- 1School of Clinical Medicine, Chengdu University of Traditional Chinese Medicine, Chengdu, China
- 2Department of Endocrinology, Hospital of Chengdu University of Traditional Chinese Medicine, Chengdu, China
- 3College of Acupuncture & Tuina, Shaanxi University of Chinese Medicine, Xianyang, China
Osteoporosis is a systemic multifactorial bone disease characterized by low bone quality and density and bone microstructure damage, increasing bone fragility and fracture vulnerability. Increased osteoclast differentiation and activity are important factors contributing to bone loss, which is a common pathological manifestation of bone diseases such as osteoporosis. TNF-a/NF-κB is an inflammatory signaling pathway with a key regulatory role in regulating osteoclast formation, and the classical pathway RANKL/RANK/OPG assists osteoclast formation. Activation of this inflammatory pathway promotes the formation of osteoclasts and accelerates the process of osteoporosis. Recent studies and emerging evidence have consistently demonstrated the potential of probiotics to modulate bone health. Secretions of Bifidobacterium, a genus of probiotic bacteria in the phylum Actinobacteria, such as short-chain fatty acids, equol, and exopolysaccharides, have indicated beneficial effects on bone health. This review discusses the molecular mechanisms of the TNF-a/NF-κB inflammatory pathway in regulating osteoclast formation and describes the secretions produced by Bifidobacterium and their potential effects on bone health through this pathway, opening up new directions for future research.
1 Introduction
Physiologically and pathologically, bone volume fraction depends mainly on the rate of bone formation by osteoblasts and the rate of resorption by osteoclasts. In most pathological bone diseases such as osteoporosis, excessive bone resorption by osteoclasts is the main cause of bone loss. At present, three main categories of drugs are used for osteoporosis treatment: anti-resorptive agents (inhibits osteoclasts), bone-forming agents (boost osteoblasts), and dual-effect drugs (both promote bone formation and inhibit osteoclasts) (1). Although they have shown good clinical efficacy, their side effects cannot be ignored (2–5). For example, bisphosphonates are a type of anti-bone resorption agent that increases the survival and activity of osteoclasts mainly by inhibiting the mevalonate biosynthetic pathway or by binding to a non-hydrolyzable analogue of ATP (6). As a first-line drug for osteoporosis, bisphosphonates have demonstrated some beneficial effects, including increasing bone density and lowering the risk of fracture (7), particularly hip fracture (8). Bisphosphonates are commonly well tolerated, but side effects can occur in up to 10% of patients, mainly including arthralgia, myalgia, and gastrointestinal discomfort (9). Other negative effects including uveitis (10), atypical femoral fractures (AFFs) (11), and osteonecrosis of the jaw (ONJ) (12), are relatively uncommon. Selective estrogen receptor modulators (SERMs) and anti-RANKL monoclonal antibodies are the other two classes of anti-resorptive drugs. SERMs include raloxifene and bazedoxifene, both of which have been shown to effectively prevent bone loss and reduce bone turnover (13). However, in a three-year clinical trial, the incidence of vasodilatation (hot flashes), leg cramps and venous thromboembolic events was significantly higher in the bazedoxifene and raloxifene groups compared with the placebo group (14). The latter is represented by denosumab, a human monoclonal antibody against RANKL, a key bone resorption mediator (15). Postmenopausal women treated with the drug showed a remarkable long-term reduction in the risk of fracture of up to 10 years (16). But, after treatment was stopped, the rate of vertebral fractures rose to the equivalent in untreated people (2). As a potent bone-forming drug, teriparatide is a good candidate for improving bone microstructure (17). However, it is only used in patients with grievous osteoporosis because it requires daily subcutaneous injection and is significantly more expensive than other osteoporosis medications (18). Romosozumab is a monoclonal antibody that binds sclerostin and has a dual regulatory effect of promoting bone formation and suppressing bone resorption (19). According to the findings of the current study, romosozumab is contraindicated for individuals with a recent history of a cardiovascular incident and should only be used with caution in patients with a high cardiovascular risk (5). The safety and affordability of the drug are key concerns for patients. Therefore, how to innovate and optimize the safety and efficacy of therapeutic drugs, while keeping them affordable to osteoporosis patients is a practical clinical problem that needs to be addressed.
In recent years, probiotics have become a research hotspot. Probiotics are described as living microorganisms, which, in sufficient quantities, provide health benefits to the host (20). Probiotics have been used as preventive and curative therapy for multiple illnesses, including diabetes (21, 22), hypothyroidism (23), Hashimoto’s thyroiditis (24), and osteoporosis (25, 26). In particular, their effects on osteoporosis are even more far-reaching (25, 27, 28). Probiotic preparations are live bacterial preparations composed of probiotics or probiotic growth-promoting substances that confer health benefits to the host, using microbiological principles (29). Among them, Bifidobacterium, Lactobacillus, Escherichia, Enterococcus, Bacillus and Streptococcus are the most commonly bacteria used in probiotic preparations (30–32). In particular, Bifidobacterium inhibits osteoclast formation to ameliorate osteoporosis. Several research and clinical studies have demonstrated that, probiotics, despite their disease-prevention and treatment effects, are not absolutely safe or without side effects (33). Probiotics may be an occasional risk factor for sepsis (34). However, in general, the benefits of probiotics outweigh the disadvantages, especially the lower incidence of adverse events in Bifidobacterium therapy (34).
RANKL/RANK/OPG has been extensively corroborated as a classical pathway for regulating osteoclast formation, but its relationship with the TNF-α/NF-κB signaling pathway has elicited great interest in recent years. Bifidobacterium, a probiotic of the intestine, has profound effects on the TNF-α/NF-κB inflammatory pathway. Its secretions, including short-chain fatty acids, equol, and exopolysaccharides have distinct effects on the aforementioned inflammatory pathway. Among them, short-chain fatty acids and equol have been extensively demonstrated to exert inhibitory effects on osteoclast formation independent of this signaling pathway. This review provides an overview of the specific mechanisms of Bifidobacterium inhibition of the TNF-α/NF-κB inflammatory pathway to affect osteoclast formation. Through this review, we attempt to provide researchers with new insights into potential targets for the development of effective therapies for osteoporosis.
2 TNF-a/NF-κB signaling pathway
TNF-α/NF-κB is a well-known inflammatory signaling pathway (35) that is implicated in the development of endocrine system illnesses, particularly osteoporosis (36, 37). The tumor necrosis factor (TNF) superfamily molecules are mostly produced by macrophages (38). Among them, TNF-α is a vigorous pro-inflammatory cytokine with a crucial role in immune function, inflammation, and regulation of cell growth, differentiation, and apoptosis (39). TNF-α requires cell surface receptors tumor necrosis factor receptor 1 (TNFR1) and tumor necrosis factor receptor 2 (TNFR2) to exert its biological effects (40). Whereas TNFR1 and TNFR2 have extracellular domains enriched with cysteine, their intracellular domains are structurally very different. Notably, TNFR1 contains a conserved 80-amino acid sequence called the cytoplasmic “death domain,” which produces a characteristic fold (41). Through this death structural domain, TNFR1 can sequentially recruit tumor necrosis factor receptor-associated death domain protein (TRADD), TNFR-associated factor 2 (TRAF2), receptor-interacting protein, and nuclear factor-κB (NF-κB) kinase inhibitor (IKK), thereby activating NF-κB (40). Contrarily, TNFR2 does not have a cytoplasmic death region sequence and recruits TNFR-associated factor 1 (TRAF1) and TNFR-associated factor 2 (TRAF2), but not TRADD (42). Despite this difference, the signaling cascades downstream of TNFR1-TRADD-TRAF2 and TNFR2-TRAF2 are similar.
NF-κB is a homodimeric and heterodimeric complex composed of five members of the Rel family, including NF-kB1 (p50), NF-kB2 (p52), RelA (p65), RelB, and c-Rel (43). These factors regulate the expression of several genes involved in immune response and numerous other cellular processes, including growth, development, and apoptosis (44). In most unstimulated cells, IkB proteins are maintained inactive in the cytoplasm by interacting with NF-kB dimers (45). Kappa B inhibitor kinase (IKK) is a heterotrimeric enzyme made up of the kinase subunits IKKa and IKKb as well as the regulatory subunit IKKγ/NEMO (46). When activated, IKK phosphorylates and degrades two important serine residues in the N-terminal regulatory domain of the NF-κB inhibitor IkB, releasing NF-κB (47). After its release, NF-κB generates cytokines such as p50 and p65, which promote its translocation to the nucleus to revive transcription (47). When NF-κB p50 and p52 are expressed, RANKL-RANK induces osteoclastogenesis (36). NF-κB promotes the activation of c-Fos (48), a member of the Fos gene family, which together with Jun proteins make up the AP-1 family of heterodimeric transcription factors (49). Without c-Fos, osteoclasts cannot develop (50). Boyce et al. demonstrated that c-Fos primarily generates and interacts with NFATc1 to initiate a transcriptional regulatory cascade, which results in upregulation of several target genes involved in osteoclast development and function (51). The TNF-α/NF-κB signaling pathway is in Figure 1.
3 RANKL/RANK/OPG signaling pathway
RANKL/RANK/OPG is the predominant signaling pathway regulating osteoclast differentiation (52). The differentiation of osteoclasts primarily involves the fusion of monocytes to multinucleated osteoclasts in response to three cytokines: macrophage colony-stimulating factor (M-CSF), nuclear factor-κB (NF-κB) ligand receptor activator (RANKL) and osteoprotegerin (OPG) (25). M-CSF (also known as colony-stimulating factor-1) regulates mononuclear phagocyte production, through a process mediated by CSF-1 receptor (CSF-1R), which is encoded by the c-FMS proto-oncogene (53). As a dimeric cytokine, it modulates the formation of many different types of cells, such as trophoblasts, macrophages, and osteoclasts (54). In the early stage of osteoclast development, M-CSF binds to c-FMS expressed on precursor cells activating their proliferation (53). A type II membrane protein with close homology to the TNFSF members TRAIL, FasL, and TNF-a (55), RANKL is expressed on osteoblasts as a membrane-associated cytokine (56). In vitro, RANKL activates mature osteoclasts in a dose-dependent manner, but can activate pre-existing osteoclasts quickly to cause bone resorption in vivo (57, 58). Additionally, it has been demonstrated that M-CSF and RANKL promote the differentiation of osteoclast precursor cells into mature and functional osteoclasts (56, 59). Collectively, CSF-1 and RANKL can stimulate the expression of genes that characterize the osteoclast lineage, including those that encode tartrate-resistant acid phosphatase, cathepsin K, and calcitonin receptor, resulting in the maturation of osteoclasts (60). Through intercellular contacts, osteoblasts express RANKL, which is recognized and binds to osteoclast precursors, which then develop into osteoclasts in the presence of M-CSF (61). Meanwhile, M-CSF strongly promotes the binding of RANK to RANKL and the formation of osteoclasts (62).
Osteoprotegerin (OPG) is a cytokine receptor protein produced by osteoblasts (63). It acts as a decoy receptor by binding to RANKL to block its interaction with its functional receptor RANK, thereby inhibiting osteoclast formation (64). OPG has also been found to cause osteoclast pseudopod disassembly and safeguard the bone cortex via pathways like the Ca-p38-MAPK signaling pathway, inhibit RANKL binding to RANK, prevent osteoblast-induced osteoclast precursor cell differentiation, and control osteoclast function (65). Li et al. demonstrated that cytokines (such as OPG and RANKL) directly interact with bone regulating proteins to enhance bone homeostasis (66). Another study confirmed that dexamethasone-induced osteoporosis can be improved by restoring OPG expression by decreasing RANKL expression (67). Hence, the ratio of OPG/RANKL determines the degree of bone resorption and the course of bone metabolism.
