- 1Lunenfeld-Tanenbaum Research Institute, Prosserman Centre for Population Health Research, Mount Sinai Hospital, and Institute of Health Policy, Management and Evaluation, University of Toronto, Toronto, ON, Canada
- 2Department of Obstetrics and Gynecology, Mount Sinai Hospital, Temerty Faculty of Medicine, University of Toronto, Toronto, ON, Canada
- 3Lunenfeld-Tanenbaum Research Institute, Mount Sinai Hospital, University of Toronto, Toronto, ON, Canada
- 4Institute of Health Policy, Management and Evaluation, Dalla Lana School of Public Health, University of Toronto, Toronto, ON, Canada
- 5The Hospital for Sick Children, Toronto, ON, Canada
- 6Departments of Physiology and Nutritional Sciences, University of Toronto, Toronto, ON, Canada
Introduction: Myostatin is a member of the transforming growth factor β superfamily, and is mainly secreted from skeletal muscle. Animal studies have demonstrated that deficiency in myostatin promotes muscle growth and protects against insulin resistance. In humans, gestational diabetes mellitus (GDM) affects fetal insulin sensitivity. Females are more insulin resistant and weigh less than males at birth. We sought to assess whether cord blood myostatin concentrations vary by GDM and fetal sex, and the associations with fetal growth factors.
Methods: In a study of 44 GDM and 66 euglycemic mother-newborn dyads, myostatin, insulin, proinsulin, insulin-like growth factor (IGF)-1, IGF-2 and testosterone were measured in cord blood samples.
Results: Cord blood myostatin concentrations were similar in GDM vs. euglycemic pregnancies (mean ± SD: 5.5 ± 1.4 vs. 5.8 ± 1.4 ng/mL, P=0.28), and were higher in males vs. females (6.1 ± 1.6 vs. 5.3 ± 1.0 ng/mL, P=0.006). Adjusting for gestational age, myostatin was negatively correlated with IGF-2 (r=-0.23, P=0.02), but not correlated with IGF-1 (P=0.60) or birth weight (P=0.23). Myostatin was strongly correlated with testosterone in males (r=0.56, P<0.001), but not in females (r=-0.08, P=0.58) (test for difference in r, P<0.001). Testosterone concentrations were higher in males vs. females (9.5 ± 6.4 vs. 7.1 ± 4.0 nmol/L, P=0.017), and could explain 30.0% (P=0.039) of sex differences in myostatin concentrations.
Discussion: The study is the first to demonstrate that GDM does not impact cord blood myostatin concentration, but fetal sex does. The higher myostatin concentrations in males appear to be partly mediated by higher testosterone concentrations. These findings shed novel insight on developmental sex differences in insulin sensitivity regulation relevant molecules.
Introduction
Myostatin or growth differentiation factor 8 is a member of the transforming growth factor β superfamily, and is mainly secreted from skeletal muscle (1). Myostatin is a strong negative regulator of skeletal muscle growth (1, 2), while inhibition of myostatin or its signaling prevents fat accumulation and improves insulin sensitivity in mice (3–8). In humans, elevated myostatin levels in skeletal muscle or circulation have been associated with obesity and insulin resistance in adults (9–12). So far, there have been no studies on whether gestational diabetes mellitus (GDM) - a common pregnancy complication (13) that has been associated with impaired fetal insulin sensitivity but enhanced fetal growth (14), may affect cord blood myostatin concentration. Little is known about whether myostatin is associated with fetal growth or fetal growth factors.
Females weigh less than males at birth, despite higher concentrations of major fetal growth factors (insulin and IGF-1), suggesting that females are intrinsically more insulin resistant than males in utero (15–17). Given sex differences in fetal growth and insulin sensitivity, we hypothesized that myostatin may exhibit sex differences and correlate with fetal growth. We are aware of only one small study (n=83) on cord blood myostatin which reported no association with fetal sex, and a negative correlation with birth weight (18).
Testosterone promotes protein synthesis, skeletal and muscle growth (19, 20). Higher cord blood testosterone concentrations have been reported in males vs. females (21). Decreased testosterone concentrations have been associated with insulin resistance and type 2 diabetes in men (22). Testosterone treatment has been associated with increased myostatin concentrations in men (23). We thus hypothesized that testosterone may mediate any potential sex difference in fetal myostatin concentration.
In view of the above discussed knowledge gaps, we sought to assess whether cord blood myostatin concentrations are affected by GDM and fetal sex, the associations with fetal growth and fetal growth factors, and the role of testosterone in mediating potential sex difference in cord blood myostatin.
Methods
Study design, participants and specimens
We recruited pregnant women at the last prenatal visit before delivery at Mount Sinai Hospital in Toronto between January 2019 and February 2020. Participants met the following inclusion criteria: (1) maternal age 20-45 years; (2) single term pregnancy (gestational age ≥37 weeks); (3) Caucasian or Asian (to limit the potential confounding effects of ethnicity). Exclusion criteria were: (1) in-vitro fertilization; (2) any known birth defects or congenital anomalies; (3) family history of type 1 diabetes; (4) any major pre-pregnancy illnesses (e.g., type 1 or 2 or unknown diabetes, hypertension); (5) preeclampsia or other severe pregnancy complications. The study was approved by the Research Ethics Board of Mount Sinai Hospital (approval number: 18-0224-E). Written informed consent was obtained from all study participants.
All pregnant women underwent a 1-hour 50 g oral glucose challenge test between 24 and 28 weeks of gestation, and those who failed the test (1-h plasma glucose ≥7.8 mmol/L) were required to undertake a 75 g 2-h oral glucose tolerance test. GDM was diagnosed if any plasma glucose value was abnormal (fasting ≥5.3 mmol/L, 1-h ≥10.6 mmol/L, 2-h ≥9.0 mmol/L) according to the Canadian Diabetes Association’s diagnostic criteria (24). Mothers who had normal values in the 50 g oral glucose challenge test were considered to be euglycemic.
A total of 44 GDM and 66 euglycemic women were recruited. Data on sociodemographic characteristics and clinical risk factors were obtained by in-person interviews and reviews of medical charts using a standardized questionnaire by a trained research staff. Cord blood samples were collected by a trained research staff in the Research Centre for Women’s and Infants’ Health BioBank at Mount Sinai Hospital immediately following birth, kept on ice, and centrifuged within 2 hours after the specimen collection. Serum (without anti-coagulant) and EDTA plasma samples were stored in multiple aliquots at -80°C until assays.
