- Tianjin Key Laboratory of Retinal Functions and Diseases, Tianjin Branch of National Clinical Research Center for Ocular Disease, Eye Institute and School of Optometry, Tianjin Medical University Eye Hospital, Tianjin, China
Retinal neovascular, neurodegenerative, and inflammatory diseases represented by diabetic retinopathy are the main types of blinding eye disorders that continually cause the increased burden worldwide. Pigment epithelium-derived factor (PEDF) is an endogenous factor with multiple effects including neurotrophic activity, anti-angiogenesis, anti-tumorigenesis, and anti-inflammatory activity. PEDF activity depends on the interaction with the proteins on the cell surface. At present, seven independent receptors, including adipose triglyceride lipase, laminin receptor, lipoprotein receptor-related protein, plexin domain-containing 1, plexin domain-containing 2, F1-ATP synthase, and vascular endothelial growth factor receptor 2, have been demonstrated and confirmed to be high affinity receptors for PEDF. Understanding the interactions between PEDF and PEDF receptors, their roles in normal cellular metabolism and the response the initiate in disease will be accommodating for elucidating the ways in which inflammation, angiogenesis, and neurodegeneration exacerbate disease pathology. In this review, we firstly introduce PEDF receptors comprehensively, focusing particularly on their expression pattern, ligands, related diseases, and signal transduction pathways, respectively. We also discuss the interactive ways of PEDF and receptors to expand the prospective understanding of PEDF receptors in the diagnosis and treatment of retinal diseases.
1 Introduction
Pigment epithelium-derived factor (PEDF), also known as serpin family F member 1 (SERPINF1), is a 50 kDa protein encoded by the SERPINE1 gene (1). The human gene encoding PEDF is located on chromosome 17p13.3 and comprises 9 exons encoding a 418 amino acid protein (NCBI Reference Sequence: NG_028180.1). PEDF belongs to the serine protease inhibitor superfamily (SERPINS), the members of which share a common structural element, a reactive center loop (RCL) (2). Most SERPINS members are serine protease inhibitors, including plasmin inhibitors, antichymotrypsin, and antitrypsin (3). However, PEDF is considered to be a noninhibitory serpin as it is activated after chymotrypsin RCL cleavage and lacks protease activity (4).
In 1991, Joyce Tombran-Tink et al. first discovered that medium from retinal pigment epithelial (RPE) cells induced Y79 retinoblastoma tumor cell differentiation and increased tumor cell neurite outgrowth. Considering these findings, they identified the protein with the neurotrophic function induced by RPE cell-conditioned medium and termed it PEDF (1, 5). In addition to exerting as a neurotrophic factor, PEDF was recognized for its other functions. One decade after its discovery, PEDF was shown to protect motor neurons from chronic glutamate-mediated neurodegeneration (6) and protect photoreceptors from light damage (7, 8). In addition, PEDF is considered to be a potent angiogenesis inhibitor, which contributes to its important role in both antiangiogenesis and antitumorigenesis (9, 10). This discovery initiated a new era of PEDF function and mechanism exploration in angiogenic and degenerative diseases, especially in diabetes and diabetic complications.
An increasing number of studies have shown that the PEDF mechanism of action and related molecular pathways are involved in mediating or inducing signal transduction. PEDF activity depends on the high affinity of PEDF for interacting proteins, namely PEDF receptors on the cell surface (11). Understanding the interactions between PEDF and PEDF receptors, their roles in normal cellular metabolism and the response the initiate in disease may help elucidate the ways in which inflammation, angiogenesis, and neurodegeneration exacerbate disease pathology. In this review, we introduce these PEDF receptors to readers in a comprehensive way and suggest the potential underlying mechanisms for diseases characterized by multipathological changes, including angiogenesis, neurodegeneration, and inflammation. This increase in understanding may lead to new approaches to combat these diseases.
2 PEDF distribution and expression
The mRNA encoding PEDF (SERPINF1 mRNA) has been identified in several kinds of normal human tissues and organs, including blood cells, plasma, lymph nodes, brain, spinal cord, heart, arteries, muscle, lung, eye, bone, colon, kidney, liver, spleen, breast, thyroid, prostate, and pancreas (12). SERPINF1 mRNA is also expressed in embryonic ocular tissues. PEDF has been found in developing cones, RPE granules, neuroblasts and the ganglion cell layer (GCL) of developing human retinas (13). PEDF can also be found in the human amniotic membrane (14). In embryonic mouse eyes, PEDF is first detected on embryonic day (E) 14.5 in RPE cells, initially in the inner plexiform layer and subsequently, on E18.5, in the GCL layer, inner nuclear layer, choroid and ciliary body (15). In mouse embryonic livers, PEDF is found on E12.5, and gradually increases and maintains high expression in the liver (16). In addition, PEDF is highly expressed in murine primary long-term hematopoietic stem cells (HSCs) (17).
PEDF levels have been shown to be decreased in angiogenic tissues or organs, such as the retinas from patients with proliferative diabetic retinopathy (PDR) (18–24). PEDF exerts antiangiogenic effects by inducing endothelial cell apoptosis (25, 26), which contributes to its antitumor effects. On the other hand, PEDF directly promotes differentiation, inhibits proliferation, mediates tumor cell apoptosis, and ultimately abrogates tumors (12), such as melanomas (27, 28), pancreatic carcinomas (29), prostate tumors (30) and ovarian tumors (31). An increasing number of studies on PEDF have shown that this protein maintains the growth and development of important organs and supports important organ functions. For example, PEDF played cardioprotective roles by increasing glucose uptake in the ischemic myocardium, an effect mediated through PI3K/AKT signaling and GLUT4 translocation (32) and enhanced cardiac function by reducing hypoxia-induced cell injury (33). Pulmonary PEDF expression was increased in idiopathic pulmonary fibrosis and inversely correlated with pulmonary microvascular density and vascular endothelial growth factor (VEGF) levels (34). Plasma PEDF levels in chronic kidney disease patients were found to be significantly increased compared with those in patients without chronic kidney disease (35). In addition, renal PEDF levels were significantly decreased in a diabetic rat model (36). Exogenously overexpressed PEDF in mice resulted in the inhibition of retinal neovascularization (RNV) in an oxygen-induced retinopathy (OIR) model (37). However, PEDF deficiency caused severe vessel loss in the retinas of the OIR model mice (38).
The PEDF receptors discovered to date include patatin-like phospholipase domain-containing protein 2 (PNPLA2)/adipose triglyceride lipase (ATGL) (39), laminin receptor (25), lipoprotein receptor-related protein 6 (LRP6) (40), plexin domain-containing 1 (PLXDC1), PLXDC2 (41), F1-ATP synthase (42), and vascular endothelial growth factor receptor 2 (VEGFR2) (43). In this review, we expound on the distribution and function of each PEDF receptor, as well as the molecular signaling pathways in which they are involved (see Table 1).
3 Receptors that bind to PEDF
3.1 Adipose triglyceride lipase
The dynamic balance between energy intake and consumption is critical for maintaining the integrity of an organism. When calorie intake exceeds energy expenditure, the remaining energy is converted into fatty acids (FAs) and stored as triglycerides (TGs). However, when energy expenditure (EE) surpasses caloric intake, TG is hydrolyzed and FAs are released (80). Dysregulated lipolysis leads to an overabundance of circulating FAs, which leads to several destructive, lipotoxic pathologic changes, such as insulin resistance, type 2 diabetes, fatty liver, nonalcoholic fatty liver disease, and inflammation (44–47). In 2004, three independent institutes identified ATGL, the main enzyme critical for the initial step of TG degradation, and named it ATGL (81), iPLA2ζ (82), and desnutrin (83). To prevent confusion, we use ATGL in the following discussion. The human gene encoding ATGL is located on chromosome 11p15.5 and includes 10 exons encoding a 504 amino acid protein (NCBI Reference Sequence: NG_023394.1). As a key participant in lipid catabolism, ATGL is highly expressed in brown adipose tissue (BAT) and white adipose tissue (WAT). However, in nonadipose tissues such as skeletal muscle (84), heart (85), liver (86), lung (87), and ocular tissues, and especially in the neural retina and RPE cells (ARPE-19 and hTERT) (39), ATGL mRNA is highly expressed, although at a lower level than in adipose tissues. In addition, researchers found that ATGL was also expressed in human Y-79 cells (11), mouse photoreceptor (661W) cells (88), a rat photoreceptor precursor (R28) cell line (39) and bovine RPE cells (89). ATGL is mainly localized in TG-rich intracellular lipid droplets (LDs), and the catalytic site in human and murine ATGL comprises a dyad consisting of serine 47 and aspartate 166 at the N-terminus of the protein (81, 90). Notably, the following factors may affect ATGL localization: the C-terminal portion includes a hydrophobic LD-binding region, and mutation in the ATGL C-terminus can negatively affect AGTL-LD binding (44). In addition, ATGL binds LC3, an autophagosome marker that promotes ATGL recruitment to LDs (47).
ATGL and hormone-sensitive lipase (HSL) are the major lipases in adipocytes in humans and mice. Several factors are involved in the regulation of ATGL activity. During catecholamine-stimulated lipolysis, for example, PKA phosphorylates α/β hydrolase domain-containing 5 (ABHD5) and promotes ATGL release from the LD surface. The direct interaction of ABHD5 with ATGL promotes the hydrolysis of triacylglycerols (TAG) (91) and broadens region-specificity of ATGL, because as it no longer exclusively engages in sn-2 reactions and engages in more sn-1 and sn-2 reactions (92). Notably, in adipocytes, the combination of an adipocyte- or FA-binding protein with ABHD5 induces ATGL activity, probably by preventing the inhibition of ATGL protease activity (93). In nonadipocytes, ubiquitin regulatory X domain-containing protein 8 (UBXD8) interacts with ATGL and causes the dissociation of CGI-58, resulting in a decrease in ATGL activity (94). ATGL activity is activated by ABHD5 and inhibited by the G0S2 protein in a noncompetitive manner (95). Specifically, G0S2 engages in direct protein-protein interactions and thus impedes substrate accessibility (96). Hypoxia-inducible gene 2 (HIG2), a HIF-1 target, has recently been identified as an inhibitor of ATGL that directly interacts with the AT GL patatin-like phospholipase domain (97, 98).
PEDF is also involved in regulating ATGL (see Figure 1). As a secreted protein, PEDF binds to ATGL on the RPE cell membrane, mediating the first step in its biological effects (33). PEDF regulates lipid metabolic functions through a concomitant elevation in the scavenger receptor CD36. The crucial roles played by PEDF are thought to be mediated through ATGL activation (99). For example, PEDF enhances the nuclear import of the predominantly cytosolic ATGL protein to promote its subsequent proteasomal degradation in the nucleus, thus diminishing ATGL protein stability in a COP1-dependent manner (99). During the development of inflammation-associated hepatic steatosis, endogenous PEDF directly interacted with ATGL and was neutralized by TNF-α, possibly in a PPARα-dependent manner (100). Endothelial damage is critical to vascular leakage and the subsequent shock in sepsis patients. Elevated serum PEDF levels in patients with sepsis induced increased extravasation, which was induced by the abnormal expression of ATGL (101). In addition, both PEDF and the PEDF-derived peptide 44mer promoted cardiac triglyceride degeneration by binding to ATGL (33).
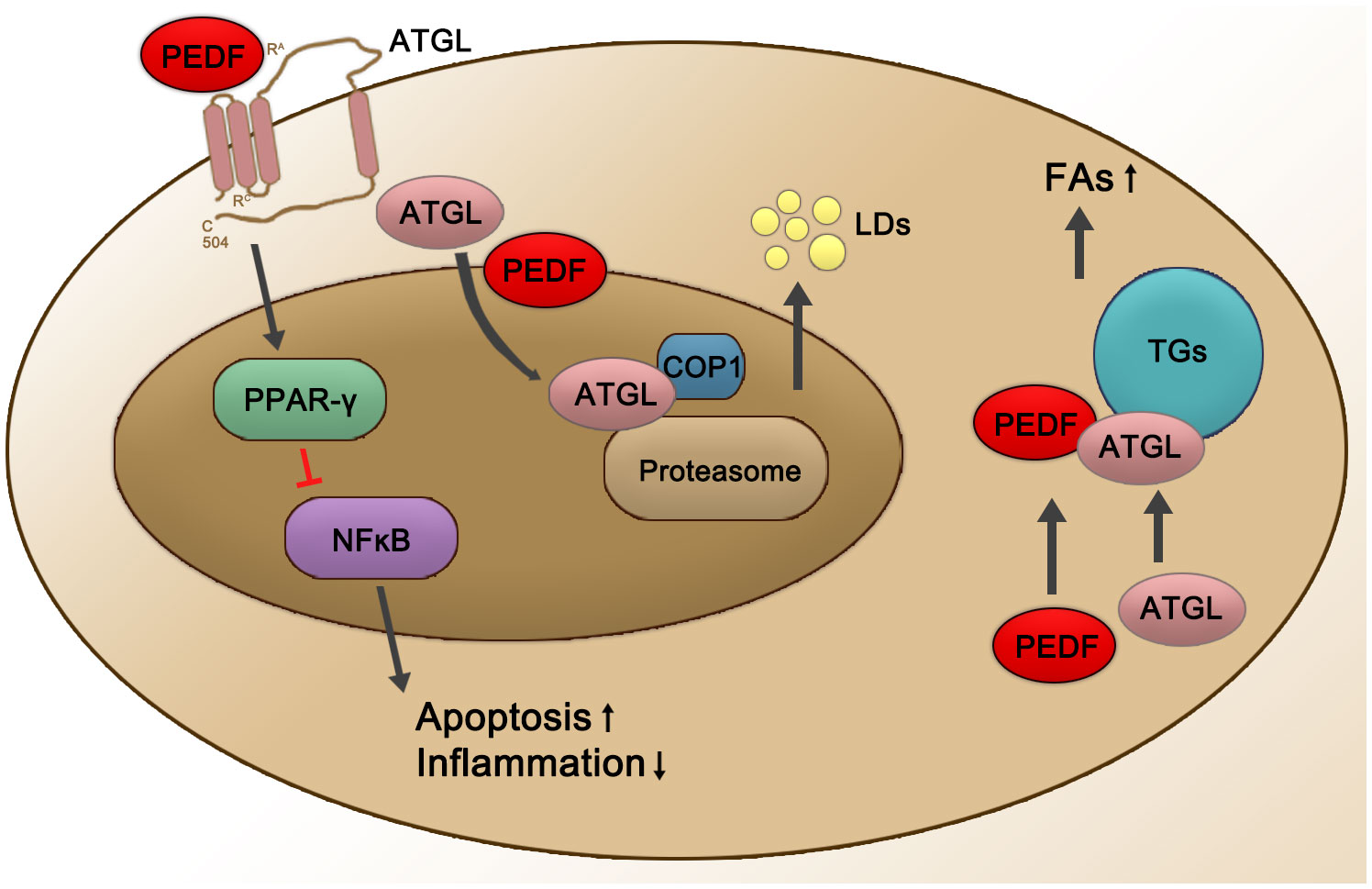
Figure 1 Schematic representation of PEDF-activated ATGL signaling. PEDF binds to the cell surface membrane receptor ATGL, which leads to the upregulation of PPARγ and then suppresses NFĸB at the transcriptional level, subsequently leading to decreased inflammation and an increased apoptosis rates. PEDF also enhances nuclear import of +predominantly cytosolic ATGL protein for its subsequent proteasomal degradation in the nucleus, thereby diminishing ATGL protein stability in a COP1-dependent manner, which enhances the release of LDs. In addition, the PEDF-ATGL interaction promotes the hydrolysis of TGs and the release of FAs. Red lines represent inhibited pathways, and black arrows show activated pathways. PPAR-γ, peroxisome proliferator-activated receptor γ; NFĸB, nuclear factor ĸB; COP1, constitutive photomorphogenic 1; LDs, lipid droplets; TGs, triglycerides; FAs, fatty acids.
