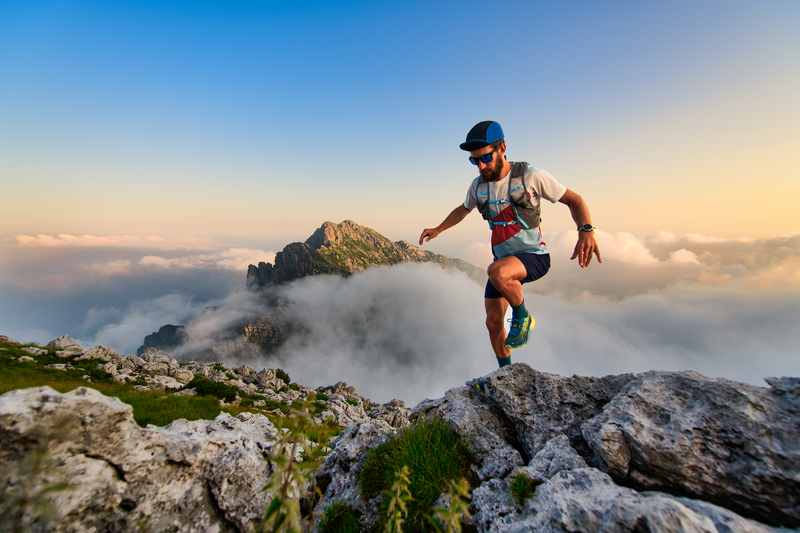
95% of researchers rate our articles as excellent or good
Learn more about the work of our research integrity team to safeguard the quality of each article we publish.
Find out more
REVIEW article
Front. Endocrinol. , 21 February 2023
Sec. Developmental Endocrinology
Volume 14 - 2023 | https://doi.org/10.3389/fendo.2023.1059854
This article is part of the Research Topic A year in review: Discussions in Developmental Endocrinology View all 5 articles
Endocrine-disrupting chemicals (EDCs) or endocrine disruptors are substances that are either naturally occurring or artificial and are released into the natural environment. Humans are exposed to EDCs through ingestion, inhalation, and skin contact. Many everyday household items, such as plastic bottles and containers, the liners of metal food cans, detergents, flame retardants, food, gadgets, cosmetics, and pesticides, contain endocrine disruptors. Each hormone has a unique chemical makeup and structural attributes. The way that endocrine hormones connect to receptors is described as a “lock and key” mechanism, with each hormone serving as the key (lock). This mechanism is enabled by the complementary shape of receptors to their hormone, which allows the hormone to activate the receptors. EDCs are described as exogenous chemicals or compounds that have a negative impact on organisms’ health by interacting with the functioning of the endocrine system. EDCs are associated with cancer, cardiovascular risk, behavioural disorders, autoimmune abnormalities, and reproductive disorders. EDCs exposure in humans is highly harmful during critical life stages. Nonetheless, the effect of EDCs on the placenta is often underestimated. The placenta is especially sensitive to EDCs due to its abundance of hormone receptors. In this review, we evaluated the most recent data on the effects of EDCs on placental development and function, including heavy metals, plasticizers, pesticides, flame retardants, UV filters and preservatives. The EDCs under evaluation have evidence from human biomonitoring and are found in nature. Additionally, this study indicates important knowledge gaps that will direct future research on the topic.
Endocrine-disrupting chemicals (EDCs) are exogenous compounds that can interact with the endocrine system in humans, altering critical biological processes such as immunity, metabolism, organogenesis, reproduction, and behavior. Several synthetic and natural chemicals can disrupt the endocrine system’s function and negatively impact the body. These compounds are known as EDCs (1). Natural or artificial substances can act as hormone disruptors. Other than these pesticides, heavy metals, and retardants, everyday products like plasticizers (food containers, flame retardants, plastic bottles, cosmetics, detergents, and toys) expose people to EDCs. Some EDCs, such as polychlorinated biphenyls (PCBs), dichlorodiphenyldichloroethylene (DDE), dioxin, organochlorine pesticides, and hexachlorobenzene (HCB), were designed to have long half-lives for industrial purposes and are known as “persistent organic pollutants” or POPs. These EDCs impede normal growth and development by interfering with genetic and epigenetic regulatory processes (2, 3).
The Environmental Protection Agency (EPA, USA) identified more than 86,000 substances as endocrine disruptors through the Toxic Substances Control Act. The effect of exposures increases during pregnancy since they may have an impact on the developing fetus and result in chronic postnatal diseases. In general, pregnant women experience rapid hormonal changes. If hormone production and secretion are interrupted, routine pregnancy maintenance and fetus nourishment become difficult (4). EDCs exposure increases a fetus’s lifetime risk of developing cognitive, behavioural, and reproductive abnormalities. According to reports, prenatal exposure to EDCs can cause disorders like cancer, autism, diabetes, infertility, and attention deficit hyperactivity disorder (5).
Immature fetuses are vulnerable to EDCs exposure because different EDCs are passed from the mother to the fetus through the placenta. Concerns regarding the contradictory effects of EDCs passed through the placenta during fetal growth have raised interest in the proper use of personal care products during placental development. Despite this, the placenta is affected by EDCs, preventing it from carrying out its normal activities. The direct effects of EDCs on the fetus are less well understood than their impact on placenta growth and function (6).
The placenta is a vital organ that performs a variety of endocrine, immunological, and physiological processes throughout pregnancy. The placenta gradually grows during the first three months of pregnancy, but starting in the fourth month, it develops simultaneously with the uterus. It is a transient organ that shares genetic characteristics with a developing infant (7). The placenta of the human body is a hemomonochorial type. It develops from a blastocyst early in gestation (trophectoderm layer). The trophoblast, which originates from the blastocyst’s outer layer, interacts with the decidual epithelium. Cytotrophoblasts (CTBs), syncytiotrophoblasts (STBs), and extravillous trophoblasts (EVTs) are the 3 primary trophoblast lineages. Recent research suggests that in the first trimester of pregnancy, EVTs invade the entire uterine vasculature, including arteries, veins, and lymphatics (8). The blastocyst’s trophectoderm cells differentiate to develop into the CTBs during placentation (first trophoblast lineage). The CTBs continue to multiply and differentiate into the STB layer and the EVTs. The uterine spiral arteries that provide oxygenated blood to the developing placenta and fetus are found in the placental bed. Throughout pregnancy, the placental bed undergoes significant modifications. Extravillous trophoblast (EVT) cells multiply from anchoring chorionic villi and invade the decidualized endometrium by two different pathways: (1) endovascular EVT cells invade the lumen of the spiral arteries, and (2) interstitial EVT cells invade the decidualized endometrium and inner myometrium. Some EVT are incorporated as intramural trophoblast into the spiral artery wall (9).
The lacunae or spaces are formed inside the syncytioblast, which are filled with maternal blood as it comes into contact with blood vessels. Lacunar networks are formed when these lacunae join together. The maternal blood that passes into and out of these networks exchanges nutrients and waste materials with the fetus, forming the foundation of uteroplacental circulation (10). The primary chorionic villi (PCV), which pierce and spread into the surrounding syncytiotrophoblast layer, originate from the cytotrophoblast layer. These structures are secondary chorionic villi (SCV) when the extraembryonic mesoderm layer forms a central mass of loose connective tissue within this PCV post-implantation. The SCV’s embryonic vasculature integrates with the embryonic mesoderm, forming tertiary chorionic villi (TCV). The syncytiotrophoblast secretes many proteins, including progesterone, human chorionic gonadotropin (HCG), and other substances (11).
To produce a cytotrophoblastic shell, the cytotrophoblast cells from the TCV advance toward the decidua basalis of the maternal uterus. Anchoring villi are the villi that attach to the decidua basalis via the cytotrophoblastic shell. Branching villi provide permeability for the interchange of metabolites between the fetal and maternal in the intervillous space by extending outward from the stem (anchoring) villi. Visualizing the villi as projections resembling trees can enable us to understand their anatomy (9). The mother’s spiral arteries undergo modification to develop low resistance and high blood flow conditions to fulfil the fetus’s needs. The mother’s spiral arteries are invaded by cytotrophoblast cells, which act as the endothelium’s replacement. They transform from epithelium to endothelium, expanding the diameter and decreasing the resistance of the blood vessels that supply the uterus with nutrient-rich blood (12). See Figure 1.
Figure 1 Stages (pre-and post-placentation) of placenta development (12).