Biochemical assays
In our research lab (Dr Lye), cord plasma myostatin (pg/mL) was measured by an enzyme-linked immunosorbent assay (ELISA) kit (Cat# DGDF80, R & D Systems, Minneapolis, USA). Cord plasma IGF-2 (ng/mL) was measured by an ELISA kit (Cat# 22-IG2HU-E01, ALPCO Diagnostics, Salem, USA). Plasma proinsulin (pmol/L) was measured by an ELISA kit (Cat# 80-PINHUT-CH01, ALPCO Diagnostics, Salem, USA). In the clinical biochemistry laboratory of Mount Sinai Hospital, cord serum glucose (in mmol/L, 1 mmol/L=18 mg/dL) was determined by an enzymatic (hexokinase) method (Roche Diagnostics, Switzerland), serum insulin (in μU/mL, 1 μU/mL=6 pmol/L) and C-peptide (in ng/mL, 1 ng/mL= 333 pmol/L) were determined by chemiluminescence immunoassays (Roche Diagnostics, Switzerland), serum IGF-1 (ng/mL) was determined by a chemiluminescent immunoassay (Liaison XL, DiaSorin, Italy). Cord serum testosterone (nmol/L) was measured by a chemiluminescent immunoassay, and cross reactivity was 18% with 11-β-hydroxy-testosterone, less than 0.16% with estradiol and progesterone, and less than 6% for other testosterone-like hormones according to the manufacturer’s instructions (Roche Diagnostics, Switzerland). The limits of detection were 0.922 pg/mL for myostatin, 0.11 mmol/L for glucose, 0.2 mU/L for insulin, 0.01 ng/mL for C-peptide, 0.455 pg/mL for proinsulin, 10 ng/mL for IGF-1, 0.02 ng/mL for IGF-2, and 0.087 nmol/L for testosterone, respectively. Intra- and inter-assay coefficients of variation were in the ranges of 1.8-6.0% for myostatin, 0.5-1.3% for glucose, 0.8-4.9% for insulin, 0.5-2.3% for C-peptide, 4.0-9.7% for proinsulin, 2.37-8.5% for IGF-1, 3.1-7.2% for IGF-2, and 2.1-18.1% for testosterone, respectively. All biomarkers were assayed in duplicates, and the average values were taken. The assay technicians were blinded to the clinical characteristics of study subjects.
Statistical analysis
The primary outcome was cord blood myostatin concentration. Birth weight z score was calculated according to the Canadian sex- and gestational age-specific birth weight standards (25). Mean±standard deviation (SD) or median (interquartile range) were presented for continuous variables. Frequency (percentage) was presented for categorical variables. Student’s t tests were conducted to compare continuous variables, and Chi-square tests or Fisher’s exact tests (where appropriate) were conducted to compare categorical variables between two groups. Pearson correlation coefficients were calculated to examine the correlations of cord blood myostatin with testosterone, fetal growth (birth weight z score) and fetal growth factors (insulin, C-peptide, proinsulin, IGF-1 and IGF-2). Log-transformed data were used for all cord blood biomarkers in t tests, correlation and regression analyses. Generalized linear regression was used to examine the determinants of cord blood myostatin. GDM status and fetal sex were the primary exposures of interest. Other covariates included maternal age, pre-pregnancy BMI (calculated from self-reported height and weight, kg/m2), ethnicity (Caucasian/Asian), education (university, yes/no), family history of type 2 diabetes (yes/no), smoking before pregnancy (yes/no), primiparity (yes/no), cesarean section (yes/no) and gestational age at delivery. Only a few mothers reported smoking (n=1) or alcohol drinking (n=2) during pregnancy, and thus not considered in data analyses. Covariates with P>0.2 that did not affect the comparisons were excluded in the parsimonious final regression models to obtain more stable effect estimates. In the presence of sex difference in cord blood myostatin concentrations, we examined the mediation effect of testosterone using the product (“Baron and Kenney”) method (26). P<0.025 was considered statistically significant in testing the primary hypothesis on the difference in cord blood myostatin concentrations by GDM status and fetal sex (Bonferroni correction for 2 tests). With Bonferroni correction for multiple tests, P<0.025 was considered statistically significant in examining the primary correlation of interest between cord blood myostatin and testosterone in sex-specific analyses. P<0.05 was considered statistically significant in other exploratory analyses. All data analyses were conducted in R Studio (Version 1.4.1717).
Results
Compared with male vs. female newborns, the mothers were more likely to be Caucasian and had higher gestational weight gain (mean ± SD: 17.4 ± 7.2 vs. 13.2 ± 6.8 kg) (Table 1). As expected, males were heavier than females at birth (3458 ± 363 vs. 3239 ± 358 g). There were no significant differences in maternal age, pre-pregnancy BMI, GDM, primiparity, family history of type 2 diabetes, smoking before pregnancy, caesarean section and gestational age at delivery between male and female newborns.
Comparing GDM vs. euglycemic pregnancies, there were no significant differences in maternal age, education, primiparity, family history of type 2 diabetes, smoking before pregnancy, caesarean section, infant sex and gestational age (Table 2). Mothers with GDM had higher pre-pregnancy BMI (25.5 vs. 22.3 kg/m2) but lower gestational weight gain (12.9 vs. 16.8 kg), and were less likely to be Caucasian (39% vs. 65%). Unexpectedly, the newborns of GDM mothers had lower average birth weight than those of euglycemic mothers (3245 ± 369 vs. 3410 ± 365 g), partly due to more Asians (61% vs. 35%) who had lower birth weights than Caucasians (3250 ± 399 vs. 3422 ± 334 g). In Caucasian subjects (n=60), the newborns of GDM mothers had similar average birth weight vs. those of euglycemic mothers (3423 ± 332 vs. 3421 ± 339, P=0.99). In Asian subjects, the newborns of GDM mothers had lower average birth weight vs. those of euglycemic mothers (3133 ± 351 vs. 3387 ± 416, P=0.026).
Adjusting for maternal and infant characteristics, male newborns had significantly higher cord blood concentrations of myostatin (mean: 6.07 vs. 5.29 ng/mL, adjusted P=0.006) and testosterone (9.53 vs. 7.14 nmol/L, adjusted P=0.017) (Table 3 and Figure 1). As expected, female newborns tended to have higher cord blood concentrations of insulin (102.8 vs. 62.9 pmol/L, adjusted P=0.074) and proinsulin (23.3 vs. 17.7 pmol/L, adjusted P=0.066). Cord blood glucose/insulin ratio - a surrogate indicator of fetal insulin sensitivity (14, 27), was higher in males vs. females (7.87 vs. 5.46, adjusted P=0.048). Cord blood myostatin and testosterone concentrations were similar in Caucasian vs. Asian newborns (all P>0.5, data not shown). There were no significant interactions between fetal sex and GDM status, or between fetal sex and ethnicity in relation to cord blood myostatin or testosterone (all P>0.1).
