- 1Department of Pharmacology and Nutritional Sciences, University of Kentucky, Lexington, KY, United States
- 2Human Movement Sciences, Darden College of Education, Old Dominion University, Norfolk, VA, United States
Polychlorinated biphenyls (PCBs) are persistent environmental organic pollutants known to have detrimental health effects. Using a mouse model, we previously demonstrated that PCB126 exposure before and during pregnancy and throughout the perinatal period adversely affected offspring glucose tolerance and/or body composition profiles. The purpose of this study was to investigate the glucose tolerance and body composition of offspring born to dams exposed to PCB126 during the nursing period only. Female ICR mice were bred, and half of the dams were exposed to either vehicle (safflower oil) or 1 µmole PCB126 per kg of body weight via oral gavage on postnatal days (PND) 3, 10, and 17 (n = 9 per group). Offspring body weight, lean and fat mass, and glucose tolerance were recorded every three weeks. PCB126 treatment did not alter dam nor offspring body weight (p > 0.05). PCB126-exposed male and female offspring displayed normal body composition (p > 0.05) relative to vehicle-exposed offspring. However, both male and female offspring that were exposed to PCB126 during the nursing period had significantly impaired glucose tolerance at 3 and 9 weeks of age (p < 0.05). At 6 and 12 weeks of age, no impairments in glucose tolerance existed in offspring (p > 0.05). Our current study demonstrates that exposure to PCB126 through the mother’s milk does not affect short- or long-term body composition but impairs glucose tolerance in the short-term.
1 Introduction
Polychlorinated biphenyls (PCBs) are a class of chlorinated hydrocarbons that were once widely manufactured for use in numerous industrial and commercial products because of their chemical stability, heat resistance, and electrical insulating properties (1). Upon accumulating evidence demonstrating the detrimental effects PCB exposure had on health, the United States banned the production of PCBs in the late 1970s (2). Despite cessation in production, PCBs continue to pose a threat to human health due to their persistent environmental presence (3). PCBs are ubiquitous and are found in almost every medium within the environment despite the fact that there are no known natural sources of the toxic compounds (4–7). Routes of PCB exposure include dermal contact, inhalation, and ingestion (3). The environmental fates of PCBs are largely determined by their chlorination pattern (6). Over 200 congeners exist and differ by their number and placement of chlorine groups on the phenyl rings. The number of chlorine group attachments and their substitutions influence the toxicity and persistence of the PCB mixtures (8). Non-ortho substituted PCBs or coplanar PCBs are more resistant to biodegradation and biotransformation (6). Thus, the introduction of such PCBs to our ecosystems through environmental uptake results in biomagnification as PCBs accumulate in adipose tissue (6, 8).
Coplanar PCBs are known as ‘dioxin-like’ PCBs because they activate the aryl hydrocarbon receptor (AhR) (9), the receptor responsible for initiating the biotransformation of many polyhalogenated xenobiotics. PCB126 (3, 3’, 4, 4’, 5-pentachlorobiphenyl) is the most potent AhR agonist among the PCB family (10). Observed epidemiologic associations between PCB exposure and diabetes prevalence (11–13), incidence (14–16), and risk (17–19) have been corroborated by laboratory investigations. Specifically, coplanar PCB exposure has been demonstrated to promote obesity (20–22), increase insulin resistance (21, 22), and impair glucose tolerance (23–26).
Further, PCBs can transfer from mother to child during the perinatal period by crossing the placenta as well as entering breastmilk (27). Because of this, understanding how early-life PCB exposure affects the developing fetus and infant is of paramount significance. The Developmental Origins of Health and Disease hypothesis, derived from the Barker hypothesis, postulates that early-life stressors influence health outcomes observed in later life (28). Research documents that the programming of disease can occur before birth and in the early postnatal period (29). However, literature scantly details the contribution of early-life PCB exposures to diabetic-like phenotypes observed in offspring. Specifically, offspring exposed to PCB126 during gestation and nursing exhibit sex-specific alterations in body composition (30). While likely acting through different mechanisms than PCB126, PCB153 exposure during gestation and lactation caused sex-specific alterations in metabolic parameters in offspring (31). PCB126 exposure during gestation results in long-lasting alterations in offspring body weight, body composition, and glucose tolerance (32). Investigation of embryonic and fetal PCB126 exposures demonstrates the ability of the toxicant to modify beta cell development (33) and the profiles of pro-inflammatory cytokines and hormones implicated in glucose regulation (34). In the current study, we exposed dams to PCB126 during the nursing period in an effort to delineate how nursing PCB126 exposures influence body composition and glucose homeostasis in offspring. Based on our previous studies of perinatal PCB126 exposure (30), we hypothesized that PCB exposure via nursing during early postnatal life will unfavorably alter offspring body composition and therefore impair offspring glucose tolerance.
1.1 Animals, breeding, and PCB treatment
Ten-week-old male and female ICR mice (Envigo, Indianapolis, IN) were housed in a pathogen-free environment in NextGen 500 cages (Allentown Inc., Allentown, NJ) under a 14:10 light/dark cycle, with temperatures ranging from 20 - 22.2˚ Celsius, and humidity ranging from 30% to 70%. The ICR stock was selected based on its exceptional reproductive abilities, extensive use in toxicological and pharmacological studies, and our previous characterizations of maternal PCB126 exposure effects on offspring glucose tolerance and body composition (30, 32). Female mice were singly housed for six days prior to mating. Each female mouse was exposed to male bedding (Sani-Chip Bedding, Teklad 7115, Envigo, Indianapolis, IN) for two days before being allowed to mate. One male mouse was placed in a cage with two female mice for 24 hours. Dams were allowed to spontaneously deliver. Litters were culled to four pups (two pups per sex) on postnatal day (PND) 1.
On PNDs 3, 10, and 17, dams were administered either tocopherol stripped safflower oil (vehicle) (Dyets #403952, Bethlehem, PA), or one micromole of PCB126 per kilogram body weight (AccuStandard Inc., New Haven, CT) dissolved in vehicle via oral gavage (n = 9 mice per treatment group). As is typical of developmental programming studies, offspring sample size indicates the number of dams/litters. Dams were selected and assigned to experimental groups according to the date of birth in order to match vehicle and PCB treatment groups as evenly as possible. Offspring exposure to vehicle or PCB126 occurred through milk during nursing. Offspring were microchipped for identification purposes (IPTT-300 Implantable Programmable Temperature Transponder, BDMS, Seaford, DE). Offspring were weaned on PND 21 and group housed with same sex pups from other dams of the same treatment group. Throughout the duration of the study, body weights were monitored weekly, and mice were fed standard chow (Teklad 2918 Global Rodent Diet, Envigo, Madison, WI) and had ad libitum access to food and water. Body weight data are provided for those weeks when the glucose tolerance testing and EchoMRI were performed. Offspring were euthanized at 42-44 weeks of age. All animal procedures performed during this study were approved by the University of Kentucky Institutional Animal Care and Use Committee.