The placenta communicates with its surroundings. The placenta must develop for a pregnancy to be successful. This delicate organ comprises multiple layers of tissue that must develop normally during pregnancy to function correctly, which could have devastating consequences for the fetus (13). Different EDCs may indirectly influence the fetus through the placenta. Protein and hormone levels will fluctuate and balance as cells develop and differentiate. The hormonal environment will no longer be in equilibrium once EDCs have crossed the placenta and reached the fetus. Due to the immature fetus’ vulnerability to EDCs, preeclampsia (PE), fetal development retardation, miscarriage, and placental disruption might occur (2). Several genetic and epigenetic factors regulate the trophoblast layer’s proliferation, apoptosis, and invasiveness. Their imbalance hinders the early vascular system from generally developing in the first trimester of pregnancy, resulting in intrauterine growth retardation (14). The placenta is particularly susceptible to endocrine disruption because of the large density of steroid hormone receptor expression in this organ. Over the last five years, research on how EDCs impair placental development has grown significantly (15).
There are numerous constraints on how the EDC can be studied regarding placenta formation and development. As a result, this article examines how EDCs affect placental growth during the first trimester of pregnancy. We first discussed the several EDCs detected in the human placenta before revising how often these EDCs are exposed and how it affects the placenta and fetal maturation. We then discussed the mechanisms of action of dichlorodiphenyltrichloroethane (DDT), cadmium, and bisphenol-A (BPA), which are early-stage environmental EDCs that change placental characteristics.
Several EDCs have been studied because they can cross the placenta, accumulate in placental tissues, and persist in fetal circulatory systems and organs. Only a small percentage of the thousands of synthetic chemicals found so far have the potential to disrupt the endocrine system. Insecticides like DDT and dieldrin, flame retardants like polybrominated diphenyl ethers, fungicides, pharmaceuticals, and additives for consumer products (benzophenone, parabens, and synthetic musks like galactoside) are just a few examples of the numerous different types of EDCs (16). DDT exposure reduces placenta cell viability in vitro at doses more than 25 μM (HTR-8/SVneo), while smaller concentrations did not affect proliferation (1 nM). DDT (doses: 1, 10, or 100 ng/ml) enhanced the expression of prostaglandin E2 (PGE2) synthase, 3β-HSD, and CYP11A1 in bovine placental explants (17). Studies have shown that various EDCs are present in pregnant women’s urine, amniotic fluid, breast milk, and serum. EDCs can enter pregnant women through several pathways, including the respiratory system, packaging, the food chain, and the skin. Pregnant women occasionally breathe the dust from spaces with wallpaper or flooring, touch objects, or consume food or drink tainted with EDCs (18).
While the source of p,p′-DDE exposure is dependent on whether p,p′-DDT exposure is active, direct contact with pesticide application and nutrition are the main ways that humans are exposed to p,p′-DDT and o,p′-DDT. Humans convert p,p′-DDT to p,p′-DDE when exposed actively, but p,p′-DDE exposure is primarily acquired from the diet. Because p,p′-DDE has a longer half-life in the environment than its counterparts, it is more detectable and has higher levels in people, which leads to bio-accumulation in the food chain. Blood levels of p,p′-DDT, p,p′-DDE, and o,p′-DDT have dropped about five times since DDT was restricted in the early 1970s (19).
The placenta and fetus typically lack the enzymatic machinery to guard against these exposures. EDCs can disturb the endocrine system by competing with endogenous steroid hormones, binding to receptors and hormone transport proteins, or altering the breakdown or generation of endogenous hormones (20). Even though the mechanism of the protein responsible for regulating the transport of drugs in the placenta is only partially understood, more studies are needed to fully comprehend how EDCs move through the placenta and whether they can change gene expression. Over the past few years, numerous clinical investigations have been carried out to assess the level of EDCs in blood or amniotic fluid (21).
EDCs, such as the metabolites of phthalates, are linked to the polymer molecules in plastic products and can gradually leak into water or food packaged in plastic. Some of these might build up in placental tissues and affect how well the placenta functions. Urine samples were tested for triclocarban (TCC) and triclosan (TCS), substances found in a wide range of consumer goods, including toothpaste, plastics, textiles, medical equipment, and soaps. TCS was found in all study participants (22). Metabolites of parabens have long been employed as preservatives in numerous everyday items. Recent studies have shown that it functions as EDCs andis present in up to 100% (methylparaben=0.26 ng/mL, ethylparaben=0.40 ng/mL; isopropylparaben=0.18 ng/mL; n-propylparaben=0.18 ng/mL; iso-butylparaben=0.07 ng/mL; n-butylparaben=0.07 ng/mL; and benzylparaben=0.18 ng/mL, respectively) of the mother urine samples analyzed (23).
According to another research, organochlorine pesticides have also been related to pre-term birth, fetal cryptorchidism, and prenatal development retardation (24). Exposure to polychlorinated biphenyls and polybrominated diphenyl ethers during the prenatal period inhibits the development of the neurological system and is linked to fetal thyroid malfunction. The placental barrier was also breached by polycyclic aromatic hydrocarbons (PAH), phenols, perchlorate, and perfluorinated chemicals found in 98 to 100% of samples from expecting mothers (25). Since BPA exhibits estrogenic action, it has the potential to disrupt endocrine pathways. It has been established that the presence of BPA (1 nM) in trophoblast cells affects DNA methylation during the first trimester of pregnancy and inhibits cellular growth. Studies showed that BPA enters the placenta and increases the level of β-HCG, which affects placental development (26).
BPA has been found in maternal and fetal serum and the human placenta and can cross the placental barrier. As a result, BPA can enter the fluids and tissues of the human womb. BPA can also be absorbed by contact or inhalation. For instance, the receipts’ thermal paper may produce this substance when it comes into contact with the epidermis (27, 28). Recent research on metabolism and toxicokinetics has revealed that BPA is rapidly absorbed orally. Once ingested, this substance is coupled with glucuronic acid in the liver. BPA glucuronate is a reliable exposure biomarker and is adequately stable (29). Although some controversial data suggests that BPA is not hazardous to human health, several recent studies have highlighted its negative consequences. BPA can accumulate in various human and animal tissues due to its lipophilic nature (logP of 3.4), impairing their physiological processes and negatively impacting health (30, 31).
IGF-2 gene methylation alterations are associated with exposure to various persistent organic pollutants (POPs), including DDTs. IGF-2 is a crucial regulator of fetal and placental development (32). The amounts of heavy metals in placental tissues have been investigated using various techniques. These heavy metals include mercury (Hg = 550 µg/m3), lead (Pb = 500 mg/kg/day), and cadmium (Cd = 0.02 µg/g), all of which have been linked to brain disorders and abnormalities in vertebrates. Even though various hormone disruptors have been identified, few human endocrine routes have been thoroughly investigated, and little is known about the impacts of epigenetics. There are many unexplored pathways and components in normal physiology. New research and new research approaches are required for this field to increase knowledge of future human diseases (33). The estrogenic signaling pathways are disrupted by a class of substances known as xenoestrogens (PCBs, BPA, and phthalates). Initial xenoestrogen exposure (~3.24 ng/day) may also prevent trophoblast penetration of the endometrium, increasing the risk of intrauterine growth restriction (IUGR), in-utero neonatal mortality, and epigenetic abnormalities in the placenta for the infant (34) (Figure 2).
Figure 2 Various groups of endocrine-disrupting chemicals (EDCs) (34).
Although heavy metal pollutants’ effects on the fetus’s development have not been examined, this study can provide insight into how metal intakes result in these effects. Cd, Pb, Hg, and As have all been identified as worldwide pollutants by the Agency for Toxic Substances and Disease Registry (ATSDR, Atlanta, GA, USA) (35). These substances are toxic to human development and cross the placenta. These metals are exposed more frequently because they are used in many residential, agricultural, technical, and medical applications (36).
Cd is one of our environment’s most prevalent and dangerous heavy metals. Decades after its toxicity was discovered, research on its toxicity is still a popular topic in systematic works. The central nervous system, liver and kidney in the placenta and fetus as teratogenicity, as well as the in-vivo studies conducted on experimental models to assess the maternal cadmium-induced toxicity (alteration in essential element homeostasis, preeclampsia, kidney injury, bone mineralization, and fertility) were summarized (37).
Several studies have reported that Cd exposure during placentation reduces the levels of membrane proteins, decreasing their transport function and increasing intracellular Cd accumulation in the placenta of Cd-treated (5 mg/kg) rats. The histopathological studies showed that exposure to Cd causes swelling in trophoblast cells and vacuolar degeneration and prevents the migration of human trophoblast cells to the placenta. Long-time exposure induces apoptosis in syncytiotrophoblast cells in-vivo rate model (38). Given that each cigarette contains 1-2 µg of Cd, numerous analyses have demonstrated a link between Cd ingested through tobacco smoke and pregnancy problems. Its precise mode of action has also been discovered (39). Furthermore, during placenta development, Cd causes the discharge of volatile substances such as cytokines from the trophoblast cells of humans and the mouse placenta (40).
