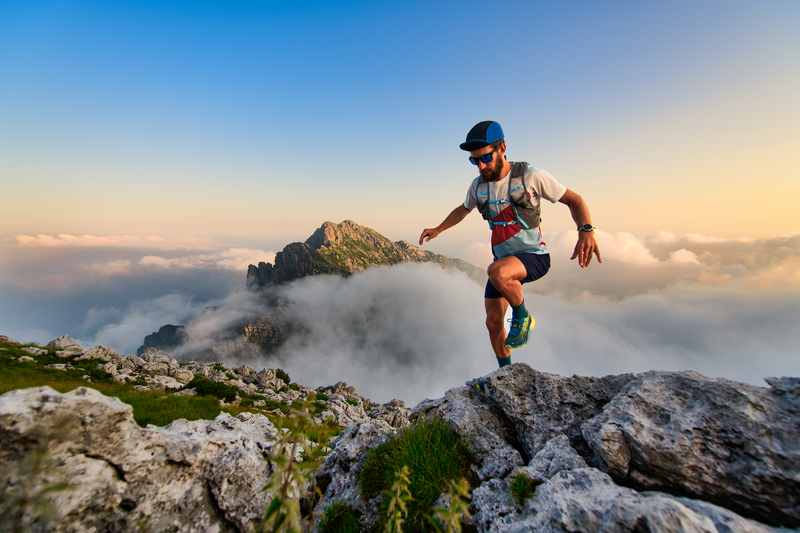
94% of researchers rate our articles as excellent or good
Learn more about the work of our research integrity team to safeguard the quality of each article we publish.
Find out more
ORIGINAL RESEARCH article
Front. Endocrinol. , 08 September 2022
Sec. Experimental Endocrinology
Volume 13 - 2022 | https://doi.org/10.3389/fendo.2022.981564
Experiments were carried out to determine whether, as with other mollusks that have been studied, the snail, Lymnaea stagnalis, can absorb, esterify and store vertebrate steroids that are present in the water. We also carried out experiments to determine whether neural tissues of the snail could be immunohistochemically stained with an antibody to human aromatase (a key enzyme that catalyzes the conversion of testosterone [T] to 17β-estradiol [E2]); and, if so, to determine the significance of such staining. Previous studies on other mollusks have reported such staining and have proposed this as decisive evidence that mollusks have the same steroid synthesis pathway as vertebrates. We found that snails absorb, esterify and retain esterified T, E2, progesterone and ethinyl-estradiol (albeit with an absorption rate about four times slower, on a weight basis, than the mussel, Mytilus edulis). We also found that not only anti-human aromatase, but also anti-human nuclear progesterone receptor (nPR) and anti-human gonadotropin-releasing hormone antibodies immunohistochemically stained snail neural cells. However, further experiments, involving gel electrophoretic separation, followed by immunostaining, of proteins extracted from the neural tissue, found at least two positively-stained bands for each antibody, none of which had masses matching the human proteins to which the antibodies had been raised. The anti-aromatase antibody even stained the 140 kDA ladder protein used as a molecular weight marker on the gels. Mass spectrometric analysis of the bands did not find any peptide sequences that corresponded to the human proteins. Our findings confirm that the presence of vertebrate-like sex steroids in molluscan tissues is not necessarily evidence of endogenous origin. The results also show that immunohistochemical studies using antibodies against human proteins are grossly non-specific and likely to have little or no value in studying steroid synthesis or activity in mollusks. Our conclusions are consistent with the fact that genes for aromatase and nPR have not been found in the genome of the snail or of any other mollusk. Our overarching conclusion, from this and our previous studies, is that the endocrinology of mollusks is not the same as that of humans or any other vertebrates and that continuing to carry out physiological and ecotoxicological studies on mollusks on the basis of this false assumption, is an unconscionable waste of resources.
Ever since the discovery of the major vertebrate sex steroids, progesterone (P), testosterone (T), and 17β-estradiol (E2), in mollusks in the late 1950s, a large body of literature has attempted to provide evidence of their role as hormones in mollusks as in vertebrates [reviewed by (1–3)]. A common line of evidence is that sex steroids are present (and, indeed, easy to measure) in tissues of a wide range of mollusks and that in some (but, it must be stressed, not all) studies, their concentrations sometimes show an association with stages of gonad development, sometimes show differences between tissues and sexes, and sometimes appear to be altered in the presence of contaminants [reviewed by (2, 4–7)]. Another line of evidence is that cells in mollusk tissues can be immunohistochemically stained with antibodies raised against mammalian proteins that are associated with sex steroid synthesis (e.g. Cytochrome P450 [CYP] 11A, CYP19A, and 17β-hydroxysteroid dehydrogenase); or steroid reception (e.g. nuclear estrogen receptor [nER]) (8–14).
In regard to the first line of evidence, it has been established, overwhelmingly, since 2000 that mollusks can readily absorb P, T, and E2 from the environment and store them and/or their metabolites for weeks to months in the form of fatty acid esters (6, 15–29). Also, ethinyl-estradiol (EE2; which is the estrogenic component of “The Pill”) has been detected in the flesh of wild-caught mollusks (30–33). It is highly improbable that the animals would have made this compound themselves as it is purely a man-made chemical. In view of such findings, and the fact that vertebrate steroids are widespread in the environment, it is legitimate to ask how much (if any at all) of the vertebrate sex steroids found in molluscan tissues are of endogenous origin.
Another problem concerning the putative role of vertebrates steroids in the mollusks is the fact that three crucial steps in the classical vertebrate steroid biosynthetic pathway - cholesterol side-chain cleavage, 17-hydroxylation, and aromatization – appear to be either absent or have very weak activity in mollusks (Supplementary Figure 1) [reviewed by (2, 3, 34–36)]. Most importantly, the protein homologues of the enzymes that catalyze the first and third of these reactions in vertebrates, CYP11A and CYP19A, respectively, as well as functional sex steroid nuclear receptors, have so far not been found in molluscan genomes (Supplementary Figure 1; Supplementary Table 1) [reviewed by (2, 3, 34–36)]. These facts are at odds, however, with the immunohistochemical evidence in the literature – which show that tissues in a range of mollusks can be stained with antibodies to human enzymes and steroid nuclear receptors.
We have recently investigated the neuronal transcriptome of the great pond snail (Lymnaea stagnalis) and confirmed the absence of several of the key protein sequences that would be required to accomplish full sex steroid biosynthesis and sex steroid receptor-mediation as found in vertebrates (Supplementary Table 2) (37). The aim of the present study was to provide further evidence for the notion that molluscan endocrinology differs from the well-characterized vertebrate endocrine system using L. stagnalis. This snail has been a widely used model organism in neuroscience, neuroendocrinology, and ecotoxicology for decades [reviewed by (38–48)], and is thus highly appropriate for such studies. To accomplish our aim, we exposed L. stagnalis to radiolabeled sex steroids (E2, T, P, and EE2) to determine if they are absorbed from water and subsequently depurated, and the rates at which these occur. Also, we performed immunohistochemistry (IHC) using commercially-available antisera generated against three mammalian proteins, CYP19A, nuclear progesterone receptor (nPR), and gonadotropin releasing hormone (GnRH), associated with vertebrate sex steroid synthesis and reception to investigate whether these yielded positive signals in the central nervous system (CNS). We also used Polyacrylamide Gel (PAGE) separation of CNS extracts, followed by Western blotting (WB), and mass spectrometry (MS) analyses to try and identify (or at least partially characterize) proteins that showed cross-reactivity with these antibodies.
17β-[2,4,6,7,16,17-3H]- E2 ([3H]-E2), [1,2,6,7-3H]-T ([3H]-T), [1,2,6,7-3H]-P ([3H]-P), and 17-[6,7-3H(N)]- EE2 ([3H]-EE2), were purchased from American Radiolabeled Chemicals, Inc. (101 ARC Dr. St. Louis, MO 63146 USA).
