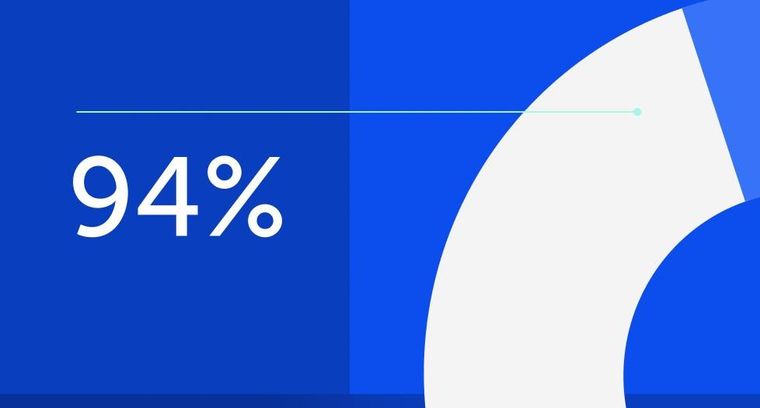
94% of researchers rate our articles as excellent or good
Learn more about the work of our research integrity team to safeguard the quality of each article we publish.
Find out more
REVIEW article
Front. Endocrinol., 07 February 2023
Sec. Cardiovascular Endocrinology
Volume 14 - 2023 | https://doi.org/10.3389/fendo.2023.1085041
Morbidity and mortality of cardiovascular diseases (CVDs) are exceedingly high worldwide. Researchers have found that the occurrence and development of CVDs are closely related to intestinal microecology. Imbalances in intestinal microecology caused by changes in the composition of the intestinal microbiota will eventually alter intestinal metabolites, thus transforming the host physiological state from healthy mode to pathological mode. Trimethylamine N-oxide (TMAO) is produced from the metabolism of dietary choline and L-carnitine by intestinal microbiota, and many studies have shown that this important product inhibits cholesterol metabolism, induces platelet aggregation and thrombosis, and promotes atherosclerosis. TMAO is directly or indirectly involved in the pathogenesis of CVDs and is an important risk factor affecting the occurrence and even prognosis of CVDs. This review presents the biological and chemical characteristics of TMAO, and the process of TMAO produced by gut microbiota. In particular, the review focuses on summarizing how the increase of gut microbial metabolite TMAO affects CVDs including atherosclerosis, heart failure, hypertension, arrhythmia, coronary artery disease, and other CVD-related diseases. Understanding the mechanism of how increases in TMAO promotes CVDs will potentially facilitate the identification and development of targeted therapy for CVDs.
The mammalian gut microbiota is the most abundant highly and diverse microbial community that resides in the gastrointestinal tract. The gut microbiome, as the “second genome”, plays an important role in dynamically integrating signals from the host and its environment, impacting both physiological homeostasis and disease emergence (1). Consequently, research on gut microbiota has become the focus of attention for many researchers. Meanwhile, in 2007, the National Institutes of Health (NIH) launched the Human Microbiome Project (HMP), aiming to elucidate microorganisms relevant to human health and disease (2). Cardiovascular diseases (CVDs) are associated with high morbidity and mortality, as well as considerable and increasing health-care costs. Based on the Global Burden of Disease (GBD) Study 2019, the total number of cases of CVDs almost doubled from 271 million in 1990 to 523 million in 2019; and the number of deaths from CVDs increased steadily from 12.1 million in 1990 to 18.6 million in 2019, accounting for 33% of all deaths globally (3). According to the European Society of Hypertension/Cardiology Guidelines, the occurrence and development of CVDs are combined effects of the risk factors, which include non-modifiable factors (age, gender, heredity) and modifiable factors (smoking, hypercholesterolemia, diabetes, lack of physical exercise, glucose intolerance, obesity), and target organ damage. Among the modifiable factors, smoking is the most important cardiovascular risk factor, and smoking cessation may be the most effective method for the prevention of CVDs among the general population (4). In recent years, metagenomic sequencing has verified that the gut microbiota is linked to many diseases, including CVDs (5).
Changes in gut microbiota alter the production of gut metabolites and thus affect the development of CVDs (6). Intestinal bacteria produce various bioactive metabolites, which can be absorbed into the enterohepatic circulation and enter the host blood circulation system, affecting host physiology directly or indirectly (7). Currently, there is growing evidence that some metabolites, such as short-chain fatty acids, bile acids (BAs), and trimethylamine N-oxide (TMAO), derived from gut microbiota, play important roles in the onset and progression of CVDs. Among them, TMAO has attracted attention recently, with evidence supporting an association between circulating levels of TMAO and increased risks of CVDs and higher all-cause mortality (8, 9). Hazen et al. reported the small-molecule metabolic profile of plasma through metabolomics and showed that the level of TMAO could be used to predict the risk of CVDs (10).
Although the role of TMAO in CVDs is well established, detailed understanding of how TMAO regulates the development and progression of CVDs has yet to be elucidated. Choline and L-carnitine are metabolized to disease-associated metabolite trimethylamine (TMA) by the gut microbiota. Subsequently, TMA is transported via the portal circulation to the liver where flavin monooxygenases (FMOs) catalyze the conversion of TMA into TMAO; however, the mechanism by which these precursors raise serum levels of TMAO and how changes in their levels contribute to CVDs remain unknown. This article reviews the involvement of TMAO in CVDs, which is helpful to understand the mechanism of TMAO leading to the development and progression of atherosclerosis, heart failure (HF), hypertension, and other CVDs. Furthermore, the solutions to regulate excessive TMAO levels from the perspective of diet, TMAO/TMA circulation, and gut microbiota regulation are reviewed, providing a promising targeted therapy for CVDs.
The human gut is colonized by approximately 100 trillion microorganisms–including beneficial bacteria, neutral bacteria, and pathogenic bacteria–which cooperate to maintain homeostasis of the gut microecology (11). Continuous improvements in next-generation sequencing technology and omic analysis methods, coupled with decreasing sequencing costs, have resulted in microbial genome sequencing becoming a rapid and convenient way to obtain detailed information on the relationship between structural changes of microbiota and human health based on gut microbiome data (7, 12). In the gut, Bacteroidetes and Firmicutes account for approximately 90% of the total gut microbial abundance, followed by Proteobacteria (5-10%), Actinobacteria (3%), and Verrucomicrobia (13). Gut microbiota plays an important role in human health through maintaining the integrity of the host intestinal epithelial barrier, digesting indigestible nutrients and providing nutrients, modulating the host innate immune system, and defending against pathogens (14). The gut microbiota colonizes and reproduces on intestinal epithelial surfaces, secreting enzymes that catalyze carbohydrate and metabolize indigestible carbohydrates into gut metabolites, vitamins, and some neurochemicals (15). Furthermore, homeostasis of gut microbiota positively affects host immuno-function and prevents invasion by pathogenic microorganisms (16). However, despite the fact that the intestinal microbiota is indispensable for maintaining lifelong health of humans, detailed understanding of the process is still at a juvenile stage. Fungi, protists, archaea, and viruses also belong to the gut microbiota, but less is known about their roles in gut homeostasis (17). Furthermore, most studies are based on snapshots of microbiome landscapes at only one time point and there is no general, effective, and systematic detection method in clinic practice.
Intestinal dysbiosis refers to imbalance in the composition and abundance of gut microbiota and is associated with numerous diseases and phenotypes (18). For healthy individuals, the gut microbiota is in dynamic equilibrium, depending mainly on genetic factors, nutrient availability, dietary habits, psychological states, etc. (19–21). Once the balance is aberrant, disorders in the composition and structure of the intestinal microbiota transform the intestinal microecological environment from healthy state to disease state, subsequently resulting in the pathogenesis of various common metabolic disorders including obesity, type 2 diabetes, non-alcoholic liver disease, cardio-metabolic diseases, and malnutrition (22, 23). CVD is one such disease, which is closely related to metabolic disorders and inflammation (24). Therefore, changes in gut microbial community structure and diversity are also one of the key factors leading to the onset and development of CVDs, as revealed by accumulating evidence (25). This review highlights the changes of certain gut microbes in patients with atherosclerosis, hypertension, heart failure, myocardial infarction, atrial fibrillation (AF), and chronic kidney disease (CKD), respectively (Supplementary Table 1). As the results shown, patients with different CVDs imply various responses in terms of gut microbial diversity, abundance, and homogeneity. For example, studies on the intestinal flora of patients with prehypertension and hypertension have found that the most significant characteristics are the reduction and uneven distribution of microbial diversity and abundance, mainly manifested in the increase of Firmicutes/Bacteroidetes ratio, the decrease of probiotics, and the increase of Prevotella and Klebsiella (26). This result has been confirmed by microbiota transplantation experiment.
Gut microbiota-derived metabolites, such as short-chain fatty acids, BAs, catechin, and TMAO, are the direct factors causing CVDs, and have been viewed as a new breakthrough for the prevention and treatment of CVDs (27, 28). TMAO keeps increasing the risks of CVDs after excluding other risk factors for CVDs, demonstrating a strong association between high circulating levels of TMAO and the risk and mortality of CVDs (29, 30). Consequently, a high circulating level of TMAO is a potential marker to better stratify CVDs risks and predict short- and long-term major adverse cardiac events (31). Understanding the specific mechanisms of TMAO in the progression and pathogenesis of CVDs will facilitate the development of new therapeutic approaches, and holds great promise for precise intervention in the occurrence of CVDs (32).
The gut microbiota is an important regulator that influences cardiovascular function through the production of intestinal metabolites such as TMAO. The structure and function of the gut microbiota varies and is affected by dietary components, thus affecting the synthesis of TMAO. TMA is a precursor to TMAO, and its dietary precursors are generally choline, L-carnitine, and betaine (33). Dietary sources of choline are abundant and include eggs, fish, grains, milk, meat, and their derived food. Some vegetables, such as soybeans and potatoes, also contain a lesser amount of choline (34). L-carnitine is predominantly contained in red meat (35), while most betaine is found in fish, beetroot, and grains grown in high osmotic environments, such as beans (36). More information on dietary precursors of TMA is shown in Table 1.
