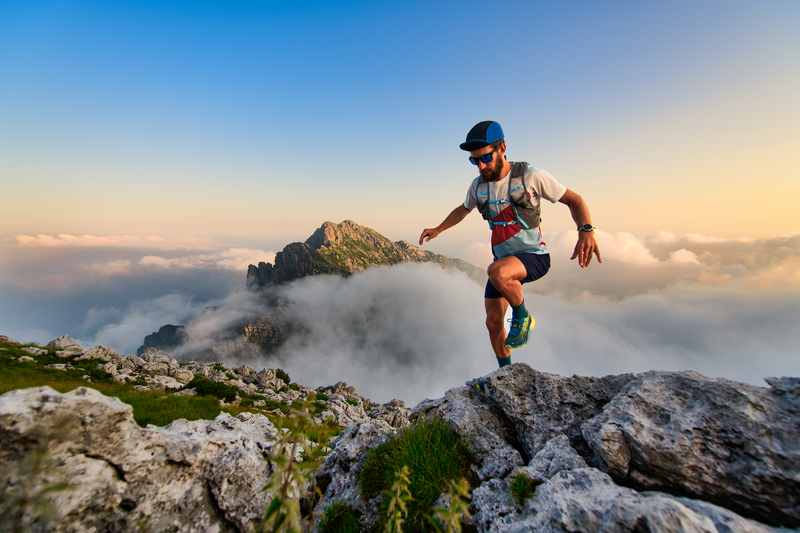
95% of researchers rate our articles as excellent or good
Learn more about the work of our research integrity team to safeguard the quality of each article we publish.
Find out more
ORIGINAL RESEARCH article
Front. Endocrinol. , 12 October 2022
Sec. Experimental Endocrinology
Volume 13 - 2022 | https://doi.org/10.3389/fendo.2022.976488
This article is part of the Research Topic Inter-Organ Communication Beyond Mammals: The Role of Tissue-Specific Cytokines View all 8 articles
Prolactin (Prl) and growth hormone (Gh) as well as insulin-like growth factor 1 (Igf1) are involved in the physiological adaptation of fish to varying salinities. The Igfs have been also ascribed other physiological roles during development, growth, reproduction and immune regulation. However, the main emphasis in the investigation of osmoregulatory responses has been the endocrine, liver-derived Igf1 route and local regulation within the liver and osmoregulatory organs. Few studies have focused on the impact of salinity alterations on the Gh/Igf-system within the neuroendocrine and immune systems and particularly in a salinity-tolerant species, such as the blackchin tilapia Sarotherodon melanotheron. This species is tolerant to hypersalinity and saline variations, but it is confronted by severe climate changes in the Saloum inverse estuary. Here we investigated bidirectional effects of increased salinity followed by its decrease on the gene regulation of prl, gh, igf1, igf2, Gh receptor and the tumor-necrosis factor a. A mixed population of sexually mature 14-month old blackchin tilapia adapted to freshwater were first exposed to seawater for one week and then to fresh water for another week. Brain, pituitary, head kidney and spleen were excised at 4 h, 1, 2, 3 and 7 days after both exposures and revealed differential expression patterns. This investigation should give us a better understanding of the role of the Gh/Igf system within the neuroendocrine and immune organs and the impact of bidirectional saline challenges on fish osmoregulation in non-osmoregulatory organs, notably the complex orchestration of growth factors and cytokines.
Mechanisms of physiological adaptations in euryhaline teleost fish species have been mainly identified in a small number of species (including some salmonids, tilapias, but also the killifish, the striped bass and the marine medaka), using individuals maintained under stable conditions or after unidirectional transfers between freshwater and seawater or vice versa (1). Euryhaline fish species can tolerate large changes in salinity by maintaining their hydromineral balance through integrated ion- and water-transporting functions in the major osmoregulatory organs (gills, intestine and kidney) that are responsive to osmosensory and endocrine stimuli (2, 3). However, as reported by Seale and Breves (1) and Blewett et al. (4), in most of these studies the experimental conditions did not consider the highly dynamic dimensions (temporal and spatial scales) of certain aquatic environments such as estuaries, which are essential for understanding the mechanisms of adaptation to increasing environmental instabilities resulting from climate change. Only a few studies in the mummichog and the Mozambique tilapia have investigated the physiological responses under tidally changing salinity regimes. As Seale and Breves (1) demonstrated in their review, the rearing or life history of an individual will influence its osmoregulatory performance: exposure to tidally saline regimes facilitate adaptation to stable hypo- or hyperosmotic environments, maintain seawater-like branchial ionocyte populations and modify the endocrine regulation of osmoregulation towards a local modulation of Prolactin (Prl) signalling (5). The relevance of studies in Mozambique tilapia (Oreochromis mossambicus) was emphasized, which demonstrated that fish can maintain osmoregulatory parameters within narrow ranges, and tilapia maintain branchial ionocyte populations in a fashion similar to seawater-acclimated fish (reviewed by 1).
Over the past 40 years, reduced rainfall, longer dry seasons and increased evaporation associated with climate change have dramatically modified the salinity levels of some West African estuaries. This is particularly the case for “inverse” estuaries such as the Sine Saloum in Senegal (6). In this complex habitat, apart from the significant spatio-temporal variations classically observed in estuaries, the Saloum estuary is inverse (with a saline gradient increasing from the embouchure to upstream). Furthermore, at the end of the dry season the salinity can reach 130 ppm, which is more than three-fold the salinity of seawater, in the most upstream areas of the river. These very particular saline spatio-temporal regimes (from 0 to 130 ppm) have affected the biodiversity of this environment. Thus, the estuarine blackchin tilapia species Sarotherodon melanotheron is one of the rare fish species that can survive in this environment (7–9). Despite some negative impacts of hypersalinity on its life-history traits (limited growth, reduced size-at-maturity, changing its fecundity), probably associated to an important allocation of energy towards osmoregulation (8), the blackchin tilapia does not migrate during the dry season (10). Moreover, genetically differentiated populations of the same species are also found in Senegal in freshwater, seawater or brackish water. Therefore, besides the Mozambique tilapia, the blackchin tilapia also constitutes an extremely interesting model to better understand the effects of salinity variations, whether they are seasonal (dry and rainy seasons), tidal (in the estuaries) or linked to long-term climatic effects (i.e. hypersalinities in the Saloum inverse estuary), on its physiology. Using different populations living under contrasted saline regimens, recent studies have begun to analyze the mechanisms of the blackchin tilapia’s extraordinary plasticity and adaptation to the significant variations in salinity, both under controlled and natural environments (9, 11–15). In addition, in West Africa, the genus Sarotherodon includes important species for fisheries in estuaries and lagoons but also for aquaculture, e.g. in Senegal and Ivory Coast.
The growth hormone (Gh)/insulin-like growth factor (Igf)1 system has been demonstrated to be involved in several physiological functions in fish (16, 17), particularly in the acclimation to osmotic change (1, 18). In both the Mozambique and blackchin tilapias, the Igfs are involved in osmoregulation (19, 20). In seawater-exposed blackchin tilapia, we have found elevated igf1, igf2 and ghr1 expressions in gills (20), which is a major osmoregulating organ (1, 21). The inverse was observed after exposure to seawater and recovery with retransfer to freshwater causing decreases in these genes. In contrast, hepatic igf1, igf2 and ghr1 mRNA decreases after seawater transfer (20). While these studies focused on liver, the source of endocrine Igf1, and osmotic active organs, much less is known with respect to the Gh/Igf system within the brain, pituitary and particularly in immune organs. Prl is essential for freshwater osmoregulation in fish (1, 22), whereas Gh is considered to be involved in seawater adaptation (23, 24). Numerous cell types within the tilapia brain express igf1 and igf2, respectively (25–27). In previous studies, we observed a differential expression of igf1 and tumor necrosis factor (tnfa) in immune organs of tilapia and rainbow trout (28, 29). In order to take into account the large variations in salinity (tidal, seasonal and/or climate change induced) that blackchin tilapia face in the conventional or inverse estuaries in which they live, we have performed here a bidirectional exposure from freshwater to seawater and back to freshwater. We then analyzed the expression changes in igf1, igf2, and ghr1 genes in whole brains, pituitaries, spleens and head kidneys as well as the expression changes in prl and gh genes in the pituitaries. In order to increase our knowledge on the complex orchestration of cytokines and growth factors in the euryhaline blackchin tilapia, we also studied tnfa gene expressions in head kidneys and spleens after seawater and freshwater exposures.