Moreover, Cd exposure during gestation in mother tissues such as maternal blood, mammary glands, and the placenta induces the production of metallothionein (MTs) in the syncytiotrophoblast and amniotic cells. The Cd-MT complexes will avoid the Cd transport from the placenta to the fetus. High MT (8-9 μg/g) will increase the formation of zinc-MT complexes. Cd may reduce the efficiency of Zn transport, such as ZnT2, ZIP14, and DMT1, and prevent the conveyance of Cd and Zn to the fetus. Even though the exact mechanism by which Cd moves through the placenta and into the fetus is still undetermined, research on rats conducted by Nakamura et al. suggests that DMT1 is the protein involved in the transport of Cd from the placenta to the fetus. On the other hand, it would appear that placental MTs would help prevent Cd transfer; however, the exact process is still unclear at this moment. According to Nakamura et al., the expression of MT genes and the level of Cd in the placental regulates the induction of metal transporter. This suggests that the mechanism of Cd mobilization and transport in the placenta depends on various metal proteins, including the MTs (41).
Hg exposure is primarily caused by eating contaminated fish and seafood, which mainly builds up in the fetal CNS (central nervous system). Ask et al. analyzed the levels of inorganic mercury (I-Hg) and methylmercury associated with selenium in the placentas of pregnant women. Increasing concentration determined their accumulation in the placenta (42). Gilman et al. discovered that women who did not consume seafood had a higher proportion of Hg in the placenta to cord blood than those who consumed. Furthermore, the placenta contains more selenium than cord blood in this population (43). However, studies have shown that Mercuric Hg does not readily pass the placental barrier but accumulates in the placenta and amnion. Further studies need to evaluate the mechanism of impact of disruption in placentation (44).
Common causes of exposure to Pb include Pb-based paint and glazed food containers. Pb also causes the fetus to undergo apoptosis and interferes with the ability of organelles like the endoplasmic reticulum and mitochondria to operate appropriately. According to publications on how metals can affect trophoblast features, prenatal exposure to Cd (0.44–2.68 ng/g) or Pb (17.17–46.86 ng/g) can cause many illnesses, including obesity, by genetic and epigenetic regulation (45). There have been numerous studies on the cytotoxic effects of Pb on fetuses in development, but little is known about the mechanisms of action at low concentrations.
Heavy metals have been shown to interfere with protein kinase C (PKC) and Ca2+ binding proteins, disrupt Ca2+ channels and pumps, and impact calcium (Ca2+) homeostasis (CaBP). Despite the modest maternal and cord blood Pb levels, there was a strong correlation between maternal blood Pb concentration (13.35 ± 0.73 nmol) and a reduction in syncytiotrophoblast Ca2+ uptake. Arsenic (As) exposure generally happens when people consume contaminated water and make pesticides. On the other hand, Zinc (Zn) is a trace element that naturally occurs in the earth, air, and food (46).
Chemicals created by humans called plasticizers are primarily employed to increase polymers’ elasticity, flexibility, color, resistance, and/or durability. The most extensively used plasticizers include phthalates, alkylphenols, and BPA. di-(2-ethylhexyl) phthalate (DEHP), butyl benzyl phthalate (BBP), dibutyl phthalate (DBP), and dimethyl phthalate (DMP) are a few of the most often used phthalates (47). Phthalates were categorized as EDCs in 2002 because they interfere with the secretion, binding, transport, synthesis, and/or removal of endogenous hormones from the body, disrupting hormonal systems. Because fetal and embryonic development is especially vulnerable, plasticizers have been shown to cross the human placenta and reach the fetus (48).
DEHP (2 mmol/L) exposure decreases cell proliferation. It reduces placenta size in mothers, which is associated with fetal growth limitation and adult disorders, according to the in-vivo and in-vitro investigation. DEHP inhibits placental cell proliferation, but the exact mechanism by which it does so is still unclear (49). Furthermore, several studies have indicated that DEHP may negatively affect fetal cellular mechanisms such as oxidative stress response, invasion, immunomodulation, endocrine function, and trophoblast differentiation, which may lead to pathologies and unfavourable pregnancy outcomes (50). Around 98–100% of pregnant women have had phthalate metabolites, including DEHP (1.7 μg/L) and MEP (monoethyl phthalate, 1.2 μg/L), found in their urine (51). Strong evidence suggests that prenatal phthalate exposure was linked to altered placental size and form. Exposure to phthalates (~3 to 30 μg/kg/d) could cause the placenta to become thicker and more circular rather than round or oval with a centrally inserted umbilical cord (52).
Numerous studies have identified a relationship between phthalate metabolites in human third-trimester urine samples and target genes involved in the trophoblast differentiation and steroidogenesis pathways. The manifestation of a gene involved in trophoblast differentiation was inversely correlated with the presence of phthalate metabolites, including; butylbenzyl phthalate (BBzP), diisobutyl phthalate (DiBP), di-n-butyl phthalate (DnBP), and DEHP (53). Additionally, mono(2-ethylhexyl) phthalate (MEHP) decreased extravillous trophoblast (EVT) invasiveness, a crucial component of placentation. In human trophoblast cells, BPA, nonylphenol (NP), and octylphenol (OP) similarly cause cytotoxicity (>500 μM) (54). According to studies, DEHP administration at GD 13 significantly reduced placenta weight. Others have found that the DEHP-treated placenta significantly decreased Esx1, Ascl2, and Fosl1 mRNA expression levels at GD 13. DEHP injection disrupted the labyrinth vascularization of the placenta, which also hampered development and induced apoptosis (55).
Numerous studies have shown that BPA exposure around the time of implantation has a negative impact on placentation and uterine renovation. The occurrence and size of placentas, fetuses, and implantations were studied in pregnant female mice exposed to 50 µg/kg BPA/day or 0.1% ethanol by oral gavage from day 1 to day 7 of gestation. Blood flow in the arteria uterine was also examined, and the results showed that the remodeling of the uterine spiral arteries (SAs) was severely compromised (56). There is strong evidence that BPA has been identified in follicular fluids, maternal, human fetal, and amniotic, as well as deposits found in placenta cells (57). In addition to blocking human trophoblast cell invasion, this chemical significantly modifies the structure of mouse placental layers and exhibits characteristics similar to those of PE (58). Recent studies indicate that BPA exposure may directly harm the placenta, leading to abnormal trophoblast cell labyrinthine development that negatively impacts trophoblast hCG production in the first trimester and elevates cell mortality. However, it has been found in multiple studies that BPA reduced HIF-1α levels and increased levels of anti-apoptotic proteins, including Hsp70 and Bcl-2 in BeWo cells, hence reducing apoptosis (59).
According to studies, BPA at low doses (1–1000 nM) could activate the ERK signaling pathway to suppress the expression of CYP11A1 and CYP19, lower placental aromatase activity, and reduce the generation of estradiol and progesterone (60). The usage of bisphenol S (BPS, 0.5 mg/kg/day), a BPA substitute, has also been linked to endocrine dysfunction and interference with trophoblast fusion in the placenta (61). It is worth noting that a neuroendocrine mechanism was hypothesized in some studies where BPA (62.5-25.0 mg/kg) exposure impacted pituitary function in infantile rats, lowering basal and GnRH-induced LH and increasing GnRH pulsatility (62).
Insect, weed, and fungal growth are all controlled by a class of organic molecules known as organochlorine pesticides (OCPs), which include carbon, hydrogen, and chlorine. According to statistics, 40% of all pesticides used in diverse pest control methods come from the chemical class of organochlorines. This broad category of chlorinated hydrocarbons includes DDT and its metabolites, hexachlorobenzene (HCB), lindane, and dieldrin. They can become active, enter the mother’s bloodstream, and eventually reach the placenta (63).
These substances can potentially impair fetal growth and the terminal stage of placental life by interfering with the placenta’s ability to produce and release hormones, and enzymes, transport nutrients, and produce waste. In the epidemiological model, high p,p’-DDT are substantially correlated with insulin-like growth factor 2 (IGF2), which may help explain fetal development restriction. Maternal organochlorine pesticide exposure levels (0.7-1.7 ng/g lw) were also linked to the hyper-methylation of placental genes (64).
