- 1Department of Structural and Functional Biology, Institute of Biology, University of Campinas, Campinas, SP, Brazil
- 2Obesity and Comorbidities Research Center, University of Campinas, Campinas, SP, Brazil
- 3Department of Physiology, Ribeirao Preto Medical School, University of Sao Paulo, Ribeirao Preto, SP, Brazil
Adipose tissue is an organ with metabolic and endocrine activity. White, brown and ectopic adipose tissues have different structure, location, and function. Adipose tissue regulates energy homeostasis, providing energy in nutrient-deficient conditions and storing it in high-supply conditions. To attend to the high demand for energy storage during obesity, the adipose tissue undergoes morphological, functional and molecular changes. Endoplasmic reticulum (ER) stress has been evidenced as a molecular hallmark of metabolic disorders. In this sense, the ER stress inhibitor tauroursodeoxycholic acid (TUDCA), a bile acid conjugated to taurine with chemical chaperone activity, has emerged as a therapeutic strategy to minimize adipose tissue dysfunction and metabolic alterations associated with obesity. In this review, we highlight the effects of TUDCA and receptors TGR5 and FXR on adipose tissue in the setting of obesity. TUDCA has been demonstrated to limit metabolic disturbs associated to obesity by inhibiting ER stress, inflammation, and apoptosis in adipocytes. The beneficial effect of TUDCA on perivascular adipose tissue (PVAT) function and adiponectin release may be related to cardiovascular protection in obesity, although more studies are needed to clarify the mechanisms. Therefore, TUDCA has emerged as a potential therapeutic strategy for obesity and comorbidities.
1 Introduction
Obesity is a global public health problem. Data from 2016 showed that about 1.9 billion adults worldwide were overweight, while 650 million were considered obese (1). Yet, studies indicate that, in 2025, almost 2.3 billion adults will be overweight; and more than 700 million will be obese (2). About public expenses with health, it is postulated that the costs of caring for obese patients will double by 2050 (US$10.1 billion) compared to 2010 (US$5.8 billion) (3). According to the Global Burden of Disease study, 4.7 million people died prematurely in 2017 as a result of obesity (4). Obesity is a risk factor for developing many disorders such as diabetes mellitus, hypertension, cardiovascular events, obstructive sleep apnea syndrome, cancer, and musculoskeletal diseases (5). Obesity also has a negative impact on quality of life and increases the costs of healthcare (6).
Adipose tissue is an endocrine organ with high metabolic activity. It corresponds to 20-28% of the body mass of healthy individuals and may represent up to 80% of the body mass in obese individuals (7). It is a specialized connective tissue composed predominantly by adipocytes and separated by a thin layer of extracellular matrix (8). It was believed that adipose tissue operated only as an energy store in the form of triglycerides, however, this tissue also works as an endocrine organ capable of secreting numerous hormones and adipokines that contribute to energy homeostasis (9). Besides these metabolism-related functions, adipose tissue regulates other physiological processes related to reproduction, immunity, angiogenesis, extracellular matrix restructuring, steroid metabolism, and body temperature (7).
White adipose tissue (WAT) is composed primarily of white and a few beige adipocytes, depending on its location. White adipocytes have a vacuole with a single lipid droplet and few cellular organelles and can vary in size according to the amount of stored triglycerides. Its main function is energy storage, but WAT also secretes a wide variety of adipokines, such as leptin and adiponectin, which are the first two proteins discovered (10). In addition, beige or brite adipocytes are generally found scattered amongst the white adipocytes and have the potential to generate heat when facing cold exposure or adrenergic receptors stimulation (11, 12). On the other hand, brown adipose tissue (BAT) adipocytes present several lipid droplets vacuolized and a large number of mitochondria, which gives the tissue a brown color. Its main function is the production of energy in the form of heat, a process accomplished by the uncoupling protein-1 (UCP-1), which is considered its phenotypic characteristic protein (10, 13).
Adipose tissues regulate energy homeostasis, providing energy in nutrient-deficient conditions and storing it in high-supply conditions (14). However, when the excess of stored energy exceeds the expenditure, obesity can be established (15). Therefore, studies involving the comprehension, prevention, and treatment of obesity are extremely important. Here, we review endoplasmic reticulum (ER) stress as a mechanism involved in adipose tissue morphological, functional and molecular changes on the setting of obesity highlighting recent advances in therapeutic actions of the tauroursodeoxycholic bile acid (TUDCA).
2 Changes in adipose tissue depots in obesity: A role for ER stress
2.1 ER stress
The ER is an important organelle of eukaryotic cells responsible for maintaining calcium homeostasis, for the biosynthesis of phospholipids and for the synthesis and folding of proteins that will be directed to the plasma membrane or to secretory vesicles (16). However, inflammation, nutrient deprivation, hypoxia, acidosis, and oxidative stress can disturb the homeostasis of ER leading to ER stress (17–19). ER stress is established when an imbalance occurs between the demand and the capacity of the ER for protein folding, resulting in the accumulation of misfolded/unfolded proteins. To normalize ER homeostasis and reestablish protein folding, cells rely on a defense mechanism called unfolded protein response (UPR) (20, 21).
UPR monitors protein folding in the ER and adjusts folding capacity to match the amount of synthesis. For this, three sensors that are present in the ER membrane are required: protein kinase R-like ER kinase (PERK), transcription factor 6 (ATF6), and inositol requiring enzyme 1α (IRE1α) (20, 21). Under physiological conditions, these sensors are bonded to a chaperone protein named as glucose-regulated protein 78 (GRP78) an ER chaperone also referred to as the immunoglobulin heavy chain binding protein (BiP), which keeps them inactivated. However, when levels of misfolded proteins increase, BiP dissociates from PERK, ATF6 and IRE1α activating three distinct signaling pathways (22). When BiP dissociates from IRE1α, IRE1α is autophosphorylated and activates the transcription factor X Box Protein 1 (XBP-1) that regulates the expression of chaperones and enzymes involved in the degradation of misfolded proteins arising from the ER (23, 24). PERK phosphorylates eukaryotic initiation factor 2 (eIF2α) and induces ATF4 translation, one of the UPR-dependent signaling proteins. In general, these pathways regulate protein synthesis rate, biosynthesis of new chaperones, protein trafficking within the ER, protein degradation, and finally, apoptosis, if ER homeostasis is not reestablished (20–22).
ER stress is linked to oxidative stress (23) as protein misfolding produces reactive oxygen species (ROS) (24, 25) that further impairs protein folding and depletes ER Ca2+ levels, aggravating ER stress (26, 27). In addition, IRE1α/XBP-1 and PERK/eIF2α induce the expression of C/EBP homologous protein (CHOP) that contribute to ROS generation and apoptosis (28).
