- 1Department of Environmental Health Research, Institute of Research and Medical Consultations Imam Abdulrahman Bin Faisal University, Dammam, Saudi Arabia
- 2UH Cleveland Medical Center, Case Western Reserve University, Cleveland, OH, United States
- 3Department of Biology, College of Science, Imam Abdulrahman Bin Faisal University, Dammam, Saudi Arabia
The timing of food intake is a key cue for circadian rhythms in humans and animals. In response to food intake, gut hormones called incretin are produced by intestinal enteroendocrine cells in a circadian rhythm that stimulates insulin secretion and regulates body weight and energy expenditure. Pregnancy is associated with the expansion of β cells, the risk of gestational diabetes mellitus, and excessive weight gain. The timing of food intake is a good way to address metabolic complications during pregnancy. The current review focuses on the circadian rhythms and biological actions of enteroendocrine hormones and their associations with pregnancy status, specifically topics like food intake and gut circadian rhythms, the circadian secretion of enteroendocrine peptides, and the effects of these factors during pregnancy.
Introduction
Endogenously developed rhythms that take place over a period of almost 24 h are known as circadian rhythms. These rhythms play a vital role in the survival and progression of life. Everyday rhythms of physiology and behavior are managed by the circadian rhythm system, which allows creatures to forecast recurring alterations in the surrounding environment and establish critical acclimatized systems. Circadian rhythms also allow for the optimal use and production of energy (1). The mapping of nearly entire sides of physiological functions (body temperature, hormone secretions, sleep–wake cycles, etc.) occurs across these 24-h rhythms. Nonetheless, circadian rhythms are often interrupted by contemporary lifestyles. These variations in circadian rhythms are found to be significant contributory factors (2).
The central pacemaker of the circadian rhythm in mammals lies in the hypothalamus, known as the suprachiasmatic nucleus (SCN). SCN plays a crucial role in the maintenance of systemic circadian rhythms and regulates peripheral tissue clocks through the secretion of endogenous regulatory factors (3). The molecular clock of the circadian system, which is present in all cells, is made up of oscillating clock-related proteins that compose transcription and translation feedback loops (TTFLs) (4). The core TTFL is composed of the transcriptional activator proteins CLOCK and BMAL1 and the repressor proteins Period-1 (PER1), Period-2 (PER2), Period-3, Cryptochrome-1, and Cryptochrome-2 (4). Other loops are coupled with the core TTFL to maintain oscillation.
The circadian rhythm is mainly entrained by environmental signals, such as light, food, and arousal stimuli. In the SCN, the circadian clock mainly responds to the light–dark cycle (Figure 1). In peripheral tissues, the circadian rhythm can be synchronized by food or temperature (4). Moreover, internal signals, such as circulating hormones, metabolites, sympathetic nervous activation, and body temperature, are significant timing cues that regulate peripheral clocks (3).
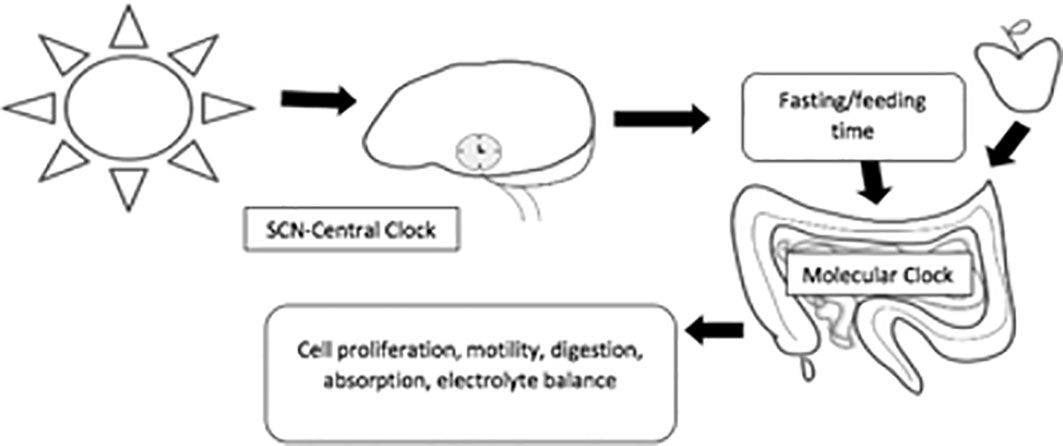
Figure 1 The main feedback loops regulating the circadian clock. In mammals, all tissues possess circadian oscillators, making the system organization highly complex. The light-entrainable pacemaker is in the suprachiasmatic nucleus (SCN) of the hypothalamus, and its function is to synchronize peripherical molecular clocks. While all these oscillate within a period close to 24 h, it is essential that they are synchronized with the external environmental conditions. Hence, the key function of the SCN clock is to receive environmental light information from the retinohypothalamic track and synchronize other molecular oscillators, both within the SCN and in peripheral organs.
The SCN is in conformance with the day–night cycles through the retina, which stimulates the pineal gland to produce the melatonin hormone (1) and also regulates the rest of the oscillators throughout the body systems to target melatonin’s receptors in different organs. A daily rhythm is adopted by the plasma melatonin, where there are high levels at night. Hence, it is known as the hormone of darkness (5, 6). The vital source of the hormones serotonin and melatonin external to the CNS is the gastrointestinal tract. A vital role is performed by these two chemicals in gastrointestinal motility (2).
Melatonin release rhythm is one of the outputs of the SCN but not the exclusive one. The C57BL6 mouse strains are deficient in melatonin synthesis due to mutations in genes coding two key enzymes of the melatonin synthesis pathway, namely, arylalkylamine-N-acetyltransferase and N-acetylserotonin-O-methyltransferase, while they have functional autonomous circadian rhythms under constant conditions (7). Inbred strains of mice (Mus musculus) kept under LD 12:12 cycles were used to study pineal gland melatonin levels (8). The results have indicated that only five inbred strains have pineal melatonin content, with higher levels during the night and lower levels during the day; the other 31 strains do not contain detectable melatonin in their pineal gland in any of the times examined. The former group includes two commonly used strains (C3H/He and CBA/Ms) and three wild-derived strains (Mol-A, Mol-Nis, MOM). C3H and CBA mice showed a similar pattern of pineal melatonin rhythm with a peak at 2 h before lights on (8, 9). These results confirm that melatonin plays a supportive instead of an essential role in maintaining circadian rhythms in mammals.
In addition to melatonin, one pathway by which the SCN is known to influence downstream processes is through the regulation of sympathetic tones (10, 11). Treatment with 6-hydroxydopamine, a neurotoxin that destroys sympathetic terminals and reduces the amplitude or abolished circadian patterns for stool number and weight, suggests that sympathetic activity is required for sustained circadian patterns of intestinal motility (12). Administration of the β-adrenergic agonist isoproterenol causes a phase-dependent shift in PER2 expression rhythms. Collectively, the data suggest that the SCN is required to maintain feeding, locomotor, and stool output rhythms during ad libitum conditions, acting at least in part through the daily activation of sympathetic activity (12).
Glucocorticoids may also mediate the actions of SCN in the intestine. The best-characterized regulation of the circadian release of glucocorticoids is via the hypothalamic–pituitary–adrenal axis controlled by the SCN (13). The extra-adrenal production of glucocorticoids may also occur in the intestine (14). In the gut, for example, steroidogenic enzymes, such as StAR, CYP11A1, and 3βHSD, have been detected in mouse gut epithelium, which can produce glucocorticoids de novo (14). Similarly, CYP11A1 and CYP11B2 are present in human colon biopsies, and human colonic tissue can produce cortisol when cultured (15). The possible functions of glucocorticoids may include the regulation of local immune homeostasis and epithelial barrier integrity (16), maturation and differentiation of epithelial cells (17), and expression of tight junction proteins (18).
Orexin and melatonin have been studied as central neuroendocrine transducers, while leptin, ghrelin, and cortisol are three peripheral hormones required in the circadian system for synchronization in mammals. They act as key internal transited messengers and inputs for other endogenous oscillators (16–18). Therefore, the exploitation of orexin receptor agonists and antagonists, such as almorexants, is helpful for narcolepsy and extensive daytime sleepiness (16).
The circadian system is formed by a network of oscillators found in central and peripheral tissues that are tightly linked to generate rhythms in vertebrates to adapt the organism to cyclic environmental changes. The nuclear receptors PPARs, REV-ERBs, and RORs are transcription factors controlled by the circadian system that regulate, among others, a large number of genes that control metabolic processes for which they have been proposed as key genes that link metabolism and temporal homeostasis (19).
Enteroendocrine cells produce over 12 different hormones reliable for some processes, such as gut motility, digestion, food absorption, metabolism, and coordination (1). The current review focuses on the hormones that exhibit circadian rhythms and their biological actions and association with pregnancy status.