The initial broodstock of blackchin tilapia, Sarotherodon melanotheron heudelotii, were collected during the rainy season (at a water salinity of 48 ‰) in Kaolack from a wild population in the Saloum estuary in Senegal by Dr. Abdou Mbow (Institut Fondamental d’Afrique Noire, Université Cheikh Anta Diop de Dakar, Senegal), and transferred in 2002 to the experimental facilities of the Centre de Coopération Internationale en Recherche Agronomique pour le Développement (CIRAD) in Montpellier (France) where they were reared in recirculating freshwater at 27 ± 1 °C under a 12 h light/12 h dark cycle. For this study, a mixed batch of sexually mature 14-month-old fish from a 4th generation progeny of the fish stocked in the CIRAD facilities was used. All the experimental procedures took place in CIRAD facilities under the Laboratory agreement for animal experimentation number A-34-172-24 and the author’s personal authorization for animal experimentation N° 35-15, both authorised by the French Government.
In order to avoid a handling stress during the freshwater to seawater or the seawater to freshwater experiments (30), the fish were kept in the freshwater aquaria, and half of the water was changed by aspiration and replaced by saline water prepared from a stock solution of 100 ‰ made from OCEAN INSTANT® salts at a rate of 0.5 ‰ per min until the experimental seawater salinity of 35 ‰ was reached, as previously described (20). Salinity was monitored daily. Similarly, for the seawater to freshwater experiment, seawater was changed by aspiration and replaced by freshwater at the same exchange rate until the 0 ‰ salinity was reached. In order to standardize the stress in all fish groups, at each salinity change (freshwater to seawater or seawater to freshwater), a freshwater change was also conducted in the freshwater control group, i.e., half of the water was changed by aspiration and replaced by freshwater.
One hundred and ten individuals were maintained in 11 freshwater aquaria of 40 l each, with 10 fish per aquarium under a 12 h/12 h light/dark cycle. Each aquarium was equipped with an individual filter, a temperature-controlled heater (27 ± 1 °C) and an aerator. Fish were fed to satiation three times a day with BIOMAR-SA food (BIOMAR-S.A., Nersac, France) until 24 h before sampling. Ten aquaria were used for the salinity challenges of the experimental fish: five aquaria were used for the five seawater sampling time points. In the other five aquaria, the seawater to freshwater retransfer was then performed after one week. The 11th aquarium was the freshwater control batch. For each sampling time point (4 h, 1, 2, 3 and 7 days after water exchange), all the ten fish from an aquarium were anaesthetized together, sacrificed and samples collected. For that purpose, one dose of 2-phenoxyethanol (Sigma, St. Louis, MO, USA) was introduced directly into the water of one aquaria to reach a dose of 2 ml/l. At each sampling time point, fish were weighted and measured. Both experimental groups were similar in size, with an average length of 11.6 ± 0.6 cm and weight of 26.2 ± 5.0 g in the seawater group and an average length of 11.8 ± 0.7 cm, and weight of 27.4 ± 4.2 g in the freshwater control group. Intact brain, pituitary, head kidney and spleen were excised and transferred into 1.5 ml of RNAlater™ (Ambion, Austin, TX, USA) for RNA preservation, kept at 4°C overnight to allow RNAse-inhibitors to act and then stored at -20°C until RNA extraction.
ZF gene and protein nomenclature rules were followed (31). TaqMan real-time PCR assays were created (Table 1) based on mRNA sequences of tilapia species, i.e., Sarotherodon melanotheron prl (32), Oreochromis mossambicus igf1 (33), igf2 (34), and RNA ribosomal 18s (rna18s) (35), and Oreochromis niloticus gh (36), ghr1 (37), tnfa (38), actin beta (actb) (39), and eukaryotic translation elongation factor 1a (eef1a) (40), as previously described (26, 28, 41–43). Taqman probes were dually labelled at the 5’ end with the fluorescent reporter dye FAM and at the 3’ end with the quencher TAMRA. Primer accuracy was verified as published for igf1, igf2, gh and actb (25, 26), and ghr1, tnfa and eef1a (20). A comparison of three different reference genes (actb, eef1a, and rna18s) demonstrated that actb was most stable across these experimental groups as previously evaluated for liver and osmoregulatory organs (20).
Total RNA was extracted using the TRIzol™reagent (Invitrogen, Merelbeke, Belgium), chloroform, isopropanol and ethanol, re-suspended in RNase free water and photospectrometrically quantified. Total RNA was treated with 1 U/μl of RQ1 RNAse-free DNAse (Catalys AG, Switzerland). Single-stranded cDNA was synthesized from 800 ng of total RNA using 1 x TaqMan® RT Buffer, MgCl2 (5.5 mM), 1.25 U/μl of MuLV reverse transcriptase, 2.5 μM of random hexamer primers, 0.4 U/μl ribonuclease inhibitor, and 500 μM of each dNTP (Applied Biosystems, Rotkreuz, Switzerland) for 10 min at 25 ˚C, 30 min at 48 ˚C and 5 min at 95 ˚C. Then 2 μl cDNA obtained from 10 ng/μl total RNA were subjected, in duplicate, to real-time PCR using an Absolute™ QPCR low ROX Mix (ABgene, UK) including Thermo-Start® DNA Polymerase, 300 nM of each primer and 150 nM of the fluorogenic probe. Amplification was performed with a total reaction volume of 10 μl in a MicroAmp Fast Optical 96-well reaction plate (Applied Biosystems). The reactions were run on the ABI 7500 Fast real-time PCR System (Applied Biosystems) under the following conditions: 15 min at 95 ˚C for enzyme activation followed by 40 cycles of 95 ˚C for 15 s and 60 ˚C for 1 min. The PCR amplification products were verified on gel electrophoresis (Figure 1) using a GeneRuler 25 bp molecular weight marker and loading buffer (both Fermentas, Le Mont sur Lausanne, Switzerland).
Figure 1 Gel electrophoresis of real-time PCR amplification products at their expected sizes between 75-97 bps (compare Table 1). Lane 1: 25 bp molecular weight marker, lanes 2-3: actb, lanes 4-5: rna18s, lanes 6-7: igf1, lanes 8-9: igf2, lanes 10-11: ghr1, lanes 12-13: gh, lanes 14-15: prl, lanes 16-17: tnfa, lane 18: 25 bp molecular weight marker.
The relative gene expression model was used to evaluate the n-fold changes in mRNA expression between experimental and control fish for each sampling time point using the ΔΔCT method (44) as established (20, 28, 29, 41–43, 45). Prior to analysis, real-time PCR assays were validated by plotting CT values against the logarithms of the dilution factors. Slope was determined and the correlation of efficiencies between compared assays confirmed as described for igf1, gh and actb (26, 46). Relative gene expression ratios (R) between treated and control groups were calculated using the formula: R = 2-ΔΔCT with ΔCT = CT (target gene) - CT (reference gene) and ΔΔCT = ΔCT (treated group) - ΔCT (untreated control group). Thus, experimentally induced changes are presented as n-fold differences relative to the corresponding untreated control set to 1. Significant differences between treated and untreated groups are designated by asterisks. Significant differences between time points are designated by different small letters. Statistical significance was calculated using the Mann-Whitney rank sum test. Statistical analyzes were performed with GraphPad Prism© 5 (Graphpad Software, Inc., San Diego, CA, USA).
In brain, ghr1 gene expression was not altered by seawater nor by freshwater (Figure 2). Brain igf1 mRNA elevated 4 h after exposition to seawater (Figure 2). At 1 and 2 days after seawater exposure, igf1 and igf2 genes significantly decreased (Figure 2). After 1 week in seawater, restitution of freshwater lowered igf1mRNA after 4 h, and after 3 and 7 days. Gene expression of igf2 mRNA decreased one day after freshwater exposure and persisted at a lower level until the end of the study (Figure 2).
Figure 2 Effects of salinity on brain ghr1, igf1, and igf2 gene expressions. Relative changes (log2, y-axis) of mRNA expression at 4 h, 1, 2, 3 and 7 days (x-axis) after exposure to seawater (white columns) and freshwater (black columns). Bars represent mean ratios between treated and control group ± SEM and asterisks show significant differences: * p < 0.05; ** p < 0.005; *** p < 0.0005. Bars within treatment groups that do not share any letters are significantly different from each other with p < 0.05, e.g., igf1 seawater expression between 4 h and 1 day, 2, 3 days and 7 days are significantly different, but between 1 day or 2 days, and 3 or 7 days are not significantly different.
From the first post-experimental day on, prl mRNA lowered during the entire seawater period. After return of freshwater, experimental group levels raised back to those of freshwater controls to become significantly lower again after 1 day for the entire freshwater period (Figure 3). At 4 h after exposure to seawater, gh mRNA increased and returned to control levels. At 2 days after freshwater exposure, gh mRNA decreased and then returned once again to the control level (Figure 3).
Figure 3 Effects of a seawater and freshwater exposure on pituitary prl and gh gene expressions. Relative changes (log 2, y-axis) of mRNA expression at 4 h, 1, 2, 3 and 7 days (x-axis) after exposure to seawater (white columns) and freshwater (black columns). Bars represent mean ratios between treated and control group ± SEM and asterisks show significant differences: * p < 0.05; *** p < 0.0005. Bars within treatment groups that do not share any letters are significantly different from each other: p < 0.05., e.g., prl seawater expression between 4 h and 1 day, 2, 3 or 7 days are significantly different, but between 2 and 3 or 7 days are not significantly different. :Starting 1 day after return of freshwater, a significant difference to untreated freshwater controls was observed, but not between time points.