OCP compound residues in the placenta of females are associated with pre-term and full-term deliveries. The glutathione and malondialdehyde were assessed as oxidative stress markers, and significantly higher levels of α-HCH (63.04 ng g-1), total HCH (41.5 ng/g lw), p,p-DDE (59.3 ng/g lw), and total DDT (5100 ng/g lipid) in the placenta were recorded (65). Similarly, in venous and umbilical cord blood from pregnant Hispanic women, biomarkers of OCPs were assessed. Low exposure to these OC chemicals during placental development may have impacted the development of the fetus. They may have caused later health issues like diabetes, obesity, cancer, and endocrine disruption (66). Another study discovered that various pesticides, such as methoxychlor (OC) and fenitrothion (OP), are human multi-resistant associate protein substrates (MRP1). MRP2 is expressed in the syncytiotrophoblast, whereas MRP1 and MRP3 are expressed in the blood vessel endothelia and the syncytiotrophoblast (67). Blood cholinesterase and tissue carboxylesterases (CEs), which are reliable markers, can determine whether an individual has been exposed to OPs. Robinson et al. (68) evaluate the results of OP exposure (median=28.5 nmol/L) on placental CE action and lipid composition in humans and hypothesize that these pesticides similarly enter the placenta. Lower CE function may have toxicological and clinical implications that can disrupt the growth and development of the fetus. Lipid profile changes suggest cytotrophoblast hyperplasia as a potential healing strategy.
In addition, the OCPs may act as endocrine disruptors leading to pre-term birth (PTB) through disturbance of the average estrogen-progesterone ratio. Significantly higher levels of α-hexa-chlor-ocyclohexane (α-HCH; 40 mg/kg/day), β-hexa-chlor-cyclohexane (β-HCH; 5 mg/kg/day), dichloro-diphenyl-dichloroethane (DDD; ≥5.83 ng/g lipid), and dichloro-diphenyl-dichloroethylene (DDE; 45.4–94.6 ng/g lipid) were found in maternal blood and placenta as well. Other studies indicate a risk of early exposure for babies because of chronic bioaccumulation and inadequate removal, which could have a negative impact on health (69). Several pesticides and their compounds can survive in the food chain due to their lipophilicity and resilience to environmental degradation (70).
Numerous types of furniture and electronic devices have been treated with brominated flame retardants (BFRs), such as polybrominated diphenyl ethers (PBDEs) and 2,4,6-tribromophenol (2,4,6-TBP), which are also used as reactive flame retardants. A class of EDCs known as PBDEs has been extensively used in electronic equipment, construction materials, foam, textile materials, and automobile parts (71). Similar chemical structures are shared by 2,4,6-TBP and PBDEs, raising questions about how they might affect thyroid hormone (TH) regulation. Numerous epidemiological studies show that exposure to PBDE during placentation affects the regulation of THs by interfering with TH serum transporters, may affect TH concentrations in the placenta, and may affect TH delivery to the fetus. Although 2-, 4-, and 6-TBP are not well-studied, data show that human exposure is widespread and ongoing, similar to PBDE exposure (72). In vitro studies have shown that using a human primary villous cytotrophoblast (CTB) model demonstrates polybrominated diphenyl ethers (BDE-47) to inhibit the ability of human EVTs in the first trimester to migrate and invade (73).
Numerous research has investigated how PBDE exposures may cause placenta abnormalities. Greater maternal PBDE levels were strongly linked to worse newborn head circumference (HC) and Apgar1 scores (73). Multiple metabolic abnormalities may alter the connections by escalating oxidative stress, regulating vasodilation, mediating neurotoxicity, and causing dysbiosis in the mother’s gut microbiota (74). When discussing the potential link between PBDEs and neurodevelopmental issues, evidence for PBDE-induced epigenetic changes in DNA methylation, chromatin dynamics, and non-coding RNA expression was examined (75). At levels greater than 10 mM (BDE-47 and BDE-99), PBDE exposure is cytotoxic in first-trimester human EVTs, substantially lowering cell viability and inducing apoptosis. The same dose of BDE-47 also changed the metabolism of lipids and cholesterol while decreasing EVTs migration and invasion (76).
Fire-master 550 (FM 550) is a commercial flame retardant mixture (brominated and organophosphate) due to widespread human exposure and structural similarities with known EDCs. In vivo, the Wistar rat model indicated that these environmental chemicals might accumulate in the placenta, and there is evidence of an impact on sex-specific behavioral effects (77). Additionally, mothers’ exposure to these contaminants during pregnancy causes the bioaccumulation of flame retardant additives (FRAs) in their systems, including the placenta, blood, and serum. One of the exposure routes for FRAs is through human breast milk and blood or serum. These FRAs pollutants are transferred to infants through the mothers’ placenta, blood, and serum (69).
Other common chemicals used as flame retardants include organophosphate flame retardants (OPFRs) and perfluoroalkyl and polyfluoroalkyl substances (PFASs). PFAS and OPFRs were first studied in pregnant women’s mid-gestational urine samples. One of the morphological outcomes, along with potential placental stress markers, was the degree of uterine artery remodeling due to cytotrophoblast (CTB) (78). In addition, rodent studies suggested that PFASs (19.62 ng/ml) interfere with thyroid and placental function. These effects have been linked to various health effects, including bladder cancer, congenital hypothyroidism, and placenta-mediated adverse pregnancy and birth outcomes, such as preeclampsia, gestational diabetes, and low birth weight (Figure 3) (81).
Figure 3 A comprehensive summary of the developmental, anatomical, and histopathological sites in the placenta that have been affected by EDCs exposure. (1). Immature placenta (left): cytotrophoblasts (CTB; grey) can differentiate into two separate lineages: invasive extravillous trophoblasts (EVT; yellow) and barrier syncytiotrophoblasts (STBs; green). The arrows represent that EVTs penetrate maternal tissues and, by modification of the spiral artery (SA), enable enhanced maternal blood flow to the placenta. (2). CTBs restore the STBs population in the mature placenta (right), while SAs that have undergone EVT remodeling fill the fetal villi in maternal blood. Placental gross mass/wet weight (grey box 1), CTB fusion/syncytialization (grey box 2), EVT invasion, and SA remodeling (grey box 3) are only a few of the changes caused by EDC; PCBs: polychlorinated biphenyls, PBDEs: polybrominated diphenyl ethers, and PFCs: perfluorinated compounds (Figure 3 modified and edited from (79, 80)).
The most often used brominated flame retardant for consumer products is tetra-Bromo-bisphenol A (TBBPA). The results of a study on the human first-trimester placental cell line HTR-8/SVneo show that TBBPA activates inflammatory pathways, specifically cytokine and prostaglandin synthesis. In HTR-8/SVneo cells, TBBPA increased interleukin (IL)-6, IL-8, and prostaglandin E2 (PGE2) release while suppressing TGF-β release (82). Similarly, TBBPA exposure (5, 10, 20, and 50 μM) was evaluated using placental explant cultures, which confirmed (at 50 μM) it induces placental inflammation, increases oxidative stress, and alters placental steroidogenesis (80). In vitro investigation revealed that TBBPA varies JEG-3 (choriocarcinoma-derived placental cells) estrogen synthesis due to its action on CYP19 protein expression. Thus this compound may interfere with normal placental development during the early stages (83).
The UV filters and preservatives included in cosmetics, sunscreens, and personal care products have been the subject of several studies, but little is known about their potential effects on the placenta. Most of the antioxidants in the placenta belong to the paraben family, and concerns have been raised regarding how they can affect EDCs. One epidemiologic study focused on placenta-specific outcomes discovered a developmental abnormality in the placenta using a positive correlation between total maternal urinary paraben levels and placental weight (84). Pharmacokinetic research in pregnant rats is the only in vivo model that investigates gestational exposure; in this investigation, ethylparaben concentrations in the placenta (~3368-53,515 ng/g) and fetal liver were three times greater than in the fetal liver suggesting placental accumulation. In addition, the negative correlation between testosterone levels and ethylparaben concentrations in human cord blood suggests a possible danger to fetal development (85).
The movement and breakdown of the parabens ethyl, butyl, methyl, and propyl (EtP, BuP, MeP, and PrP), as well as their metabolite para-hydroxybenzoic acid (PHBA) via the human placenta, are demonstrated by an ex vivo human placental perfusion model (86). When excrement and amniotic fluid were tested for 2,4- and 2,5-dichlorophenols, bisphenol A, benzophenone-3, triclosan, and ethyl-, butyl-, and methyl and propyl, parabens, it was determined that amniotic fluid was positive for paraben (87).
