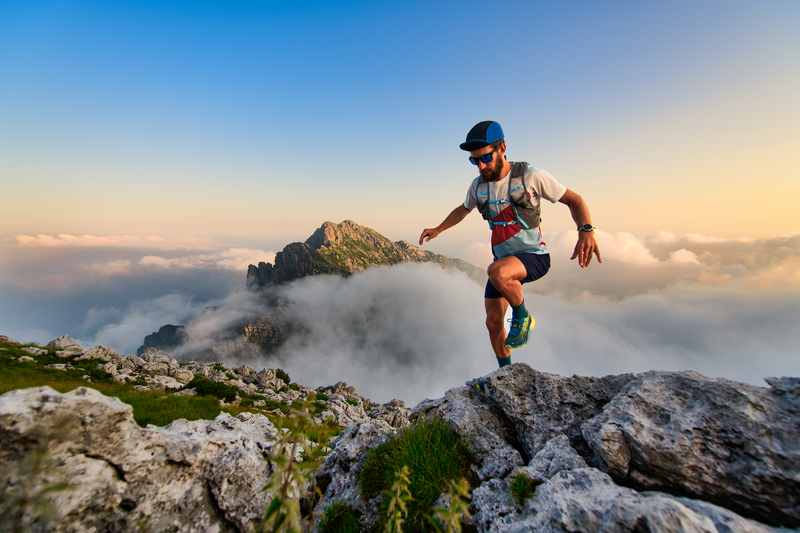
94% of researchers rate our articles as excellent or good
Learn more about the work of our research integrity team to safeguard the quality of each article we publish.
Find out more
REVIEW article
Front. Endocrinol. , 13 September 2022
Sec. Experimental Endocrinology
Volume 13 - 2022 | https://doi.org/10.3389/fendo.2022.975509
This article is part of the Research Topic Inter-Organ Communication Beyond Mammals: The Role of Tissue-Specific Cytokines View all 8 articles
Circadian rhythm, an innate 24-h biological clock, regulates several mammalian physiological activities anticipating daily environmental variations and optimizing available energetic resources. The circadian machinery is a complex neuronal and endocrinological network primarily organized into a central clock, suprachiasmatic nucleus (SCN), and peripheral clocks. Several small molecules generate daily circadian fluctuations ensuring inter-organ communication and coordination between external stimuli, i.e., light, food, and exercise, and body metabolism. As an orchestra, this complex network can be out of tone. Circadian disruption is often associated with obesity development and, above all, with diabetes and cardiovascular disease onset. Moreover, accumulating data highlight a bidirectional relationship between circadian misalignment and cardiometabolic disease severity. Food intake abnormalities, especially timing and composition of meal, are crucial cause of circadian disruption, but evidence from preclinical and clinical studies has shown that food could represent a unique therapeutic approach to promote circadian resynchronization. In this review, we briefly summarize the structure of circadian system and discuss the role playing by different molecules [from leptin to ghrelin, incretins, fibroblast growth factor 21 (FGF-21), growth differentiation factor 15 (GDF15)] to guarantee circadian homeostasis. Based on the recent data, we discuss the innovative nutritional interventions aimed at circadian re-synchronization and, consequently, improvement of cardiometabolic health.
Most organisms, from bacteria to plants and humans, coordinate their physiological function and behavior with the fluctuating environment in a 24-h daily cycle (1–3). This biological daily rhythm, called circadian rhythm from Latin words circa dies (meaning approximately day), is the adaptative response to Earth’s rotation in order to maximize the use of existing resources ensuring the survival. Light, referred also as photic zeitgeber (zeitgeber from German “time givers”), is the main external stimulus that regulates autonomous circadian oscillations (4), but food (5), exercise (6), and social activities (7) also act as zeitgeber (Figure 1). In humans, sleep–wake cycle, the best known circadian rhythm, is characterized by a long diurnal active period and a shorter nocturnal sleeping time (8).