Exposure to seawater lowered the ghr1 gene expression in the pituitary after 1 day, which was compensated to elevated levels after 7 days (Figure 4). Restitution of freshwater did not significantly alter ghr1 mRNA as compared to the control fish (set as 1) (Figure 4). After exposure to seawater, pituitary igf1mRNA was elevated at 4 h, 3 and 7 days (Figure 4). The maintenance for 7 days in seawater followed by the exposure to freshwater resulted in elevated igf1 mRNA levels already after 4 hours, which remained elevated for the rest of the experimental period. The igf2 mRNA levels did not significantly differ from those of the control fish, but differed between time points (Figure 4).
Figure 4 Effects of exposure to seawater and freshwater on pituitary ghr1, igf1, and igf2 gene expressions. Relative changes (log2, y-axis) of mRNA expression at 4 h, 1, 2, 3 and 7 days (x-axis) after exposure to seawater (white columns) and freshwater (black columns). Bars represent mean ratios between treated and control group ± SEM and asterisks show significant differences: * p < 0.05; ** p < 0.005; *** p < 0.0005. Bars within treatment groups that do not share any letters are significantly different from each other: p < 0.05, e.g., igf2 seawater expression between 4 h and 1 or 2 days are significantly different, but between 4 h and 3 or 7 days and between 1 or 2 days and 3 or 7 days are not significantly different.
In the head kidney, ghr1 expression was elevated 1 day after seawater challenge while no significant changes compared to the freshwater controls (set as 1) occurred at the other times points. After 7 days in seawater, however, the ghr gene expression was lower than at all other time points, also after restitution of freshwater (Figure 5). Four hours after exposure to seawater, igf1 mRNA increased and persisted with a tendency to elevation becoming significant again after 3 and 7 days (Figure 5). After the return to freshwater, igf1 gene expression was similarly elevated throughout the entire treatment period (Figure 5). While at the other time points no significant changes in igf2 expression were observed, it decreased at 1 and 2 days after return to freshwater and recovered after 3 days. No significant changes occurred for tnfa (Figure 5).
Figure 5 Effects of a seawater and freshwater exposure on the head kidney ghr1, igf1, igf2, and tnfa gene expressions. Relative changes (log2, y-axis) of mRNA expression at 4 h, 1, 2, 3 and 7 days (x-axis) after exposure to seawater (white columns) and freshwater (black columns). Bars represent mean ratios between treated and control group ± SEM and asterisks show significant differences: * p < 0.05; *** p< 0.0005. Bars within treatment groups that do not share any letters are significantly different from each other: p < 0.05, e.g., ghr1 seawater expression between 4 hours, 1, 2 or 3 days and 7 days are significantly different, but between 4 h and 1 day, 2 or 3 days are not significantly different.
In the spleen, ghr1 and igf2 expressions were not significantly different to control levels (set as 1), but differed between time points (Figure 6). After exposure to seawater, igf1 mRNA decreased after 4 h and 1 day, which reversed by the return of freshwater to elevated levels after 2 and 7 days (Figure 6). After exposure to seawater, tnfa gene expression differed between the time points until it significantly decreased after 7 days. After return of freshwater, no significant differences to the control fish levels occurred, but between time points (Figure 6).
Figure 6 Effects of a seawater and freshwater exposures on the spleen ghr1, igf1, and igf2 and tnfa gene expressions. Relative changes (log2, y-axis) of mRNA expression at 4 h, 1, 2, 3 and 7 days (x-axis) after exposure to seawater (white columns) and restitution of freshwater (black columns). Bars represent mean ratios between treated and control groups ± SEM and asterisks show significant differences: * p < 0.05; ** p < 0.005. Bars within treatment groups that do not share any letters are significantly different from each other: p < 0.05, e.g., tnfa seawater expression after 7 days was significantly lower than in untreated controls, and differed to fish after 1 day in seawater, but not after 4 h, 2 or 3 days.
Osmoregulation is an essential physiological adaptation required for health and reproduction of numerous euryhaline fish species (23, 47–51). The blackchin tilapia is an euryhaline species, which has evolved particular physiological adaptations to drastic salinity changes (52). Nevertheless, climate changes appear to severely impact fish physiology (53). Salinity changes occur in lakes, rivers and estuarines’ ecosystems in West Africa where increased salinities, due to the combined effects of reduced freshwater inflow and water evaporation on the one hand (12), and on the other hand seasonal rainfalls decrease water salinity (9). Increasing drought periods particularly affect some Sahelian estuaries, which is the case in the Sine Saloum River of Senegal (6, 8, 11, 13).
Environmental salinity exerts pronounced effects on somatic growth and on the Gh/Igf1 axis of the juvenile Mozambique tilapias (5, 54). To date, main emphasis on osmoregulatory responses of the Gh/Igf1 system has been laid on the endocrine, liver-derived Igf1 route (5, 55, 56), and on local regulation within the liver and osmoregulatory organs (20, 55–58), as well as gh and prl expressions in the pituitary (5, 54). Nevertheless, environmental alterations of the Gh/Igf-system also impact other physiological systems including the immune system [for reviews see: (59– 64)]. We here explored the effects of seawater and freshwater exposures on the igf1, igf2 and ghr1 genes in brain, pituitary and immune organs and additionally, gh and prl genes in the pituitary and tnfa gene in the immune organs head kidney and spleen.
In our preceding study, we found elevated expressions of the igf1, igf2 and ghr1 genes in the gills of the blackchin tilapia after exposure to seawater and in part, after returning to freshwater (20). Likewise, ghr1 expression was upregulated in freshwater gills in wild sockeye salmon (Oncorhynchus nerka) (65). Thus, there exists evidence for a differential involvement of the Gh/Igf-system in osmoregulation at extrahepatic sites, but the data set is limited. Most recently, the Gh/Igf1 axis together with cortisol were found to be strongly involved in seawater adaptation and osmoregulation in Atlantic salmon (Salmo salar) as observed for muscle tissue (66). A particular local role of igf1 was proposed for fast signalling on the salt secretory system in euryhaline teleosts in response to salinity stress (58). Both igf1 mRNA and Igf1 peptide were detected in the majority of ionocytes of developing and adult Nile tilapia (27). These gills ionocytes are in combination with prolactin signalling crucial for salinity adaptation in euryhaline fish (1). In the present study, we focused on brain, pituitary and the immune organs head kidney and spleen.
Four hours after exposure to seawater, brain igf1 mRNA was elevated, but subsequently both, igf1 and igf2 mRNA levels decreased from day 1 onwards, and levels remained low after return of freshwater, while ghr1 mRNA was not affected. In brain neurons of juvenile and, to a lesser extent adult Nile tilapia, igf1 expression was detected in different regions including cerebellum (27, 67), periventricular zone and optic tectum (41). In our preceding study, transfer to seawater lowered igf1 mRNA in the intestine at 4 h, which increased in a stepwise manner becoming significant at 2 days. After exposure to freshwater, intestinal igf1 gene was elevated during the entire experimental period (20). Specific alterations upon hypoosmotic stress such as disturbed gut-brain neurotransmission, impaired intestinal barrier integrity, and inflammatory reactions were recently described in the yellowfin seabream (Acanthopagrus latus), including changes in the tight junction protein ZO-2 and interleukin 1 beta (il1b) (68). An evolutionary well-conserved role of Igf1 was suggested for paracellular transport and organization of epithelia such as formation of tight junctions (e.g., 69–71). Similarly, the importance of Igf1 for intercellular junctions and tissue architecture was proposed for liver (72, 73), the main source of endocrine Igf1. In addition, in human lymph nodes, our group had detected Igf1 immunoreactivity in high-endothelial venules, site of lymphodiapedesis, which we interpreted as particular relevance of Igf1 for maintenance and restoration of cellular integrity and tissue organization (74). These rudimentary data might point to a role of Igf1 also in the brain-gut-axis to cope with alterations of the water salinity environment via intercellular connections.
Significantly lower igf2 gene expressions compared to control fish were observed in the present study after seawater and freshwater exposure exclusively in the brain. In adult Mozambique tilapia, igf2 expression was widespread in neurons, meninges and choroid plexus of diverse brain regions, which was interpreted as a role in the differentiation, maintenance and regeneration of neurons, particularly for the continued post-embryonic life-long brain growth in many teleost fishes (75). However, this was even lower than igf1 expression as measured by absolute transcript quantification (26). In the present study, there was a general trend to lower levels of igf2 compared to control fish in brain, which was also observed in the other organs after either treatment. Lower igf2 in contrast to igf1 gene expressions were also observed in seabream juveniles (Sparus aurata) one hour after stress conditions of salinity, temperature and ammonia, whereby igf1 was emphasized to be more under control of Gh (76). Organ specific alterations of igf1 and igf2 after Gh treatment were observed in rainbow trout (Oncorhynchus mykiss) (77), Nile tilapia pituitary and immune organs (28, 43).