It was shown through an in vitro investigation that butylparaben exposure reduces cell proliferation while inducing cell death and endoplasmic reticulum stress (200 μM) in HTR-8/SVneo cell lines (88) see Table 1. Apoptosis is triggered, and HTR8/SVneo cell proliferation is inhibited by the popular UV filter 4-MBC (95). Additional epidemiological studies have looked at the primary forms of parabens in pregnant women and their relationships with thyroid and reproductive hormones, suggesting alterations for methyl and butyl parabens (105). A few parabens are currently prohibited from cosmetics and cannot be used on newborns, toddlers, or children under three’s diaper area. Parabens, like methyl-, ethyl-, propyl and-butyl-parabens, are safe (106). Ultraviolet filters classified as EDCs with relation to the disruption of the hypothalamic-pituitary-gonadal (HPG) system include cinnamonate derivatives, camphor derivatives, and benzophenone (BP)-type. In several in vivo, in vitro, and in silico bioassays, BPs were found to have multiple endocrine-disrupting effects on the androgen receptor (AR), estrogen receptor (ER), progesterone receptor (PR), and other nuclear receptors (107).
Table 1 Worldwide In vitro and In vivo studies on EDCs exposure impact on placenta and placenta development.
This study highlights the current understanding of placental outcomes following EDC exposure by reviewing epidemiological in vivo and in vitro data. The use of supraphysiological dose regimens, which are often greater than human exposures and represent directly deleterious impacts, is one of the significant drawbacks. Human biomonitoring and in vivo pharmacokinetic data should be used to develop predictive physiologically-based toxicokinetic mathematical models to establish environmentally relevant dosage strategies. These doses should be used in conjunction with in vitro models that more closely replicate the in vivo placental microenvironments, such as 3-dimensional models that recreate cell-to-cell interactions and add tissue shear stress and extracellular matrix components. These alternative in vitro models should be utilized in conjunction with in vivo research incorporating suitable animal models to capture more human-representative toxicokinetic profiles or anatomical features. Unfortunately, the data on animal model utilization is lacking on this topic.
Finally, more research is needed to provide more exact effect estimates in risk assessment epidemiological investigations. Nonetheless, given the potential early impacts of endocrine disruptors on child health, pregnant women, infants, and young children should be given special care. This study also uncovers significant knowledge gaps that will guide future research.
Study conception and design: YY and YW. Drafting of the manuscript: FG, KL, and RD. Critical revision: YY, FG and YW. Approve final version: All authors.
The authors declare that the research was conducted in the absence of any commercial or financial relationships that could be construed as a potential conflict of interest.
All claims expressed in this article are solely those of the authors and do not necessarily represent those of their affiliated organizations, or those of the publisher, the editors and the reviewers. Any product that may be evaluated in this article, or claim that may be made by its manufacturer, is not guaranteed or endorsed by the publisher.
1. Melnick R, Lucier G, Wolfe M, Hall R, Stancel G, Prins G, et al. Summary of the national toxicology program's report of the endocrine disruptors low-dose peer review. Environ Health Perspectives (2002) 110(4):427–31. doi: 10.1289/ehp.02110427
2. Tang ZR, Xu XL, Deng SL, Lian ZX, Yu K. Oestrogenic endocrine disruptors in the placenta and the fetus. Int J Mol Sci (2020) 21(4):1519. doi: 10.3390/ijms21041519
3. Kumar M, Sarma DK, Shubham S, Kumawat M, Verma V, Prakash A, et al. Environmental endocrine-disrupting chemical exposure: Role in non-communicable diseases. Front Public Health (2020) 8:553850. doi: 10.3389/fpubh.2020.553850
4. Rosenfeld CS, Cooke PS. Endocrine disruption through membrane estrogen receptors and novel pathways leads to rapid toxicological and epigenetic effects. J Steroid Biochem Mol Biol (2019) 187:106–17. doi: 10.1016/j.jsbmb.2018.11.007
5. Braun JM. Early-life exposure to EDCs: Role in childhood obesity and neurodevelopment. Nat Rev Endocrinol (2017) 13(3):161–73. doi: 10.1038/nrendo.2016.186
6. Yang C, Song G, Lim W. A mechanism for the effect of endocrine disrupting chemicals on placentation. Chemosphere (2019) 231:326–36. doi: 10.1016/j.chemosphere.2019.05.133
8. Velicky P, Windsperger K, Petroczi K, Pils S, Reiter B, Weiss T, et al. Pregnancy-associated diamine oxidase originates from extravillous trophoblasts and is decreased in early-onset preeclampsia. Sci Rep (2018) 8(1):1–11. doi: 10.1038/s41598-018-24652-0
9. Knöfler M, Haider S, Saleh L, Pollheimer J, Gamage TK, James J. Human placenta and trophoblast development: Key molecular mechanisms and model systems. Cell Mol Life Sci (2019) 76(18):3479–96. doi: 10.1007/s00018-019-03104-6
10. Turco MY, Moffett A. Development of the human placenta. Development (2019) 146(22):dev163428. doi: 10.1242/dev.163428
11. Cindrova-Davies T, Sferruzzi-Perri AN. Human placental development and function. in seminars in cell & developmental biology. Elsevier (UK): Academic Press (2022) 131:6677. doi: 10.1016/j.semcdb.2022.03.039
12. Reijnders IF, Mulders A. G. M. G. J., Koster MPH, Koning AHJ, Frudiger A, Willemsen SP, et al. New imaging markers for preconception and first-trimester uteroplacental vascularization. Placenta (2018) 61:96–102. doi: 10.1016/j.placenta.2017.11.013
13. Dusza HM, Janssen E, Kanda R, Legler J. Method development for effect-directed analysis of endocrine disrupting compounds in human amniotic fluid. Environ Sci Technol (2019) 53(24):14649–59. doi: 10.1021/acs.est.9b04255
14. Huppertz B, Weiss G, Moser G. Trophoblast invasion and oxygenation of the placenta: measurements versus presumptions. J Reprod Immunol (2014) 101:74–9. doi: 10.1016/j.jri.2013.04.003
15. Montes-Grajales D, Olivero-Verbel J. EDCs DataBank: a 3D-structure database of endocrine disrupting chemicals. Toxicology (2015) 327:87–94. doi: 10.1016/j.tox.2014.11.006
16. Monneret C. What is an endocrine disruptor? Comptes Rendus Biol (2017) 340(9-10):403–5. doi: 10.1016/j.crvi.2017.07.004
17. Derfoul A, Lin FJ, Awumey EM, Kolodzeski T, Hall DJ, Tuan RS. Estrogenic endocrine disruptive components interfere with calcium handling and differentiation of human trophoblast cells. J Cell Biochem (2003) 89(4):755–70. doi: 10.1002/jcb.10558