ATGL is regulated by many factors at the transcriptional and post-transcriptional levels. In mice, ATGL controls energy supply and systemically hydrolyzes FAs, thereby influencing mitochondrial β-oxidation and the activation of peroxisome proliferator-activated receptors (PPARs) (102). ATGL decreases PPAR-γ signaling in the brain and suppresses appetite. Additionally, ATGL-deficient humans typically have obesity (103). At the transcriptional level, tumor necrosis factor-α (TNF-α) and insulin decrease ATGL expression, respectively. In this process, PPAR-γ interacts with the ATGL promoter and induces ATGL mRNA expression (104). Under starvation conditions, forkhead box protein O1 (FoxO1) showed increased affinity for the ATGL promoter (105), and this affinity was decreased by an increase in insulin level (106). Regarding the posttranscriptional phosphorylation of ATGL, studies with mice have shown that ATGL phosphorylation at Ser406 either by protein kinase A (PKA) or adenosine 5’-monophosphate-activated protein7 kinase (AMPK) increased ATGL activity (107, 108). In addition, fat-specific protein 27 (Fsp27) reduced ATGL transcript levels by regulating the affinity of early growth response proteins for the ATGL promoter in human adipocytes and thus decreased ATGL-mediated lipolysis (109, 110) (see Figure 1).
3.2 Lipoprotein receptor-related protein 6
Lipoprotein receptor-related protein 6 (LRP6) was first isolated by Sheryl D. Brown et al., who reported the chromosomal location of LRP6 on human chromosome 12 in 1998 (111). The low-density lipoprotein receptor (LDLR) family comprises cell surface receptors, and several members are involved in numerous signaling pathways and are expressed in various target organs (112). LRP6 is a unique member of the LDL receptor gene family, as indicated by sequence comparisons, and exhibits the closest relationship to the LRP5 gene, a possible therapeutic target for type 1 diabetes (113). Both LRP5 and LRP6 function as coreceptors in the Wnt/β-catenin pathway (111); nevertheless, studies have indicated that LRP5 and LRP6 act distinctly owing to differences in their tissue distribution and affinity for individual Wnt ligands (114). LRP5 and LRP6 are structurally related, exhibiting approximately 71% homology at the nucleotide level, and are broadly expressed in humans, including the hippocampus, renal tubular cells, hepatocytes, intestinal epithelial cells, osteoblasts, and osteoclasts (115). Although LRP5 and LRP6 are functionally and structurally similar, LRP6 is more closely related to glucose and lipid metabolism signaling pathways and plays a more crucial role in developmental processes than LRP5 (116–118).
The human LRP6 (hLRP6) gene is located on chromosome 12p13.2, is 150 kb long and consists of 23 exons. hLRP6 is highly evolutionarily conserved, with negligible differences observed among Drosophila, Xenopus and Mus (119). LRP6 is highly expressed in the heart, brain, placenta, spleen, testes and ovaries but is expressed at low levels in the liver, skeletal muscle, prostate and colon mucosa (111). Mice show LRP6 expression levels in the heart, brain, lungs and kidneys similar to those in humans (111). According to a βgeo reporter activity assay, LRP6 is an endogenous gene and has been detected in all types of developing embryo cells (120).
In mice, LRP6 is essential to the canonical and noncanonical Wnt signaling pathways. When the LRP6 gene carries an insertion mutation and an individual Wnt gene is mutated, Lrp6-/- homozygous embryos present developmental defects (120). Mice that lack LRP6 genes typically exhibit mid-/hindbrain defects, posterior limb truncations, and abnormal limb patterning, similar to Wnt7a-mutant mice (48). In addition, LRP6 plays an important role in the mouse forebrain (121). In Alzheimer’s disease (AD), a neurodegenerative disorder characterized by deficient cognitive processes and the accumulation of amyloid precursor protein (APP) and amyloid-β, LRP6 mutations are considered to be pathogenic factors. Notably, the conditional deletion of the LRP6 gene in mouse forebrain neuronal cells led to the destruction of synaptic integrity and memory loss in an age-dependent manner, potentially due to increased processing of APP in to amyloid-β (51). In addition, De Ferrari GV et al. showed that in two brain bank data series, a single nucleotide polymorphism (SNP) in exon 18 of LRP6 was associated with Alzheimer’s disease (49).
Recently, a reduction in Wnt-mediated transcription resulting from mutations in LRP6 has been confirmed to lead to several features of metabolic syndrome, including atherosclerosis (50), diabetes (55), hyperlipidemia (50, 53), and hypertension (54), but not obesity. Coronary artery disease (CAD) is usually caused by several risk factors of metabolic syndrome. In 2007, Mani et al. reported on a family with autosomal dominant early CAD, characterized by the features of hyperlipidemia, hypertension, and osteoporosis, which were found to be associated with a short piece of chromosome 12p; specifically, the group identified a mutation in LPR6 that encoded a coreceptor in the Wnt signaling pathway (52). The identified mutation damaged the Wnt signaling pathway in vitro because an arginine residue in the epidermal growth factor-like (EGF-like) domain was replaced with a cysteine residue. This finding encouraged the investigators to focus on the relationships between LRP6 and various human diseases, especially atherosclerosis (51).
After the binding of the Wnt ligand, the Fzd/LRP6 receptor and the scaffold protein Dishevelled (Dvl) form a signaling body that is endocytosed. The signaling body induced glycogen synthase kinase 3 (GSK3)-mediated phosphorylation of LRP6 on the PPPSP motif, which initiated the phosphorylation of adjacent S/T clusters through casein kinase 1γ (CK1γ) (122, 123). In addition to GSK3, other kinases phosphorylate the LRP6-PPPSP motif in a Wnt-independent manner. For example, due to the initiation of phosphorylation of cyclin-dependent kinase 14 (CDK14/PFTK1) and its regulators, mitotic cyclin Y and cyclin Y-like 1 (CCNY/CCNYL1), the ability of LRP6 to respond to Wnt ligands is greatest during the G2/M period (123–127). Phosphorylated LRP6 recruits a polyprotein called the “destruction complex”, which includes the scaffold protein Axin and Adenomatous Polyposis Coli(APC) tumor suppressors, the kinases CK1α and GSK3, and the protein E3 ubiquitin ligase β-transduction protein repeat sequence (β-TrCP). In the absence of Wnt ligands, CK1α and GSK3 in the destruction complex phosphorylate β-catenin, inducing β-TrCP-mediated degradation by the proteasome. Wnt/LRP6 signaling inhibits destruction complex activity through at least two mechanisms. First, GSK3 is the product of inhibited LRP6 phosphorylation. Second, the signaling body matures into a multivesicular body, in which the destruction complex is isolated with LRP6. Wnt/LRP6 signaling is enhanced by members of the R-sponge protein (RSPO) family of secreted proteins, which bind to the LGR4/5 receptor and the E3 protein ligase RNF43/ZNRF3 to stabilize the Wnt receptor on the cell surface (54, 128–133).
The canonical Wnt signaling pathway is a conservative intracellular signaling pathway. When the receptor complex on the cell surface, specifically, the Wnt-Fzd-LRP5/6 complex, binds to a Wnt ligand, the canonical Wnt pathway is rapidly activated. Then, Wnt phosphorylation of the cytoplasmic tail of the Fzd-LRP5/6 complex triggers the recruitment and phosphorylation of disheveled protein (also known as Dishevelled, Dvl) in the cytoplasm (134, 135). Subsequently, Axin is recruited to the receptor complex to inhibit its decomposition and the phosphorylation of β-catenin. The stable nuclear translocation of soluble β-catenin in the cytoplasm promotes the activation of the downstream Wnt/β-catenin signaling pathway via the transcription and translation of target genes (134, 135). In the absence of Wnt ligands or in the presence of Wnt receptor inhibitors, β-catenin is bound by Axin, Axin and casein kinase Ia (CKIa) and glycogen synthase kinase 3β (GSK3β); moreover, two APC protein kinases combine to form a destruction complex (134). CKIa and GSK3β mediate the phosphorylation of β-catenin in the cytoplasm. Phosphorylated β-catenin is recognized by b-TrCP and induces ubiquitination, which ultimately leads to the degradation of β-catenin (136). Many inhibitors of the Wnt signaling pathway, including Dickkopf WNT Signaling Pathway Inhibitor 1, DKK-1 (137), R-spondins (138), Norrin (139), endostatin (140), PEDF (40), and very low-density lipoprotein receptor, have been identified (141, 142). These negative regulatory molecules of the Wnt signaling pathway ultimately lead to the transcription of target genes, inducing cell proliferation, differentiation, or apoptosis (see Figure 2).
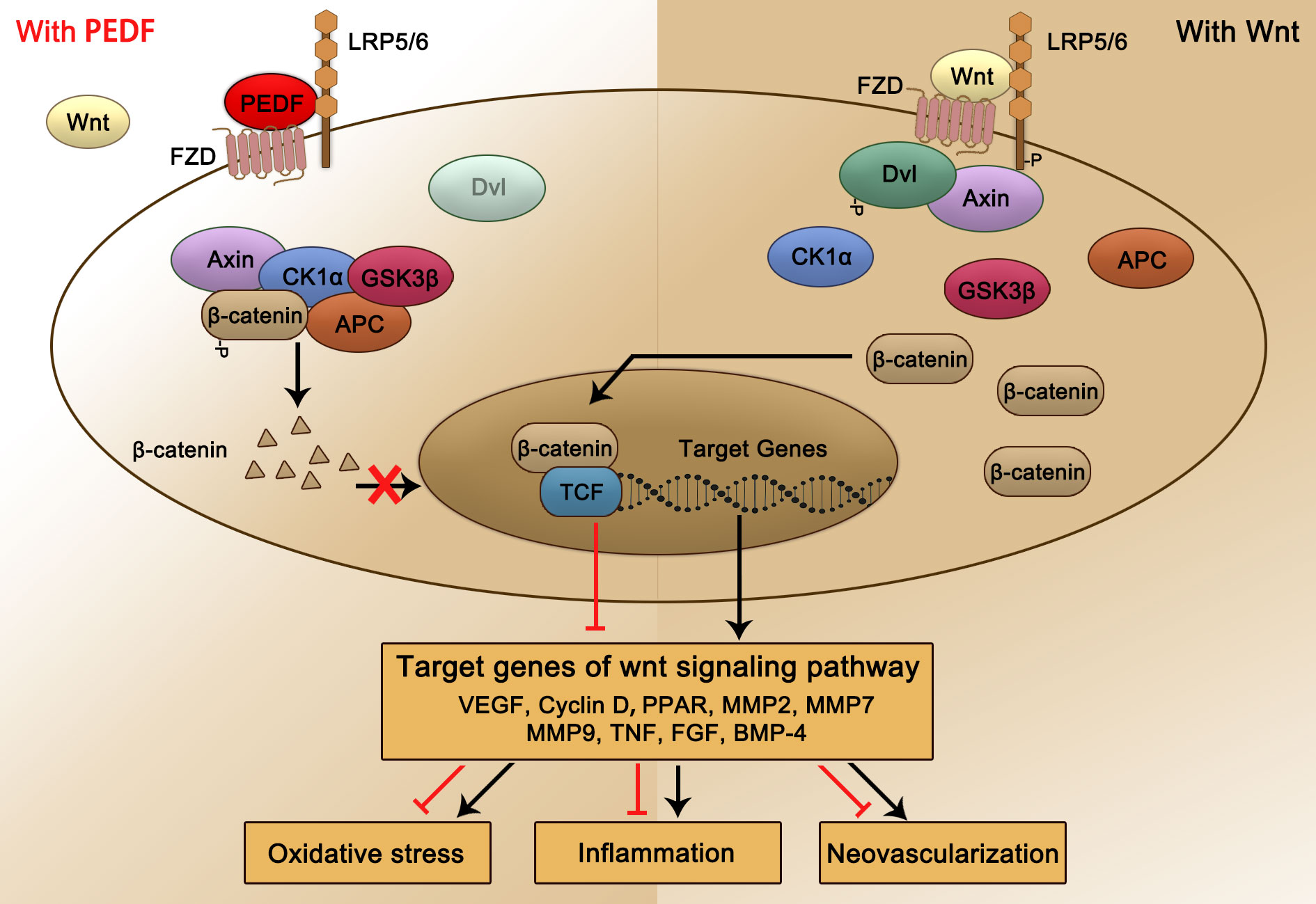
Figure 2 Schematic representation of PEDF-activated LRP6 signaling. PEDF binds to the Fzd-LRP5/6 complex and activates the canonical Wnt/β-catenin pathway. The key protein Dvl is recruited and phosphorylated, subsequently forming a destruction complex that also includes Axin, CKIa, GSK3β, and APC tumor suppressors and inhibiting the phosphorylation and nuclear translocation of β-catenin, which is bound. This process leads to changes in target gene transcription and expression, which reduce the occurrence of relevant pathological consequences, such as oxidative stress, inflammation, and NV. Dvl, dishevelled; CKIa, casein kinase Ia; GSK3β, glycogen synthase kinase 3β.