Figure 1 Schematic representation of the circadian system. (A) Circadian system is a complex communication network that allows to combine different signaling, photic and no-photic zeitgebers, maximizing the use of resources and safeguarding the survival. Light, converted into a neural input by melanopsin and rod and cone photoreceptors, is the fundamental activator of central circadian pacemaker, suprachiasmatic nucleus (SCN). Melatonin, secreted by pineal gland, is a main mediator of SCN action, which is also influenced by food, exercise, and social activities (no-photic zeitgebers). Several biomolecules, synchronizing signal, ensure the bidirectional relationship between master clock and peripheral organs. (B) Circadian rhythm is regulated by a complex molecular system in master and peripheral clocks. The fundamental player of this complex molecular system is represented by heterodimeric complexes CLOCK-BMAL1 that enhances daytime expression of PER and CRY factors, which translocate into the nucleus and suppress CLOCK–BMAL1 activity. During the night, PER and CRY are degraded, and a new cycle begins. Additionally, REV-ERB and ROR are also circadian regulator that respectively represses and enhances BMAL1 expression. Additionally, sirtuins, whose activity is directly correlated to NAD+/NADH, regulate CLOCK-BMAL1 action. In addition, genetic components, gender, and age influence circadian rhythms and contribute to define individual circadian cycle. This complex network can break down, and circadian misalignment is an important risk factor for the development of cardiometabolic pathologies. Recent data highlight that nutrition could be a crucial metronome able to lead circadian re-synchronization: high-calorie breakfast associated with reduced food intake at dinner, supplementation with nutritional compounds, and, above all, time-restricted energy (TRE) could have beneficial action counteracting excessive weight gain and protection from cardiometabolic diseases (modified by Servier Medical Art by Servier is licensed under a Creative Commons Attribution 3.0 Unported License).
Individual differences in sleep–wake rhythm identify different chronotypes (9): morning chronotype (morningness) describes the preference to wake up early and to achieve physical and intellectual peak during the morning, while evening chronotype (eveningness) is typical of subjects that wake up late and prefer work in the later part of the day. Most individuals are characterized by an intermediate chronotype (10–12).
Emerging evidence has indicated that genetic component, gender, and age affect individual chronotype (13–15). In particular, numerous data obtained using animal models and performed clinical studies have demonstrated how, before menopause, women are more frequently associated with morning chronotypes (16, 17). Menopause is related to circadian abnormalities and sleep disturbance (18, 19).
Moreover, in developed countries, socioeconomic organization contributes to the modification of circadian rhythm. In details, the extension of working hours to night with shift work, the different time of food consumption, the artificial light, and, in the last two decades, the use of electronic media (internet/mobile phones/electronic gaming/on demand television) enhance the disruption of circadian cycle (20–22). A large number of individuals, adolescents and above post-adolescent individuals, usually prefer nocturnal activities during weekends, while during weekdays, they follow a different sleep–wake cycle (23–25). The result of this discrepancy between biological and social time, work, and free days is sleep deprivation. This condition, defined as “social jetlag” by Till Roenneberg, is an important cause involved in the onset of obesity and metabolic and cardiovascular diseases (26).
Emerging data highlight a bidirectional relationship between circadian misalignment and cardiometabolic health: circadian disruption promotes the onset of obesity and its comorbidities, including diabetes and cardiovascular diseases; on the other hand, these pathologies exacerbate circadian alterations creating a vicious cycle (27–29). The close interconnection between circadian system and cardiometabolic state assumes a communication network, capable of sending mutual feedbacks from circadian system to different organs and vice versa, exists (30–32). This communication network integrates peripheral signals, such as glucose levels, lipid absorption, or blood pressure oscillations, guaranteeing metabolic homeostasis (27).
In this review, we briefly analyzed the principal characteristics of the circadian system focusing our attention on biomolecules, in particular hormones, adipokines, and hepatokines, that join in the communication network and act as synchronizing signals that regulate circadian rhythm and eating. Moreover, we also discussed innovative nutritional strategies aiming at the re-synchronizing of the circadian clock and proposed as a new therapeutic approach to attenuate cardiometabolic pathologies.
The circadian system, responsible for rhythmicity and synchronization of physiological functions, is organized into two different compartments: the central clock, also called central circadian pacemaker or master clock, and several peripheral clocks that regulate daily physiological fluctuations of different tissues (32, 33).