4 TNF-α/NF-κB signaling pathway and RANKL/RANK/OPG signaling pathway
Inflammation is closely linked to osteoporosis. TNF-α is a potent pro-inflammatory cytokine (39), and IL-6 is a “classical” bone resorption pro-inflammatory cytokine (68). It has been demonstrated that IL-1, a pro-inflammatory cytokine, promotes osteoclast production, which in turn stimulates bone resorption (69). TNF-α stimulates inflammatory cytokine mRNA transcription, which results in the production of IL-6 (70). It has been discovered that recombinant human tumor necrosis factor (rTNF-α) naturally induces IL-1 in the body (71). Furthermore, IL-1 can induce the expression of TNF-α via an autocrine mechanism (72) and IL-1 induces the production of IL-6 (73). Thus, inflammatory cytokines can not only promote bone resorption alone, but their mutual activation can enhance the activation of TNF-α/NF-κB signaling pathway, activate osteoclast-related genes, and enhance bone resorption, which can be seriously detrimental to osteoporotic patients. The cytokines TNF-α, IL-6, and IL-1 cause a significant augmentation of osteoclasts and a suppression of osteoblast activity when RANKL is present (74). IL-17 is an another pro-inflammatory cytokine that promotes bone resorption via upregulating RANKL (75). IL-6 trans-signaling directly increases RANKL on fibroblast-like synovial cells and is involved in the induction of RANKL by TNF and IL-17 (76). In addition, IL-6 and TNF-α can synergistically activate NF-κB (77). Ciucci et al. further ascertained that bone marrow CD4+ T cells belong to a distinct subpopulation of osteoclastic T cells termed Th17 TNF-α (+) cells that can generate IL-17 and TNF-α (78). These cells move to the bone marrow amid chronic inflammation, where they facilitate the recruitment of inflammatory monocytes (mainly osteoclast progenitors) (78). In an inflammatory event, immune system cells, such as T cells, B cells, macrophages, and dendritic cells, become activated and release inflammatory cytokines, which are among the most crucial mediators in bone immunology (74). Activated T cells are particularly significant mediators because they increase the production of the so-called bone resorbing cytokines, including TNF- and RANKL (74). Thus, the formation of osteoclasts is closely associated with chronic inflammation. In addition to stimulating osteoclast formation through the NF-κB signaling pathway, TNF-α can also mediate RANK ligands activation of osteoclast formation via an autocrine mechanism (79). The combination of TNF-α and RANKL greatly stimulated osteoclast formation and significantly up-regulated osteoclast mRNA markers (37).
To sum up, inhibiting the TNF-α/NF-κB inflammatory pathway can impede the formation of osteoclasts. Multiple studies (80, 81) have demonstrated a strong link between inflammation and osteoclast formation. The important role of the NK-kB transcription factor family in inflammation and innate immunity has also been elucidated (82, 83). Inhibition of osteoclast formation via the NF-kB pathway has also been reported. For example, preparations of Zanthoxylum piperitum (84), Sophorae flos (85), and Bajijiasu (86), were shown to inhibit the RANKL-induced NF-κB/NFATc1 pathway in osteoblasts to hinder bone resorption. As a pro-inflammatory cytokine, TNF-α promotes the production of osteoclasts by activating the NF-κB pathway, synergizing RANKL cytokines, and facilitating and enhancing RANK-RANKL binding. In periodontitis, down-regulating TNF-α, alveolar bone loss was delayed (87). In addition, Yao et al. explained the regulation of TNF-α-induced osteoclast formation (88). The effect of RANKL/RANK/OPG on osteoclasts has also been confirmed by many investigators (52, 89, 90). TNF-α/NF-κB signaling pathway and RANKL/RANK/OPG signaling pathway induced-osteoclast have been shown in Figure 2.
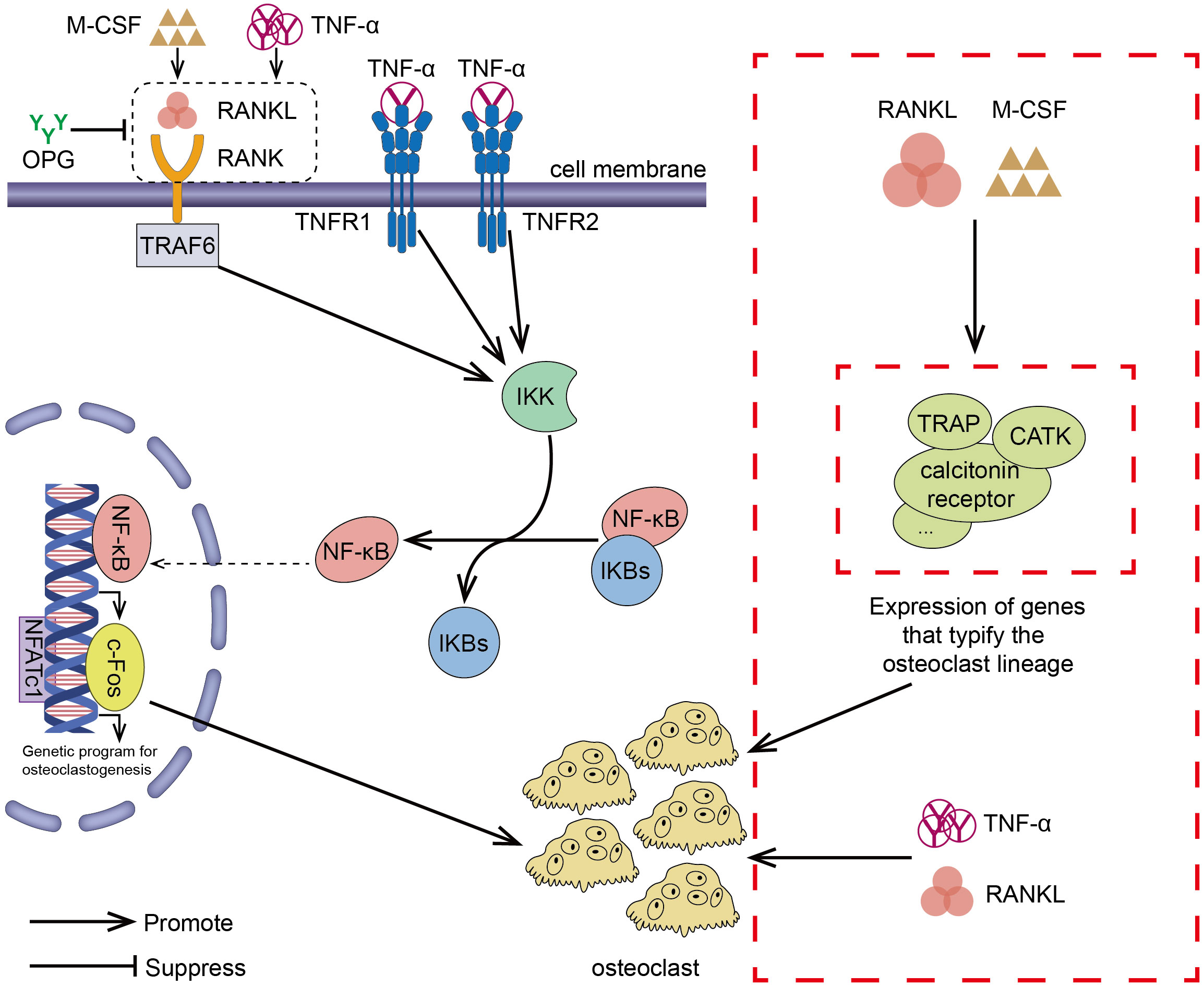
Figure 2 RANKL/RANK/OPG is the most important signaling pathway regulating osteoclasts formation. The interaction between RANK and RANKL (which can exacerbate this effect in the presence of M-CSF) promotes the recruitment of the TRAF family bridging proteins, one of which TRAF6 contributes to OC formation and activation of the NF-κB signaling pathway, leading to the transcription of genes involved in OC formation and OC production. OPG is a decoy receptor that binds RANKL and can block the binding and activation of RANK and RANKL, reducing OC production. TNF-α/NF-κB is an inflammatory signaling pathway. In the presence of TNF-α, NF-κB pathway is activated, OC production is increased, and the interaction between RANKL and RANK is enhanced, which results in activation of the downstream signaling pathways. In addition to the above-mentioned effects, TNF-α also synergizes with RANKL and directly promotes OC production. In the presence of RANKL and M-CSF, the expression of genes involved in OC formation leading to the development of mature OC.
In recent years, the impact of probiotics on bone health has become a hotspot of research. Current research suggests that probiotics regulate bone metabolism through different mechanisms, including intestinal barrier permeability (91), metabolite production (92), the immune response (93), and inflammation (94). Postmenopausal bone mineral density loss, which is associated with estrogen deficiency (95), was effectively reduced by probiotic supplementation, which also improved bone turnover in 78 postmenopausal patients with osteoporosis for more than a year (96). In another trial, probiotics significantly increased total hip bone density compared with placebo and modulated gut microbiota in postmenopausal women (97). In response to the aforementioned pathways, recent reports show that probiotics, particularly Bifidobacterium, have more benefits for bone health (Table 1).
5 Bifidobacterium
The most prevalent phyla of the human gut microbiota are Firmicutes, Bacteroidetes, and Actinobacteria (105). The phyla Firmicutes and Bacteroidetes collectively account for 90% of colonic microbiota (60–75% and 30–40%, respectively) (106, 107). By comparison, the phylum Actinomycetes is a smaller but essential component for preserving intestinal homeostasis (108). Bifidobacterium, which belongs to the phylum Actinobacteria (105), was first isolated from the feces of healthy breastfed infants by a French pediatrician Tissier and named Bifidobacterium because of its commonly bifurcated ends (109). Bifidobacterium can produce short-chain fatty acids (SCFA) (110), equol (Eq) (111), exopolysaccharides (112) and many other substances that can affect osteoclast formation, which is shown in Figure 3.
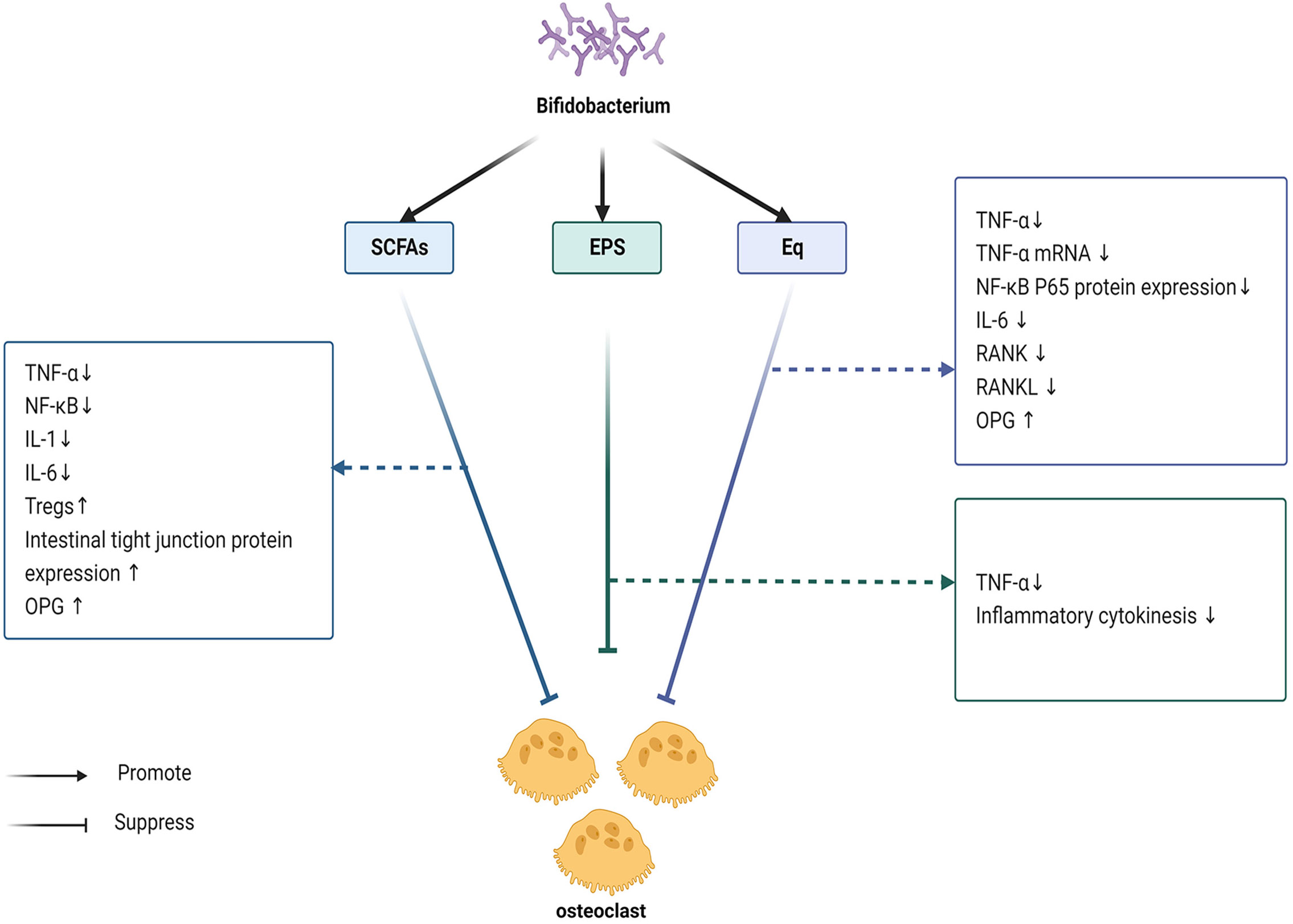
Figure 3 Bifidobacterium mainly produces SCFA, Eq and EPS. These three substances inhibit the TNF-α/NF-κB signaling pathway, reduces the production of inflammatory mediators, and blocks the activation of inflammatory mediators, thereby preventing the formation of OC. SCFA can promote the production of Treg cells, indirectly inhibit the TNF-α/NF-κB signaling pathway, and also regulate OC formation through Treg cells. In addition, SCFA plays a role in the maintenance of the intestinal mucosal barrier, blocking the entry of inflammatory factors into the bloodstream, and reducing inflammation, leading to the inhibition of OC formation. More importantly, it has been shown that SCFA also increases the production of OPG. Eq also decreases the production of inflammatory factors, such as IL-6, inhibits RANK and RANKL, and can upregulate OPG expression. EPS mainly inhibits the production of inflammatory factors.