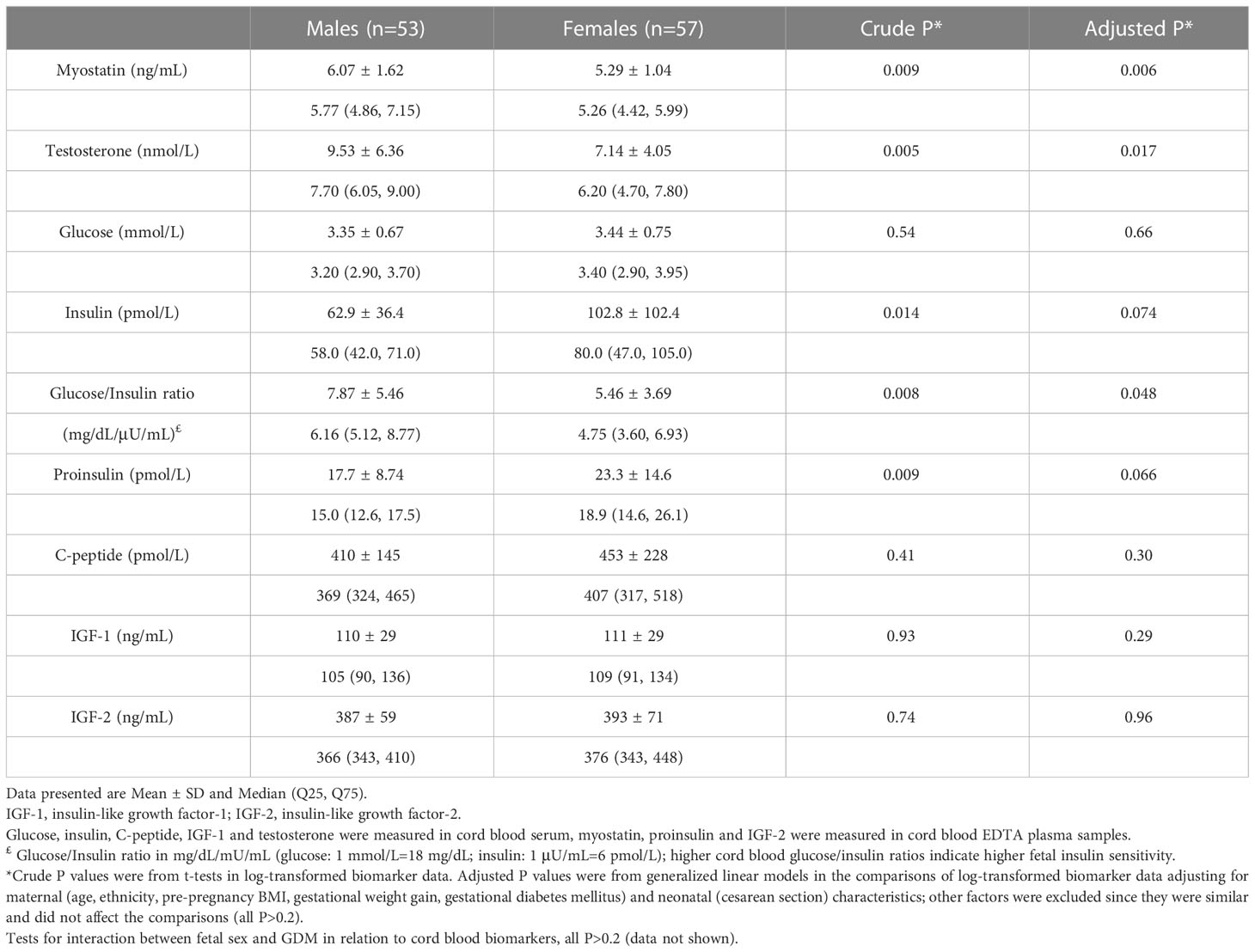
Table 3 Cord blood concentrations of myostatin, testosterone and fetal growth factors comparing male vs. female newborns.
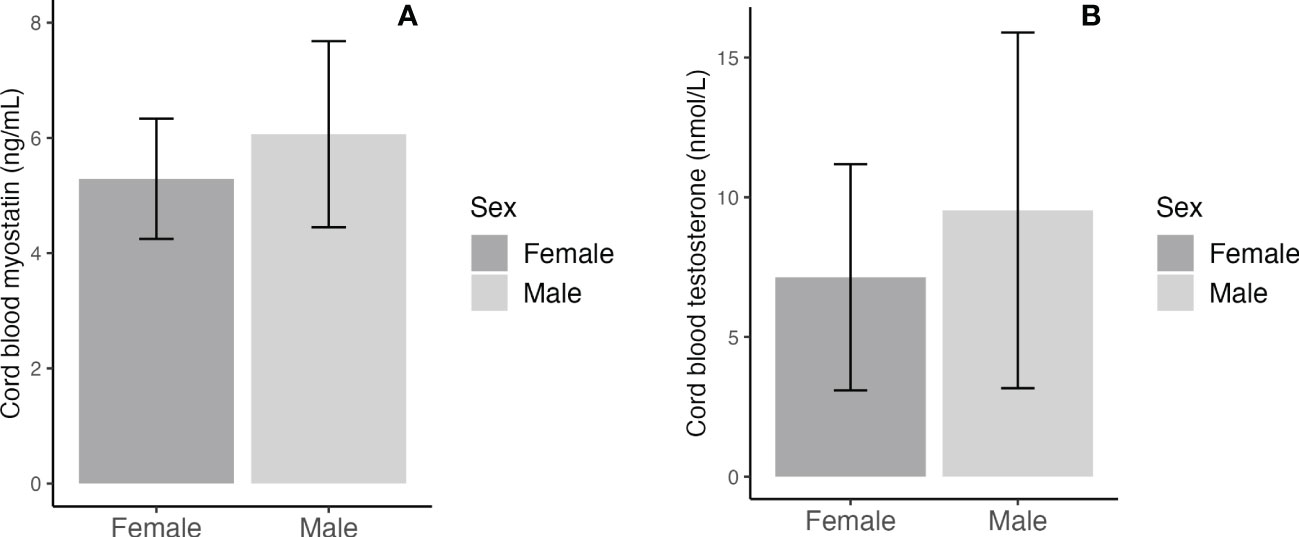
Figure 1 Sex differences in cord blood concentrations of myostatin (A) and testosterone (B); the error bars represent the mean and 95% confidence intervals.