These dietary components are metabolized to TMA under the participation of gut microbiota. However, despite the gut microbiota being instrumental in this process, only a few gut microbes have genes that utilize dietary precursors. Rath et al. found that less than 1% of the microbes in stool samples encoded the choline-utilizing TMA lyase system (CutC/D) and the carnitine Rieske-type oxygenase/reductase system (CntA/B), which depends on the availability of molecular oxygen (42). YeaW/X, which is a homolog of CntA/B, can also metabolize carnitine, choline, γ-butylbetaine, and betaine to generate TMA (43). Identification of these enzymes has made it possible to determine which bacteria synthesize TMA by using whole-genome sequencing analysis. The genes CntA/B have been detected in the genus Acinetobacter (e.g. Acinetobacter calcoaceticus, Acinetobacter baumannii), while the CutC/D genes have been detected in the genus Pelobacter (e.g. Pelobacter acetylenicus, Pelobacter carbinolicus) (44). These bacteria grow in different conditions, as shown in Supplementary Table 2. Consequently, different dietary precursors are degraded by different gut bacteria. Rath et al. showed that carnivores and omnivores had more CntA/B and CutC/D genes in their gut bacteria compared with herbivores, and these genes were found in more than 80 percent of gut bacteria. Overall, potential TMA-forming bacteria adapted to various nutritional conditions, although they were not evenly distributed (42). Most TMA produced in the gut is absorbed into the blood intravenously and into the liver by portal vein circulation. The liver is the home of TMA processing. Flavin-containing monooxygenases (FMOs) are abundant in liver microparticles and oxidize TMA to TMAO. For this catalytic reaction, FMOs require the cofactor flavin adenine dinucleotide and depend on nicotinamide adenine dinucleotide phosphate or nicotinamide adenine dinucleotide as a coenzyme (45). The reaction involves inserting an oxygen atom from O2 into TMA, while the other oxygen atom is reduced to water (46). FMOs in the liver are crucial in the production of TMAO, and experiments showed that FMO3 knockdown mice had decreased circulating levels of TMAO (32). Ultimately, TMAO is distributed throughout the body, being eliminated via the kidneys or accumulating in tissues (47).
TMA is a volatile gas that smells fishy, while TMAO is a less volatile solid with the formula (CH3)3 NO (48), as shown in Figure 1. TMAO has a high turnover rate, with more than 90% excreted in the urine after being metabolized by the kidney (49). A small amount of TMAO is excreted by respiration and feces, and the rest is reduced to TMA by TMAO reductase and re-enters the circulation. TMAO-an important stabilizer of protein and nucleic acid-stabilizes proteins by modifying their polarity, and indirectly affects the balance between unfolded and folded conformations (50). The physiological functions of TMAO in the host are determined by these features, such as influencing the protein unfolding or endoplasmic reticulum stress response in cells (51).
Figure 1 Molecular structure of TMAO. The formula is C3H9NO, which consists of three carbon atoms, nine hydrogen atoms, one nitrogen atom and one oxygen atom.
Studies have found that high levels of TMAO are closely related to the occurrence of atherosclerosis, HF, hypertension, and other CVDs (Figure 2). In this context, lifestyle, gender, drugs, age, and microbiota have a synergistic impact on the levels of TMAO (52). Dietary pattern plays a crucial role in human microbiota (53). Western diets are characterized by animal products containing large amounts of TMA precursors, and not only affect the gut microbiota but also increase levels of TMAO (54). Exercise also affects the integrity of the gut and the intestinal microbiota, and was found to effectively decrease TMAO levels (55). Considering the characteristics of TMAO formation, the activity of FMOs also affects TMAO levels. FMO3 has the highest activity among all types of FMOs capable of oxidizing TMA to TMAO. Males were reported to have lower expression levels of FMO3 compared with females, which resulted in differences in TMAO levels (56). The gender specificity in TMAO levels has been demonstrated in the livers of mice, but no difference in TMAO levels has been detected between human men and women (57). Drugs have been shown to have an impact on the human gut microbiota (58). For example, in human experiments conducted by Tang et al., TMAO plasma levels were markedly decreased after administration of antibiotics but returned to the previous level after withdrawal of antibiotics (59). In addition, plasma TMAO levels have been reported to gradually increase with age, and this process is regulated by changes in diet and an increase in abundance of TMA-producing bacteria (60).
Figure 2 The biogenesis and metabolism of TMAO. Dietary choline or L-carnitine in food was metabolized to TMA by gut microbiota, which converted to TMAO by FMO3 in the liver. TMAO was closely related to the development of atherosclerosis, HF, hypertension and other CVDs.
The mechanism of TMAO resulting in atherosclerosis, HF, and hypertension has gradually been clarified (Supplementary Table 3).
Atherosclerosis, which is the major risk factor of CVDs, is characterized by foam cell formation, inflammatory cell recruitment, and endothelial dysfunction (61). A high level of TMAO is thought to contribute to atherosclerosis, leading to a long-term risk of CVDs and poor prognosis in patients (32). The mechanism by which TMAO promotes the complex pathological processes of atherosclerosis is gradually being elucidated and includes cholesterol accumulation, activation of pro-inflammatory pathways, endothelial dysfunction, and thrombosis (62) (Figure 3).
Figure 3 The role of TMAO in onset and progression of atherosclerosis. High circulating levels of TMAO inhibited RCT, and accumulated cholesterol, which promoted the formation of foam cells; TMAO induced inflammatory responses, resulting in increased production of pro-inflammatory cytokines such as TNF-α and IL-1B; TMAO caused endothelial dysfunction and platelet activation, and ultimately contributed to the development of atherosclerosis.
There are multiple ways in which TMAO affects cholesterol metabolism and promotes the formation of foam cells. The reverse cholesterol transport (RCT) pathway involving high-density lipoprotein (HDL) is an important process to maintain the balance of cholesterol metabolism, and promotes the efflux of free cholesterol from macrophages (63). Studies found that HDL in the plasma of CVD patients with high TMAO levels was significantly reduced, resulting in inhibition of the RCT pathway and cholesterol accumulation in macrophages (64). TMAO also upregulated the expression of scavenger receptor cluster of differentiation 36 (CD36) and scavenger receptor-A1 (SR-A1) in macrophages, and induced cholesterol flow to macrophages. Wang et al. measured the contents of several scavenger receptors of macrophages in ApoE-/- mice and found that CD36 and SRA1 levels were increased in macrophages in TMAO-treated mice compared with control mice (65). Under the interference of these factors, cholesterol-overloaded macrophages developed into foam cells, which is one of the earliest cellular hallmarks of atherosclerosis (66). Furthermore, TMAO is closely related to BA metabolism, negatively impacting the main pathway of cholesterol elimination in humans. Cholesterol is concentrated in the liver by the RCT pathway and then secreted into the bile in the form of unesterified cholesterol by the ATP-binding cassette (ABC) transporters G5 (ABCG5) and G8 (ABCG8) (67). Alternatively, cholesterol can be synthesized as BAs, which are then transported to bile via a bile salt export pump (BSEP) and finally excreted with feces (68). In addition to the hepatic cholesterol excretion pathway, the ABG5/G8 heterodimer promotes cholesterol excretion from the intestinal cells directly to the intestinal lumen. In C57BL/6J mice, TMAO significantly decreased the expression of ABCG5/G8 (69). Moreover, TMAO activated the farnesoid X receptor (FXR) and small heterodimer partner (SHP), downregulating the expression of hepatic BA synthase cytochrome P450 family 7 subfamily A member 1 (CYP7A1), cytochrome P450 family 27 subfamily A member 1 (CYP27A1), and BA transporter organic anion-transporting polypeptide (OATP) in ApoE-/- mice (70). Inhibition of the related enzymes reduced cholesterol elimination, causing the accumulation of cholesterol in the liver. Cholesterol then re-entered the blood through the low-density lipoprotein (LDL) cycle, which increased foam cell formation and accelerated the process of atherosclerosis.
Atherosclerosis is also considered a chronic inflammatory disease because the endothelial inflammatory damage is ongoing throughout atherosclerosis progression (71). High TMAO levels were found to induce activation of the nuclear factor-kappa B (NF-κB) pathway and increase the expression of proinflammatory genes including those encoding inflammatory cytokines, adhesion molecules, and chemokines (72). Similar results were reported in the study of Chen et al., where high TMAO levels increased the expression of tumor necrosis factor-α (TNF-α) and interleukin-6 (IL-6), but inhibited the expression of anti-inflammatory cytokine interleukin-10 (IL-10), thereby accelerating cellular inflammation in obese mice treated with a Western diet (73). Furthermore, in vitro studies of cultured endothelial progenitor cells (EPCs) by Chou et al. showed that TMAO not only promoted cellular inflammation but also accounted for oxidative stress, thus playing an indirect role in promoting expression of inflammatory cytokines (74).
In human umbilical vein endothelial cells (HUVECs) and aortas from ApoE-/- mice, TMAO increased the concentrations of reactive oxygen species (ROS), especially superoxide, by inhibiting the SIRT3-SOD2-mitochondrial ROS signaling pathway (75). The increase of ROS and TMAO-derived oxidative stress-related molecule malondialdehyde (MDA) impaired the activity of endothelial nitric oxide synthase (eNOS), contributing to decreased nitric oxide (NO) bioavailability and endothelial dysfunction in aged rats (76, 77). In addition, thioredoxin-interacting protein (TXNIP) is an important protein that mediates ROS and the NOD-like receptor protein 3 (NLRP3) inflammasome (78). Upregulation of TXNIP by ROS stimulated the NLRP3 inflammasome, which activated the protease caspase-1 to facilitate the release of inflammatory cytokines such as interleukin-18 (IL-18) and interleukin-1β (IL-1β) and triggered inflammatory responses. Activated inflammatory factors also aggravated the damage caused by oxidative stress in a positive feedback manner. For example, TNF-α and IL-6 reduced the production of NO by inhibiting the phosphorylation of eNOS (79). Oxidative stress and inflammation reinforced each other in a continuous cycle that accelerated atherosclerosis (80).