For IHC, WB, and MS experiments, L. stagnalis specimens were obtained from the laboratory-bred stocks of the Balaton Limnological Research Institute (Hungary). Snails were kept in large holding tanks (with a 100 individuals/tank stocking density) containing 10L oxygenated artificial snail water with low copper content at a constant temperature of 20°C ( ± 1°C) on a light:dark regime of 12 h:12 h. Specimens were fed on lettuce ad libitum three times a week. All animals used in the experiment originated from the same breeding cohort and were thus all of the same age (five months old, mature snails). All procedures were performed according to the protocols approved by the Scientific Committee of Animal Experimentation of the Balaton Limnological Research Institute (VE-I-001/01890-10/2013).
The steroid uptake and depuration experiments were carried out at the Cefas, Weymouth Laboratory in the UK. L. stagnalis specimens were supplied by Blades Biological (Kent, UK). The husbandry of the snails used in the experiments was very similar to that described above. Briefly, L. stagnalis were housed in 100 L glass aquaria (stocking density 3.6 [snails]/L; culture media was dechlorinated ambient tap water) in controlled temperature room at 20 ± 1°C, and 16 hour light: dark photoperiod with light of natural wavelength (400-700nm) and an intensity of 400-500 Lux. Specimens were fed a diet of non-organic round lettuce ad libitum.
The experiment was designed to investigate uptake, metabolism, and depuration of radioactive-labeled steroids by L. stagnalis. The methodology was based on previously published protocols (15–18) with slight modifications (e.g., freshwater instead of seawater, shorter incubation and depuration period) - the details are provided in Table 1. All procedures involved placing the animals in containers with freshwater (pre-aerated water), adding labelled steroids to the water using ethanol as a carrier (<0.01% final solvent concentration), and then taking 1 mL water samples (immediately mixed with 7 mL scintillation fluid) every two hours to measure the amount of radioactivity remaining in the water. In all cases, three sorption control vessels (radioactive steroid but no animals) were in place to determine how much radioactivity was being lost to sorption to the vessels. Scintillation counting was carried out with color quench correction on a Tricarb 2910 scintillation counter (PerkinElmer). For each steroid exposure, five animals (one from each replicate) were immediately frozen after exposure (day 0 of depuration) and the remaining five were placed in a fresh container with 4 L aerated freshwater for depuration under semi-static conditions. The animals were sampled and frozen on day 10.
The rates at which individual snails initially cleared steroids from water (i.e. clearance rates) were calculated for each steroid as follows. The percentage radiolabel remaining in the water from each treatment was first corrected for loss of radiolabel due to sorption. Label disappearance data were fitted to linear decay curves using Sigmaplot (Systat Software Inc, TW4 6JQ, London, UK.). The fitted curves were used to calculate the proportion of radiolabel that had been removed from the water between 0 and 1.5 h. This proportion (r) was used in the following equation to derive the ‘clearance rate’ of an individual snail (mL animal-1h-1) at the start of each exposure period:
where r = proportion of radiolabel removed over the first 1.5 h; V= total volume of water in the container (mL); n = number of animals in the container.
For extraction of radioactive steroids from snail tissues, the animals sampled after the 8 h of exposure (i.e. day 0) and depuration (i.e. day 10) were defrosted at room temperature, shucked, blotted dry, and weighed. The tissues were extracted with methanol and ethyl acetate using Method 2 as described previously (15). All extracts had a final volume of 5 mL and were stored at −20°C.
To separate the free, water-soluble, and esterified metabolites, a portion of each extract (800 μL) was evaporated with nitrogen gas and partitioned using the separation method described before (15). Briefly, the dried extracts were shaken twice with a heptane/80% ethanol mix. The highly lipophilic steroid esters partitioned into the heptane phase. The 80% ethanol fraction contained the free (i.e. non-conjugated) and water-soluble metabolites. This was dried completely, reconstituted in water, and extracted with diethyl ether resulting in the separation of the water-soluble metabolites from the free steroids. Saponification of the heptane fraction to release the free steroids was also performed as described previously. A sample (500 μL) of each fraction was mixed with 7 mL scintillation fluid for counting.
The whole CNS was dissected from individual snails (n=5). Tissue fixation, sectioning, and IHC procedure were implemented following a previously published protocol (49). Briefly, CNSs were pinned out on a Sylgard-coated dish containing 4% paraformaldehyde in 0.1 M phosphate buffer (pH=7.4) overnight at 4°C. After washing with phosphate-buffered saline (PBS; 137 mM NaCl, 10 mM Na2HPO4, 1.8 mM KH2PO4, pH=7.4), fixed tissues were cryoprotected in 20% glucose solution for 4 hours at room temperature and embedded into Cryomatrix (#6769006, Thermo Scientific). A series of 12-14 μm-thick cryostat sections were cut and thaw-mounted onto gelatin-aluminium-coated slides. In the case of the comparison of specific anti-Lymnaea GnRH/corazonin (ly-GnRH/CRZ) antibody and anti-human GnRH antibody, alternating sections were made. Next, the samples were incubated with PBS containing 0.25% Triton X-100 and 0.25% bovine serum albumin (BSA) (PBS-TX-BSA) for 1 h at 4°C.
The sections were then incubated for 24 h at 4°C with different primary antibodies diluted in PBS-TX-BSA (Table 2). After washing with PBS, the samples were incubated for 5 h at 4°C with the respective secondary antibodies diluted in PBS-TX-BSA (Table 2). The slides were washed with PBS and cover-slipped with fluorescent mounting medium (#S3023, Dako). The stained tissues were analyzed with a TCS SP8 DMI laser confocal scanning microscope (Leica Microsystems, Germany) equipped with appropriate wavelength-filter configuration settings and a transmitted light detector (BioMarker Ltd, Hungary). Image processing was performed by LasX software (Leica Microsystems, Germany). In the negative control experiments, the sections were only incubated with the secondary antibody and yielded no signal (not shown). The specificity of the anti-ly-GnRH/CRZ antibody (preadsorption with hemocyanin from giant keyhole limpet (KLH), preadsorption with synthetic peptide) was determined previously (49). In some cases, the anti-human GnRH antibody was preadsorbed with 10 µM synthetic human GnRH peptide (#PEP-10933, Thermo Fisher Scientific) overnight at 4°C and applied to the sections resulted in the complete abolishment of the staining (not shown). Given the lack of commercially available proteins, preadsorption control was not performed for the anti-human CYP19A and anti-human nPR antibodies reflecting the methods in the relevant previous papers (8, 10–12, 50).
The whole CNS was dissected from the animals (n=12). The CNSs were pooled and extracted following our previously published protocol (51). Briefly, the sample was homogenized in 1 mL lysis buffer (1 M TRIS (pH=6.8), 2% SDS, 10% glycerol, 1% mercaptoethanol, 10% protease inhibitory cocktail (#P2714-1BTL, Sigma-Aldrich)) with a Dounce homogenizer and then further extracted with ultra-sonication. After centrifugation (16,000×g for 5 min at 4°C), the supernatant was placed in a new tube. 160 µL of the supernatant was diluted with 5x sample buffer (0.1 M TRIS (pH=6.8), 0.5 M DTT, 2% SDS, 10% glycerol, 1 mM EDTA, 0.1% bromophenol blue) and denatured for 5 min at 95°C.
For the WB analysis, 5 µL samples were run three times on a 10% SDS-PAGE, each with the ProSieve™ QuadColor™ Protein Marker (#00193837, Lonza). In the parallel SDS-PAGE, 20 µL samples were run three times for the MS analysis. The gel then was blotted for two hours onto a nitrocellulose membrane (#GE10600002, Sigma-Aldrich) following the standard semi-dry blotting protocol, while the parallel gel was stained with Coomassie brilliant blue following the standard protocol. The membrane was incubated for 3 h with a blocking solution (10% non-fat dry milk powder and 6% BSA in Tris-buffered saline (TBS; 20 mM Tris, 150 mM NaCl, pH=7.6)), then washed thoroughly (3 x 5 min in TBS with 1% Tween-20 (TBST), then 3 x 5 min in TBS). The membrane was then divided into three distinct pieces and incubated overnight at 4°C with the respective primary antibodies (Table 2), each diluted 1:1000 in TBST-6% BSA. After washing (3 x 5 min in TBST, then 3 x 5 min in TBS), the membranes were incubated for 3 h at room temperature with a goat anti-rabbit IgG secondary antibody conjugated with HRP (#172-1019, BioRad) or with a donkey anti-mouse IgG secondary antibody conjugated with HRP (#170-6516, BioRad) – each diluted 1:3000 in TBST-2% non-fat dry milk powder. After washing (3 x 5 min in TBST, 3 x 5 min in TBS), the chemiluminescence was visualized with Western Blotting Luminol Reagent (#sc-2048, Santa Cruz Biotechnology) on an Azure 300 (AZI300-01) system, resulting in digital images. In the negative control experiments, the samples were only probed with the secondary antibody and yielded no bands (not shown). In some cases, the anti-human GnRH antibody was preadsorbed with 10 μM of synthetic human GnRH peptide overnight at 4°C. This resulted in no bands (not shown). Similarly to the IHC method, preadsorption control was not performed for the anti-human CYP19A and anti-human nPR antibodies reflecting the methods in the relevant previous papers (10, 11, 50). Method optimization for Western blotting with the anti-human GnRH antibody to be able to investigate the possible marking at ~1.3 kDa is presented in the Supplementary Information.