Cord blood myostatin concentrations were similar in GDM vs. euglycemic pregnancies (5.50 vs. 5.77 ng/mL, adjusted P=0.28, Table 4). There were no significant differences in cord blood concentrations of testosterone, IGF-1 and IGF-2 comparing the newborns of GDM vs. euglycemic mothers. Similarly, in male newborns, there were no significant differences in cord blood concentrations of myostatin (5.94 vs. 6.13 ng/mL, adjusted P=0.57) and testosterone (9.74 vs. 9.43 nmol/L, adjusted P=0.56) comparing GDM vs. euglycemic pregnancies. The newborns of GDM mothers tended to have higher insulin (120.4 vs. 60.1 pmol/L, adjusted P=0.07) and proinsulin (24.5 vs. 18.0 pmol/L, adjusted P=0.14) concentrations, although the differences did not reach statistical significance. Similarly, cord blood concentrations tended to be higher for insulin (90.5 vs. 52.2 pmol/L, P=0.026) and C-peptide (462 vs. 385 pmol/L, P=0.08) comparing GDM and euglycemic pregnancies in male newborns,
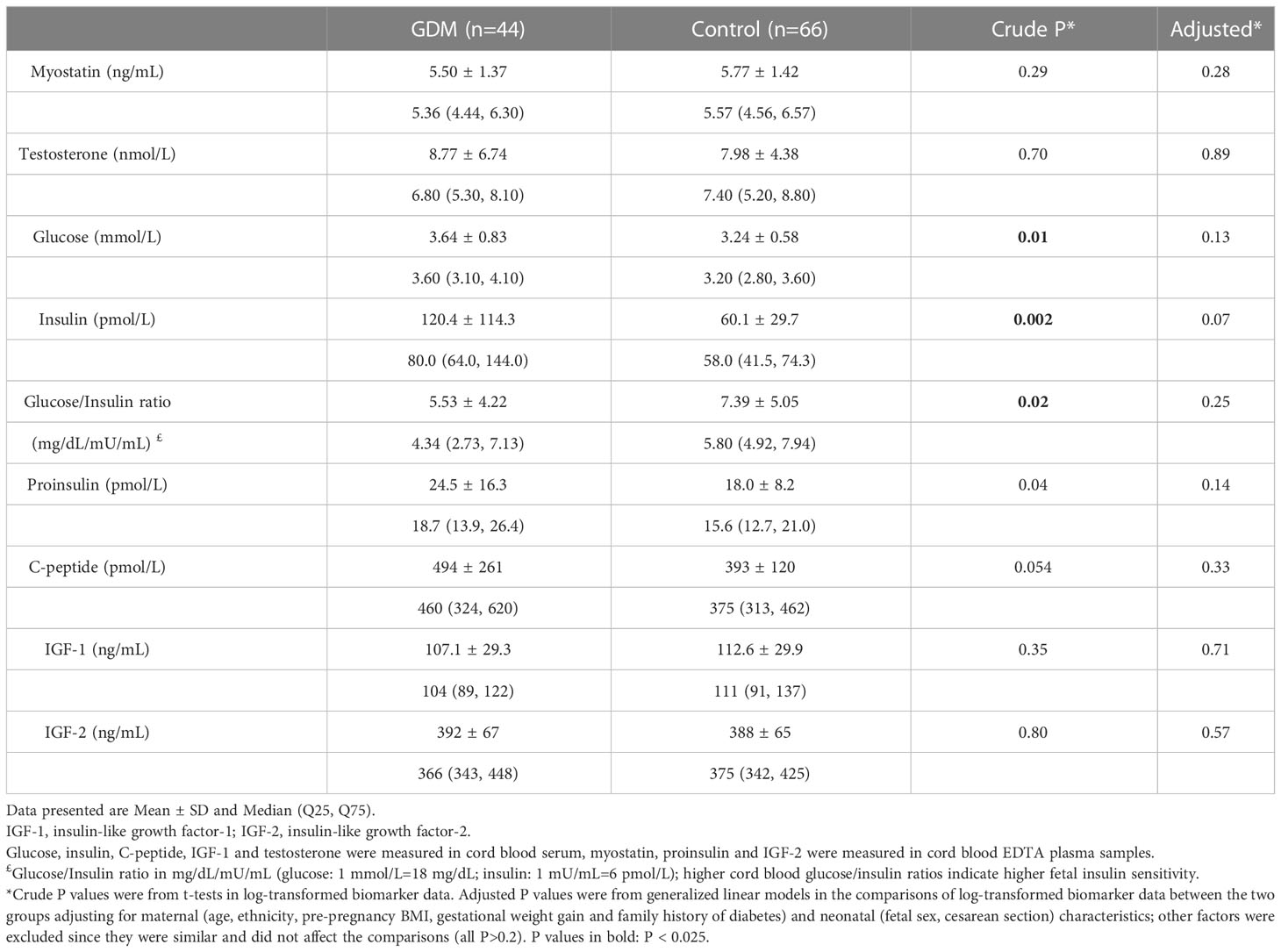
Table 4 Cord blood concentrations of myostatin, testosterone and fetal growth factors comparing GDM vs. euglycemic pregnancies.
Adjusting for gestational age at blood sampling, cord blood myostatin was positively correlated with testosterone in males (r=0.56, P <0.001), but not in females (r=-0.076, P=0.58) (Fisher’s z test for difference in correlation coefficients, P<0.001) (Table 5 and Figure 2). In the total study sample, cord blood myostatin was negatively correlated with IGF-2 (r=-0.23, P=0.02), but not correlated with birth weight (z score), IGF-1, insulin, proinsulin or C-peptide. Cord blood myostatin was not correlated with glucose/insulin ratio in males (P=0.46) or females (P=0.35).
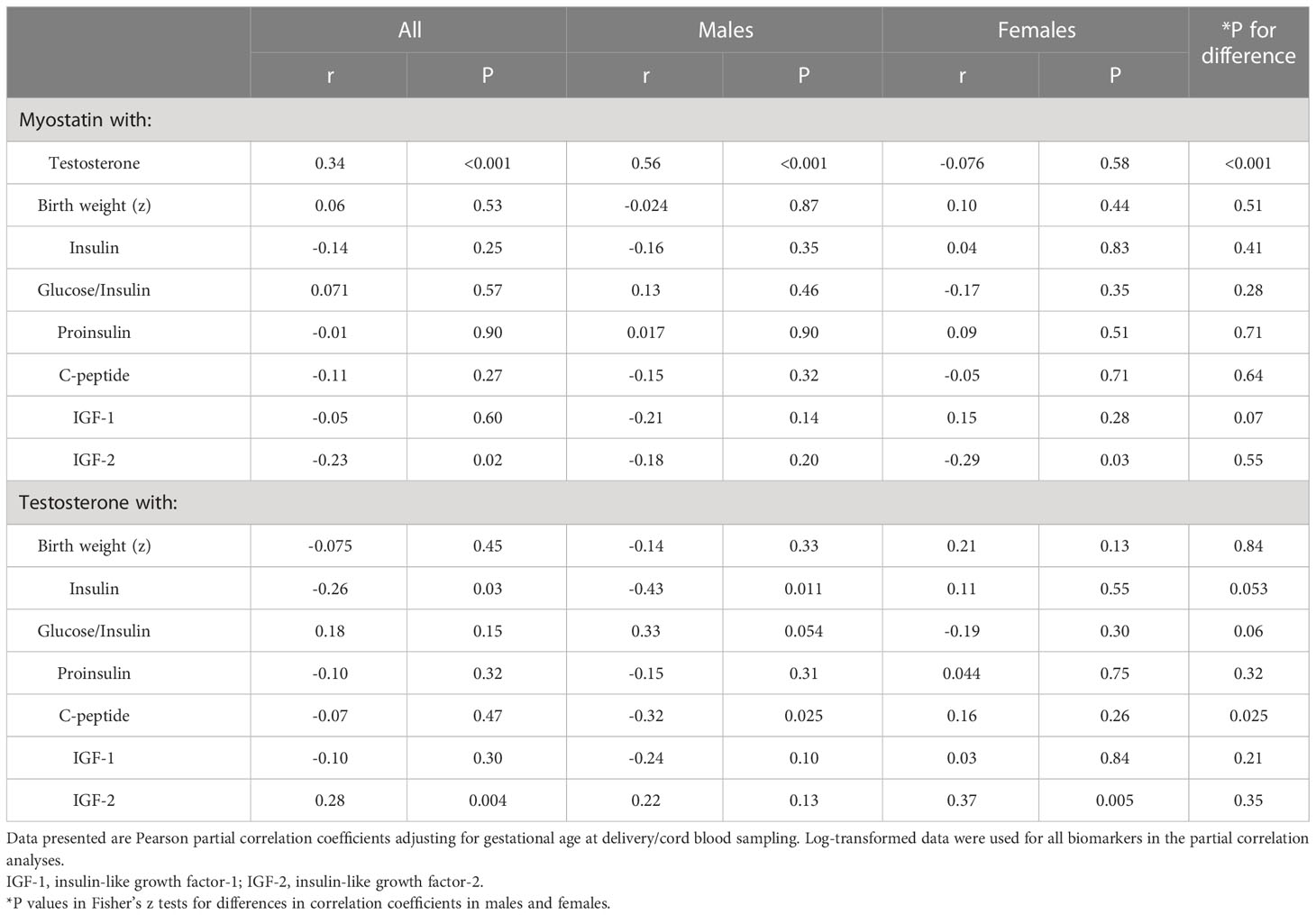
Table 5 Correlations of cord blood myostatin with testosterone, birth weight (z), glucose/insulin ratio and fetal growth factors.