1.2 Glucose tolerance testing
Every three weeks, beginning on PND 21, glucose tolerance tests were performed on offspring. Prior to testing, animals were fasted for three hours and had their tail vein pricked. An intraperitoneal injection of two grams per kilogram body weight of dextrose (Bimeda Inc., Le Sueur, MN) was given to one mouse of each sex per litter. Prior to the injection (0 minutes) and 15, 30, 60, and 120 minutes post-injection, a hand-held glucometer (Bayer Breeze 2, Bayer Health Care LLC, Tarrytown, NY) was used to quantify blood glucose levels. The total area under the curve (AUC) for blood glucose levels were calculated using the ‘Area Below Curves’ function in SigmaPlot 14.0.
1.3 Body composition analyses
Total fat mass, lean mass, and water were measured in live, conscious male and female offspring by nuclear magnetic resonance (EchoMRI; Echo Medical Systems, Houston, TX) every three weeks.
1.4 Statistics
All analyses were completed using SigmaPlot 14.0. Dam body weight was analyzed using two-factor repeated measures ANOVA and offspring body weight, body composition, glucose tolerance testing, and AUC data were analyzed using two-factor ANOVA, where the factors of analysis were treatment and time or sex. Fisher’s Least Significance Difference post-hoc testing was employed upon the detection of interactions between the factors. Significance was set at α < 0.05 for all comparisons. When parameters set for normality were not met (p < 0.05), data were natural log or square root transformed. Upon data transformations, dams body weight data (depicted in Figure 1A), offspring 6-week-old lean mass data (depicted in Figure 2D), and offspring 9-week-old lean mass data (depicted in Figure 2F) still failed to obtain equal variances between samples. Certain data failed to obtain a normal distribution following transformation: offspring 9-week-old body weight (depicted in Figure 1D), 12-week-old offspring lean mass data (depicted in Figure 2H), offspring 6-week-old 0 min and 9-week-old 120 min glucose tolerance (respectively depicted in Figures 3C, E). As a result, the probability (p) values reported in Figures 1A, D, 2D, F, H, 3C at 0 min, and Figure 3E at 120 min were obtained using untransformed data.
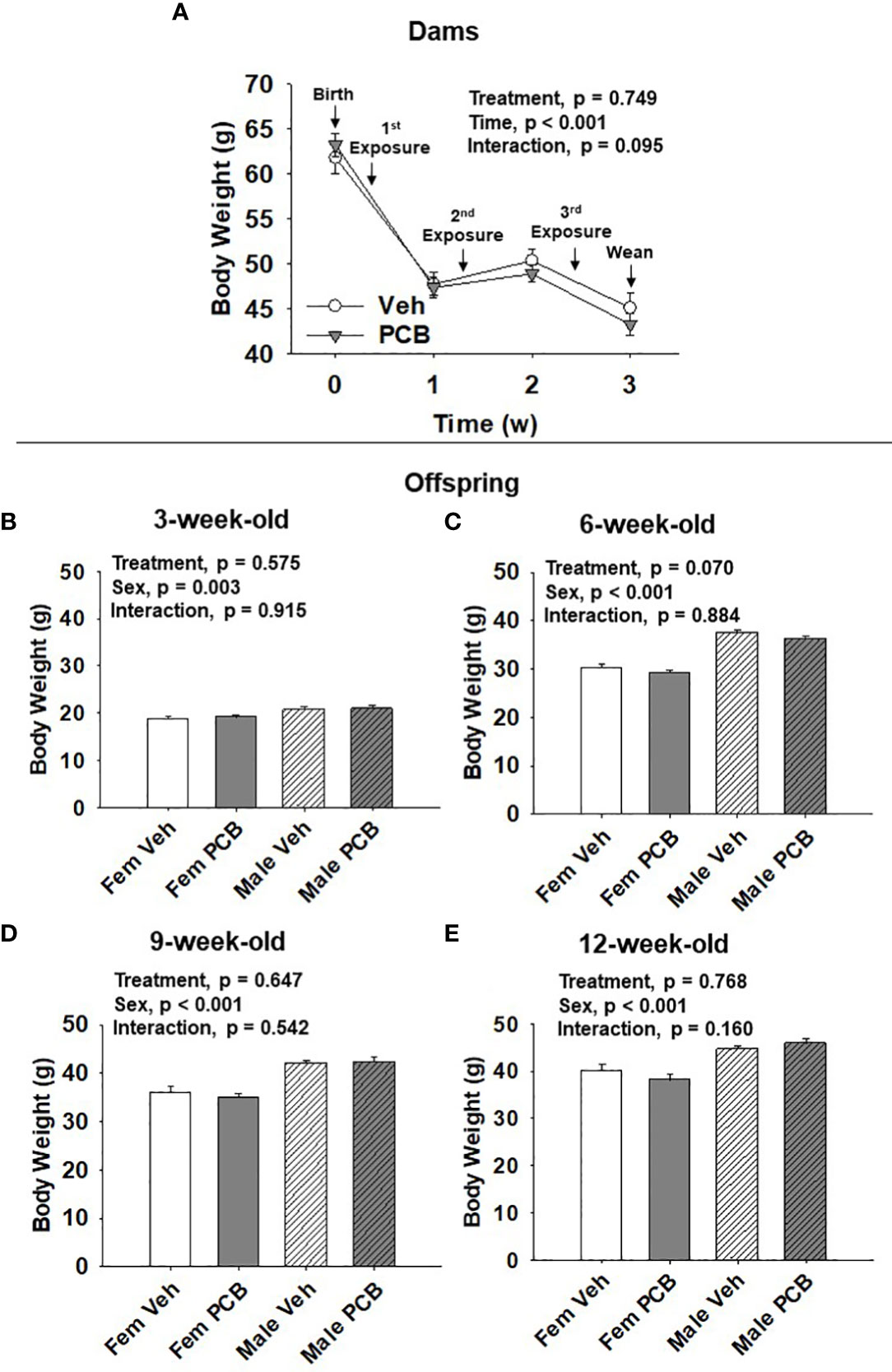
Figure 1 PCB126 exposure during the nursing period does not affect dam or offspring body weight. (A) The body weight of vehicle and PCB126-treated dams from parturition to weaning are shown. Dams received treatments respective to their experimental grouping 3, 10, and 17 days after delivery. Dam body weight values are representative of the mean ± SEM (n = 9 mice per group). The body weight of (B) 3-week-old (C) 6-week-old, (D) 9-week-old, and (E) 12-week-old offspring born to dams exposed to PCB126 or vehicle during the nursing period are shown. Offspring body weight values are provided as means of litter means ± SEM (n = 9 per group). Two-factor repeated measures ANOVA and two-factor ANOVA were respectively used to analyze dam and offspring body weight.