The intestine hormone-producing enteroendocrine cells are distributed lengthwise along the epithelium layer through the gastrointestinal (GI) tract (1). The gut hormones regulate secretory and motility functions in the GI tract. Furthermore, they control appetite and energy expenditure, mainly via the gut–brain axis, as well as glucose homeostasis through effects on pancreatic hormone secretion (2, 20). Knowledge of the physiology of intestine hormones reveals the advancement of two categories of antidiabetes treatments, such as dipeptidyl peptidase-4 inhibitors, a drug that was approved for obesity. Another drug is glucagon-like peptide-1 (GLP-1) mimetics (21, 22).
Gut functions and circadian rhythms
In addition to central clocks, peripheral biological clocks are present in the majority of tissues, if not all of them, such as the gut, liver, retina, and heart (23). Robust circadian oscillations are demonstrated by the explants and cultured cells extracted from those tissues in gene expression (24). The expression of these clock genes and their rhythmic regulation are not unique to the SCN but instead are widely distributed in many cells and tissues. For instance, the period genes are expressed and rhythmically regulated in a variety of peripheral tissues including the liver, lung, and skeletal muscle (25). Various gut functions are controlled by the molecular clock, either dependent or independent of the SCN. These include endobiotic and xenobiotic detoxification, nutrient absorption, and motility of large intestines (26).
Different biological rhythms are also shown by the gut. Vital core activities of the gut are influenced by diurnal oscillations, such as maintaining and changing the protective barrier, motility, secretions, and gut microbiota (27).
Circadian rhythm stability is pivotal for the maintenance of mucosal barrier function. Circadian rhythm disruption increases intestinal necroptosis, thus rendering the gut epithelium more susceptible to inflammatory processes (28). It has been found that disturbances in circadian oscillators may directly and indirectly (through melatonin and other circulatory elements) affect protecting barriers in the mucosal layer of the GI and cell multiplication (29). A potential relationship between shifting the job and development of duodenal ulcers was recently reported by Pietroiusti et al. (27). It was asserted by the authors that in comparison with daytime workers, there was a higher incidence of duodenal ulcers in shift workers (27). Nonetheless, there continues to be a weak understanding of the mechanisms responsible for this occurrence. A reduction in circulating melatonin because of the shift work in the night may be a contributing factor to this phenomenon. Melatonin application accelerates gastric ulcer healing and can physiologically regulate antioxidative enzyme activity and increase gastric blood flow level (29).
There is a marked circadian variation in gastric luminal human trefoil protein (TFF2) in young healthy volunteers with peak levels present during the night. It is demonstrated that the TFF2 rhythm is impaired in cohorts of individuals known to suffer gastric symptoms of Helicobacter pylori infection (30). In the elderly, the amplitude of the circadian rhythm of TFF2 is considerably decreased, which may influence the protective systems of the GI mucosa (30, 31).
Secretory changes in the gut are also influenced by circadian rhythms, particularly modifications to HCl release (32, 33). Gastroesophageal reflux disease (GERD) is a common illness related to gastric hypersecretion. GERD patients often make complaints about recurring reflux symptoms at night. Their quality of life is adversely affected by night-time GERD, as it creates pain and disrupts sleep, which has an impact on the mental and physical activity of the person the following day. Furthermore, there are certain GERD patients who are taking antisecretory medicines, such as PPIs, and for them, a description of the nocturnal acid breakthrough phenomenon has been provided (33, 34).
Clinical investigations have been provided as support for the significance of the relationship between the molecular clock and colonic motility in clinical investigations that depict a widespread incidence of functional colonic motility disorder because of the interference in circadian rhythms (34). The relationship between alternative jobs and the existence of efficient bowel dysfunction was examined by Nojkov et al. (33). This study comprised 399 nurses that were involved in patient care. The groups examined included 214 of them during the day period, 110 nurses from the night period, and 75 who practiced alternating shifts. It was found that alternating shifts can have a substantial effect on the efficient illness of the bowels.
The aging process has one of the most powerful impacts on the circadian mechanism. To sum up, it was noticed that the following changes occurred in the circadian system: 1) age-linked modifications in the central biological clock, such as a reduced expression of AVP and VIP neuropeptides that led to a decline in circadian electrical activity amplitude and lessened sensitivity to the melatonin hormone in the SCN; 2) age-linked modifications in diurnal entrainment (a reduced response to the stimulus of light and nutrition entrainment and a decrease in melatonin concentrations in circulation); 3) changes in the genetic factor clock because of aging (lower expression of significant genes in both the master clock and peripheral tissue); and 4) incidences of efficient GI illnesses because of aging and the neurodegenerative effect of cholinergic degeneration (35–37).
Bacteroidetes and Firmicutes dominate the gut microbiota. Nonetheless, there are considerable interindividual variations in microbial composition (38). The source of this variation is the integrated impact of host genetics, physical location, aging, diet, lifestyle (39), and pet ownership (40). Confusion prevails regarding most of these factors.
It is important to have a balanced gut microbial constitution in host physiology, and it is believed that compositional disturbance leads to several illnesses. For example, it is believed that obesity causes a considerable decrease in Bacteroidetes and a subsequent elevation in Firmicutes (41).
Circadian rhythms exist in prokaryotes as well as in Cyanobacteria (42). Diurnal sequences of light and warmth are faced by free-living bacteria; hence, their circadian rhythm permits them to forecast and adapt to variations in ecological statuses.
The time-of-day-based constitution of the rat fecal microbiota was reported by Thaiss et al. (43). Routine oscillation is experienced by over 15% of identified operational taxonomical units (OTUs) in their comparative richness. These include many species, such as Lactobacillus reuteri and Dehalobacterium spp., which are part of Clostridiales, Lactobacillales, and Bacteroidales. Later on, two more groups offered further support. According to Zarrinpar et al. (44), there is a cyclic nature in 17% of OTUs. These everyday modifications are evident in the variety and constitution of the GI microbiota (45). There is a diurnal variation in the gastrointestinal ecosystem, which is subject to the time of the day and the status of food and fasting. However, the host circadian rhythms are also influenced by the microbiota (46). If the diet is adjusted, the constitution of the gut microbiome can rapidly change, which may cause the circadian rhythmicity to be modified (47). Night-shift work and induced chronodisruption have been linked in various studies to colorectal and stomach cancer (48). It is suggested that colorectal cancer can be developed as a result of the changes in the microbiota following a disruption to circadian rhythms. An irregular composition of the microbiota environment in mice was related to inflammation and tumors (49).
Circadian rhythm and enteric endocrine hormones: glucose-dependent insulinotropic polypeptide
Enteroendocrine K cells situated in the duodenum and proximal jejunum secrete glucose-dependent insulinotropic polypeptide (GIP), which is a gastric inhibitory hormone made up of 42 amino acids. The incretin effect is the process of a glucose-dependent insulin release that is regulated by GIP and glucagon-like peptides. GIP plays a significant role as an incretin hormone that regulates the levels of blood glucose. GIP and GLP-1 are secreted as endocrine hormones in the blood after eating (Table 1) and are responsible for up to 70% of insulin release as a response to meal intake (50–52). Diurnal fluctuations are shown (Table 1) following the secretion of GLP-1 right before their active period, and increased responses are shown at ZT16 in rats (52).
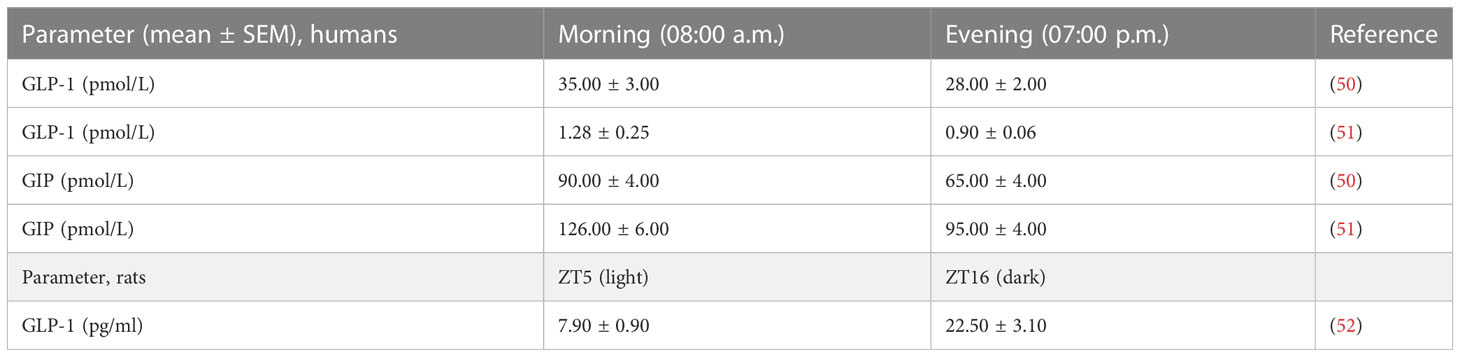
Table 1 Circadian variations of some incretin hormones after 30 min of ingestion of standardized mixed meal in healthy men or after 10 min of oral glucose load in rats.