3.3 Plexin domain-containing 1 and plexin domain-containing 2
Plexin domain-containing 1 (PLXDC1), also known as tumor endothelial marker 7, is a member of the tumor vascular endothelial marker family (143). The genes encoding PLXDC1 are highly expressed during tumor angiogenesis and enriched in many types of tumor endothelial cells (144), including glioblastoma endothelium (57, 63), ovarian cancer (62), gastric cancer (60), renal cell carcinoma (61), colorectal cancer (64), lung cancer (58), breast cancer (56), and osteosarcoma (59). PLXDC1 has been found to be highly expressed in the endothelial cells in the ocular disease DR context and is highly specific to diseased blood vessels (145). PLXDC1 has also been shown to be expressed in rodent retinal ganglion cells (RGCs) (145). Transmission electron microscopy has revealed that PLXDC1 was mainly expressed at tight junctions between endothelial cells, and some PLXDC1 expression was detected on the surface of the blood vessel lumen (146).
PLXDC1 is a novel LRP1-regulated cell-signaling protein. Regulation of PLXDC1 activity by LRP1 was confirmed in CHO cells, and in extracts of RAW264.7 cells and mouse liver, PLXDC1 coimmunoprecipitated with LRP1 (147). The Nidogen/PLXDC1 interaction enhanced cell spreading in PLXDC1-transfected 293T cells, suggesting that Nidogen is a candidate ligand for PLXDC1 (148). Akash Nanda et al. identified cortactin as a protein candidate for binding to the extracellular region of both PLXDC1 and its closest homolog, which were all expressed in the tumor endothelium (146).
Plexin domain-containing 2 (PLXDC2) is a largely uncharacterized transmembrane protein with a homologous nidogen region and a plexin repeat in its extracellular domain. In mice, PLXDC2-betageo expression is prominent in the brain, including in the cortical hem, midbrain-hindbrain boundary, and midbrain floorplate, during midembryonic stages (E9.5-E11.5). On E15.5, PLXDC2 expression was apparent in a large number of discrete nuclei and structures throughout the brain, including the glial wedge and derivatives of the cortical hem. PLXDC2 was also found to be expressed in other tissues, most notably the limbs, lung buds and developing heart, as well as in the spinal cord and dorsal root ganglia (149). In humans, PLXDC2 has been found to be expressed in endothelial cells of the tumor stroma, neural progenitor cells, and pluripotent stem cells and in human hepatocellular carcinoma (HCC) tissues, including HCC cells, tumor vascular endothelial cells, and some infiltrating cells (150).
The PLXDC2 level is elevated during tumor angiogenesis (143). PLXDC2, a mitogen for neural progenitors, has been shown to be a novel regulator of the development of different brain regions involved in the coordinated control of cell proliferation and cell fate along and across the neuraxis (151). PLXDC2 expression increases during cellular senescence and is a marker of adult stem cells (152). In addition, PLXDC2 has been associated with CAD (72), ovarian cancer (73), diabetes mellitus (71), DR (68), testicular germ cell tumors (74), Sotos syndrome (66), ischemic stroke (70), laryngeal squamous cell carcinoma (69), breast cancer (56), and colorectal tumors (65, 67).
In 2014, Guo Cheng et al. identified PEDF binding to PLXDC1 and PLXDC2 on the surface of 293T cells (see Figure 3). PEDF interacted with PLXDC1 to promote the secretion of interleukin-10 by macrophages, which exerted neuroprotective effects on rat RGCs, and PEDF combined with PLXDC2 promoted apoptosis in endothelial cells (41). With few reports on the role or mechanism of PEDF and PLXDC in DR or neovascular fundus diseases, PLXDC1 has been shown to inhibit cell proliferation and invasion in tumor cells, promote endothelial cell separation in mice. With a protective effect on RGCs, PLXDC1 is likely to be an important receptor for PEDF, particularly in fundus diseases. In addition to PEDF, other molecules are ligands of PLXDC1 or PLXDC2. Moreover, members of the Wnt family (Wnt3a, Wnt5a and Wnt8b) show a striking overlap with PLXDC2 expression in certain areas (69). Cortactin can bind to the extracellular terminus of PLXDC2 (152). PLXDC2 has also been shown to be an activating ligand for ADGRD1 on cumulus cells (153).
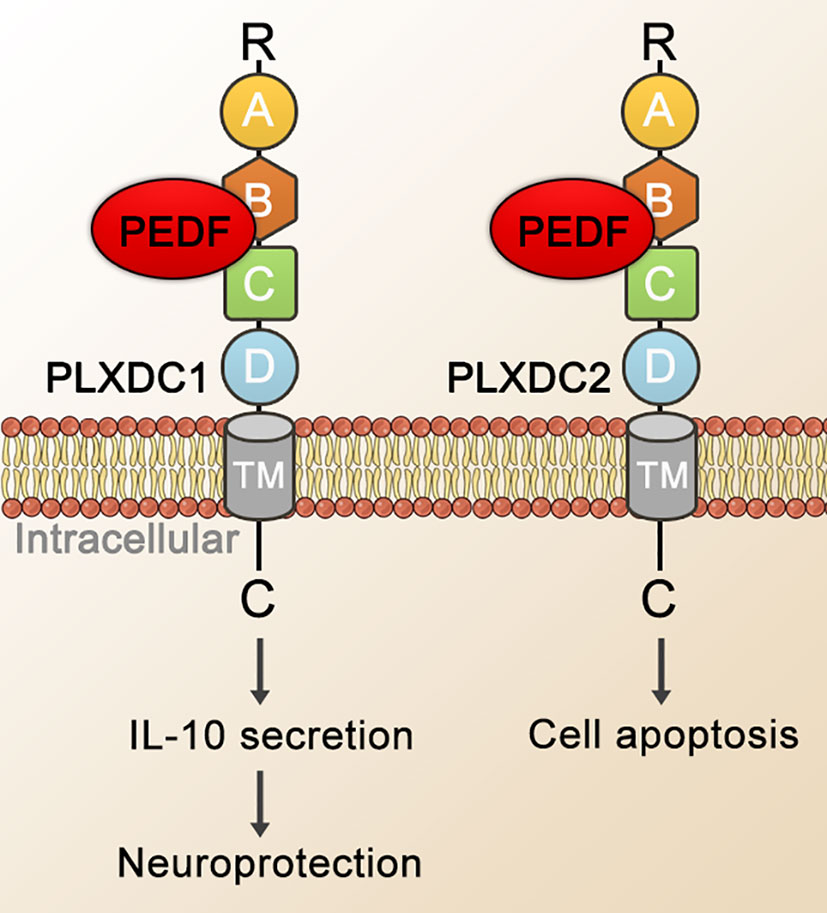
Figure 3 Schematic representation of PEDF-activated PLXDC1 or PLXDC2 signaling. PEDF binds to the cell-surface homologous membrane proteins PLXDC1 and PLXDC2 through their extracellular domains. Domain B plays an important role in binding PEDF. The TM and the C-terminus of these receptors mediate downstream signaling. Ultimately, this signal transduction mechanism stimulates the PLXDC1-dependent secretion of IL-10, which exerts neuroprotective and apoptosis-promoting effects in combination with PLXDC2. TM, transmembrane domain; IL-10, interleukin-10.
3.4 Laminin receptor
The laminin receptor (LR) is a laminin-binding protein with a molecular weight of approximately 67 kDa and is a nonintegrin cell surface receptor of the extracellular matrix (154). LR contributes to laminin binding, ribosome biosynthesis, cytoskeletal organization, and nuclear function, and it controls key cellular processes, including cell adhesion, migration, proliferation and survival, protein synthesis, development, and differentiation (76). Research on LR is mainly focused on its role on the development of tumors, specifically its promotion of tumor cell adhesion to the basement membrane, which is the first step in the tumor cell invasion and metastasis. Therefore, compared with normal cells, LR in tumor cells is overexpressed, and its overexpression is considered to be a molecular marker of cancer metastasis and aggressiveness, including breast cancer, lung cancer, ovarian cancer, prostate cancer, gastric cancer, thyroid cancer, leukemia and lymphoma (75, 76).
In vitro yeast two-hybrid screening experiments confirmed that LR is a cell membrane surface receptor of PEDF. Researchers have also determined that the PEDF isoform that interacts with LR consists of 34 peptides. Surface plasmon resonance analysis showed that PEDF interacts with LR, and PEDF also interacts with endothelial cells. LR-plasma membrane binding induces endothelial cell apoptosis and inhibits endothelial cell migration and the formation of vascular networks in vitro (25). The combination of the PEDF 34mer and LR may also mediate endothelial cell apoptosis through the LR-JNK-PPAR-γ-FasL-caspase 8 pathway, thereby exerting an anti-NV effect in the retina. In apoptotic cells, the PEDF 34mer binds to LR to phosphorylate JNK/MAPK, which then activates the transcription factor PPARγ through PPARγ ligand binding and nuclear translocation. In the nucleus, PPAR-γ and RXR combine in the form of heterodimers and recruit specific nuclear coactivators that activate the transcription of target FasL genes in a ligand-dependent manner. Both PPAR-γ and RXR promote the transcription mechanism initiated at FasL gene promoters, ultimately inducing the expression of FasL protein (155) (see Figure 4).
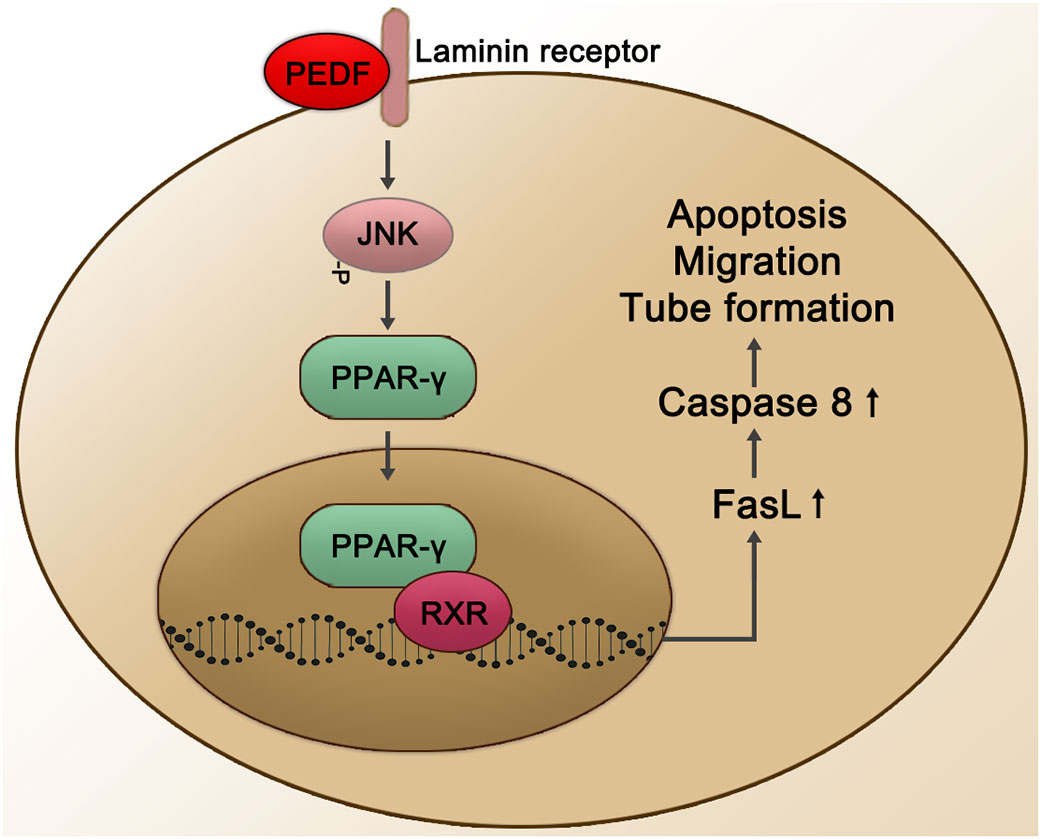
Figure 4 Schematic representation of the PEDF-activated laminin receptor. The PEDF 34mer binds to LR to activate the JNK-PPAR-γ-dependent signal transduction pathway. Subsequently, the combination of PPAR-γ and RXR is activated in the nucleus, promoting the transcription mechanism of FasL gene promoters and affecting biological processes through the upregulation of Caspase 8, such as cell apoptosis, migration, and tube formation. JNK, Jun N-terminal kinase; RXR, retinoid X receptor.