For MS identification, the respective bands were excised from the parallel SDS-PAGE gel with a razorblade and placed in Eppendorf tubes. After de-staining and cleaning, the in-gel digestion was performed with Trypsin/Lys-C Mix (#V5072, Promega) based on the supplier technical bulletin (fast digestion protocol). Peptides were cleaned on 3 mL SPE tubes (HLB 60 mg; Waters, Milford, USA), and the eluted samples were concentrated with an Eppendorf Concentrator plus system (Eppendorf, Hamburg, Germany). The concentrated peptides were resolved in 100 µL water containing 0.1% formic acid. The proteomics analysis was performed with Bruker EASY-nLC equipment coupled with a nano-ESI MS instrument (Bruker Maxis 4G UHR-QTOF). Three μL aliquots of the samples were injected and separated on a home-made C18 analytical column (3 μm, 75 μm x 150 mm) using a gradient elution at a flow rate of 250 nL min-1. Eluents had the following composition: A (aqueous formic acid solution: 0.1%) and B (acetonitrile/formic acid: v/v 99.9/0.1%). The scanning range was 250-2800 m/z. The flow of nebulizer gas was 4 L min-1 on 0.6 bar and the temperature was set at 200°C. The capillary voltage was 4.5 kV, and the top 15 peptides were fragmented with the CID fragmentation cell. For protein identification, data were processed with Data Analysis 3.4 software. The identification was carried out with a Mascot server V2.4.1 and PEAKS Studio Xpro software on the Swiss-Prot database. Searching parameters were set to allow one missed cleavage site, accepting 50 ppm mass error at the MS1 and 0.3 Da at the MS2 mode.
Figure 1 shows the disappearance of radioactive E2, P, T, and EE2 from water in five vessels containing two live snails each. There was a marked decrease in radioactivity in all the vessels containing snails with the uptake rate of P being marginally fastest. The amounts of radioactivity remaining in the water after 8 h was ~80% for E2, ~70% for P, ~78% for T, and ~75% for EE2 treatments. In the first 1.5 h, the calculated clearance rates ranged from 3.7 mL animal-1 h-1 for E2 to 5.6 mL animal-1 h-1 for P (Table 3).
Figure 1 Radioactive steroid removal from water by L. stagnalis during an 8 h exposure to a concentration of 2.6 µCi L-1 [3H]-E2 (A), 2.7 µCi L-1 [3H]-P (B), 3.5 µCi L-1 [3H]-T (C), and 2.7 µCi L-1 [3H]-EE2 (D). Exposure (●) and sorption control (○) data are presented as mean % total ± S.E.M (n=5 exposure vessels with 2 animals each and n=3 control vessels).
Extraction and phase separation of the metabolites in the snail tissues showed the presence of a high proportion of radioactivity (40, 50 and 85%) for P, E2, and T, respectively, in the lipid-rich heptane fraction where esterified steroids would be expected to accumulate (Figure 2). The proportion of EE2 that had been potentially esterified was, as expected (18), much lower (8%). Most of the rest of the radioactivity was recovered in the 80% ethanol fraction which contains free steroids. Only a small proportion of radioactivity was found in the water-soluble fraction. After ten days of depuration, approximately 70% of the radioactivity was still present in the animals (Figure 2). The proportion of steroids in the form of ester (i.e. in the heptane fraction v. the 80% ethanol fraction) was higher for all steroids except EE2 - implying the preferential depuration of the free steroids. Only preliminary characterization of the steroids that were present in the ester fraction (following saponification to release the fatty acid moieties) was carried out. This is shown in Supplementary Figure 3. There was evidence that most of the E2 radioactivity (but not all) eluted in the expected elution position of standard E2. The T radioactivity showed two major peaks, one of which eluted with T standard, the other being consistent with the elution position of 5α-DHT. The P radioactivity eluted as two peaks that were consistent with the 5α-reduced steroids identified in the study on the blue mussel Mytilus edulis (17). However, it must be noted that these identifications are only tentative. There was insufficient activity in the EE2 samples to obtain a meaningful separation.
Figure 2 Radioactive residues of four steroids from L. stagnalis (whole tissue extracts) on day 0 (i.e. directly after the 8 h of exposure) and day 10 (i.e. after depuration) with day 0 being treated as 100%. The proportions of ester (black), free (light grey), and water-soluble (dark gray) that were found in the extracts on days 0 and 10 are also shown. Data are presented as mean percentage of the total radioactivity in each extract (n=5 for each time point, except for P, where n=4 on day10).
Despite the lack of CYP19A and nPR genes in L. stagnalis (Supplementary Table 2), our IHC investigations with the anti-human CYP19A and anti-human nPR antibodies yielded a positive signal in the CNS (Figure 3). CYP19A-immunopositive neurons were detected in the paired cerebral (CG) (Figure 3A1), paired pleural (PlG) (Figure 3A2), paired parietal (PaG) (Figure 3A3), unpaired visceral (VG) (Figure 3A3), and paired pedal (PeG) (not shown) ganglia. Neurons labeled by the anti-nPR antibody were observed in the PeG (Figure 3B1), VG (Figures 3B2, B3), and PaG (not shown).
Figure 3 Representative CYP19A (A1-A3) and nPR (B1-B3) immunolabeling (red) in the CNS. The anti-human CYP19A antibody yielded a positive signal, for example, in the right cerebral (A1), right pleural (A2), and right parietal (A3) ganglia, while the anti-human nPR antibody gave immunoreactivity, for example, in the left pedal (B1) and visceral (B2, B3) ganglia. Arrowhead: labeled neuron(s); asterisk: unlabeled neuron(s); np: neuropil. Scale=100 µm (A1, A3, B2, B3) and 50 µm (A2, B1).
Both specific and non-specific antibodies yielded a positive signal in the CNS (Figure 4), however, with a highly different distribution: there were neurons/neuron clusters labeled by both antibodies, while other cells were labeled only by the specific snail or the non-specific human antibody (Figure 4A).
Figure 4 Immunolabeling by the specific anti-ly-GnRH/CRZ antibody [the antigen was a synthetic undecapeptide [CNYHFSNGWYA-amide] corresponding to ly-GnRH/CRZ (49, 52–54)] and/or the non-specific anti-human GnRH antibody (the antigen was a synthetic decapeptide [pQHWSYGLRPG-amide] corresponding to human GnRH) in the CNS. (A) Schematic CNS map (dorsal view) showing the distribution of neurons labeled in the left and right buccal (BG), cerebral (CG), pedal (PeG), pleural (PlG), parietal (PaG), and visceral (VG) ganglia. Neurons labeled by the specific, the non-specific, and both antibodies are indicated with red, blue, and purple symbols, respectively. Some unlabeled but identified large neurons (e.g., CGC) are included for providing reference points for anatomical orientation. (B-G) Representative micrographs made by alternating sectioning showing the comparative immunolabeling (red). In the right CG (B, C), both specific (B) and non-specific (C) antibodies yielded a signal, in part the same neurons were labeled (indicated by asterisks). The giant VD1 neuron (marked by a triangle) of the VG (D, E) and the giant RPD2 neuron (marked by a circle) of the right PaG (F, G) were labeled only by the non-specific antibody (E, G). Scale=50 µm. B1-B4, identified buccal feeding motoneurons; CGC, cerebral giant cell; CDCs, caudo-dorsal cells; al, anterior lobe; vl, ventral lobe; LPeD1, left pedal dorsal 1 neuron; RPeD1, right pedal dorsal 1 neuron; CAcl, cerebral A-cluster; PeAcl, pedal A-cluster; Ecl, E-cluster; SC, statocyst; Mcl, M-cluster; Bcl, B-cluster; VD1, visceral dorsal 1 neuron; RPD2, right parietal dorsal 2 neuron; np, neuropil.