2.2 ER stress in WAT and BAT during obesity
ER stress has been associated with dysfunctional WAT and BAT during obesity. In WAT, high-fat diet (HFD)-induced obesity enhances expression of UPR markers such as PERK, ATF-6, IRE1-α, ATF-4, and CHOP (29–31). Furthermore, ER stress affects adipokine secretion and action by impairing adiponectin synthesis and secretion (30, 31) and by inducing leptin resistance (32). Lipogenesis and adipogenesis are also modulated by ER stress (33). In line with this, activation of ER stress increases triglycerides, SREBP-1c, and FAS in human mature adipocytes (34). CHOP upregulation limits beige adipocyte differentiation by inhibiting UCP-1, Cox8b, Cidea, Prdm16, and PGC-1α (35), while CHOP deficiency upregulates PPARγ and adiponectin (29). IRE1α impedes beige fat activation in white and beige adipocytes, degrades PGC1-α mRNA, and limits thermogenesis (36).
During obesity, ER stress can be a mechanism potentiating proinflammatory state in WAT and UPR pathways converge with inflammatory signaling pathways (37, 38). CHOP deficiency enhances M2 macrophages abrogating WAT inflammation (29). In addition, IRE1α favors M1/M2 adipose tissue macrophages polarization and impairs WAT browning (30). Therefore, ER stress can modulate WAT adipocytes morphology and WAT inflammation in the setting of obesity.
In humans and in animal models of obesity, density and thermogenic activity of brown adipocytes is lower, as they go through a process called whitening, due but not limited to the excess of lipids (39, 40). Enlarged white-like brown adipocytes may present accumulation of large lipid droplets, mitochondrial dysfunction, and oxidative stress (41–44). The inflammatory profile triggered in BAT during obesity reduces the expression of UCP-1 and BAT thermogenic activity. Furthermore, inflammatory mediators have been shown to prevent the expansion of BAT in obesity, by promoting cell apoptosis via tumor necrosis factor-α (TNF-α) or reducing tissue proliferation, inhibiting catecholamine signaling (45).
In BAT of obese mice, genes related to ER stress and UPR such as GRP78, CHOP, ATF4 and ATF6 are enhanced while thermogenic genes UCP-1 and PGC1-α are reduced; induction of ER stress resulted in brown adipocytes apoptosis in vitro and in vivo (46). UCP-1 deficiency increases CHOP and XBP1 transcript levels and eIF2α phosphorylation, linking deficient thermogenesis to ER stress in BAT (47). However, the role of UPR response elements in BAT are still under investigation. The IRE1α/XBP1 pathway seem to be highly activated compared with other UPR branches during the induction of UCP-1 transcription in BAT, and its induction mechanism is independent of ER stress (48). Also, in brown adipocytes, PERK has a function independent of UPR, and seems to be essential for mitochondrial thermogenesis (49). Therefore, the role of ER stress in BAT in response to metabolic challenges as obesity still needs to be clarified.
2.3 Ectopic fat
In addition to classic fat depots, adipose tissue can accumulate ectopically during the development of obesity, which can contribute to impaired function in the deposited tissues (50), such as blood vessels and liver (51). In this sense, it is known that a progressive increase in the deposition of lipids in the liver leads to inflammation, hepatocellular degeneration, and collagen deposition, resulting in non-alcoholic steatohepatitis (NASH) (52), which, if not reversed, leads to irreversible liver cirrhosis and also increases the risk for the occurrence of hepatocellular carcinoma (53, 54). ER stress and UPR pathways are molecular mechanisms associated with NASH. CHOP, Bip, IRE1α and XBP1 are involved in hepatic lipid metabolism (55). In the livers of obese mice, ER stress associated with IRE1α and PERK activation, and CHOP upregulation activate the NLRP3 inflammasome and induce hepatocyte inflammation and apoptosis (56). Therefore, long-term ER stress leads can result in liver injury in obesity. The importance of ER stress to hepatic lipid metabolism in obesity and NASH was the topic of a recent review (57).
The obesity has also been demonstrated to alter the perivascular adipose tissue (PVAT) (58, 59). PVAT presents morphological characteristics that vary according to the vascular bed, resembling WAT in mesenteric arteries and abdominal aorta or BAT/beige in thoracic aorta (60–62). PVAT synthesizes and releases a variety of vasoactive substances that paracrinally influence peripheral vascular resistance and, therefore, blood pressure. PVAT may lose its vasoregulatory capacity due to a decrease in the release of vasodilating adipokines (63, 64). The expansion of PVAT in obesity is associated with immune cells infiltration, reduced adiponectin and increased leptin secretion, greater ROS production, and inducible nitric oxide synthase (iNOS) expression (63–67). In PVAT depots, ER stress is associated with the expression of pro-inflammatory factors including NF-kB, impaired vascular function (vasodilation and contraction) and atherosclerotic plaque destabilization (68, 69).
Given the metabolic and cardiovascular importance of PVAT and other ectopic fat depots, further studies need to be conducted in order to better elucidate the impact and pathophysiological of obesogenic diets and ER stress in ectopic fat. It is also relevant to identify pharmacological tools targeting ER stress in PVAT that could be a coadjuvant approach to the treatment and prevention of vascular complications associated with cardiometabolic diseases.
3 Actions of TUDCA in adiposity
3.1 Molecular mechanisms of TUDCA
Bile acids (BAs) are synthesized in hepatocytes by the enzyme cholesterol 7α-hydroxylase (CYP7A1) from cholesterol, generating primary BAs such as cholic acid (CA) and chenodeoxycholic acid (CDCA). In the intestine, through deconjugation, oxidation and epimerization reactions carried out by the intestinal microbiota, primary BAs give rise to secondary BAs such as deoxycholic acid (DCA), lithocholic acid (LCA) and ursodeoxycholic acid (UDCA). After the formation of secondary BAs, some BAs are conjugated in hepatocytes with the amino acids glycine as glycocholic acid (GCA), or taurine, as TUDCA, which makes these compounds obtain greater solubility and ionization in the intestinal lumen (70, 71).
At first, it was believed that BAs served only to assist in the process of digestion of lipids and fat-soluble vitamins (71, 72). However, recent data show that these molecules can regulate lipid and glycemic metabolism, BA synthesis, and the immune system, presenting metabolic and endocrine functions (71–73). These effects result from signaling through receptors such as the Farnesoid X receptor (FXR), the G protein-coupled BA receptor (TGR5) and Sphingosine-1-phosphate receptor 2 (S1PR2) (72–74). FXR is a nuclear receptor while TGR5 and S1PR2 are membrane receptors. These three receptors are classically expressed in the liver and digestive tract, but also have been identified in other tissues such as the heart, blood vessels, and adipocytes (75, 76). Additionally, preadipocytes and mature adipocytes express other factors involved in BA metabolism, such as BSEP (bile salt exporting pump), a hepatic protein that functions as a bile salt export pump in liver cells mediating BA transport into the bile canaliculi (77, 78). BAs conjugation with taurine increases affinity to the membrane and nuclear receptors (75).
Inhibition of ER stress could be a therapeutic intervention against morphofunctional alterations of overall adipose tissue depots in obesity. In this sense, the ER stress inhibitor TUDCA, a BA conjugated to taurine with chemical chaperone activity, has emerged as a therapeutic strategy to minimize adipose tissue dysfunction and metabolic alterations associated with obesity (79, 80).