The adenohypophyseal hormones Prl and Gh are involved in the physiological adaptation to varying salinities (5, 20, 49, 55, 56, 58, 66, 78). In the present study, we observed a decrease in prl mRNA levels in the blackchin tilapia starting from the first post-experimental day after seawater exposure and during the entire seawater period, which back in freshwater raised to baseline control levels. This is well in line with the observation in isolated Prl cells that the salinity acclimation history of fish influences the osmotic responsiveness. For instance, baseline gene expressions of prl177 and prl188 were 30-fold higher in Prl cells isolated from Mozambique tilapia acclimated to freshwater versus fish acclimated to seawater. These Prl cells were less responsive to hyposmotic stimulation with regard to prl mRNA levels (79), which might explain, even with respect to species differences, that the freshwater-acclimated blackchin tilapia in our study did not strongly react after their return from seawater back to freshwater. Thus, differences in responses of Prls and prls to extracellular osmolality between tilapia species may be linked, at least in part, to the observed inter-species difference in salinity tolerance as reviewed by Seale and co-workers (2020).
Return of freshwater elevated prl gene expressions after 4 h and lowered them again for 7 days. Most recently, Prl has been proposed to promote freshwater acclimation in mummichogs by orchestrating the expression of solute transporters/channels, water channels, and tight-junction proteins. In freshwater-type ionocytes, ovine Prl stimulated the expression of Na+/Cl- cotransporter 2 and aquaporin 3, whereas it lowered gene expressions in seawater-type ionocytes of the Na+/K+/2Cl- cotransporter 1, cystic fibrosis transmembrane regulator 1, and claudin 10f (80). In mammals, Prl is the central hormone to promote during pregnancy mammary cell proliferation and differentiation to prepare for lactation. From an evolutionary aspect, secretion of a mineral-enriched solution from skin glands to protect soil-laid eggs from heat and dryness was described for amphibians and reptiles (81–83). Prl is a pleiotropic hormone, which was found to be produced also at extrapituitary sites and to act on the brain in manifold ways (for review: 84).
In contrast to prl mRNA, seawater challenge increased gh mRNA at 4 h whereas freshwater exposure decreased it. Our results are well in line with higher gh expressions found in seawater than in freshwater exposed Mozambique tilapia (5). The data regarding Gh levels under salinity changes are, however, contradictory, whereby several studies proposed gh mRNA as a better indicator of growth than Gh serum levels (5, 54, 85). As also demonstrated in Mozambique tilapia individuals under controlled conditions (86), populations of blackchin tilapia living in seawater had a better growth rate than those living in freshwater (8, 11). Individual growth rates in that study were calculated by the ratio between fish length and the actual fish age in years estimated by counting the macro-increments on whole otoliths according to the validated method developed by Panfili and co-workers (2004). In contrast, those living under hypersaline conditions (Saloum estuary) exhibited the lowest growth rate, probably reflecting the energy costs of adapting to extreme salinities (11). In the pituitary of these individuals, gh expression was higher in populations of blackchin tilapias adapted to seawater than in those living in freshwater, brackish or hypersaline environments (11). In that population study, gh expression levels seemed to be better related to growth rates in each environment than to salinities. Nevertheless, important variations of both prl1 and gh gene expressions were reported between individuals for each population.
In the preceding study on osmoregulatory organs (20), salinity challenges did not cause a significant change in renal igf1 mRNA. In the gills, igf1 mRNA was decreased after 2 days in seawater to become elevated after 3 and 7 days. We found igf1 mRNA expression in the blackchin tilapia pituitary elevated after 4 h of exposure to seawater and then again after 3 and 7 days, remaining high when re-exposed to freshwater, while igf2 mRNA was not altered. Pituitary ghr1 in seawater followed somewhat the trend of igf1 with levels increasing only after 3 days, but upon freshwater exposure, ghr levels differed only slightly between groups. Similarly, in the pituitary of seawater-acclimated Mozambique tilapia, ghr1 and igf1 increased, but with no difference in gh gene suggesting that local Prl-releasing peptide and dopamine 2 receptor may control and regulate prl gene expression in the pituitary (87). Nevertheless, in those pituitary hormone cell types supposed to be differentially involved in a broad range of physiological actions including osmoregulation (88), igf1 gene or Igf1 peptide were not detected in freshwater-reared Nile tilapia, i.e., the somatolactin cells in the pars intermedia and the prolactin cells in the rostral pars distalis (89).
On the contrary, pronounced Igf1 immunoreactivity in pituitary adrenocorticotrophic hormone (ACTH) cells was well preserved throughout evolution (61, 62), which was interpreted as a particular need of ACTH cells for maintenance and restoration (89), also under salinity stress for a healthy and functioning immune axis (87). Most recently, the importance of ACTH in electrolyte homeostasis via the mineralocorticoid 2 receptor was emphasized (90).
Alterations of natural salinities have been proposed to impair the immune system of fish (91). Increased salinity in Nile tilapia (92), did not significantly affect any immune system assays, whereas decreased salinity was accompanied by lymphopenia, neutrophilia and monocytosis in the peripheral blood without modifying total cell volume, plasma protein or cortisol levels. Phagocytosis was increased without change of the phagocytic index. Transforming growth factor beta (tgfb) transcription increased, but transcription of il1b did not change. In that study, acute salinity changes appeared to trigger reactive dysregulation of the immune response in tilapia, which in combination with additional stressors could make fish more susceptible to infectious diseases (92). For instance, in the intestine of Amyloodinum ocellatum-infected juvenile European sea bass (Dicentrarchus labrax), cytokines including tnfa increased, which was proposed to help fish to cope with the infection (93). Interestingly, in head kidney and spleen of Yersinia ruckeri-challenged rainbow trout (Oncorhynchus mykiss), igf1 and tnfa were simultaneously elevated while again igf2 expression was, if at all affected, lowered (29).
Very few data exist on the local role of Igf1 and even less for Igf2, in fish immune organs generally. In the present study, 4 h after exposure to seawater, igf1 expression in the head kidney increased and remained elevated until the end of the experiment, and this was the case also after return of freshwater. No significant changes were observed in tnfa expression, whereby a similar tendency to elevated levels in seawater and lowered levels in freshwater was observed and likewise for ghr1. In spleen, tnfa expression was lower only after 7 days in seawater, but there was again a similar tendency to lower levels in seawater seen equally for ghr1. After exposure to seawater, igf1 mRNA decreased, which reversed when returned to freshwater reaching levels that became significantly elevated after 2 and 7 days.
The role of the Gh/Igf-system has been under debate for the fish immune system for a long time (reviewed by 64). In gh-overexpressing Mozambique tilapia, igf1 expression was lower than in the spleen of control fish (43). Recently, a differential role of cytokines and the Gh/Igf axis has been emphasized from in vitro studies in Atlantic salmon (94) and in vivo studies in zebrafish (95).
The present study contributes tissue specific effects on gene expressions of the igfs and tnfa, which have been proposed to exert opposite effects in several studies. More recently in the Nile tilapia (On), two tnf isoforms, On-tnfa and On-tnfb, have been identified in a wide range of tissues (96). Experimental infection with Streptococcus agalactiae significantly increased On-tnfa and On-tnfb mRNA levels and Tnf proteins in spleen lymphocytes during the primary response stage of adaptive immunity suggesting the possible involvement of On-tnfa and On-tnfb in the adaptive immune response of Nile tilapia. Both transcripts increased following activation of spleen lymphocytes by the T cell specific mitogen phytoagglutinine. Finally, recombinant On-Tnfa and On-Tnfb could induce apoptosis of head kidney leukocytes in Nile tilapia, whereby On-tnfb, but not On-tnfa promoted apoptosis by activating caspase-8 in target cells (96). While both, tnfa and tnfb, were postulated to be clearly involved as pro-inflammatory cytokines in the lymphocyte-mediated adaptive immune response of Nile tilapia by initiating apoptosis (96), more specific data are required in the future in a comparative approach. So far, Tnfa has been proposed in human patients as potential antagonistic pro-inflammatory cytokine counteracting some beneficial effects of Igf1 and impairing the activity of the Gh/Igf axis, e.g., in neurological and immunological disorders (reviewed by 97, 98).