In the BG, only the specific antibody yielded an immunopositive signal (e.g., B3 and B4 motoneurons). In the CG, a small subset of caudo-dorsal cells (CDCs) as well as small-sized neurons in the metacerebrum located close to the ventral lobe (vl) and in the anterior lobe (al) were detected by both antibodies (Figures 4B, C). A group of medium-sized neurons in the A-cluster (CAcl) and the large majority of CDCs were labeled with the specific antibody. Furthermore, some dispersed, small-sized (unidentified) neurons were detected by the human antibody.
In the PeG, several small- and medium-sized neurons in the A- (PeAcl) and E (Ecl) –clusters, some dispersed (unidentified) neurons, as well as the left serotonergic giant cell (LPeD1) and the right dopaminergic giant neuron (RPeD1) could be labeled by the specific antibody. The human antibody yielded immunoreactivity in pedal lb (Pe lb) clusters as well as in some small (unidentified) neurons around the LPeD1 and RPeD1.
The PlG and the left PaG contained only (unidentified) cells labeled by the specific antibody. Neurons in the right RPaG and VG ganglia were labeled by only the specific (e.g., B-cluster [Bcl], and M-cluster [Mcl]) or only the non-specific (e.g., visceral dorsal one [VD1] – Figure 4E; right parietal dorsal two [RPD2] – Figure 4G) antibody.
To determine which molecules were labeled by the human antibodies, we performed PAGE and WB analysis on the CNS homogenate (Figure 5). The anti-human CYP19A antibody yielded one discrete (~30 kDa) and two amorphous (~65 kDa and ~140 kDa) bands. Interestingly, it also marked the 140 kDa marker protein. Instead of the expected band at 1.3 kDa [corresponding to the known mass value of the active ly-GnRH/CRZ peptide (52)], the anti-human GnRH antibody gave two discrete bands with much higher mass value (~50 kDa and ~100 kDa). The anti-human nPR antibody resulted in three discrete bands (~30 kDa, ~50 kDa, and ~60 kDa). The ~30 kDa band was marked by both the anti-human CYP19A and anti-human nPR antibodies, while the ~50 kDa band was marked by both anti-human GnRH and anti-human nPR antibodies.
Figure 5 Western blot analysis of the CNS homogenate with the anti-human CYP19A, anti-human GnRH, and anti-human nPR antibodies. The anti-CYP19A antibody yielded one discrete (~30 kDa) and two amorphous (~65 kDa and ~140 kDa) bands, moreover, it marked the 140 kDa marker protein. The anti-GnRH antibody gave two discrete bands (~50 kDa and ~100 kDa), while the anti-nPR antibody resulted in three discrete bands (~30 kDa, ~50 kDa, and ~60 kDa).
The subsequent MS identification gave no significant match between the fragments obtained from the bands and the three human protein sequences (or their in silico predicted fragments). The proteins that were positively identified in the bands are presented in Table 4. However, there was no evidence that any of these proteins were the ones that cross-reacted with the relevant antibodies.
Although vertebrate sex steroids are present in molluscan tissues, it has been argued that they are so readily absorbed from the environment and can be stored for such a long time, that their presence is very poor evidence of endogenous synthesis [reviewed by (4)]. To provide further evidence for this, we exposed L. stagnalis to radiolabeled sex steroids and investigated their uptake, metabolism, and depuration. We confirmed that snails do indeed, like many other mollusks, absorb vertebrate steroids. Compared to M. edulis (15–18), the rate was approximately ten times slower on a per animal basis. On a gram for gram basis, however, the difference was c. four times slower [e.g., the clearance rate for E2 was previously demonstrated to be c. 40 mL animal-1 h-1 for a single mussel (average 3 g wet weight of tissue) (15); for L. stagnalis (average 1 g wet weight of tissue), the clearance rate of E2 was 3.7 mL animal-1 h-1]. The fact that mussels are filter feeders and continuously pump water through their gills probably accounts for their overall faster rate of absorption. In mussels, not only was the rate of uptake faster, but also the pattern of disappearance of the steroids from the water fitted hyperbolic or exponential decay functions. In L. stagnalis, in contrast, the pattern was linear, except for perhaps P. However, this is probably a result of snail experiments being shorter (8h v. 24h or 48h) and the rate of uptake being slower. Little is known about the mechanism of uptake, although for water-living mollusks it is probably by passive diffusion across the gills. However, sex steroids have been shown to be detected in land-living snails as well (55–57), it is likely that they can also enter the animal by ingestion.
We have confirmed not only that L. stagnalis snails can absorb the natural steroids E2, T and P from the water, but also that they are able to esterify them. Just as in mussels (18), EE2 appeared to be the least easily esterified steroid. Since P has no hydroxyl groups by which it can be esterified, the presence of radioactivity in the ester fraction implied that this steroid had to have been metabolized following uptake. The most likely reactions [based on the steroids previously identified in mussels (17, 25)] are 5α-reduction of the A ring and conversion of the oxo-groups on carbons 3 and 20 to 3β- and 20β-hydroxyl groups, respectively.
Finally, we showed that the steroid radioactivity that could be recovered from the snail tissues was depurated relatively slowly (only c. 30% loss over ten days) – implying a half-life of at least two weeks for all four steroids. This is consistent with studies on mussels that showed an initial rapid loss of free steroid and then a very slow loss of the esterified steroid.
As mentioned in the Introduction section, another key line of evidence that, on the surface, appears to support similarities between the molluscan and the vertebrate endocrine systems is that antibodies raised against human proteins that are associated with sex steroid synthesis or receptor-mediation bind to cells in molluscan tissues. Such positive staining with anti-human CYP19A (i.e. aromatase) antibodies (8, 10–12) has been claimed as evidence for the presence of this enzyme in these animals; despite the aromatase gene not being found in the genome of any species outside chordates (2, 3, 36, 58–61). Our own neuronal transcriptome analysis of L. stagnalis also failed to reveal CYP19A (Supplementary Table 2) (37), yet, like the other studies, we also found positive staining with an anti-human CYP19A. To add complexity to this puzzle, several studies have reported the presence of ‘aromatase activity’ in mollusks (12, 55, 62–66). However, such evidence was mainly obtained indirectly with a non-specific assay [already reviewed by (2, 3)]. In only one case (66) was endogenously-derived estrogen production specifically demonstrated, but the yields were extremely low (<0.1%), suggesting it was due to the now well-recognized phenomenon of enzyme promiscuity (67, 68) (i.e. one or other CYP enzyme having a weak affinity for T or androstenedione that is partially able to catalyze their conversion into estrogens), rather than to the possibility that CYP19A has somehow been missed in numerous previous investigations of molluscan genomes.
We further chose to investigate whether an anti-human nPR antibody gave a signal in the CNS. To the best of our knowledge, the only previous study to look at the immunostaining of an antibody to the nPR in a mollusk was on the common octopus (Octopus vulgaris). Like ours, this study also yielded positive staining (50). As with CYP19A, the nPR gene is not present in mollusks (2, 3, 36, 58, 61), the L. stagnalis transcriptome data also confirming its absence (Supplementary Table 2) (37). This again calls into question the meaningfulness of positive immunostaining with certain vertebrate antibodies for identifying or localizing specific proteins in molluscan tissues.