A diurnal pattern is also shown by insulin secretion, with increased secretion taking place in terms of the active/feeding period in humans and rodents (53). In addition, it has been demonstrated in earlier studies that routine insulin-releasing rhythms exhibit a more distinct response to oral feeding than IV feeding, which shows that incretin hormones play a vital role in promoting circadian insulin release (53). The stimulation of GIP receptors (GIPR) on pancreatic β cells is found to regulate GIP (54). In addition, it has been shown in other studies that GIPR signals exhibit favorable effects that support mineral deposition in bones (55).
It is interesting to note that when the eating–fasting plan is modified for the same experimental animals, a parallel shift in peak GIP secretion occurs (54), which indicates that the major zeitgeber for the diurnal rhythm in GIP is the intake of nutrients. Variations in the pattern of GIP circadian secretion in diabetic, obese, or lower body-weight individuals indicate that the release of hormones may be affected by metabolic status (40).
A specific effect of GIP to stimulate the secretion of intestinal GLP-1 was demonstrated in vivo in rats (55). This enteroendocrine loop between the duodenal peptide GIP and the ileal GLP-1 may account for some of the early rises in the secretion of GLP-1 observed in response to nutrient ingestion.
Glucagon-like peptide-1
GLPs are released by enteroendocrine L cells found in the distal small part and large intestine (54). It is the enteroendocrine L cells that create this insulinotropic peptide. GLP-1 is an incretin hormone, and similar to GIP, it is secreted soon after a carbohydrate meal and taken to support insulin secretion, which also supports the release of glucose-dependent insulin. Food intake, stomach emptying, and glucagon release are restricted by GLP-1, which brings about β-cell differentiation, propagation, and cell neogenesis. The expression of the GLP-1 receptors occurs in different metabolically active tissues, which triggers various biological effects throughout different organ systems (54).
Research on humans indicates that GLP-1 release is temporally regulated, showing that GLP-1 has higher efficiency during the early hours of the day in comparison with the late hours of the day for participants that are administered similar meal programs with varying periods of fasting (50). A distinct release of GLP-1, depending on the time of the day, was decreased when subjects came across nocturnal light (56). Phase-delayed circadian misalignment increased rapid eye movement sleep and sleeping metabolic rate, increased glucose and decreased GLP-1 concentrations, and increased carbohydrate oxidation that may create a health risk through a metabolic disturbance (56). It has now been determined with carefully regulated studies of rats and mice that a GLP-1 release adopts a substantial 24-h secretory manner in reaction to similar levels of glucose that are given by following a similar duration of fasting (52, 57). In rodents, it was determined that the peak level of GLP-1 release was at the start of the dark/eating phase, while the lowest release occurred at the start of the light/fasting phase (52, 57), which means that the key zeitgeber of the L cell is nutrient intake, demonstrating the existence of a diurnal rhythm in GLP-1 secretory responses to an oral glucose load in rats, with increased release immediately preceding the normal feeding period. This profile of GLP-1 release correlated with the pattern in insulin secretion, and both rhythms were completely inverted in animals subjected to a 12-h feeding cycle disruption and abolished in rats maintained under constant light conditions (52). Interestingly, the intestinal microbiome was established to be an integral component of the pathway regulating diurnal GLP-1 release (57).
It was demonstrated that the core SNARE protein syntaxin-1a (syn1a), which is expressed by murine ileal L cells, plays an essential role in secretagogue-induced exocytosis of GLP-1 (58). Munc18-1 (Syntaxin binding protein-1), a regulator of membrane fusion, further controls vesicle docking and secretion by interacting with syntaxin-1 and accompanies it to the plasma membrane (37, 59).
Clinical studies found that the GLP-1 secretory rhythm of obese individuals is lost, providing support to the idea that obesogenic feeding also affects diurnal GLP-1 secretion (60). In addition, GLP-1 secretory rhythms have also been modified in morbidly obese T2D patients in comparison to a group having standard tolerance for glucose (60). Exposing rat’s GLUTag L cells to palmitate as a saturated fatty acid, which was a key constituent of the obesogenic Western diet, impairs circadian glucagon-like peptide-1 secretion (61).
The rhythm in GLP-1 release was paralleled by identical peak and trough insulin responses by β cells (52). Continuous GLP-1 receptor activation also increases insulin synthesis and β-cell proliferation and neogenesis (62). Exposure of human islets to melatonin for 12 h increases the sensitivity of the β cell to the stimulatory effects of GLP-1 (63). Thus, it appears likely that the human β cell shows a circadian pattern in its response to GLP-1 (63).
In chronotherapy, medicine is administrated at a specific time of the day, maximizing therapeutic response(s) and minimizing negative effects by benefiting from the circadian rhythms in physiology (Table 2). The dual GLP-1R/GIPR agonist DA-JC1 has been found to exert a stronger hypoglycemic effect than a GLP-1R agonist alone and could effectively improve the decline of learning and memory and circadian rhythm disorders induced by Aβ31-35 in mice (76).
Oxyntomodulin
A circadian pattern of release is shown by OXM, which is co-produced with GLP-1 in the L cell in the intestine, where the peak release occurs between the dark/active period (77). OXM functions through the receptors of both hormones GLP-1 and glucagon receptors by decreasing body weight and enhancing glucose metabolism (77, 78). The homeostatic functions of OXM on the body’s energy are exerted by enhancing the use of energy through the glucagon receptor and reducing energy intake, most likely through hypothalamic and area postrema activation by GLP-1R signaling (79, 80). Acute OXM infusion improves glucose tolerance in T2DM patients making dual agonists of the glucagon receptors and GLP-1R new promising treatments for diabetes and obesity with the potential for weight loss and glucose lowering superior to that of GLP-1R agonists (81, 82).
Peptide YY
Peptide YY (PYY) is another anorexigenic hormone that is released by the L cells. In humans, the peak levels occur during the day (83). PYY can be characterized as having a meal-driven diurnal rhythm, as illustrated by significant correlations between PYY and meal timing as well as caloric load of a meal eliciting postprandial responses and contributing to the 24-h profile (84). Fasting as well as postprandial PYY concentrations has been shown to be depressed in obese and elevated in energy-deficient women (84). Interestingly, Guo et al. (85) found a negative correlation between fasting PYY and resting metabolic rate in humans. It is imperative to perform additional studies to explain the function of the circadian release of PYY to balance the diurnal rhythm of energy production in the body. Digestion is slowed down by PYY, which functions like an “ileal brake” in order to induce a better rate of absorbing nutrients (86). The highest concentrations are reached by PYY up to 2 h postprandially, which helps in meal cessation (87). Therefore, PYY is recognized as a satiety hormone.
Neurotensin
Neurotensin is a hormone produced by N cells found in the distal part of the small intestine and facilitates fat ingestion and absorption (88), which in turn stimulates neurotensin release in animals and humans (89). It has been found that neurotensin also exhibits circadian rhythms. There was a significant 24-h rhythm in the levels of neurotensin in groups of rats maintained under constant illumination or a 12:12 light:dark cycle and fasted for either 24 h or provided food ad libitum. The levels of neurotensin were the highest during the early morning (04:00–08:00 h) and the lowest during the afternoon (12:00–16:00 h) in rat small intestines (90). There is a close relationship between neurotensin, intestinal GLP-1, and PYY, and the three peptide-regulating hormones are expressed, preserved, and secreted at the same time to manage peripheral and central metabolic targets (91). The essential processes of digestion and nutrient absorption that take place in the small intestine are dependent on the constant proliferation and differentiation of intestinal epithelial cells (84). This continuous self-renewal process is mediated by intestinal stem cells, which integrate dietary signals to maintain intestinal homeostasis. Both neurotensin and GLP-2 induce mucosal growth and proliferation and promote intestinal repair after inflammatory damage (92). At the molecular level, the GLP-1 receptor and the neurotensin receptor 1, which is dominating in the periphery, are coupled to Gs and Gq/11, respectively, which are signaling pathways that are well established to act synergistically (93).
Enteroendocrine hormones and pregnancy
Normal pregnancy requires a functional circadian system (94–97), and circadian disruption (Figure 2) can perturb pregnancy outcomes (98, 99). At approximately day 7 of pregnancy, mice shift their activity to a time that is 4 h earlier than for non-pregnant animals. In women, the timing of sleep onset is earlier during the first 27 weeks of gestation (100). In women, the onset of labor most commonly occurs between midnight and 4:00 a.m (101). Similarly, rodents are most likely to deliver pups at around dawn (101, 102). In pregnant rats, the ablation of SCN abolishes the preferential clustering of pup deliveries around dawn (103). Finally, women who work at night or on rotating shifts are 60% more likely to miscarry or deliver preterm than women who work day shifts (104, 105).