TMAO may lead to endothelial dysfunction. Endothelial tight junction proteins play a barrier role in regulating cell permeability and maintaining cell polarity (81). TMAO activated the expression of the inflammatory mediator high mobility group box 1 (HMGB1), which stimulated multiple receptors such as toll-like receptor 4 (TLR4), thus mediating extracellular signal-regulated kinases 1 and 2 (ERK1 and ERK2) and the NF-κB signaling pathway (82). Activation of this pathway destroyed the expression of tight junction proteins such as zonula occludens-2 (ZO-2), occludin, and vascular endothelial cadherin (VE-cadherin) in endothelial cells, which increased endothelial cell permeability. Gaps between the endothelial cells allowed LDL to easily enter the intima and be oxidized to oxidized low-density lipoprotein (ox-LDL) (83). In addition, TMAO also activated proteinase C, and induced functional damage of vascular endothelial cells through the PKC/NF-κB pathway. With the induction of the NF-κB signaling pathway in HUVECs, the subsequent upregulation of expression of vascular cell adhesion molecule-1 (VCAM-1) and intercellular adhesion molecule-1 (ICAM-1) enhanced the adhesion of monocytes to the vascular cells (84). Activated monocytes were recruited to the intima and differentiated into macrophages, accelerating endothelial dysfunction (85). In addition, TMAO inhibited endothelial cell proliferation and impaired endothelial self-repairment (86). Platelet hyperreactivity and thrombosis are also associated with TMAO (87). According to experiments by Zhu et al., TMAO improved the expression of adenosine diphosphate (ADP) and thrombin to enhance a release of Ca2+ from intracellular calcium stores, causing platelet aggregation and enhancing thrombosis potential (88).
TMAO, as one of the independent risk factors of CVDs, is widely distributed in human tissues and body fluids such as urine, blood, feces, bile, and semen. Once TMAO formation/concentration is elevated, it may increase the risks of atherosclerosis for healthy individuals (48). However, owing to the complexity of individual physiological characteristics, the threshold concentration of TMAO that causes atherosclerosis is not clear.
HF is defined as a group of syndromes caused by structural or functional damage that results in impaired ventricular filling or cardiac output, and which involves multi-organ and systemic neurohumoral dysfunction (89). As the terminal stage of various CVDs, HF is associated with a persistently high rate of morbidity, hospital readmission, and mortality in patients and has become a major public health problem worldwide, with more than 60 million cases diagnosed globally (47). Although there is an active search for effective treatments of HF, up to 50% of diagnosed patients have died in the past 30 years, indicating that the pathogenesis of this disease has not yet been fully elucidated (90). Accumulating evidence has indicated that TMAO is involved in the progression of HF (91). A clinical study in patients with HF revealed that plasma TMAO levels were positively associated with the risk of chronic HF, suggesting that TMAO might be a new target for the treatment of HF (49, 92). In addition to inducing HF by promoting atherosclerotic progression, elevated plasma TMAO levels may accelerate myocardial hypertrophy, exacerbate mitochondrial dysfunction, and induce transverse-tubule (T-tubule) remodeling in cardiomyocytes (47, 93). These pathophysiological mechanisms have the potential to form a vicious circle that further aggravates the development of HF (Figure 4).
Figure 4 Molecular mechanisms of HF progression regulated by TMAO. In cardiomyocytes, TMAO activated TGF-β1/Smad3 pathway and increased expression levels of ANP and β-MHC, which caused myocardial hypertrophy and fibrosis; TMAO decreased energy metabolism and mitochondrial function by inhibiting the activity of pyruvate, leading to myocardial damage; TMAO also negatively affected the intracellular Ca2+ processing and myocardial contraction.
TMAO significantly increased the mRNA and protein levels of the hypertrophic markers atrial natriuretic peptide (ANP) and β-myosin heavy chain (β-MHC), causing myocardial hypertrophy and fibrosis (94). In mice fed a high choline diet, myocardial hypertrophy induced by TMAO was achieved through the involvement of transforming growth factor-β1 (TGF-β1) and small mothers against decapentaplegic (Smad), an intermediate effector molecule in the TGF-β superfamily signaling pathway (95). High levels of TMAO activated the TGF-β1 receptor, and then Smad3 was phosphorylated at its C-terminus by the TGF-β1 receptor. Subsequently, phosphorylated Smad3 bound to Smad4 (the partner Smad) and was transported from the cytoplasm to the nucleus to activate the transcription of downstream target genes related to ANP and β-MHC (96). In addition, TMAO induced myocardial inflammation, which may lead to myocardial fibrosis and cardiac dysfunction in western diet-induced obese mice (97). Zhang et al. found that mice fed on diets supplemented with high sugar and fat had elevated plasma TMAO levels, accompanied with increased TNF-α levels and decreased IL-10 levels, which led to myocardial fibrosis (98).
The accumulation of TMAO in cardiac tissue led to disturbed energy metabolism and impaired myocardial function (99). Savi et al. found that TMAO induced mitochondrial dysfunction, thereby decreasing contractility of cardiomyocytes (100). Mitochondria in cardiomyocytes consume pyruvate and fatty acids to generate energy, which maintains the physiological function of the heart. However, TMAO inhibited the activity of pyruvate dehydrogenase (PDH) and weakened the oxidation of pyruvate in mitochondria, which impaired production of ATP (101). The decrease in ATP resulted in a decline in contractile capacity of the cardiomyocytes. TMAO also caused glycogen accumulation and lipofuscin-like pigment deposition in the paranuclear area of cardiomyocytes, which generated damage to mitochondria, the proteasome system, and lysosomal function (102).
TMAO disturbed myocardial excitation-contraction coupling by inducing Ca2+ dysfunction (103). Microtubules formed by the polymerization of α- and β-tubulin dimers are ubiquitous cytoskeletal fibers in cells and are instrumental in maintaining ventricular shape (104). In a HF model with coarctation of the aorta, mice fed a high-cholesterol diet had higher TMAO levels and accelerated adverse ventricular remodeling (105). Jin et al. reported the same conclusion, with TMAO promoting tubulin densification and increased polymerization and redistribution of proteins such as Junctophilin-2 (JPH2). The translocated JPH2 spanned T-tubule and sarcoplasmic reticulum (SR) membranes and enabled T-tubule remodeling in cardiomyocytes (106, 107). The remodeling of T-tubules destroys accurate communication between L-type voltage-gated Ca2+ channels (VGCCs), located predominantly on the T-tubule membrane, and type 2 ryanodine receptor (RyR2), a Ca2+-release channel located on the sarcoplasmic reticulum (108). This disrupted communication leads to a massive efflux of Ca2+. The Ca2+ dysfunction impairs the excitation-contraction coupling of the myocardium and worsens HF.
In addition to myocardial injury, TMAO indirectly affects the progression of HF by influencing renal function (109). Tang et al. reported that a high level of TMAO increased Smad3 phosphorylation, which promoted renal interstitial fibrosis and dysfunction. Consequently, sodium and water were retained in the kidneys, which increased cardiac preload and exacerbated HF (110).
Based on the role of TMAO in the progression of HF, regulating TMAO levels is a new and attractive therapeutic target for HF treatment (111). However, considering the diversity of gut microbiota and TMAO levels in patients with HF, specific treatment strategies need to be developed.
By 2025, the total number of patients with hypertension is expected to reach 1.56 billion and the average age of patients tends to be younger (112). More than 95% of hypertension is related to lifestyle, hence a healthy lifestyle tends to better control the onset and development of CVDs (113). Several dietary interventions, such as the Mediterranean diet (Med-Diet) and the Dietary Approaches to Stop Hypertension (DASH) diet, have shown that a high intake of fruits, vegetables, and fiber is conducive to alleviating hypertension (114). However, there are other factors involved in the development of hypertension, and studies have found a link between the gut microbiome, microbial metabolites, and hypertension (115). In the hypertensive rat model, Yang et al. found that microbial abundance and diversity were reduced (116). Meanwhile, a meta-analysis of population-based studies found that individuals with high TMAO levels were more likely to develop hypertension compared with those with low TMAO levels (117). TMAO prolongs the hypertensive effect of angiotensin II (Ang II) and increases water reabsorption and has emerged as a potential biomarker of hypertension (Figure 5).
Figure 5 Summary schemes illustrating the involvement of TMAO in hypertension. TMAO prolonged the hypertensive effect of Ang II, and elevated blood pressure via RAAS; TMAO stimulated the secretion of AVP, increasing water reabsorption through the ‘TMAO-AVP-AQP-2 Axis’, which contributed to the progression of hypertension.
Ang II is the main effector of the renin-angiotensin-aldosterone system (RAAS), and studies have shown that Ang II promoted the development of hypertension through RAAS system (118). In hypertensive mice induced by Ang II, TMAO changed the structure of Ang II by enhancing folding and processing of proteins, thereby prolonging the duration of hypertensive effect (119). In the adrenal medulla, Ang II promoted the synthesis of norepinephrine, which contracted the vascular arteriolar smooth muscle and increased blood pressure; meanwhile, in the adrenal cortex, Ang II stimulated the secretion of aldosterone, thereby increasing the absorption of water and sodium in collecting duct principal cells (120).
In a spontaneous hypertensive rat model, TMAO increased plasma osmotic pressure and water reabsorption via the ‘TMAO-AVP-(AQP-2) axis’, which contributed to hypertension (121). High TMAO levels promoted the elevation of plasma osmotic pressure, increasing the secretion of arginine vasopressin (AVP) from the posterior pituitary. The effects of AVP were initiated through the binding of AVP to the type-2 vasopressin receptor (V2R) on the basolateral membrane of principal cells in the kidney collecting duct (122). This binding activated cyclic adenosine monophosphate/protein kinase A (cAMP/PKA) signaling pathway, resulting in the translocation of aquaporin-2 (AQP2) from the vesicle to the apical plasma membrane and markedly increasing the water reabsorption of principal cells (123). AVP also stimulated the type-1 vasopressin receptor (V1R) of vascular smooth muscle to cause vasoconstriction and increase blood pressure. Additionally, TMAO induced hypertension by accelerating vascular endothelial dysfunction (124).
Arrhythmia, which primarily includes AF, ventricular arrhythmia (VA), and atrioventricular block, is emerging as an intractable CVD that leads to HF or sudden cardiac death (62). Meng et al. first proposed that TMAO may be a target to treat arrhythmia (125). A study in a community cohort of elderly participants demonstrated that there was a positive association between plasma TMAO levels and long-term incident AF in patients with suspected stable angina (126). TMAO also promoted the development of VA in various ways and several of these reports highlighted ischemia-induced VA. Gong et al. found that TMAO promoted the release of pro-inflammatory cytokines such as IL-1β and TNF-α to stimulate the cardiac autonomic nervous system (CANS) and worsen ischemia-induced VA (127). In addition, TMAO significantly promoted ischemic-induced VA by modifying the left stellate ganglion (LSG) either directly or through the ‘gut-brain-heart’ axis (125).