3.5 VEGFR2
Three VEGFR subtypes are encoded by different genes: VEGFR1 (Flt-1 in mice) and VEGFR2 (Flk-1; KDR) are structurally similar, while VEGFR3 (Flt-4) carries proteolytically cleaved extracellular domains (156–158). VEGF receptors are highly expressed in endothelial cells, hematopoietic cells, macrophages, and smooth muscle cells (159–161). VEGF-A isoforms drive physiological and pathophysiological angiogenesis via VEGFR1 and VEGFR2 signaling, while lymphatic angiogenesis is mediated by VEGF-C/D isoforms via VEGFR3 (162). VEGFR2 is a large membrane protein consisting of seven extracellular immunoglobulin (Ig)-like domains, a single transmembrane helix, and a split intracellular kinase domain. VEGF-A is an endogenous agonist of VEGFR2 that binds to ligand binding sites in Ig-like domain 2 (D2) and D3 on the basis of the stoichiometry of one VEGF-A dimer to one VEGFR dimer (163, 164).
VEGF-A, VEGFR1, and VEGFR2 play important roles in physiological and pathological angiogenesis, including tumor angiogenesis. Although VEGF-A isoforms exhibit similar VEGFR2-binding properties, activation of VEGFR2 is a complex multistep process. The VEGFR2 signaling pathway is critical for controlling vascular endothelial cell activity, migration, and permeability (165, 166). In cancer cells, transcription factors induced by hypoxia-inducible factors rapidly increase gene transcription rates, resulting in elevated levels of VEGF gene expression while enhancing the stability of VEGF mRNA (78, 79). The direct result of VEGF-VEGFR binding is endothelial cell budding, increased vascular permeability, and increased expression of tissue matrix metalloproteinases, resulting in extracellular matrix digestion. These processes lead to the movement of endothelial and pericyte cells, eventually leading to vasodilation and the formation of pathological vascular networks.
In 2006, Jianxing Ma demonstrated that PEDF inhibited VEGFR2 (167). PEDF inhibits VEGF expression at the transcriptional level by inhibiting hypoxia-induced increases in VEGF promoter activity, HIF-1 nuclear translocation, and mitogen-activated protein kinase phosphorylation. Other investigators have reported that PEDF exerts the anti-VEGF actions by binding to its key angiogenic receptor, VEGF-R2 (43). This binding inhibits receptor activation and ultimately reverses critical downstream VEGF-A signaling by reducing VEGF-R2 tyrosine kinase activity and inhibiting two phosphorylation sites in VEGF-induced HUVECs (Y951 is critical for cell migration and pathological angiogenesis, and Y1175 phosphorylates downstream signaling). Inhibition of VEGF angiogenesis signaling molecules such as PI3K, PLC-γ, FAK and Src may regulate many aspects of endothelial cell biology, including cell proliferation, migration, and survival. Behzad Shahbazi et al. also showed that PEDF binds to the VEGFR2, as well as laminin receptor much stronger than ATP synthase β-subunit, VEGFR1, and LRP6 using molecular docking, molecular dynamics (MD) simulation, and Molecular mechanics/Poisson-Boltzmann surface area (MM/PBSA) approach. Besides, the N-terminal of PEDF owns the most important enactment in the interaction with its receptors (168).
3.6 F1-ATP synthase
F-ATP is an ATP synthase in the bacterial plasma membrane, the eukaryotic mitochondrial inner membrane and chloroplast thylakoid membrane. F-ATPase leverages the membrane proton gradient to drive ATP synthesis, passing the passive proton flux through the membrane along its electrochemical gradient, and releasing newly formed ATP from the active site of F-ATP synthase using the energy released by transport reaction (169). F-ATP synthase is composed of two domains, F0-ATP synthase and F1-ATP synthase. In previous studies on angiogenesis, lipoprotein metabolism, innate immunity, hypertension or food intake regulation, F1-ATP synthase was found to be expressed only in cell mitochondria and was used to synthesize ATP that was needed by cells(77). Luigi Notari et al. demonstrated for the first time that PEDF binds F1 ATP synthase b subunit on the endothelial cell surface and inhibits endothelial extracellular ATP synthesis (170). In addition, F1-ATP synthase can inhibit cell growth, proliferation, and migration and directly induce multiple forms of cell death (171).
4 Therapeutic roles of PEDF and its receptors in ocular fundus diseases
Millions of people worldwide are affected by ocular fundus diseases such as DR, age-related macular degeneration (AMD), retinal vein occlusion (RVO), retinal artery occlusion (RAO), and fundus tumors. Inflammation, oxidative stress, neurodegeneration, and neovascularization (NV) are involved in the development of these diseases. As an endogenous multifunctional factor, defects or deficiencies that alter PEDF expression have been shown to be closely related to the progression of some of the abovementioned diseases. Related studies have shown that PEDF levels are markedly decreased in the vitreous, retinas and aqueous humors of diabetic patients with proliferative DR compared with those of either nondiabetic individuals or diabetic patients with nonproliferative DR (18, 24, 172). The high-glucose environment in diabetic patients directly downregulates the expression of PEDF in RPE cells, resulting in the loss of its inhibitory effect on angiogenesis. Other studies have shown that the early postnatal retinal vascularization occurs at a faster rate in PEDF-deficient mice, and NV has been observed in the OIR model (26, 38). Injection of exogenous PEDF into the vitreous can significantly inhibit this pathological change (173). These studies have shown that an increase or decrease in PEDF expression is likely important to the development of retinopathy of prematurity (ROP) lesions and abnormal vascular changes. Notably, Holekamp et al. and Park et al., studying neovascular age-related macular degeneration (nAMD) patients and an argon laser-induced choroidal neovascularization (CNV) model in BN rats confirmed that decreased PEDF expression levels were associated with oxidative damage to RPE cells and may lead to CNV formation, which is a key factor in AMD pathological changes (37, 174). Moreover, studies with proliferative vitreoretinopathy (PVR) patients have shown increased levels of PEDF in the vitreous (175), suggesting that PEDF may act through a positive regulatory feedback loop to counteract the angiogenic response and inhibit the activity of fibrotic factors. The dynamic changes in PEDF levels in the abovementioned case studies suggested its potential therapeutic role in related fundus diseases. Clarifying the mechanism and molecular pathway of signal transduction mediated or induced by the membrane surface receptor of PEDF will contribute to the in-depth understanding of the pathological mechanism of fundus lesions and provide new ideas for disease intervention and treatment.
There are extensive reports on the role of PEDF and ATGL in ophthalmology. As mentioned above, ATGL is expressed in a variety of cells in the eye. In the natural retina, ATGL is distributed in the RPE, the inner segments of photoreceptors, and inner nuclear and RGC layers (39). Western blots of ARPE-19 cells and R28 cells membrane fractions yield a single 83-kDa band representing an immunoreactive protein. Specific biotinylation of cell-surface proteins enables ATGL labeling in ARPE-19 cells, and kinetic experiments have shown that fluorescein-labeled human recombinant PEDF binds to cell-surface proteins with a structure similar to that of ATGL. Based on these findings, ATGL is considered to be a plasma membrane protein that interacts with PEDF in these retinal cells (39). ATGL shows phospholipase A2 (PLA2) activity, which is enhanced after binding to PEDF, resulting in the release of active nonesterified fatty acids (NEFAs) and lysophosphatidic acid (LPA), which can be a second messenger or interact with C protein-coupled receptors (107). PEDF increases the PLA activity of ATGL, which plays a potential role in regulating the survival-promoting effect of PEDF on R28 cells (39). Retinal NV is a factor in a variety of eye-blinding diseases, such as DR and ROP. PEDF possibly exerts its antiangiogenic effects by interacting with ATGL in endothelial cells, as indicated by PEDF upregulation of FasL expression by regulating NF-κB in a PNPLA2-dependent manner (102, 103). Ocular neurodegenerative diseases such as glaucoma, hypertensive and ischemic retinopathies are results of the death of RGCs. Müller cells endow neonatal RGCs with trophic properties, and Müller cell-derived PEDF is secreted to support the regeneration of damaged RGCs. Researchers have shown that PEDF secreted by Müller cells promoted RGC survival through the engagement of ATGL (104). R28 cells express neuronal genes and carry functional cell-surface ATGL. PEDF specifically binds to the L4 region in ATGL, which is between Ile193 and Leu249, and thus protects R28 cells from undergoing apoptosis. In other words, ATGL, especially its L4 ectodomain, is essential to the anti-apoptotic and survival effects of PEDF on retinal cells (105).
LRP6 is one of the most widely studied receptors of PEDF, and it plays an important role in the occurrence and progression of DR. Notably, compared with that in nondiabetic controls, the activation of the Fzd-LRP5/6 coreceptor complex in the retina of patients with nonproliferative DR was significantly increased. Studies have shown that in two different recently developed ocular vessel models: OIR mice, which develop ischemia-induced retinal NV, or Vldlr−/− mice, which develop subretinal NV, the Wnt signaling pathway was activated, and β-catenin was translocated into the nucleus and negatively correlated with the retinal PEDF level (40). Intravitreal injection of PEDF significantly inhibited Wnt signaling pathway activation in this mouse model retina by binding to LRP6 with high affinity and subsequently regulating the expression of downstream target genes, including VEGF, PPAR, MMP2, MMP9, TNF, FGF and BMP-4. As a result, the increase in the oxidative stress response, the increase in the inflammatory response level and the formation of retinal NV in the pathogenesis of DR were attenuated, demonstrating a therapeutic role in DR.
The function and mechanism of PEDF action in fundus diseases mediated through other receptors have not been fully studied. Recent studies, however, have shown that in animal models of retinal NV (an OIR model and a laser-induced CNV model), both the PEDF 34mer and PEDF polypeptide 336 (PEDF 336) exhibit high affinity for LR (176). In a CNV model, PEDF 34mer spot treatment significantly inhibited the formation of CNV through its interaction with LR. In the OIR model, PEDF 336 bound LR with high affinity and inhibited retinal NV (176). In choroidal and retinal endothelial cells, VEGF induced LR expression, which can be inhibited by PEDF 336 (177). In addition, although the roles and mechanisms of PEDF and PLXDC in the eye have rarely been reported, considering that PEDF-activated PLXDC1 protected rat RGCs and that the combination of PEDF and PLXDC2 induced apoptosis of endothelial cells (41), these two membrane receptors may be important to PEDF effects on fundus diseases. They are therefore worthy of further study by researchers. The correlation between VEGFR2 activation and NV has been widely recognized. Zhang et al. proposed that the competitive blockade of VEGF-VEGFR-2 binding by PEDF may be crucial for PEDF effects on VEGF-induced permeability and angiogenesis in retinal capillary endothelial cells (167). Besides, PEDF bound specifically to the extracellular domain of VEGF-R2 and prevented activation of an intrinsic tyrosine kinase, disrupting the flow of VEGF-A downstream signals in primate retinal vascular endothelial cells (43). Furthermore, F1-ATP synthase inhibited cell growth, proliferation, and migration by inhibiting extracellular ATP synthesis and directly induced death in several cell types (19). Therefore, the interaction between PEDF and F1-ATP synthase may reasonably explain for the inhibitory effect of PEDF on NV (42).
5 Conclusion
PEDF receptors are widely expressed in ocular tissues and exhibit important physiological and pathological significance. These characteristics implicate the importance of basic research and clinical application of PEDF in the diagnosis, pathogenesis and drug development of DR. DR is a preventable but incurable type of blindness. At present, intravitreal injection of anti-VEGF drugs is an effective treatment method to control retinal NV in patients with DR. Among the many types of anti-VEGF drugs, monoclonal antibody anti-VEGF drugs (such as bevacizumab and ranibizumab) target VEGFR2 and have been designed to antagonize the binding of VEGF and VEGFR2. However, these anti-VEGF treatments still present certain limitations. A number of clinical studies have shown that the effect of anti-VEGF treatment in the clinic was clearly inferior to that obtained in Phase III clinical trials. After intravitreal injection, vision initially improved compared with that at the baseline, but it continued to decrease, not maintaining the initial benefits. Moreover, not all patients responded to anti-VEGF treatment. Exploring new treatments for retinal NV, reducing the number of intravitreal injections by optimizing treatment methods, and administering sustained-release preparations/eye drops have always been the research focus of ophthalmologists and scientific researchers.
Since it was discovered in 1989, PEDF has shown great potential as a drug target for the treatment of DR due to its antiangiogenic, anti-inflammatory, neuroprotective, and neurotrophic effects. With increasing attention on PEDF and the gradual deepening of relevant exploration, an increasing number of researchers have focused on mechanistic research, drug development and clinical transformation of PEDF-specific peptides with biological effects and have clarified the high-affinity structure of PEDF and PEDF receptors. Domains and specific amino acid sequences are helpful to locate PEDF-derived peptides that can truly perform a certain biological function. The therapeutic effect of PEDF-derived peptides is clear and focused, and as a therapeutic drug, its administration method is more adaptable and efficient. For example, drug loaded exosomes or nanomaterials exert therapeutic effects of the PEDF polypeptide to a greater extent than other methods. Therefore, it is important to accelerate the clinical translation and application of PEDF peptides.