To study these enigmatic findings further, we compared the immunohistochemical staining of a specific snail antibody with that of a non-specific vertebrate one for ly-GnRH/CRZ neuropeptide in the CNS. When GnRH/CRZ was first described in O. vulgaris, the peptide was named ‘invertebrate GnRH’ based on the sequence similarity to human GnRH and its effects observed in the reproductive system (69). Since then we know that these peptides are multifunctional (i.e. they do not have a specific reproductive function as seen in vertebrates) and should most likely be called CRZs (49, 52–54, 70–74). Because of the GnRH term, some previous papers have investigated invertebrate GnRH/CRZ peptides with antibodies raised against vertebrate (tunicate, mammalian, chicken, fish) GnRHs (75–79). Since these investigations yielded a positive signal in the CNS, we expected immunostaining in L. stagnalis with the non-specific vertebrate antibody. Although it did give a signal in the CNS, it could not label several genuine ly-GnRH/CRZ-immunopositive cells identified previously (49), but labeled several non-invGnRH/CRZ-containing neurons. This, on the one hand, confirms the need for using appropriate antibodies in order to prevent false conclusions (e.g., inferred functions from the distribution) and, on the other hand, highlights that wrong naming schemes (i.e. nominative determinism) in molluscan research can also contribute to using inappropriate antibodies. To further characterize the proteins that were being labeled by the vertebrate antibodies, we performed PAGE, WB, and MS analyses on a homogenate derived from the CNS. Although WB analyses have previously been performed, subsequent MS identification studies had not been done before. A WB analysis of the ovary and testis homogenates of the mussel M. galloprovincialis with an anti-human CYP19A antibody revealed one discrete band at ~60 kDa corresponding to the band of rat CYP19A (10, 11). In contrast, our analysis with an anti-human CYP19A antibody yielded one discrete (~30 kDa) and two amorphous (~65 kDa and ~140 kDa) bands. The antibody also labeled the protein used as the 140 kDa marker. A previous WB investigation of the ovary of O. vulgaris with an anti-chicken nPR antibody revealed one discrete band at ~70 kDa (50). In contrast, our analysis with the anti-human nPR antibody yielded three discrete bands (~30 kDa, ~50 kDa, and ~60 kDa).
Since there is a slight sequence similarity between ly-GnRH/CRZ and human GnRH, we did not exclude the possibility that the non-specific antibody can in some cases recognize ly-GnRH/CRZ neuropeptide resulting in some immunopositive-neurons that were also marked by the specific snail antibody. However, instead of one expected band at ~1.3 kDa, the anti-human GnRH antibody gave two discrete bands with much higher mass values (~50 kDa and ~100 kDa) clearly indicating a non-specific signal.
The subsequent MS analysis of the bands found no sequences that were homologous to those found in vertebrate CYP19A, nPR, and GnRH. Although the homolog searching with the immunogen of the anti-human nPR in the L. stagnalis sequence data revealed three hits (Supplementary Information), they showed no significant match with the fragments obtained from the ~50 kDa band. We could also identify some proteins that may be marked by the antibodies, but this needs to be investigated further.
In summary, we showed that snails, like all other mollusks that have previously been studied, can absorb and accumulate vertebrate steroids. This confirms that the presence of vertebrate sex steroids in molluscan tissues is not necessarily evidence of endogenous production. We also show that the three antibodies that we tested all positively stained two or more proteins in the snail, including, in the case of the CYP19 antibody, one of the molecular weight marker proteins. This clearly highlights that immunostaining with antibodies (especially polyclonal ones) against vertebrate proteins is a highly unreliable procedure for identifying or localizing specific proteins in invertebrate tissues. Our results add to a growing body of literature questioning whether natural selection in mollusks would have favored the evolution of a steroid-based endocrine system. Besides sex steroids (progestogens, androgens, and estrogens), glucocorticoids and mineralocorticoids are the main vertebrate steroid hormones. To the best of our knowledge, there is no evidence that mollusks have 11-hydroxylase and 21-hydroxylase activity (i.e. no corticosterone and cortisol production) or aldosterone synthase activity (i.e. no aldosterone production). Furthermore, the protein homologues of the enzymes that catalyze these reactions (CYP21, CYP11B1, and CYP11B2) are not present in molluscan genomes (80, 81). Also, there is no evidence that mollusks have corticoid receptors (NR3C1 and NR3C2) – the NR3C receptor family is vertebrate-specific (58, 61, 82). Instead of vertebrate-like steroids, we would like to highlight the potential role of sterols as hormones in molluscan endocrinology, though not necessarily in relation to reproduction. For example, non-aromatized steroids (epidioxysterols) have been identified in Aplysia (83). Moreover, since aromatized long-chained steroids (e.g., geodisterol) have been firmly identified in sponges and cnidarians (84, 85),they may also be present in mollusks. The potential role of sterols is supported by a recent finding that inhibition of 5α-reductase, that is known to use sterols, as well as steroids as substrates, caused marked malformations in shell morphology during the development of two freshwater gastropods (86). It can be speculated that in mollusks, some of those sterols might bind to nuclear receptors from the NR1H or NR1I/J/K families. In our opinion, this is a better direction to search for nuclear receptor-based signaling in lophotrochozoans than to continually carry out research on the basis that their endocrine systems are the same as in humans.
We hope that readers will take away the message that research that is carried out on the unquestioning assumption that mollusks are ‘honorary humans’ constitutes a massive waste of resources.
The raw data supporting the conclusions of this article will be made available by the authors, without undue reservation.
IF: Conceptualization, Investigation, Writing - original draft, Data curation, Visualization, Funding acquisition. TS: Investigation, Writing - review & editing, Data curation, Visualization. BK: Investigation, Writing - review & editing. AT: Investigation, Writing - review & editing. JS: Investigation, Writing - review & editing, Data curation, Funding acquisition. AC: Data curation, Visualization, Writing - review & editing. IK: Investigation, Writing - review & editing, Supervision, Funding acquisition. AS: Conceptualization, Methodology, Writing - review & editing. ZP: Conceptualization, Methodology, Writing - review & editing, Data curation, Visualization, Supervision, Funding acquisition. All authors contributed to the article and approved the submitted version.
This work was supported by the National Brain Project (#2017-1.2.1 NKP-2017-00002; ZP); Hungarian Scientific Research Fund (#138039; ZP); Bolyai Foundation (#BO/00646/21/8; ZP); László János Doctoral Scholarship (#498/2021/PTE DOK; IF); New National Excellence Program (ÚNKP-20-3-II-PTE-888; IF) and Cooperative Doctoral Programme for Doctoral Scholarships (KDP-2020-1018493; IF) of the Ministry for Innovation and Technology from the source of the National Research, Development and Innovation Fund; Scholarship for National Young Talents (NTP-NFTÖ-21-B-0212; IF); Defra (#CB0485); Cefas internal funds (Cefas Seedcorn); and Thematic Excellence Program (#TKP2021-EGA-17; JS).
The authors thank Prof. Pei-San Tsai for kindly providing the specific GnRH/CRZ antibody. The research was performed in collaboration with Mass Spectrometry Core Facility at the Szentágothai Research Centre of the University of Pécs.
The authors declare that the research was conducted in the absence of any commercial or financial relationships that could be construed as a potential conflict of interest.
All claims expressed in this article are solely those of the authors and do not necessarily represent those of their affiliated organizations, or those of the publisher, the editors and the reviewers. Any product that may be evaluated in this article, or claim that may be made by its manufacturer, is not guaranteed or endorsed by the publisher.