CAD is a CVD with the highest morbidity and mortality in the world, and is closely related to intestinal microbiota dysbiosis (128). According to the research, gut microbiota may be an important diagnostic marker for CAD in the future (129). As an active metabolite synthesized by intestinal microbiota, the increased level of TMAO is associated with increased risks of CAD and all-cause mortality (130, 131). In addition, Guo et al. reported that associations of TMAO with CAD were sex-related (132). However, although TMAO may be a potential target for the prevention and treatment of CAD, current immature conclusions limit clinical applications at present.
In addition to CVDs, TMAO promotes numerous related diseases, such as diabetes, CKD, etc., which have a burden of cardiovascular complications (133, 134). Li et al. showed that elevation of plasma TMAO increased the chance of developing gestational diabetes by 22% (135). TMAO stimulated gluconeogenesis and caused insulin resistance, which promoted the progression of diabetes (136). As a major risk factor for CVDs and cardiovascular complications, the relationship between plasma TMAO concentrations and the increased risks of CVDs in individuals with type 1 and 2 diabetes has been reported (137–139). According to a study by Winther et al., higher concentrations of plasma TMAO were related to mortality, CVDs, and poor renal outcome in patients with type 1 diabetes (137). Similar results were reported in the clinical study of patients with type 2 diabetes, where increased TMAO levels are independently associated with risks of adverse cardiovascular events (138, 139). Furthermore, some studies on the relationship between TMAO levels and CVDs identified kidney function as an important confounder, owing to a degree of decline in the renal function of patients with CVDs (140). Elevated TMAO levels damaged renal medulla cells and influenced glomerular filtration, which caused water and sodium retention and impaired the kidneys (30). Another study suggested that plasma TMAO levels were inversely correlated with long-term survival in patients with CKD (141). Despite these positive findings, additional research is needed to clarify the specific mechanism of TMAO involvement in diseases related to CVDs.
Increasing numbers of experiments that are aimed at altering host gut microbiota and interfering with the TMAO synthesis pathway as therapeutic means have been conducted to prevent and treat CVDs. Considering the multifactorial nature of TMAO generation, many strategies can be pursued to reduce the level of TMA/TMAO in patients with CVDs, such as lifestyle changes, use of antibiotics and functional foods, development of related inhibitors, and improvement of gut microbiota (Supplementary Table 4).
Choline, phosphatidylcholine, and L-carnitine are the precursors of TMA and are found in high levels in the daily diet, especially in red meat (142). Reducing the intake of red meat can theoretically reduce the level of TMA in the body, however, there is no universal international control standard. Besides, a vegetarian lifestyle, with multiple health benefits, also reduces the risk of CVDs (143).
Numerous studies have shown that the use of antibiotics can significantly reduce circulating TMAO levels, possibly due to changes in the gut microbiota composition. However, the use of antibiotics also poses concerns, such as transient changes in circulating TMAO levels, increased risk of bacterial resistance, and other antibiotic safety problems (144). According to one study, older women who took antibiotics for years were prone to inflammation caused by intestinal microbiota translocation (145). Therefore, the use of antibiotics needs caution.
Animal experiments showed that inhibition of choline TMA lyase by 3,3-dimethyl-1-butanol (DMB) could reduce plasma TMAO levels and aortic lesions in mice; however, human clinical experiments have not been performed (146). Another blocking method is to inhibit hepatic FMO3 activity. Chen et al. found that using 3,3′-diindolyl methane or indole-3-carbinol to inhibit FMO3 could be a suitable target to control TMAO production (147).
Probiotics are beneficial living microbes and represent a great way to improve gut microbiota. In contrast to antibiotics, probiotics are inexpensive, noninvasive, and have few side effects. Multiple studies have shown the positive effects of probiotics in reducing TMA levels and alleviating CVDs, with the probiotics including Enterobacter aerogenes, Lactobacillus rhamnosus, Saccharomyces boulardii, and Bifidobacterium animalis subsp. lactis (148–151). Probiotics present an easy way to reduce possible cardiovascular risks and further studies are underway to identify more microbial strains with such benefits.
Prebiotics include galactooligosaccharides, fructooligosaccharides, inulin, dietary fiber, etc. that are utilized by host microbes (152, 153). Prebiotics can promote the growth of beneficial bacteria in the gut and decrease bacteria able to transform precursors into TMA. For example, mice fed resveratrol had remodeled gut microbiota and decreased production of TMA (154).
FMT was successfully applied in animal models and restored the structure of the gut microbiota in mice (155). However, an experiment on humans showed that FMT normalized insulin sensitivity of obese subjects with metabolic syndrome, but the effects were short-term (156). FMT has great potential as a treatment for CVDs, and further studies are needed to increase its efficacy and safety in reducing the risks of CVDs (157).
In conclusion, high concentrations of TMAO are associated with the development of various CVDs, such as atherosclerosis, hypertension, HF, arrhythmia, and CAD. However, although there are many reports on the harmful effects of TMAO, there are still some studies suggesting that TMAO plays a beneficial role. One experiment suggested that TMAO enhanced the stability of cellular proteins, which protected cells against the deleterious effects of various stressors. Furthermore, TMAO alleviated the formation of aortic atherosclerotic lesions in ApoE-/- mice. Therefore, additional research is needed to determine the role of TMAO in CVDs.
CVDs remain the leading cause of death globally, despite the availability of numerous clinical treatments for CVDs. Gut microbiota and TMAO affect CVDs in various ways and interact with each other, and this link provides an opportunity to identify TMAO as a new therapeutic target to treat CVDs. Many studies have modulated the diversity of gut microbiota and TMAO/TMA levels in patients through dietary intervention, probiotics, and FMT. These strategies regulate gut microbial structure, reduce TMAO levels, and reduce the risk of CVDs. However, the complex composition of gut microbiota means that the gut microbiota that contributes most to CVDs and the specific mechanism of TMAO are not fully understood. Current understanding is predominantly based on animal experiments. Consequently, longitudinal studies in humans are needed to determine the role of gut microbiota and TMAO in CVDs and facilitate the development of better therapeutic strategies.
JZ, ZZ, MH and H-XH wrote the original draft. E-HL, P-BW, XL, Y-TW, X-CC, J-QT and M-YZ revised the manuscript. LX and X-XK conceived the manuscript. All authors agreed to be accountable for the content of the work. All authors contributed to the article and approved the submitted version.
The research is financially supported by the Shuangchuang Ph.D award of Jiangsu Province (No.JSSCBS20211252), the initializing Fund of Xuzhou Medical University (No.D2020013), the National College Students of Innovation and Entrepreneurship Training Program in Jiangsu Province (No.202110313048Y) and the Natural Science Foundation of Jiangsu Higher Education Institutions of China (No.22KJB310021 and No.22KJB520040).
The authors declare that the research was conducted in the absence of any commercial or financial relationships that could be construed as a potential conflict of interest.
All claims expressed in this article are solely those of the authors and do not necessarily represent those of their affiliated organizations, or those of the publisher, the editors and the reviewers. Any product that may be evaluated in this article, or claim that may be made by its manufacturer, is not guaranteed or endorsed by the publisher.
The Supplementary Material for this article can be found online at: https://www.frontiersin.org/articles/10.3389/fendo.2023.1085041/full#supplementary-material
1. Qin J, Li R, Raes J, Arumugam M, Burgdorf KS, Manichanh C, et al. A human gut microbial gene catalogue established by metagenomic sequencing. Nature (2010) 464:59–65. doi: 10.1038/nature08821
2. Group NHW, Peterson J, Garges S, Giovanni M, McInnes P, Wang L, et al. The NIH human microbiome project. Genome Res (2009) 19:2317–23. doi: 10.1101/gr.096651.109
3. Roth GA, Mensah GA, Johnson CO, Addolorato G, Ammirati E, Baddour LM, et al. Global burden of cardiovascular diseases and risk factors, 1990–2019 update from the GBD 2019 study. J Am Coll Cardiol (2020) 76:2982–3021. doi: 10.1016/j.jacc.2020.11.010
4. Cuspidi C, Tadic M, Grassi G, Mancia G. Treatment of hypertension: The ESH/ESC guidelines recommendations. Pharmacol Res (2018) 128:315–21. doi: 10.1016/j.phrs.2017.10.003
5. Lau K, Srivatsav V, Rizwan A, Nashed A, Liu R, Shen R, et al. Bridging the gap between gut microbial dysbiosis and cardiovascular diseases. Nutrients (2017) 9:859. doi: 10.3390/nu9080859
6. Peng J, Xiao X, Hu M, Zhang X. Interaction between gut microbiome and cardiovascular disease. Life Sci (2018) 214:153–7. doi: 10.1016/j.lfs.2018.10.063
7. Fan Y, Pedersen O. Gut microbiota in human metabolic health and disease. Nat Rev Microbiol (2021) 19:55–71. doi: 10.1038/s41579-020-0433-9