Whole salmonid fry showed a small upregulation of igf1 receptor expression during bacterial and viral infections. In particular, Yersinia ruckeri-challenge strongly downregulated gene expressions of igf1 and its receptors in head kidney and spleen and of igf2 in spleen of adult fish, which highlights a strong repression of IGF-signalling in primary immune tissues (99). The data set is still generally too scarce in light of the important role of fish species in aquaculture and wildlife research. In a study on salinity and the immune health system in Nile tilapia (100), fish reared at 16-ppt salt showed better performance than the population at 20 ppt with a lower mortality and higher expression of ion-regulated genes, stress-related genes in the gills and pro-inflammatory genes il1b and interleukin 8 (il8) in the liver. Elevated kidney-immune-related genes at 20-ppt salt were proposed to indicate that a higher salinity may predispose the fish to infection and to increased mortality. On the contrary, as proposed by Choi etal. (92) rapid decreases in salinity but not increases lead to immune dysregulation in Nile tilapia. In a recent study in olive flounder (Paralichthys olivaceus), after transfer from seawater to freshwater, there was no difference in cortisol levels between active and passive coping flounder while the prolactin level of freshwater-acclimated active coping flounder was higher than of passive acting flounder (101). This underlines the complexity of the orchestration of the neuroendocrine and the immune system.
While this study describes salinity-related changes in genes of the hormones and growth factors in euryhaline tilapia, brain, pituitary, head kidney, and spleen, the amounts of mRNA may not necessarily reflect the amounts of encoded peptides and their activities. Future studies are encouraged to address peptides or activities of hormones and cytokines as well as the osmoregulatory status and immune functions of the fish.
Our study describes the effects of a bidirectional exposure and acclimation from freshwater to seawater and back to freshwater challenges on the transcripts of several genes associated with osmoregulation and immunity, igf1, igf2, and ghr1 in whole brains, pituitaries, spleens and head kidneys as well as the changes in prl and gh in the pituitaries and tnfa expressions in head kidneys and spleens by quantitative PCR in a strongly euryhaline tilapia species. Our results show the influence of salinity-related changes on mRNA contents of several endocrine and immune genes: in brain, 1 or 2 days after seawater and freshwater exposure, respectively, igf1 and igf2 genes were decreased. In the pituitary, prl gene expression was lowered over the entire seawater period and transiently returned to control levels after a return to freshwater. Gene expressions of gh and igf1 increased immediately after seawater challenge while the ghr1 gene was lowered after 1 day. In the head kidney, igf1 was elevated over the entire period, also in freshwater, but in spleen, decreased in seawater and then also increased in freshwater. These results aim at contributing to a more differentiated understanding of the involvement and role of the Gh/Igf-system within the neuro-endocrine and immune organs and the impact of bidirectional saline challenges in organs other than osmoregulatory organs, notably the complex orchestration of growth factors and cytokines.
The raw data supporting the conclusions of this article will be made available by the authors, without undue reservation.
The animal study was reviewed and approved by CIRAD facilities under the Laboratory agreement for animal experimentation number A-34-172-24.
J-FB, HD’C, EE, KL and NSh designed the study. GA, J-FB, AC, HD’C, EE, OF, KL, FM, NSe, and NSh performed experiments. J-FB, HD’C, EE, KL, and OR revised the manuscript. All authors contributed to the article and approved the submitted version.
Work was supported by the Swiss National Foundation and the Prof. Dr. med. Karl and Rena Theiler-Haag Foundation.
Thanks are due to Manfred Reinecke, Institute of Anatomy, University of Zurich, for the initiation of the original salinity-project. The authors are grateful to Marc Cannone, Aquaculture Unit UPR20, Centre de Coopération Internationale en Recherche Agronomique pour le Développement, Campus International Baillarguet, Montpellier, France for excellent and friendly support.
The authors declare that the research was conducted in the absence of any commercial or financial relationships that could be construed as a potential conflict of interest.
All claims expressed in this article are solely those of the authors and do not necessarily represent those of their affiliated organizations, or those of the publisher, the editors and the reviewers. Any product that may be evaluated in this article, or claim that may be made by its manufacturer, is not guaranteed or endorsed by the publisher.
1. Seale AP, Breves JP. Endocrine and osmoregulatory responses to tidally-changing salinities in fishes. Gen Comp Endocrinol (2022) 326:114071. doi: 10.1016/j.ygcen.2022.114071
2. Evans DH, Piermarini PM, Choe KP. The multifunctional fish gill: dominant site of gas exchange, osmoregulation, acid-base regulation, and excretion of nitrogenous waste. Physiol Rev (2005) 85:97–177. doi: 10.1152/physrev.00050.2003
3. McCormick SD, Bradshaw D. Hormonal control of salt and water balance in vertebrates. Gen Comp Endocrinol (2006) 147:3–8. doi: 10.1016/j.ygcen.2005.12.009
4. Blewett TA, Binning SA, Weinrauch AM, Ivy CM, Rossi GS, Borowiec BG, et al. Physiological and behavioural strategies of aquatic animals living in fluctuating environments. J Exp Biol (2022) 225(9):jeb242503. doi: 10.1242/jeb.242503
5. Moorman BP, Yamaguchi Y, Lerner DT, Grau EG, Seale AP. Rearing Mozambique tilapia in tidally-changing salinities: effects on growth and the growth hormone/insulin-like growth factor I axis. Comp Biochem Physiol A Mol Integr Physiol (2016) 198:8–14. doi: 10.1016/j.cbpa.2016.03.014
6. Panfili J, Thior D, Ecoutin J-M, Ndiaye P, Albaret J-J. Influence of salinity on the size at maturity for fish species reproducing in contrasting West African estuaries. J Fish Biol (2006) 69:95–113. doi: 10.1111/j.1095-8649.2006.01069.x
7. Simier M, Blanc L, Alioume C, Diouf PS, Albaret JJ. Spatial and temporal structure of fish assemblages in an “inverse estuary”, the sine saloum system (Senegal). Estuary Coast Shelf Sci (2004) 59:69e86. doi: 10.1016/j.ecss.2003.08.002
8. Panfili J, Mbow A, Durand J-D, Diop K, Diouf K, Thior D, et al. Influence of salinity on the life-history traits of the West African black-chinned tilapia (Sarotherodon melanotheron): comparison between the Gambia and saloum estuaries. Aquat. Living Resour (2004) 17:65–74. doi: 10.1051/alr:2004002
9. Ouattara N’G., Bodinier C, Nègre-Sadargues G, D’Cotta H, Messad S, Charmantier G, et al. Changes in gill ionocyte morphology and function following transfer from fresh water to hypersaline waters in the tilapia Sarotherodon melanotheron. Aquaculture (2009) 290:155–64. doi: 10.1016/j.aquaculture.2009.01.025
10. Diouf K, Panfili J, Labonne M, Aliaume C, Tomas J, Do Chi T. Effects of salinity on strontium: calcium ratios in the otoliths of the West African blackchinned tilapia sarotherodon melanotheron in a hypersaline estuary. Environment. Biol Fish (2006) 77:9e20. doi: 10.1007/s10641-006-9048-x