The Supplementary Material for this article can be found online at: https://www.frontiersin.org/articles/10.3389/fendo.2022.981564/full#supplementary-material
1. Scott AP. Do mollusks use vertebrate sex steroids as reproductive hormones? II. critical review of the evidence that steroids have biological effects. Steroids (2013) 78:268–81. doi: 10.1016/j.steroids.2012.11.006
2. Scott AP. Do mollusks use vertebrate sex steroids as reproductive hormones? Part i. critical appraisal of the evidence for the presence, biosynthesis and uptake of steroids. Steroids (2012) 77:1450–68. doi: 10.1016/j.steroids.2012.08.009
3. Fodor I, Urban P, Scott AP, Pirger Z. A critical evaluation of some of the recent so-called ‘Evidence’ for the involvement of vertebrate-type sex steroids in the reproduction of mollusks. Mol Cell Endocrinol (2020) 516:110949. doi: 10.1016/j.mce.2020.110949
4. Scott AP. Is there any value in measuring vertebrate steroids in invertebrates? Gen Comp Endocrinol (2018) 265:77–82. doi: 10.1016/j.ygcen.2018.04.005
5. Fernandes D, Loi B, Porte C. Biosynthesis and metabolism of steroids in molluscs. J Steroid Biochem Mol Biol (2011) 127(3-5):189–95. doi: 10.1016/j.jsbmb.2010.12.009
6. Giusti A, Joaquim-Justo C. Esterification of vertebrate like steroids in molluscs: A target of endocrine disruptors? Comp Biochem Physiol C Toxicol Pharmacol (2013) 158(4):187–98. doi: 10.1016/j.cbpc.2013.08.003
7. Janer G, Porte C. Sex steroids and potential mechanisms of non-genomic endocrine disruption in invertebrates. Ecotoxicology (2007) 16(1):145–60. doi: 10.1007/s10646-006-0110-4
8. Osada M, Tawarayama H, Mori K. Estrogen synthesis in relation to gonadal development of Japanese scallop, Patinopecten yessoensis: Gonadal profile and immunolocalization of P450 aromatase and estrogen. Comp Biochem Physiol B Biochem Mol Biol (2004) 139:123–8. doi: 10.1016/j.cbpc.2004.07.002
9. Osada M, Takamura T, Sato H, Mori K. Vitellogenin synthesis in the ovary of scallop, Patinopecten yessoensis: Control by estradiol-17β and the central nervous system. J Exp Zool A Comp Exp Biol (2003) 299(2):172–9. doi: 10.1002/jez.a.10276
10. Rosati L, Agnese M, Abagnale L, Aniello F, Andreuccetti P, Prisco M. The mussel Mytilus galloprovincialis in the bay of Naples: New insights on oogenic cycle and its hormonal control. Anat Rec (2019) 302(6):1039–49. doi: 10.1002/ar.24075
11. Prisco M, Agnese M, De Marino A, Andreuccetti P, Rosati L. Spermatogenic cycle and steroidogenic control of spermatogenesis in Mytilus galloprovincialis collected in the bay of Naples. Anat Rec (2017) 300:1881–94. doi: 10.1002/ar.23626
12. Matsumoto T, Osada M, Osawa Y, Mori K. Gonadal estrogen profile and immunohistochemical localization of steroidogenic enzymes in the oyster and scallop during sexual maturation. Comp Biochem Physiol B Biochem Mol Biol (1997) 118:811–7. doi: 10.1016/S0305-0491(97)00233-2
13. Martinez AA, Munoz YR, Serrano FS, Garcia PM. Immunolocalization of cholesterol side chain cleavage enzyme (P450scc) in Mytilus galloprovincialis and its induction by nutritional levels. J Comp Physiol B Biochem Syst Environ (2008) 178(5):647–54. doi: 10.1007/s00360-008-0256-x
14. Di Cosmo A, Di Cristo C, Paolucci M. A estradiol-17β receptor in the reproductive system of the female of Octopus vulgaris: Characterization and immunolocalization. Mol Reprod Dev (2002) 61(3):367–75. doi: 10.1002/mrd.10014
15. Schwarz TI, Katsiadaki I, Maskrey BH, Scott AP. Mussels (Mytilus spp.) display an ability for rapid and high capacity uptake of the vertebrate steroid, estradiol-17β from water. J Steroid Biochem Mol Biol (2017) 165(Pt B):407–20. doi: 10.1016/j.jsbmb.2016.08.007
16. Schwarz TI, Katsiadaki I, Maskrey BH, Scott AP. Rapid uptake, biotransformation, esterification and lack of depuration of testosterone and its metabolites by the common mussel, Mytilus spp. J Steroid Biochem Mol Biol (2017) 171:54–65. doi: 10.1016/j.jsbmb.2017.02.016
17. Schwarz TI, Katsiadaki I, Maskrey BH, Scott AP. Uptake and metabolism of water-borne progesterone by the mussel, Mytilus spp. (Mollusca). J Steroid Biochem Mol Biol (2018) 178:13–21. doi: 10.1016/j.jsbmb.2017.10.016
18. Katsiadaki I, Schwarz TI, Cousins ARO, Scott AP. The uptake of ethinyl-estradiol and cortisol from water by mussels (Mytilus spp.). Front Endocrinol (2021) 12:794623. doi: 10.3389/fendo.2021.794623
19. Gooding MP, LeBlanc GA. Biotransformation and disposition of testosterone in the Eastern mud snail Ilyanassa obsoleta. Gen Comp Endocrinol (2001) 122(2):172–80. doi: 10.1006/gcen.2001.7630
20. Leblanc GA, Gooding MP, Sternberg RM. Testosterone-fatty acid esterification: A unique target for the endocrine toxicity of tributyltin to gastropods. Integ Comp Biol (2005) 45(1):81–7. doi: 10.1093/icb/45.1.81
21. Labadie P, Peck M, Minier C, Hill EM. Identification of the steroid fatty acid ester conjugates formed in vivo in Mytilus edulis as a result of exposure to estrogens. Steroids (2007) 72(1):41–9. doi: 10.1016/j.steroids.2006.10.003
22. Puinean A-M, Labadie P, Hill EM, Osada M, Kishida M, Nakao R, et al. Laboratory exposure to 17β-estradiol fails to induce vitellogenin and estrogen receptor gene expression in the marine invertebrate Mytilus edulis. Aquat Toxicol (2006) 79:376–83. doi: 10.1016/j.aquatox.2006.07.006
23. Janer G, Mesia-Vela S, Porte C, Kauffman FC. Esterification of vertebrate-type steroids in the Eastern oyster (Crassostrea virginica). Steroids (2004) 69(2):129–36. doi: 10.1016/j.steroids.2003.12.002
24. Janer G, Sternberg RM, LeBlanc GA, Porte C. Testosterone conjugating activities in invertebrates: Are they targets for endocrine disruptors? Aquat Toxicol (2005) 71(3):273–82. doi: 10.1016/j.aquatox.2004.11.024
25. Dimastrogiovanni G, Fernandes D, Bonastre M, Porte C. Progesterone is actively metabolized to 5α-Pregnane-3,20-Dione and 3β-Hydroxy-5α-Pregnan-20-One by the marine mussel Mytilus galloprovincialis. Aquat Toxicol (2015) 165:93–100. doi: 10.1016/j.aquatox.2015.05.018
26. Peck MR, Labadie P, Minier C, Hill EM. Profiles of environmental and endogenous estrogens in the zebra mussel Dreissena polymorpha. Chemosphere (2007) 69(1):1–8. doi: 10.1016/j.chemosphere.2007.04.082
27. Guercia C, Cianciullo P, Porte C. Analysis of testosterone fatty acid esters in the digestive gland of mussels by liquid chromatography-high resolution mass spectrometry. Steroids (2017) 123:67–72. doi: 10.1016/j.steroids.2017.05.008
28. Giusti A, Ducrot V, Joaquim-Justo C, Lagadic L. Testosterone levels and fecundity in the hermaphroditic aquatic snail Lymnaea stagnalis exposed to testosterone and endocrine disruptors. Environ Toxicol Chem (2013) 32(8):1740–5. doi: 10.1002/etc.2234
29. Le Curieux-Belfond O, Fievet B, Séralini GE, Mathieu M. Short-term bioaccumulation, circulation and metabolism of estradiol-17β in the oyster. Crassostrea gigas J Exp Mar Biol Ecol (2005) 325:125–33. doi: 10.1016/j.jembe.2005.04.027
30. Lu M, Horiguchi T, Shiraishi H, Shibata Y, Abo M, Okubo A, et al. Identification and quantitation of steroid hormones in marine gastropods by GC/MS. Bunseki Kagaku (2001) 50(4):247–55. doi: 10.2116/bunsekikagaku.50.247
31. Pojana G, Gomiero A, Jonkers N, Marcomini A. Natural and synthetic endocrine disrupting compounds (Edcs) in water, sediment and biota of a coastal lagoon. Environ Int (2007) 33(7):929–36. doi: 10.1016/j.envint.2007.05.003
32. Hallmann A, Smolarz K, Konieczna L, Zabrzanska S, Belka M, Baczek T. LC-MS measurment of free steroids in mussels (Mytilus trossulus) from the southern Baltic Sea. J Pharm BioMed (2016) 117:311–5. doi: 10.1016/j.jpba.2015.09.013
33. Almeida A, Silva MG, Soares A, Freitas R. Concentrations levels and effects of 17α-ethinylestradiol in freshwater and marine waters and bivalves: A review. Environ Res (2020) 185:109316. doi: 10.1016/j.envres.2020.109316
34. Horiguchi T, Ohta Y. A critical review of sex steroid hormones and the induction mechanisms of imposex in gastropod mollusks. In: Saber Saleuddin ABL, Orchard I, editors. Advances in invertebrate (Neuro)Endocrinology, 1st edition. Boca Raton: Apple Academic Press (2020).