Regarding the study of PEDF receptors alone, the roles of LRP6, ATGL and LR in the pathogenesis of DR are relatively clear. For example, the expression levels of LRP6 and ATGL are clearly related to DR pathogenesis, the severity of the disease, and the VEGF expression level. Therefore, the PEDF receptor may be used as a predictive biomarker for the development and progression of DR, which can help in the diagnosis of the disease. In addition, PEDF receptors are expressed not only in eye tissues but also in various tissues of the body, such as heart, bone marrow, kidney and so on. The study of the mechanism of PEDF receptors in nonocular diseases can broaden the horizons of researchers and help their exploration into DR pathogenesis more comprehensively.
There are still certain limitations to the development of treatments that target PEDF receptors. PEDF binds to different receptors and may exert the same effect. For example, by binding to ATGL or LRP6, PEDF exerts anti-inflammatory effects. If ATGL is blocked, LRP6 may continue to compensate and have the inflammatory functions. In addition, most PEDF receptors are not specific receptors and blocking these receptors will affect the physiological functions of other ligands bound to them.
Besides, although PEDF has shown strong therapeutic potential in the ophthalmology field, only one Phase I clinical study has shown that an adenovirus carrying PEDF exerted a therapeutic effect in patients with advanced neovascular age-related macular degeneration. To mediate a biological effect, PEDF must first be ligated to its cell membrane surface receptor. Moreover, PEDF binds to a specific domain of the PEDF receptor or a regulatory site to mediate its biological function. Through the identification of these binding sites, the true value of receptor research in the development of corresponding DR treatments can be demonstrated.
Author contributions
MX designed this study. MX and XC collected the data and wrote the manuscript. ZY collected the details of tables, figures, generated the tables, figures, figure legends, and wrote the first draft of the manuscript. XL reviewed and revised the manuscript and was also involved with the manuscript development, proofreading and approved the final version of the manuscript. All authors contributed to the article and approved the submitted version.
Funding
This work is supported by National Natural Science Foundation of China (82171085), National Natural Science Foundation of China (81900891) and Tianjin Research Innovation Project for Postgraduate Students (2021YJSB271).
Acknowledgments
All authors would like to thank Professor Joyce Tombran-Tink for her guidance on the structure and logic of the manuscript.
Conflict of interest
The authors declare that the research was conducted in the absence of any commercial or financial relationships that could be construed as a potential conflict of interest.
Publisher’s note
All claims expressed in this article are solely those of the authors and do not necessarily represent those of their affiliated organizations, or those of the publisher, the editors and the reviewers. Any product that may be evaluated in this article, or claim that may be made by its manufacturer, is not guaranteed or endorsed by the publisher.
Glossary
References
1. Tombran-Tink J, Chader GG, Johnson LV. PEDF: a pigment epithelium-derived factor with potent neuronal differentiative activity. Exp Eye Res (1991) 53:411–4. doi: 10.1016/0014-4835(91)90248-D
2. Lucas A, Yaron JR, Zhang L, Ambadapadi S. Overview of serpins and their roles in biological systems. Methods In Mol Biol (Clifton N.J.) (2018) 1826:1–7. doi: 10.1007/978-1-4939-8645-3_1
3. Sanrattana W, Maas C, De Maat S. SERPINs-from trap to treatment. Front Med (Lausanne) (2019) 6:25. doi: 10.3389/fmed.2019.00025
4. Tombran-Tink J. The neuroprotective and angiogenesis inhibitory serpin, PEDF: new insights into phylogeny, function, and signaling. Front Biosci (2005) 10:2131–49. doi: 10.2741/1686
5. Steele FR, Chader GJ, Johnson LV, Tombran-Tink J. Pigment epithelium-derived factor: neurotrophic activity and identification as a member of the serine protease inhibitor gene family. Proc Natl Acad Sci USA (1993) 90:1526–30. doi: 10.1073/pnas.90.4.1526
6. Bilak MM, Corse AM, Bilak SR, Lehar M, Tombran-Tink J, Kuncl RW. Pigment epithelium-derived factor (PEDF) protects motor neurons from chronic glutamate-mediated neurodegeneration. J Neuropathol Exp Neurol (1999) 58:719–28. doi: 10.1097/00005072-199907000-00006
7. Cayouette M, Smith SB, Becerra SP, Gravel C. Pigment epithelium-derived factor delays the death of photoreceptors in mouse models of inherited retinal degenerations. Neurobiol Dis (1999) 6:523–32. doi: 10.1006/nbdi.1999.0263
8. Cao W, Tombran-Tink J, Elias R, Sezate S, Mrazek D, Mcginnis JF. In Vivo Protection of photoreceptors from light damage by pigment epithelium-derived factor. Invest Ophthalmol Vis Sci (2001) 42:1646–52. doi: 10.1007/978-1-4615-1355-1_14
9. Dawson DW, Volpert OV, Gillis P, Crawford SE, Xu H, Benedict W, et al. Pigment epithelium-derived factor: a potent inhibitor of angiogenesis. Science (1999) 285:245–8. doi: 10.1126/science.285.5425.245
10. Franco-Chuaire ML, Ramirez-Clavijo S, Chuaire-Noack L. Pigment epithelium-derived factor: clinical significance in estrogen-dependent tissues and its potential in cancer therapy. Iran J Basic Med Sci (2015) 18:837–55. doi: 10.1016/j.mce.2009.12.001
11. Alberdi E, Aymerich MS, Becerra SP. Binding of pigment epithelium-derived factor (PEDF) to retinoblastoma cells and cerebellar granule neurons. evidence for a PEDF receptor. J Biol Chem (1999) 274:31605–12. doi: 10.1074/jbc.274.44.31605
12. Broadhead ML, Dass CR, Choong PF. Cancer cell apoptotic pathways mediated by PEDF: prospects for therapy. Trends Mol Med (2009) 15:461–7. doi: 10.1016/j.molmed.2009.08.003
13. Karakousis PC, John SK, Behling KC, Surace EM, Smith JE, Hendrickson A, et al. Localization of pigment epithelium derived factor (PEDF) in developing and adult human ocular tissues. Mol Vis (2001) 7:154–63. doi: 10.1017/S0952523801184166
14. Niknejad H, Yazdanpanah G, Kakavand M. Extract of fetal membrane would inhibit thrombosis and hemolysis. Med Hypotheses (2015) 85:197–202. doi: 10.1016/j.mehy.2015.04.030
15. Behling KC, Surace EM, Bennett J. Pigment epithelium-derived factor expression in the developing mouse eye. Mol Vis (2002) 8:449–54. doi: 10.1016/S0960-9822(02)01286-1
16. Sawant S, Aparicio S, Tink AR, Lara N, Barnstable CJ, Tombran-Tink J. Regulation of factors controlling angiogenesis in liver development: a role for PEDF in the formation and maintenance of normal vasculature. Biochem Biophys Res Commun (2004) 325:408–13. doi: 10.1016/j.bbrc.2004.10.041
17. Rörby E, Billing M, Dahl M, Warsi S, Andradottir S, Miharada K, et al. The stem cell regulator PEDF is dispensable for maintenance and function of hematopoietic stem cells. Sci Rep (2017) 7:10134. doi: 10.1038/s41598-017-09452-2
18. Spranger J, Osterhoff M, Reimann M, Mohlig M, Ristow M, Francis MK, et al. Loss of the antiangiogenic pigment epithelium-derived factor in patients with angiogenic eye disease. Diabetes (2001) 50:2641–5. doi: 10.2337/diabetes.50.12.2641
19. Ogata N, Nishikawa M, Nishimura T, Mitsuma Y, Matsumura M. Inverse levels of pigment epithelium-derived factor and vascular endothelial growth factor in the vitreous of eyes with rhegmatogenous retinal detachment and proliferative vitreoretinopathy. Am J Ophthalmol (2002) 133:851–2. doi: 10.1016/S0002-9394(02)01406-X
20. Ogata N, Nishikawa M, Nishimura T, Mitsuma Y, Matsumura M. Unbalanced vitreous levels of pigment epithelium-derived factor and vascular endothelial growth factor in diabetic retinopathy. Am J Ophthalmol (2002) 134:348–53. doi: 10.1016/S0002-9394(02)01568-4
21. Guan M, Yam HF, Su B, Chan KP, Pang CP, Liu WW, et al. Loss of pigment epithelium derived factor expression in glioma progression. J Clin Pathol (2003) 56:277–82. doi: 10.1136/jcp.56.4.277
22. Boehm BO, Lang G, Feldmann B, Kurkhaus A, Rosinger S, Volpert O, et al. Proliferative diabetic retinopathy is associated with a low level of the natural ocular anti-angiogenic agent pigment epithelium-derived factor (PEDF) in aqueous humor. a pilot study. Horm Metab Res (2003) 35:382–6. doi: 10.1055/s-2003-41362
23. Boehm BO, Lang G, Volpert O, Jehle PM, Kurkhaus A, Rosinger S, et al. Low content of the natural ocular anti-angiogenic agent pigment epithelium-derived factor (PEDF) in aqueous humor predicts progression of diabetic retinopathy. Diabetologia (2003) 46:394–400. doi: 10.1007/s00125-003-1040-9
24. Yokoi M, Yamagishi S, Saito A, Yoshida Y, Matsui T, Saito W, et al. Positive association of pigment epithelium-derived factor with total antioxidant capacity in the vitreous fluid of patients with proliferative diabetic retinopathy. Br J Ophthalmol (2007) 91:885–7. doi: 10.1136/bjo.2006.110890
25. Bernard A, Gao-LI J, Franco CA, Bouceba T, Huet A, Li Z. Laminin receptor involvement in the anti-angiogenic activity of pigment epithelium-derived factor. J Biol Chem (2009) 284:10480–90. doi: 10.1074/jbc.M809259200
26. He X, Cheng R, Benyajati S, Ma JX. PEDF and its roles in physiological and pathological conditions: implication in diabetic and hypoxia-induced angiogenic diseases. Clin Sci (Lond) (2015) 128:805–23. doi: 10.1042/CS20130463
27. Garcia M, Fernandez-Garcia NI, Rivas V, Carretero M, Escamez MJ, Gonzalez-Martin A, et al. Inhibition of xenografted human melanoma growth and prevention of metastasis development by dual antiangiogenic/antitumor activities of pigment epithelium-derived factor. Cancer Res (2004) 64:5632–42. doi: 10.1158/0008-5472.CAN-04-0230
28. Yang H, Xu Z, Iuvone PM, Grossniklaus HE. Angiostatin decreases cell migration and vascular endothelium growth factor (VEGF) to pigment epithelium derived factor (PEDF) RNA ratio in vitro and in a murine ocular melanoma model. Mol Vis (2006) 12:511–7. doi: 10.1016/j.visres.2005.12.018
29. Uehara H, Miyamoto M, Kato K, Ebihara Y, Kaneko H, Hashimoto H, et al. Expression of pigment epithelium-derived factor decreases liver metastasis and correlates with favorable prognosis for patients with ductal pancreatic adenocarcinoma. Cancer Res (2004) 64:3533–7. doi: 10.1158/0008-5472.CAN-03-3725
30. Guan M, Jiang H, Xu C, Xu R, Chen Z, Lu Y. Adenovirus-mediated PEDF expression inhibits prostate cancer cell growth and results in augmented expression of PAI-2. Cancer Biol Ther (2007) 6:419–25. doi: 10.4161/cbt.6.3.3757
31. Cheung LW, Au SC, Cheung AN, Ngan HY, Tombran-Tink J, Auersperg N, et al. Pigment epithelium-derived factor is estrogen sensitive and inhibits the growth of human ovarian cancer and ovarian surface epithelial cells. Endocrinology (2006) 147:4179–91. doi: 10.1210/en.2006-0168
32. Yuan Y, Liu X, Miao H, Huang B, Liu Z, Chen J, et al. PEDF increases GLUT4-mediated glucose uptake in rat ischemic myocardium via PI3K/AKT pathway in a PEDFR-dependent manner. Int J Cardiol (2019) 283:136–43. doi: 10.1016/j.ijcard.2019.02.035
33. Zhang H, Sun T, Jiang X, Yu H, Wang M, Wei T, et al. PEDF and PEDF-derived peptide 44mer stimulate cardiac triglyceride degradation via ATGL. J Transl Med (2015) 13:68. doi: 10.1186/s12967-015-0432-1
34. Cosgrove GP, Brown KK, Schiemann WP, Serls AE, Parr JE, Geraci MW, et al. Pigment epithelium-derived factor in idiopathic pulmonary fibrosis: a role in aberrant angiogenesis. Am J Respir Crit Care Med (2004) 170:242–51. doi: 10.1164/rccm.200308-1151OC
35. Shiga Y, Miura S, Mitsutake R, Yamagishi S, Saku K. Significance of plasma levels of pigment epithelium-derived factor as determined by multidetector row computed tomography in patients with mild chronic kidney disease and/or coronary artery disease. J Int Med Res (2011) 39:880–90. doi: 10.1177/147323001103900322
36. Wang JJ, Zhang SX, Lu K, Chen Y, Mott R, Sato S, et al. Decreased expression of pigment epithelium-derived factor is involved in the pathogenesis of diabetic nephropathy. Diabetes (2005) 54:243–50. doi: 10.2337/diabetes.54.1.243
37. Park K, Jin J, Hu Y, Zhou K, Ma JX. Overexpression of pigment epithelium-derived factor inhibits retinal inflammation and neovascularization. Am J Pathol (2011) 178:688–98. doi: 10.1016/j.ajpath.2010.10.014