Neurons and glia localized in the suprachiasmatic nucleus (SCN), a tiny region of the hypothalamus above the optic chiasm, play the role of the master clock (34). As previously reported, light represents the fundamental activator of SCN. Classically, melanopsin, a member of the G-protein-coupled receptor family, is a fundamental player involved in SCN activation. Indeed, melanopsin, expressed by photosensitive retinal ganglion cells, acts a photopigment and converts photic energy into a neural signal (4, 35). Recently though, different sophisticated studies have demonstrated that classic retinal photoreceptors, namely, rod and cone photoreceptors, are also implicated in transmission of photic information to SCN (36, 37). Accumulating data suggest that rod photoreceptors are responsible for the SCN stimulation under low light conditions, while input from cones to SCN is essential during twilight transitions, characterized by a shift to shorter wavelengths (38). Additionally, UV-sensitive cone photoreceptors induce SCN neuron response, without melanopsin and rod photoreceptor signaling (37). Therefore, the conversion of photic energy into a neural signal is a combined result of different inputs from all three photoreceptor classes, namely, melanopsin, rod, and cone (4) (Figure 1A).
A complex neural network, existing mainly between SCN and the other hypothalamic nuclei in addition to areas of the thalamus, midbrain, and hindbrain, facilities the integration of retina stimuli with other inputs, such as feeding, body temperature, and blood pressure alterations (8, 39, 40).
In human, SCN action is partially mediated by neurohormone melatonin, mainly secreted during darkness by pineal gland and for reason usually called night hormone. If the absence of light promotes melatonin production, melanopsin inhibits it (41). Light wavelength is a crucial parament for the inhibition action of melanopsin; indeed, artificial light inhibits it, while short light wavelength, i.e., blue light, promotes it (42–44). This aspect is extremely relevant considering that in our modern society, blue light is becoming progressively more prominent (45, 46).
Tissues rhythmicity is controlled not only by the SCN but also by specific peripheral clocks capable of combining SCN inputs with several different signals, including food intake, temperature, sympathetic and parasympathetic innervations, and endocrinological and inflammatory signaling (Figure 1A).
In both compartments, master and peripheral clocks, a complex transcription–translation feedback loops (TTFLs) generate the circadian rhythm (Figure 1B).
The core of this molecular machine is formed by two transcription factors present in all cells of the body and respectively called circadian locomotor output cycles kaput (CLOCK) and brain and muscle ARNT-like 1 (BMAL1) that bind to E-boxes in the promoter region of genes. Period circadian regulators 1–3 (PER1, ID:5187; PER2, ID:8864; and PER3, ID:8863) and cryptochrome circadian regulators 1 and 2 (CYR1, ID:1407; CYR2, ID:1408) enhance their transcription (47–50) (Figure 1B).
PER-CYR protein heterodimers translocate into the nucleus and repress CLOCK-BMAL1 activity. When PER and CYR levels are sufficiently low, heterodimers are degraded, and a new cycle restarts. In normal condition, during day/wake time, CLOCK-BMAL1 heterodimer is active, while in night/sleep time, CLOCK-BMAL1 complex is repressed (51). Different studies have pointed out how the cellular redox state, i.e., the ratio between the oxidized and reduced form of adenine dinucleotide (NAD+/NADH), regulating the deacetylase activity of sirtuins, plays a critical role in TTFLs system. SIRT1 and SIRT7 directly deacetylate BMAL1 and CRY, and their activity fluctuates daily (52–55). Moreover, CLOCK-BMAL1 also induces the expression of the nuclear receptors reverse erythroblastoma-erbα/β (REV-ERBα, ID:9572; REV-ERBβ, ID:9975) and retinoic-acid-related orphan genes (RORα, ID:6095, RORβ, ID:6096; and RORγ, ID:6097) that respectively act as inhibitor and activator of BMAL1 (ID:406) expression (56, 57). This complex molecular network controls the expression of several genes (Figure 1B).