11. Tine M, de Lorgeril J, Diop K, Bonhomme F, Panfili J, Durand J-D. Growth hormone and prolactin-1 gene transcription in natural populations of the blackchinned tilapia sarotherodon melanotheron acclimatised to different salinities. Comp Biochem Physiol Part B (2007) 147:541.e549. doi: 10.1016/j.cbpb.2007.03.010
12. Tine M, de Lorgeril J, D’Cotta H, Pepey E, Bonhomme F, Baroiller JF, et al. Transcriptional responses of the black-chinned tilapia Sarotherodon melanotheron to salinity extremes. Mar Genomics (2008) 1:37–46. doi: 10.1016/j.margen.2008.06.001
13. Tine M, McKenzie DJ, Bonhomme F, Durand JD. Salinity-related variation in gene expression in wild populations of the black-chinned tilapia from various West African coastal marine, estuarine and freshwater habitats. Estuarine Coast Shelf Sci (2011) 91:102.e109. doi: 10.1016/j.ecss.2010.10.015
14. Tine M, Guinand B, Durand JD. Variation in gene expression along a salinity gradient in wild populations of the euryhaline black-chinned tilapia Sarotherodon melanotheron. J Fish Biol (2012) 80:785–801. doi: 10.1111/j.1095-8649.2012.03220.x
15. Lorin-Nebel C, Avarre JC, Faivre N, Wallon S, Charmantier G. Osmoregulatory strategies in natural populations of the black-chinned tilapia sarotherodon melanotheron exposed to extreme salinities in West African estuaries. J Comp Physiol B (2012) 182:771–80. doi: 10.1007/s00360-012-0657-8
16. Chandhini S, Trumboo B, Jose S, Varghese T, Rajesh M, Kumar VJR. Insulin-like growth factor signalling and its significance as a biomarker in fish and shellfish research. Fish Physiol Biochem (2021) 47:1011–31. doi: 10.1007/s10695-021-00961-6
17. Wood AW, Duan C, Bern HA. Insulin-like growth factor signaling in fish. Int Rev Cytol (2005) 243:215–85. doi: 10.1016/S0074-7696(05)43004-1
18. Sakamoto T, McCormick SD. Prolactin and growth hormone in fish osmoregulation. Gen Comp Endocrinol (2006) 147:24–30. doi: 10.1016/j.ygcen.2005.10.008
19. Breves JP, Hirano T, Grau EG. Ionoregulatory and endocrine responses to disturbed salt and water balance in Mozambique tilapia exposed to confinement and handling stress. Comp Biochem Physiol A. Mol Integr Physiol (2010) 155:294–300. doi: 10.1016/j.cbpa.2009.10.033
20. Link K, Berishvili G, Shved N, D’Cotta H, Baroiller J-F, Reinecke M, et al. Seawater and freshwater challenges affect the insulin-like growth factors IGF-I and IGF-II in liver and osmoregulatory organs of the tilapia. Mol Cell Endocrinol (2010) 327:40–6. doi: 10.1016/j.mce.2010.05.011
21. Lin Y-T, Lee T-H. Rapid response of osmotic stress transcription factor 1 (OSTF1) expression to salinity challenge in gills of marine euryhaline milkfish (Chanos chanos). PloS One (2022) 17:e0271029. doi: 10.1371/journal.pone.0271029
22. Manzon LA. The role of prolactin in fish osmoregulation: a review. Gen Com. Endocrinol (2002) 125:291–310. doi: 10.1016/j.ygcen.2022.114051
23. Sakamoto T, Hirano T. Expression of insulin-like growth factor I gene in osmoregulatory organs during seawater adaptation of the salmonid fish: possible mode of osmoregulatory action of growth hormone. Proc Nat Acad Sci (1993) 90:1912–6. doi: 10.1073/pnas.90.5.1912
24. McCormick SD. Endocrine control of osmoregulation in teleost fish. Am Zool (2001) 41:781–94. doi: 10.1016/S0074-7696(05)43004-1
25. Caelers A, Schmid AC, Hrusovsky A, Reinecke M. Insulin-like growth factor II mRNA is expressed in neurones of the brain of the bony fish Oreochromis mossambicus, the tilapia. Eur J Neurosci (2003) 18(2):355–63. doi: 10.1046/j.1460-9568.2003.02761.x
26. Caelers A, Berishvili G, Meli ML, Eppler E, Reinecke M. Establishment of a real-time RT-PCR for the determination of absolute amounts of IGF-I and IGF-II gene expression in liver and extrahepatic sites of the tilapia. gen. Comp Endocrinol (2004) 137:196–204. doi: 10.1016/j.ygcen.2004.03.006
27. Berishvili G, Shved N, Eppler E, Clota F, Baroiller J-F, Reinecke M. Organ-specific expression of IGF-I during early development of bony fish as revealed in the tilapia, oreochromis niloticus, by in situ hybridisation and immunohistochemistry: Indication for the particular importance of local IGF-I. Cell Tissue Res (2006) 325:287–301. doi: 10.1007/s00441-005-0133-9
28. Shved N, Berishvili G, Mazel P, Baroiller JF, Eppler E. Growth hormone (GH) treatment acts on the endocrine and autocrine/paracrine GH/IGF-axis and on TNF-α expression in bony fish pituitary and immune organs. Fish Shellfish Immunol (2011) 31:944–52. doi: 10.1016/j.fsi.2011.08.012
29. Wenger M, Shved N, Akgül G, Caelers A, Casanova A, Segner H, et al. Developmental oestrogen exposure differentially modulates IGF-I and TNF-α expression levels in immune organs of Yersinia ruckeri-challenged young adult rainbow trout (Oncorhynchus mykiss). Gen Comp Endocrinol (2014) 205:168–75. doi: 10.1016/j.ygcen.2014.05.022
30. Auperin B, Baroiller JF, Ricordel MJ, Fostier A, Prunet P. Effect of confinement stress on circulating levels of growth hormone and two prolactins in freshwater-adapted tilapia (Oreochromis niloticus). Gen Comp Endocrinol (1997) 108:35–44. doi: 10.1006/gcen.1997.6938
31. The Zebrafish Information Network (ZFIN). (2022). Available at: https://zfin.org.
32. Meng QH, Li SF, Fan WJ. GenBank accession no. GQ359823 Vol. 1. Bethesda, MD, USA: National Library of Medicine (2009).
33. Reinecke M, Schmid A, Ermatinger R, Loffing-Cueni D. Insulin-like growth factor I in the teleost Oreochromis mossambicus, the tilapia: Gene sequence, tissue expression, and cellular localization. Endocrinology (1997) 138:3613–9. doi: 10.1210/endo.138.9.5375
34. Chen JY, Tsai HL, Chang CY, Wang JI, Shen SC, Wu JL. Isolation and characterization of tilapia (Oreochromis mossambicus) insulin-like growth factors gene and proximal promotor region. DNA Cell Biol (1998) 17:359–76. doi: 10.1089/dna.1998.17.359
35. Rodgers BD, Weber GM, Kelley KM, Levine MA. Prolonged fasting and cortisol reduce myostatin mRNA levels in tilapia larvae; short-term fasting elevates. Am J Physiol Regul Integr Comp Physiol (2003) 284:1277–86. doi: 10.1152/ajpregu.00644.2002
36. Rentier-Delrue F, Swennen D, Prunet P, Lion M, Martial JA. Tilapia prolactin: molecular cloning of two cDNAs and expression in Escherichia coli. DNA (1989) 8:261–70. doi: 10.1089/dna.1.1989.8.261
37. Jiao B, Huang X, Chan CB, Zhang L, Wang D, Cheng CH. The co-existence of two growth hormone receptors in teleost fish and their differential signal transduction, tissue distribution and hormonal regulation of expression in seabream. J Mol Endocrinol (2006) 36:23–40. doi: 10.1677/jme.1.01945
38. Praveen K, Leary JH 3rd, Evans DL, Jaso-Friedmann L. Molecular cloning of cellular apoptosis susceptibility (CAS) gene in Oreochromis niloticus and its proposed role in regulation of non-specific cytotoxic cell (NCC) functions. Fish Shellfish Immunol (2006) 20:647–55. doi: 10.1016/j.fsi.2005.06.010
39. Hwang GL, Rahman AM, Razak AS, Sohm F, Farahmand H, Smith A, et al. Isolation and characterisation of tilapia beta-actin promoter and comparison of its activity with carp beta-actin promoter. Biochim Biophys Acta (2003) 1625:11–8. doi: 10.1016/S0167-4781(02)00534-1
40. Mochida K, Matsubara T. Molecular cloning of an elongation factor 1 alpha and its mRNA localization in testis of the Nile tilapia Orechromis niloticus. Fish. Sci (2002) 68:830–7.