38. Huang Q, Wang S, Sorenson CM, Sheibani N. PEDF-deficient mice exhibit an enhanced rate of retinal vascular expansion and are more sensitive to hyperoxia-mediated vessel obliteration. Exp Eye Res (2008) 87:226–41. doi: 10.1016/j.exer.2008.06.003
39. Notari L, Baladron V, Aroca-Aguilar JD, Balko N, Heredia R, Meyer C, et al. Identification of a lipase-linked cell membrane receptor for pigment epithelium-derived factor. J Biol Chem (2006) 281:38022–37. doi: 10.1074/jbc.M600353200
40. Park K, Lee K, Zhang B, Zhou T, He X, Gao G, et al. Identification of a novel inhibitor of the canonical wnt pathway. Mol Cell Biol (2011) 31:3038–51. doi: 10.1128/MCB.01211-10
41. Cheng G, Zhong M, Kawaguchi R, Kassai M, Al-Ubaidi M, Deng J, et al. Identification of PLXDC1 and PLXDC2 as the transmembrane receptors for the multifunctional factor PEDF. Elife (2014) 3:e05401. doi: 10.7554/eLife.05401
42. Deshpande M, Notari L, Subramanian P, Notario V, Becerra SP. Inhibition of tumor cell surface ATP synthesis by pigment epithelium-derived factor: implications for antitumor activity. Int J Oncol (2012) 41:219–27. doi: 10.3892/ijo.2012.1431
43. Zhang M, Tombran-Tink J, Yang S, Zhang X, Li X, Barnstable CJ. PEDF is an endogenous inhibitor of VEGF-R2 angiogenesis signaling in endothelial cells. Exp Eye Res (2021) 213:108828. doi: 10.1016/j.exer.2021.108828
44. Schweiger M, Schoiswohl G, Lass A, Radner FP, Haemmerle G, Malli R, et al. The c-terminal region of human adipose triglyceride lipase affects enzyme activity and lipid droplet binding. J Biol Chem (2008) 283:17211–20. doi: 10.1074/jbc.M710566200
45. Soni KG, Mardones GA, Sougrat R, Smirnova E, Jackson CL, Bonifacino JS. Coatomer-dependent protein delivery to lipid droplets. J Cell Sci (2009) 122:1834–41. doi: 10.1242/jcs.045849
46. Ellong EN, Soni KG, Bui QT, Sougrat R, Golinelli-Cohen MP, Jackson CL. Interaction between the triglyceride lipase ATGL and the Arf1 activator GBF1. PloS One (2011) 6:e21889. doi: 10.1371/journal.pone.0021889
47. Martinez-Lopez N, Garcia-Macia M, Sahu S, Athonvarangkul D, Liebling E, Merlo P, et al. Autophagy in the CNS and periphery coordinate lipophagy and lipolysis in the brown adipose tissue and liver. Cell Metab (2016) 23:113–27. doi: 10.1016/j.cmet.2015.10.008
48. Yang Y, Niswander L. Interaction between the signaling molecules WNT7a and SHH during vertebrate limb development: dorsal signals regulate anteroposterior patterning. Cell (1995) 80:939–47. doi: 10.1016/0092-8674(95)90297-X
49. De Ferrari GV, Papassotiropoulos A, Biechele T, Wavrant De-Vrieze ,F, Avila ME, Major MB, et al. Common genetic variation within the low-density lipoprotein receptor-related protein 6 and late-onset alzheimer's disease. Proc Natl Acad Sci U.S.A. (2007) 104:9434–9. doi: 10.1073/pnas.0603523104
50. Sarzani R, Salvi F, Bordicchia M, Guerra F, Battistoni I, Pagliariccio G, et al. Carotid artery atherosclerosis in hypertensive patients with a functional LDL receptor-related protein 6 gene variant. Nutr Metab Cardiovasc Dis (2011) 21:150–6. doi: 10.1016/j.numecd.2009.08.004
51. Go GW. Low-density lipoprotein receptor-related protein 6 (LRP6) is a novel nutritional therapeutic target for hyperlipidemia, non-alcoholic fatty liver disease, and atherosclerosis. Nutrients (2015) 7:4453–64. doi: 10.3390/nu7064453
52. Mani A, Radhakrishnan J, Wang H, Mani A, Mani MA, Nelson-Williams C, et al. LRP6 mutation in a family with early coronary disease and metabolic risk factors. Science (2007) 315:1278–82. doi: 10.1126/science.1136370
53. Huertas-Vazquez A, Plaisier C, Weissglas-Volkov D, Sinsheimer J, Canizales-Quinteros S, Cruz-Bautista I, et al. TCF7L2 is associated with high serum triacylglycerol and differentially expressed in adipose tissue in families with familial combined hyperlipidaemia. Diabetologia (2008) 51:62–9. doi: 10.1007/s00125-007-0850-6
54. Piao S, Lee SH, Kim H, Yum S, Stamos JL, Xu Y, et al. Direct inhibition of GSK3beta by the phosphorylated cytoplasmic domain of LRP6 in wnt/beta-catenin signaling. PloS One (2008) 3:e4046. doi: 10.1371/journal.pone.0004046
55. Liu PH, Chang YC, Jiang YD, Chen WJ, Chang TJ, Kuo SS, et al. Genetic variants of TCF7L2 are associated with insulin resistance and related metabolic phenotypes in Taiwanese adolescents and Caucasian young adults. J Clin Endocrinol Metab (2009) 94:3575–82. doi: 10.1210/jc.2009-0609
56. Davies G, Cunnick GH, Mansel RE, Mason MD, Jiang WG. Levels of expression of endothelial markers specific to tumour-associated endothelial cells and their correlation with prognosis in patients with breast cancer. Clin Exp Metastasis (2004) 21:31–7. doi: 10.1023/B:CLIN.0000017168.83616.d0
57. Beaty RM, Edwards JB, Boon K, Siu IM, Conway JE, Riggins GJ. PLXDC1 (TEM7) is identified in a genome-wide expression screen of glioblastoma endothelium. J Neurooncol (2007) 81:241–8. doi: 10.1007/s11060-006-9227-9
58. Pierce A, Barron N, Linehan R, Ryan E, O'driscoll L, Daly C, et al. Identification of a novel, functional role for S100A13 in invasive lung cancer cell lines. Eur J Cancer (2008) 44:151–9. doi: 10.1016/j.ejca.2007.10.017
59. Halder C, Ossendorf C, Maran A, Yaszemski M, Bolander ME, Fuchs B, et al. Preferential expression of the secreted and membrane forms of tumor endothelial marker 7 transcripts in osteosarcoma. Anticancer Res (2009) 29:4317–22. doi: 10.1007/978-3-642-72707-8_4
60. Zhang ZZ, Hua R, Zhang JF, Zhao WY, Zhao EH, Tu L, et al. TEM7 (PLXDC1), a key prognostic predictor for resectable gastric cancer, promotes cancer cell migration and invasion. Am J Cancer Res (2015) 5:772–81.
61. Li XH, Yang CZ, Wang J. Network spatio-temporal analysis predicts disease stage-related genes and pathways in renal cell carcinoma. Genet Mol Res (2016) 15. doi: 10.4238/gmr.15028061
62. Kim GH, Won JE, Byeon Y, Kim MG, Wi TI, Lee JM, et al. Selective delivery of PLXDC1 small interfering RNA to endothelial cells for anti-angiogenesis tumor therapy using CD44-targeted chitosan nanoparticles for epithelial ovarian cancer. Drug Delivery (2018) 25:1394–402. doi: 10.1080/10717544.2018.1480672
63. Falchetti ML, D'alessandris QG, Pacioni S, Buccarelli M, Morgante L, Giannetti S, et al. Glioblastoma endothelium drives bevacizumab-induced infiltrative growth via modulation of PLXDC1. Int J Cancer (2019) 144:1331–44. doi: 10.1002/ijc.31983
64. Pietrzyk L, Wdowiak P. Serum TEM5 and TEM7 concentrations correlate with clinicopathologic features and poor prognosis of colorectal cancer patients. Adv Med Sci (2019) 64:402–8. doi: 10.1016/j.advms.2019.07.001
65. Rmali KA, Puntis MC, Jiang WG. Prognostic values of tumor endothelial markers in patients with colorectal cancer. World J Gastroenterol (2005) 11:1283–6. doi: 10.3748/wjg.v11.i9.1283
66. Visser R, Gijsbers A, Ruivenkamp C, Karperien M, Reeser HM, Breuning MH, et al. Genome-wide SNP array analysis in patients with features of sotos syndrome. Horm Res Paediatr (2010) 73:265–74. doi: 10.1159/000284391
67. Greening DW, Kapp EA, Ji H, Speed TP, Simpson RJ. Colon tumour secretopeptidome: insights into endogenous proteolytic cleavage events in the colon tumour microenvironment. Biochim Biophys Acta (2013) 1834:2396–407. doi: 10.1016/j.bbapap.2013.05.006
68. Hosseini SM, Boright AP, Sun L, Canty AJ, Bull SB, Klein BE, et al. The association of previously reported polymorphisms for microvascular complications in a meta-analysis of diabetic retinopathy. Hum Genet (2015) 134:247–57. doi: 10.1007/s00439-014-1517-2
69. Kim JS, Kim SY, Lee M, Kim SH, Kim SM, Kim EJ. Radioresistance in a human laryngeal squamous cell carcinoma cell line is associated with DNA methylation changes and topoisomerase II alpha. Cancer Biol Ther (2015) 16:558–66. doi: 10.1080/15384047.2015.1017154
70. O'connell GC, Petrone AB, Treadway MB, Tennant CS, Lucke-Wold N, Chantler PD, et al. Machine-learning approach identifies a pattern of gene expression in peripheral blood that can accurately detect ischaemic stroke. NPJ Genom Med (2016) 1:16038. doi: 10.1038/npjgenmed.2016.38
71. Li J, Wei J, Xu P, Yan M, Li J, Chen Z, et al. Impact of diabetes-related gene polymorphisms on the clinical characteristics of type 2 diabetes Chinese han population. Oncotarget (2016) 7:85464–71. doi: 10.18632/oncotarget.13399
72. Azzam SK, Osman WM, Lee S, Khalaf K, Khandoker AH, Almahmeed W, et al. Genetic associations with diabetic retinopathy and coronary artery disease in emirati patients with type-2 diabetes mellitus. Front Endocrinol (Lausanne) (2019) 10:283. doi: 10.3389/fendo.2019.00283
73. Bates M, Spillane CD, Gallagher MF, Mccann A, Martin C, Blackshields G, et al. The role of the MAD2-TLR4-MyD88 axis in paclitaxel resistance in ovarian cancer. PloS One (2020) 15:e0243715. doi: 10.1371/journal.pone.0243715
74. Qin G, Mallik S, Mitra R, Li A, Jia P, Eischen CM, et al. MicroRNA and transcription factor co-regulatory networks and subtype classification of seminoma and non-seminoma in testicular germ cell tumors. Sci Rep (2020) 10:852. doi: 10.1038/s41598-020-57834-w
75. Pesapane A, Di Giovanni C, Rossi FW, Alfano D, Formisano L, Ragno P, et al. Discovery of new small molecules inhibiting 67 kDa laminin receptor interaction with laminin and cancer cell invasion. Oncotarget (2015) 6:18116–33. doi: 10.18632/oncotarget.4016
76. Pesapane A, Ragno P, Selleri C, Montuori N. Recent advances in the function of the 67 kDa laminin receptor and its targeting for personalized therapy in cancer. Curr Pharm Des (2017) 23:4745–57. doi: 10.2174/1381612823666170710125332
77. Champagne E, Martinez LO, Collet X, Barbaras R. Ecto-F1Fo ATP synthase/F1 ATPase: metabolic and immunological functions. Curr Opin Lipidol (2006) 17:279–84. doi: 10.1097/01.mol.0000226120.27931.76
78. Ikeda E, Achen MG, Breier G, Risau W. Hypoxia-induced transcriptional activation and increased mRNA stability of vascular endothelial growth factor in C6 glioma cells. J Biol Chem (1995) 270:19761–6. doi: 10.1074/jbc.270.34.19761
79. Forsythe JA, Jiang BH, Iyer NV, Agani F, Leung SW, Koos RD, et al. Activation of vascular endothelial growth factor gene transcription by hypoxia-inducible factor 1. Mol Cell Biol (1996) 16:4604–13. doi: 10.1128/MCB.16.9.4604
80. Schreiber R, Xie H, Schweiger M. Of mice and men: the physiological role of adipose triglyceride lipase (ATGL). Biochim Biophys Acta Mol Cell Biol Lipids (2019) 1864:880–99. doi: 10.1016/j.bbalip.2018.10.008
81. Zimmermann R, Strauss JG, Haemmerle G, Schoiswohl G, Birner-Gruenberger R, Riederer M, et al. Fat mobilization in adipose tissue is promoted by adipose triglyceride lipase. Science (2004) 306:1383–6. doi: 10.1126/science.1100747
82. Jenkins CM, Mancuso DJ, Yan W, Sims HF, Gibson B, Gross RW. Identification, cloning, expression, and purification of three novel human calcium-independent phospholipase A2 family members possessing triacylglycerol lipase and acylglycerol transacylase activities. J Biol Chem (2004) 279:48968–75. doi: 10.1074/jbc.M407841200
83. Villena JA, Roy S, Sarkadi-Nagy E, Kim KH, Sul HS. Desnutrin, an adipocyte gene encoding a novel patatin domain-containing protein, is induced by fasting and glucocorticoids: ectopic expression of desnutrin increases triglyceride hydrolysis. J Biol Chem (2004) 279:47066–75. doi: 10.1074/jbc.M403855200
84. Jocken JW, Smit E, Goossens GH, Essers YP, Van Baak MA, Mensink M, et al. Adipose triglyceride lipase (ATGL) expression in human skeletal muscle istype I (oxidative) fiber specific. Histochem Cell Biol (2008) 129:535–8. doi: 10.1007/s00418-008-0386-y
85. Kienesberger PC, Pulinilkunnil T, Sung MM, Nagendran J, Haemmerle G, Kershaw EE, et al. Myocardial ATGL overexpression decreases the reliance on fatty acid oxidation and protects against pressure overload-induced cardiac dysfunction. Mol Cell Biol (2012) 32:740–50. doi: 10.1128/MCB.06470-11
86. Ong KT, Mashek MT, Bu SY, Greenberg AS, Mashek DG. Adipose triglyceride lipase is a major hepatic lipase that regulates triacylglycerol turnover and fatty acid signaling and partitioning. Hepatology (2011) 53:116–26. doi: 10.1002/hep.24006