8. Gruppen EG, Garcia E, Connelly MA, Jeyarajah EJ, Otvos JD, Bakker SJL, et al. TMAO is associated with mortality: Impact of modestly impaired renal function. Sci Rep (2017) 7:13781. doi: 10.1038/s41598-017-13739-9
9. Roberts AB, Gu X, Buffa JA, Hurd AG, Wang Z, Zhu W, et al. Development of a gut microbe-targeted nonlethal therapeutic to inhibit thrombosis potential. Nat Med (2018) 24:1407–17. doi: 10.1038/s41591-018-0128-1
10. Brown JM, Hazen SL. The gut microbial endocrine organ: Bacterially derived signals driving cardiometabolic diseases. Annu Rev Med (2015) 66:343–59. doi: 10.1146/annurev-med-060513-093205
11. Maynard CL, Elson CO, Hatton RD, Weaver CT. Reciprocal interactions of the intestinal microbiota and immune system. Nature (2012) 489:231–41. doi: 10.1038/nature11551
12. Moore-Connors JM, Dunn KA, Bielawski JP, Van Limbergen J. Novel strategies for applied metagenomics. Inflamm Bowel Dis (2016) 22:709–18. doi: 10.1097/MIB.0000000000000717
13. Cardinelli CS, Sala PC, Alves CC, Torrinhas RS, Waitzberg DL. Influence of intestinal microbiota on body weight gain: A narrative review of the literature. Obes Surg (2015) 25:346–53. doi: 10.1007/s11695-014-1525-2
14. Gomaa EZ. Human gut Microbiota/Microbiome in health and diseases: A review. Antonie Van Leeuwenhoek (2020) 113:2019–40. doi: 10.1007/s10482-020-01474-7
15. Lin L, Zhang J. Role of intestinal microbiota and metabolites on gut homeostasis and human diseases. BMC Immunol (2017) 18:2. doi: 10.1186/s12865-016-0187-3
16. Xiao HW, Cui M, Li Y, Dong JL, Zhang SQ, Zhu CC, et al. Gut microbiota-derived indole 3-propionic acid protects against radiation toxicity via retaining acyl-CoA-Binding protein. Microbiome (2020) 8:69. doi: 10.1186/s40168-020-00845-6
17. Sarangi AN, Goel A, Aggarwal R. Methods for studying gut microbiota: A primer for physicians. J Clin Exp Hepatol (2019) 9:62–73. doi: 10.1016/j.jceh.2018.04.016
18. Garg Y, Kanwar N, Dodiya H, Chopra S, Tambuwala M, Bhatia A, et al. Microbiome medicine: Microbiota in development and management of cardiovascular diseases. Endocr Metab Immune Disord Drug Targets (2022) 22:1344–56. doi: 10.2174/1871530322666220624161712
19. Iddir M, Brito A, Dingeo G, Fernandez Del Campo SS, Samouda H, La Frano MR, et al. Strengthening the immune system and reducing inflammation and oxidative stress through diet and nutrition: Considerations during the COVID-19 crisis. Nutrients (2020) 12:1562. doi: 10.3390/nu12061562
20. Kumar A, Chidambaram V, Mehta JL. Vegetarianism, microbiota and cardiovascular health: Looking back, and forward. Eur J Prev Cardiol (2022) 29:1895–910. doi: 10.1093/eurjpc/zwac128
21. Lach G, Schellekens H, Dinan TG, Cryan JF. Anxiety, depression, and the microbiome: A role for gut peptides. Neurotherapeutics (2018) 15:36–59. doi: 10.1007/s13311-017-0585-0
22. Spor A, Koren O, Ley R. Unravelling the effects of the environment and host genotype on the gut microbiome. Nat Rev Microbiol (2011) 9:279–90. doi: 10.1038/nrmicro2540
23. Kang Y, Cai Y. Gut microbiota and obesity: Implications for fecal microbiota transplantation therapy. Hormones (Athens) (2017) 16:223–34. doi: 10.14310/horm.2002.1742
24. Sanchez-Rodriguez E, Egea-Zorrilla A, Plaza-Díaz J, Aragón-Vela J, Muñoz-Quezada S, Tercedor-Sánchez L, et al. The gut microbiota and its implication in the development of atherosclerosis and related cardiovascular diseases. Nutrients (2020) 12:605. doi: 10.3390/nu12030605
25. Zhu B, Ren H, Xie F, An Y, Wang Y, Tan Y. Trimethylamine n-oxide generated by the gut microbiota: Potential atherosclerosis treatment strategies. Curr Pharm Des (2022) 28:2914–19. doi: 10.2174/1381612828666220919085019
26. Li J, Zhao F, Wang Y, Chen J, Tao J, Tian G, et al. Gut microbiota dysbiosis contributes to the development of hypertension. Microbiome (2017) 5:14. doi: 10.1186/s40168-016-0222-x
27. Lu Y, Zhang Y, Zhao X, Shang C, Xiang M, Li L, et al. Microbiota-derived short-chain fatty acids: Implications for cardiovascular and metabolic disease. Front Cardiovasc Med (2022) 9:900381. doi: 10.3389/fcvm.2022.900381
28. Zhou X, Jin M, Liu L, Yu Z, Lu X, Zhang H. Trimethylamine n-oxide and cardiovascular outcomes in patients with chronic heart failure after myocardial infarction. ESC Heart Failure (2020) 7:188–93. doi: 10.1002/ehf2.12552
29. Sanchez-Gimenez R, Ahmed-Khodja W, Molina Y, Peiro OM, Bonet G, Carrasquer A, et al. Gut microbiota-derived metabolites and cardiovascular disease risk: A systematic review of prospective cohort studies. Nutrients (2022) 14:2654. doi: 10.3390/nu14132654
30. Nam HS. Gut microbiota and ischemic stroke: The role of trimethylamine n-oxide. J Stroke (2019) 21:151–9. doi: 10.5853/jos.2019.00472
31. Guasti L, Galliazzo S, Molaro M, Visconti E, Pennella B, Gaudio GV, et al. TMAO as a biomarker of cardiovascular events: A systematic review and meta-analysis. Intern Emerg Med (2021) 16:201–7. doi: 10.1007/s11739-020-02470-5
32. Duttaroy AK. Role of gut microbiota and their metabolites on atherosclerosis, hypertension and human blood platelet function: A review. Nutrients (2021) 13:144. doi: 10.3390/nu13010144
33. He S, Jiang H, Zhuo C, Jiang W. Trimethylamine/Trimethylamine-N-Oxide as a key between diet and cardiovascular diseases. Cardiovasc Toxicol (2021) 21:593–604. doi: 10.1007/s12012-021-09656-z
34. Borges NA, Stenvinkel P, Bergman P, Qureshi AR, Lindholm B, Moraes C, et al. Effects of probiotic supplementation on trimethylamine-N-Oxide plasma levels in hemodialysis patients: A pilot study. Probiotics Antimicrob Proteins (2019) 11:648–54. doi: 10.1007/s12602-018-9411-1
35. Koeth RA, Lam-Galvez BR, Kirsop J, Wang Z, Levison BS, Gu X, et al. L-carnitine in omnivorous diets induces an atherogenic gut microbial pathway in humans. J Clin Invest (2019) 129:373–87. doi: 10.1172/JCI94601
36. Zeisel SH, Warrier M. Trimethylamine n-oxide, the microbiome, and heart and kidney disease. Annu Rev Nutr (2017) 37:157–81. doi: 10.1146/annurev-nutr-071816-064732
37. Wiedeman AM, Barr SI, Green TJ, Xu Z, Innis SM, Kitts DD. Dietary choline intake: Current state of knowledge across the life cycle. Nutrients (2018) 10:1513. doi: 10.3390/nu10101513
38. Arias N, Arboleya S, Allison J, Kaliszewska A, Higarza SG, Gueimonde M, et al. The relationship between choline bioavailability from diet, intestinal microbiota composition, and its modulation of human diseases. Nutrients (2020) 12:2340. doi: 10.3390/nu12082340
39. Aldana-Hernandez P, Azarcoya-Barrera J, van der Veen JN, Leonard KA, Zhao YY, Nelson R, et al. Dietary phosphatidylcholine supplementation reduces atherosclerosis in ldlr-/- Male Mice2. J Nutr Biochem (2021) 92:108617. doi: 10.1016/j.jnutbio.2021.108617
40. Wang C, Ma C, Gong L, Dai S, Li Y. Preventive and therapeutic role of betaine in liver disease: A review on molecular mechanisms. Eur J Pharmacol (2021) 912:174604. doi: 10.1016/j.ejphar.2021.174604
41. Du J, Zhang P, Luo J, Shen L, Zhang S, Gu H, et al. Dietary betaine prevents obesity through gut microbiota-drived MicroRNA-378a family. Gut Microbes (2021) 13:1–19. doi: 10.1080/19490976.2020.1862612
42. Rath S, Rud T, Pieper DH, Vital M. Potential TMA-producing bacteria are ubiquitously found in Mammalia. Front Microbiol (2019) 10:2966. doi: 10.3389/fmicb.2019.02966
43. Koeth RA, Levison BS, Culley MK, Buffa JA, Wang Z, Gregory JC, et al. Gamma-butyrobetaine is a proatherogenic intermediate in gut microbial metabolism of l-carnitine to TMAO. Cell Metab (2014) 20:799–812. doi: 10.1016/j.cmet.2014.10.006
44. Jameson E, Quareshy M, Chen Y. Methodological considerations for the identification of choline and carnitine-degrading bacteria in the gut. Methods (2018) 149:42–8. doi: 10.1016/j.ymeth.2018.03.012
45. Romero E, Gómez Castellanos JR, Gadda G, Fraaije MW, Mattevi A. Same substrate, many reactions: Oxygen activation in flavoenzymes. Chem Rev (2018) 118:1742–69. doi: 10.1021/acs.chemrev.7b00650
46. Reis RAG, Li H, Johnson M, Sobrado P. New frontiers in flavin-dependent monooxygenases. Arch Biochem Biophys (2021) 699:108765. doi: 10.1016/j.abb.2021.108765
47. Lv S, Wang Y, Zhang W, Shang H. Trimethylamine oxide: A potential target for heart failure therapy. Heart (2022) 108:917–22. doi: 10.1136/heartjnl-2021-320054
48. Ufnal M, Zadlo A, Ostaszewski R. TMAO: A small molecule of great expectations. Nutrition (2015) 31:1317–23. doi: 10.1016/j.nut.2015.05.006
49. Bennett BJ, de Aguiar Vallim TQ, Wang Z, Shih DM, Meng Y, Gregory J, et al. Trimethylamine-N-Oxide, a metabolite associated with atherosclerosis, exhibits complex genetic and dietary regulation. Cell Metab (2013) 17:49–60. doi: 10.1016/j.cmet.2012.12.011
50. Ma J, Pazos IM, Gai F. Microscopic insights into the protein-stabilizing effect of trimethylamine n-oxide (TMAO). Proc Natl Acad Sci USA (2014) 111:8476–81. doi: 10.1073/pnas.1403224111
51. Lupachyk S, Watcho P, Stavniichuk R, Shevalye H, Obrosova IG. Endoplasmic reticulum stress plays a key role in the pathogenesis of diabetic peripheral neuropathy. Diabetes (2013) 62:944–52. doi: 10.2337/db12-0716
52. Thomas MS, Fernandez ML. Trimethylamine n-oxide (TMAO), diet and cardiovascular disease. Curr Atheroscler Rep (2021) 23:12. doi: 10.1007/s11883-021-00910-x
53. Zeisel SH, Mar MH, Howe JC, Holden JM. Concentrations of choline-containing compounds and betaine in common foods. J Nutr (2003) 133:1302–7. doi: 10.1093/jn/133.5.1302
54. Yoo W, Zieba JK, Foegeding NJ, Torres TP, Shelton CD, Shealy NG, et al. High-fat diet-induced colonocyte dysfunction escalates microbiota-derived trimethylamine n-oxide. Science (2021) 373:813–8. doi: 10.1126/science.aba3683
55. Dannenberg L, Zikeli D, Benkhoff M, Ahlbrecht S, Kelm M, Levkau B, et al. Targeting the human microbiome and its metabolite TMAO in cardiovascular prevention and therapy. Pharmacol Ther (2020) 213:107584. doi: 10.1016/j.pharmthera.2020.107584
56. Ripp SL, Itagaki K, Philpot RM, Elfarra AA. Species and sex differences in expression of flavin-containing monooxygenase form 3 in liver and kidney microsomes. Drug Metab Dispos (1999) 27:46–52.