3.2 Effects of TUDCA and its receptors in obesity
TUDCA was recently demonstrated to be an important mediator of beneficial metabolic effects on diet-induced obesity models, as by activating FXR and TGR5, taurine-conjugated BAs can improve glucose homeostasis, lipid metabolism and BAT thermogenesis (81, 82).
The activation of TGR5 increases energy expenditure in HFD-induced obese mice, limiting obesity and insulin resistance (83). TGR5 signals through cyclic adenosine monophosphate/protein kinase A (cAMP/PKA) and deiodinase-2 activation in mouse and human brown adipocytes and increased thermogenic activity (84–86). This pathway can also mediate beiging of WAT by increasing mitochondrial content and fission in white adipocytes from HFD mice (85). In BAT, TGR-5 induces thermogenesis (83). FXR activation can reduce both weight gain and inflammatory markers in WAT of HFD-induced obese mice, as well as limit the expansion of WAT under obesogenic conditions (87–89). Therefore, BA receptors activation seems to limit lipid accumulation, inflammation and metabolic changes in WAT during obesity. However, it was demonstrated that FXR-deficiency can attenuate WAT expansion, body weight and insulin resistance in mouse models of genetic and diet-induced obesity (90). Controversial data is also observed in ectopic fat as reduced or increased fat liver accumulation was observed following FXR activation (88, 89). Therefore, further studies are needed to clear the molecular mechanism of BA receptors in adipocytes during obesity.
Our group has demonstrated that TUDCA has a beneficial effect on glucose-induced insulin secretion through TGR5/PKA signaling in beta cells (91). Interestingly, increased insulin secretion is induced by TUDCA in isolated pancreatic islets under thapsigargin-induced ER stress (72). Therefore, it is likely that ER stress inhibition in beta cells is involved in TUDCA effects. In agreement, by modulating ER stress, administration of TUDCA in obese and type 2 diabetic mice normalized glycemia, restored insulin sensitivity in liver, muscle and adipose tissues, as well as reduced fatty liver disease (92). Of note, TUDCA may act as an insulin receptor (IR) agonist, which, in addition, can contribute to its beneficial effects on insulin sensitivity (93).
TUDCA treatment of human adipose derived stem cells (hASCs) significantly decreases ER stress marker GRP78, adipogenic markers such as PPARγ and glycerol-3-phosphate dehydrogenase 1 (GPDH), and lipid accumulation (94) suggesting that ER inhibition by TUDCA is associated with decreased adipogenesis. Such actions of TUDCA were similar to the ER stress inhibitor 4-phenyl butyric acid (PBA). Further, TUDCA or PBA treatment decreased ER stress markers, free cholesterol, inflammatory cytokines level, and NF-kB activity in WAT of HFD-induced obese mice (37). Finally, ER stress inhibition induced by TUDCA is associated with the inhibition of ROS production and attenuated cleaved-caspase-3 expression, resulting in an antiapoptotic effect in white adipocytes exposed to high glucose (95). In BAT, ER stress inhibition is associated with increased UCP-1 and thermogenesis (96, 97). The major effects of TUDCA on WAT and BAT are summarized in Figure 1.
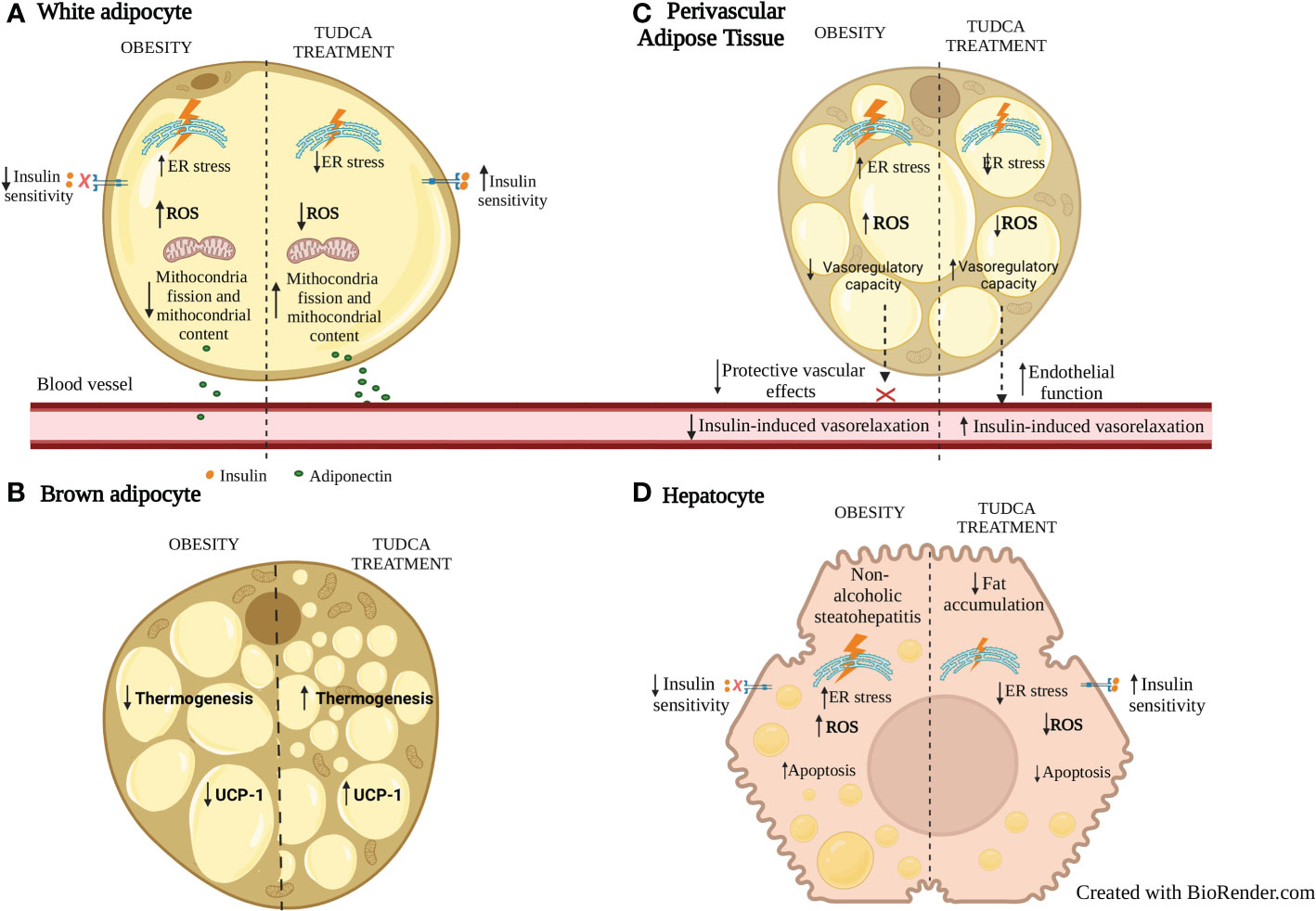
Figure 1 Effects of TUDCA treatment on WAT, BAT, PVAT and liver fat during obesity. (A) In WAT adipocytes, ER stress inhibition with TUDCA is associated with increased mitochondrial fission and content, decreased ROS, and increased insulin sensitivity and adiponectin secretion. (B) In BAT, TUDCA increases thermogenesis and expression of UCP-1. (C) In PVAT, ER stress inhibition with TUDCA is associated with decreased ROS, increased anticontractile and endothelial function. (D) In hepatocytes, in addition to inhibiting ER and oxidative stress, TUDCA treatment increases insulin sensitivity, decreases fat accumulation and adipocyte apoptosis. ER: endoplasmic reticulum; ROS: reactive oxygen species. UCP-1: uncoupling protein-1.