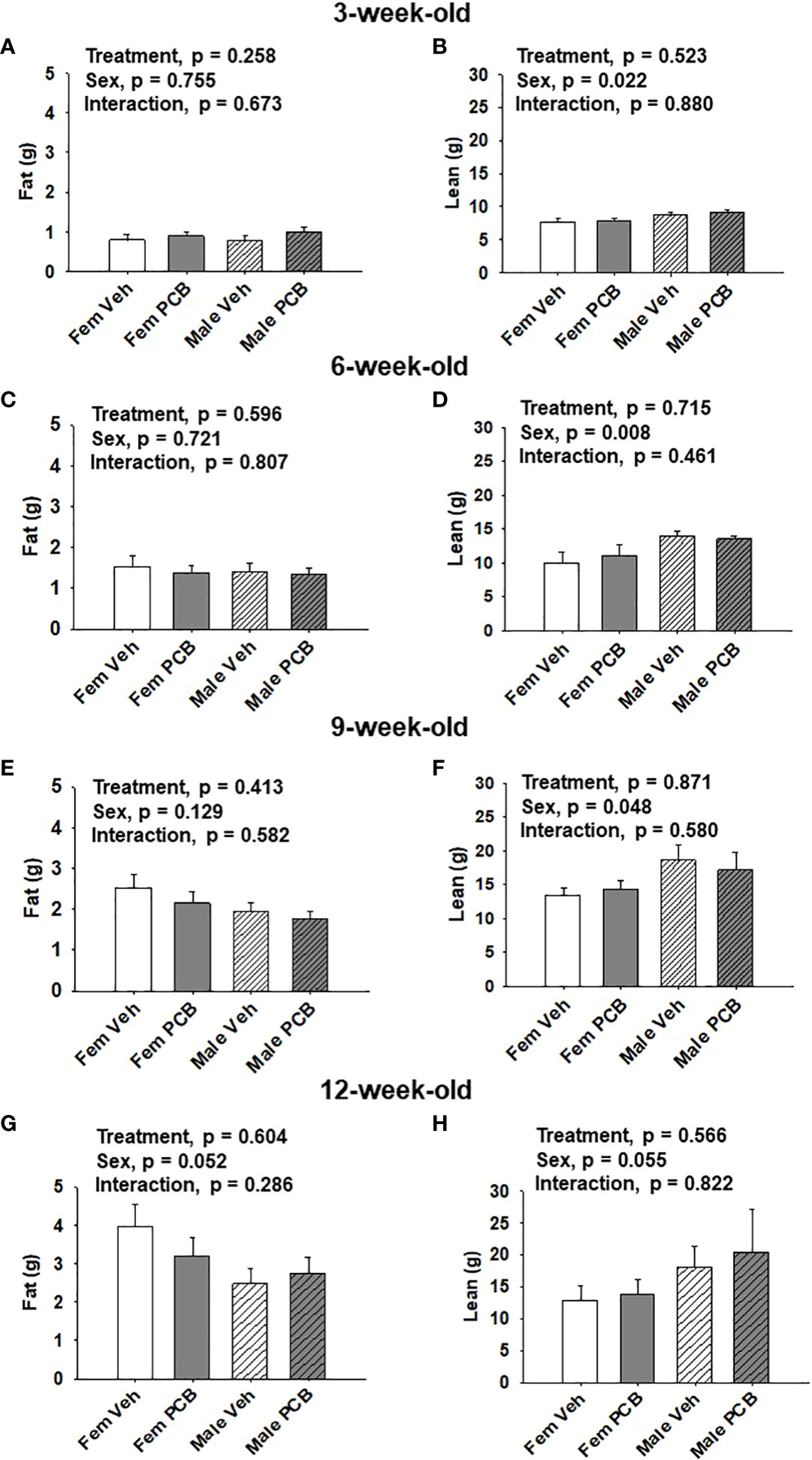
Figure 2 Maternal PCB126 exposure during the nursing period does not alter body composition. Body composition (lean and fat mass) of offspring was measured at 3, 6, 9, and 12 weeks of age via EchoMRI. Shown are the offspring data from 3 (A, B), 6 (C, D), 9 (E, F) and 12 (G, H) weeks of age. Fat mass and lean mass values are presented as the mean of litter means ± SEM (n = 9 per group). Data shown were analyzed using two-factor ANOVA.