5.1 Short chain fatty acids
SCFAs are molecules less than 6 carbon atoms in size (C1-C6) (113). Intestinal bacteria degrade dietary fiber into products such as butyrate, propionate, and acetate so on, which are collectively called SCFAs (114). Acetate, fructose, and lactate can be produced during the fermentation of dietary fiber by Bifidobacterium, but not butyrate (115). However, in hybrid cultures composed of Bifidobacterium and E. coli or A. caccae, the addition of dietary fiber results in the production of butyrate (115). This study shows that SCFAs not only directly affect the TNF-α/NF-κB inflammatory pathway, but also inhibit TNF-α/NF-κB-mediated inflammation by promoting the secretion of regulatory T cells and increasing intestinal tight junction protein expression, thereby controlling osteoclast formation. In addition, osteoclasts can be regulated by Treg cells independent of this pathway. Of course, the direct effect of SCFAs on osteoclasts cannot be ignored.
There are substantial reports on anti-inflammatory properties of SCFAs, including the results of basic experiments done to inhibit the TNF-α/NF-κB inflammatory pathway. At low concentrations, acetic acid, propionic acid, and butyric acid exert potent anti-inflammatory effects by inhibiting the generation of pro-inflammatory agents, such as NO, TNF-α, IL-1, and IL-6 (116). Downar et al. showed that pretreatment of human umbilical vein endothelial cells with butyric acid suppressed TNF-α-induced activation of NF-κB (117). In another experiment, all SCFAs dose-dependently decreased NF-κB reporter activity in Colo320DM cells and 30 mmol/L of acetate, propionate, and butyrate reduced LPS-stimulated TNF-α release from neutrophils (118). Certainly, by directly blocking NF-kB, SCFAs may also alleviate S. aureus-induced inflammatory response (119). In mice maintained in germ-free environment and fed with 150 mM SCFA via drinking water for three weeks, SCFA, either alone or in combination (SCFA mixture), was found to increase the frequency and amount of cTreg (120). Foxp3+ CD4+ regulatory T cells (Tregs) are a subset of immune cells that regulate tissue inflammation (121). Treg cells can restrain the function of effector T cells, thus reducing the output of TNF-α (122). They also produce interleukin-10 (IL-10), which limits environmental interface inflammation (123) and inhibits osteoclastogenesis (124). Bogdan et al. demonstrated that recombinant mouse IL-10 effectively inhibited the capacity of murine peritoneal macrophages to release TNF-α (125). The anti-inflammatory properties of Treg cells not only confer an advantage, but they can also affect osteoclast formation independent of the TNF-α/NF-κB pathway. Tregs cells can also inhibit macrophage colony-stimulating factor and RANKL to promote osteoclast formation in a dose-dependent manner (126). Compared with wild-type litter controls, FoxP3-Tg mice had higher bone mass, indicating that Treg cells can regulate bone resorption in vivo (127). However, no reduction in osteoclast production was seen when butyric acid or propionic acid was added 24 or 48 hours after osteoclast differentiation, and acetate alone had no significant effect on osteoclast differentiation (128). Thus, SCFAs may inhibitory effect on osteoclast production but not on osteoclasts that have already been generated.
TNF-α, which may be linked to pathogenic intestinal inflammation, can affect the shape and function of tight junctions, impairing epithelial barrier function (129). Damage to the intestinal mucosal barrier aggravates the TNF-α/NF-κB-induced pro-osteoclastogenic pathway, creating a vicious cycle of inflammation and epithelial injury. Therefore, maintenance of good integrity and proper permeability of the intestinal mucosal barrier is crucial to intestinal health and protection from other diseases. In particular, intestinal permeability is maintained in normally functioning tight junctions (130). The expression of tight junction proteins, which are essential for preserving intestinal epithelial permeability, is elevated in the presence of SCFAs (131, 132). Damage to the tight junction barrier allows toxic materials to enter the body, which can cause inflammation and over-activation of the mucosal immune system (133). Additional evidence indicates that SCFAs play an important protective role in the intestinal mucosal barrier. Intestinal development in piglets may be aided by gastric infusion of SCFAs, particularly at high SCFAs concentrations, by improving intestinal shape, lowering the percentage of apoptotic cells, and maintaining intestinal barrier function (134). Another study demonstrated that oral or direct enteral drip treatment with SCFAs enhanced the proliferation of intestinal epithelial cells (135). As a result, SCFAs play a key role in maintaining intestinal epithelial stability, reducing inflammation, and preventing osteoclast formation.
SCFAs have also been shown to directly regulate osteoclasts. By stimulating human osteoblasts to produce more OPG, sodium butyrate inhibited the development of osteoclasts (136). Nonetheless, treatment with butyrate increased cell cycle arrest and drastically diminished cell proliferation in MG-63 osteoblasts (137). Therefore, the effect of SCFAs on the formation of osteoblasts are still inconclusive. However, its inhibitory effect on bone resorption in cellular experiments or animal experiments is well supported. In RAW264 cells, sodium butyrate blocks the expression of osteoclast-specific mRNA and nuclear factor-κB (NF-κB) ligand (RANKL) receptor activator-stimulated osteoclast formation (138). Mice treated with SCFAs and fed a high-fiber diet had much more bone mass and were protected from inflammation-induced bone loss (139).
In summary, SCFAs play an important role in inhibiting the TNF-α/NF-κB inflammatory signaling pathway, regulates osteoclast formation in other ways, and exerts a considerable direct effect on osteoclasts.
5.2 Equol and TNF-α/NF-κB signaling pathway
Equol, with a chemical formula C15H14O3, was initially discovered and clarified by Marrian and Haslewood (140). Equol, a soy glycosides metabolite, is categorized as a polyphenolic compound (isoflavone found in plants and foods) (141). In comparison to soy sapogenins, equol has greater estrogenic activity and a stronger affinity for estrogen receptors (142). Soybean isoflavones have been shown to improve ovx-induced osteoporosis (143). They can generate the metabolite equol in the intestine, which exerts therapeutic effect on bone metabolism (144).
Equol had been demonstrated to suppress the activation of the TNF-α/NF-κB inflammatory pathway by several authors. In addition, like SCFAs, it has been shown to exert anti-inflammatory effects independent of this pathway, further supporting its role in the inhibition of osteoclast formation. Equol dramatically reduces the level of pro-inflammatory cytokine TNF-α in mice treated with lipopolysaccharide (LPS) (145, 146). Additionally, in LPS-stimulated murine macrophages, equol dose-dependently reduced TNF-α production and TNF-α mRNA expression (146). Moreover, equol may drastically lower NF-κB P65 protein expression by suppressing the activation of the NF-κB pathway (147). Subedi et al. showed that treatment with equol decreased LPS-induced production of pro-inflammatory cytokines (such as TNF-α and IL-6) and treatment of cells (pretreated with LPS) with equol at doses of 10 and 20 µM, significantly suppressed NF-κB activity (148). Among them, IL-6 is a classical factor that promotes osteoclast formation (68), which further supports the inhibitory effect of equol on osteoclast formation.
Meanwhile, its direct regulation of osteoclasts has also received much scholarly attention in recent years. Equol (0.5 mg/day subcutaneously) treatment prevented bone loss in the femur and other bones in body of ovx mice (149). In another study, however, it was discovered that equol had no particular benefit on whole-body bone density, but had a special advantage in the femur, where it inhibited bone loss throughout, proximally and distally (150). The substance probably works better on some parts of the bone than others. But it is certain that equol plays a key role in promoting bone healing and inhibiting osteoclast formation. In severe osteoporosis presenting 10 weeks after oophorectomy, the administration of equol intervention promoted fracture healing by enhancing bone trabecular structure and raising endosteal healing tissue (151). More importantly, it reduced the expression of specific genes (e.g. Fos) in osteoblasts (152). In the classical pathway of osteoclast formation, qRT-PCR confirmed that treatment of ovx-induced rats with equol revealed decreased RANKL and RANK mRNA expression levels and upregulated OPG expression levels (153). Thus, Eq can regulate the balance between OPG and RANKL and inhibit bone loss caused by osteoclasts.
5.3 Exopolysaccharides and TNF-α/NF-κB signaling pathway
Exopolysaccharides (EPS) are lengthy polysaccharide chains that are loosely linked to the microbial cell wall and thus can easily be discharged into the nearby local milieu (154). The effect of exopolysaccharides on this signaling pathway is still relatively limited, but it has been found that it can exert an inhibitory effect on osteoclasts to some extent.
The inhibition of inflammation by EPS is bidirectional. Large molecular weight and neutrally charged EPS exert their immunosuppressive effects by preventing the release of pro-inflammatory molecules (112). EPS with small molecular weights and negative charges can boost the immune system by prompting immune cells to release cytokines, including IL-10, IL-12, and TNF-α (155). Bifidobacterium 35624, which produces EPS, more effectively suppresses the pro-inflammatory response compared with Bifidobacterium 35624, which is deficient in EPS (156). And, this study showed that the former can reverse the increase in the pro-osteoclastogenic cytokine IL-17, which is induced in the absence of EPS (156). One recent study suggests that EPS acts mainly by preventing the fusion of early osteoclast precursors, without significantly affecting the resorptive activity of mature osteoclasts (154). In contrast, mice that received peroxisulfated exopolysaccharides (OS-EPS) had more osteoclasts on the surface of their trabecular bones (157). Notably, in vitro, the early stage of osteoclast precursor adhesion was prevented by OS-EPS, thereby preventing the cell fusion stage (157). Therefore, the effects of extracellular polysaccharides on osteoclasts are still inconclusive, and further research is needed to explain these relationships and explore the conditions under which extracellular polysaccharides are beneficial for bone health.
6 Analysis and future outlooks
Osteoporosis has become a major global public health problem, with a significant economic burden on health care systems. It is a bone disease that is characterized by low bone mass and microstructural degradation, which promote bone fragility and, consequently, increase the fracture risk (158). In previous studies (25, 159), the therapeutic potential of probiotics in bone health has been demonstrated, including their positive effect on osteoporosis. However, few studies have examined the relationship between specific genera of bacteria and osteoporosis, and certainly even fewer articles have discussed their specific mechanisms of action. Therefore, in this paper, we sought to fill this gap by reviewing the mechanisms of Bifidobacterium inhibition of the TNF-α/NF-κB inflammatory pathway to prevent osteoclast formation. In germ-free mice, higher bone mass is linked to changes in the immunological state, which is reflected by decreased expression of inflammatory cytokines in bone (25). Bifidobacterium can be used to inhibit the formation of osteoclasts by altering the inflammatory immune status of bones through its secretory products.