TUDCA treatment also has been suggested as beneficial for ectopic fat during obesity (Figure 1). Reduced ER stress markers PERK and IRE-1α phosphorylation is demonstrated in the liver of diabetic ob/ob mice associated with resolution of fat liver disease (92). TUDCA can limit hepatocyte lipoapoptosis by suppressing phosphorylation of eIF2α, XBP1 splicing, BiP and ATF4 expression (98). TUDCA may also have beneficial effects on PVAT. In line with this, TUDCA reduced ER stress markers GRP78 and ATF4 on PVAT from type 2 diabetic mice which was associated with improved endothelial function and reduced vascular stiffness (99). The ex vivo exposure to palmitate or thapsigargin both impaired insulin-induced vasorelaxation in the aorta which was prevented by TUDCA, associated with reduced expression of ER markers IRE1α and eIF-2α phosphorylation in PVAT (100). However, the mechanisms associated with TUDCA-induced PVAT mediated protective vascular effects still need to be addressed. As TUDCA increases adiponectin production in obese mice and in adipocytes (76, 101) this can be an additional beneficial mechanism associated with cardiovascular protection. Future studies need to be addressed to elucidate mechanisms of metabolic and vascular effects of TUDCA, focused on the involvement of ER stress inhibition and/or direct effects of this BA.
4 Conclusion
Here, we summarize the main findings on the effects of TUDCA and receptors TGR5 and FXR on adipose tissue in the setting of obesity. TUDCA has been demonstrated to limit metabolic disturbs associated with obesity by inhibiting lipid accumulation, ER stress, inflammation, and apoptosis in adipocytes. TUDCA also improves insulin sensitivity in obese mice and may act as an IR agonist. The beneficial effect of TUDCA on PVAT function and adiponectin release may be related to cardiovascular protection in obesity, although more studies are needed to clarify the mechanisms. A limitation of the studies is to identify possible direct effects of TUDCA independent of ER stress inhibition, which are interesting for elucidating possible therapeutic targets in obesity and other diseases.
As summarized in this review, TUDCA can be considered a multi-targeted therapy, as this BA modulates glucose and lipid metabolism, inflammatory response, adipogenesis, macrophage differentiation and other general metabolic responses. TUDCA has been approved by the US administration for clinical use in diseases such as cholelithiasis and cholestatic liver disease (102, 103). Therefore, it may be suggested that TUDCA treatment could be also an important therapeutic agent for obesity and comorbidities.
Author contributions
IF and AD contributed to the study conception, design and writing. JS, BA, TR, JC, and EC contributed to the writing and reviewing. KO made the figures. All authors contributed to the article and approved the submitted version.
Funding
This work was supported by Fundação de Amparo à Pesquisa do Estado de São Paulo- FAPESP (grant numbers 2018/26080-4, 2019/15164-5, 2020/05146-7 and 2021/02734-8) and Conselho Nacional de Desenvolvimento Científico e Tecnológico- CNPq (308682/2019-0).
Conflict of interest
The authors declare that the research was conducted in the absence of any commercial or financial relationships that could be construed as a potential conflict of interest.
Publisher’s note
All claims expressed in this article are solely those of the authors and do not necessarily represent those of their affiliated organizations, or those of the publisher, the editors and the reviewers. Any product that may be evaluated in this article, or claim that may be made by its manufacturer, is not guaranteed or endorsed by the publisher.
Abbreviations
ATF6, transcription factor 6; BAs, bile acid; BAT, brown adipose tissue; BiP, binding immunoglobulin protein; CA, cholic acid; CDCA, chenodeoxycholic acid; CIDEA; cell death-inducing DNA fragmentation factor alpha-like effector A; Cox8b, Cytochrome c oxidase subunit 8b; CYP7A1, cholesterol 7α-hydroxylase; DCA, deoxycholic acid; ER, endoplasmic reticulum; eIF2α, eukaryotic initiation factor 2 alpha; GPDH, glycerol-3-phosphate dehydrogenase; GRP78, glucose-regulated protein-78; hASCs, human adipose derived stem cells; iNOS, inducible nitric oxide synthase; IRE1α, inositol-requiring enzyme 1α; LCA, lithocholic acid; NASH, non-alcoholic steatohepatitis; NLRP3, NOD- LRR- and pyrin domain-containing protein 3; Pgc1a, Peroxisome proliferator-activated receptor-gamma coactivator; PPARγ, peroxisome proliferator-activated receptor γ; PVAT, perivascular adipose tissue; PERK, protein kinase R (PKR)-like endoplasmic reticulum kinase; ROS, reactive oxygen species; TUDCA, tauroursodeoxycholic acid; TNF-α, tumor necrosis factor-α; UCP-1, uncoupling protein-1; UDCA, ursodeoxycholic acid; UPR, unfolded protein response; WAT, white adipose tissue; XBP-1, X Box Protein 1; Cytochrome c oxidase subunit 8b (Cox8b); Peroxisome proliferator-activated receptor-gamma coactivator (Pgc1a); cell death-inducing DNA fragmentation factor alpha-like effector A (CIDEA).
References
2. World Health Organization. The State of Food Security and Nutrition in the World 2019: Safeguarding Against Economic Slowdowns and Downturns. Rome: Food and Agriculture Organization of the United Nations (FAO); (2019).