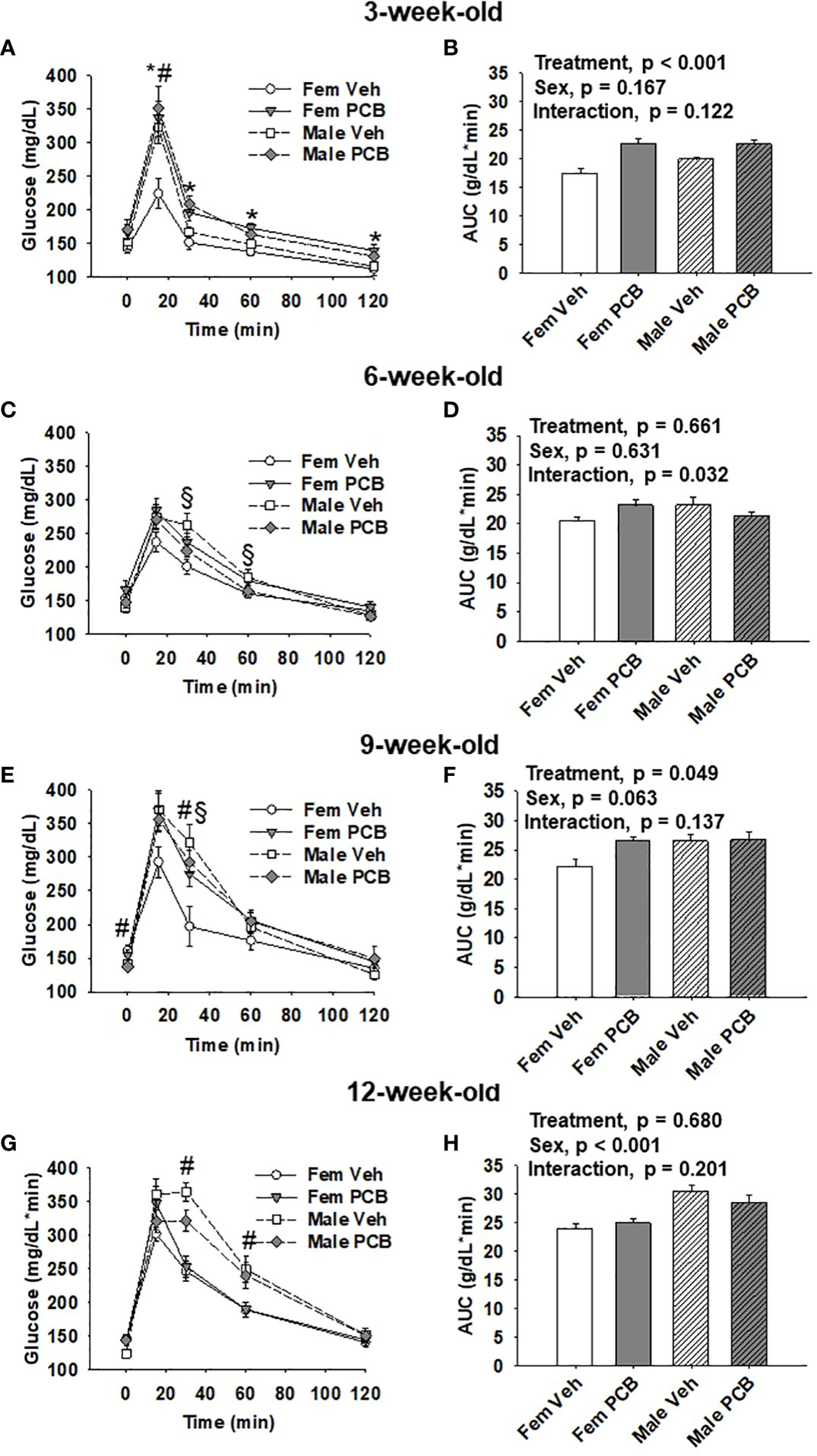
Figure 3 Offspring born to PCB126-exposed dams exhibit short-term impairments in glucose tolerance. Measurements of glucose levels in offspring are shown in response to intraperitoneal glucose injection. The glucose tolerance testing of (A) 3-week-old, (C) 6-week-old, (E) 9-week-old, and (G) 12-week-old vehicle- and PCB-exposed offspring. Significant sex-specific differences and treatment differences in glucose tolerance were respectively observed in male offspring and PCB126-exposed offspring (A, E, and G; p < 0.05 for all). Total AUC of (B) 3-week-old and (D) 6-week-old, (F) 9-week-old, and (H) 12-week-old vehicle- and PCB-exposed offspring blood glucose levels are shown. Significant differences in treatment were observed in offspring at 3 weeks of age (B, p < 0.001) and 9 weeks of age (D, p = 0.049), while significant differences in sex was observed at 12 weeks of age (H, p < 0.001). Values are shown as the mean ± SEM (n = 9 per group). Two-factor ANOVA was used to analyze glucose tolerance and AUC data. Specifically, two-factor ANOVA was used at each time-point for glucose tolerance data analyses. The # sign designates a main effect of sex while the * designates a main effect of PCB treatment (P < 0.05). Significant interactions between treatment and sex are indicated by §.
2 Results
2.1 Dam and offspring body weight
Dam body weight changed significantly over time (Figure 1A, p < 0.001) respective to weight loss after delivery and while nursing. PCB treatment was found to not significantly affect dam body weight (Figure 1A, p = 0.749). No interaction between treatment and time in dam body weight was observed (Figure 1A, p = 0.095). Offspring body weights were measured from 3 to 12 weeks of age. Body weight gain was apparent as offspring aged (Figures 1B–E). Male offspring weighed significantly more than female offspring throughout the study (Figure 1B, p = 0.003; Figure 1C, p < 0.001; Figure 1D, p < 0.001; and Figure 1E, p < 0.001). Nursing exposure to PCB126 did not result in any difference in the body weight of offspring during the study (Figure 1B, p = 0.575; Figure 1C, p = 0.070; Figure 1D, p = 0.647; and Figure 1E, p = 0.768). No interactions between sex and treatment were observed in regards to offspring body weight (Figure 1B, p = 0.915; Figure 1C, p = 0.884; Figure 1D, p = 0.542; and Figure 1E, p = 0.160).
2.2 Offspring body composition
No differences in offspring fat or lean mass respective to PCB treatment were observed throughout the study (Figure 2A, p = 0.258; Figure 2B, p = 0.523; Figure 2C, p = 0.596; Figure 2D, p = 0.715; Figure 2E, p = 0.413; Figure 2F, p = 0.871; Figure 2G, p = 0.604; and Figure 2H, p = 0.566). However, sex-specific differences in lean mass amount were present at 3, 6, and 9 weeks of age, where female offspring had lower lean mass compared to male offspring (Figure 2B, p = 0.022; Figure 2D, p = 0.008; and Figure 2F, p = 0.048). At 12 weeks of age, marginal sex effects were observed where female offspring exhibit a trend towards increased fat mass compared to male offspring (Figure 2G, p = 0.052) and male offspring exhibit a trend towards higher lean mass compared to female offspring (Figure 2H, p = 0.055). No interactions between sex and treatment were detected regarding offspring lean and fat mass profiles (Figure 2A, p = 0.673; Figure 2B, p = 0.880; Figure 2C, p = 0.807; Figure 2D, p = 0.461; Figure 2E, p = 0.582; Figure 2F, p = 0.580; Figure 2G, p = 0.286; and Figure 2H, p = 0.822).
2.3 Offspring glucose tolerance
At 3 weeks of age, postnatal PCB exposure caused significant impairments in glucose disposal at all time-points during testing beyond fasting in both female and male offspring compared to vehicle control offspring (Figure 3A, p < 0.05). At 15 minutes following the glucose challenge, male offspring exhibited significantly elevated blood glucose levels when compared to female offspring (Figure 3A, p < 0.05). Glucose disposal curves generated from glucose tolerance testing were summarized using AUC, and both male and female PCB-exposed offspring had higher AUC values than those vehicle-exposed offspring (Figure 3B, p < 0.001). Additionally, no sex differences or interactions were detected in the AUC data (Figure 3B, p > 0.100). At six weeks of age, no differences in glucose disposal of offspring respective to treatment or sex were observed (Figure 3C, p > 0.05). Cross-over interactions between sex and treatment were detected at 30 minutes and 60 minutes during testing (Figure 3C, p = 0.009 and p = 0.027 respectively). Likewise, AUC values for offspring did not differ based on treatment or sex (Figure 3D, p > 0.600), but a significant interaction between treatment and sex was detected (Figure 3D, p = 0.032). At 9 weeks of age, treatment did not influence glucose disposal profiles at individual time-points during testing (Figure 3E, p > 0.05). An interaction between treatment and sex was detected at the 30-minute time-point (Figure 3E, p = 0.030), where female offspring exposed to PCB exhibited pronounced elevations in blood glucose levels when compared to vehicle-exposed female offspring (p = 0.025). While AUC data did not reveal any significant differences in offspring AUC values based on sex (Figure 3F, p = 0.063), PCB-exposed offspring had higher AUC values when compared to vehicle controls (Figure 3F, p = 0.049). No interactions between sex and treatment were detected (Figure 3F, p = 0.137). Glucose tolerance testing at 12 weeks of age yielded no significant differences between groups respective to treatment (Figure 3G, p > 0.1). Offspring AUC data at 12 weeks of age demonstrate that glucose disposal differed by sex (Figure 3H, p < 0.001), not treatment (Figure 3H, p = 0.680). No interactions between treatment and sex were detected (Figure 3H, p = 0.201).