41. Shved N, Berishvili G, D’Cotta H, Baroiller J-F, Eppler E, Segner H, et al. Ethinylestradiol (EE2) differentially interferes with insulin-like growth factor I (IGF-I) in liver and extrahepatic sites during development of male and female bony fish. J Endocrinol (2007) 195:513–23. doi: 10.1677/JOE-07-0295
42. Shved N, Berishvili G, Häusermann E, D’Cotta H, Baroiller JF, Eppler E. Challenge with 17alpha-ethinylestradiol (EE2) during early development persistently impairs growth, differentiation, and local expression of IGF-I and IGF-II in immune organs of tilapia. Fish Shellfish Immunol (2009) 26:524–30. doi: 10.1016/j.fsi.2009.02.003
43. Eppler E, Berishvili G, Mazel P, Caelers A, Hwang G, Maclean N, et al. Distinct organ-specific up- and down-regulation of IGF-I and IGF-II mRNA in various organs of a GH-overexpressing transgenic Nile tilapia. Transgenic Res (2010) 19:231–40. doi: 10.1007/s11248-009-9314-8
44. Livak KJ, Schmittgen TD. Analysis of relative gene expression data using real-time quantitative PCR and the 2(-delta delta C(T)) method. Methods (2001) 25:402–8. doi: 10.1006/meth.2001.1262
45. Berishvili G, Baroiller J-F, Eppler E, Reinecke M. Insulin-like growth factor-3 (IGF-3) in male and female gonads of the tilapia: development and regulation by growth hormone (GH) and 17α-ethinylestradiol (EE2). Gen Comp Endocrinol (2010) 167:128–34. doi: 10.1016/j.ygcen.2010.01.023
46. Caelers A, Maclean N, Hwang G, Eppler E, Reinecke M. Expression of endogenous and exogenous growth hormone (GH) messenger (m) RNA in a GH-transgenic tilapia (Oreochromis niloticus). Transgenic Res (2005) 14(1):95–104. doi: 10.1007/s11248-004-5791-y
47. Culbert BM, Regish AM, Hall DJ, McCormick SD, Bernier NJ. Neuroendocrine regulation of plasma cortisol levels during smoltification and seawater acclimation of Atlantic salmon. Front Endocrinol (2022) 13:859817. doi: 10.3389/fendo.2022.859817
48. Liebert AM, Schreck CB. Effects of acute stress on osmoregulation, feed intake, IGF-1, and cortisol in yearling steelhead trout (Oncorhynchus mykiss) during seawater adaptation. Gen Comp Endocrinol (2006) 148:195–202. doi: 10.1016/j.ygcen.2006.03.002
49. Seale AP, Pavlosky KK, Celino-Brady FT, Yamaguchi Y, Breves JP, Lerner DT. Systemic versus tissue-level prolactin signaling in a teleost during a tidal cycle. J Comp Phys (2019) 189:581–94. doi: 10.1007/s00360-019-01233-9
50. Seidelin M, Madsen SS. Endocrine control of Na+,K+-ATPase and chloride cell development in brown trout (Salmo trutta): interaction of insulin-like growth factor-I with prolactin and growth hormone. J Endocrinol (1999) 162:127–35. doi: 10.1677/joe.0.1620127
51. Tipsmark CK, Madsen SS. Distinct hormonal regulation of na(+), k(+)-atpase genes in the gill of Atlantic salmon (Salmo salar l.). J Endocrinol (2009) 203:301–10. doi: 10.1677/JOE-09-0281
52. Guèye M, Tine M, Kantoussan J, Ndiaye P, Thiaw OT, Albaret JJ. Comparative analysis of reproductive traits in black-chinned tilapia females from various coastal marine, estuarine and freshwater ecosystems. PloS One (2012) 7:e29464. doi: 10.1371/journal.pone.0029464
53. Servili A, Canario AVM, Mouchel O, Muñoz-Cueto JA. Climate change impacts on fish reproduction are mediated at multiple levels of the brain-pituitary-gonad axis. Gen Comp Endocrinol (2020) 291:113439. doi: 10.1016/j.ygcen.2020.113439
54. Magdeldin S, Uchida K, Hirano T, Grau EG, Abdelfattah A, Nozaki M. Effects of environmental salinity on somatic growth and growth hormone/insulin-like growth factor-I axis in juvenile tilapia Oreochromis mossambicus. Fish. Sci (2007) 73:1025–34. doi: 10.1111/j.1444-2906.2007.01432.x
55. Mohammed-Geba K, Mancera JM, Martínez-Rodríguez G. Acclimation to different environmental salinities induces molecular endocrine changes in the GH/IGF-I axis of juvenile gilthead sea bream (Sparus aurata l.). J Comp Physiol B (2015) 185:87–101. doi: 10.1007/s00360-014-0871-7
56. Mohammed-Geba K, González AA, Suárez RA, Galal-Khallaf A, Martos-Sitcha JA, Ibrahim HM, et al. Molecular performance of prl and Gh/Igf1 axis in the Mediterranean meagre, Argyrosomus regius, acclimated to different rearing salinities. Fish Physiol Biochem (2017) 43:203–16. doi: 10.1007/s10695-016-0280-9
57. Mancera JM, McCormick SD. Evidence for growth hormone/insulin-like growth factor I axis regulation of seawater acclimation in the euryhaline teleost Fundulus heteroclitus. Gen Comp Endocrinol (1998) 111:103–12. doi: 10.1006/gcen.1998.7086
58. Yan J-J, Lee Y-C, Tsou Y-L, Tseng Y-C, Hwang P-P. Insulin-like growth factor 1 triggers salt secretion machinery in fish under acute salinity stress. J. Endocrinol (2020) 246:277–88. doi: 10.1530/JOE-20-0053
59. Segner H, Eppler E, Reinecke M. The impact of environmental hormonally active substances on the endocrine and immune system of fish. Review. Book chapter in: Reinecke M, Zaccone G, Kapoor BG (Eds.) Fish Endocrinology (2006) 2 (Plymouth, USA: Science Publishers, Enfield (NH), Jersey), 809–65.
60. Reinecke M. Influences of the environment on the endocrine and paracrine fish growth hormone-insulin-like growth factor-I system. J. Fish Biol (2010) 76:1233–54. doi: 10.1111/j.1095-8649.2010.02605.x
61. Eppler E. Morphological aspects of the pituitary as the central regulatory organ in the hypothalamic-pituitary-gonad (HPG) axis in fish. In: recent advances in fish reproductive biology. In: García Ayala A, Meseguer-Peñalver J, Chaves-Pozo E, editors. Research signpost. Kerala, India: Trivandrum (2010). p. 1–22.
62. Eppler E. The insulin-like growth factor I (IGF-I) within the bony fish pituitary: new morpho-functional and phylogenetic aspects. Special issue: ‘Paracrine processes in the pituitary gland’. Open Neuroendocrinol. J (2011) 4:43–50. doi: 10.2174/1876528901104010043
63. Reindl KM, Sheridan M. Peripheral regulation of the growth hormone-insulin-like growth factor system in fish and other vertebrates. Biochem Physiol A Mol Integr Physiol (2012) 163(3-4):231–45. doi: 10.1016/j.cbpa.2012.08.003
64. Franz AC, Faass O, Köllner B, Shved N, Link K, Casanova A, et al. Endocrine and local IGF-I in the bony fish immune system. Biol (Basel) (2016) 5:9. doi: 10.3390/biology5010009
65. Flores AM, Shrimpton JM, Patterson DA, Hills JA, Cooke SJ, Yada T, et al. Physiological and molecular endocrine changes in maturing wild sockeye salmon, Oncorhynchus nerka, during ocean and river migration. J Comp Physiol B (2012) 182:77–90. doi: 10.1007/s00360-011-0600-4
66. Bortoletti M, Maccatrozzo L, Peruzzi S, Espen J, Strand T, Jobling M, et al. Dietary effects on biomarkers of growth, stress, and welfare of diploid and triploid Atlantic salmon (Salmo salar) during parr-smolt transformation. Aquaculture Rep 24 (2022) 101123. doi: 10.1016/j.aqrep.2022.101123
67. Shved N, Berishvili G, D’Cotta H, Baroiller J-F, Eppler E, Segner H, et al. A survey on the expression of IGF-I in the early developing bony fish with special emphasis on the tilapia, Oreochromis niloticus. Trends in comparative endocrinology and neurobiology. In: Larhammar D, Flik G, Roubos E, Schoofs L, Vaudry H, editors. Ann. N.Y. Acad. Sci., New York Academy of Sciences, New York, USA 1040 (2006). p. 469–71.
68. Lin G, Li S, Huang J, Gao D, Lu J. Hypoosmotic stress induced functional alternations of intestinal barrier integrity, inflammatory reactions, and neurotransmission along gut-brain axis in the yellowfin seabream (Acanthopagrus latus). Fish Physiol Biochem (2021) 47:1725–38. doi: 10.1007/s10695-021-01011-x
69. Paye JMD, Akers RM, Huckle WR, Forsten-Williams K. Autocrine production of insulin-like growth factor-I (IGF-I) affects paracellular transport across epithelial cells in vitro. Cell Commun Adhes (2007) 14:85–98. doi: 10.1080/15419060701463116
70. Ko JA, Murata S, Nishida T. Up-regulation of the tight-junction protein ZO-1 by substance p and IGF-1in A431 cells. Cell Biochem Funct (2009) 27:388–94. doi: 10.1002/cbf.1587
71. Mitsui R, Fujita-Yoshigaki J, Narita T, Matsuki-Fukushima M, Satoh K, Qi B, et al. Maintenance of paracellular barrier function by insulin-like growth factor-I in submandibular gland cells. Arch Oral Biol (2010) 55:963–9. doi: 10.1016/j.archoralbio.2010.07.023
72. Tutau F, Rodriguez-Ortigosa C, Puche JE, Juanarena N, Monreal I, Garcia Fernandez M, et al. Enhanced actions of insulin-like growth factor-I and interferon-alpha co-administration in experimental cirrhosis. Liver Int (2009) 29:37–46. doi: 10.1111/j.1478-3231.2008.01770.x
73. Lara-Diaz VJ, Castilla-Cortazar I, Martin-Estal I, Garcia-Magarino M, Aguirre GA, Puche JE, et al. IGF-1modulates gene expression of proteins involved in inflammation, cytoskeleton, and liver architecture. J Physiol Biochem (2017) 73:245–58. doi: 10.1007/s13105-016-0545-x
74. Oberlin D, Fellbaum C, Eppler E. Insulin-like growth factor I (IGF-I) mRNA and peptide are expressed in the human lymph node and distinctly defined to subtypes of macrophages, antigen-presenting cells, lymphocytes and endothelial cells. Immunology (2009) 128:342–50. doi: 10.1111/j.1365-2567.2009.03136.x
75. Caelers A, Schmid AC, Hrusovsky A, Reinecke M. Insulin-like growth factor II mRNA is expressed in neurones of the brain of the bony fish Oreochromis mossambicus, the tilapia. Eur J Neurosci (2003) 18:355–63. doi: 10.1046/j.1460-9568.2003.02761.x
76. Zarantoniello M, Bortoletti M, Olivotto I, Ratti S, Poltronieri C, Negrato E, et al. Salinity, temperature and ammonia acute stress response in seabream (Sparus aurata) juveniles: A multidisciplinary study. Animals (2021) 11:97–112. doi: 10.3390/ani11010097
77. Biga PR, Schelling GT, Hardy RW, Cain KD, Overturf K, Ott TL. The effects of recombinant bovine somatotropin (rbST) on tissue IGF-I, IGF-I receptor, and GH mRNA levels in rainbow trout, Oncorhynchus mykiss. Gen. Comp Endocrinol (2004) 135:324–3. doi: 10.1016/j.ygcen.2003.10.014
78. Seale AP, Riley LG, Leedom TA, Kajimura S, Dores RM, Hirano T, et al. Effects of environmental osmolality on release of prolactin, growth hormone and ACTH from the tilapia pituitary. Gen Comp Endocrinol (2002) 128:91–101. doi: 10.1016/S0016-6480(02)00027-8
79. Seale AP, Malintha GHW, Celino-Brady FT, Head T, Belcaid M, Yamaguchi Y, et al. Transcriptional regulation of prolactin in a euryhaline teleost: Characterisation of gene promoters in silico and transcriptome analyses. J Neuroendocrinol (2020) 32:e12905. doi: 10.1111/jne.12905
80. Breves JP, Puterbaugh KM, Bradley SE, Hageman AE, Verspyck AJ, Shaw LH, et al. Molecular targets of prolactin in mummichogs (Fundulus heteroclitus): Ion transporters/channels, aquaporins, and claudins. Gen Comp Endocrinol (2022) 325:114051. doi: 10.1016/j.ygcen.2022.114051
81. Oftedal OT. The mammary gland and its origin during synapsid evolution. J Mammary Gland Biol Neoplasia (2002) 7:225–52.