87. Al-Zoughbi W, Pichler M, Gorkiewicz G, Guertl-Lackner B, Haybaeck J, Jahn SW, et al. Loss of adipose triglyceride lipase is associated with human cancer and induces mouse pulmonary neoplasia. Oncotarget (2016) 7:33832–40. doi: 10.18632/oncotarget.9418
88. Desjardin JT, Becerra SP, Subramanian P. Searching for alternatively spliced variants of phospholipase domain-containing 2 (Pnpla2), a novel gene in the retina. J Clin Exp Ophthalmol (2013) 4:295. doi: 10.4172/2155-9570.1000295
89. Subramanian P, Notario PM, Becerra SP. Pigment epithelium-derived factor receptor (PEDF-r): a plasma membrane-linked phospholipase with PEDF binding affinity. Adv Exp Med Biol (2010) 664:29–37. doi: 10.1007/978-1-4419-1399-9_4
90. Rydel TJ, Williams JM, Krieger E, Moshiri F, Stallings WC, Brown SM, et al. The crystal structure, mutagenesis, and activity studies reveal that patatin is a lipid acyl hydrolase with a ser-asp catalytic dyad. Biochemistry (2003) 42:6696–708. doi: 10.1021/bi027156r
91. Yamaguchi T, Omatsu N, Morimoto E, Nakashima H, Ueno K, Tanaka T, et al. CGI-58 facilitates lipolysis on lipid droplets but is not involved in the vesiculation of lipid droplets caused by hormonal stimulation. J Lipid Res (2007) 48:1078–89. doi: 10.1194/jlr.M600493-JLR200
92. Eichmann TO, Kumari M, Haas JT, Farese RV, Jr., Zimmermann, R., Lass A, et al. Studies on the substrate and stereo/regioselectivity of adipose triglyceride lipase, hormone-sensitive lipase, and diacylglycerol-o-acyltransferases. J Biol Chem (2012) 287:41446–57. doi: 10.1074/jbc.M112.400416
93. Hofer P, Boeszoermenyi A, Jaeger D, Feiler U, Arthanari H, Mayer N, et al. Fatty acid-binding proteins interact with comparative gene identification-58 linking lipolysis with lipid ligand shuttling. J Biol Chem (2015) 290:18438–53. doi: 10.1074/jbc.M114.628958
94. Olzmann JA, Richter CM, Kopito RR. Spatial regulation of UBXD8 and p97/VCP controls ATGL-mediated lipid droplet turnover. Proc Natl Acad Sci U.S.A. (2013) 110:1345–50. doi: 10.1073/pnas.1213738110
95. Cornaciu I, Boeszoermenyi A, Lindermuth H, Nagy HM, Cerk IK, Ebner C, et al. The minimal domain of adipose triglyceride lipase (ATGL) ranges until leucine 254 and can be activated and inhibited by CGI-58 and G0S2, respectively. PloS One (2011) 6:e26349. doi: 10.1371/journal.pone.0026349
96. Yang X, Lu X, Lombes M, Rha GB, Chi YI, Guerin TM, et al. The G(0)/G(1) switch gene 2 regulates adipose lipolysis through association with adipose triglyceride lipase. Cell Metab (2010) 11:194–205. doi: 10.1016/j.cmet.2010.02.003
97. Zhang X, Saarinen AM, Hitosugi T, Wang Z, Wang L, Ho TH, et al. Inhibition of intracellular lipolysis promotes human cancer cell adaptation to hypoxia. Elife (2017) 6. doi: 10.7554/eLife.31132
98. Padmanabha Das KM, Wechselberger L, Liziczai M, De La Rosa Rodriguez M, Grabner GF, Heier C, et al. Hypoxia-inducible lipid droplet-associated protein inhibits adipose triglyceride lipase. J Lipid Res (2018) 59:531–41. doi: 10.1194/jlr.M082388
99. Niyogi S, Ghosh M, Adak M, Chakrabarti P. PEDF promotes nuclear degradation of ATGL through COP1. Biochem Biophys Res Commun (2019) 512:806–11. doi: 10.1016/j.bbrc.2019.03.111
100. Yang Z, Sun J, Ji H, Shi XC, Li Y, Du ZY, et al. Pigment epithelium-derived factor improves TNFalpha-induced hepatic steatosis in grass carp (Ctenopharyngodon idella). Dev Comp Immunol (2017) 71:8–17. doi: 10.1016/j.dci.2017.01.016
101. He T, Hu J, Yan G, Li L, Zhang D, Zhang Q, et al. Pigment epithelium-derived factor regulates microvascular permeability through adipose triglyceride lipase in sepsis. Clin Sci (Lond) (2015) 129:49–61. doi: 10.1042/CS20140631
102. Schweiger M, Paar M, Eder C, Brandis J, Moser E, Gorkiewicz G, et al. G0/G1 switch gene-2 regulates human adipocyte lipolysis by affecting activity and localization of adipose triglyceride lipase. J Lipid Res (2012) 53:2307–17. doi: 10.1194/jlr.M027409
103. Albert JS, Yerges-Armstrong LM, Horenstein RB, Pollin TI, Sreenivasan UT, Chai S, et al. Null mutation in hormone-sensitive lipase gene and risk of type 2 diabetes. N Engl J Med (2014) 370:2307–15. doi: 10.1056/NEJMoa1315496
104. Kim JY, Tillison K, Lee JH, Rearick DA, Smas CM. The adipose tissue triglyceride lipase ATGL/PNPLA2 is downregulated by insulin and TNF-alpha in 3T3-L1 adipocytes and is a target for transactivation by PPARgamma. Am J Physiol Endocrinol Metab (2006) 291:E115–27. doi: 10.1152/ajpendo.00317.2005
105. Chakrabarti P, English T, Karki S, Qiang L, Tao R, Kim J, et al. SIRT1 controls lipolysis in adipocytes via FOXO1-mediated expression of ATGL. J Lipid Res (2011) 52:1693–701. doi: 10.1194/jlr.M014647
106. Chakrabarti P, Kandror KV. FoxO1 controls insulin-dependent adipose triglyceride lipase (ATGL) expression and lipolysis in adipocytes. J Biol Chem (2009) 284:13296–300. doi: 10.1074/jbc.C800241200
107. Pagnon J, Matzaris M, Stark R, Meex RC, Macaulay SL, Brown W, et al. Identification and functional characterization of protein kinase a phosphorylation sites in the major lipolytic protein, adipose triglyceride lipase. Endocrinology (2012) 153:4278–89. doi: 10.1210/en.2012-1127
108. Kim SJ, Tang T, Abbott M, Viscarra JA, Wang Y, Sul HS. AMPK phosphorylates Desnutrin/ATGL and hormone-sensitive lipase to regulate lipolysis and fatty acid oxidation within adipose tissue. Mol Cell Biol (2016) 36:1961–76. doi: 10.1128/MCB.00244-16
109. Grahn THM, Kaur R, Yin J, Schweiger M, Sharma VM, Lee MJ, et al. Fat-specific protein 27 (FSP27) interacts with adipose triglyceride lipase (ATGL) to regulate lipolysis and insulin sensitivity in human adipocytes. J Biol Chem (2014) 289:12029–39. doi: 10.1074/jbc.M113.539890
110. Singh M, Kaur R, Lee MJ, Pickering RT, Sharma VM, Puri V, et al. Fat-specific protein 27 inhibits lipolysis by facilitating the inhibitory effect of transcription factor Egr1 on transcription of adipose triglyceride lipase. J Biol Chem (2014) 289:14481–7. doi: 10.1074/jbc.C114.563080
111. Brown SD, Twells RC, Hey PJ, Cox RD, Levy ER, Soderman AR, et al. Isolation and characterization of LRP6, a novel member of the low density lipoprotein receptor gene family. Biochem Biophys Res Commun (1998) 248:879–88. doi: 10.1006/bbrc.1998.9061
112. Dieckmann M, Dietrich MF, Herz J. Lipoprotein receptors–an evolutionarily ancient multifunctional receptor family. Biol Chem (2010) 391:1341–63. doi: 10.1515/bc.2010.129
113. Hey PJ, Twells RC, Phillips MS, Yusuke N, Brown SD, Kawaguchi Y, et al. Cloning of a novel member of the low-density lipoprotein receptor family. Gene (1998) 216:103–11. doi: 10.1016/S0378-1119(98)00311-4
114. Kelly OG, Pinson KI, Skarnes WC. The wnt co-receptors Lrp5 and Lrp6 are essential for gastrulation in mice. Development (2004) 131:2803–15. doi: 10.1242/dev.01137
115. Wang ZM, Luo JQ, Xu LY, Zhou HH, Zhang W. Harnessing low-density lipoprotein receptor protein 6 (LRP6) genetic variation and wnt signaling for innovative diagnostics in complex diseases. Pharmacogenomics J (2018) 18:351–8. doi: 10.1038/tpj.2017.28
116. Macdonald BT, Tamai K, He X. Wnt/beta-catenin signaling: components, mechanisms, and diseases. Dev Cell (2009) 17:9–26. doi: 10.1016/j.devcel.2009.06.016
117. Zhong Z, Baker JJ, Zylstra-Diegel CR, Williams BO. Lrp5 and Lrp6 play compensatory roles in mouse intestinal development. J Cell Biochem (2012) 113:31–8. doi: 10.1002/jcb.23324
118. Joiner DM, Ke J, Zhong Z, Xu HE, Williams BO. LRP5 and LRP6 in development and disease. Trends Endocrinol Metab (2013) 24:31–9. doi: 10.1016/j.tem.2012.10.003
119. Cheng Z, Biechele T, Wei Z, Morrone S, Moon RT, Wang L, et al. Crystal structures of the extracellular domain of LRP6 and its complex with DKK1. Nat Struct Mol Biol (2011) 18:1204–10. doi: 10.1038/nsmb.2139
120. Pinson KI, Brennan J, Monkley S, Avery BJ, Skarnes WC. An LDL-receptor-related protein mediates wnt signalling in mice. Nature (2000) 407:535–8. doi: 10.1038/35035124
121. Liu CC, Tsai CW, Deak F, Rogers J, Penuliar M, Sung YM, et al. Deficiency in LRP6-mediated wnt signaling contributes to synaptic abnormalities and amyloid pathology in alzheimer's disease. Neuron (2014) 84:63–77. doi: 10.1016/j.neuron.2014.08.048
122. Davidson G, Wu W, Shen J, Bilic J, Fenger U, Stannek P, et al. Casein kinase 1 gamma couples wnt receptor activation to cytoplasmic signal transduction. Nature (2005) 438:867–72. doi: 10.1038/nature04170
123. Niehrs C, Shen J. Regulation of Lrp6 phosphorylation. Cell Mol Life Sci (2010) 67:2551–62. doi: 10.1007/s00018-010-0329-3
124. Davidson G, Shen J, Huang YL, Su Y, Karaulanov E, Bartscherer K, et al. Cell cycle control of wnt receptor activation. Dev Cell (2009) 17:788–99. doi: 10.1016/j.devcel.2009.11.006
125. Niehrs C, Acebron SP. Mitotic and mitogenic wnt signalling. EMBO J (2012) 31:2705–13. doi: 10.1038/emboj.2012.124
126. Huang YL, Anvarian Z, Doderlein G, Acebron SP, Niehrs C. Maternal Wnt/STOP signaling promotes cell division during early xenopus embryogenesis. Proc Natl Acad Sci USA (2015) 112:5732–7. doi: 10.1073/pnas.1423533112
127. Koch S, Acebron SP, Herbst J, Hatiboglu G, Niehrs C. Post-transcriptional wnt signaling governs epididymal sperm maturation. Cell (2015) 163:1225–36. doi: 10.1016/j.cell.2015.10.029
128. Aberle H, Bauer A, Stappert J, Kispert A, Kemler R. Beta-catenin is a target for the ubiquitin-proteasome pathway. EMBO J (1997) 16:3797–804. doi: 10.1093/emboj/16.13.3797
129. Niehrs C, Acebron SP. Wnt signaling: multivesicular bodies hold GSK3 captive. Cell (2010) 143:1044–6. doi: 10.1016/j.cell.2010.12.003
130. Taelman VF, Dobrowolski R, Plouhinec JL, Fuentealba LC, Vorwald PP, Gumper I, et al. Wnt signaling requires sequestration of glycogen synthase kinase 3 inside multivesicular endosomes. Cell (2010) 143:1136–48. doi: 10.1016/j.cell.2010.11.034
131. Stamos JL, Weis WI. The beta-catenin destruction complex. Cold Spring Harb Perspect Biol (2013) 5:a007898. doi: 10.1101/cshperspect.a007898
132. Vinyoles M, Del Valle-Perez B, Curto J, Vinas-Castells R, Alba-Castellon L, Garcia, et al. Multivesicular GSK3 sequestration upon wnt signaling is controlled by p120-catenin/cadherin interaction with LRP5/6. Mol Cell (2014) 53:444–57. doi: 10.1016/j.molcel.2013.12.010
133. Acebron SP, Niehrs C. Beta-Catenin-Independent roles of Wnt/LRP6 signaling. Trends Cell Biol (2016) 26:956–67. doi: 10.1016/j.tcb.2016.07.009
134. Logan CY, Nusse R. The wnt signaling pathway in development and disease. Annu Rev Cell Dev Biol (2004) 20:781–810. doi: 10.1146/annurev.cellbio.20.010403.113126
135. Bilic J, Huang YL, Davidson G, Zimmermann T, Cruciat CM, Bienz M, et al. Wnt induces LRP6 signalosomes and promotes dishevelled-dependent LRP6 phosphorylation. Science (2007) 316:1619–22. doi: 10.1126/science.1137065
136. Zeng X, Tamai K, Doble B, Li S, Huang H, Habas R, et al. A dual-kinase mechanism for wnt co-receptor phosphorylation and activation. Nature (2005) 438:873–7. doi: 10.1038/nature04185
137. Mao B, Wu W, Li Y, Hoppe D, Stannek P, Glinka A, et al. LDL-receptor-related protein 6 is a receptor for dickkopf proteins. Nature (2001) 411:321–5. doi: 10.1038/35077108
138. Kim KA, Zhao J, Andarmani S, Kakitani M, Oshima T, Binnerts ME, et al. R-spondin proteins: a novel link to beta-catenin activation. Cell Cycle (2006) 5:23–6. doi: 10.4161/cc.5.1.2305
139. Ye X, Wang Y, Cahill H, Yu M, Badea TC, Smallwood PM, et al. Norrin, frizzled-4, and Lrp5 signaling in endothelial cells controls a genetic program for retinal vascularization. Cell (2009) 139:285–98. doi: 10.1016/j.cell.2009.07.047
140. Hanai J, Gloy J, Karumanchi SA, Kale S, Tang J, Hu G, et al. Endostatin is a potential inhibitor of wnt signaling. J Cell Biol (2002) 158:529–39. doi: 10.1083/jcb.200203064
141. Chen Y, Hu Y, Lu K, Flannery JG, Ma JX. Very low density lipoprotein receptor, a negative regulator of the wnt signaling pathway and choroidal neovascularization. J Biol Chem (2007) 282:34420–8. doi: 10.1074/jbc.M611289200
142. Lee K, Shin Y, Cheng R, Park K, Hu Y, Mcbride J, et al. Receptor heterodimerization as a novel mechanism for the regulation of wnt/beta-catenin signaling. J Cell Sci (2014) 127:4857–69. doi: 10.1242/jcs.149302
143. Carson-Walter EB, Watkins DN, Nanda A, Vogelstein B, Kinzler KW, St Croix B. Cell surface tumor endothelial markers are conserved in mice and humans. Cancer Res (2001) 61:6649–55.