3. Rtveladze K, Marsh T, Webber L, Kilpi F, Levy D, Conde W, et al. Health and economic burden of obesity in Brazil. PloS One (2013) 8. doi: 10.1371/journal.pone.0068785
4. Dai H, Alsalhe TA, Chalghaf N, Riccò M, Bragazzi NL, Wu J. The global burden of disease attributable to high body mass index in 195 countries and territories, 1990-2017: An analysis of the global burden of disease study. PloS Med (2020) 17(7):e1003198. doi: 10.1371/journal.pmed.1003198
5. Singh GM, Danaei G, Farzadfar F, Stevens GA, Woodward M, Wormser D, et al. The age-specific quantitative effects of metabolic risk factors on cardiovascular diseases and diabetes: a pooled analysis. PloS One (2013) 8(7):e65174. doi: 10.1371/journal.pone.0065174
6. Kolotkin RL, Andersen JR. A systematic review of reviews: exploring the relationship between obesity, weight loss and health-related quality of life. Clin Obes (2017) 7(5):273–89. doi: 10.1111/cob.12203
7. Frigolet ME, Gutiérrez-Aguilar R. The colors of adipose tissue. Gac Med Mex (2020) 156:142–9. doi: 10.24875/GMM.M20000356
8. Bastard JP, Fève B. Physiology and physiopathology of adipose tissue. Springer-Verlag (2013) 2:1–437. doi: 10.1007/978-2-8178-0343-2
9. Wozniak SE, Gee LL, Wachtel MS, Frezza EE. Adipose tissue: The new endocrine organ? a review article. Dig Dis Sci (2009) 54:1847–56. doi: 10.1007/s10620-008-0585-3
10. Saely CH, Geiger K, Drexel H. Brown versus white adipose tissue: A mini-review. Gerontology (2011) 58:15–23. doi: 10.1159/000321319
11. Cinti S. Between brown and white: Novel aspects of adipocyte differentiation. Ann Med (2011) 43. doi: 10.3109/07853890.2010.535557
12. Unamuno X, Gómez-Ambrosi J, Rodríguez A, Becerril S, Frühbeck G, Catalán V. Adipokine dysregulation and adipose tissue inflammation in human obesity. Eur J Clin Invest (2018) 48(9):e12997. doi: 10.1111/eci.12997
13. Villarroya F, Cereijo R, Gavaldà-Navarro A, Villarroya J, Giralt M. Inflammation of brown/beige adipose tissues in obesity and metabolic disease. J Intern Med (2018) 284:492–504. doi: 10.1111/joim.12803
14. Choe SS, Huh JY, Hwang IJ, Kim JI, Kim JB. Adipose tissue remodeling: Its role in energy metabolism and metabolic disorders. Front Endocrinol (2016) 7:30. doi: 10.3389/fendo.2016.00030
15. Kahn CR, Wang G, Lee KY. Altered adipose tissue and adipocyte function in the pathogenesis of metabolic syndrome. J Clin Invest (2019) 129:3990–4000. doi: 10.1172/JCI129187
16. Lin JH, Walter P, Yen TS. Endoplasmic reticulum stress in disease pathogenesis. Annu Rev Pathol (2008) 3:399–425. doi: 10.1146/annurev.pathmechdis.3.121806.151434
17. Tarayre JP, Aliaga M, Barbara M, Tisné-Versailles J. Hypotheses on a possible role of some mediators in various inflammatory reactions on mouse ear. Methods Find Exp Clin Pharmacol (1988) 10(10):623–7.
18. Dong L, Krewson EA, Yang LV. Acidosis activates endoplasmic reticulum stress pathways through GPR4 in human vascular endothelial cells. Int J Mol Sci (2017) 18(2):278. doi: 10.3390/ijms18020278
19. Balsa E, Soustek MS, Thomas A, Cogliati S, García-Poyatos C, Martín-García E, et al. ER and nutrient stress promote assembly of respiratory chain supercomplexes through the PERK-eIF2α axis. Mol Cell (2019) 74(5):877–90.e6. doi: 10.1016/j.molcel.2019.03.031
20. Chadwick SR, Lajoie P. Endoplasmic reticulum stress coping mechanisms and lifespan regulation in health and diseases. Front Cell Dev Biol (2019) 7:84. doi: 10.3389/fcell.2019.00084
21. Chipurupalli S, Samavedam U, Robinson N. Crosstalk between ER stress, autophagy and inflammation. Front Med (2021) 8:758311. doi: 10.3389/fmed.2021.758311
22. Adams CJ, Kopp MC, Larburu N, Nowak PR, Ali MMU. Structure and molecular mechanism of ER stress signaling by the unfolded protein response signal activator IRE1. Front Mol Biosci (2019) 6:11. doi: 10.3389/fmolb.2019.00011
23. Chaudhari N, Talwar P, Parimisetty A, Lefebvre d’Hellencourt C, Ravanan P. A molecular web: endoplasmic reticulum stress, inflammation, and oxidative stress. Front Cell Neurosci (2014) 8:213. doi: 10.3389/fncel.2014.00213
24. Zeeshan HM, Lee GH, Kim HR, Chae HJ. Endoplasmic reticulum stress and associated ROS. Int J Mol Sci (2016) 17:327. doi: 10.3390/ijms17030327
25. Benjamin P, Jonathan S. Weissman; oxidative protein folding in eukaryotes: mechanisms and consequences. J Cell Biol (2004) 164(3):341–6. doi: 10.1083/jcb.200311055
26. Malhi H, Kaufman RJ. Endoplasmic reticulum stress in liver disease. J Hepatol (2011) 54(4):795–809. doi: 10.1016/j.jhep.2010.11.005
27. Bhandary B, Marahatta A, Kim HR, Chae HJ. An involvement of oxidative stress in endoplasmic reticulum stress and its associated diseases. Int J Mol Sci (2013) 14:434–56. doi: 10.3390/ijms14010434
28. Oyadomari S, Mori M. Roles of CHOP/GADD153 in endoplasmic reticulum stress. Cell Death Differ (2004) 11(4):381–9. doi: 10.1038/sj.cdd.4401373
29. Suzuki T, Gao J, Ishigaki Y, Kondo K, Sawada S, Izumi T, et al. ER stress protein CHOP mediates insulin resistance by modulating adipose tissue macrophage polarity. Cell Rep (2017) 18(8):2045–57. doi: 10.1016/j.celrep.2017.01.076
30. Shan B, Wang X, Wu Y, Xu C, Xia Z, Dai J, et al. The metabolic ER stress sensor IRE1α suppresses alternative activation of macrophages and impairs energy expenditure in obesity. Nat Immunol (2017) 18(5):519–29. doi: 10.1038/ni.3709
31. Torre-Villalvazo I, Bunt AE, Alemán G, Marquez-Mota CC, Diaz-Villaseñor A, Noriega LG, et al. Adiponectin synthesis and secretion by subcutaneous adipose tissue is impaired during obesity by endoplasmic reticulum stress. J Cell Biochem (2018) 119(7):5970–84. doi: 10.1002/jcb.26794
32. Hosoi T, Sasaki M, Miyahara T, Hashimoto C, Matsuo S, Yoshii M, et al. Endoplasmic reticulum stress induces leptin resistance. Mol Pharmacol (2008) 74(6):1610–9. doi: 10.1124/mol.108.050070