82. Oftedal OT, Dhouailly D. Evo-devo of the mammary gland. J Mammary Gland Biol Neoplasia (2013) 18:105–20.
83. Spina E, Cowin P. Embryonic mammary gland development. Semin Cell Development Biol (2021) 114:83–92.
84. Costa-Brito AR, Gonçalves I, Santos CRA. The brain as a source and a target of prolactin in mammals. Neural Regen. Res (2022) 17:1695–702. doi: 10.4103/1673-5374.332124
85. Riley LG, Hirano T, Grau EG. Effects of transfer from seawater to fresh water on the growth hormone/insulin-like growth factor-I axis and prolactin in the tilapia, Oreochromis mossambicus. Comp Biochem Physiol B Biochem Mol Biol (2003) 136:647–55. doi: 10.1016/S1096-4959(03)00246-X
86. Sparks RT, Shepherd BS, Ron B, Richman NH, Riley LG, Iwama GK, et al. Effects of environmental salinity and 17 alpha-methyltestosterone on growth and oxygen consumption in the tilapia. Oreochromis mossambicus. Comp Biochem Physiol (2003) 136:657–65. doi: 10.1016/S1096-4959(03)00245-8
87. Aruna A, Nagarajan G, Chang C-F. The acute salinity changes activate the dual pathways of endocrine responses in the brain and pituitary of tilapia. Gen Comp Endocrinol (2015) 211:154–64. doi: 10.1016/j.ygcen.2014.12.005
88. Ocampo Daza D, Larhammar D. Evolution of the growth hormone, prolactin, prolactin 2 and somatolactin family. Gen Comp Endocrinol (2018) 264:94–112. doi: 10.1016/j.ygcen.2018.01.007
89. Eppler E, Shved N, Moret O, Reinecke M. IGF-I is distinctly located in the bony fish pituitary as revealed for Oreochromis niloticus, the Nile tilapia, using real-time RT-PCR, in situ hybridisation and immunohistochemistry. Gen Comp Endocrinol (2007) 150:87–95. doi: 10.1016/j.ygcen.2006.07.013
90. Lu Y, Shi S, Jin X, He J, Yin Z. Domestication of farmed fish via the attenuation of stress responses mediated by the hypothalamus–pituitary–inter-renal endocrine axis. Front. Endocrinol (2022). doi: 10.3389/fendo.2022.923475
91. Birrer SC, Reusch TBH, Roth O. Salinity change impairs pipefish immune defence. Fish Shellfish Immunol (2012) 33:1238–48. doi: 10.1016/j.fsi.2012.08.028
92. Choi K, Cope WG, Harms CA, Law JM. Rapid decreases in salinity, but not increases, lead to immune dysregulation in Nile tilapia, Oreochromis niloticus (L.). J Fish. Dis (2013) 36:389–99. doi: 10.1111/j.1365-2761.2012.01417.x
93. Nozzi V, Strofaldi S, Forner Piquer I, Di Crescenzo D, Olivotto I, Carnevali O. Amyloodinum ocellatum in Dicentrarchus labrax: Study of infection in salt water and freshwater aquaponics. Fish Shellfish Immunol (2016) 57:179–85. doi: 10.1016/j.fsi.2016.07.036
94. Pontigo JP, Vargas-Chacoff L. Growth hormone (GH) and growth hormone release factor (GRF) modulate the immune response in the SHK-1 cell line and leukocyte cultures of head kidney in Atlantic salmon. Gen Comp Endocrinol (2021) 300:113631. doi: 10.1016/j.ygcen.2020.113631
95. Yi CC, Liu C-H, Chuang K-P, Chang Y-T, Hu S-Y. A potential probiotic Chromobacterium aquaticum with bacteriocin-like activity enhances the expression of indicator genes associated with nutrient metabolism, growth performance and innate immunity against pathogen infections in zebrafish (Danio rerio). Fish Shellfish Immunol (2019) 93:124–34. doi: 10.1016/j.fsi.2019.07.042
96. Li K, Qiu H, Yan J, Shen X, Wei X, Duan M, et al. The involvement of TNF-alpha and TNF-beta as proinflammatory cytokines in lymphocyte mediated adaptive immunity of Nile tilapia by initiating apoptosis. Dev Comp Immunol (2021) 115:103884. doi: 10.1016/j.dci.2020.103884
97. Duan R, Wang N, Shang Y, Li H, Liu Q, Li L, et al. TNF-α (G-308A) polymorphism, circulating levels of TNF-α and IGF-1: Risk factors for ischemic stroke-an updated meta-analysis. Front Aging Neurosci (2022) 14:831910. doi: 10.3389/fnagi.2022.831910
98. Witkowska-Sędek E, Pyrżak B. Chronic inflammation and the growth hormone/insulin-like growth factor-1 axis. Cent. Eur J Immunol (2020) 45:469–75. doi: 10.5114/ceji.2020.103422
99. Alzaid A, Martin SAM, Macqueenb DJ. The complete salmonid IGF-IR gene repertoire and its transcriptional response to disease. Sci Rep (2016) 6:34806. doi: 10.1038/srep34806
100. El-Leithy AAA, Hemeda SA, Abd El Naby WSH, El Nahas AF, Hassan SAH, Awad ST, et al. Optimum salinity for Nile tilapia (Oreochromis niloticus) growth and mRNA transcripts of ion-regulation, inflammatory, stress- and immune-related genes. Fish Physiol Biochem (2019) 45:1217–32. doi: 10.1007/s10695-019-00640-7
Keywords: euryhaline teleost, growth hormone, insulin-like growth factors, cytokines, brain, pituitary, head kidney, spleen
Citation: Link K, Shved N, Serrano N, Akgül G, Caelers A, Faass O, Mouttet F, Raabe O, D’Cotta H, Baroiller J-F and Eppler E (2022) Effects of seawater and freshwater challenges on the Gh/Igf system in the saline-tolerant blackchin tilapia (Sarotherodon melanotheron). Front. Endocrinol. 13:976488. doi: 10.3389/fendo.2022.976488
Received: 23 June 2022; Accepted: 12 September 2022;
Published: 12 October 2022.
Edited by:
Emilio J. Vélez, Institut National de recherche pour l’agriculture, l’alimentation et l’environnement (INRAE), FranceReviewed by:
Pedro Miguel Guerreiro, University of Algarve, PortugalCopyright © 2022 Link, Shved, Serrano, Akgül, Caelers, Faass, Mouttet, Raabe, D’Cotta, Baroiller and Eppler. This is an open-access article distributed under the terms of the Creative Commons Attribution License (CC BY). The use, distribution or reproduction in other forums is permitted, provided the original author(s) and the copyright owner(s) are credited and that the original publication in this journal is cited, in accordance with accepted academic practice. No use, distribution or reproduction is permitted which does not comply with these terms.
*Correspondence: Elisabeth Eppler, ZWxpc2FiZXRoLmVwcGxlckB1bmliZS5jaA==
†Present address: Elisabeth Eppler, Institute of Anatomy and Cell Biology, Martin-Luther-University of Halle-Wittenberg, Halle (Saale), Germany
‡These authors share first authorship
§These authors share senior authorship
Disclaimer: All claims expressed in this article are solely those of the authors and do not necessarily represent those of their affiliated organizations, or those of the publisher, the editors and the reviewers. Any product that may be evaluated in this article or claim that may be made by its manufacturer is not guaranteed or endorsed by the publisher.
Research integrity at Frontiers
Learn more about the work of our research integrity team to safeguard the quality of each article we publish.