Recent data indicate that in mammalians, 3%–16% of all mRNA display circadian expression and, in particular, 6%–10% hepatic mRNA and 10%–20% of white adipose mRNA have rhythmic daily expression (58–61). This circadian expression primarily affects hormones, enzymes, and transcription factors involved in macronutrient intake and metabolism, as comprehensively discussed by Brubaker et al. in a recent review (62).
Cyclic food accessibility acts as zeitgeber as light/dark cycle, and already at the beginning of the last century, Richter observed increased locomotion in rats during the hours preceding food intake (63). This phenomenon, called food anticipatory activity (FAA), is an important component of circadian rhythms but is not directly regulated by SCN activity, as demonstrated by studies performed using animal models with SCN ablation (64–66). FFA is generated by food-entrainable oscillators (FEOs) whose brain localization is unknown (67).
Moreover, food intake is mainly controlled by carbohydrates, fat, and protein, and by hedonic factors, from taste and appearance to social and emotional factors. Homeostatic stimuli generated by peripheral tissues are integrated by the arcuate nucleus (ARC), the periventricular nucleus (PVN), and the lateral hypothalamus, while mesolimbic circuits, formed mainly by the dopaminergic neurons of the ventral tegmental area (VTA) and the nucleus accumbens (NAc), receive hedonic inputs (39, 68–70). Thus, circadian food intake is a complex result of central signals produced not only by SCN neurons but also by FEOs and by peripheral energetic inputs.
Not only food intake but also numerous cardiac parameters have a peculiar daily rhythm: blood pressure and heart rate are characterized by daily fluctuations (71–73). Moreover, numerous epidemiological studies have revealed that various adverse cardiac events, i.e., myocardial ischemia, sudden cardiac death, and ventricular fibrillation, occur more frequently during the morning (74–76). At molecular levels, different components of the cardiovascular system express circadian genes from smooth muscle cells (77, 78) to cardiomyocytes (79, 80). In addition, different studies performed using murine models have indicated that about 13% of cardiac genes and 8% of cardiac proteins have a circadian expression pattern (81, 82). It is important to note that several data, obtained using mice having mutant circadian genes only in the heart, have demonstrated that heart rate, cardiac metabolism and contractility, and cardiac function are mainly synchronized by the cardiomyocyte circadian clock and not by SCN (83–86). But as observed by Scheer et al., natural light exposure raises sleeping heart rate, emphasizing the role of master clock on cardiac homeostasis (87). Moreover, Curtis et al. have demonstrated that in mice, BMAL1 gene knockout abolishes the circadian rhythm of blood pressure and contemporarily reduces production of catecholamines (88). Recently, Sedova et al. have tested melatonin integration as therapeutic treatment against ischemia/reperfusion injury (89).
In the next sub-sections, we will examine the action of main biomolecules involved in the circadian regulation of feeding and cardiometabolic processes (Figure 1).
Leptin, the most well-known adipokine, is mainly synthesized by white adipocytes and rhythmically secreted during the day with a peak during sleep/inactive phase (90, 91). Leptin’s circadian rhythm is controlled both by SCN, through sympathetic inputs to adipocytes (92); moreover, recently, Luo et al. have demonstrated how dopaminergic neurons localized at the peri-SCN area contribute to leptin expression (93). In addition, food intake regulates leptin secretion: while meal increases levels of this adipokine, fasting has an opposite effect. The correlation between nutritional status and leptin’s secretion reveals the main function of this hormone: suppressing hunger, as suggested by the Greek origin of its name “leptos,” namely, thin (94, 95). ARC, a major target of leptin, is a complex hypothalamic nucleus having a crucial role on different physiological processes, from feeding to reproduction. ARC functions are guaranteed by specialized neuron subtypes; in particular, control of feeding is mainly ensured by two different neuronal subpopulations having opposite role: proopiomelanocortin/cocaine-amphetamine-related transcript (POMC/CART)-positive neurons transmit anorexigenic inputs, while neuropeptide Y (NPY)-/agounti-related peptide (AgRP)-positive neurons regulate orexigenic stimulus. Leptin binding to its receptor inhibits NPY/AgRP neurons, whereas enhances POMC/CART activity triggering the secretion of anorexigenic peptide alpha-it melanocyte stimulating hormone (α-MSH) (96). Binding between melanocortin 4 receptor (MC4R), expressed on distinct second-order neurons in PVN, and α-MSH suppresses food intake (97). Moreover, leptin also influences hedonic response to food: Domingos et al. have proven that leptin inhibits mesolimbic dopamine neuronal activity, decreasing the reward value of sucrose (98), and recently, Omran et al. have proposed that leptin reduces the pleasure of food activating via inhibitory GABA neurons in VTA that directly regulate dopamine neurons (99). It is important to note that satiety induced by the melanocortin system is antagonized by NPY/AgRP neurons. As demonstrated by Ollmann et al., AgRP plays as a competitive antagonist of the MC4R (100).