35. Balbi T, Ciacci C, Canesi L. Estrogenic compounds as exogenous modulators of physiological functions in molluscs: Signaling pathways and biological responses. Comp Biochem Physiol C Toxicol Pharmacol (2019) 222:135–44. doi: 10.1016/j.cbpc.2019.05.004
36. Fodor I, Pirger Z. From dark to light - an overview of over 70 years of endocrine disruption research on marine mollusks. Front Endocrinol (2022) 13:903575. doi: 10.3389/fendo.2022.903575
37. Fodor I, Koene JM, Pirger Z. Neuronal transcriptome analysis of a widely recognised molluscan model organism highlights the absence of key proteins involved in the de novo synthesis and receptor-mediation of sex steroids in vertebrates. Malacologia (2021) 64(1):69–77. doi: 10.4002/040.064.0103
38. Fodor I, Hussein AA, Benjamin PR, Koene JM, Pirger Z. The unlimited potential of the great pond snail, Lymnaea stagnalis. eLife (2020) 9:e56962. doi: 10.7554/eLife.56962
39. Fodor I, Svigruha R, Kemenes G, Kemenes I, Pirger Z. The great pond snail (Lymnaea stagnalis) as a model of ageing and age-related memory impairment: An overview. J Gerontol A Biol Sci Med Sci (2021) 76(6):975–82. doi: 10.1093/gerona/glab014
40. Amorim J, Abreu I, Rodrigues P, Peixoto D, Pinheiro C, Saraiva A, et al. Lymnaea stagnalis as a freshwater model invertebrate for ecotoxicological studies. Sci Total Environ (2019) 669:11–28. doi: 10.1016/j.scitotenv.2019.03.035
41. Koene JM. Neuro-endocrine control of reproduction in hermaphroditic freshwater snails: Mechanisms and evolution. Front Behav Neurosci (2010) 4:167. doi: 10.3389/fnbeh.2010.00167
42. Di Cristo C, Koene JM. Neurobiology of reproduction in mollusks: Mechanisms and evolution. In: Byrne JH, editor. The Oxford handbook of invertebrate neurobiology. Oxford, UK: Oxford University Press (2017).
43. Rivi V, Benatti C, Colliva C, Radighieri G, Brunello N, Tascedda F, et al. Lymnaea stagnalis as model for translational neuroscience research: From pond to bench. Neurosci Biobehav Rev (2020) 108:602–16. doi: 10.1016/j.neubiorev.2019.11.020
44. Rivi V, Benatti C, Lukowiak K, Colliva C, Alboni S, Tascedda F, et al. What can we teach Lymnaea and what can Lymnaea teach us? Biol Rev Camb Philos Soc (2021) 96(4):1590–602. doi: 10.1111/brv.12716
45. Benjamin PR, Kemenes G, Staras K. Molluscan nervous systems. eLS (2021) 2:1–15. doi: 10.1002/9780470015902.a0029277
46. Pirger Z, Zrinyi Z, Maasz G, Molnar E, Kiss T. Pond snail reproduction as model in the environmental risk assesment: Reality and doubts. In: Ray S, editor. Biological resources of water. London, UK: IntechOpen (2018). p. 33–53.
48. Nakai J, Chikamoto N, Fujimoto K, Totani Y, Hatakeyama D, Dyakonova VE, et al. Insulin and memory in invertebrates. Front Behav Neurosci (2022) 16:882932. doi: 10.3389/fnbeh.2022.882932
49. Fodor I, Zrinyi Z, Urban P, Herczeg R, Buki G, Koene JM, et al. Identification, presence, and possible multifunctional regulatory role of invertebrate gonadotropin-releasing Hormone/Corazonin molecule in the great pond snail (Lymnaea stagnalis). Gen Comp Endocrinol (2020) 299:113621. doi: 10.1016/j.ygcen.2020.113621
50. Di Cosmo A, Paolucci M, Di Cristo C, Botte V, Ciarcia G. Progesterone receptor in the reproductive system of the female of Octopus vulgaris: Characterization and immunolocalization. Mol Reprod Dev (1998) 50(4):451–60. doi: 10.1002/(SICI)1098-2795(199808)50:4<451::AID-MRD9>3.0.CO;2-H
51. Hernadi L, Pirger Z, Kiss T, Nemeth J, Mark L, Kiss P, et al. The presence and distribution of pituitary adenylate cyclase activating polypeptide and its receptor in the snail Helix pomatia. Neurosci (2008) 155(2):387–402. doi: 10.1016/j.neuroscience.2008.05.003
52. Fodor I, Svigruha R, Bozso Z, Toth GK, Osugi T, Yamamoto T, et al. Functional characterization and related evolutionary implications of invertebrate gonadotropin-releasing Hormone/Corazonin in a well-established model species. Sci Rep (2021) 11(1):10028. doi: 10.1038/s41598-021-89614-5
53. Tsai PS, Sun B, Rochester JR, Wayne NL. Gonadotropin-releasing hormone-like molecule is not an acute reproductive activator in the gastropod, Aplysia californica. Gen Comp Endocrinol (2010) 166(2):280–8. doi: 10.1016/j.ygcen.2009.09.009
54. Jung LH, Kavanaugh SI, Sun B, Tsai PS. Localization of a molluscan gonadotropin-releasing hormone in Aplysia californica by in situ hybridization and immunocytochemistry. Gen Comp Endocrinol (2014) 195:132–7. doi: 10.1016/j.ygcen.2013.11.007
55. Le Guellec D, Thiard M-C, Remy-Martin JP, Deray A, Gomot L, Adessi GL. In vitro metabolism of androstenedione and identification of endogenous steroids in Helix aspersa. Gen Comp Endocrinol (1987) 66:425–33. doi: 10.1016/0016-6480(87)90253-x
56. Bose R, Majumdar C, Bhattacharya S. Steroids in Achatina fulica (Bowdich): Steroid profile in haemolymph and in vitro release of steroids from endogenous precursors by ovotestis and albumen gland. Comp Biochem Physiol C Pharmacol Toxicol (1997) 116(3):179–82. doi: 10.1016/S0742-8413(96)00163-6
57. Bamidele JA, Idowu AB, Ademolu KO, Agbaje AA, Ajayi AO. Concentrations of steroids in the giant African land snail (Archahatina marginata) at different reproductive phases. Molluscan Res (2015) 1(1):46–51.