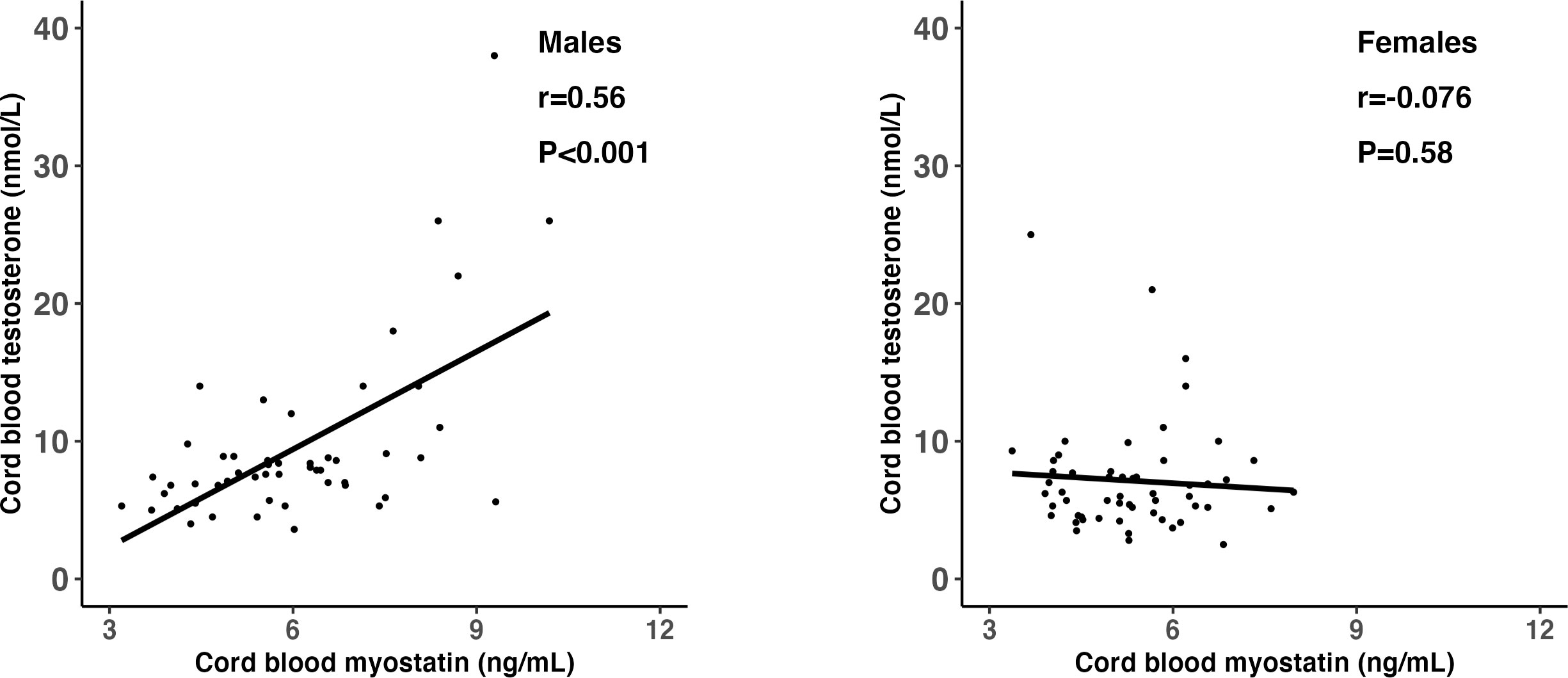
Figure 2 Scatter plots illustrating the differential correlations (r) between cord blood myostatin and testosterone in males and females; Fisher’s Z test for difference in r (correlation coefficient): P<0.001.
Adjusting for gestational age at blood sampling, cord blood testosterone was positively correlated with IGF-2 (r=0.28, P=0.004), but not correlated with IGF-1 (r=-0.10, P=0.30) or birth weight (r=-0.075, P=0.45) in the total study sample (Table 5). Cord blood testosterone was negatively correlated with insulin in males (r=-0.43, P=0.011), but not in females (r=0.11, P=0.55) (Fisher’s z test for difference in correlation coefficients, P=0.053). Similarly, testosterone was negatively correlated with C-peptide (r=-0.32, P=0.025) in males, but not in females (r=0.16, P=0.26) (Fisher’s z test for difference in correlation coefficients, P=0.025).
There were no significant differences in the correlations of cord blood myostatin and testosterone with birth weight and fetal growth factors by GDM status or ethnicity (data not shown).
In the total study sample, birth weight z score was positively correlated with cord blood proinsulin (r=0.24, P=0.01) and IGF-1 (r=0.31, P=0.001), and tended to be positively correlated with C-peptide (r=0.17, P=0.08), but not correlated with IGF-2 (r=0.01, P=0.94).
Overall, male sex was associated with a 0.60 increase in cord blood myostatin concentration z score (P=0.002). Mediation analysis demonstrated that cord blood testosterone could explain a 0.18 increase in cord blood myostatin z score (30.0%, P=0.039) in males vs. females (Table 6). Cord blood testosterone or myostatin could not explain the sex difference in cord blood glucose/insulin ratio or birth weight (all P>0.1 for mediation effect, results not shown).