Furthermore, various studies have highlighted the correlation between plasma leptin levels and hypertension development (101, 102). Interestingly, Han et al. have demonstrated that in normal mice, central administration of a leptin receptor antagonist abrogates diurnal rise of blood pressure, while in obese mice, leptin mediates the diurnal blood pressure elevation, promoting tumor necrosis factor-α (TNFα) signaling (103).
Not only leptin but also ghrelin, known as hunger hormone, affects ARC neurons regulating food intake and body composition (104). Ghrelin is primarily secreted by oxyntic cells of the gastric mucosa, and its action is due to acylation process that allows its binding to growth hormone secretagogue receptor (GHSR), highly expressed in ARC (104–106). However, it is important to note that GHSR is differently expressed in ARC neuronal populations: about 90% of NPY/AgRP expressed GHSR compared to ~10% POMC neurons (107, 108). Consequently, ghrelin mainly activates NPY/AgRP neurons promoting food intake (109). Plasma ghrelin levels continuously fluctuate during the day, and nutritional status is the main factor responsible of this fluctuation: fasting raises the activity of oxyntic cells, while food intake plays in opposite manner, decreasing ghrelin levels (105, 110, 111). In addition, in rodents and human, ghrelin’s peak is observed during the inactive phase, while in the active phase of circadian cycle, ghrelin is low (104, 112, 113).
In addition, different intestine biomolecules secreted after food intake influence circadian rhythm. In 1932, La Barre hypothesized the existence of these molecules and coined the word “incretin,” and about 40 years later, Dupre et al. discovered the duodenal glucose-dependent insulinotropic polypeptide, GIP, and only in 1987, Holst identified glucagon-like peptide-1 (GLP-1) (114–117). Both GIP and GLP-1 contribute to maintain diurnal euglycemia; GIP promotes insulin production, while GLP-1 also strongly reduces glucagon secretion (118). The circadian release of GLP-1 by enteroendocrine L cells is well established, and GLP-1 peak is observed in the morning at the onset of the active period (dark for mice and light for humans), while circadian GIP secretion by proximal K cells is partially controversial (119–121). Recently, the study performed by Martchenko et al. have demonstrated that in normal male and female mice, both GLP-1 and GIP release are characterized by 24-h rhythm, whereas in obese mice, circadian secretion of GLP-1 is fundamental to control insulin action (122, 123). However, the most important result of these works concerns the role played by intestinal microbiome: performing experiments using antibiotic-induced microbial depletion in germ-free mice, Martchenko et al. observed that diurnal GLP-1 release was regulated by intestinal microbiota (122). This observation is recently confirmed by the study coordinated by Grasset, which showed how GLP-1 release in mice is the result of a specific pattern of clock gene expression, in particular BMAL1, and oscillation of some ileum bacteria, including Lachnospiraceae (124). Additionally, Grasset et al. have proven the role of master clock on GLP-1 signal: the disruption of gut–brain axis by monolateral subdiaphragmatic vagotomy impairs diurnal rhythmicity of GLP-1 and consequently its action. It is important to note that GLP-1 crosses the blood–brain and, above all, that GLP-1 is synthetized by preproglucagon-neurons (PPG) in the nucleus of the solitary tract (125, 126). PPG neurons project to several nuclei that express the GLP-1 receptor, including hindbrain, hypothalamus, and mesolimbic brain areas. These nuclei are involved in reward circuit (127, 128), and thus, GLP-1 influences hedonic eating, reducing palatable food intake as reported in a recent review presented by Eren-Yazicioglu et al. (129). Furthermore, several emerging data, obtained from treated different animal models with GLP-1 agonists, suggest that GLP-1 signaling plays an important action in drug addiction, i.e., cocaine (130), amphetamine and alcohol (129). Data obtained from studying cocaine abuse are extremely remarkable (130), suggesting that cocaine activates PPG in the nucleus of the solitary tract by an increase in plasma and central corticosterone levels (131). Consequently, the GLP-1 receptor pathway in midbrain and forebrain areas is activated and acts as a negative-feedback response (132). Obviously, further studies focusing on the relationship between GLP-1 central signaling–corticosterone axis and seeking behaviors must be performed. In any case, GLP-1 signaling plays an important role in psychological stress responses.