3 Discussion
Type 2 diabetes (T2D), a disease in which affected individuals have elevated blood glucose levels due to insulin impairments, is one of the leading causes of death globally (35). Progressive elevations in the prevalence of T2D within child and adolescent populations (36) have gathered the attention of many to the etiology of the disease. Risk factors for T2D have extended beyond hallmarked contributions of genetics and lifestyle and now include environmental exposures (37). Despite piqued interest, research has failed to sufficiently detail the underlying mechanisms by which environmental exposure to PCBs contribute to the developmental programming of T2D. The current study used glucose tolerance testing to assess diabetes risk in offspring in relation to PCB126 exposure during nursing (early postnatal life). Results from the present study demonstrate that offspring exposed to PCB126 during the nursing period exhibited short-term impairments in early-life glucose tolerance despite displaying normal body composition and body weight gain while aging to 12 weeks. This finding extends the current body of etiological evidence supporting the contribution of early-life PCB exposure to the development of diabetic-like phenotypes (30–34), as well as provides insight into understanding how the timing of PCB exposure during development affects diabetic-like outcomes observed later in life.
Previously, we conducted dosing studies to understand the effects of perinatal PCB exposure on offspring diabetes and obesity health outcomes in which ICR dams were exposed to 0, 0.5, or 1 micromole of PCB126 per kilogram body weight via oral gavage at a frequency of once per every 14 days beginning two days before mating. There were a total of three exposures: once prior to mating, during gestation, and during nursing (30), and our goal in the current study was to replicate the number of exposures but during the nursing period only. Despite differences in exposure paradigms between our previous work and the current study, our current observation of comparable body weights between animals exposed to vehicle and PCB126 during the nursing period are corroborated by our finding from our preceding study when dams were exposed to PCB126 throughout the perinatal period (30). Mennigen et al. (38) found the pre-weaning and adolescent body weights of Sprague-Dawley rats exposed during gestation days 16 and 18 to Aroclor 1221, a commercial PCB mixture, were not significantly different from that of the control animals. Offspring gestational PCB mixture exposure occurred through placental transfer of dams exposed intraperitoneally to 1 milligram/kilogram of Aroclor 1221 (38). Interestingly, in the same study, the body weights of offspring of the F2 and F3 generations were elevated as a result of the F0 PCB gestational exposure (38). In C57BL/6 J mouse dams exposed to 3.0 micrograms/kilogram body weight of dioxin via oral gavage during gestation day 12.5 of pregnancy, toxicant exposure was not shown to affect the body weight of exposed offspring as they aged (39). This lack of effect may be due to a single exposure. Independent of perinatal exposures, PCBs have been demonstrated to increase body weight (20, 22, 40–42). Studies demonstrating the impact of PCB exposure to body composition indicate that PCB-induced obesity may occur via alteration of adipocyte development (20, 21, 43, 44) and death (44). However, the route, dose, and timing of coplanar/non-coplanar PCBs or mixtures exposures must be considered to avoid overinterpretation of the comparisons to previous studies.
Although excess body weight has been linked to diabetes development and progression, body composition analyses provide a more comprehensive understanding of diabetes risk and glycemic control. Individuals possessing increased fat mass and reduced lean mass may be more susceptible to hyperglycemia. In adult male mice, direct PCB exposure has been demonstrated to increase fat mass (42). Here, we report no differences in the early-life body composition of offspring exposed to PCB126 during the nursing period. These findings are in contrast to our previous observations of increased adiposity in female offspring and decreased lean mass in male offspring when we used a different dosing paradigm and PCBs were given prior to and during pregnancy and during nursing (30). Recent work from our research group demonstrates consistent and pronounced alterations in offspring later-life body composition as a result of exposure to PCBs solely during gestation, where 4-month-old adult offspring exposed to PCB126 exhibited significantly reduced lean mass profiles when compared to vehicle-exposed offspring (32). Unlike the effects of gestational PCB exposure on offspring lean mass profiles, offspring fat mass profiles respective to PCB exposure were modulated by the presence or absence of nuclear factor erythroid-2-related factor 2 (Nrf2) (32), a key transcription factor responsible for regulating the detoxification of coplanar PCBs. Those adult offspring born to Nrf2 knockout dams exposed to PCBs during pregnancy exhibited significantly increased adiposity when compared to vehicle control offspring (32). Taken together, this suggests that in utero PCB exposure, or a combination of timed exposures during development, is more detrimental than nursing exposure alone as it pertains to obesity risk, or body composition changes, associated with early-life PCB exposure.
Iterations of in vivo PCB-induced glucose impairments have been extended to in vitro situations, as PCB exposure has been shown to inhibit insulin-stimulated glucose uptake in adipocytes (23, 42) and hepatocytes (42). Interestingly, in myotubes, PCB126 exposure does not affect insulin-stimulated glucose uptake, but has been demonstrated to reduce basal glucose uptake (26). In the present study, following glucose challenges, we observed impairments in the glucose disposal of offspring exposed to PCB126 during the nursing period compared to vehicle controls. Dams in this study were exposed to PCB126 or vehicle on PNDs 3, 10, and 17. It is important to note that the maternal dose at PND17 may not contribute substantial levels of PCBs to the offspring as they likely would consume little milk after this age. Our observation is largely consistent with the aforementioned in vitro studies as we demonstrate the ability of PCBs to alter glucose tolerance. Here, our observation of the PCB-induced glucose impairment in offspring was short-term. Specifically, both male and female offspring nursed to PCB-exposed dams had significantly elevated blood glucose levels at weaning (Figure 3). By twelve weeks of age, neither male nor female offspring presented any impairments in response to the PCB exposure (Figure 3). We previously demonstrated that PCB126 exposure prior to and during gestation (not during nursing) significantly impairs glucose disposal after a glucose challenge in animals (32). This suggests that the in utero period is the most susceptible window for long-lasting PCB-induced glucose intolerance because nursing only PCB exposure caused glucose tolerance that was reversible over time. In mice, persistent oral Aroclor 1254 exposure induced glucose intolerance and hyperinsulinemia while cessation of the exposure abolished PCB-induced detriments (45). Such data could explain the lack of permanence in the glucose impairments observed in the offspring of the current study, as PCB exposure through the dams’ milk serves as a persistent direct exposure for offspring from birth until weaning.