144. Bagley RG, Rouleau C, Weber W, Mehraein K, Smale R, Curiel M, et al. Tumor endothelial marker 7 (TEM-7): a novel target for antiangiogenic therapy. Microvasc Res (2011) 82:253–62. doi: 10.1016/j.mvr.2011.09.004
145. Yamaji Y, Yoshida S, Ishikawa K, Sengoku A, Sato K, Yoshida A, et al. TEM7 (PLXDC1) in neovascular endothelial cells of fibrovascular membranes from patients with proliferative diabetic retinopathy. Invest Ophthalmol Vis Sci (2008) 49:3151–7. doi: 10.1167/iovs.07-1249
146. Nanda A, Buckhaults P, Seaman S, Agrawal N, Boutin P, Shankara S, et al. Identification of a binding partner for the endothelial cell surface proteins TEM7 and TEM7R. Cancer Res (2004) 64:8507–11. doi: 10.1158/0008-5472.CAN-04-2716
147. Gaultier A, Simon G, Niessen S, Dix M, Takimoto S, 3rd Cravatt BF, et al. LDL receptor-related protein 1 regulates the abundance of diverse cell-signaling proteins in the plasma membrane proteome. J Proteome Res (2010) 9:6689–95. doi: 10.1021/pr1008288
148. Lee HK, Seo IA, Park HK, Park HT. Identification of the basement membrane protein nidogen as a candidate ligand for tumor endothelial marker 7 in vitro and in vivo. FEBS Lett (2006) 580:2253–7. doi: 10.1016/j.febslet.2006.03.033
149. Miller SF, Summerhurst K, Runker AE, Kerjan G, Friedel RH, Chedotal A, et al. Expression of Plxdc2/TEM7R in the developing nervous system of the mouse. Gene Expr Patterns (2007) 7:635–44. doi: 10.1016/j.modgep.2006.12.002
150. Yamamoto N, Eguchi A, Hirokawa Y, Ogura S, Sugimoto K, Iwasa M, et al. Expression pattern of plexin domain containing 2 in human hepatocellular carcinoma. Monoclon Antib Immunodiagn Immunother (2020) 39:57–60. doi: 10.1089/mab.2019.0050
151. Miller-Delaney SF, Lieberam I, Murphy P, Mitchell KJ. Plxdc2 is a mitogen for neural progenitors. PloS One (2011) 6:e14565. doi: 10.1371/journal.pone.0014565
152. Schwarze SR, Fu VX, Desotelle JA, Kenowski ML, Jarrard DF. The identification of senescence-specific genes during the induction of senescence in prostate cancer cells. Neoplasia (2005) 7:816–23. doi: 10.1593/neo.05250
153. Bianchi E, Sun Y, Almansa-Ordonez A, Woods M, Goulding D, Martinez-Martin N, et al. Control of oviductal fluid flow by the G-protein coupled receptor Adgrd1 is essential for murine embryo transit. Nat Commun (2021) 12:1251. doi: 10.1038/s41467-021-21512-w
154. Digiacomo V, Meruelo D. Looking into laminin receptor: critical discussion regarding the non-integrin 37/67-kDa laminin receptor/RPSA protein. Biol Rev Camb Philos Soc (2016) 91:288–310. doi: 10.1111/brv.12170
155. Gong Q, Qiu S, Li S, Ma Y, Chen M, Yao Y, et al. Proapoptotic PEDF functional peptides inhibit prostate tumor growth–a mechanistic study. Biochem Pharmacol (2014) 92:425–37. doi: 10.1016/j.bcp.2014.09.012
156. Koch S, Claesson-Welsh L. Signal transduction by vascular endothelial growth factor receptors. Cold Spring Harb Perspect Med (2012) 2:a006502. doi: 10.1101/cshperspect.a006502
157. Smith GA, Fearnley GW, Tomlinson DC, Harrison MA, Ponnambalam S. The cellular response to vascular endothelial growth factors requires co-ordinated signal transduction, trafficking and proteolysis. Biosci Rep (2015) 35. doi: 10.1042/BSR20150171
158. Peach CJ, Mignone VW, Arruda MA, Alcobia DC, Hill SJ, Kilpatrick LE, et al. Molecular pharmacology of VEGF-a isoforms: binding and signalling at VEGFR2. Int J Mol Sci (2018) 19. doi: 10.3390/ijms19041264
159. Kabrun N, Buhring HJ, Choi K, Ullrich A, Risau W, Keller G. Flk-1 expression defines a population of early embryonic hematopoietic precursors. Development (1997) 124:2039–48. doi: 10.1242/dev.124.10.2039
160. Ishida A, Murray J, Saito Y, Kanthou C, Benzakour O, Shibuya M, et al. Expression of vascular endothelial growth factor receptors in smooth muscle cells. J Cell Physiol (2001) 188:359–68. doi: 10.1002/jcp.1121
161. Witmer AN, Dai J, Weich HA, Vrensen GF, Schlingemann RO. Expression of vascular endothelial growth factor receptors 1, 2, and 3 in quiescent endothelia. J Histochem Cytochem (2002) 50:767–77. doi: 10.1177/002215540205000603
162. Simons M, Gordon E, Claesson-Welsh L. Mechanisms and regulation of endothelial VEGF receptor signalling. Nat Rev Mol Cell Biol (2016) 17:611–25. doi: 10.1038/nrm.2016.87
163. Leppanen VM, Prota AE, Jeltsch M, Anisimov A, Kalkkinen N, Strandin T, et al. Structural determinants of growth factor binding and specificity by VEGF receptor 2. Proc Natl Acad Sci USA (2010) 107:2425–30. doi: 10.1073/pnas.0914318107
164. Brozzo MS, Bjelic S, Kisko K, Schleier T, Leppanen VM, Alitalo K, et al. Thermodynamic and structural description of allosterically regulated VEGFR-2 dimerization. Blood (2012) 119:1781–8. doi: 10.1182/blood-2011-11-390922
165. Shweiki D, Itin A, Soffer D, Keshet E. Vascular endothelial growth factor induced by hypoxia may mediate hypoxia-initiated angiogenesis. Nature (1992) 359:843–5. doi: 10.1038/359843a0
166. Mcmahon G. VEGF receptor signaling in tumor angiogenesis. Oncologist (2000) 5 Suppl 1:3–10. doi: 10.1634/theoncologist.5-suppl_1-3
167. Zhang SX, Wang JJ, Gao G, Parke K, Ma JX. Pigment epithelium-derived factor downregulates vascular endothelial growth factor (VEGF) expression and inhibits VEGF-VEGF receptor 2 binding in diabetic retinopathy. J Mol Endocrinol (2006) 37:1–12. doi: 10.1677/jme.1.02008
168. Shahbazi B, Arab SS, Mafakher L, Azadmansh K, Teimoori-Toolabi L. Computational assessment of pigment epithelium-derived factor as an anti-cancer protein during its interaction with the receptors. J Biomol Struct Dyn (2022), 1–17. doi: 10.1080/07391102.2022.2069863
169. Nirody JA, Budin I, Rangamani P. ATP synthase: evolution, energetics, and membrane interactions. J Gen Physiol (2020) 152. doi: 10.1085/jgp.201912475
170. Notari L, Arakaki N, Mueller D, Meier S, Amaral J, Becerra SP. Pigment epithelium-derived factor binds to cell-surface F(1)-ATP synthase. FEBS J (2010) 277:2192–205. doi: 10.1111/j.1742-4658.2010.07641.x
171. Qiu F, Zhang H, Yuan Y, Liu Z, Huang B, Miao H, et al. A decrease of ATP production steered by PEDF in cardiomyocytes with oxygen-glucose deprivation is associated with an AMPK-dependent degradation pathway. Int J Cardiol (2018) 257:262–71. doi: 10.1016/j.ijcard.2018.01.034
172. Zheng B, Li T, Chen H, Xu X, Zheng Z. Correlation between ficolin-3 and vascular endothelial growth factor-to-pigment epithelium-derived factor ratio in the vitreous of eyes with proliferative diabetic retinopathy. Am J Ophthalmol (2011) 152:1039–43. doi: 10.1016/j.ajo.2011.05.022
173. Wang Y, Lu Q, Gao S, Zhu Y, Gao Y, Xie B, et al. Pigment epithelium-derived factor regulates glutamine synthetase and l-glutamate/l-aspartate transporter in retinas with oxygen-induced retinopathy. Curr Eye Res (2015) 40:1232–44. doi: 10.3109/02713683.2014.990639
174. Holekamp NM, Bouck N, Volpert O. Pigment epithelium-derived factor is deficient in the vitreous of patients with choroidal neovascularization due to age-related macular degeneration. Am J Ophthalmol (2002) 134:220–7. doi: 10.1016/S0002-9394(02)01549-0
175. Abu El-Asrar AM, Imtiaz Nawaz M, Kangave D, Siddiquei MM, Geboes K. Osteopontin and other regulators of angiogenesis and fibrogenesis in the vitreous from patients with proliferative vitreoretinal disorders. Mediators Inflammation (2012) 2012:493043. doi: 10.1155/2012/493043
176. Sheibani N, Wang S, Darjatmoko SR, Fisk DL, Shahi PK, Pattnaik BR, et al. Novel anti-angiogenic PEDF-derived small peptides mitigate choroidal neovascularization. Exp Eye Res (2019) 188:107798. doi: 10.1016/j.exer.2019.107798
Keywords: pigment epithelium-derived factor (PEDF), receptor, adipose triglyceride lipase (ATGL), laminin receptor (LR), lipoprotein receptor-related protein 6 (LRP6), plexin domain-containing (PLXDC), F1-ATP synthase, vascular endothelial growth factor receptor 2 (VEGFR2)
Citation: Xu M, Chen X, Yu Z and Li X (2023) Receptors that bind to PEDF and their therapeutic roles in retinal diseases. Front. Endocrinol. 14:1116136. doi: 10.3389/fendo.2023.1116136
Received: 07 December 2022; Accepted: 04 April 2023;
Published: 17 April 2023.
Edited by:
Rajashekhar Gangaraju, University of Tennessee Health Science Center (UTHSC), United StatesReviewed by:
Vicente Bermúdez, Instituto de Investigaciones Bioquímicas de Bahía Blanca (INIBIBB), ArgentinaGao Guoquan, Zhongshan School of Medicine, Sun Yat-sen University, China
Copyright © 2023 Xu, Chen, Yu and Li. This is an open-access article distributed under the terms of the Creative Commons Attribution License (CC BY). The use, distribution or reproduction in other forums is permitted, provided the original author(s) and the copyright owner(s) are credited and that the original publication in this journal is cited, in accordance with accepted academic practice. No use, distribution or reproduction is permitted which does not comply with these terms.
*Correspondence: Xiaorong Li, bGl4aWFvcm9uZ0B0bXUuZWR1LmNu
†These authors have contributed equally to this work