57. Schiattarella GG, Sannino A, Toscano E, Giugliano G, Gargiulo G, Franzone A, et al. Gut microbe-generated metabolite trimethylamine-N-Oxide as cardiovascular risk biomarker: A systematic review and dose-response meta-analysis. Eur Heart J (2017) 38:2948–56. doi: 10.1093/eurheartj/ehx342
58. Ferrer M, Méndez-García C, Rojo D, Barbas C, Moya A. Antibiotic use and microbiome function. Biochem Pharmacol (2017) 134:114–26. doi: 10.1016/j.bcp.2016.09.007
59. Tang WHW, Wang Z, Levison BS, Koeth RA, Britt EB, Fu X, et al. Intestinal microbial metabolism of phosphatidylcholine and cardiovascular risk. N Engl J Med (2013) 368:1575–84. doi: 10.1056/NEJMoa1109400
60. Rath S, Rox K, Kleine Bardenhorst S, Schminke U, Dörr M, Mayerle J, et al. Higher trimethylamine-N-Oxide plasma levels with increasing age are mediated by diet and trimethylamine-forming bacteria. MSystems (2021) 6:e0094521. doi: 10.1128/mSystems.00945-21
61. Gui T, Shimokado A, Sun Y, Akasaka T, Muragaki Y. Diverse roles of macrophages in atherosclerosis: From inflammatory biology to biomarker discovery. Mediators Inflammation (2012) 2012:693083. doi: 10.1155/2012/693083
62. Zhou W, Cheng Y, Zhu P, Nasser MI, Zhang X, Zhao M. Implication of gut microbiota in cardiovascular diseases. Oxid Med Cell Longev (2020) 2020:5394096. doi: 10.1155/2020/5394096
63. Lee-Rueckert M, Escola-Gil JC, Kovanen PT. HDL functionality in reverse cholesterol transport-challenges in translating data emerging from mouse models to human disease. Biochim Biophys Acta (2016) 1861:566–83. doi: 10.1016/j.bbalip.2016.03.004
64. Koeth RA, Wang Z, Levison BS, Buffa JA, Org E, Sheehy BT, et al. Intestinal microbiota metabolism of l-carnitine, a nutrient in red meat, promotes atherosclerosis. Nat Med (2013) 19:576–85. doi: 10.1038/nm.3145
65. Wang Z, Klipfell E, Bennett BJ, Koeth R, Levison BS, Dugar B, et al. Gut flora metabolism of phosphatidylcholine promotes cardiovascular disease. Nature (2011) 472:57–63. doi: 10.1038/nature09922
66. Janeiro MH, Ramírez MJ, Milagro FI, Martínez JA, Solas M. Implication of trimethylamine n-oxide (TMAO) in disease: Potential biomarker or new therapeutic target. Nutrients (2018) 10:1398. doi: 10.3390/nu10101398
67. Tall AR, Yvan-Charvet L. Cholesterol, inflammation and innate immunity. Nat Rev Immunol (2015) 15:104–16. doi: 10.1038/nri3793
68. Kubitz R, Droge C, Stindt J, Weissenberger K, Haussinger D. The bile salt export pump (BSEP) in health and disease. Clin Res Hepatol Gastroenterol (2012) 36:536–53. doi: 10.1016/j.clinre.2012.06.006
69. Canyelles M, Tondo M, Cedó L, Farràs M, Escolà-Gil JC, Blanco-Vaca F. Trimethylamine n-oxide: A link among diet, gut microbiota, gene regulation of liver and intestine cholesterol homeostasis and HDL function. Int J Mol Sci (2018) 19:3228. doi: 10.3390/ijms19103228
70. Ding L, Chang M, Guo Y, Zhang L, Xue C, Yanagita T, et al. Trimethylamine-N-Oxide (TMAO)-induced atherosclerosis is associated with bile acid metabolism. Lipids Health Dis (2018) 17:286. doi: 10.1186/s12944-018-0939-6
71. Tousoulis D, Oikonomou E, Economou EK, Crea F, Kaski JC. Inflammatory cytokines in atherosclerosis: Current therapeutic approaches. Eur Heart J (2016) 37:1723–32. doi: 10.1093/eurheartj/ehv759
72. Zhang X, Gérard P. Diet-gut microbiota interactions on cardiovascular disease. Comput Struct Biotechnol J (2022) 20:1528–40. doi: 10.1016/j.csbj.2022.03.028
73. Liu X, Shao Y, Tu J, Sun J, Li L, Tao J, et al. Trimethylamine-N-Oxide-Stimulated hepatocyte-derived exosomes promote inflammation and endothelial dysfunction through nuclear factor-kappa b signaling. Ann Transl Med (2021) 9:1670. doi: 10.21037/atm-21-5043
74. Chou RH, Chen CY, Chen IC, Huang HL, Lu YW, Kuo CS, et al. Trimethylamine n-oxide, circulating endothelial progenitor cells, and endothelial function in patients with stable angina. Sci Rep (2019) 9:4249. doi: 10.1038/s41598-019-40638-y
75. Zhou S, Xue J, Shan J, Hong Y, Zhu W, Nie Z, et al. Gut-Flora-Dependent metabolite trimethylamine-N-Oxide promotes atherosclerosis-associated inflammation responses by indirect ROS stimulation and signaling involving AMPK and SIRT1. Nutrients (2022) 14:3338. doi: 10.3390/nu14163338
76. Li T, Chen Y, Gua C, Li X. Elevated circulating trimethylamine n-oxide levels contribute to endothelial dysfunction in aged rats through vascular inflammation and oxidative stress. Front Physiol (2017) 8:350. doi: 10.3389/fphys.2017.00350
77. Sun X, Jiao X, Ma Y, Liu Y, Zhang L, He Y, et al. Trimethylamine n-oxide induces inflammation and endothelial dysfunction in human umbilical vein endothelial cells via activating ROS-TXNIP-NLRP3 inflammasome. Biochem Biophys Res Commun (2016) 481:63–70. doi: 10.1016/j.bbrc.2016.11.017
78. Fusco R, Siracusa R, Genovese T, Cuzzocrea S, Di Paola R. Focus on the role of NLRP3 inflammasome in diseases. Int J Mol Sci (2020) 21:4223. doi: 10.3390/ijms21124223
79. Lee J, Lee S, Zhang H, Hill MA, Zhang C, Park Y. Interaction of IL-6 and TNF-alpha contributes to endothelial dysfunction in type 2 diabetic mouse hearts. PloS One (2017) 12:e0187189. doi: 10.1371/journal.pone.0187189
80. Raggi P, Genest J, Giles JT, Rayner KJ, Dwivedi G, Beanlands RS, et al. Role of inflammation in the pathogenesis of atherosclerosis and therapeutic interventions. Atherosclerosis (2018) 276:98–108. doi: 10.1016/j.atherosclerosis.2018.07.014
81. Singh GB, Zhang Y, Boini KM, Koka S. High mobility group box 1 mediates TMAO-induced endothelial dysfunction. Int J Mol Sci (2019) 20:3570. doi: 10.3390/ijms20143570
82. Yang WS, Han NJ, Kim JJ, Lee MJ, Park SK. TNF-α activates high-mobility group box 1 - toll-like receptor 4 signaling pathway in human aortic endothelial cells. Cell Physiol Biochem (2016) 38:2139–51. doi: 10.1159/000445570
83. Miteva K, Madonna R, De Caterina R, Linthout SV. Innate and adaptive immunity in atherosclerosis. Vascul Pharmacol (2018) 107:67–77. doi: 10.1016/j.vph.2018.04.006
84. Ma G, Pan B, Chen Y, Guo C, Zhao M, Zheng L, et al. Trimethylamine n-oxide in atherogenesis: Impairing endothelial self-repair capacity and enhancing monocyte adhesion. Biosci Rep (2017) 37:BSR20160244. doi: 10.1042/BSR20160244
85. Seldin MM, Meng Y, Qi H, Zhu W, Wang Z, Hazen SL, et al. Trimethylamine n-oxide promotes vascular inflammation through signaling of mitogen-activated protein kinase and nuclear factor-κB. J Am Heart Assoc (2016) 5:e002767. doi: 10.1161/JAHA.115.002767
86. Pan B, Ma Y, Ren H, He Y, Wang Y, Lv X, et al. Diabetic HDL is dysfunctional in stimulating endothelial cell migration and proliferation due to down regulation of SR-BI expression. PloS One (2012) 7:e48530. doi: 10.1371/journal.pone.0048530
87. Zhu W, Buffa JA, Wang Z, Warrier M, Schugar R, Shih DM, et al. Flavin monooxygenase 3, the host hepatic enzyme in the metaorganismal trimethylamine n-Oxide-Generating pathway, modulates platelet responsiveness and thrombosis risk. J Thromb Haemost (2018) 16:1857–72. doi: 10.1111/jth.14234
88. Zhu W, Gregory JC, Org E, Buffa JA, Gupta N, Wang Z, et al. Gut microbial metabolite TMAO enhances platelet hyperreactivity and thrombosis risk. Cell (2016) 165:111–24. doi: 10.1016/j.cell.2016.02.011
89. Zhao P, Zhao S, Tian J, Liu X. Significance of gut microbiota and short-chain fatty acids in heart failure. Nutrients (2022) 14:3758. doi: 10.3390/nu14183758
90. Bell DSH, Goncalves E. Heart failure in the patient with diabetes: Epidemiology, aetiology, prognosis, therapy and the effect of glucose-lowering medications. Diabetes Obes Metab (2019) 21:1277–90. doi: 10.1111/dom.13652
91. Anderson KM, Ferranti EP, Alagha EC, Mykityshyn E, French CE, Reilly CM. The heart and gut relationship: A systematic review of the evaluation of the microbiome and trimethylamine-N-Oxide (TMAO) in heart failure. Heart Fail Rev (2022) 27:2223–49. doi: 10.1007/s10741-022-10254-6
92. Trøseid M, Ueland T, Hov JR, Svardal A, Gregersen I, Dahl CP, et al. Microbiota-dependent metabolite trimethylamine-N-Oxide is associated with disease severity and survival of patients with chronic heart failure. J Intern Med (2015) 277:717–26. doi: 10.1111/joim.12328
93. Organ CL, Otsuka H, Bhushan S, Wang Z, Bradley J, Trivedi R, et al. Choline diet and its gut microbe-derived metabolite, trimethylamine n-oxide, exacerbate pressure overload-induced heart failure. Circ Heart Fail (2016) 9:e002314. doi: 10.1161/CIRCHEARTFAILURE.115.002314