Discussion
Main findings
Our study is the first to demonstrate that GDM does not affect cord blood myostatin concentration, whereas fetal sex does. Cord blood myostatin concentrations were significantly higher in males vs. females. Approximately 30% of the sex difference in cord blood myostatin concentrations can be explained by testosterone. Interestingly, cord blood testosterone and myostatin was positively correlated in males only, suggesting a male-specific androgen up-regulation of myostatin secretion in early life in humans.
GDM and cord blood myostatin
Cord blood myostatin concentrations were similar in GDM vs. controls. Skeletal muscle is the dominant source of circulating myostatin (1); skeletal muscle-specific expression of myostatin is about 50-100 fold higher than adipose tissue-specific expression (28). Whether GDM affects skeletal muscle mass remains controversial; similar or lower lean mass has been reported in the neonates of GDM vs. controls (29, 30). Although GDM has been associated with increased fat mass in the offspring (29, 30), myostatin expression in both visceral and subcutaneous fat was similar in obese vs. lean subjects (11), and circulating myostatin concentration does not appear to be correlated with fat mass (12).
Cord blood myostatin, fetal sex and testosterone
We observed significantly higher cord blood myostatin concentrations in males vs. females. In contrast, a smaller study (n=83) reported no significant difference in cord blood myostatin concentrations in males vs. females (18). The reasons for the discrepant findings may be partly due to the differences in sample size and detection method (Sandwich ELISA in our study vs. competitive ELISA kit in their study). Our study is consistent with an adult study reporting higher myostatin concentrations in males vs. females (4.3 ng/mL vs. 3.3 ng/mL) using the same ELISA kit as in our study (R&D Systems) (12).
Interestingly, cord blood myostatin was positively correlated with testosterone in males but not in females. Testosterone is an anabolic hormone promoting protein synthesis and skeletal muscle growth (19). In contrast, myostatin inhibits skeletal muscle growth (1, 2). Our data support the hypothesis that testosterone may up-regulate myostatin (and thus may counteract the effect of testosterone) in males. This observation is in line with a study in male mice reporting that the inhibition of testosterone production or androgen receptor signaling could down-regulate myostatin gene expression and protein synthesis in androgen responsive muscles (31). An adult study showed that both testosterone and myostatin concentrations were higher in young vs. old men, and testosterone treatment resulted in higher myostatin concentrations (23), indicating that testosterone may up-regulate myostatin secretion in men. Our data suggest that testosterone may up-regulate fetal myostatin secretion in males, and may partly mediate the higher fetal (cord blood) myostatin concentrations in males in humans.
Myostatin, testosterone and fetal insulin sensitivity
Our study confirmed that GDM and female sex were associated with lower fetal insulin sensitivity as indicated by lower cord blood glucose/insulin ratios and higher insulin and proinsulin concentrations (14, 15). Neither myostatin nor testosterone could explain the differences in glucose/insulin ratios by fetal sex or GDM in mediation analyses, suggesting neither may explain such differences. GDM was not associated with cord blood testosterone, consistent with a previous study (32). Testosterone tended to be positively correlated with fetal insulin sensitivity (glucose/insulin) in males (P=0.054). This is in line with a previous study reporting that testosterone replacement therapy improved insulin resistance in adult men (22).
Myostatin and testosterone in relation to fetal growth and fetal growth factors
We observed a negative correlation between cord blood myostatin and IGF-2, but no correlation with IGF-1 or birth weight. Myostatin appears to be regulated by growth hormone in hypophysectomised mice (33) and hypopituitary adults (34), suggesting myostatin may play a role in fetal growth. A previous study reported an inverse correlation between cord blood myostatin and birth weight in 83 newborns (r=-0.40, P=0.001) (18). The reasons for the different findings may be partly due to the differences in study population and detection methods (Sandwich vs. competitive ELISA). Their study included 23 large-for-gestational-age (LGA, birth weight z score >2) and 60 appropriate-for-gestational-age infants (birth weight z score from -1 to 1) (18), and the larger differences in birth weight may render a greater power to identify a significant correlation between cord blood myostatin and birth weight. None of our newborns could be identified as LGA if we used the same definition as in their study. On the other hand, we did observe a negative correlation between cord blood myostatin and IGF-2, suggesting a possible negative effect on fetal growth. IGF-2 plays a pivotal role in fetal growth (35, 36). Our observation is in line with two animal studies reporting that IGF-2 expression was greater in mice with myostatin mutation (37), and IGF-2 expression was inhibited in myoblast cultures with treatment of recombinant myostatin (38). Overall, our data are somewhat uncertain concerning the role of myostatin in fetal growth. We could not rule out the possibility of a false negative finding, and larger studies are warranted to clarify the role of myostatin in fetal growth.
Testosterone was positively correlated with IGF-2, but not correlated to IGF-1 or birth weight. IGF-2 is a fetal growth factor important for early embryonic fetal growth, and its correlation with birth weight tends to be much weaker than IGF-1 (35, 36). We failed to detect a positive correlation between IGF-2 and birth weight, probably due to the relative small sample size. The lack of correlation between cord blood testosterone and birth weight is consistent with the results in a previous study (32). As expected, birth weight was positively correlated with cord blood proinsulin, C-peptide and IGF-1, consistent with the findings in previous studies (39, 40).
Interestingly, we observed a negative correlation between cord blood testosterone and insulin or C-peptide in males but not in females. More studies from other independent cohorts are warranted to confirm this novel observation suggesting that testosterone may play a sex dimorphic role in insulin secretion during fetal life in humans.
Limitations
There are some study limitations. Firstly, the modest sample size allowed for the detection of modest/large differences, and was underpowered to detect small differences. With the study sample size (44 GDM, 66 controls; 53 males, 57 females) with alpha error at 0.025, we had a power of >=91% to detect a 0.7 SD or greater difference in cord blood myostatin concentrations between GDM and controls, or between males and females. The study power was >78% to detect an absolute correlation coefficient of 0.4 or greater in sex-specific analyses with alpha error at 0.025. Secondly, cord serum testosterone was measured by chemiluminescence immunoassay rather than mass spectrometry - the golden standard (much more costly) method. Due to cross reactivity with testosterone-like molecules, the observed cord serum testosterone concentrations could have been inflated to some extent. However, such noise random variations would only tend to decrease the probability of detecting a significant association. Lastly, the observational nature of the study precluded the possibility of conclusive causal inference.
In conclusion, GDM does not affect cord blood myostatin concentration, but fetal sex does. The higher myostatin concentrations in males may be partly mediated by testosterone. The male-only positive correlation between cord blood testosterone and myostatin suggests a male-specific role of androgen in up-regulating fetal myostatin secretion in humans.
Data availability statement
The datasets presented in this article are not readily available because Access to deidentified research data must be approved by the research ethics board. Requests to access the datasets should be directed to emNsdW9AbHVuZW5mZWxkLmNh.
Ethics statement
The studies involving human participants were reviewed and approved by Research Ethics Board of Mount Sinai Hospital University of Toronto. The patients/participants provided their written informed consent to participate in this study.