SCFAs can directly inhibit the classical pathway of inflammation, the TNF-α/NF-κB signaling pathway, to strongly inhibit inflammation. It can also promote the secretion of Tregs cells, regulate inflammation through the immune system, increase the expression of intestinal tight junction proteins, block the invasion of harmful substances through the intestinal mucosa, and effectively control inflammatory response. In the immune system, regulatory T cells (Treg cells) expressing the transcription factor Foxp3 have been shown to act as inhibitors of inflammatory response in the gut, and helper T cells 17 (Th17) are pro-inflammatory cells (160). Under inflammatory conditions, Foxp3 expression on Treg cells is lost, and this allows transdifferentiate of the cells into Th17 cells (161). The balance between Th17 cells and Treg cells influences the pathogenesis of osteoporosis. Increased Th17 cell frequency has been linked to the occurrence of bone resorption (162). Th17 also secretes high quantities of IL-17, NF-kB ligand receptor activator (RANKL), and TNF as well as low levels of interferon gamma, making it the most osteolytic subpopulation of T CD4+ cells (IFNγ) (163, 164). A subpopulation of immune cells known as Treg cells inhibits the differentiation and functionality of Th17 cells (165). In addition, Treg cells can inhibit OC differentiation and bone resorption by releasing TGF-β1 and IL-10 (166). It has been reported that enrichment of SCFA-producing probiotics downregulates intestinal epithelial permeability and restores the Treg/Th17 cell ratio (27). Butyric acid, in particular, is implicated in the control of Treg/Th17 balance and prevents the formation of inflammation in colonic mucosa (167). This protective effect on the intestinal mucosa contributes to the reduction in inflammation and formation of osteoclasts. These effects are mediated by the TNF-α/NF-κB inflammatory pathway. For instance, Th17 secrete high levels of NF-kB ligand receptor activator (RANKL) and TNF, and Treg inhibits Th17 cells thereby indirectly regulating the TNF-α/NF-κB signaling inflammatory pathway. Treg also inhibits osteoclast formation, and further studies are needed to expand our understanding on this. High concentration of SCFAs can inhibit bone growth. By blocking osteoblast-specific factors, high dose of sodium butyrate prevents the differentiation and mineralization of the ROS17/2.8 rat osteoblast line (168). Therefore, further investigations are advocated to determine the optimal concentration of SCFAs for the treatment of osteoporosis. On the side, butyrate also has the ability to modulate bone anabolic metabolism through Treg cell-mediated generation of Wnt10b from CD8+ T cells (169). This demonstrates the ability of short-chain fatty acids in bone formation, and perhaps Bifidobacterium could be drugs that have the dual effect of stimulating bone formation and inhibiting bone resorption.
There have been several reports about the effect of SCFAs on osteoclast formation (128, 136, 139). However, few scholars have explored the effect of equol and exopolysaccharides on osteoclast formation. Currently, few microorganisms have been identified to produce equol, and Bifidobacterium is one of them (111). Equol can inhibits the TNF-α/NF-κB pathway to exert anti-inflammatory effects and reduce the pro-osteoclastogenic function of inflammatory cytokines (145–148, 170). Ovariectomy model (OVX) mice showed significantly reduced bone mineral density (BMD) and bone mineral content (BMC) compared with sham-operated animals, and 0.5 mg/d Eq treatment preserved bone mass (149). Furthermore, it has stronger estrogenic activity and may be a potential agent for treatment of postmenopausal osteoporosis (171). Equol is produced in the gastrointestinal system by soy glycosides, however, its metabolism in humans differs among individuals (172). This may alter the efficacy of the drug leading to different responses in various patients with osteoporosis, but this concept has not been sufficiently studied. Therefore, further clinical evaluation and analysis is still needed. In vitro experiments have demonstrated that EPS can potentially prevent osteoclast formation, however, the optimal way to use EPS in humans that guarantees stable inhibition of osteoclast formation needs to be further explored (154).
Currently, Bifidobacterium is now an important product in the market. Compared with other microbial workhorses, engineered Bifidobacterium’s produces numerous bioproducts with additional benefit while using fewer resources (173). The Bifid shunt, which generates higher number of CoA predecessors for the bioproduction of polyketide products and fatty acid biosynthesis, is one of the crucial metabolic processes in Bifidobacterium (174). Although clostridia can also produce SCFAs and Eq (111, 175), it is thought to cause pathogenicity (176). Safety assessment of Bifidobacterium species identified only 2 cases of mild functional intestinal obstruction (177) and 1 case of sepsis (178), and these results demonstrate that probiotic preparations possess pathogenic risks. Therefore, there is need to balance between the risk and the cost-benefit and safety in the clinical treatment of patients to reduce the incidence of adverse events.
Inflammatory bowel disease (IBD) has been linked to increased risk of bone mineral loss and osteoporosis (179). Bifidobacterium lactis BL-99 can be used to prevent the development of osteoporosis in patients with ulcerative colitis (UC) by shaping the intestinal flora and inhibiting the production of inflammatory cytokines (180). Probiotics have shown potential for the treatment of IBD, and therefore, the authors suggest that fecal transplants could be used in the future to regulate intestinal flora to improve the symptoms of IBD (181).. Studies have demonstrated that extensive changes occur in the structure of the intestinal flora of rats after ovariectomy (182). Compared with healthy individuals, patients with osteoporosis or bone loss showed significant changes in gut microbial species (183). This suggested a correlation between osteoporosis and the constitution as well as functionality of the intestinal flora. It is possible that fecal colony transplantation technique may be an effective treatment for patients with osteoporosis. However, FMT is processed by collecting therapeutic stools from normal individuals and its treatment success depends largely on the quality of the donor’s gut microbes. Therefore, appropriate selection of donors is crucial. Nevertheless, there are many challenges affecting the adoption of this technology (184). Further animal experiments and clinical studies are needed to clarify this.
7 Conclusion
In this review, we summarized the mechanisms by which Bifidobacterium bifidum regulates osteoclast formation by inhibiting the TNF-α/NF-κB inflammatory pathway. Its effects are mediated by its secreted products, including short-chain fatty acids, equol, and exopolysaccharides.
The role of probiotics in osteoporosis is increasingly being studied. Activation of inflammatory factors associated with osteoporosis such as TNF-α, NF-κB, IL-1, IL-6, and IL-17 has been to be involved in the physiology of pro-osteoclast formation. Inhibition of the TNF-α/NF-κB signaling pathway prevented nuclear transfer of NF-κB and blocked the transcription of regulatory proteins associated with osteoclasts. Bifidobacterium secretions block the initiation of inflammation and inhibits osteoclast formation to improve osteoporosis symptoms. In addition, the Bifidobacterium secretions can regulate RANKL/RANK/OPG, the most important signaling pathway of osteoclasts, via the TNF-α/NF-κB signaling pathway, which block the synergistic effect of TNF-α on RANKL and reduce the binding of RANK to RANKL to regulate the formation of osteoclasts. In this way, it prevents bone loss caused by the bone resorption induced by osteoclasts. Although clinically effective osteoporosis treatment drugs are available, their safety and efficacy are not satisfactory, especially in patients with severe osteoporosis. Therefore, there is a need to actively search for more effective treatments with fewer side effects and more cost-effective. The data described in this review demonstrated that Bifidobacterium might be a good treatment agent.
Author contributions
YW and YY conceived the study and finished drafting the article. LW and YC polished up the article. XH, LS, and HC processed the table and pictures. QC provided guidance and resolved disagreements. All authors approved it for publication.
Funding
This work was supported by the project of construction and regional promotion and application of the intelligent management innovation system of TCM for metabolic diseases based on Internet of Things Technology (CKY2021088).
Acknowledgments
We are grateful to Bio Render for supplying the drawing platform. We thank Home for Researchers editorial team (www.home-for-researchers.com) for language editing service.
Conflict of interest
The authors declare that the research was conducted in the absence of any commercial or financial relationships that could be construed as a potential conflict of interest.
Publisher’s note
All claims expressed in this article are solely those of the authors and do not necessarily represent those of their affiliated organizations, or those of the publisher, the editors and the reviewers. Any product that may be evaluated in this article, or claim that may be made by its manufacturer, is not guaranteed or endorsed by the publisher.
References
1. Langdahl B, Ferrari S, Dempster DW. Bone modeling and remodeling: Potential as therapeutic targets for the treatment of osteoporosis. Ther Adv Musculoskelet Dis (2016) 8(6):225–35. doi: 10.1177/1759720X16670154
2. Cummings SR, Ferrari S, Eastell R, Gilchrist N, Jensen JB, McClung M, et al. Vertebral fractures after discontinuation of denosumab: A Post hoc analysis of the randomized placebo-controlled freedom trial and its extension. J Bone Miner Res (2018) 33(2):190–8. doi: 10.1002/jbmr.3337
3. Mosca L, Grady D, Barrett-Connor E, Collins P, Wenger N, Abramson BL, et al. Effect of raloxifene on stroke and venous thromboembolism according to subgroups in postmenopausal women at increased risk of coronary heart disease. Stroke (2009) 40(1):147–55. doi: 10.1161/STROKEAHA.108.518621
4. Schilcher J, Koeppen V, Aspenberg P, Michaëlsson K. Risk of atypical femoral fracture during and after bisphosphonate use. Acta Orthop (2015) 86(1):100–7. doi: 10.3109/17453674.2015.1004149
5. Fixen C, Tunoa J. Romosozumab: A review of efficacy, safety, and cardiovascular risk. Curr Osteoporos Rep (2021) 19(1):15–22. doi: 10.1007/s11914-020-00652-w
6. Xu XL, Gou WL, Wang AY, Wang Y, Guo QY, Lu Q, et al. Basic research and clinical applications of bisphosphonates in bone disease: What have we learned over the last 40 years? J Transl Med (2013) 11:303. doi: 10.1186/1479-5876-11-303
7. McClung M, Harris ST, Miller PD, Bauer DC, Davison KS, Dian L, et al. Bisphosphonate therapy for osteoporosis: Benefits, risks, and drug holiday. Am J Med (2013) 126(1):13–20. doi: 10.1016/j.amjmed.2012.06.023