There are many positive outcomes of nursing for mothers and babies. Health benefits observed in adults that were breastfed include, but are not limited to, increased cognitive functioning, decreased obesity in childhood and adulthood, and reduced T2D risk (46, 47), thus, suggesting a beneficial developmental impact of breastfeeding on health span. Additionally, moms that breastfeed their infants have reduced rates of cancer, obesity, T2D, and heart disease after parturition when compared to those women who did not (46). While beneficial effects of nursing are apparent, adverse effects associated with toxicant exposure have also been observed (48). Despite potential risks that may occur via increased exposure to pollutants, health care providers encourage mothers to breastfeed as the benefits outweigh the risks.
Although we found that short-term early-life impairments in offspring glucose tolerance results from PCB126 exposure during the nursing period, more work is needed to understand how and why each period of perinatal PCB exposure collectively and independently influence diabetes risk in offspring. A limitation of our work is that we did not assess the PCB126 body burden after exposure in dams and offspring. Future studies will address this limitation by quantifying the amount of PCB126 accumulated in dam milk and offspring adipose tissue and serum. Exposing dams daily or on a weekly basis starting at PND0 could also affect the offspring metabolic outcomes differently. Additionally, the study could have benefited from more robust metabolic phenotyping and mechanistic assays, such as quantification of insulin resistance and adipocyte dysfunction to better understand observed offspring health outcomes. This is a limitation of the current work, and these experiments could be included in future studies. Regardless, we feel the body weight, body composition, and glucose tolerance data collected following nursing only exposure to PCB126 contribute important information to the field.
In conclusion, this study demonstrates that glucose impairments that occurred in offspring exposed to PCB126 during nursing were short-term. Thus, when compared to our previous work (30, 32) these results suggest that in utero exposure potentially poses more detrimental health effects on offspring when compared to nursing exposure. The short-term early life impairments are transient and disappear as offspring age. This suggests a direct toxicity effect, but it is uncertain whether there will be residual effects much later in life. While toxicological exposures during the nursing period may pose a threat to long-term health outcomes of offspring, the benefits of breastfeeding outweigh the risks. Although toxic exposures during nursing have been shown to be less harmful than in utero exposure, our work demonstrates they should not be ignored given that nursing and in utero exposure often co-exist. Therefore, knowledge of the physiological interaction between the two is warranted.
Data availability statement
The raw data supporting the conclusions of this article will be made available by the authors, without undue reservation.
Ethics statement
The animal study was reviewed and approved by University of Kentucky Institutional Animal Care and Use Committee.
Author contributions
BR and KS both made substantial contributions and share equal first authorship. SN, HS, and KP contributed to the conception and design of this study. KS, SN, and MW contributed to data acquisition. BR, KS, and SN contributed to data analysis. BR, KS, SN, LR, CR, HS, and KP contributed to the interpretation of the data. BR, KS, SN, MW, LR, CR, HS, and KP drafted and critically revised the manuscript, gave final approval, and agree to be accountable for all aspects of work ensuring integrity and accuracy. All authors contributed to the article and approved the submitted version.
Funding
Research reported was supported by the National Institute of Environmental Health Sciences (P42ES007380) and the National Institute of General Medical Sciences (P20GM103527). BR was supported by the Southern Regional Education Board Doctoral Scholars Program. KS was supported by the Undergraduate Summer Research Training Program in Cardiovascular Research funded by the American Heart Association (17UFEL33490002). MW was supported by the Summer Undergraduate Research in Environmental Health Sciences Program funded by the National Institute of Environmental Health Sciences (R25ES027684). LR was supported by an American Heart Association Postdoctoral Fellowship (15POST25110002). The content is solely the responsibility of the authors and does not necessarily represent the official views of the National Institutes of Health.
Acknowledgments
We would like to thank M. Paul Murphy for his useful discussions that contributed to the generation of this manuscript. Portions of this manuscript will be published online by the University of Kentucky as a section of BR’s PhD dissertation in order to fulfill the requirements of her dissertation.
Conflict of interest
The authors declare that the research was conducted in the absence of any commercial or financial relationships that could be construed as a potential conflict of interest.
Publisher’s note
All claims expressed in this article are solely those of the authors and do not necessarily represent those of their affiliated organizations, or those of the publisher, the editors and the reviewers. Any product that may be evaluated in this article, or claim that may be made by its manufacturer, is not guaranteed or endorsed by the publisher.
References
1. Erickson MD, Kaley RG 2nd. Applications of polychlorinated biphenyls. Environ Sci Pollut Res Int (2011) 18(2):135–51. doi: 10.1007/s11356-010-0392-1
2. Markowitz G. From industrial toxins to worldwide pollutants: A brief history of polychlorinated biphenyls. Public Health Rep (2018) 133(6):721–5. doi: 10.1177/0033354918801578
3. Carpenter DO. Polychlorinated biphenyls (PCBs): Routes of exposure and effects on human health. Rev Environ Health (2006) 21(1):1–24. doi: 10.1515/REVEH.2006.21.1.1
4. Atlas E, Giam CS. Global transport of organic pollutants: Ambient concentrations in the remote marine atmosphere. Science (1981) 211(4478):163. doi: 10.1126/science.211.4478.163
5. Bacon CE, Jarman WM, Costa DP. Organochlorine and polychlorinated biphenyl levels in pinniped milk from the Arctic, the Antarctic, California and Australia. Chemosphere (1992) 24(6):779–91. doi: 10.1016/0045-6535(92)90538-3
6. Beyer A, Biziuk M. Environmental fate and global distribution of polychlorinated biphenyls. Rev Environ Contam Toxicol (2009) 201:137–58. doi: 10.1007/978-1-4419-0032-6_5
7. Wassermann M, Wassermann D, Cucos S, Miller HJ. World PCBs map: Storage and effects in man and his biologic environment in the 1970s. Ann N Y Acad Sci (1979) 320:69–124. doi: 10.1111/j.1749-6632.1979.tb13137.x
8. ATSDR CfDCaP. Toxicological profile for polychlorinated biphenyls (PCBs). Atlanta, GA: U.S. Department of Health and Human Services, Public Health Service (2000). Available at: http://www.atsdr.cdc.gov/toxprofiles/tp17.pdf.