Author contributions
RH, LB, ZP, KM, JK, SL and Z-CL conceived the study. RH, MK, KM, JK, SL and Z-CL contributed to the acquisition of research data. RH conducted the literature review, data analysis and drafted the article. All authors contributed in revising the article critically for important intellectual content, and approved the final version for publication. Z-CL is the guarantor of this work, has full access to all the data in the study and takes responsibility for the integrity of the data and the accuracy of the data analysis. All authors approved the submitted version.
Funding
Supported by research grants from the Canadian Institutes of Health Research (grant # 158616, 155955 and FDN-143262) and the Ministry of Science and Technology of China (grant # 2019YFA0802501).
Conflict of interest
The authors declare that the research was conducted in the absence of any commercial or financial relationships that could be construed as a potential conflict of interest.
Publisher’s note
All claims expressed in this article are solely those of the authors and do not necessarily represent those of their affiliated organizations, or those of the publisher, the editors and the reviewers. Any product that may be evaluated in this article, or claim that may be made by its manufacturer, is not guaranteed or endorsed by the publisher.
Abbreviations
BMI, body mass index; GDM, Gestational diabetes mellitus; IGF-1, insulin-like growth factor-1; IGF-2, insulin-like growth factor-2.
References
1. McPherron AC, Lawler AM, Lee SJ. Regulation of skeletal muscle mass in mice by a new TGF-beta superfamily member. Nature (1997) 387(6628):83–90. doi: 10.1038/387083a0
2. Thomas M, Langley B, Berry C, Sharma M, Kirk S, Bass J, et al. Myostatin, a negative regulator of muscle growth, functions by inhibiting myoblast proliferation. J Biol Chem (2000) 275(51):40235–43. doi: 10.1074/jbc.M004356200
3. McPherron AC, Lee SJ. Suppression of body fat accumulation in myostatin-deficient mice. J Clin Invest (2002) 109(5):595–601. doi: 10.1172/jci13562
4. Guo T, Jou W, Chanturiya T, Portas J, Gavrilova O, McPherron AC. Myostatin inhibition in muscle, but not adipose tissue, decreases fat mass and improves insulin sensitivity. PloS One (2009) 4(3):e4937. doi: 10.1371/journal.pone.0004937
5. Bernardo BL, Wachtmann TS, Cosgrove PG, Kuhn M, Opsahl AC, Judkins KM, et al. Postnatal PPARdelta activation and myostatin inhibition exert distinct yet complimentary effects on the metabolic profile of obese insulin-resistant mice. PloS One (2010) 5(6):e11307. doi: 10.1371/journal.pone.0011307
6. Burgess K, Xu T, Brown R, Han B, Welle S. Effect of myostatin depletion on weight gain, hyperglycemia, and hepatic steatosis during five months of high-fat feeding in mice. PloS One (2011) 6(2):e17090. doi: 10.1371/journal.pone.0017090
7. Wilkes JJ, Lloyd DJ, Gekakis N. Loss-of-function mutation in myostatin reduces tumor necrosis factor alpha production and protects liver against obesity-induced insulin resistance. Diabetes (2009) 58(5):1133–43. doi: 10.2337/db08-0245
8. Coleman SK, Rebalka IA, D'Souza DM, Deodhare N, Desjardins EM, Hawke TJ. Myostatin inhibition therapy for insulin-deficient type 1 diabetes. Sci Rep (2016), 6:32495. doi: 10.1038/srep32495
9. Hittel DS, Berggren JR, Shearer J, Boyle K, Houmard JA. Increased secretion and expression of myostatin in skeletal muscle from extremely obese women. Diabetes (2009) 58(1):30–8. doi: 10.2337/db08-0943
10. Chen MJ, Han DS, Yang JH, Yang YS, Ho HN, Yang WS. Myostatin and its association with abdominal obesity, androgen and follistatin levels in women with polycystic ovary syndrome. Hum Reprod (2012) 27(8):2476–83. doi: 10.1093/humrep/des209
11. Amor M, Itariu BK, Moreno-Viedma V, Keindl M, Jürets A, Prager G, et al. Serum myostatin is upregulated in obesity and correlates with insulin resistance in humans. Exp Clin Endocrinol Diabetes (2019) 127(8):550–6. doi: 10.1055/a-0641-5546
12. Tanaka M, Masuda S, Yamakage H, Inoue T, Ohue-Kitano R, Yokota S, et al. Role of serum myostatin in the association between hyperinsulinemia and muscle atrophy in Japanese obese patients. Diabetes Res Clin Pract (2018) 142:195–202. doi: 10.1016/j.diabres.2018.05.041
13. Sacks DA, Hadden DR, Maresh M, Deerochanawong C, Dyer AR, Metzger BE, et al. Frequency of gestational diabetes mellitus at collaborating centers based on IADPSG consensus panel-recommended criteria: The hyperglycemia and adverse pregnancy outcome (HAPO) study. Diabetes Care (2012) 35(3):526–8. doi: 10.2337/dc11-1641
14. Luo ZC, Delvin E, Fraser WD, Audibert F, Deal CI, Julien P, et al. Maternal glucose tolerance in pregnancy affects fetal insulin sensitivity. Diabetes Care (2010) 33(9):2055–61. doi: 10.2337/dc10-0819
15. Shields BM, Knight B, Hopper H, Hill A, Powell RJ, Hattersley AT, et al. Measurement of cord insulin and insulin-related peptides suggests that girls are more insulin resistant than boys at birth. Diabetes Care (2007) 30(10):2661–6. doi: 10.2337/dc06-1501
16. Ibáñez L, Sebastiani G, Lopez-Bermejo A, Díaz M, Gómez-Roig MD, de Zegher F. Gender specificity of body adiposity and circulating adiponectin, visfatin, insulin, and insulin growth factor-I at term birth: relation to prenatal growth. J Clin Endocrinol Metab (2008) 93(7):2774–8. doi: 10.1210/jc.2008-0526
17. Wilkin TJ, Murphy MJ. The gender insulin hypothesis: why girls are born lighter than boys, and the implications for insulin resistance. Int J Obes (Lond) (2006) 30(7):1056–61. doi: 10.1038/sj.ijo.0803317
18. de Zegher F, Pérez-Cruz M, Díaz M, Gómez-Roig MD, López-Bermejo A, Ibáñez L. Less myostatin and more lean mass in large-born infants from nondiabetic mothers. J Clin Endocrinol Metab (2014) 99(11):E2367–71. doi: 10.1210/jc.2014-2334
19. Herbst KL, Bhasin S. Testosterone action on skeletal muscle. Curr Opin Clin Nutr Metab Care (2004) 7(3):271–7. doi: 10.1097/00075197-200405000-00006