8. Jha S, Wang Z, Laucis N, Bhattacharyya T. Trends in media reports, oral bisphosphonate prescriptions, and hip fractures 1996-2012: An ecological analysis. J Bone Miner Res (2015) 30(12):2179–87. doi: 10.1002/jbmr.2565
9. Langdahl BL. Overview of treatment approaches to osteoporosis. Br J Pharmacol (2021) 178(9):1891–906. doi: 10.1111/bph.15024
10. Gupta S, Onkar A, Vashisht T. Zoledronic acid induced unilateral anterior uveitis. Indian J Ophthalmol (2020) 68(9):2002–3. doi: 10.4103/ijo.IJO_1654_19
11. Abrahamsen B, Eiken P, Prieto-Alhambra D, Eastell R. Risk of hip, subtrochanteric, and femoral shaft fractures among mid and long term users of alendronate: Nationwide cohort and nested case-control study. BMJ (2016) 353:i3365. doi: 10.1136/bmj.i3365
12. Khan AA, Morrison A, Kendler DL, Rizzoli R, Hanley DA, Felsenberg D, et al. Case-based review of osteonecrosis of the jaw (Onj) and application of the international recommendations for management from the international task force on onj. J Clin Densitom (2017) 20(1):8–24. doi: 10.1016/j.jocd.2016.09.005
13. Miller PD, Chines AA, Christiansen C, Hoeck HC, Kendler DL, Lewiecki EM, et al. Effects of bazedoxifene on bmd and bone turnover in postmenopausal women: 2-yr results of a randomized, double-blind, placebo-, and active-controlled study. J Bone Miner Res (2008) 23(4):525–35. doi: 10.1359/jbmr.071206
14. Silverman SL, Christiansen C, Genant HK, Vukicevic S, Zanchetta JR, de Villiers TJ, et al. Efficacy of bazedoxifene in reducing new vertebral fracture risk in postmenopausal women with osteoporosis: Results from a 3-year, randomized, placebo-, and active-controlled clinical trial. J Bone Miner Res (2008) 23(12):1923–34. doi: 10.1359/jbmr.080710
15. Deeks ED. Denosumab: A review in postmenopausal osteoporosis. Drugs Aging (2018) 35(2):163–73. doi: 10.1007/s40266-018-0525-7
16. Cummings SR, San Martin J, McClung MR, Siris ES, Eastell R, Reid IR, et al. Denosumab for prevention of fractures in postmenopausal women with osteoporosis. N Engl J Med (2009) 361(8):756–65. doi: 10.1056/NEJMoa0809493
17. Saag KG, Shane E, Boonen S, Marín F, Donley DW, Taylor KA, et al. Teriparatide or alendronate in glucocorticoid-induced osteoporosis. N Engl J Med (2007) 357(20):2028–39. doi: 10.1056/NEJMoa071408
18. Blick SK, Dhillon S, Keam SJ. Teriparatide: A review of its use in osteoporosis. Drugs (2008) 68(18):2709–37. doi: 10.2165/0003495-200868180-00012
19. Cosman F, Crittenden DB, Adachi JD, Binkley N, Czerwinski E, Ferrari S, et al. Romosozumab treatment in postmenopausal women with osteoporosis. N Engl J Med (2016) 375(16):1532–43. doi: 10.1056/NEJMoa1607948
20. Hill C, Guarner F, Reid G, Gibson GR, Merenstein DJ, Pot B, et al. Expert consensus document. the international scientific association for probiotics and prebiotics consensus statement on the scope and appropriate use of the term probiotic. Nat Rev Gastroenterol Hepatol (2014) 11(8):506–14. doi: 10.1038/nrgastro.2014.66
21. Kijmanawat A, Panburana P, Reutrakul S, Tangshewinsirikul C. Effects of probiotic supplements on insulin resistance in gestational diabetes mellitus: A double-blind randomized controlled trial. J Diabetes Investig (2019) 10(1):163–70. doi: 10.1111/jdi.12863
22. Sato J, Kanazawa A, Azuma K, Ikeda F, Goto H, Komiya K, et al. Probiotic reduces bacterial translocation in type 2 diabetes mellitus: A randomised controlled study. Sci Rep (2017) 7(1):12115. doi: 10.1038/s41598-017-12535-9
23. Su X, Zhao Y, Li Y, Ma S, Wang Z. Gut dysbiosis is associated with primary hypothyroidism with interaction on gut-thyroid axis. Clin Sci (Lond) (2020) 134(12):1521–35. doi: 10.1042/cs20200475
24. Virili C, Fallahi P, Antonelli A, Benvenga S, Centanni M. Gut microbiota and hashimoto's thyroiditis. Rev Endocr Metab Disord (2018) 19(4):293–300. doi: 10.1007/s11154-018-9467-y
25. Collins FL, Rios-Arce ND, Schepper JD, Parameswaran N, McCabe LR. The potential of probiotics as a therapy for osteoporosis. Microbiol Spectr (2017) 5(4). doi: 10.1128/microbiolspec.BAD-0015-2016
26. Paccou J. Nutritional facets of osteoporosis management: Can probiotics help? Joint Bone Spine (2020) 87(2):115–7. doi: 10.1016/j.jbspin.2019.06.007
27. Sun P, Zhang C, Huang Y, Yang J, Zhou F, Zeng J, et al. Jiangu granule ameliorated ovx rats bone loss by modulating gut microbiota-Scfas-Treg/Th17 axis. BioMed Pharmacother (2022) 150:112975. doi: 10.1016/j.biopha.2022.112975
28. Knudsen JK, Leutscher P, Sørensen S. Gut microbiota in bone health and diabetes. Curr Osteoporos Rep (2021) 19(4):462–79. doi: 10.1007/s11914-020-00629-9
29. Salminen S, Ouwehand A, Benno Y, Lee YK. Probiotics: How should they be defined? Trends Food Sci Technol (1999) 10(3):107–10. doi: 10.1016/S0924-2244(99)00027-8
30. Jin LZ, Marquardt RR, Zhao X. A strain of enterococcus faecium (18c23) inhibits adhesion of enterotoxigenic escherichia coli K88 to porcine small intestine mucus. Appl Environ Microbiol (2000) 66(10):4200–4. doi: 10.1128/AEM.66.10.4200-4204.2000
31. Gibson GR, Roberfroid MB. Dietary modulation of the human colonic microbiota: Introducing the concept of prebiotics. J Nutr (1995) 125(6):1401–12. doi: 10.1093/jn/125.6.1401
32. Alvarez-Olmos MI, Oberhelman RA. Probiotic agents and infectious diseases: A modern perspective on a traditional therapy. Clin Infect Dis (2001) 32(11):1567–76. doi: 10.1086/320518
33. Didari T, Solki S, Mozaffari S, Nikfar S, Abdollahi M. A systematic review of the safety of probiotics. Expert Opin Drug Saf (2014) 13(2):227–39. doi: 10.1517/14740338.2014.872627
34. Redman MG, Ward EJ, Phillips RS. The efficacy and safety of probiotics in people with cancer: A systematic review. Ann Oncol (2014) 25(10):1919–29. doi: 10.1093/annonc/mdu106
35. Qu R, Chen X, Hu J, Fu Y, Peng J, Li Y, et al. Ghrelin protects against contact dermatitis and psoriasiform skin inflammation by antagonizing tnf-α/Nf-κb signaling pathways. Sci Rep (2019) 9(1):1348. doi: 10.1038/s41598-018-38174-2
36. Xing L, Bushnell TP, Carlson L, Tai Z, Tondravi M, Siebenlist U, et al. Nf-kappab P50 and P52 expression is not required for rank-expressing osteoclast progenitor formation but is essential for rank- and cytokine-mediated osteoclastogenesis. J Bone Miner Res (2002) 17(7):1200–10. doi: 10.1359/jbmr.2002.17.7.1200
37. Luo G, Li F, Li X, Wang ZG, Zhang B. Tnf−α and rankl promote osteoclastogenesis by upregulating rank Via the Nf−κb pathway. Mol Med Rep (2018) 17(5):6605–11. doi: 10.3892/mmr.2018.8698
38. Carswell EA, Old LJ, Kassel RL, Green S, Fiore N, Williamson B. An endotoxin-induced serum factor that causes necrosis of tumors. Proc Natl Acad Sci U.S.A. (1975) 72(9):3666–70. doi: 10.1073/pnas.72.9.3666
39. Baud V, Karin M. Signal transduction by tumor necrosis factor and its relatives. Trends Cell Biol (2001) 11(9):372–7. doi: 10.1016/s0962-8924(01)02064-5
40. Aggarwal BB. Signalling pathways of the tnf superfamily: A double-edged sword. Nat Rev Immunol (2003) 3(9):745–56. doi: 10.1038/nri1184
41. Lavrik I, Golks A, Krammer PH. Death receptor signaling. J Cell Sci (2005) 118(Pt 2):265–7. doi: 10.1242/jcs.01610
42. Brenner D, Blaser H, Mak TW. Regulation of tumour necrosis factor signalling: Live or let die. Nat Rev Immunol (2015) 15(6):362–74. doi: 10.1038/nri3834
43. Pomerantz JL, Baltimore D. Two pathways to nf-kappab. Mol Cell (2002) 10(4):693–5. doi: 10.1016/s1097-2765(02)00697-4
44. Hayden MS, Ghosh S. Signaling to nf-kappab. Genes Dev (2004) 18(18):2195–224. doi: 10.1101/gad.1228704
45. Oeckinghaus A, Ghosh S. The nf-kappab family of transcription factors and its regulation. Cold Spring Harb Perspect Biol (2009) 1(4):a000034. doi: 10.1101/cshperspect.a000034
46. Ghosh S, Karin M. Missing pieces in the nf-κb puzzle. Cell (2002) 109(2, Supplement 1):S81–96. doi: 10.1016/S0092-8674(02)00703-1
47. Karin M, Delhase M. The I kappa b kinase (Ikk) and nf-kappa b: Key elements of proinflammatory signalling. Semin Immunol (2000) 12(1):85–98. doi: 10.1006/smim.2000.0210
48. Yamashita T, Yao Z, Li F, Zhang Q, Badell IR, Schwarz EM, et al. Nf-kappab P50 and P52 regulate receptor activator of nf-kappab ligand (Rankl) and tumor necrosis factor-induced osteoclast precursor differentiation by activating c-fos and Nfatc1. J Biol Chem (2007) 282(25):18245–53. doi: 10.1074/jbc.M610701200
49. Shaulian E, Karin M. Ap-1 as a regulator of cell life and death. Nat Cell Biol (2002) 4(5):E131–6. doi: 10.1038/ncb0502-e131
50. Wang ZQ, Ovitt C, Grigoriadis AE, Möhle-Steinlein U, Rüther U, Wagner EF. Bone and haematopoietic defects in mice lacking c-fos. Nature (1992) 360(6406):741–5. doi: 10.1038/360741a0
51. Matsuo K, Galson DL, Zhao C, Peng L, Laplace C, Wang KZ, et al. Nuclear factor of activated T-cells (Nfat) rescues osteoclastogenesis in precursors lacking c-fos. J Biol Chem (2004) 279(25):26475–80. doi: 10.1074/jbc.M313973200
52. Boyce BF, Xing L. The Rankl/Rank/Opg pathway. Curr Osteoporos Rep (2007) 5(3):98–104. doi: 10.1007/s11914-007-0024-y
53. Dai XM, Ryan GR, Hapel AJ, Dominguez MG, Russell RG, Kapp S, et al. Targeted disruption of the mouse colony-stimulating factor 1 receptor gene results in osteopetrosis, mononuclear phagocyte deficiency, increased primitive progenitor cell frequencies, and reproductive defects. Blood (2002) 99(1):111–20. doi: 10.1182/blood.v99.1.111
54. Bartocci A, Mastrogiannis DS, Migliorati G, Stockert RJ, Wolkoff AW, Stanley ER. Macrophages specifically regulate the concentration of their own growth factor in the circulation. Proc Natl Acad Sci U.S.A. (1987) 84(17):6179–83. doi: 10.1073/pnas.84.17.6179
55. Wong BR, Josien R, Lee SY, Sauter B, Li HL, Steinman RM, et al. Trance (Tumor necrosis factor [Tnf]-related activation-induced cytokine), a new tnf family member predominantly expressed in T cells, is a dendritic cell-specific survival factor. J Exp Med (1997) 186(12):2075–80. doi: 10.1084/jem.186.12.2075
56. Yasuda H, Shima N, Nakagawa N, Yamaguchi K, Kinosaki M, Mochizuki S, et al. Osteoclast differentiation factor is a ligand for Osteoprotegerin/Osteoclastogenesis-inhibitory factor and is identical to Trance/Rankl. Proc Natl Acad Sci U.S.A. (1998) 95(7):3597–602. doi: 10.1073/pnas.95.7.3597
57. Burgess TL, Qian Y, Kaufman S, Ring BD, Van G, Capparelli C, et al. The ligand for osteoprotegerin (Opgl) directly activates mature osteoclasts. J Cell Biol (1999) 145(3):527–38. doi: 10.1083/jcb.145.3.527
58. Fuller K, Murphy C, Kirstein B, Fox SW, Chambers TJ. Tnfalpha potently activates osteoclasts, through a direct action independent of and strongly synergistic with rankl. Endocrinology (2002) 143(3):1108–18. doi: 10.1210/endo.143.3.8701
59. Lacey DL, Timms E, Tan HL, Kelley MJ, Dunstan CR, Burgess T, et al. Osteoprotegerin ligand is a cytokine that regulates osteoclast differentiation and activation. Cell (1998) 93(2):165–76. doi: 10.1016/s0092-8674(00)81569-x
60. Boyle WJ, Simonet WS, Lacey DL. Osteoclast differentiation and activation. Nature (2003) 423(6937):337–42. doi: 10.1038/nature01658
61. Suda T, Takahashi N, Udagawa N, Jimi E, Gillespie MT, Martin TJ. Modulation of osteoclast differentiation and function by the new members of the tumor necrosis factor receptor and ligand families. Endocr Rev (1999) 20(3):345–57. doi: 10.1210/edrv.20.3.0367