94. Li Z, Wu Z, Yan J, Liu H, Liu Q, Deng Y, et al. Gut microbe-derived metabolite trimethylamine n-oxide induces cardiac hypertrophy and fibrosis. Lab Invest (2019) 99:346–57. doi: 10.1038/s41374-018-0091-y
95. Li X, Geng J, Zhao J, Ni Q, Zhao C, Zheng Y, et al. Trimethylamine n-oxide exacerbates cardiac fibrosis via activating the NLRP3 inflammasome. Front Physiol (2019) 10:866. doi: 10.3389/fphys.2019.00866
96. Khalil H, Kanisicak O, Prasad V, Correll RN, Fu X, Schips T, et al. Fibroblast-specific TGF-β-Smad2/3 signaling underlies cardiac fibrosis. J Clin Invest (2017) 127:3770–83. doi: 10.1172/JCI94753
97. Chen K, Zheng X, Feng M, Li D, Zhang H. Gut microbiota-dependent metabolite trimethylamine n-oxide contributes to cardiac dysfunction in Western diet-induced obese mice. Front Physiol (2017) 8:139. doi: 10.3389/fphys.2017.00139
98. Zhang H, Meng J, Yu H. Trimethylamine n-oxide supplementation abolishes the cardioprotective effects of voluntary exercise in mice fed a Western diet. Front Physiol (2017) 8:944. doi: 10.3389/fphys.2017.00944
99. Lu X, Liu J, Zhou B, Wang S, Liu Z, Mei F, et al. Microbial metabolites and heart failure: Friends or enemies? Front Microbiol (2022) 13:956516. doi: 10.3389/fmicb.2022.956516
100. Savi M, Bocchi L, Bresciani L, Falco A, Quaini F, Mena P, et al. Trimethylamine-N-Oxide (TMAO)-induced impairment of cardiomyocyte function and the protective role of urolithin b-glucuronide. Molecules (2018) 23:549. doi: 10.3390/molecules23030549
101. Makrecka-Kuka M, Volska K, Antone U, Vilskersts R, Grinberga S, Bandere D, et al. Trimethylamine n-oxide impairs pyruvate and fatty acid oxidation in cardiac mitochondria. Toxicol Lett (2017) 267:32–8. doi: 10.1016/j.toxlet.2016.12.017
102. Jung T, Bader N, Grune T. Lipofuscin: Formation, distribution, and metabolic consequences. Ann N Y Acad Sci (2007) 1119:97–111. doi: 10.1196/annals.1404.008
103. Witkowski M, Witkowski M, Friebel J, Buffa JA, Li XS, Wang Z, et al. Vascular endothelial tissue factor contributes to trimethylamine n-Oxide-Enhanced arterial thrombosis. Cardiovasc Res (2022) 118:2367–84. doi: 10.1093/cvr/cvab263
104. Roll-Mecak A. The tubulin code in microtubule dynamics and information encoding. Dev Cell (2020) 54:7–20. doi: 10.1016/j.devcel.2020.06.008
105. Manolis AA, Manolis TA, Melita H, Manolis AS. Gut microbiota and cardiovascular disease: Symbiosis versus dysbiosis. Curr Med Chem (2022) 29:4050–77. doi: 10.2174/0929867328666211213112949
106. Jin B, Ji F, Zuo A, Liu H, Qi L, He Y, et al. Destructive role of TMAO in T-tubule and excitation-contraction coupling in the adult cardiomyocytes. Int Heart J (2020) 61:355–63. doi: 10.1536/ihj.19-372
107. Wang Y, Chen B, Huang CK, Guo A, Wu J, Zhang X, et al. Targeting calpain for heart failure therapy: Implications from multiple murine models. JACC Basic Transl Sci (2018) 3:503–17. doi: 10.1016/j.jacbts.2018.05.004
108. Frisk M, Koivumäki JT, Norseng PA, Maleckar MM, Sejersted OM, Louch WE. Variable T-tubule organization and Ca2+ homeostasis across the atria. Am J Physiol Heart Circ Physiol (2014) 307:H609–20. doi: 10.1152/ajpheart.00295.2014
109. Suzuki T, Heaney LM, Bhandari SS, Jones DJL, Ng LL. Trimethylamine n-oxide and prognosis in acute heart failure. Heart (2016) 102:841–8. doi: 10.1136/heartjnl-2015-308826
110. Tang WHW, Wang Z, Kennedy DJ, Wu Y, Buffa JA, Agatisa-Boyle B, et al. Gut microbiota-dependent trimethylamine n-oxide (TMAO) pathway contributes to both development of renal insufficiency and mortality risk in chronic kidney disease. Circ Res (2015) 116:448–55. doi: 10.1161/CIRCRESAHA.116.305360
111. Salzano A, Cassambai S, Yazaki Y, Israr MZ, Bernieh D, Wong M, et al. The gut axis involvement in heart failure: Focus on trimethylamine n-oxide. Heart Fail Clin (2020) 16:23–31. doi: 10.1016/j.hfc.2019.08.001
112. Zhang WQ, Wang YJ, Zhang A, Ding YJ, Zhang XN, Jia QJ, et al. TMA/TMAO in hypertension: Novel horizons and potential therapies. J Cardiovasc Transl Res (2021) 14:1117–24. doi: 10.1007/s12265-021-10115-x
113. Verhaar BJH, Prodan A, Nieuwdorp M, Muller M. Gut microbiota in hypertension and atherosclerosis: A review. Nutrients (2020) 12:2982. doi: 10.3390/nu12102982
114. Estruch R, Ros E, Salas-Salvadó J, Covas MI, Corella D, Arós F, et al. Primary prevention of cardiovascular disease with a Mediterranean diet supplemented with extra-virgin olive oil or nuts. N Eng J Med (2018) 378:e34. doi: 10.1056/NEJMoa1800389
115. Luo Q, Hu Y, Chen X, Luo Y, Chen J, Wang H. Effects of gut microbiota and metabolites on heart failure and its risk factors: A two-sample mendelian randomization study. Front Nutr (2022) 9:899746. doi: 10.3389/fnut.2022.899746
116. Yang T, Santisteban MM, Rodriguez V, Li E, Ahmari N, Carvajal JM, et al. Gut dysbiosis is linked to hypertension. Hypertension (2015) 65:1331–40. doi: 10.1161/HYPERTENSIONAHA.115.05315
117. Ge X, Zheng L, Zhuang R, Yu P, Xu Z, Liu G, et al. The gut microbial metabolite trimethylamine n-oxide and hypertension risk: A systematic review and dose-response meta-analysis. Adv Nutr (2020) 11:66–76. doi: 10.1093/advances/nmz064
118. Shanks J, Ramchandra R. Angiotensin II and the cardiac parasympathetic nervous system in hypertension. Int J Mol Sci (2021) 22:12305. doi: 10.3390/ijms222212305
119. Ufnal M, Jazwiec R, Dadlez M, Drapala A, Sikora M, Skrzypecki J. Trimethylamine-N-Oxide: A carnitine-derived metabolite that prolongs the hypertensive effect of angiotensin II in rats. Can J Cardiol (2014) 30:1700–5. doi: 10.1016/j.cjca.2014.09.010
120. Te RL, van Esch JH, Roks AJ, van den Meiracker AH, Danser AH. Hypertension: Renin-Angiotensin-Aldosterone system alterations. Circ Res (2015) 116:960–75. doi: 10.1161/CIRCRESAHA.116.303587
121. Liu M, Han Q, Yang J. Trimethylamine-N-Oxide (TMAO) increased aquaporin-2 expression in spontaneously hypertensive rats. Clin Exp Hypertens (2019) 41:312–22. doi: 10.1080/10641963.2018.1481420
122. Ishikawa SE. Hyponatremia associated with heart failure: Pathological role of vasopressin-dependent impaired water excretion. J Clin Med (2015) 4:933–47. doi: 10.3390/jcm4050933
123. Ando F. Activation of AQP2 water channels by protein kinase a: Therapeutic strategies for congenital nephrogenic diabetes insipidus. Clin Exp Nephrol (2021) 25:1051–6. doi: 10.1007/s10157-021-02108-6
124. Jaworska K, Huc T, Samborowska E, Dobrowolski L, Bielinska K, Gawlak M, et al. Hypertension in rats is associated with an increased permeability of the colon to TMA, a gut bacteria metabolite. PloS One (2017) 12:e0189310. doi: 10.1371/journal.pone.0189310
125. Meng G, Zhou X, Wang M, Zhou L, Wang Z, Wang M, et al. Gut microbe-derived metabolite trimethylamine n-oxide activates the cardiac autonomic nervous system and facilitates ischemia-induced ventricular arrhythmia via two different pathways. EBioMedicine (2019) 44:656–64. doi: 10.1016/j.ebiom.2019.03.066
126. Svingen GFT, Zuo H, Ueland PM, Seifert R, Løland KH, Pedersen ER, et al. Increased plasma trimethylamine-N-Oxide is associated with incident atrial fibrillation. Int J Cardiol (2018) 267:100–6. doi: 10.1016/j.ijcard.2018.04.128
127. Gong D, Zhang L, Zhang Y, Wang F, Zhao Z, Zhou X. Gut microbial metabolite trimethylamine n-oxide (TMAO) is related to thrombus formation in atrial fibrillation patients. Am J Med Sci (2019) 358:422–8. doi: 10.1016/j.amjms.2019.09.002
128. Papadopoulos PD, Tsigalou C, Valsamaki PN, Konstantinidis TG, Voidarou C, Bezirtzoglou E. The emerging role of the gut microbiome in cardiovascular disease: Current knowledge and perspectives. Biomedicines (2022) 10:948. doi: 10.3390/biomedicines10050948
129. Li XS, Obeid S, Klingenberg R, Gencer B, Mach F, Räber L, et al. Gut microbiota-dependent trimethylamine n-oxide in acute coronary syndromes: A prognostic marker for incident cardiovascular events beyond traditional risk factors. Eur Heart J (2017) 38:814–24. doi: 10.1093/eurheartj/ehw582
130. Dong Z, Zheng S, Shen Z, Luo Y, Hai X. Trimethylamine n-oxide is associated with heart failure risk in patients with preserved ejection fraction. Lab Med (2021) 52:346–51. doi: 10.1093/labmed/lmaa075