20. Davis SR, Wahlin-Jacobsen S. Testosterone in women–the clinical significance. Lancet Diabetes Endocrinol (2015) 3(12):980–92. doi: 10.1016/s2213-8587(15)00284-3
21. Barry JA, Hardiman PJ, Siddiqui MR, Thomas M. Meta-analysis of sex difference in testosterone levels in umbilical cord blood. J Obstet Gynaecol (2011) 31(8):697–702. doi: 10.3109/01443615.2011.614971
22. Rao PM, Kelly DM, Jones TH. Testosterone and insulin resistance in the metabolic syndrome and T2DM in men. Nat Rev Endocrinol (2013) 9(8):479–93. doi: 10.1038/nrendo.2013.122
23. Lakshman KM, Bhasin S, Corcoran C, Collins-Racie LA, Tchistiakova L, Forlow SB, et al. Measurement of myostatin concentrations in human serum: Circulating concentrations in young and older men and effects of testosterone administration. Mol Cell Endocrinol (2009) 302(1):26–32. doi: 10.1016/j.mce.2008.12.019
24. Berger H, Gagnon R, Sermer M, Basso M, Bos H, Brown RN, et al. Diabetes in pregnancy. J Obstet Gynaecol Can (2016) 38(7):667–679.e1. doi: 10.1016/j.jogc.2016.04.002
25. Kramer M, Platt R, Wen S, Joseph K, Allen A, Abrahamowicz M, et al. Fetal/Infant health study group of the Canadian perinatal surveillance system a new and improved population-based Canadian reference for birth weight for gestational age. Pediatrics (2001) 108(2):E35. doi: 10.1542/peds.108.2.e35
26. VanderWeele TJ. Mediation analysis: A practitioner's guide. Annu Rev Public Health (2016) 37:17–32. doi: 10.1146/annurev-publhealth-032315-021402
27. Setia S, Sridhar MG, Bhat V, Chaturvedula L, Vinayagamoorti R, John M. Insulin sensitivity and insulin secretion at birth in intrauterine growth retarded infants. Pathology (2006) 38(3):236–8. doi: 10.1080/00313020600696256
28. Allen DL, Cleary AS, Speaker KJ, Lindsay SF, Uyenishi J, Reed JM, et al. Myostatin, activin receptor IIb, and follistatin-like-3 gene expression are altered in adipose tissue and skeletal muscle of obese mice. Am J Physiol Endocrinol Metab (2008) 294(5):E918–27. doi: 10.1152/ajpendo.00798.2007
29. Okereke NC, Uvena-Celebrezze J, Hutson-Presley L, Amini SB, Catalano PM. The effect of gender and gestational diabetes mellitus on cord leptin concentration. Am J Obstet Gynecol (2002) 187(3):798–803. doi: 10.1067/mob.2002.125887
30. Durnwald C, Huston-Presley L, Amini S, Catalano P. Evaluation of body composition of large-for-gestational-age infants of women with gestational diabetes mellitus compared with women with normal glucose tolerance levels. Am J Obstet Gynecol (2004) 191(3):804–8. doi: 10.1016/j.ajog.2003.11.033
31. Dubois V, Laurent MR, Sinnesael M, Cielen N, Helsen C, Clinckemalie L, et al. A satellite cell-specific knockout of the androgen receptor reveals myostatin as a direct androgen target in skeletal muscle. FASEB J (2014) 28(7):2979–94. doi: 10.1096/fj.14-249748
32. Gemer O, Sevillia J, Zalis J, Segal S. Umbilical cord androgens in infants of diabetic mothers. Arch Gynecol Obstet (1997) 259(3):139–41. doi: 10.1007/bf02505322
33. Oldham JM, Osepchook CC, Jeanplong F, Falconer SJ, Matthews KG, Conaglen JV, et al. The decrease in mature myostatin protein in male skeletal muscle is developmentally regulated by growth hormone. J Physiol (2009) 587(3):669–77. doi: 10.1113/jphysiol.2008.161521
34. Liu W, Thomas SG, Asa SL, Gonzalez-Cadavid N, Bhasin S, Ezzat S. Myostatin is a skeletal muscle target of growth hormone anabolic action. J Clin Endocrinol Metab (2003) 88(11):5490–6. doi: 10.1210/jc.2003-030497
35. DeChiara TM, Efstratiadis A, Robertson EJ. A growth-deficiency phenotype in heterozygous mice carrying an insulin-like growth factor II gene disrupted by targeting. Nature (1990) 345(6270):78–80. doi: 10.1038/345078a0
36. Ong K, Kratzsch J, Kiess W, Costello M, Scott C, Dunger D. Size at birth and cord blood levels of insulin, insulin-like growth factor I (IGF-I), IGF-II, IGF-binding protein-1 (IGFBP-1), IGFBP-3, and the soluble IGF-II/mannose-6-phosphate receptor in term human infants. the ALSPAC study team. Avon longitudinal study of pregnancy and childhood. J Clin Endocrinol Metab (2000) 85(11):4266–9. doi: 10.1210/jcem.85.11.6998
37. Clark DL, Clark DI, Hogan EK, Kroscher KA, Dilger AC. Elevated insulin-like growth factor 2 expression may contribute to the hypermuscular phenotype of myostatin null mice. Growth Horm IGF Res (2015) 25(5):207–18. doi: 10.1016/j.ghir.2015.06.007
38. Miyake M, Hayashi S, Taketa Y, Iwasaki S, Watanabe K, Ohwada S, et al. Myostatin down-regulates the IGF-2 expression via ALK-smad signaling during myogenesis in cattle. Anim Sci J (2010) 81(2):223–9. doi: 10.1111/j.1740-0929.2009.00725.x
39. Luo ZC, Nuyt AM, Delvin E, Audibert F, Girard I, Shatenstein B, et al. Maternal and fetal IGF-I and IGF-II levels, fetal growth, and gestational diabetes. J Clin Endocrinol Metab (2012) 97(5):1720–8. doi: 10.1210/jc.2011-3296
Keywords: gestational diabetes mellitus, myostatin, testosterone, insulin-like growth factor, sex difference
Citation: Huang R, Kibschull M, Briollais L, Pausova Z, Murphy K, Kingdom J, Lye S and Luo Z-C (2023) Cord blood myostatin concentrations by gestational diabetes mellitus and fetal sex. Front. Endocrinol. 14:1018779. doi: 10.3389/fendo.2023.1018779
Received: 13 August 2022; Accepted: 25 January 2023;
Published: 15 February 2023.
Edited by:
Jeff M. P. Holly, University of Bristol, United KingdomReviewed by:
Kaiping Yang, Western University, CanadaQiong Luo, Zhejiang University, China
Johan Verhaeghe, KU Leuven Research and Development, Belgium
Copyright © 2023 Huang, Kibschull, Briollais, Pausova, Murphy, Kingdom, Lye and Luo. This is an open-access article distributed under the terms of the Creative Commons Attribution License (CC BY). The use, distribution or reproduction in other forums is permitted, provided the original author(s) and the copyright owner(s) are credited and that the original publication in this journal is cited, in accordance with accepted academic practice. No use, distribution or reproduction is permitted which does not comply with these terms.
*Correspondence: Zhong-Cheng Luo, emNsdW9AbHVuZW5mZWxkLmNh