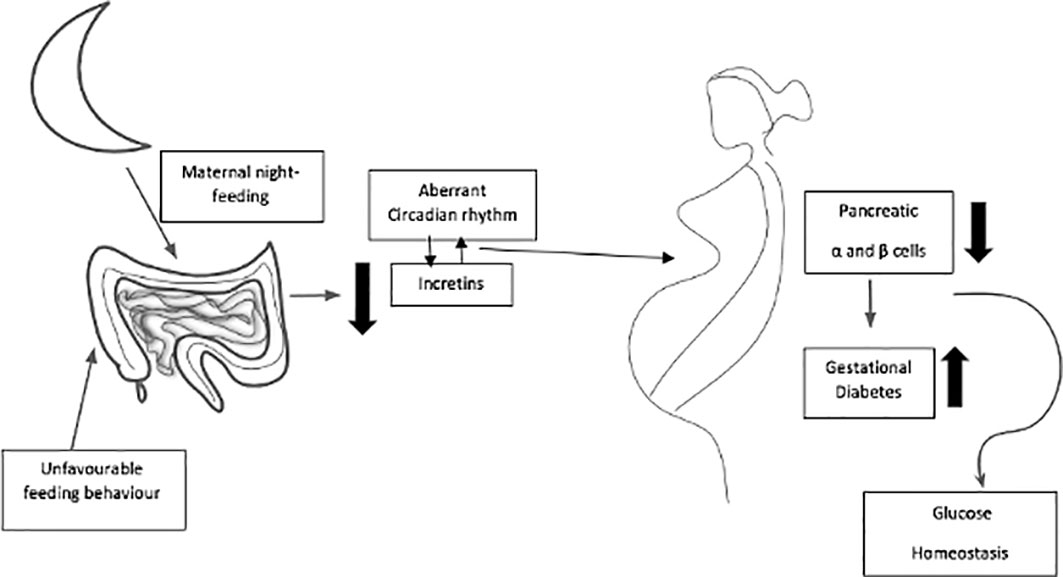
Figure 2 Maternal dark-period energy intake at night-time can together affect circadian rhythm of incretins, glucose tolerance and function of β-cells in pregnancy, and expose to high possibility of acquiring gestational diabetes mellitus.
The enteroendocrine hormones PYY, GLP-1, and GLP-2 are co-secreted from the gut L cells (91) and have been linked with gut growth (16, 106) and increased capacity for nutrient absorption (98). Circadian rhythms of enteroendocrine hormones could be an additional mechanism to support pregnancy, increasing the surface area and gut capacity to process nutrients from more food. On day 4, pregnant rats had the largest ascending colon circumferences, with higher values in the duodenum and descending colon than in controls (107). These data may reflect the earliest pregnancy adaptations to rapidly increasing capacity, especially of the cecum to hold more food and facilitate microbe diversity changes (108). Hormonal (PYY/GLP-1) stimulation is needed to initiate and maintain gut growth upon extensive organ remodeling (99, 109). PYY and GLP-1 are co-secreted, with GLP-2 (91) having been previously linked with gut growth in adult female mice and rats (110, 111). PYY and GLP-2 cause substantial intestinal hypertrophy, which highlights the role of L cells and their secretory products after gut surgeries for body mass reduction, as these techniques lead to rapidly increased concentrations of appetite-regulating hormones (112) and may stimulate intestinal growth in attempts to regenerate the remaining gut tissues (112).
In the initial days of gestation, maternal metabolism is an anabolism process that manages the higher requirement of energy for fetal and placental growth in the later stages of gestation. Multiple biomolecules including glucose, fatty acids, ketone bodies, and hormones collectively contribute toward these metabolic adaptations. This is because of elevated concentrations of circulating blood glucose to meet the increasing requirements of the growth and metabolism of both placental and fetus development and metabolism (113). Changes in maternal release and sensitivity of insulin that take place during different stages of pregnancy are important for regulating these changes in energy utilization (113). It has been determined that there is a greater prevalence of insulin resistance and maternal obesity during pregnancy (114). Research carried out in North America and Scandinavia (115) shows the adverse effects of these alterations on carbohydrate metabolism, fetal development in utero, and neonatal health subsequently. Excess maternal weight gain in pregnancy contributes to a glycemic environment and insulin resistance that affects fetal growth (95). Maternal GLP-1 might be involved in mechanisms that compensate for the pregnancy-related increase in glycemia and insulin resistance, suggesting a role of this peptide in maternal metabolism and weight and fetal growth (116). Lowered levels of GIP and GLP-1 may play an important role in the abnormality of glucose regulation following pregnancy. Serum GIP and GLP-1 levels were inversely associated with gestational diabetes mellitus (GDM), and participants with lower levels of GIP and GLP-1 had a seven-fold higher risk of developing GDM compared with the higher levels, suggesting that there is an independent, inverse association between fasting incretins and higher risk of GDM (114).
It has been demonstrated in experimental studies on mice that the quantity of maternal intestinal GLP-1-secreting L cells in the maternal intestine increases during pregnancy (117). Pregnancy is associated with the physiological and reversible expansion of β-cell mass (117). Incretin hormones inhibit β-cell apoptosis and stimulate proliferation, resulting in the development of β-cell mass (54). Islet adaptations to pregnancy were explored in C57BL6/J mice lacking functional receptors for GLP-1 and gastric inhibitory polypeptide (GIP). The data collected indicated that GLP-1 but not GIP is a key mediator of β-cell mass expansion and related adaptations in pregnancy, triggered in part by the generation of intra-islet GLP-1 (117).
Alterations in circadian rhythms are brought about by maternal adjustment (117–121), with significant modifications in the expression pattern of diurnal clock genes (122). The variations in mother gene expressions of peripheral clocks throughout gestation specifically bring about downstream changes in the diurnal expression of particular metabolism genes, such as the glucoregulatory genes Pck1, Gk, and G6Pase, to ensure a healthy pregnancy (122). This shows that when there are interruptions in circadian rhythms during pregnancy, women may be at risk of acquiring metabolic diseases and experiencing negative effects of gestation. It was determined that night-shift pregnant employees face more risks of prematurity, miscarriage, hypertensive disorders, and low birth weights (123). These results are not only relevant to night-shift pregnant employees but are likewise applicable to pregnant women population who consume high-energy intakes in the evening or in their night shift, with possible chronodisruption. The human body is evolved for rest at night. Observations in pregnant women are in agreement with evidence in men, indicating that food timing and the amount of dietary carbohydrates could affect glucose metabolism (123–125). A dietary carbohydrate constitution (for example, highly vs. poorly digestible carbohydrates) has a significant impact on the clock mediating glucose homeostasis (125).
Maternal dark-period energy intake and the volume and form of intaking carbohydrates at night can together affect glucose tolerance and the function of β cells in pregnancy and exposure to the high possibility of acquiring gestational diabetes mellitus. Here, the triggered or natural circadian release of enteroendocrine hormones may play a role as an insulinotropic. A significant research topic that is of interest to scientists and nutritionists/dietitians focuses on nutritional programs to attain optimal results in pregnancy. It seems that the timing of intaking energy and diet constituents could be used to mitigate metabolic disturbances that may happen throughout pregnancy, in addition to the safety impact on gestation results. It can be concluded from the few studies presented that intaking a high-energy source at nighttime and its diet component may contribute to impaired energy and glucose metabolism and reproductive hormone disruption during pregnancy (126, 127). In pregnant animals, it was found that disturbed intake timing, or feeding (during the day period by nocturnal rodents), gave rise to an irregular circadian rhythm in the mother and fetus (127) and was capable of altering the microbiota profiles to give rise to metabolic disorders (128). However, studies that translate these mechanisms to pregnant women have not yet been carried out.
The importance of the circadian clock in maintaining human health is now widely acknowledged. Circadian rhythms can act as therapeutical targets, therefore managing the factors that influence the clocks. Some of the potentials as chronotherapeutic agents are flavonoids. Nobiletin, a polymethoxy flavone obtained from dried citrus peel, known as Citri Reticulatae Pericarpium fruit, was identified as a particularly effective clock amplitude-enhancing small molecule and can directly affect the mammalian circadian system (128–130). Time-restricted feeding partially restores diurnal rhythms of the ileal microbiome (131). Interestingly, regular fasting periods may provide physiological benefits such as improved circadian rhythmicity and modulation of the gut microbiota (127).
Conclusion
It is demonstrated in the current review that under physiological conditions, the enteroendocrine hormones GLP-1, neurotensin, GIP, PYY, and OXM play a role in regulating vital metabolic functions through circadian secretion rhythms. A synergistic effect may be indicated by their secretion, co-expression, and action on metabolism when administered in pharmacological doses. A vital theme of research for scientists is considering diet programming as a new strategic policy to achieve optimal pregnancy results. Changes in circadian patterns are brought about by maternal modification during gestation, with significant differences in circadian clock gene expression. Disturbances in metabolism in pregnancy that may lead to negative results could be addressed by possible novel methods of programming the timing of energy intake and diet components.
Author contributions
All authors contributed to the article and approved the submitted version.
Conflict of interest
The authors declare that the research was conducted in the absence of any commercial or financial relationships that could be construed as a potential conflict of interest.
Publisher’s note
All claims expressed in this article are solely those of the authors and do not necessarily represent those of their affiliated organizations, or those of the publisher, the editors and the reviewers. Any product that may be evaluated in this article, or claim that may be made by its manufacturer, is not guaranteed or endorsed by the publisher.