18. Kezios KL, Liu X, Cirillo PM, Cohn BA, Kalantzi OI, Wang Y, et al. Dichlorodiphenyltrichloroethane (DDT), DDT metabolites and pregnancy outcomes. Reprod Toxicol (2013) 35:156–64. doi: 10.1016/j.reprotox.2012.10.013
19. Shekhar S, Sood S, Showkat S, Lite C, Chandrasekhar A, Vairamani M, et al. Detection of phenolic endocrine disrupting chemicals (EDCs) from maternal blood plasma and amniotic fluid in Indian population. Gen Comp Endocrinol (2017) 241:100–7. doi: 10.1016/j.ygcen.2016.05.025
20. Basak S, Das MK, Duttaroy AK. Plastics-derived endocrine-disrupting compounds and their effects on early development. Congenital Disabil Res (2020) 112(17):1308–25. doi: 10.1002/bdr2.1741
21. Pycke BFG, Geer LA, Dalloul M, Abulafia O, Jenck AM, Halden RU. Human fetal exposure to triclosan and triclocarban in an urban population from brooklyn, new York. Environ Sci Technol (2014) 48:8831–8. doi: 10.1021/es501100w
22. Valle-Sistac J, Molins-Delgado D, Diaz M, Ibanez L, Barcelo D, et al. Determination of parabens and benzophenone-type UV filters in human placenta. the first description of the existence of benzyl paraben and benzophenone-4. Environ Int (2016) 88:243–9. doi: 10.1016/j.envint.2015.12.034
23. Woods MM, Lanphear BP, Braun JM, McCandless LC. Gestational exposure to endocrine disrupting chemicals about infant birth weight: A Bayesian analysis of the HOME study. Environ Health (2017) 16(1):1–12. doi: 10.1186/s12940-017-0332-3
24. Guo LC, Pan S, Yu S, Liu T, Xiao J, Zhu B, et al. Human sex hormone-disrupting effects of new flame retardants and their interactions with polychlorinated biphenyls, polybrominated diphenyl ethers, a case study in south China. Environ Sci Technol (2018) 52(23):13935–41. doi: 10.1021/acs.est.8b01540
25. Basak S, Srinivas V, Duttaroy AK. Bisphenol-A impairs cellular function and alters DNA methylation of Stress pathway genes in first-trimester trophoblast cells. Reprod Toxicol (2018) 1(82):72–9. doi: 10.1016/j.reprotox.2018.10.009
26. Ziv-Gal A, Flaws JA. Evidence for bisphenol a-induced female infertility: a review (2007–2016). Fertil Steril (2016) 106(4):827–56. doi: 10.1016/j.fertnstert.2016.06.027
27. Margel D, Fleshner NE. Oral contraceptive use is associated with prostate cancer: an ecological study. BMJ Open (2011) 1(2):e000311. doi: 10.1136/bmjopen-2011-000311
28. Hormann AM, Vom Saal FS, Nagel SC, Stahlhut RW, Moyer CL, Ellersieck MR, et al. Holding thermal receipt paper and eating food after using handsanitizer results in high serum bioactive and total urine levels of bisphenol a (BPA). PloS One (2014) 9(10):e110509. doi: 10.1371/journal.pone.0110509
29. Ndaw S, Remy A, Jargot D, Robert A. Occupational exposure of cashiers to bisphenol a via thermal paper: Urinary biomonitoring study. Int Arch Occup Environ Health (2016) 89(6):935–46. doi: 10.1007/s00420-016-1132-8
30. Cimmino I, Fiory F, Perruolo G, Miele C, Beguinot F, Formisano P, et al. Potential mechanisms of bisphenol a (BPA) contributing to human disease. Int J Mol Sci (2020) 21(16):5761. doi: 10.3390/ijms21165761
31. Kim S, Cho YH, Lee I, Kim W, Won S, Ku JL, et al. Prenatal exposure to persistent organic pollutants and methylation of LINE-1and imprinted genes in the placenta: A CHECK cohort study. Environ Int (2018) 119: 398–406. doi: 10.1016/j.envint.2018.06.039
32. Street ME, Bernasconi S. Endocrine-disrupting chemicals in human fetal growth. Int J Mol Sci (2020) 21(4):1430. doi: 10.37247/PAMB.1.2020.42
33. Susiarjo M, Sasson I, Mesaros C, Bartolomei MS. Bisphenol exposure disrupts genomic imprinting in the mouse. PloS Genet (2013) 9(4):e1003401. doi: 10.1371/journal.pgen.1003401
34. Hanna CW, Bloom MS, Robinson WP, Kim D, Parsons PJ, et al. DNA Methylation changes in whole blood is associated with exposure to the environmental contaminants, mercury, lead, cadmium and bisphenol a, in women undergoing ovarian stimulation for IVF. Hum Reprod (2012) 27:1401–10. doi: 10.1093/humrep/des038
35. Ashizawa A, Faroon O, Ingerman L, Jenkins K, Tucker P, Wright S. Draft Toxicological Profile for Cadmium. Agency for Toxic Substances and Disease Registry (ATSDR). Atlanta, GA, USA (2008).
36. Jacobo-Estrada T, Santoyo-Sánchez M, Thévenod F, Barbier O. Cadmium handling, toxicity and molecular targets involved during pregnancy: Lessons from experimental models. Int J Mol Sci (2017) 18(7):1590. doi: 10.3390/ijms18071590
37. Geng HX, Wang L. Cadmium: Toxic effects on placental and embryonic development. Environ Toxicol Pharmacol (2019) 67:102–7. doi: 10.1016/j.etap.2019.02.006
38. Punshon T, Li Z, Jackson BP, Parks WT, Romano M, Conway D, et al. Placental metal concentrations concerning placental growth, efficiency and birth weight. Environ Int (2019) 126:533–42. doi: 10.1016/j.envint.2019.01.063
39. Hu J, Wang H, Hu YF, Xu XF, Chen YH, Xia MZ, et al. Cadmium induces inflammatory cytokines by activating akt signaling in mouse placenta and human trophoblast cells. Placenta (2018) 65:7–14. doi: 10.1016/j.placenta.2018.03.008
40. Nakamura M, Yamanaka H, Oguro A, Imaoka S. Bisphenol a induces Nrf2-dependent drug-metabolizing enzymes through nitrosylation of Keap1. Drug Metab Pharmacokinet (2018) 33(4):194–202. doi: 10.1016/j.dmpk.2018.04.003
41. Espart A, Artime S, Tort-Nasarre G, Yara-Varón E. Cadmium exposure during pregnancy and lactation: materno-fetal and newborn repercussions of cd (II), and cd–metallothionein complexes. Metallomics (2018) 10(10):1359–67. doi: 10.1039/C8MT00174J
42. Fry RC, Bangma J, Szilagyi J, Rager JE. Developing novel in vitro methods for the risk assessment of developmental and placental toxicants in the environment. Toxicol Appl Pharmacol (2019) 378:114635. doi: 10.1016/j.taap.2019.114635
43. Ask K, Akesson A, Berglund M, Vahter M. Inorganic mercury and methylmercury in placentas of Swedish women. Environ Health Perspect (2002) 110(5):523–6. doi: 10.1289/ehp.02110523
44. Gilman CL, Soon R, Sauvage L, Ralston NV, Berry MJ. Umbilical cord blood and placental mercury, selenium and selenoprotein expression about maternal fish consumption. J Trace Elements Med Biol (2015) 30:17–24. doi: 10.1016/j.jtemb.2015.01.006
45. Czajkowska M, Chrobaczynska M, Suprewicz K, Ciaglo A, Stawarz R, Formicki G, et al. (2010). Accumulation of mercury in placenta and amnion of women from upper silesian region–Poland, in: Proceedings of the 15th International Conference on Heavy Metals in the Environment, Gdansk, Poland, 1923 September 2010 Żaneta B, Beyer A, Klimaszewska K, Namieśnik J, Tobiszewski M, Rutkiewicz I, et al Eds. Gdansk University of Technology: Gdansk, Poland pp. 547–50.