33. Menikdiwela KR, Guimarães JP, Ramalingam L, Kalupahana N, Dufour JM, Washburn L, et al. Mechanisms linking endoplasmic reticulum (ER) stress and microRNAs to adipose tissue dysfunction in obesity. Crit Rev Biochem Mol Biol (2021) 56:5. doi: 10.1080/10409238.2021.1925219
34. Zhu W, Niu X, Wang M, Li Z, Jiang HK, Li C, et al. Endoplasmic reticulum stress may be involved in insulin resistance and lipid metabolism disorders of the white adipose tissues induced by high-fat diet containing industrial trans-fatty acids. Diabetes Metab Syndr Obes (2019) 29:12. doi: 10.2147/DMSO.S218336
35. Lee JM, Park S, Lee D, Ginting RP, Lee MR, Lee MW, et al. Reduction in endoplasmic reticulum stress activates beige adipocytes differentiation and alleviates high fat diet-induced metabolic phenotypes. Biochim Biophys Acta Mol Basis Dis (2021) 1867(5):166099. doi: 10.1016/j.bbadis.2021.166099
36. Chen Y, Wu Z, Huang S, Wang X, He S, Liu L, et al. Adipocyte IRE1α promotes PGC1α mRNA decay and restrains adaptive thermogenesis. Nat Metab (2022) 4:1166–84. doi: 10.1038/s42255-022-00631-8
37. Chen Y, Wu Z, Zhao S, Xiang R. Chemical chaperones reduce ER stress and adipose tissue inflammation in high fat diet-induced mouse model of obesity. Sci Rep (2016) 6:1–8. doi: 10.1038/srep27486
38. Kawasaki N, Asada R, Saito A, Kanemoto S, Imaizumi K. Obesity-induced endoplasmic reticulum stress causes chronic inflammation in adipose tissue. Sci Rep (2012) 2:1–7. doi: 10.1038/srep00799
39. McGregor RA, Kwon EY, Shin SK, Jung UJ, Kim E, Park JHY, et al. Time-course microarrays reveal modulation of developmental, lipid metabolism and immune gene networks in intrascapular brown adipose tissue during the development of diet-induced obesity. Int J Obes (2013) 37:1524–31. doi: 10.1038/ijo.2013.52
40. Vitali A, Murano I, Zingaretti MC, Frontini A, Ricquier D, Cinti S. The adipose organ of obesity-prone C57BL/6J mice is composed of mixed white and brown adipocytes. J Lipid Res (2012) 53(4):619–29. doi: 10.1194/jlr.M018846
41. Stephens M, Ludgate M, Rees DA. Brown fat and obesity: the next big thing? Clin Endocrinol (2011) 74(6):661–70. doi: 10.1111/j.1365-2265.2011
42. Bournat JC, Brown CW. Mitochondrial dysfunction in obesity. Curr Opin Endocrinol Diabetes Obes (2010) 17(5):446–52. doi: 10.1097/MED.0b013e32833c3026
43. Shimizu I, Walsh K. The whitening of brown fat and its implications for weight management in obesity. Curr Obes Rep (2015) 4:224–9. doi: 10.1007/s13679-015-0157-8
44. Gao P, Jiang Y, Wu H, Sun F, Li Y, He H, et al. Inhibition of mitochondrial calcium overload by SIRT3 prevents obesity- or age-related whitening of brown adipose tissue. Diabetes (2020) 2:165–80. doi: 10.2337/db19-0526
45. Alcalá M, Calderon-Dominguez M, Serra D, Herrero L, Viana M. Mechanisms of impaired brown adipose tissue recruitment in obesity. Front Physiol (2019) 10:94. doi: 10.3389/fphys.2019.00094
46. Liu Z, Gu H, Gan L, Xu Y, Feng F, Saeed M, et al. Reducing Smad3/ATF4 was essential for Sirt1 inhibiting ER stress-induced apoptosis in mice brown adipose tissue. Oncotarget (2017) 8:9267–79. doi: 10.18632/oncotarget.14035
47. Bond LM, Burhans MS, Ntambi JM. Uncoupling protein-1 deficiency promotes brown adipose tissue inflammation and ER stress. PloS One (2018) 13(11):e0205726. doi: 10.1371/journal.pone.0205726
48. Asada R, Kanemoto S, Matsuhisa K, Hino K, Cui M, Cui X, et al. IRE1α-XBP1 is a novel branch in the transcriptional regulation of Ucp1 in brown adipocytes. Sci Rep (2015) 5:16580. doi: 10.1038/srep16580
49. Kato H, Okabe K, Miyake M, Hattori K, Fukaya T, Tanimoto K, et al. ER-resident sensor PERK is essential for mitochondrial thermogenesis in brown adipose tissue. Life Sci Alliance (2020) 3(3):e201900576. doi: 10.26508/lsa.201900576
50. Ferrara D, Montecucco F, Dallegri F, Carbone F. Impact of different ectopic fat depots on cardiovascular and metabolic diseases. J Cell Physiol (2019) 234:21630–41. doi: 10.1002/jcp.28821
51. Camporez JP, Wang Y, Faarkrog K, Chukijrungroat N, Petersen KF, Shulman GI. Mechanism by which arylamine n-acetyltransferase 1 ablation causes insulin resistance in mice. Proc Natl Acad Sci U.S.A. (2017) 114(52):E11285–92. doi: 10.1073/pnas.1716990115
52. Camargo FN, Matos SL, Araujo LCC, Carvalho CRO, Amaral AG, Camporez JP. Western Diet-fed ApoE knockout Male mice as an experimental model of non-alcoholic steatohepatitis. Curr Issues Mol Biol (2022) 44(10):4692–703. doi: 10.3390/cimb44100320
53. Arab JP, Arrese M, Trauner M. Recent insights into the pathogenesis of nonalcoholic fatty liver disease. Annu Rev Pathol Mech Dis (2018) 13:321–50. doi: 10.1146/annurev-pathol-020117-043617
54. Buzzetti E, Pinzani M, Tsochatzis EA. The multiple-hit pathogenesis of non-alcoholic fatty liver disease (NAFLD). Metabolism (2016) 65:1038–48. doi: 10.1016/j.metabol.2015.12.012
55. Lee AH, Scapa EF, Cohen DE, Glimcher LH. Regulation of hepatic lipogenesis by the transcription factor XBP1. Science (2008) 320(5882):1492–6. doi: 10.1126/science.1158042
56. Lebeaupin C, Proics E, de Bieville CH, Rousseau D, Bonnafous S, Patouraux S, et al. ER stress induces NLRP3 inflammasome activation and hepatocyte death. Cell Death Dis (2015) 6(9):e1879. doi: 10.1038/cddis.2015.248
57. Zheng W, Sun Q, Li L, Cheng Y, Chen Y, Lv M, et al. Role of endoplasmic reticulum stress in hepatic glucose and lipid metabolism and therapeutic strategies for metabolic liver disease. Int Immunopharmacol (2022) 113(Pt B):109458. doi: 10.1016/j.intimp.2022.109458
58. Chang L, Milton H, Eitzman DT, Eugene Chen Y. Paradoxical roles of perivascular adipose tissue in atherosclerosis and hypertension. Circ J (2013) 77:11–8. doi: 10.1253/circj.CJ-12-1393