9. Okey AB, Riddick DS, Harper PA. The ah receptor: Mediator of the toxicity of 2,3,7,8-tetrachlorodibenzo-p-dioxin (TCDD) and related compounds. Toxicol Lett (1994) 70(1):1–22. doi: 10.1016/0378-4274(94)90139-2
10. Van den Berg M, Birnbaum LS, Denison M, De Vito M, Farland W, Feeley M, et al. The 2005 world health organization reevaluation of human and mammalian toxic equivalency factors for dioxins and dioxin-like compounds. Toxicol Sci (2006) 93(2):223–41. doi: 10.1093/toxsci/kfl055
11. Lee DH, Lee IK, Song K, Steffes M, Toscano W, Baker BA, et al. A strong dose-response relation between serum concentrations of persistent organic pollutants and diabetes: Results from the national health and examination survey 1999-2002. Diabetes Care (2006) 29(7):1638–44. doi: 10.2337/dc06-0543
12. Silverstone AE, Rosenbaum PF, Weinstock RS, Bartell SM, Foushee HR, Shelton C, et al. Polychlorinated biphenyl (PCB) exposure and diabetes: Results from the anniston community health survey. Environ Health Perspect (2012) 120(5):727–32. doi: 10.1289/ehp.1104247
13. Tanaka T, Morita A, Kato M, Hirai T, Mizoue T, Terauchi Y, et al. Congener-specific polychlorinated biphenyls and the prevalence of diabetes in the saku control obesity program (SCOP). Endocrine J (2011) 58(7):589–96. doi: 10.1507/endocrj.K10E-361
14. Lee DH, Steffes MW, Sjödin A, Jones RS, Needham LL, Jacobs DR Jr. Low dose of some persistent organic pollutants predicts type 2 diabetes: A nested case-control study. Environ Health Perspect (2010) 118(9):1235–42. doi: 10.1289/ehp.0901480
15. Vasiliu O, Cameron L, Gardiner J, Deguire P, Karmaus W. Polybrominated biphenyls, polychlorinated biphenyls, body weight, and incidence of adult-onset diabetes mellitus. Epidemiology (2006) 17(4):352–9. doi: 10.1097/01.ede.0000220553.84350.c5
16. Wang SL, Tsai PC, Yang CY, Guo YL. Increased risk of diabetes and polychlorinated biphenyls and dioxins: A 24-year follow-up study of the yucheng cohort. Diabetes Care (2008) 31(8):1574–9. doi: 10.2337/dc07-2449
17. Aminian O, Moinfar Z, Eftekhari S, Esser A, Schettgen T, Felten M, et al. Association of plasma levels of lipid and polychlorinated biphenyls in Iranian adult. Heliyon (2020) 6(4):e03775–e. doi: 10.1016/j.heliyon.2020.e03775
18. Chen J-W, Wang S-L, Liao P-C, Chen HY, Ko Y-C, Lee C-C. Relationship between insulin sensitivity and exposure to dioxins and polychlorinated biphenyls in pregnant women. Environ Res (2008) 107(2):245–53. doi: 10.1016/j.envres.2008.01.004
19. Singh K, Chan HM. Association of blood polychlorinated biphenyls and cholesterol levels among Canadian Inuit. Environ Res (2018) 160:298–305. doi: 10.1016/j.envres.2017.10.010
20. Arsenescu V, Arsenescu RI, King V, Swanson H, Cassis LA. Polychlorinated biphenyl-77 induces adipocyte differentiation and proinflammatory adipokines and promotes obesity and atherosclerosis. Environ Health Perspect (2008) 116(6):761–8. doi: 10.1289/ehp.10554
21. Kim HY, Kwon WY, Kim YA, Oh YJ, Yoo SH, Lee MH, et al. Polychlorinated biphenyls exposure-induced insulin resistance is mediated by lipid droplet enlargement through Fsp27. Arch Toxicol (2017) 91(6):2353–63. doi: 10.1007/s00204-016-1889-2
22. Loiola RA, Dos Anjos FM, Shimada AL, Cruz WS, Drewes CC, Rodrigues SF, et al. Long-term in vivo polychlorinated biphenyl 126 exposure induces oxidative stress and alters proteomic profile on islets of langerhans. Sci Rep (2016) 6:27882. doi: 10.1038/srep27882
23. Baker NA, English V, Sunkara M, Morris AJ, Pearson KJ, Cassis LA. Resveratrol protects against polychlorinated biphenyl-mediated impairment of glucose homeostasis in adipocytes. J Nutr Biochem (2013) 24(12):2168–74. doi: 10.1016/j.jnutbio.2013.08.009
24. Baker NA, Karounos M, English V, Fang J, Wei Y, Stromberg A, et al. Coplanar polychlorinated biphenyls impair glucose homeostasis in lean C57BL/6 mice and mitigate beneficial effects of weight loss on glucose homeostasis in obese mice. Environ Health Perspect (2013) 121(1):105–10. doi: 10.1289/ehp.1205421
25. Jackson EN, Thatcher SE, Larian N, English V, Soman S, Morris AJ, et al. Effects of aryl hydrocarbon receptor deficiency on PCB-77-Induced impairment of glucose homeostasis during weight loss in Male and female obese mice. Environ Health Perspect (2019) 127(7):077004. doi: 10.1289/EHP4133
26. Mauger JF, Nadeau L, Caron A, Chapados NA, Aguer C. Polychlorinated biphenyl 126 exposure in L6 myotubes alters glucose metabolism: A pilot study. Environ Sci Pollut Res Int (2016) 23(8):8133–40. doi: 10.1007/s11356-016-6348-3
27. DeKoning EP, Karmaus W. PCB Exposure in utero and via breast milk. a review. J Expo Anal Environ Epidemiol (2000) 10(3):285–93. doi: 10.1038/sj.jea.7500090
28. Wadhwa PD, Buss C, Entringer S, Swanson JM. Developmental origins of health and disease: Brief history of the approach and current focus on epigenetic mechanisms. Semin Reprod Med (2009) 27(5):358–68. doi: 10.1055/s-0029-1237424
29. Preston JD, Reynolds LJ, Pearson KJ. Developmental origins of health span and life span: A mini-review. Gerontology (2018) 64(3):237–45. doi: 10.1159/000485506
30. Rashid CS, Carter LG, Hennig B, Pearson KJ. Perinatal polychlorinated biphenyl 126 exposure alters offspring body composition. J Pediatr Biochem (2013) 3(1):47–53. doi: 10.3233/JPB-120072