62. Arai F, Miyamoto T, Ohneda O, Inada T, Sudo T, Brasel K, et al. Commitment and differentiation of osteoclast precursor cells by the sequential expression of c-fms and receptor activator of nuclear factor kappab (Rank) receptors. J Exp Med (1999) 190(12):1741–54. doi: 10.1084/jem.190.12.1741
63. Simonet WS, Lacey DL, Dunstan CR, Kelley M, Chang MS, Lüthy R, et al. Osteoprotegerin: A novel secreted protein involved in the regulation of bone density. Cell (1997) 89(2):309–19. doi: 10.1016/s0092-8674(00)80209-3
64. Anderson DM, Maraskovsky E, Billingsley WL, Dougall WC, Tometsko ME, Roux ER, et al. A homologue of the tnf receptor and its ligand enhance T-cell growth and dendritic-cell function. Nature (1997) 390(6656):175–9. doi: 10.1038/36593
65. Zhao H, Liu X, Zou H, Dai N, Yao L, Gao Q, et al. Osteoprotegerin induces podosome disassembly in osteoclasts through calcium, erk, and P38 mapk signaling pathways. Cytokine (2015) 71(2):199–206. doi: 10.1016/j.cyto.2014.10.007
66. Li Z, Hou WS, Escalante-Torres CR, Gelb BD, Bromme D. Collagenase activity of cathepsin K depends on complex formation with chondroitin sulfate. J Biol Chem (2002) 277(32):28669–76. doi: 10.1074/jbc.M204004200
67. Zhao Y, Wang HL, Li TT, Yang F, Tzeng CM. Baicalin ameliorates dexamethasone-induced osteoporosis by regulation of the Rank/Rankl/Opg signaling pathway. Drug Des Devel Ther (2020) 14:195–206. doi: 10.2147/DDDT.S225516
68. Ishimi Y, Miyaura C, Jin CH, Akatsu T, Abe E, Nakamura Y, et al. Il-6 is produced by osteoblasts and induces bone resorption. J Immunol (1990) 145(10):3297–303. doi: 10.4049/jimmunol.145.10.3297
69. Pfeilschifter J, Chenu C, Bird A, Mundy GR, Roodman GD. Interleukin-1 and tumor necrosis factor stimulate the formation of human osteoclastlike cells in vitro. J Bone Miner Res (1989) 4(1):113–8. doi: 10.1002/jbmr.5650040116
70. Tanaka T, Narazaki M, Kishimoto T. Il-6 in inflammation, immunity, and disease. Cold Spring Harb Perspect Biol (2014) 6(10):a016295. doi: 10.1101/cshperspect.a016295
71. Dinarello CA, Cannon JG, Wolff SM, Bernheim HA, Beutler B, Cerami A, et al. Tumor necrosis factor (Cachectin) is an endogenous pyrogen and induces production of interleukin 1. J Exp Med (1986) 163(6):1433–50. doi: 10.1084/jem.163.6.1433
72. Zelová H, Hošek J. Tnf-α signalling and inflammation: Interactions between old acquaintances. Inflammation Res (2013) 62(7):641–51. doi: 10.1007/s00011-013-0633-0
73. Taniguchi T, Matsuzaki N, Jo T, Saji F, Taga T, Hirano T, et al. Interleukin-1 (Il-1)-Induced il-6- and il-6-Receptor-Mediated release of human chorionic gonadotropin by choriocarcinoma cell lines (Jar and hccm-5) activates adenosine 3',5'-Monophosphate-Independent signal transduction pathway. J Clin Endocrinol Metab (1992) 74(6):1389–95. doi: 10.1210/jcem.74.6.1317386
74. Pietschmann P, Mechtcheriakova D, Meshcheryakova A, Föger-Samwald U, Ellinger I. Immunology of osteoporosis: A mini-review. Gerontology (2016) 62(2):128–37. doi: 10.1159/000431091
75. Lee Y. The role of interleukin-17 in bone metabolism and inflammatory skeletal diseases. BMB Rep (2013) 46(10):479–83. doi: 10.5483/BMBRep.2013.46.10.141
76. Hashizume M, Hayakawa N, Mihara M. Il-6 trans-signalling directly induces rankl on fibroblast-like synovial cells and is involved in rankl induction by tnf-alpha and il-17. Rheumatol (Oxford) (2008) 47(11):1635–40. doi: 10.1093/rheumatology/ken363
77. De Simone V, Franzè E, Ronchetti G, Colantoni A, Fantini MC, Di Fusco D, et al. Th17-type cytokines, il-6 and tnf-α synergistically activate Stat3 and nf-kb to promote colorectal cancer cell growth. Oncogene (2015) 34(27):3493–503. doi: 10.1038/onc.2014.286
78. Ciucci T, Ibáñez L, Boucoiran A, Birgy-Barelli E, Pène J, Abou-Ezzi G, et al. Bone marrow Th17 tnfα cells induce osteoclast differentiation, and link bone destruction to ibd. Gut (2015) 64(7):1072–81. doi: 10.1136/gutjnl-2014-306947
79. Zou W, Hakim I, Tschoep K, Endres S, Bar-Shavit Z. Tumor necrosis factor-alpha mediates rank ligand stimulation of osteoclast differentiation by an autocrine mechanism. J Cell Biochem (2001) 83(1):70–83. doi: 10.1002/jcb.1202
80. Zeng XZ, Zhang YY, Yang Q, Wang S, Zou BH, Tan YH, et al. Artesunate attenuates lps-induced osteoclastogenesis by suppressing Tlr4/Traf6 and Plcγ1-Ca(2+)-Nfatc1 signaling pathway. Acta Pharmacol Sin (2020) 41(2):229–36. doi: 10.1038/s41401-019-0289-6
81. Wu L, Luo Z, Liu Y, Jia L, Jiang Y, Du J, et al. Aspirin inhibits rankl-induced osteoclast differentiation in dendritic cells by suppressing nf-κb and Nfatc1 activation. Stem Cell Res Ther (2019) 10(1):375. doi: 10.1186/s13287-019-1500-x
82. Hoesel B, Schmid JA. The complexity of nf-κb signaling in inflammation and cancer. Mol Cancer (2013) 12:86. doi: 10.1186/1476-4598-12-86
83. DiDonato JA, Mercurio F, Karin M. Nf-κb and the link between inflammation and cancer. Immunol Rev (2012) 246(1):379–400. doi: 10.1111/j.1600-065X.2012.01099.x
84. Kim MH, Lee H, Ha IJ, Yang WM. Zanthoxylum piperitum alleviates the bone loss in osteoporosis Via inhibition of rankl-induced c-Fos/Nfatc1/Nf-κb pathway. Phytomedicine (2021) 80:153397. doi: 10.1016/j.phymed.2020.153397
85. Kim JM, Lee JH, Lee GS, Noh EM, Song HK, Gu DR, et al. Sophorae flos extract inhibits rankl-induced osteoclast differentiation by suppressing the nf-κb/Nfatc1 pathway in mouse bone marrow cells. BMC Complement Altern Med (2017) 17(1):164. doi: 10.1186/s12906-016-1550-x
86. Hong G, Zhou L, Shi X, He W, Wang H, Wei Q, et al. Bajijiasu abrogates osteoclast differentiation via the suppression of rankl signaling pathways through nf-κb and nfat. Int J Mol Sci (2017) 18(1). doi: 10.3390/ijms18010203
87. Garcia VG, Knoll LR, Longo M, Novaes VC, Assem NZ, Ervolino E, et al. Effect of the probiotic saccharomyces cerevisiae on ligature-induced periodontitis in rats. J Periodontal Res (2016) 51(1):26–37. doi: 10.1111/jre.12274
88. Yao Z, Getting SJ, Locke IC. Regulation of tnf-induced osteoclast differentiation. Cells (2021) 11(1). doi: 10.3390/cells11010132
89. Boyce BF, Xing L. Functions of Rankl/Rank/Opg in bone modeling and remodeling. Arch Biochem Biophys (2008) 473(2):139–46. doi: 10.1016/j.abb.2008.03.018
90. Hofbauer LC, Schoppet M. Clinical implications of the Osteoprotegerin/Rankl/Rank system for bone and vascular diseases. JAMA (2004) 292(4):490–5. doi: 10.1001/jama.292.4.490
91. Li JY, Chassaing B, Tyagi AM, Vaccaro C, Luo T, Adams J, et al. Sex steroid deficiency-associated bone loss is microbiota dependent and prevented by probiotics. J Clin Invest (2016) 126(6):2049–63. doi: 10.1172/JCI86062
92. Kwon Y, Park C, Lee J, Park DH, Jeong S, Yun CH, et al. Regulation of bone cell differentiation and activation by microbe-associated molecular patterns. Int J Mol Sci (2021) 22(11). doi: 10.3390/ijms22115805
93. Sapra L, Shokeen N, Porwal K, Saini C, Bhardwaj A, Mathew M, et al. Bifidobacterium longum ameliorates ovariectomy-induced bone loss Via enhancing anti-osteoclastogenic and immunomodulatory potential of regulatory b cells (Bregs). Front Immunol (2022) 13:875788. doi: 10.3389/fimmu.2022.875788
94. Ohlsson C, Engdahl C, Fåk F, Andersson A, Windahl SH, Farman HH, et al. Probiotics protect mice from ovariectomy-induced cortical bone loss. PloS One (2014) 9(3):e92368. doi: 10.1371/journal.pone.0092368
95. McClung MR, Pinkerton JV, Blake J, Cosman FA, Lewiecki M, Shapiro M, et al. Management of osteoporosis in postmenopausal women: The 2021 position statement of the north American menopause society. Menopause (2021) 28(9):973–97. doi: 10.1097/gme.0000000000001831
96. Lambert MNT, Thybo CB, Lykkeboe S, Rasmussen LM, Frette X, Christensen LP, et al. Combined bioavailable isoflavones and probiotics improve bone status and estrogen metabolism in postmenopausal osteopenic women: A randomized controlled trial. Am J Clin Nutr (2017) 106(3):909–20. doi: 10.3945/ajcn.117.153353
97. Takimoto T, Hatanaka M, Hoshino T, Takara T, Tanaka K, Shimizu A, et al. Effect of bacillus subtilis c-3102 on bone mineral density in healthy postmenopausal Japanese women: A randomized, placebo-controlled, double-blind clinical trial. Biosci Microbiota Food Health (2018) 37(4):87–96. doi: 10.12938/bmfh.18-006
98. Kim DE, Kim JK, Han SK, Jang SE, Han MJ, Kim DH. Lactobacillus plantarum Nk3 and bifidobacterium longum Nk49 alleviate bacterial vaginosis and osteoporosis in mice by suppressing nf-κb-Linked tnf-α expression. J Med Food (2019) 22(10):1022–31. doi: 10.1089/jmf.2019.4419
99. Rodrigues FC, Castro AS, Rodrigues VC, Fernandes SA, Fontes EA, de Oliveira TT, et al. Yacon flour and bifidobacterium longum modulate bone health in rats. J Med Food (2012) 15(7):664–70. doi: 10.1089/jmf.2011.0296
100. Ricoldi MST, Furlaneto FAC, Oliveira LFF, Teixeira GC, Pischiotini JP, Moreira ALG, et al. Effects of the probiotic bifidobacterium animalis subsp. lactis on the non-surgical treatment of periodontitis. a histomorphometric, microtomographic and immunohistochemical study in rats. PloS One (2017) 12(6):e0179946. doi: 10.1371/journal.pone.0179946
101. Parvaneh K, Ebrahimi M, Sabran MR, Karimi G, Hwei AN, Abdul-Majeed S, et al. Probiotics (Bifidobacterium longum) increase bone mass density and upregulate sparc and bmp-2 genes in rats with bone loss resulting from ovariectomy. BioMed Res Int (2015) 2015:897639. doi: 10.1155/2015/897639
102. Tomofuji T, Ekuni D, Azuma T, Irie K, Endo Y, Yamamoto T, et al. Supplementation of broccoli or bifidobacterium longum-fermented broccoli suppresses serum lipid peroxidation and osteoclast differentiation on alveolar bone surface in rats fed a high-cholesterol diet. Nutr Res (2012) 32(4):301–7. doi: 10.1016/j.nutres.2012.03.006
103. Oliveira LF, Salvador SL, Silva PH, Furlaneto FA, Figueiredo L, Casarin R, et al. Benefits of bifidobacterium animalis subsp. lactis probiotic in experimental periodontitis. J Periodontol (2017) 88(2):197–208. doi: 10.1902/jop.2016.160217
104. Roberts JL, Liu G, Darby TM, Fernandes LM, Diaz-Hernandez ME, Jones RM, et al. Bifidobacterium adolescentis supplementation attenuates fracture-induced systemic sequelae. BioMed Pharmacother (2020) 132:110831. doi: 10.1016/j.biopha.2020.110831
105. Hidalgo-Cantabrana C, Delgado S, Ruiz L, Ruas-Madiedo P, Sánchez B, Margolles A. Bifidobacteria and their health-promoting effects. Microbiol Spectr (2017) 5(3). doi: 10.1128/microbiolspec.BAD-0010-2016
106. Rinninella E, Raoul P, Cintoni M, Franceschi F, Miggiano GAD, Gasbarrini A, et al. What is the healthy gut microbiota composition? a changing ecosystem across age, environment, diet, and diseases. Microorganisms (2019) 7(1). doi: 10.3390/microorganisms7010014
107. Arumugam M, Raes J, Pelletier E, Le Paslier D, Yamada T, Mende DR, et al. Enterotypes of the human gut microbiome. Nature (2011) 473(7346):174–80. doi: 10.1038/nature09944
108. Binda C, Lopetuso LR, Rizzatti G, Gibiino G, Cennamo V, Gasbarrini A. Actinobacteria: A relevant minority for the maintenance of gut homeostasis. Dig Liver Dis (2018) 50(5):421–8. doi: 10.1016/j.dld.2018.02.012
109. Oyetayo VO, Oyetayo FL. Potential of probiotics as biotherapeutic agents targeting the innate immune system. Afr J Biotechnol (2005) 4(2):123–7.