59. Victorio JA, Guizoni DM, Freitas IN, Araujo TR, Davel AP. Effects of high-fat and high-Fat/High-Sucrose diet-induced obesity on PVAT modulation of vascular function in Male and female mice. Front Pharmacol (2021) 10:720224. doi: 10.3389/fphar.2021.720224
60. Fitzgibbons TP, Kogan S, Aouadi M, Hendricks GM, Straubhaar J, Czech MP. Similarity of mouse perivascular and brown adipose tissues and their resistance to diet-induced inflammation. Am J Physiol - Hear Circ Physiol (2011) 301(4):H1425–37. doi: 10.1152/ajpheart.00376.2011
61. Police SB, Thatcher SE, Charnigo R, Daugherty A, Cassis LA. Obesity promotes inflammation in periaortic adipose tissue and angiotensin ii-induced abdominal aortic aneurysm formation. Arterioscler Thromb Vasc Biol (2009) 29. doi: 10.1161/ATVBAHA.109.192658
62. Padilla J, Jenkins NT, Vieira-Potter VJ, Harold Laughlin M. Divergent phenotype of rat thoracic and abdominal perivascular adipose tissues. Am J Physiol - Regul Integr Comp Physiol (2013) 304(7):R543–52. doi: 10.1152/ajpregu.00567.2012
63. Aghamohammadzadeh R, Unwin RD, Greenstein AS, Heagerty AM. Effects of obesity on perivascular adipose tissue vasorelaxant function: Nitric oxide, inflammation and elevated systemic blood pressure. J Vasc Res (2016) 52:299–305. doi: 10.1159/000443885
64. Greenstein AS, Khavandi K, Withers SB, Sonoyama K, Clancy O, Jeziorska M, et al. Local inflammation and hypoxia abolish the protective anticontractile properties of perivascular fat in obese patients. Circulation (2009) 119:1661–70. doi: 10.1161/CIRCULATIONAHA.108.821181
65. Gómez-Hernández A, Beneit N, Díaz-Castroverde S, Escribano Ó. Differential role of adipose tissues in obesity and related metabolic and vascular complications. Int J Endocrinol (2016). doi: 10.1155/2016/1216783
66. Araujo HN, Victorio JA, Valgas da Silva CP, Sponton ACS, Vettorazzi JF, de Moraes C, et al. Anti-contractile effects of perivascular adipose tissue in thoracic aorta from rats fed a high-fat diet: role of aerobic exercise training. Clin Exp Pharmacol Physiol (2018) 45(3):293–302. doi: 10.1111/1440-1681.12882
67. Victorio JA, Davel AP. Perivascular adipose tissue oxidative stress on the pathophysiology of cardiometabolic diseases. Curr Hypertens Rev (2020) 16(3):192–200. doi: 10.2174/1573402115666190410153634
68. Wang M, Xing J, Liu M, Gao M, Liu Y, Li X, et al. Deletion of seipin attenuates vascular function and the anticontractile effect of perivascular adipose tissue. Front Cardiovasc Med (2021) 8:706924. doi: 10.3389/fcvm.2021.706924
69. Ying R, Li SW, Chen JY, Zhang HF, Yang Y, Gu ZJ, et al. Endoplasmic reticulum stress in perivascular adipose tissue promotes destabilization of atherosclerotic plaque by regulating GM-CSF paracrine. J Transl Med (2018) 16(1):105. doi: 10.1186/s12967-018-1481-z
70. Ackerman HD, Gerhard GS. Bile acids in neurodegenerative disorders. Front Aging Neurosci (2016) 8:263. doi: 10.3389/fnagi.2016.00263
71. Hylemon PB, Zhou H, Pandak WM, Ren S, Gil G, Dent P. Bile acids as regulatory molecules. J Lipid Res (2009) 50:1509–20. doi: 10.1194/jlr.R900007-JLR200
72. Lee YY, Hong SH, Lee YJ, Chung SS, Jung HS, Park SG, et al. Tauroursodeoxycholate (TUDCA), chemical chaperone, enhances function of islets by reducing ER stress. Biochem Biophys Res Commun (2010) 397(4):735–9. doi: 10.1016/j.bbrc.2010.06.022
73. Kawamata Y, Fujii R, Hosoya M, Harada M, Yoshida H, Miwa M, et al. A G protein-coupled receptor responsive to bile acids. J Biol Chem (2003) 278:9435–40. doi: 10.1074/jbc.M209706200
74. Hanafi NI, Mohamed AS, Kadir SHSA, Othman MHD. Overview of bile acids signaling and perspective on the signal of ursodeoxycholic acid, the most hydrophilic bile acid, in the heart. Biomolecules (2018) 8(4):159. doi: 10.3390/biom8040159
75. Wan YJY, Sheng L. Regulation of bile acid receptor activity. Liver Res (2018) 2(4):180–5. doi: 10.1016/j.livres.2018.09.008
76. Schmid A, Schlegel J, Thomalla M, Karrasch T, Schäffler A. Evidence of functional bile acid signaling pathways in adipocytes. Mol Cell Endocrinol (2019) 483:1–10. doi: 10.1016/j.mce.2018.12.006
77. Hayashi H, Sugiyama Y. 4-phenylbutyrate enhances the cell surface expression and the transport capacity of wild-type and mutated bile salt export pumps. Hepatology (2007) 45:1506–16. doi: 10.1002/hep.21630
78. Telbisz Á, Homolya L. Recent advances in the exploration of the bile salt export pump (BSEP/ABCB11) function. Expert Opin Ther Targets (2016) 20:501–14. doi: 10.1517/14728222.2016.1102889
79. Yang JS, Kim JT, Jeon J, Park HS, Kang GH, Park KS, et al. Changes in hepatic gene expression upon oral administration of taurine-conjugated ursodeoxycholic acid in ob/ob mice. PloS One (2010) 5:1–10. doi: 10.1371/journal.pone.0013858
80. Kusaczuk M. Tauroursodeoxycholate-bile acid with chaperoning activity: Molecular and cellular effects and therapeutic perspectives. Cells (2019) 8(12):1471. doi: 10.3390/cells8121471
81. Dos Reis AT, Roberta Rodrigues MM, Lourençoni AB, Monali Barreto Dos SL, Fernandes BM, Alves da SJJ, et al. Tauroursodeoxycholic acid improves glucose tolerance and reduces adiposity in normal protein and malnourished mice fed a high-fat diet. Food Res Int (2022) 156:111331. doi: 10.1016/j.foodres.2022.111331
82. Münzker J, Haase N, Till A, Sucher R, Haange SB, Nemetschke L, et al. Functional changes of the gastric bypass microbiota reactivate thermogenic adipose tissue and systemic glucose control via intestinal FXR-TGR5 crosstalk in diet-induced obesity. Microbiome (2022) 10(1):96. doi: 10.1186/s40168-022-01264-5
83. Watanabe M, Houten SM, Mataki C, Christoffolete MA, Kim BW, Sato H, et al. Bile acids induce energy expenditure by promoting intracellular thyroid hormone activation. Nature (2006) 439(7075):484–9. doi: 10.1038/nature04330