131. Almesned MA, Prins FM, Lipšic E, Connelly MA, Garcia E, Dullaart RPF, et al. Temporal course of plasma trimethylamine n-oxide (TMAO) levels in ST-elevation myocardial infarction. J Clin Med (2021) 10:5677. doi: 10.3390/jcm10235677
132. Guo F, Zhou J, Li Z, Yu Z, Ouyang D. The association between trimethylamine n-oxide and its predecessors choline, l-carnitine, and betaine with coronary artery disease and artery stenosis. Cardiol Res Pract (2020) 2020:5854919. doi: 10.1155/2020/5854919
133. Xu KY, Xia GH, Lu JQ, Chen MX, Zhen X, Wang S, et al. Impaired renal function and dysbiosis of gut microbiota contribute to increased trimethylamine-N-Oxide in chronic kidney disease patients. Sci Rep (2017) 7:1445. doi: 10.1038/s41598-017-01387-y
134. Kovacic JC, Castellano JM, Farkouh ME, Fuster V. The relationships between cardiovascular disease and diabetes: Focus on pathogenesis. Endocrinol Metab Clin North Am (2014) 43:41–57. doi: 10.1016/j.ecl.2013.09.007
135. Li P, Zhong C, Li S, Sun T, Huang H, Chen X, et al. Plasma concentration of trimethylamine-N-Oxide and risk of gestational diabetes mellitus. Am J Clin Nutr (2018) 108:603–10. doi: 10.1093/ajcn/nqy116
136. Coutinho-Wolino KS, de F Cardozo LFM, de Oliveira Leal V, Mafra D, Stockler-Pinto MB. Can diet modulate trimethylamine n-oxide (TMAO) production? What Do we Know so Far? Eur J Nutr (2021) 60:3567–84. doi: 10.1007/s00394-021-02491-6
137. Winther SA, Øllgaard JC, Tofte N, Tarnow L, Wang Z, Ahluwalia TS, et al. Utility of plasma concentration of trimethylamine n-oxide in predicting cardiovascular and renal complications in individuals with type 1 diabetes. Diabetes Care (2019) 42:1512–20. doi: 10.2337/dc19-0048
138. Winther SA, Øllgaard JC, Hansen TW, von Scholten BJ, Reinhard H, Ahluwalia TS, et al. Plasma trimethylamine n-oxide and its metabolic precursors and risk of mortality, cardiovascular and renal disease in individuals with type 2-diabetes and albuminuria. PloS One (2021) 16:e0244402. doi: 10.1371/journal.pone.0244402
139. Croyal M, Saulnier PJ, Aguesse A, Gand E, Ragot S, Roussel R, et al. Plasma trimethylamine n-oxide and risk of cardiovascular events in patients with type 2 diabetes. J Clin Endocrinol Metab (2020) 105:dgaa188. doi: 10.1210/clinem/dgaa188
140. Oellgaard J, Winther SA, Hansen TS, Rossing P, von Scholten BJ. Trimethylamine n-oxide (TMAO) as a new potential therapeutic target for insulin resistance and cancer. Curr Pharm Des (2017) 23:3699–712. doi: 10.2174/1381612823666170622095324
141. Cho CE, Caudill MA. Trimethylamine-N-Oxide: Friend, foe, or simply caught in the cross-fire? Trends Endocrinol Metab (2017) 28:121–30. doi: 10.1016/j.tem.2016.10.005
142. Din AU, Hassan A, Zhu Y, Yin T, Gregersen H, Wang G. Amelioration of TMAO through probiotics and its potential role in atherosclerosis. Appl Microbiol Biotechnol (2019) 103:9217–28. doi: 10.1007/s00253-019-10142-4
143. Wang H, Luo Q, Ding X, Chen L, Zhang Z. Trimethylamine n-oxide and its precursors in relation to blood pressure: A mendelian randomization study. Front Cardiovasc Med (2022) 9:922441. doi: 10.3389/fcvm.2022.922441
144. Winkel P, Hilden J, Hansen JF, Kastrup J, Kolmos HJ, Kjøller E, et al. Clarithromycin for stable coronary heart disease increases all-cause and cardiovascular mortality and cerebrovascular morbidity over 10Years in the CLARICOR randomised, blinded clinical trial. Int J Cardiol (2015) 182:459–65. doi: 10.1016/j.ijcard.2015.01.020
145. Heianza Y, Zheng Y, Ma W, Rimm EB, Albert CM, Hu FB, et al. Duration and life-stage of antibiotic use and risk of cardiovascular events in women. Eur Heart J (2019) 40:3838–45. doi: 10.1093/eurheartj/ehz231
146. Wang Z, Roberts AB, Buffa JA, Levison BS, Zhu W, Org E, et al. Non-lethal inhibition of gut microbial trimethylamine production for the treatment of atherosclerosis. Cell (2015) 163:1585–95. doi: 10.1016/j.cell.2015.11.055
147. Simó C, García-Cañas V. Dietary bioactive ingredients to modulate the gut microbiota-derived metabolite TMAO. new opportunities for functional food development. Food Funct (2020) 11:6745–76. doi: 10.1039/d0fo01237h
148. Qiu L, Yang D, Tao X, Yu J, Xiong H, Wei H. Enterobacter aerogenes ZDY01 attenuates choline-induced trimethylamine n-oxide levels by remodeling gut microbiota in mice. J Microbiol Biotechnol (2017) 27:1491–9. doi: 10.4014/jmb.1703.03039
149. Gan XT, Ettinger G, Huang CX, Burton JP, Haist JV, Rajapurohitam V, et al. Probiotic administration attenuates myocardial hypertrophy and heart failure after myocardial infarction in the rat. Circ Heart Fail (2014) 7:491–9. doi: 10.1161/CIRCHEARTFAILURE.113.000978
150. Costanza AC, Moscavitch SD, Faria Neto HCC, Mesquita ET. Probiotic therapy with saccharomyces boulardii for heart failure patients: A randomized, double-blind, placebo-controlled pilot trial. Int J Cardiol (2015) 179:348–50. doi: 10.1016/j.ijcard.2014.11.034
151. Quin C, Estaki M, Vollman DM, Barnett JA, Gill SK, Gibson DL. Probiotic supplementation and associated infant gut microbiome and health: A cautionary retrospective clinical comparison. Sci Rep (2018) 8:8283. doi: 10.1038/s41598-018-26423-3
152. Swanson KS, Gibson GR, Hutkins R, Reimer RA, Reid G, Verbeke K, et al. The international scientific association for probiotics and prebiotics (ISAPP) consensus statement on the definition and scope of synbiotics. Nat Rev Gastroenterol Hepatol (2020) 17:687–701. doi: 10.1038/s41575-020-0344-2
153. da Silva TF, Casarotti SN, de Oliveira GLV, Penna ALB. The impact of probiotics, prebiotics, and synbiotics on the biochemical, clinical, and immunological markers, as well as on the gut microbiota of obese hosts. Crit Rev Food Sci Nutr (2021) 61:337–55. doi: 10.1080/10408398.2020.1733483
154. Chen ML, Yi L, Zhang Y, Zhou X, Ran L, Yang JD, et al. Resveratrol attenuates trimethylamine-N-Oxide (TMAO)-induced atherosclerosis by regulating TMAO synthesis and bile acid metabolism via remodeling of the gut microbiota. MBio (2016) 7:e02210–15. doi: 10.1128/mBio.02210-15
155. Du D, Tang W, Zhou C, Sun X, Wei Z, Zhong J, et al. Fecal microbiota transplantation is a promising method to restore gut microbiota dysbiosis and relieve neurological deficits after traumatic brain injury. Oxid Med Cell Longev (2021) 2021:5816837. doi: 10.1155/2021/5816837
156. Vrieze A, Van Nood E, Holleman F, Salojärvi J, Kootte RS, Bartelsman JFWM, et al. Transfer of intestinal microbiota from lean donors increases insulin sensitivity in individuals with metabolic syndrome. Gastroenterology (2012) 143:913–6. doi: 10.1053/j.gastro.2012.06.031
Keywords: gut microbiota, metabolite, cardiovascular disease, trimethylamine N-oxide, targeted therapy
Citation: Zhen J, Zhou Z, He M, Han H-X, Lv E-H, Wen P-B, Liu X, Wang Y-T, Cai X-C, Tian J-Q, Zhang M-Y, Xiao L and Kang X-X (2023) The gut microbial metabolite trimethylamine N-oxide and cardiovascular diseases. Front. Endocrinol. 14:1085041. doi: 10.3389/fendo.2023.1085041
Received: 31 October 2022; Accepted: 26 January 2023;
Published: 07 February 2023.
Edited by:
Tarunveer Singh Ahluwalia, Steno Diabetes Center Copenhagen (SDCC), DenmarkReviewed by:
Piotr Konopelski, Medical University of Warsaw, PolandCopyright © 2023 Zhen, Zhou, He, Han, Lv, Wen, Liu, Wang, Cai, Tian, Zhang, Xiao and Kang. This is an open-access article distributed under the terms of the Creative Commons Attribution License (CC BY). The use, distribution or reproduction in other forums is permitted, provided the original author(s) and the copyright owner(s) are credited and that the original publication in this journal is cited, in accordance with accepted academic practice. No use, distribution or reproduction is permitted which does not comply with these terms.
*Correspondence: Xing-Xing Kang, eHhrYW5nQHh6aG11LmVkdS5jbg==; Lei Xiao, bHhpYW9AY3VtdC5lZHUuY24=
† These authors have contributed equally to this work
Disclaimer: All claims expressed in this article are solely those of the authors and do not necessarily represent those of their affiliated organizations, or those of the publisher, the editors and the reviewers. Any product that may be evaluated in this article or claim that may be made by its manufacturer is not guaranteed or endorsed by the publisher.
Research integrity at Frontiers
Learn more about the work of our research integrity team to safeguard the quality of each article we publish.