110. Tsukuda N, Yahagi K, Hara T, Watanabe Y, Matsumoto H, Mori H, et al. Key bacterial taxa and metabolic pathways affecting gut short-chain fatty acid profiles in early life. ISME J (2021) 15(9):2574–90. doi: 10.1038/s41396-021-00937-7
111. Setchell KD, Clerici C. Equol: History, chemistry, and formation. J Nutr (2010) 140(7):1355S–62S. doi: 10.3945/jn.109.119776
112. López P, Monteserín DC, Gueimonde M, de los Reyes-Gavilán CG, Margolles A, Suárez A, et al. Exopolysaccharide-producing bifidobacterium strains elicit different in vitro responses upon interaction with human cells. Food Res Int (2012) 46(1):99–107. doi: 10.1016/j.foodres.2011.11.020
113. Yao Y, Cai X, Fei W, Ye Y, Zhao M, Zheng C. The role of short-chain fatty acids in immunity, inflammation and metabolism. Crit Rev Food Sci Nutr (2022) 62(1):1–12. doi: 10.1080/10408398.2020.1854675
114. Sivaprakasam S, Prasad PD, Singh N. Benefits of short-chain fatty acids and their receptors in inflammation and carcinogenesis. Pharmacol Ther (2016) 164:144–51. doi: 10.1016/j.pharmthera.2016.04.007
115. Belenguer A, Duncan SH, Calder AG, Holtrop G, Louis P, Lobley GE, et al. Two routes of metabolic cross-feeding between bifidobacterium adolescentis and butyrate-producing anaerobes from the human gut. Appl Environ Microbiol (2006) 72(5):3593–9. doi: 10.1128/AEM.72.5.3593-3599.2006
116. Liu T, Li J, Liu Y, Xiao N, Suo H, Xie K, et al. Short-chain fatty acids suppress lipopolysaccharide-induced production of nitric oxide and proinflammatory cytokines through inhibition of nf-κb pathway in Raw264.7 cells. Inflammation (2012) 35(5):1676–84. doi: 10.1007/s10753-012-9484-z
117. Zapolska-Downar D, Siennicka A, Kaczmarczyk M, Kołodziej B, Naruszewicz M. Butyrate inhibits cytokine-induced vcam-1 and icam-1 expression in cultured endothelial cells: The role of nf-kappab and pparalpha. J Nutr Biochem (2004) 15(4):220–8. doi: 10.1016/j.jnutbio.2003.11.008
118. Tedelind S, Westberg F, Kjerrulf M, Vidal A. Anti-inflammatory properties of the short-chain fatty acids acetate and propionate: A study with relevance to inflammatory bowel disease. World J Gastroenterol (2007) 13(20):2826–32. doi: 10.3748/wjg.v13.i20.2826
119. Park JW, Kim HY, Kim MG, Jeong S, Yun CH, Han SH. Short-chain fatty acids inhibit staphylococcal lipoprotein-induced nitric oxide production in murine macrophages. Immune Netw (2019) 19(2):e9. doi: 10.4110/in.2019.19.e9
120. Smith PM, Howitt MR, Panikov N, Michaud M, Gallini CA, Bohlooly YM, et al. The microbial metabolites, short-chain fatty acids, regulate colonic treg cell homeostasis. Science (2013) 341(6145):569–73. doi: 10.1126/science.1241165
121. Ali N, Rosenblum MD. Regulatory T cells in skin. Immunology (2017) 152(3):372–81. doi: 10.1111/imm.12791
122. Zeng G, Jin L, Ying Q, Chen H, Thembinkosi MC, Yang C, et al. Regulatory T cells in cancer immunotherapy: Basic research outcomes and clinical directions. Cancer Manag Res (2020) 12:10411–21. doi: 10.2147/CMAR.S265828
123. Rubtsov YP, Rasmussen JP, Chi EY, Fontenot J, Castelli L, Ye X, et al. Regulatory T cell-derived interleukin-10 limits inflammation at environmental interfaces. Immunity (2008) 28(4):546–58. doi: 10.1016/j.immuni.2008.02.017
124. Takayanagi H. New immune connections in osteoclast formation. Ann N Y Acad Sci (2010) 1192:117–23. doi: 10.1111/j.1749-6632.2009.05303.x
125. Bogdan C, Vodovotz Y, Nathan C. Macrophage deactivation by interleukin 10. J Exp Med (1991) 174(6):1549–55. doi: 10.1084/jem.174.6.1549
126. Zaiss MM, Axmann R, Zwerina J, Polzer K, Gückel E, Skapenko A, et al. Treg cells suppress osteoclast formation: A new link between the immune system and bone. Arthritis Rheum (2007) 56(12):4104–12. doi: 10.1002/art.23138
127. Zaiss MM, Sarter K, Hess A, Engelke K, Böhm C, Nimmerjahn F, et al. Increased bone density and resistance to ovariectomy-induced bone loss in Foxp3-transgenic mice based on impaired osteoclast differentiation. Arthritis Rheum (2010) 62(8):2328–38. doi: 10.1002/art.27535
128. Yan J, Takakura A, Zandi-Nejad K, Charles JF. Mechanisms of gut microbiota-mediated bone remodeling. Gut Microbes (2018) 9(1):84–92. doi: 10.1080/19490976.2017.1371893
129. Schmitz H, Fromm M, Bentzel CJ, Scholz P, Detjen K, Mankertz J, et al. Tumor necrosis factor-alpha (Tnfalpha) regulates the epithelial barrier in the human intestinal cell line ht-29/B6. J Cell Sci (1999) 112(Pt 1):137–46. doi: 10.1242/jcs.112.1.137
130. Zihni C, Mills C, Matter K, Balda MS. Tight junctions: From simple barriers to multifunctional molecular gates. Nat Rev Mol Cell Biol (2016) 17(9):564–80. doi: 10.1038/nrm.2016.80
131. Ma X, Fan PX, Li LS, Qiao SY, Zhang GL, Li DF. Butyrate promotes the recovering of intestinal wound healing through its positive effect on the tight junctions. J Anim Sci (2012) 90 Suppl 4:266–8. doi: 10.2527/jas.50965
132. Saitou M, Furuse M, Sasaki H, Schulzke JD, Fromm M, Takano H, et al. Complex phenotype of mice lacking occludin, a component of tight junction strands. Mol Biol Cell (2000) 11(12):4131–42. doi: 10.1091/mbc.11.12.4131
133. Suzuki T. Regulation of intestinal epithelial permeability by tight junctions. Cell Mol Life Sci (2013) 70(4):631–59. doi: 10.1007/s00018-012-1070-x
134. Diao H, Jiao AR, Yu B, Mao XB, Chen DW. Gastric infusion of short-chain fatty acids can improve intestinal barrier function in weaned piglets. Genes Nutr (2019) 14:4. doi: 10.1186/s12263-019-0626-x
135. Ichikawa H, Shineha R, Satomi S, Sakata T. Gastric or rectal instillation of short-chain fatty acids stimulates epithelial cell proliferation of small and Large intestine in rats. Dig Dis Sci (2002) 47(5):1141–6. doi: 10.1023/a:1015014829605
136. Katono T, Kawato T, Tanabe N, Suzuki N, Iida T, Morozumi A, et al. Sodium butyrate stimulates mineralized nodule formation and osteoprotegerin expression by human osteoblasts. Arch Oral Biol (2008) 53(10):903–9. doi: 10.1016/j.archoralbio.2008.02.016
137. Chang MC, Tsai YL, Liou EJ, Tang CM, Wang TM, Liu HC, et al. Effect of butyrate on collagen expression, cell viability, cell cycle progression and related proteins expression of mg-63 osteoblastic cells. PloS One (2016) 11(11):e0165438. doi: 10.1371/journal.pone.0165438
138. Rahman MM, Kukita A, Kukita T, Shobuike T, Nakamura T, Kohashi O. Two histone deacetylase inhibitors, trichostatin a and sodium butyrate, suppress differentiation into osteoclasts but not into macrophages. Blood (2003) 101(9):3451–9. doi: 10.1182/blood-2002-08-2622
139. Lucas S, Omata Y, Hofmann J, Böttcher M, Iljazovic A, Sarter K, et al. Short-chain fatty acids regulate systemic bone mass and protect from pathological bone loss. Nat Commun (2018) 9(1):55. doi: 10.1038/s41467-017-02490-4
140. Marrian GF, Haslewood GA. Equol, a new inactive phenol isolated from the ketohydroxyoestrin fraction of mares' urine. Biochem J (1932) 26(4):1227–32. doi: 10.1042/bj0261227
141. Setchell KD, Clerici C, Lephart ED, Cole SJ, Heenan C, Castellani D, et al. S-equol, a potent ligand for estrogen receptor beta, is the exclusive enantiomeric form of the soy isoflavone metabolite produced by human intestinal bacterial flora. Am J Clin Nutr (2005) 81(5):1072–9. doi: 10.1093/ajcn/81.5.1072
142. Schmitt E, Dekant W, Stopper H. Assaying the estrogenicity of phytoestrogens in cells of different estrogen sensitive tissues. Toxicol In Vitro (2001) 15(4-5):433–9. doi: 10.1016/s0887-2333(01)00048-0
143. Ishimi Y. Soybean isoflavones in bone health. Forum Nutr (2009) 61:104–16. doi: 10.1159/000212743
144. Setchell KD, Brown NM, Lydeking-Olsen E. The clinical importance of the metabolite equol-a clue to the effectiveness of soy and its isoflavones. J Nutr (2002) 132(12):3577–84. doi: 10.1093/jn/132.12.3577
145. Lu C, Gao R, Zhang Y, Jiang N, Chen Y, Sun J, et al. S-equol, a metabolite of dietary soy isoflavones, alleviates lipopolysaccharide-induced depressive-like behavior in mice by inhibiting neuroinflammation and enhancing synaptic plasticity. Food Funct (2021) 12(13):5770–8. doi: 10.1039/d1fo00547b
146. Kang JS, Yoon YD, Han MH, Han SB, Lee K, Kang MR, et al. Estrogen receptor-independent inhibition of tumor necrosis factor-alpha gene expression by phytoestrogen equol is mediated by blocking nuclear factor-kappab activation in mouse macrophages. Biochem Pharmacol (2005) 71(1-2):136–43. doi: 10.1016/j.bcp.2005.10.009
147. Shi J, Ji A, Cao Z, Cao R, Li D, Yang R, et al. [Equol induced apoptosis of human breast cancer mda-Mb-231 cell by inhibiting the expression of nuclear factor-kappab]. Wei Sheng Yan Jiu (2011) 40(1):95–8. Available at: https://pubmed.ncbi.nlm.nih.gov/21434324/.