46. Park SS, Skaar DA, Jirtle RL, Hoyo C. Epigenetics, obesity and early-life cadmium or lead exposure. Epigenomics (2017) 9(1):57–75. doi: 10.2217/epi-2016-0047
47. Lafond J, Hamel A, Takser L, Vaillancourt C, Mergler D. Low environmental contamination by lead in pregnant women affects calcium transfer in human placental syncytiotrophoblasts. J Toxicol Environ Health Part A (2004) 67(14):1069–79. doi: 10.1080/15287390490452263
48. Pérez-Albaladejo E, Fernandes D, Lacorte S, Porte C. Comparative toxicity, oxidative stress and endocrine disruption potential of plasticizers in JEG-3 human placental cells. Toxicol Vitro (2017) 38:41–8. doi: 10.1016/j.tiv.2016.11.003
49. Martínez-Razo LD, Martínez-Ibarra A, Vázquez-Martínez ER, Cerbón M. Di-(2-ethylhexyl) phthalate and mono (2-ethylhexyl) phthalate's impact on placental development, function, and pathophysiology. Environ Int (2021) 146:106228. doi: 10.1016/j.envint.2020.106228
50. Sun CC, Zhao S, Chu LL, Zhang SY, Li YL, Sun MF, et al. Di (2-ethyl-hexyl) phthalate disrupts placental growth in a dual-blocking mode. J Hazardous Mater (2022) 421:126815. doi: 10.1016/j.jhazmat.2021.126815
51. Cantonwine DE, Meeker JD, Ferguson KK, Mukherjee B, Hauser R, McElrath TF. Urinary concentrations of bisphenol a and phthalate metabolites measured during pregnancy and risk of preeclampsia. Environ Health Perspect (2016) 124(10):1651–5. doi: 10.1289/EHP188
52. Zhu YD, Gao H, Huang K, Zhang YW, Cai XX, Yao HY, et al. Prenatal phthalate exposure and placental size and shape at birth: a cohort study. Environ Res (2018) 160:239–46. doi: 10.1016/j.envres.2017.09.012
53. Adibi JJ, Whyatt RM, Hauser R, Bhat HK, Davis BJ, Calafat AM, et al. Transcriptional biomarkers of steroidogenesis and trophoblast differentiation in the placenta concerning prenatal phthalate exposure. Environ Health Perspect (2010) 118(2):291–6. doi: 10.1289/ehp.0900788
54. Zong T, Lai L, Hu J, Guo M, Li M, Zhang L, et al. Maternal exposure to di-(2-Ethylhexyl) phthalate disrupts placental growth and development in pregnant mice. J Hazardous Mater (2015) 297:25–33. doi: 10.1016/j.jhazmat.2015.04.065
55. Müller JE, Meyer N, Santamaria CG, Schumacher A, Luque EH, Zenclussen ML, et al. Bisphenol a exposure during early pregnancy impairs uterine spiral artery remodeling and provokes intrauterine growth restriction in mice. Sci Rep (2018) 8(1):1–10. doi: 10.1038/s41598-018-27575-y
56. Ejaredar M, Lee Y, Roberts DJ, Sauve R, Dewey D. Bisphenol a exposure and children's behaviour: A systematic review. J Exposure Sci Environ Epidemiol (2017) 27:175–83. doi: 10.1038/jes.2016.8
57. Ye Y, Tang Y, Xiong Y, Feng L, Li X. Bisphenol a exposure alters placentation and causes preeclampsia-like features in pregnant mice involved in the reprogramming of DNA methylation of WNT2. Fed Am Societies Exp Biol J (2019) 33(2):2732–42. doi: 10.1096/fj.201800934RRR
58. Ponniah M, Billett EE, De Girolamo LA. Bisphenol a increases BeWo trophoblast survival in stress-induced paradigms through the regulation of oxidative stress and apoptosis. Chem Res Toxicol (2015) 28(9):pp.1693–1703. doi: 10.1021/acs.chemrestox.5b00093
59. Chu P, Yang Z, Huang H, Chang A, Cheng Y, Wu G, et al. Low-dose bisphenol a activates the ERK signaling pathway and attenuates steroidogenic gene expression in human placental cells. Biol Reprod (2018) 98:250–8. doi: 10.1093/biolre/iox162
60. Parent AS, Franssen D, Fudvoye J, Gerard A, Bourguignon JP. Developmental variations in environmental influences including endocrine disruptors on pubertal timing and neuroendocrine control: Revision of human observations and mechanistic insight from rodents. Front Neuroendocrinol (2015) 38:12–36. doi: 10.1016/j.yfrne.2014.12.004
61. Anand M, Agarwal P, Singh L. Persistent organochlorine pesticides and oxidant/antioxidant status in the placental tissue of the women with full-term and pre-term deliveries. Toxicol Res (2015) 4:326–32. doi: 10.1039/C4TX00094C
62. Pathak R, Mustafa M, Ahmed RS, Tripathi AK, Guleria K, Banerjee BD. Association between recurrent miscarriages and organochlorine pesticide levels. Clin Biochem (2010) 43:131–5. doi: 10.1016/j.clinbiochem.2009.09.019
63. Sexton K, Salinas JJ, McDonald TJ, Gowen RM, Miller RP, McCormick JB, et al. Biomarker measurements of prenatal exposure to polychlorinated biphenyls (PCB) in umbilical cord blood from postpartum Hispanic women in brownsville, Texas. J Toxicol Environ Health Part A (2013) 76(22):1225–35. doi: 10.1080/15287394.2013.848744
64. Magnarelli G. Exposición ambiental a plaguicidas: biomarcadores en matrices de la tríada madre-placenta-feto. Acta Bioquímica Clínica Latinoamericana (2015) 49(1):39–53.
65. Vera B, Santa Cruz S, Magnarelli G. Plasma cholinesterase and carboxylesterase activities and nuclear and mitochondrial lipid composition of the human placenta are associated with maternal pesticide exposure. Reprod Toxicol (2012) 34(3):402–7. doi: 10.1016/j.reprotox.2012.04.007
66. Tyagi V, Garg N, Mustafa MD, Banerjee BD, Guleria K. Organochlorine pesticide levels in maternal blood and placental tissue concerning pre-term birth: a recent trend in north Indian population. Environ Monit Assess (2015) 187(7):1–9. doi: 10.1007/s10661-015-4369-x
67. Xu J, Fang R, Wang H, Sun DX, Yang J, Huang X, et al. A review of environmental metabolism disrupting chemicals and effect biomarkers associating disease risks: Where exposomics meets metabolomics. Environ Int (2022) 158:106941. doi: 10.1016/j.envint.2021.106941
68. Leonetti C, Butt CM, Hoffman K, Miranda ML, Stapleton HM. Concentrations of polybrominated diphenyl ethers (PBDEs) and 2, 4, 6-tribromophenol in human placental tissues. Environ Int (2016) 88:23–9. doi: 10.1016/j.envint.2015.12.002
69. Robinson JF, Kapidzic M, Hamilton EG, Chen H, Puckett KW, Zhou Y, et al. Genomic profiling of BDE-47 effects on human placental cytotrophoblasts. Toxicol Sci (2019) 167(1):pp.211–226. doi: 10.1093/toxsci/kfy230
70. Gill HK, Garg H. Pesticide: environmental impacts and management strategies. Pesticides-toxic Aspects (2014) 8:187. doi: 10.5772/57399
71. Wang Y, Wang Q, Zhou L, Zeng Z, Zhao C, You L, et al. Metabolomics insights into the prenatal exposure effects of polybrominated diphenyl ethers on neonatal birth outcomes. Sci Total Environ (2022) 836:155601. doi: 10.1016/j.scitotenv.2022.155601
72. Poston RG, Saha RN. Epigenetic effects of polybrominated diphenyl ethers on human health. Int J Environ Res Public Health (2019) 16(15):2703. doi: 10.3390/ijerph16152703
73. Baldwin KR, Phillips AL, Horman B, Arambula SE, Rebuli ME, Stapleton HM, et al. Sex-specific placental accumulation and behavioral effects of developmental firemaster 550 exposure in wistar rats. Sci Rep (2017) 7(1):1–13. doi: 10.1038/s41598-017-07216-6
74. Gravel S, Aubin S, Labrèche F. Assessment of occupational exposure to organic flame retardants: A systematic review. Ann Work Exposures Health (2019) 63(4):pp.386–406. doi: 10.1093/annweh/wxz012
75. Lopez-Espinosa MJ, Costa O, Vizcaino E, Murcia M, Fernandez-Somoano A, Inãiguez C, et al. Prenatal exposure to polybrominated flame retardants and fetal growth in the INMA cohort (Spain). Environ Sci Technol (2015) 49(16):pp.10108–10116. doi: 10.1021/acs.est.5b01793
76. Varshavsky JR, Robinson JF, Zhou Y, Puckett KA, Kwan E, Buarpung S, et al. Organophosphate flame retardants, highly fluorinated chemicals, placental development and disease biomarkers during mid-gestation. Toxicol Sci (2021) 181(2):215–28. doi: 10.1093/toxsci/kfab028
77. Blake BE, Fenton SE. Early life exposure to per-and polyfluoroalkyl substances (PFAS) and latent health outcomes: A review including the placenta as a target tissue and possible driver of peri-and postnatal effects. Toxicology (2020) 443:152565. doi: 10.1016/j.tox.2020.152565
78. Park HR, Kamau PW, Korte C, Loch-Caruso R. Tetrabromobisphenol a activates inflammatory pathways in human first-trimester extravillous trophoblasts in vitro. Reprod Toxicol (2014) 1(50):154–62. doi: 10.1016/j.reprotox.2014.10.005
79. Honkisz E, Wójtowicz AK. Modulating estradiol synthesis and aromatase activity in human choriocarcinoma JEG-3 cells exposed to tetrabromobisphenol a. Toxicol Vitro (2015) 29(1):44–50. doi: 10.1016/j.tiv.2014.09.003