84. Broeders EPM, Nascimento EBM, Havekes B, Brans B, Roumans KHM, Tailleux A, et al. The bile acid chenodeoxycholic acid increases human brown adipose tissue activity. Cell Metab (2015) 22(3):418–26. doi: 10.1016/j.cmet.2015.07.002
85. Velazquez-Villegas LA, Perino A, Lemos V, Zietak M, Nomura M, Pols TWH, et al. TGR5 signalling promotes mitochondrial fission and beige remodelling of white adipose tissue. Nat Commun (2018) 9(1):245. doi: 10.1038/s41467-017-02068-0
86. Saito M, Matsushita M, Yoneshiro T, Okamatsu-Ogura Y. Brown adipose tissue, diet-induced thermogenesis, and thermogenic food ingredients: From mice to men. Front Endocrinol (Lausanne) (2020) 11:222. doi: 10.3389/fendo.2020.00222
87. McIlvride S, Nikolova V, Fan HM, McDonald JAK, Wahlström A, Bellafante E, et al. Obeticholic acid ameliorates dyslipidemia but not glucose tolerance in mouse model of gestational diabetes. Am J Physiol Endocrinol Metab (2019) 317(2):E399–410. doi: 10.1152/ajpendo.00407.2018
88. van Zutphen T, Stroeve JHM, Yang J, Bloks VW, Jurdzinski A, Roelofsen H, et al. FXR overexpression alters adipose tissue architecture in mice and limits its storage capacity leading to metabolic derangements. J Lipid Res (2019) 60(9):1547–61. doi: 10.1194/jlr.M094508
89. Haczeyni F, Poekes L, Wang H, Mridha AR, Barn V, Geoffrey Haigh W, et al. Obeticholic acid improves adipose morphometry and inflammation and reduces steatosis in dietary but not metabolic obesity in mice. Obesity (2017) 25(1):155–65. doi: 10.1002/oby.21701
90. Prawitt J, Abdelkarim M, Stroeve JH, Popescu I, Duez H, Velagapudi VR, et al. Farnesoid X receptor deficiency improves glucose homeostasis in mouse models of obesity. Diabetes (2011) 60(7):1861–71. doi: 10.2337/db11-0030
91. Vettorazzi JF, Ribeiro RA, Borck PC, Branco RC, Soriano S, Merino B, et al. The bile acid TUDCA increases glucose-induced insulin secretion via the cAMP/PKA pathway in pancreatic beta cells. Metabolism (2016) 65(3):54–63. doi: 10.1016/j.metabol.2015.10.021
92. Ozcan U, Yilmaz E, Ozcan L, Furuhashi M, Vaillancourt E, Smith RO, et al. Chemical chaperones reduce ER stress and restore glucose homeostasis in a mouse model of type 2 diabetes. Science (2006) 313:1137–40. doi: 10.1126/science.1128294
93. da Silva JA Jr, Figueiredo LS, Chaves JO, Oliveira KM, Carneiro EM, Abreu PA, et al. Effects of tauroursodeoxycholic acid on glucose homeostasis: Potential binding of this bile acid with the insulin receptor. Life Sci (2021) 285:120020. doi: 10.1016/j.lfs.2021.120020
94. Cha BH, Kim JS, Chan Ahn J, Kim HC, Kim BS, Han DK, et al. The role of tauroursodeoxycholic acid on adipogenesis of human adipose-derived stem cells by modulation of ER stress. Biomaterials (2014) 35:2851–8. doi: 10.1016/j.biomaterials.2013.12.067
95. Li A, Zhang S, Li J, Liu K, Huang F, Liu B. Metformin and resveratrol inhibit Drp1-mediated mitochondrial fission and prevent ER stress-associated NLRP3 inflammasome activation in the adipose tissue of diabetic mice. Mol Cell Endocrinol (2016) 434:36–47. doi: 10.1016/j.mce.2016.06.008
96. Contreras C, Fondevila MF, López M. Hypothalamic GRP78, a new target against obesity? Adipocyte (2018) 7(1):63–6. doi: 10.1080/21623945.2017.1405878
97. Jeong HW, Choi RH, Koh HJ. Obesity-induced TRB3 negatively regulates brown adipose tissue function in mice. Biochem Biophys Res Commun (2021) 547:29–35. doi: 10.1016/j.bbrc.2021.01.103
98. Cho EJ, Yoon JH, Kwak MS, Jang ES, Lee JH, Yu SJ, et al. Tauroursodeoxycholic acid attenuates progression of steatohepatitis in mice fed a methionine-choline-deficient diet. Dig Dis Sci (2014) 59(7):1461–74. doi: 10.1007/s10620-014-3217-0
99. Battson ML, Lee DM, Jarrell DK, Hou S, Ecton KE, Phan AB, et al. Tauroursodeoxycholic acid reduces arterial stiffness and improves endothelial dysfunction in type 2 diabetic mice. J Vasc Res (2017) 54(5):280–7. doi: 10.1159/000479967
100. Xu X, Chen Y, Song J, Hou F, Ma X, Liu B, et al. Mangiferin suppresses endoplasmic reticulum stress in perivascular adipose tissue and prevents insulin resistance in the endothelium. Eur J Nutr (2018) 57(4):1563–75. doi: 10.1007/s00394-017-1441-z
101. Vettorazzi JF, Kurauti MA, Soares GM, Borck PC, Ferreira SM, Branco RCS, et al. Bile acid TUDCA improves insulin clearance by increasing the expression of insulin-degrading enzyme in the liver of obese mice. Sci Rep (2017) 7(1):14876. doi: 10.1038/s41598-017-13974-0
102. Hetz C, Zhang K, Kaufman RJ. Mechanisms, regulation and functions of the unfolded protein response. Nat Rev Mol Cell Biol (2020) 21:421–38. doi: 10.1038/s41580-020-0250-z
Keywords: TUDCA, tauroursodeoxycholic acid, obesity, endoplasmic reticulum (ER) stress, adipose tissue, perivascular adipose tissue, adipocyte
Citation: Freitas IN, da Silva JA Jr, Oliveira KM, Lourençoni Alves B, Dos Reis Araújo T, Camporez JP, Carneiro EM and Davel AP (2023) Insights by which TUDCA is a potential therapy against adiposity. Front. Endocrinol. 14:1090039. doi: 10.3389/fendo.2023.1090039
Received: 04 November 2022; Accepted: 10 February 2023;
Published: 21 February 2023.
Edited by:
Bruno Melo Carvalho, Universidade de Pernambuco, BrazilReviewed by:
Elisa Villalobos, Newcastle University, United KingdomXavier Prieur, U1087 Institut du Thorax (INSERM), France
Copyright © 2023 Freitas, da Silva Jr, Oliveira, Lourençoni Alves, Dos Reis Araújo, Camporez, Carneiro and Davel. This is an open-access article distributed under the terms of the Creative Commons Attribution License (CC BY). The use, distribution or reproduction in other forums is permitted, provided the original author(s) and the copyright owner(s) are credited and that the original publication in this journal is cited, in accordance with accepted academic practice. No use, distribution or reproduction is permitted which does not comply with these terms.
*Correspondence: Ana Paula Davel, YW5hZGF2ZWxAdW5pY2FtcC5icg==