References
1. Konturek PC, Brzozowski T, Konturek SJ. Gut clock: implication of circadian rhythms in the gastrointestinal tract. J Physiol Pharmacol (2011) 62(2):139–50.
2. Codoñer-Franch P, Gombert M. Circadian rhythms in the pathogenesis of gastrointestinal diseases. World J Gastroenterol (2018) 24(38):4297–303. doi: 10.3748/wjg.v24.i38.4297
3. Dibner C, Schibler U, Albrecht U. The mammalian circadian timing system: organization and coordination of central and peripheral clocks. Annu Rev Physiol (2010) 72:517–49. doi: 10.1146/annurev-physiol-021909-135821
4. Gekakis N, Staknis D, Nguyen HB, Davis FC, Wilsbacher LD, King DP, et al. Role of the CLOCK protein in the mammalian circadian mechanism. Science (1998) 280:1564–9. doi: 10.1126/science.280.5369.1564
5. Bubenik GA, Konturek SJ. Melatonin and aging: prospects for human treatment. J Physiol Pharmacol (2011) 62(1):13–9.
6. Abbas A, Raju J, Milles J, Ramachandran S. A circadian rhythm sleep disorder: melatonin resets the biological clock. J R Coll Physicians Edinb (2010) 40(4):311–3. doi: 10.4997/JRCPE.2010.406
7. Zhang Z, Silveyra E, Jin N, Ribelayga CP. A congenic line of the C57BL/6J mouse strain that is proficient in melatonin synthesis. J Pineal Res (2018) 65(3):e12509. doi: 10.1111/jpi.12509
8. Goto M, Oshima I, Tomita T, Ebihara S. Melatonin content of the pineal gland in different mouse strains. J Pineal Res (1989) 7:195–204. doi: 10.1111/j.1600-079X.1989.tb00667.x
9. Kennaway DJ. Melatonin research in mice: a review. Chronobiol Int (2019) 36(9):1167–83. doi: 10.1080/07420528.2019.1624373
10. Brusco LI, García-Bonacho M, Esquifino AI, Cardinali DP. Diurnal rhythms in norepinephrine and acetylcholine synthesis of sympathetic ganglia, heart and adrenals of aging rats: effect of melatonin. J Auton Nerv Syst (1998) 74:49 –61. doi: 10.1016/S0165-1838(98)00134-9
11. Vujovic N, Davidson AJ, Menaker M. Sympathetic input modulates, but does not determine, phase of peripheral circadian oscillators. Am J Physiol Regul Integr Comp Physiol (2008) 295:R355–R3560. 34. doi: 10.1152/ajpregu.00498.2007
12. Malloy JN, Paulose JK, Li Y, Cassone VM. Circadian rhythms of gastrointestinal function are regulated by both central and peripheral oscillators. Am J Physiol Gastrointest Liver Physiol (2012) 303(4):G461–73. doi: 10.1152/ajpgi.00369.2011
13. Miller WL, Auchus RJ. The molecular biology, biochemistry, and physiology of human steroidogenesis and its disorders. Endocr Rev (2011) 32:81–151. doi: 10.1210/er.2010-001
14. Cima I, Corazza N, Dick B, Fuhrer A, Herren S, Jakob S, et al. Intestinal epithelial cells synthesize glucocorticoids and regulate T cell activation. J Exp Med (2004) 200(12):1635–46. doi: 10.1084/jem.20031958
15. Sidler D, Renzulli P, Schnoz C, Berger B, Schneider-Jakob S, Flück C, et al. Colon cancer cells produce immunoregulatory glucocorticoids. Oncogene (2011) 30(21):2411–9. doi: 10.1038/onc.2010.629
16. Kostadinova F, Schwaderer J, Sebeo V, Brunner T. Why does the gut synthesize glucocorticoids? Ann Med (2014) 46:490–7. doi: 10.3109/07853890.2014.932920
17. Boivin MA, Ye D, Kennedy JC, Al-Sadi R, Shepela C, Ma TY. Mechanism of glucocorticoid regulation of the intestinal tight junction barrier. Am J Physiol Gastrointest Liver Physiol (2007) 292:G590–8. doi: 10.1152/ajpgi.00252.2006
18. Lu L, Li T, Williams G, Petit E, Borowsky M, Walker WA. Hydrocortisone induces changes in gene expression and differentiation in immature human enterocytes. Am J Physiol Gastrointest Liver Physiol (2011) 300:G425–32. doi: 10.1152/ajpgi.00011.2010
19. Gómez-Boronat M, De Pedro N, Alonso-Gómez ÁL, Delgado MJ, Isorna E. Nuclear receptors (PPARs, REV-ERBs, RORs) and clock gene rhythms in goldfish (Carassius auratus) are differently regulated in hypothalamus and liver. Front Physiol (2022) 13:903799. doi: 10.3389/fphys.2022.903799
20. Lee C, Etchegaray JP, Cagampang FR, Loudon AS, Reppert SM. Posttranslational mechanisms regulate the mammalian circadian clock. Cell (2001) 107(7):855–67. doi: 10.1016/s0092-8674(01)00610-9
21. Thompson MD, Xhaard H, Sakurai T, Rainero I, Kukkonen JP. OX1 and OX2 orexin/hypocretin receptor pharmacogenetics. Front Neurosci (2014) 8:57. doi: 10.3389/fnins.2014.00057
22. Isorna E, de Pedro N, Valenciano AI, Alonso-Gómez Á.L, Delgado MJ. Interplay between the endocrine and circadian systems in fishes. J Endocrinol (2017) 232(3):R141–59. doi: 10.1530/JOE-16-0330
23. Pardini L, Kaeffer B, Trubuil A, Bourreille A, Galmiche JP. Human intestinal circadian clock: expression of clock genes in colonocytes lining the crypt. Chronobiol Int (2005) 22(6):951–61. doi: 10.1080/07420520500395011
24. Pan X, Hussain MM. Clock is important for food and circadian regulation of macronutrient absorption in mice. J Lipid Res (2009) 50(9):1800–13. doi: 10.1194/jlr.M900085-JLR200
25. Yamazaki S, Numano R, Abe M, Hida A, Takahashi R, Ueda M, et al. Resetting central and peripheral circadian oscillators intransgenic rats. Science (2000) 288:682–5. doi: 10.1126/science.288.5466.682
26. Bron R, Furness JB. Rhythm of digestion: keeping time in the gastrointestinal tract. Clin Exp Pharmacol Physiol (2009) 36(10):1041–8. doi: 10.1111/j.1440-1681.2009.05254.x
27. Pietroiusti A, Forlini A, Magrini A, Galante A, Coppeta L, Gemma G, et al. Shift work increases the frequency of duodenal ulcer in h pylori infected workers. Occup Environ Med (2006) 63(11):773–5. doi: 10.1136/oem.2006.027367
28. Pagel R, Bär F, Schröder T, Sünderhauf A, Künstner A, Ibrahim SM, et al. Circadian rhythm disruption impairs tissue homeostasis and exacerbates chronic inflammation in the intestine. FASEB J (2017) 31(11):4707–19. doi: 10.1096/fj.201700141RR
29. Magierowski M, Jasnos K, Brzozowska I, Drozdowicz D, Sliwowski Z, Nawrot E, et al. Melatonina jako czynnik leczniczy wzgledem wrzodów zoładka w warunkach eksperymentalnej cukrzycy [Melatonin as a therapeutic factor in gastric ulcer healing under experimental diabetes]. Przegl Lek (2013) 70(11):942–6.