80. Gingrich J, Ticiani E, Veiga-Lopez A. Placenta disrupted: endocrine disrupting chemicals and pregnancy. Trends Endocrinol Metab (2020) 31(7):pp.508–524. doi: 10.1016/j.tem.2020.03.003
81. Arita Y, Pressman M, Getahun D, Menon R, Peltier MR. Effect of tetrabromobisphenol a on the expression of biomarkers for inflammation and neurodevelopment by the placenta. Placenta (2018) 68:33–9. doi: 10.1016/j.placenta.2018.06.306
82. Wang J, Pan L, Wu S, Lu L, Xu Y, Zhu Y, et al. Recent advances on endocrine disrupting effects of UV filters. Int J Environ Res Public Health (2016) 13(8):782. doi: 10.3390/ijerph13080782
83. Vela-Soria F, Gallardo-Torres ME, Ballesteros O, Díaz C, Pérez J, Navalón A, et al. Assessment of parabens and ultraviolet filters in human placenta tissue by ultrasound-assisted extraction and ultra-high performance liquid chromatography-tandem mass spectrometry. J Chromatogr (2017) 1487:153–61. doi: 10.1016/j.chroma.2017.01.041
84. Andersen MHG, Zuri G, Knudsen LE, Mathiesen L. Placental transport of parabens was studied using an ex-vivo human perfusion model. Placenta (2021) 115:121–8. doi: 10.1016/j.placenta.2021.09.010
85. Philippat C, Wolff MS, Calafat AM, Ye X, Bausell R, Meadows M, et al. Prenatal exposure to environmental phenols: concentrations in amniotic fluid and variability in urinary concentrations during pregnancy. Environ Health Perspect (2013) 121(10):1225–31. doi: 10.1289/ehp.1206335
86. Yang C, Lim W, Bazer FW, Song G. Butylparaben promotes apoptosis in human trophoblast cells through increased oxidative stress-induced endoplasmic reticulum stress. Environ Toxicol (2018) 33(4):436–45. doi: 10.1002/tox.22529
87. Yang C, Lim W, You S, Song G. 4-methyl benzylidene-camphor inhibits proliferation and induces reactive oxygen species-mediated apoptosis of human trophoblast cells. Reprod Toxicol (2019) 84:49–58. doi: 10.1016/j.reprotox.2018.12.011
88. Aker AM, Watkins DJ, Johns LE, Ferguson KK, Soldin OP, Del Toro LV, et al. Phenols and parabens concerning reproductive and thyroidHormones in pregnant women. Environ Res (2016) 151:30–7. doi: 10.1016/j.envres.2016.07.002
89. Gonçalves BM, Graceli JB, da Rocha PB, Tilli HP, Vieira EM, de Sibio MT, et al. Placental model as an important tool to study maternal-fetal interface. Reprod Toxicol (2022) 112:7–13. doi: 10.1016/j.reprotox.2022.06.005
90. Profita M, Fabbri E, Spisni E, Valbonesi P. Comparing effects and action mechanisms of BPA and BPS on HTR-8/SVneo placental cells. Biol Reprod (2021) 105(5):1355–64. doi: 10.1093/biolre/ioab139
91. Yang C, Lim W, Bazer FW, Song G. Homosalate aggravates the invasion of human trophoblast cells and regulates intracellular signaling pathways, including PI3K/AKT and MAPK pathways. Environ pollut (2018) 243:1263–73. doi: 10.1016/j.envpol.2018.09.092
92. Gingrich J, Pu Y, Roberts J, Karthikraj R, Kannan K, Ehrhardt R, et al. Gestational bisphenol s impairs placental endocrine function and the fusogenic trophoblast signaling pathway. Arch Toxicol (2018) 92:1861–76. doi: 10.1007/s00204-018-2191-2
93. Zota AR, Geller RJ, Romano LE, Coleman-Phox K, Adler NE, Parry E, et al. Association between persistent endocrine-disrupting chemicals (PBDEs, OH-PBDEs, PCBs, and PFASs) and biomarkers of inflammation and cellular ageing during pregnancy and postpartum. Environ Int (2018) 115:9–20. doi: 10.1016/j.envint.2018.02.044
94. Eskenazi B, Rauch SA, Tenerelli R, Huen K, Holland NT, Lustig RH, et al. In utero and childhood DDT, DDE, PBDE and PCBs exposure and sex hormones in adolescent boys: The CHAMACOS study. Int J Hygiene Environ Health (2017) 220(2):364–72. doi: 10.1016/j.ijheh.2016.11.001
95. Kolatorova L, Duskova M, Vitku J, Starka L. Prenatal exposure to bisphenols and parabens and impacts on human physiology. Physiol Res (2017) 66:S305–15. doi: 10.33549/physiolres.933723
96. Forte M, Mita L, Cobellis L, Merafina V, Specchio R, Rossi S, et al. Triclosan and bisphenol a affect decidualization of human endometrial stromal cells. Mol Cell Endocrinol (2016) 15;422:74–83. doi: 10.1016/j.mce.2015.11.017
97. Crain DA, Janssen SJ, Edwards TM, Heindel J, Ho SM, Hunt P, et al. Female reproductive disorders: The roles of endocrine-disrupting compounds and developmental timing. Fertil Steril (2008) 90(4):911–40. doi: 10.1016/j.fertnstert.2008.08.067
98. LaRocca J, Binder AM, McElrath TF, Michels KB. First-trimester urine concentrations of phthalate metabolites and phenols and placenta miRNA expression in a cohort of US women. Environ Health Perspect (2016) 124(3):380–7. doi: 10.1289/ehp.1408409
99. Zhao M, Zhang Y, Zhuang S, Zhang Q, Lu C, Liu W. Disruption of the hormonal network and the enantioselectivity of bifenthrin in trophoblast: maternalfetal health risk of chiral pesticides. Environ Sci Technol (2014) 48(14):8109–16. doi: 10.1021/es501903b
100. Chen K, Cheng M, Jing Y, Chiu DT, Shiao M, Chen J. Resveratrol ameliorates metabolic disorders and muscle wasting in streptozotocin-induced diabetic rats (vol 301, pg E853, 2011). Am J Physiol-endocrinol Metab (2012) 302(3):E388. doi: 10.1152/ajpendo.00048.2011
101. Lee DH, Jacobs DR. Methodological issues in human studies of endocrine disrupting chemicals. Rev Endocrine Metab Disord (2015) 16(4):289–97. doi: 10.1007/s11154-016-9340-9
102. Gonzalez-Garcia B, Olave ME, Ramos-Martinez E, Gonzalez-Horta C, Levario- Carrillo M, Sanchez-Ramirez B. Decrease of muscarinic cholinergic-receptors expression in placenta from rats exposed to methyl parathion. Hum Exp Toxicol (2008) 27:241e246. doi: 10.1177/0960327108091863
103. Helmestam M, Stavreus-Evers A, Olovsson M. Cadmium chloride alters mRNA levels of angiogenesis-related genes in primary human endometrial endothelial cells grown in vitro. Reprod Toxicol (2010) 30(3):370–6. doi: 10.1016/j.reprotox.2010.05.003
104. Xu LC, Sun H, Chen JF, Bian Q, Qian J, Song L, et al. Evaluation of androgen receptor transcriptional activities of bisphenol a, octylphenol and nonylphenol in vitro. Toxicology (2005) 216(2-3):197–203. doi: 10.1016/j.tox.2005.08.006
105. Midic U, Goheen B, Vincent KA, VandeVoort CA, Latham KE. Changes in gene expression following long-term in vitro exposure of Macaca mulatta trophoblast stem cells to biologically relevant levels of endocrine disruptors. Reprod Toxicol (2018) 77:154–65. doi: 10.1016/j.reprotox.2018.02.012
106. De Aguiar Greca SC, Kyrou I, Pink R, Randeva H, Grammatopoulos D, Silva E, et al. Involvement of the endocrine-disrupting chemical bisphenol a (BPA) in human placentation. J Clin Med (2020) 9(2):405. doi: 10.3390/jcm9020405
Keywords: endocrine disrupting chemicals, placental development, bisphenol A, DDT, heavy metals, VU filters
Citation: Yan Y, Guo F, Liu K, Ding R and Wang Y (2023) The effect of endocrine-disrupting chemicals on placental development. Front. Endocrinol. 14:1059854. doi: 10.3389/fendo.2023.1059854
Received: 02 October 2022; Accepted: 03 February 2023;
Published: 21 February 2023.
Edited by:
Arnab Banerjee, Birla Institute of Technology and Science, IndiaReviewed by:
Taisen Iguchi, Graduate University for Advanced Studies (Sokendai), JapanCopyright © 2023 Yan, Guo, Liu, Ding and Wang. This is an open-access article distributed under the terms of the Creative Commons Attribution License (CC BY). The use, distribution or reproduction in other forums is permitted, provided the original author(s) and the copyright owner(s) are credited and that the original publication in this journal is cited, in accordance with accepted academic practice. No use, distribution or reproduction is permitted which does not comply with these terms.
*Correspondence: Yichao Wang, d3ljXzAwMDAwQGpsdS5lZHUuY24=
Disclaimer: All claims expressed in this article are solely those of the authors and do not necessarily represent those of their affiliated organizations, or those of the publisher, the editors and the reviewers. Any product that may be evaluated in this article or claim that may be made by its manufacturer is not guaranteed or endorsed by the publisher.
Research integrity at Frontiers
Learn more about the work of our research integrity team to safeguard the quality of each article we publish.