31. van Esterik JC, Verharen HW, Hodemaekers HM, Gremmer ER, Nagarajah B, Kamstra JH, et al. Compound- and sex-specific effects on programming of energy and immune homeostasis in adult C57BL/6JxFVB mice after perinatal TCDD and PCB 153. Toxicol Appl Pharmacol (2015) 289(2):262–75. doi: 10.1016/j.taap.2015.09.017
32. Rice BB, Ngo Tenlep SY, Tolaymat O, Alvi AT, Slone FR, Crosby CL, et al. Lack of offspring Nrf2 does not exacerbate the detrimental metabolic outcomes caused by In utero PCB126 exposure. Front Endocrinol (2021) 12:777831–. doi: 10.3389/fendo.2021.777831
33. Timme-Laragy AR, Sant KE, Rousseau ME, diIorio PJ. Deviant development of pancreatic beta cells from embryonic exposure to PCB-126 in zebrafish. Comp Biochem Physiol C Toxicol Pharmacol (2015) 178:25–32. doi: 10.1016/j.cbpc.2015.08.012
34. Ahmed RG, El-Gareib AW, Shaker HM. Gestational 3,3',4,4',5-pentachlorobiphenyl (PCB 126) exposure disrupts fetoplacental unit: Fetal thyroid-cytokines dysfunction. Life Sci (2018) 192:213–20. doi: 10.1016/j.lfs.2017.11.033
35. Khan MAB, Hashim MJ, King JK, Govender RD, Mustafa H, Al Kaabi J. Epidemiology of type 2 diabetes - global burden of disease and forecasted trends. J Epidemiol Glob Health (2020) 10(1):107–11. doi: 10.2991/jegh.k.191028.001
36. Wilmot E, Idris I. Early onset type 2 diabetes: Risk factors, clinical impact and management. Ther Adv Chronic Dis (2014) 5(6):234–44. doi: 10.1177/2040622314548679
37. Murea M, Ma L, Freedman BI. Genetic and environmental factors associated with type 2 diabetes and diabetic vascular complications. Rev Diabetic Stud RDS (2012) 9(1):6–22. doi: 10.1900/RDS.2012.9.6
38. Mennigen JA, Thompson LM, Bell M, Tellez Santos M, Gore AC. Transgenerational effects of polychlorinated biphenyls: 1. development and physiology across 3 generations of rats. Environ Health (2018) 17(1):18. doi: 10.1186/s12940-018-0362-5
39. Sugai E, Yoshioka W, Kakeyama M, Ohsako S, Tohyama C. In utero and lactational exposure to 2,3,7,8-tetrachlorodibenzo- p -dioxin modulates dysregulation of the lipid metabolism in mouse offspring fed a high-calorie diet. J Appl Toxicol JAT (2014) 34 296–306. doi: 10.1002/jat.2881
40. Wahlang B, Falkner KC, Gregory B, Ansert D, Young D, Conklin DJ, et al. Polychlorinated biphenyl 153 is a diet-dependent obesogen that worsens nonalcoholic fatty liver disease in male C57BL6/J mice. J Nutr Biochem (2013) 24(9):1587–95. doi: 10.1016/j.jnutbio.2013.01.009
41. Zhang S, Wu T, Chen M, Guo Z, Yang Z, Zuo Z, et al. Chronic exposure to aroclor 1254 disrupts glucose homeostasis in Male mice via inhibition of the insulin receptor signal pathway. Environ Sci Technol (2015) 49(16):10084–92. doi: 10.1021/acs.est.5b01597
42. Wu H, Yu W, Meng F, Mi J, Peng J, Liu J, et al. Polychlorinated biphenyls-153 induces metabolic dysfunction through activation of ROS/NF-κB signaling via downregulation of HNF1b. Redox Biol (2017) 12:300–10. doi: 10.1016/j.redox.2017.02.026
43. Chi Y, Lin Y, Zhu H, Huang Q, Ye G, Dong S. PCBs-high-fat diet interactions as mediators of gut microbiota dysbiosis and abdominal fat accumulation in female mice. Environ Pollut (2018) 239:332–41. doi: 10.1016/j.envpol.2018.04.001
44. Kim YA, Kim HY, Oh YJ, Kwon WY, Lee MH, Bae JY, et al. Polychlorinated biphenyl 138 exposure-mediated lipid droplet enlargement endows adipocytes with resistance to TNF-α-induced cell death. Toxicol Lett (2018) 292:55–62. doi: 10.1016/j.toxlet.2018.04.029
45. Xi Z, Fang L, Xu J, Li B, Zuo Z, Lv L, et al. Exposure to aroclor 1254 persistently suppresses the functions of pancreatic β-cells and deteriorates glucose homeostasis in male mice. Environ Pollut (2019) 249:822–30. doi: 10.1016/j.envpol.2019.03.101
46. Binns C, Lee M, Low WY. The long-term public health benefits of breastfeeding. Asia Pac J Public Health (2016) 28(1):7–14. doi: 10.1177/1010539515624964
47. Westerfield KL, Koenig K, Oh R. Breastfeeding: Common questions and answers. Am Fam Physician (2018) 98(6):368–73.
Keywords: obesity, polychlorinated biophenyls, diabetes, mice, developmental programming of adult disease, DOHaD (development origins of health and disease), lactation
Citation: Rice BB, Sammons KW, Ngo Tenlep SY, Weltzer MT, Reynolds LJ, Rashid CS, Swanson HI and Pearson KJ (2023) Exposure to PCB126 during the nursing period reversibly impacts early-life glucose tolerance. Front. Endocrinol. 14:1085958. doi: 10.3389/fendo.2023.1085958
Received: 31 October 2022; Accepted: 03 March 2023;
Published: 22 March 2023.
Edited by:
Rebecca Simmons, University of Pennsylvania, United StatesReviewed by:
Kimberly Keil Stietz, University of Wisconsin-Madison, United StatesSara Pinney, Children’s Hospital of Philadelphia, United States
Copyright © 2023 Rice, Sammons, Ngo Tenlep, Weltzer, Reynolds, Rashid, Swanson and Pearson. This is an open-access article distributed under the terms of the Creative Commons Attribution License (CC BY). The use, distribution or reproduction in other forums is permitted, provided the original author(s) and the copyright owner(s) are credited and that the original publication in this journal is cited, in accordance with accepted academic practice. No use, distribution or reproduction is permitted which does not comply with these terms.
*Correspondence: Kevin J. Pearson, a2V2aW4ucGVhcnNvbkB1a3kuZWR1
†These authors have contributed equally to this work and share first authorship