30. Johns CE, Newton JL, Westley BR, May FE. The diurnal rhythm of the cytoprotective human trefoil protein TFF2 is reduced by factors associated with gastric mucosal damage: ageing, helicobacter pylori infection, and sleep deprivation. Am J Gastroenterol (2005) 100(7):1491–7. doi: 10.1111/j.1572-0241.2005.41859.x
31. Scheving LA. Biological clocks and the digestive system. Gastroenterology (2000) 119(2):536–49. doi: 10.1053/gast.2000.9305
32. Pácha J, Sumová A. Circadian regulation of epithelial functions in the intestine. Acta Physiol (Oxf) (2013) 208(1):11–24. doi: 10.1111/apha.12090
33. Nojkov B, Rubenstein JH, Chey WD, Hoogerwerf WA. The impact of rotating shift work on the prevalence of irritable bowel syndrome in nurses. Am J Gastroenterol (2010) 105(4):842–7. doi: 10.1038/ajg.2010.48
34. Zhen Lu W, Ann Gwee K, Yu Ho K. Functional bowel disorders in rotating shift nurses may be related to sleep disturbances. Eur J Gastroenterol Hepatol (2006) 18(6):623–7. doi: 10.1097/00042737-200606000-00008
35. Gibson EM, Williams WP 3rd, Kriegsfeld LJ. Aging in the circadian system: considerations for health, disease prevention and longevity. Exp Gerontol (2009) 44(1-2):51–6. doi: 10.1016/j.exger.2008.05.007
36. Hofman MA, Swaab DF. Living by the clock: the circadian pacemaker in older people. Ageing Res Rev (2006) 5(1):33–51. doi: 10.1016/j.arr.2005.07.001
37. Campbell JR, Martchenko A, Sweeney ME, Maalouf MF, Psichas A, Gribble FM, et al. Essential role of syntaxin-binding protein-1 in the regulation of glucagon-like peptide-1 secretion. Endocrinology (2020) 161(5):bqaa039. doi: 10.1210/endocr/bqaa039
38. Yatsunenko T, Rey FE, Manary MJ, Trehan I, Dominguez-Bello MG, Contreras M, et al. Human gut microbiome viewed across age and geography. Nature (2012) 486(7402):222–7. doi: 10.1038/nature11053
39. Annalisa N, Alessio T, Claudette TD, Erald V, Antonino de L, Nicola DD. Gut microbioma population: an indicator really sensible to any change in age, diet, metabolic syndrome, and life-style. Mediators Inflamm (2014) 2014:901308. doi: 10.1155/2014/901308
40. Song SJ, Lauber C, Costello EK, Lozupone CA, Humphrey G, Berg-Lyons D, et al. Cohabiting family members share microbiota with one another and with their dogs. Elife (2013) 2:e00458. doi: 10.7554/eLife.00458
41. Zhao L. The gut microbiota and obesity: from correlation to causality. Nat Rev Microbiol (2013) 11(9):639–47. doi: 10.1038/nrmicro3089
42. Grobbelaar N, Huang TC, Lin HY, Chow TJ. Dinitrogen-fixing endogenous rhythm in synechococcus RF-1. FEMS Microbiol Lett (1986) 37(2):173–7. doi: 10.1111/j.1574-6968.1986.tb01788.x
43. Thaiss CA, Zeevi D, Levy M, Zilberman-Schapira G, Suez J, Tengeler AC, et al. Transkingdom control of microbiota diurnal oscillations promotes metabolic homeostasis. Cell (2014) 159(3):514–29. doi: 10.1016/j.cell.2014.09.048
44. Zarrinpar A, Chaix A, Yooseph S, Panda S. Diet and feeding pattern affect the diurnal dynamics of the gut microbiome. Cell Metab (2014) 20(6):1006–17. doi: 10.1016/j.cmet.2014.11.008
45. Voigt RM, Forsyth CB, Green SJ, Engen PA, Keshavarzian A. Circadian rhythm and the gut microbiome. Int Rev Neurobiol (2016) 131:193–205. doi: 10.1016/bs.irn.2016.07.002
46. Liang X, Bushman FD, FitzGerald GA. Rhythmicity of the intestinal microbiota is regulated by gender and the host circadian clock. Proc Natl Acad Sci U.S.A (2015) 112(33):10479–84. doi: 10.1073/pnas.1501305112
47. Murakami M, Tognini P, Liu Y, Eckel-Mahan KL, Baldi P, Sassone-Corsi P. Gut microbiota directs PPARγ-driven reprogramming of the liver circadian clock by nutritional challenge. EMBO Rep (2016) 17(9):1292–303. doi: 10.15252/embr.201642463
48. Gyarmati G, Turner MC, Castaño-Vinyals G, Espinosa A, Papantoniou K, Alguacil J, et al. Night shift work and stomach cancer risk in the MCC-Spain study. Occup Environ Med (2016) 73(8):520–7. doi: 10.1136/oemed-2016-103597
49. Zackular JP, Baxter NT, Iverson KD, Sadler WD, Petrosino JF, Chen GY, et al. The gut microbiome modulates colon tumorigenesis. mBio (2013) 4(6):e00692– 13. doi: 10.1128/mBio.00692-13
50. Lindgren O, Mari A, Deacon CF, Carr RD, Winzell MSörhede, Vikman J, et al. Differential islet and incretin hormone responses in morning versus afternoon after standardized meal in healthy men. J Clin Endocrinol Metab (2009) 94(8):2887–92. doi: 10.1210/jc.2009-0366
51. Kessler K, Hornemann S, Petzke KJ, Kemper M, Kramer A, Pfeiffer AF, et al. The effect of diurnal distribution of carbohydrates and fat on glycaemic control in humans: a randomized controlled trial. Sci Rep (2017) 7:44170. doi: 10.1038/srep44170
52. Gil-Lozano M, Mingomataj EL, Wu WK, Ridout SA, Brubaker PL. Circadian secretion of the intestinal hormone GLP-1 by the rodent l cell. Diabetes (2014) 63(11):3674–85. doi: 10.2337/db13-1501
53. Peschke E, Peschke D. Evidence for a circadian rhythm of insulin release from perifused rat pancreatic islets. Diabetologia (1998) 41(9):1085–92. doi: 10.1007/s001250051034
54. Baggio LL, Drucker DJ. Biology of incretins: GLP-1 and GIP. Gastroenterology (2007) 132(6):2131–57. doi: 10.1053/j.gastro.2007.03.054
55. Roberge JN, Brubaker PL. Regulation of intestinal proglucagon-derived peptide secretion by glucose-dependent insulinotropic peptide in a novel enteroendocrine loop. Endocrinology (1993) 133(1):233–40. doi: 10.1210/endo.133.1.8319572
56. Gonnissen HK, Rutters F, Mazuy C, Martens EA, Adam TC, Westerterp-Plantenga MS. Effect of a phase advance and phase delay of the 24-h cycle on energy metabolism, appetite, and related hormones. Am J Clin Nutr (2012) 96(4):689–97. doi: 10.3945/ajcn.112.037192
57. Martchenko SE, Martchenko A, Cox BJ, Naismith K, Waller A, Gurges P, et al. Circadian GLP-1 secretion in mice is dependent on the intestinal microbiome for maintenance of diurnal metabolic homeostasis. Diabetes (2020) 69(12):2589–602. doi: 10.2337/db20-0262
58. Wheeler SE, Stacey HM, Nahaei Y, Hale SJ, Hardy AB, Reimann F, et al. The SNARE protein syntaxin-1a plays an essential role in biphasic exocytosis of the incretin hormone glucagon-like peptide 1. Diabetes (2017) 66(9):2327–38. doi: 10.2337/db16-1403
59. Han GA, Malintan NT, Saw NM, Li L, Han L, Meunier FA, et al. Munc18-1 domain-1 controls vesicle docking and secretion by interacting with syntaxin-1 and chaperoning it to the plasma membrane. Mol Biol Cell (2011) 22(21):4134–49. doi: 10.1091/mbc.E11-02-0135
60. Galindo Muñoz JS, Jiménez Rodríguez D, Hernández Morante JJ. Diurnal rhythms of plasma GLP-1 levels in normal and overweight/obese subjects: lack of effect of weight loss. J Physiol Biochem (2015) 71(1):17–28. doi: 10.1007/s13105-014-0375-7
61. Martchenko A, Oh RH, Wheeler SE, Gurges P, Chalmers JA, Brubaker PL. Suppression of circadian secretion of glucagon-like peptide-1 by the saturated fatty acid, palmitate. Acta Physiol (Oxf) (2018) 222(4):e13007. doi: 10.1111/apha.13007
62. Doyle ME, Egan JM. Mechanisms of action of glucagon-like peptide 1 in the pancreas. Pharmacol Ther (2007) 113(3):546–93. doi: 10.1016/j.pharmthera.2006.11.007
63. Costes S, Boss M, Thomas AP, Matveyenko AV. Activation of melatonin signaling promotes β-cell survival and function. Mol Endocrinol (2015) 29(5):682–92. doi: 10.1210/me.2014-1293
64. Mingrone G, Nolfe G, Gissey GC, Iaconelli A, Leccesi L, Guidone C, et al. Circadian rhythms of GIP and GLP1 in glucose-tolerant and in type 2 diabetic patients after biliopancreatic diversion. Diabetologia (2009) 52(5):873–81. doi: 10.1007/s00125-009-1288-9
65. Eissele R, Göke R, Willemer S, Harthus HP, Vermeer H, Arnold R, et al. Glucagon-like peptide-1 cells in the gastrointestinal tract and pancreas of rat, pig and man. Eur J Clin Invest (1992) 22(4):283–91. doi: 10.1111/j.1365-2362.1992.tb01464.x
66. Goyal RK, Guo Y, Mashimo H. Advances in the physiology of gastric emptying. Neurogastroenterol Motil (2019) 31(4):e13546. doi: 10.1111/nmo.13546