58. Markov GV, Gutierreze-Mazariegos J, Pitrat D, Billas IML, Bonneton F, Moras D, et al. Origin of an ancient Hormone/Receptor couple revealed by resurrection of an ancestral estrogen. Sci Adv (2017) 3:e1601778. doi: 10.1126/sciadv.1601778
59. Callard GV, Tarrant AM, Novillo A, Yacci P, Ciaccia L, Vajda S, et al. Evolutionary origins of the estrogen signaling system: Insights from amphioxus. J Steroid Biochem Mol Biol (2011) 127(3-5):176–88. doi: 10.1016/j.jsbmb.2011.03.022
60. Castro LF, Santos MM, Reis-Henriques MA. The genomic environment around the aromatase gene: Evolutionary insights. BMC Evol Biol (2005) 5:43. doi: 10.1186/1471-2148-5-43
61. Baker ME. Steroid receptors and vertebrate evolution. Mol Cell Endocrinol (2019) 496:110526. doi: 10.1016/j.mce.2019.110526
62. Carreau S, Drosdowsky M. The in vitro biosynthesis of steroids by the gonad of the cuttlefish (Sepia officinalis). Gen Comp Endocrinol (1977) 33:554–65. doi: 10.1016/0016-6480(77)90116-2
63. Morcillo Y, Ronis MJJ, Porte C. Effects of tributyltin on the phase I testosterone metabolism and steroid titres of the clam Ruditapes decussata. Aquat Toxicol (1998) 42:1–13. doi: 10.1016/S0166-445X(97)00102-1
64. Goto Y, Kajiwara M, Yanagisawa Y, Hirose H, Yoshimi T, Umemura M, et al. Detection of vertebrate-type steroid hormones and their converting activities in the neogastropod Thais clavigera (Küster, 1858). J Molluscan Stud (2012) 78:197–204. doi: 10.1093/mollus/eys001
65. Le Curieux-Belfond O, Moslemi S, Mathieu M, Séralini GE. Androgen metabolism in oyster Crassostrea gigas: Evidence for 17β-HSD activities and characterization of an aromatase-like activity inhibited by pharmacological compounds and a marine pollutant. J Steroid Biochem Mol Biol (2001) 78:359–66. doi: 10.1016/s0960-0760(01)00109-1
66. Hallmann A, Konieczna L, Swiezak J, Milczarek R, Smolarz K. Aromatisation of steroids in the bivalve Mytilus trossulus. PeerJ (2019) 7:e6953. doi: 10.7717/peerj.6953
67. Atkins WM. Biological messiness vs. biological genius: Mechanistic aspects and roles of protein promiscuity. J Steroid Biochem Mol Biol (2015) 151:3–11. doi: 10.1016/j.jsbmb.2014.09.010
68. Lathe R, Kotelevtsev Y, Mason JI. Steroid promiscuity: Diversity of enzyme action. preface. J Steroid Biochem Mol Biol (2015) 151:1–2. doi: 10.1016/j.jsbmb.2015.01.008
69. Iwakoshi E, Takuwa-Kuroda K, Fujisawa Y, Hisada M, Ukena K, Tsutsui K, et al. Isolation and characterization of a GnRH-like peptide from Octopus vulgaris. Biochem Biophys Res Comm (2002) 291(5):1187–93. doi: 10.1006/bbrc.2002.6594
70. Hauser F, Grimmelikhuijzen CJP. Evolution of the AKH/Corazonin/ACP/GnRH receptor superfamily and their ligands in the Protostomia. Gen Comp Endocrinol (2014) 209:35–49. doi: 10.1016/j.ygcen.2014.07.009
71. Plachetzki DC, Tsai PS, Kavanaugh SI, Sower SA. Ancient origins of metazoan gonadotropin-releasing hormone and their receptors revealed by phylogenomic analyses. Gen Comp Endocrinol (2016) 234:10–9. doi: 10.1016/j.ygcen.2016.06.007
72. Tsai PS. Gonadotropin-releasing hormone by any other name would smell as sweet. Gen Comp Endocrinol (2018) 264:58–63. doi: 10.1016/j.ygcen.2017.09.010
73. Minakata H, Shigeno S, Kano N, Haraguchi S, Osugi T, Tsutsui K. Octopus gonadotrophin-releasing hormone: A multifunctional peptide in the endocrine and nervous systems of the cephalopod. J Neuroendocrinol (2009) 21(4):322–6. doi: 10.1111/j.1365-2826.2009.01852.x
74. Sakai T, Yamamoto T, Matsubara S, Kawada T, Satake H. Invertebrate gonadotropin-releasing hormone receptor signaling and its relevant biological actions. Int J Mol Sci (2020) 21(22):8544. doi: 10.3390/ijms21228544
75. Amano M, Moriyama S, Okubo K, Amiya N, Takahashi A, Oka Y. Biochemical and immunohistochemical analyses of a GnRH-like peptide in the neural ganglia of the pacific abalone Haliotis discus hannai (Gastropoda). Zool Sci (2010) 27(8):656–61. doi: 10.2108/zsj.27.656
76. Nakamura S, Osada M, Kijima A. Involvement of gnrh neuron in the spermatogonial proliferation of the scallop, Patinopecten yessoensis. Mol Reprod Dev (2007) 74(1):108–15. doi: 10.1002/mrd.20544
77. Zhang L, Wayne NL, Sherwood NM, Postigo HR, Tsai PS. Biological and immunological characterization of multiple GnRH in an opisthobranch mollusk, Aplysia californica. Gen Comp Endocrinol (2000) 118(1):77–89. doi: 10.1006/gcen.2000.7457
78. Tsai PS, Maldonado TA, Lunden JB. Localization of gonadotropin-releasing hormone in the central nervous system and a peripheral chemosensory organ of Aplysia californica. Gen Comp Endocrinol (2003) 130(1):20–8. doi: 10.1016/s0016-6480(02)00519-1
79. Di Cosmo A, Di Cristo C. Neuropeptidergic control of the optic gland of Octopus vulgaris: FMRF-amide and GnRH immunoreactivity. J Comp Neurol (1998) 398(1):1–12. doi: 10.1002/(sici)1096-9861(19980817)398:1<1::aid-cne1>3.0.co;2-5
80. Markov GV, Tavares R, Dauphin-Villemant C, Demeneix BA, Baker ME, Laudet V. Independent elaboration of steroid hormone signaling pathways in metazoans. Proc Natl Acad Sci USA (2009) 106(29):11913–8. doi: 10.1073/pnas.0812138106
81. Nelson DR, Goldstone JV, Stegeman JJ. The cytochrome P450 genesis locus: The origin and evolution of animal cytochrome P450s. Philos Trans R Soc Lond B Biol Sci (2013) 368(1612):20120474. doi: 10.1098/rstb.2012.0474
82. Taubenheim J, Kortmann C, Fraune S. Function and evolution of nuclear receptors in environmental-dependent postembryonic development. Front Cell Dev Biol (2021) 9:653792. doi: 10.3389/fcell.2021.653792
83. Pereira RB, Andrade PB, Valentao P. Chemical diversity and biological properties of secondary metabolites from Sea hares of Aplysia genus. Mar Drugs (2016) 14(2):39. doi: 10.3390/md14020039
84. Wang G-Y-S, Crews P. Geodisterol, a novel polyoxygenated sterol with an aromatic a ring from the tropical marine sponge Geodia sp. Tetrahedron Lett (1996) 37:8145–6. doi: 10.1016/0040-4039(96)01894-1
85. Yan XH, Liu HL, Huang H, Li XB, Guo YW. Steroids with aromatic a-rings from the hainan soft coral Dendronephthya studeri ridley. J Nat Prod (2011) 74(2):175–80. doi: 10.1021/np100562n
Keywords: mollusk, Lymnaea stagnalis, neuroendocrine, sex steroid uptake and metabolism, immunohistochemistry, western blot, mass spectrometry
Citation: Fodor I, Schwarz T, Kiss B, Tapodi A, Schmidt J, Cousins ARO, Katsiadaki I, Scott AP and Pirger Z (2022) Studies on a widely-recognized snail model species (Lymnaea stagnalis) provide further evidence that vertebrate steroids do not have a hormonal role in the reproduction of mollusks. Front. Endocrinol. 13:981564. doi: 10.3389/fendo.2022.981564
Received: 29 June 2022; Accepted: 22 August 2022;
Published: 08 September 2022.
Edited by:
Pei-San Tsai, University of Colorado Boulder, United StatesReviewed by:
Roger P. Croll, Dalhousie University, Canada;Tyrone Hayes, University of California, Berkeley, United StatesCopyright © 2022 Fodor, Schwarz, Kiss, Tapodi, Schmidt, Cousins, Katsiadaki, Scott and Pirger. This is an open-access article distributed under the terms of the Creative Commons Attribution License (CC BY). The use, distribution or reproduction in other forums is permitted, provided the original author(s) and the copyright owner(s) are credited and that the original publication in this journal is cited, in accordance with accepted academic practice. No use, distribution or reproduction is permitted which does not comply with these terms.
*Correspondence: István Fodor, Zm9kb3IuaXN0dmFuQGJsa2kuaHU=
†These authors have contributed equally to this work
Disclaimer: All claims expressed in this article are solely those of the authors and do not necessarily represent those of their affiliated organizations, or those of the publisher, the editors and the reviewers. Any product that may be evaluated in this article or claim that may be made by its manufacturer is not guaranteed or endorsed by the publisher.
Research integrity at Frontiers
Learn more about the work of our research integrity team to safeguard the quality of each article we publish.