.103.015628
113. Nikitenko LL, Brown NS, Smith DM, MacKenzie IZ, Bicknell R, Rees MC, et al. Differential and cell-specific expression of calcitonin receptor-like receptor and receptor activity modifying proteins in the human uterus. Mol Hum Reprod (2001) 7(7):655–64. doi: 10.1093/molehr/7.7.655
114. Wilson C, Nikitenko LL, Sargent IL, Rees MC. Adrenomedullin: multiple functions in human pregnancy. Angiogenesis (2004) 7(3):203–12. doi: 10.1007/s10456-004-4183-5
115. Di Iorio R, Marinoni E, Letizia C, Cosmi EV. Adrenomedullin in perinatal medicine. Regul Pept (2003) 112(1-3):103–13. doi: 10.1016/S0167-0115(03)00028-4
116. Lenhart PM, Caron KM. Adrenomedullin and pregnancy: perspectives from animal models to humans. Trends Endocrinol Metab (2012) 23(10):524–32. doi: 10.1016/j.tem.2012.02.007
117. Matson BC, Caron KM. Adrenomedullin and endocrine control of immune cells during pregnancy. Cell Mol Immunol (2014) 11(5):456–9. doi: 10.1038/cmi.2014.71
118. Li M, Schwerbrock NM, Lenhart PM, Fritz-Six KL, Kadmiel M, Christine KS, et al. Fetal-derived adrenomedullin mediates the innate immune milieu of the placenta. J Clin Invest (2013) 123(6):2408–20. doi: 10.1172/JCI67039
119. Li SC, Lan KC, Hung HN, Huang WT, Lai YJ, Cheng HH, et al. HSPA4 is a biomarker of placenta accreta and enhances the angiogenesis ability of vessel endothelial cells. Int J Mol Sci (2022) 23(10). doi: 10.3390/ijms23105682
120. Duzyj CM, Buhimschi IA, Laky CA, Cozzini G, Zhao G, Wehrum M, et al. Extravillous trophoblast invasion in placenta accreta is associated with differential local expression of angiogenic and growth factors: a cross-sectional study. BJOG (2018) 125(11):1441–8. doi: 10.1111/1471-0528.15176
121. Alzoubi O, Maaita W, Madain Z, Alzoubi M, Sweis JJG, Arar AR, et al. Association between placenta accreta spectrum and third-trimester serum levels of vascular endothelial growth factor, placental growth factor, and soluble fms-like tyrosine kinase-1: A meta-analysis. J Obstet Gynaecol Res (2022) 48(9):2363–76. doi: 10.1111/jog.15330
122. Liu R, Liang L, Freed EF, Gill RT. Directed evolution of CRISPR/Cas systems for precise gene editing. Trends Biotechnol (2021) 39(3):262–73. doi: 10.1016/j.tibtech.2020.07.005
Keywords: placenta accreta spectrum, animal model, placenta development, mouse placenta, trophoblast invasion, decidual deficiency
Citation: Ma Y, Hu Y and Ma J (2023) Animal models of the placenta accreta spectrum: current status and further perspectives. Front. Endocrinol. 14:1118168. doi: 10.3389/fendo.2023.1118168
Received: 07 December 2022; Accepted: 20 March 2023;
Published: 08 May 2023.
Edited by:
Senthilkumar Palaniappan, Karpagam Academy of Higher Education, IndiaCopyright © 2023 Ma, Hu and Ma. This is an open-access article distributed under the terms of the Creative Commons Attribution License (CC BY). The use, distribution or reproduction in other forums is permitted, provided the original author(s) and the copyright owner(s) are credited and that the original publication in this journal is cited, in accordance with accepted academic practice. No use, distribution or reproduction is permitted which does not comply with these terms.
*Correspondence: Yongyan Hu, aHV5eWdAMTYzLmNvbQ==; Jingmei Ma, amluZ21laW1hQGJqbXUuZWR1LmNu