67. Nyström T, Gutniak MK, Zhang Q, Zhang F, Holst JJ, Ahrén B, et al. Effects of glucagon-like peptide-1 on endothelial function in type 2 diabetes patients with stable coronary artery disease. Am J Physiol Endocrinol Metab (2004) 287(6):E1209– 15. doi: 10.1152/ajpendo.00237.2004
68. Pyke C, Heller RS, Kirk RK, Ørskov C, Reedtz-Runge S, Kaastrup P, et al. GLP-1 receptor localization in monkey and human tissue: novel distribution revealed with extensively validated monoclonal antibody. Endocrinology (2014) 155(4):1280–90. doi: 10.1210/en.2013-1934
69. Tanaka A, Node K. Clinical application of glucagon-like peptide-1 receptor agonists in cardiovascular disease: lessons from recent clinical cardiovascular outcomestrials. Cardiovasc Diabetol (2018) 17:85. doi: 10.1186/s12933-018-0731-y
70. Biancolin AD, Martchenko A, Mitova E, Gurges P, Michalchyshyn E, Chalmers JA, et al. The core clock gene, Bmal1, and its downstream target, the SNARE regulatory protein secretagogin, are necessary for circadian secretion of glucagon-like peptide-1. Mol Metab (2020) 31:124–37. doi: 10.1016/j.molmet.2019.11.004
71. Campbell JE, Drucker DJ. Pharmacology, physiology, and mechanisms of incretin hormone action. Cell Metab (2013) 17(6):819–37. doi: 10.1016/j.cmet.2013.04.008
72. Mansur SA, Mieczkowska A, Flatt PR, Chappard D, Irwin N, Mabilleau G. Sitagliptin alters bone composition in high-Fat-Fed mice. Calcif Tissue Int (2019) 104(4):437–448. doi: 10.1007/s00223-018-0507-0
73. Gupta NA, Mells J, Dunham RM, Grakoui A, Handy J, Saxena NK, et al. Glucagon-like peptide-1 receptor is present on human hepatocytes and has a direct role in decreasing hepatic steatosis in vitro by modulating elements of the insulin signaling pathway. Hepatology (2010) 51(5):1584–92. doi: 10.1002/hep.23569
74. Yang Q, Wang F. Successful pregnancy after improving insulin resistance with the glucagon-like peptide-1 analogue in a woman with polycystic ovary syndrome: a case report and review of the literature. Gynecol Obstet Invest (2016) 81(5):477–80. doi: 10.1159/000446951
75. Cork SC, Richards JE, Holt MK, Gribble FM, Reimann F, Trapp S. Distribution and characterisation of glucagon-like peptide-1 receptor expressing cells in the mouse brain. Mol Metab (2015) 4(10):718–31. doi: 10.1016/j.molmet.2015.07.008
76. Wang L, Zhang R, Hou X, Wang C, Guo S, Ning N, et al. DA-JC1 improves learning and memory by antagonizing Aβ31-35- induced circadian rhythm disorder. Mol Brain (2019) 12(1):14. doi: 10.1186/s13041-019-0432-9
77. Landgraf D, Tsang AH, Leliavski A, Koch CE, Barclay JL, Drucker DJ, et al. Oxyntomodulin regulates resetting of the liver circadian clock by food. Elife (2015) 4:e06253. doi: 10.7554/eLife.06253
78. Briere DA, Bueno AB, Gunn EJ, Michael MD, Sloop KW. Mechanisms to elevate endogenous GLP-1 beyond injectable GLP-1 analogs and metabolic surgery. Diabetes (2018) 67(2):309–20. doi: 10.2337/db17-0607
79. Baggio LL, Huang Q, Brown TJ, Drucker DJ. Oxyntomodulin and glucagon-like peptide-1 differentially regulate murine food intake and energy expenditure. Gastroenterology (2004) 127(2):546–58. doi: 10.1053/j.gastro.2004.04.063
80. Du X, Kosinski JR, Lao J, Shen X, Petrov A, Chicchi GG, et al. Differential effects of oxyntomodulin and GLP-1 on glucose metabolism. Am J Physiol Endocrinol Metab (2012) J303(2):E265–71. doi: 10.1152/ajpendo.00142.2012
81. Parlevliet ET, Heijboer AC, Schröder-van der Elst JP, Havekes LM, Romijn JA, Pijl H, et al. Oxyntomodulin ameliorates glucose intolerance in mice fed a high- fat diet. Am J Physiol Endocrinol Metab (2008) 294(1):E142–7. doi: 10.1152/ajpendo.00576.2007
82. Pocai A. Action and therapeutic potential of oxyntomodulin. Mol Metab (2013) 3(3):241–51. doi: 10.1016/j.molmet.2013.12.001
83. Hill BR, De Souza MJ, Williams NI. Characterization of the diurnal rhythm of peptide YY and its association with energy balance parameters in normal-weight premenopausal women. Am J Physiol Endocrinol Metab (2011) 301(2):E409–15. doi: 10.1152/ajpendo.00171.2011
84. Scheid JL, Williams NI, West SL, VanHeest JL, De Souza MJ. Elevated PYY is associated with energy deficiency and indices of sub-clinical disordered eating in exercising women with hypothalamic amen-orrhea. Appetite (2009) 52:184–92. doi: 10.1016/j.appet.2008.09.016
85. Guo Y, Ma L, Enriori PJ, Koska J, Franks PW, Brookshire T, et al. Physiological evidence for the involvement of peptide YY in the regulation of energy homeostasis in humans. Obes (Silver Spring) (2006) 14:1562–70. doi: 10.1038/oby.2006.180
86. Huda MS, Wilding JP, Pinkney JH. Gut peptides and the regulation of appetite. Obes Rev (2006) 7:163–82. doi: 10.1111/j.1467-789X.2006.00245.x
87. Adrian TE, Ferri GL, Bacarese-Hamilton AJ, Fuessl HS, Polak JM, Bloom SR. Human distribution and release of a putative new gut hormone, peptide YY. Gastroenterology (1985) 89:1070 –1077. doi: 10.1016/0016-5085(85)90211-2
88. Armstrong MJ, Parker MC, Ferris CF, Leeman SE. Neurotensin stimulates [3H]oleic acid translocation across rat small intestine. Am J Physiol (1986) 251:G823–829. doi: 10.1152/ajpgi.1986.251.6.G823
89. Rosell S, Rokaeus A. The effect of ingestion of amino acids, glucose and fat on circulating neurotensin-like immunoreactivity (NTLI) in man. Acta Physiologica Scandinavica (1979) 107:263–7. doi: 10.1111/j.1748-1716.1979.tb06472.x
90. Ferris CF, George JK, Albers HE. Circadian rhythm of neurotensin levels in rat small intestine. Regul Pept (1986) 15(4):285–92. doi: 10.1016/0167-0115(86)90158-8
91. Grunddal KV, Ratner CF, Svendsen B, Sommer F, Engelstoft MS, Madsen AN, et al. Neurotensin is coexpressed, coreleased, and acts together with GLP-1 and PYY in enteroendocrine control of metabolism. Endocrinology (2016) 157(1):176–94. doi: 10.1210/en.2015-1600
92. Rock SA, Jiang K, Wu Y, Liu Y, Li J, Weiss HL, et al. Neurotensin regulates proliferation and stem cell function in the small intestine in a nutrient-dependent manner. Cell Mol Gastroenterol Hepatol (2022) 13(2):501–16. doi: 10.1016/j.jcmgh.2021.09.006
93. Hauge M, Vestmar MA, Husted AS, Ekberg JP, Wright MJ, Di Salvo J, et al. GPR40 (FFAR1) - combined gs and gq signaling in vitro is associated with robust incretin secretagogue action ex vivo and in vivo. Mol Metab (2015) 4:3–14. doi: 10.1016/j.molmet.2014.10.002
94. Lain KY, Catalano PM. Metabolic changes in pregnancy. Clic Obstet Gynecol (2007) 50(4):938–48. doi: 10.1097/GRF.0b013e31815a5494
95. Rayan EA, Enns L. Role of gestational hormones in the induction of insulin resistance. J Clin Endocrinol Metab (1988) 67(2):341–7. doi: 10.1210/jcem-67-2-341
96. Miller BH, Olson SL, Turek FW, Levine JE, Horton TH, Takahashi JS. Circadian clock mutation disrupts estrous cyclicity and maintenance of pregnancy. Curr Biol (2004) 14:1367–73. doi: 10.1016/j.cub.2004.07.055
97. Perez S, Murias L, Fernandez-Plaza C, Diaz I, Gonzalez C, Otero J, et al. Evidence for clock genes circadian rhythms in human full-term placenta. Syst Biol Reprod Med (2015) 61:360–6. doi: 10.3109/19396368.2015.1069420
98. Vilches N, Spichiger C, Mendez N, Abarzua-Catalan L, Galdames HA, Hazlerigg DG, et al. Gestational chronodisruption impairs hippocampal expression of NMDA receptor subunits Grin1b/Grin3a and spatial memory in the adult offspring. PloS One (2014) 9:e91313. doi: 10.1371/journal.pone.0091313
99. Fernandez RC, Marino JL, Varcoe TJ, Davis S, Moran LJ, Rumbold AR, et al. Fixed or rotating night shift work undertaken by women: implications for fertility and miscarriage. Semin Reprod Med (2016) 34:74–82. doi: 10.1055/s-0036-1571354
100. Martin-Fairey CA, Zhao P, Wan L, Roenneberg T, Fay J, Ma X, et al. Pregnancy induces an earlier chronotype in both mice and women. J Biol Rhythms (2019) 34(3):323–31. doi: 10.1177/0748730419844650
101. Cooperstock M, England JE, Wolfe RA. Circadian incidence of premature rupture of the membranes in term and preterm births. Obstet Gynecol (1987) 69:936–41.