148. Subedi L, Ji E, Shin D, Jin J, Yeo JH, Kim SY. Equol, a dietary daidzein gut metabolite attenuates microglial activation and potentiates neuroprotection in vitro. Nutrients (2017) 9(3). doi: 10.3390/nu9030207
149. Fujioka M, Uehara M, Wu J, Adlercreutz H, Suzuki K, Kanazawa K, et al. Equol, a metabolite of daidzein, inhibits bone loss in ovariectomized mice. J Nutr (2004) 134(10):2623–7. doi: 10.1093/jn/134.10.2623
150. Ohtomo T, Uehara M, Peñalvo JL, Adlercreutz H, Katsumata S, Suzuki K, et al. Comparative activities of daidzein metabolites, equol and O-desmethylangolensin, on bone mineral density and lipid metabolism in ovariectomized mice and in osteoclast cell cultures. Eur J Nutr (2008) 47(5):273–9. doi: 10.1007/s00394-008-0723-x
151. Kolios L, Sehmisch S, Daub F, Rack T, Tezval M, Stuermer KM, et al. Equol but not genistein improves early metaphyseal fracture healing in osteoporotic rats. Planta Med (2009) 75(5):459–65. doi: 10.1055/s-0029-1185380
152. Lin IC, Yamashita S, Murata M, Kumazoe M, Tachibana H. Equol suppresses inflammatory response and bone erosion due to rheumatoid arthritis in mice. J Nutr Biochem (2016) 32:101–6. doi: 10.1016/j.jnutbio.2016.02.012
153. Wu B. Opg/Rank/Rankl signal transduction pathway in the mechanism of equol on postmenopausal osteoporosis [master]. Third Military Medical University (2015).
154. Wallimann A, Hildebrand M, Groeger D, Stanic B, Akdis CA, Zeiter S, et al. An exopolysaccharide produced by bifidobacterium longum 35624® inhibits osteoclast formation Via a Tlr2-dependent mechanism. Calcif Tissue Int (2021) 108(5):654–66. doi: 10.1007/s00223-020-00790-4
155. Hidalgo-Cantabrana C, López P, Gueimonde M, de Los Reyes-Gavilán CG, Suárez A, Margolles A, et al. Immune modulation capability of exopolysaccharides synthesised by lactic acid bacteria and bifidobacteria. Probiotics Antimicrob Proteins (2012) 4(4):227–37. doi: 10.1007/s12602-012-9110-2
156. Schiavi E, Gleinser M, Molloy E, Groeger D, Frei R, Ferstl R, et al. The surface-associated exopolysaccharide of bifidobacterium longum 35624 plays an essential role in dampening host proinflammatory responses and repressing local Th17 responses. Appl Environ Microbiol (2016) 82(24):7185–96. doi: 10.1128/AEM.02238-16
157. Velasco CR, Baud'huin M, Sinquin C, Maillasson M, Heymann D, Colliec-Jouault S, et al. Effects of a sulfated exopolysaccharide produced by altermonas infernus on bone biology. Glycobiology (2011) 21(6):781–95. doi: 10.1093/glycob/cwr002
158. Cummings SR, Melton LJ. Epidemiology and outcomes of osteoporotic fractures. Lancet (2002) 359(9319):1761–7. doi: 10.1016/s0140-6736(02)08657-9
159. Jafarnejad S, Djafarian K, Fazeli MR, Yekaninejad MS, Rostamian A, Keshavarz SA. Effects of a multispecies probiotic supplement on bone health in osteopenic postmenopausal women: A randomized, double-blind, controlled trial. J Am Coll Nutr (2017) 36(7):497–506. doi: 10.1080/07315724.2017.1318724
160. Arpaia N, Campbell C, Fan X, Dikiy S, van der Veeken J, deRoos P, et al. Metabolites produced by commensal bacteria promote peripheral regulatory T-cell generation. Nature (2013) 504(7480):451–5. doi: 10.1038/nature12726
161. Komatsu N, Okamoto K, Sawa S, Nakashima T, Oh-hora M, Kodama T, et al. Pathogenic conversion of Foxp3+ T cells into Th17 cells in autoimmune arthritis. Nat Med (2014) 20(1):62–8. doi: 10.1038/nm.3432
162. Schmidt T, Schwinge D, Rolvien T, Jeschke A, Schmidt C, Neven M, et al. Th17 cell frequency is associated with low bone mass in primary sclerosing cholangitis. J Hepatol (2019) 70(5):941–53. doi: 10.1016/j.jhep.2018.12.035
163. Polanczyk MJ, Carson BD, Subramanian S, Afentoulis M, Vandenbark AA, Ziegler SF, et al. Cutting edge: Estrogen drives expansion of the Cd4+Cd25+ regulatory T cell compartment. J Immunol (2004) 173(4):2227–30. doi: 10.4049/jimmunol.173.4.2227
164. Sato K, Suematsu A, Okamoto K, Yamaguchi A, Morishita Y, Kadono Y, et al. Th17 functions as an osteoclastogenic helper T cell subset that links T cell activation and bone destruction. J Exp Med (2006) 203(12):2673–82. doi: 10.1084/jem.20061775
165. Pacifici R. Role of T cells in ovariectomy induced bone loss–revisited. J Bone Miner Res (2012) 27(2):231–9. doi: 10.1002/jbmr.1500
166. Luo CY, Wang L, Sun C, Li DJ. Estrogen enhances the functions of Cd4(+)Cd25(+)Foxp3(+) regulatory T cells that suppress osteoclast differentiation and bone resorption in vitro. Cell Mol Immunol (2011) 8(1):50–8. doi: 10.1038/cmi.2010.54
167. Zhang M, Zhou Q, Dorfman RG, Huang X, Fan T, Zhang H, et al. Butyrate inhibits interleukin-17 and generates tregs to ameliorate colorectal colitis in rats. BMC Gastroenterol (2016) 16(1):84. doi: 10.1186/s12876-016-0500-x
168. Morozumi A. High concentration of sodium butyrate suppresses osteoblastic differentiation and mineralized nodule formation in Ros17/2.8 cells. J Oral Sci (2011) 53(4):509–16. doi: 10.2334/josnusd.53.509
169. Tyagi AM, Yu M, Darby TM, Vaccaro C, Li JY, Owens JA, et al. The microbial metabolite butyrate stimulates bone formation Via T regulatory cell-mediated regulation of Wnt10b expression. Immunity (2018) 49(6):1116–31 e7. doi: 10.1016/j.immuni.2018.10.013
170. Bandara M, Arun SJ, Allanson M, Widyarini S, Chai Z, Reeve VE. Topical isoflavonoids reduce experimental cutaneous inflammation in mice. Immunol Cell Biol (2010) 88(7):727–33. doi: 10.1038/icb.2010.26
171. Wang J, Xu J, Wang B, Shu FR, Chen K, Mi MT. Equol promotes rat osteoblast proliferation and differentiation through activating estrogen receptor. Genet Mol Res (2014) 13(3):5055–63. doi: 10.4238/2014.July.4.21
172. Rowland IR, Wiseman H, Sanders TA, Adlercreutz H, Bowey EA. Interindividual variation in metabolism of soy isoflavones and lignans: Influence of habitual diet on equol production by the gut microflora. Nutr Cancer (2000) 36(1):27–32. doi: 10.1207/s15327914nc3601_5
173. Chen J, Chen X, Ho CL. Recent development of probiotic bifidobacteria for treating human diseases. Front Bioeng Biotechnol (2021) 9:770248. doi: 10.3389/fbioe.2021.770248
174. Wang Q, Xu J, Sun Z, Luan Y, Li Y, Wang J, et al. Engineering an in vivo ep-bifido pathway in escherichia coli for high-yield acetyl-coa generation with low Co(2) emission. Metab Eng (2019) 51:79–87. doi: 10.1016/j.ymben.2018.08.003
175. Verhaar BJH, Hendriksen HMA, de Leeuw FA, Doorduijn AS, van Leeuwenstijn M, Teunissen CE, et al. Gut microbiota composition is related to ad pathology. Front Immunol (2021) 12:794519. doi: 10.3389/fimmu.2021.794519
176. Hatheway CL. Toxigenic clostridia. Clin Microbiol Rev (1990) 3(1):66–98. doi: 10.1128/CMR.3.1.66
177. Kitajima H, Sumida Y, Tanaka R, Yuki N, Takayama H, Fujimura M. Early administration of bifidobacterium breve to preterm infants: Randomised controlled trial. Arch Dis Child Fetal Neonatal Ed (1997) 76(2):F101–7. doi: 10.1136/fn.76.2.F101
178. Ohishi A, Takahashi S, Ito Y, Ohishi Y, Tsukamoto K, Nanba Y, et al. Bifidobacterium septicemia associated with postoperative probiotic therapy in a neonate with omphalocele. J Pediatr (2010) 156(4):679–81. doi: 10.1016/j.jpeds.2009.11.041
179. van Hogezand RA, Hamdy NA. Skeletal morbidity in inflammatory bowel disease. Scand J Gastroenterol Suppl (2006) 243):59–64. doi: 10.1080/00365520600664276
180. Lan H, Liu WH, Zheng H, Feng H, Zhao W, Hung WL, et al. Bifidobacterium lactis bl-99 protects mice with osteoporosis caused by colitis Via gut inflammation and gut microbiota regulation. Food Funct (2022) 13(3):1482–94. doi: 10.1039/d1fo02218k
181. Ghouri YA, Richards DM, Rahimi EF, Krill JT, Jelinek KA, DuPont AW. Systematic review of randomized controlled trials of probiotics, prebiotics, and synbiotics in inflammatory bowel disease. Clin Exp Gastroenterol (2014) 7:473–87. doi: 10.18632/aging.103290
182. Ma S, Qin J, Hao Y, Shi Y, Fu L. Structural and functional changes of gut microbiota in ovariectomized rats and their correlations with altered bone mass. Aging (Albany NY) (2020) 12(11):10736–53. doi: 10.18632/aging.103290
183. Wang J, Wang Y, Gao W, Wang B, Zhao H, Zeng Y, et al. Diversity analysis of gut microbiota in osteoporosis and osteopenia patients. PeerJ (2017) 5:e3450. doi: 10.7717/peerj.3450
Keywords: Bifidobacterium, TNF-α, NF-κB, inflammation, osteoclast
Citation: Wu Y, Yang Y, Wang L, Chen Y, Han X, Sun L, Chen H and Chen Q (2023) Effect of Bifidobacterium on osteoclasts: TNF-α/NF-κB inflammatory signal pathway-mediated mechanism. Front. Endocrinol. 14:1109296. doi: 10.3389/fendo.2023.1109296
Received: 27 November 2022; Accepted: 14 February 2023;
Published: 09 March 2023.
Edited by:
Abdul Malik Tyagi, Central Drug Research Institute (CSIR), IndiaReviewed by:
Rupesh K. Srivastava, All India Institute of Medical Sciences, IndiaFarhath Sultana, Icahn School of Medicine at Mount Sinai, United States
Copyright © 2023 Wu, Yang, Wang, Chen, Han, Sun, Chen and Chen. This is an open-access article distributed under the terms of the Creative Commons Attribution License (CC BY). The use, distribution or reproduction in other forums is permitted, provided the original author(s) and the copyright owner(s) are credited and that the original publication in this journal is cited, in accordance with accepted academic practice. No use, distribution or reproduction is permitted which does not comply with these terms.
*Correspondence: Qiu Chen, Y2hlbnFpdTEwMDVAY2R1dGNtLmVkdS5jbg==
†These authors contributed equally to this work and share first authorship