102. Viswanathan N, Davis FC. Timing of birth in Syrian hamsters. Biol Reprod (1992) 47:6–10. doi: 10.1095/biolreprod47.1.6
103. Reppert SM, Henshaw D, Schwartz WJ, Weaver DR. The circadian-gated timing of birth in rats: disruption by maternal SCN lesions or by removal of the fetal brain. Brain Res (1987) 403:398–402. doi: 10.1016/0006-8993(87)90084-9
104. Bonzini M, Palmer KT, Coggon D, Carugno M, Cromi A, Ferrario MM. Shift work and pregnancy outcomes: a systematic review with meta-analysis of currently available epidemiological studies. BJOG (2011) 118:1429–37. doi: 10.1111/j.1471-0528.2011.03066.x
105. Lin YC, Chen MH, Hsieh CJ, Chen PC. Effect of rotating shift work on childbearing and birth weight: a study of women working in a semiconductor manufacturing factory. World J Pediatr (2011) 7:129–35. doi: 10.1007/s12519-011-0265-9
106. Drucker DJ, Erlich P, Asa SL, Brubaker PL. Induction of intestinal epithelial proliferation by glucagon-like peptide 2. PNAS (1996) 93:7911–6. doi: 10.1073/pnas.93.15.7911
107. Ghatei MA, Goodlad RA, Taheri S, Mandir N, Brynes AE, Jordinson M, et al. Proglucagon-derived peptides in intestinal epithelial proliferation: glucagon-like peptide-2 is a major mediator of intestinal epithelial proliferation in rats. Digestive Dis Sci (2001) 46:1255–63. doi: 10.1023/A:1010615429639
108. Johnson ML, Saffrey MJ, Taylor VJ. Gastrointestinal capacity, gut hormones and appetite change during rat pregnancy and lactation. Reproduction (2019) 157(5):431–43. doi: 10.1530/REP-18-0414
109. Mann PE, Huynh K, Widmer G. Maternal high fat diet and its consequence on the gut microbiome: a rat model. Gut Microbes (2018) 9:143–54. doi: 10.1080/19490976.2017.1395122
110. Gomez G, Zhang T, Rajaraman S, Thakore KN, Yanaihara N, Townsend CM, et al. Intestinal peptide YY – ontogeny of gene-expression in rat bowel and trophic actions on rat and mouse bowel. Am J Physiol (1995) 268:G71–81. doi: 10.1152/ajpgi.1995.268.1.G71
111. Chandarana K, Gelegen C, Karra E, Choudhury AI, Drew ME, Fauveau V, et al. Diet and gastrointestinal bypass-induced weight loss: the roles of ghrelin and peptide YY. Diabetes (2011) 60:810–8. doi: 10.2337/db10-0566
112. leRoux CW, Borg C, Wallis K, Vincent RP, Bueter M, Goodlad R, et al. Gut hypertrophy after gastric bypass is associated with increased glucagon-like peptide 2 and intestinal crypt cell proliferation. Ann Surg (2010) 252:50–56114. doi: 10.1097/SLA.0b013e3181d3d21f
113. Zeng Z, Liu F, Li S. Metabolic adaptations in pregnancy: a review. Ann Nutr Metab (2017) 70:59–65. doi: 10.1159/000459633
114. Mosavat M, Omar SZ, Jamalpour S, Tan PC. Serum glucose-dependent insulinotropic polypeptide (GIP) and glucagon-like peptide-1 (GLP-1) in association with the risk of gestational diabetes: a prospective case-control study. J Diabetes Res (2020) 2020:9072492. doi: 10.1155/2020/9072492
115. Ørskou J, Kesmodel U, Henriksen TB, Secher NJ. An increasing proportion of infants weigh more than 4000 grams at birth. Acta Obstet Gynecol Scand (2001) 80(10):931–6. doi: 10.1034/j.1600-0412.2001.801010.x
116. Valsamakis G, Margeli A, Vitoratos N, Boutsiadis A, Sakkas EG, Papadimitriou G, et al. The role of maternal gut hormones in normal pregnancy: fasting plasma active glucagon-like peptide 1 level is a negative predictor of fetal abdomen circumference and maternal weight change. Eur J Endocrinol (2010) 162(5):897–903. doi: 10.1530/EJE-10-0047
117. Moffett RC, Vasu S, Thorens B, Drucker DJ, Flatt PR. Incretin receptor null mice reveal key role of GLP-1 but not GIP in pancreatic beta cell adaptation to pregnancy. PloS One (2014) 9(6):e96863. doi: 10.1371/journal.pone.0096863
118. Anini Y, Hansotia T, Brubaker PL. Muscarinic receptors control postprandial release of glucagon-like peptide-1: in vivo and in vitro studies in rats. Endocrinology (2002) 143(6):2420–6. doi: 10.1210/endo.143.6.8840
119. Johnston JD, Ordovás JM, Scheer FA, Turek FW. Circadian rhythms, metabolism, and chrononutrition in rodents and humans. Adv Nutr (2016) 7(2):399–406. doi: 10.3945/an.115.010777
120. Guerrero-Vargas NN, Espitia-Bautista E, Buijs RM, Escobar C. Shift-work: is time of eating determining metabolic health? evidence from animal models. Proc Nutr Soc (2018) 77(3):199–215. doi: 10.1017/S0029665117004128
121. Wharfe MD, Mark PJ, Wyrwoll CS, Smith JT, Yap C, Clarke MW, et al. Pregnancy-induced adaptations of the central circadian clock and maternal glucocorticoids. J Endocrinol (2016) 228(3):135–47. doi: 10.1530/JOE-15-0405
122. Gamble KL, Resuehr D, Johnson CH. Shift work and circadian dysregulation of reproduction. Front Endocrinol (Lausanne) (2013) 4:92. doi: 10.3389/fendo.2013.00092
123. Gallant A, Lundgren J, Drapeau V. Nutritional aspects of late eating and night eating. Curr Obes Rep (2014) 3(1):101–7. doi: 10.1007/s13679-013-0081-8
124. Ribas-Latre A, Eckel-Mahan K. Interdependence of nutrient metabolism and the circadian clock system: importance for metabolic health. Mol Metab (2016) 5(3):133–152. doi: 10.1016/j.molmet.2015.12.006
125. Loy SL, Loo RSX, Godfrey KM, Chong YS, Shek LP, Tan KH, et al. Chrononutrition during pregnancy: a review on maternal night- time eating. Nutrients (2020) 12(9):2783. doi: 10.3390/nu12092783
126. Varcoe TJ, Gatford KL, Kennaway DJ. Maternal circadian rhythms and the programming of adult health and disease. Am J Physiol Regul Integr Comp Physiol (2018) 314(2):R231–41. doi: 10.1152/ajpregu.00248.2017
127. Paoli A, Tinsley G, Bianco A, Moro T. The influence of meal frequency and timing on health in humans: the role of fasting. Nutrients (2019) 11(4):719. doi: 10.3390/nu11040719
128. Neba Ambe GNN, Breda C, Bhambra AS, Arroo RRJ. Effect of the citrus flavone nobiletin on circadian rhythms and metabolic syndrome. Molecules (2022) 27:7727. doi: 10.3390/molecules27227727
129. Liu F, Zhang X, Zhao B, Tan X, Wang L, Liu X. Role of food phytochemicals in the modulation of circadian clocks. J Agric Food Chem (2019) 67:8735–9. doi: 10.1021/acs.jafc.9b02263
130. Shinozaki A, Misawa K, Ikeda Y, Haraguchi A, Kamagata M, Tahara Y, et al. Potent effects of flavonoid nobiletin on amplitude, period, and phase of the circadian clock rhythm in PER2::LUCIFERASE mouse embryonic fibroblasts. PloS One (2017) 12:e0170904. doi: 10.1371/journal.pone.0170904
Keywords: enteroendocrine cells, circadian rhythm, hormone, intestine, pregnancy, human
Citation: Homeida AM, Homeida MA and Al-Suhaimi EA (2023) Circadian hormone secretion of enteroendocrine cells: implication on pregnancy status. Front. Endocrinol. 14:1106382. doi: 10.3389/fendo.2023.1106382
Received: 23 November 2022; Accepted: 18 April 2023;
Published: 10 May 2023.
Edited by:
Dionisio Pedro Amorim Neto, Campinas State University, BrazilReviewed by:
Anthony Hiu King Tsang, University of Cambridge, United KingdomPaulla Rodrigues, Ciência e Tecnologia de Rondônia - IFRO, Brazil
Copyright © 2023 Homeida, Homeida and Al-Suhaimi. This is an open-access article distributed under the terms of the Creative Commons Attribution License (CC BY). The use, distribution or reproduction in other forums is permitted, provided the original author(s) and the copyright owner(s) are credited and that the original publication in this journal is cited, in accordance with accepted academic practice. No use, distribution or reproduction is permitted which does not comply with these terms.
*Correspondence: Abdelgadir M. Homeida, aG9tZWlkYTUyQGhvdG1haWwuY29t