In addition to adipokines and gastrokines, hepatokines are also mediators of the circadian rhythm. Different nutritional inputs affect the circadian secretion of fibroblast growth factor 21 (FGF-21), a hepatokine discovered in 2000, which is secreted by other tissues from adipose tissue to skeletal muscle (133, 134). Several researchers, including Yu et al., have observed that circulating FGF-21 levels have a characteristic diurnal rhythm in humans peaking around midnight (135, 136). As other biomolecules, food intake influences FGF-21 secretion; in particular, prolonged hunger, protein restriction, or carbohydrate-rich diets potently stimulate circulating FGF-21 in humans (137). Transcription factor peroxisome proliferator-activated receptor α (PPARα) is the main mediator of different nutritional states; in fact, PPARα binding FGF21 (ID: 26291) promotor region enhances its expression (138–140). Metabolically circulating FGF-21 stimulates insulin-dependent glucose uptake in peripheral tissues, preventing excessive hyperglycemia after food intake (141–143). Interestingly, several studies also performed using transgenic mice models have shown how FGF-21 decreased sweet and alcohol intake, acting on hypothalamic neurons and regulating food intake. Matsui et al. have revealed that FGF-21 acts as a sweet-intake inhibitor on hypothalamic oxytocin neurons (144), while Jensen-Cody et al. have demonstrated that FGF-21, after binding to its receptor, β-Klotho (KLB) co-receptor, activates glutamatergic neurons in the ventromedial hypothalamus (VMH), suppressing sweet intake (145). However, KLB receptor is also expressed in the SCN and in the dorsal vagal complex of the hindbrain, and Bookout et al., using different mouse models, have shown that the binding of FGF-21 to its receptor in the brain enhances an adaptive starvation response, characterized by an increased systemic corticosterone level, an alteration of metabolism and light/dark cycle activity (146). Additionally, Bookout et al. have observed that in the SCN, the expression of the neuropeptide vasopressin is suppressed by FGF-21 (146).
Vasopressin (AVP) is a neurotransmitter secreted by ~20% of SNC neurons, and AVP neurons are important components of circadian pacemaker (147–149). CLOCK/BMAL1 complex binds the AVP (ID:551) promoter region and induces AVP synthesis (150, 151), while CRY1 and CRY2 repress it (152). AVP function strictly correlates with metabolic and behavioral rhythm of the first hours of active and inactive phases. In fact, activation of SCN-AVP neurons, projecting to the organum vasculosum lamina terminalis, influences the anticipatory thirst prior to sleep (153). But above all, AVP promotes cortisol peak before awakening.
In humans, cortisol is secreted by adrenal glands in a circadian manner even if different stress conditions modify its secretion (154). In normal conditions, cortisol levels rise at the end of the sleep phase, reaching the peak in the first hour after waking; this crucial point is known as “cortisol awakening response,” underlining the role of cortisol in the beginning of the active phase (154, 155). Cortisol expression is directly regulated by adrenocorticotrophic hormone (ACTH) whose secretion is controlled by AVP and corticotropin-releasing hormone (CRH), produced by the hypothalamic paraventricular nucleus (156, 157). It is important to note that in both nocturnal and diurnal animals, AVP is released during the light period, but AVP action is different: in nocturnal animals, like mice, AVP inhibits CRH neurons, while in diurnal animals, AVP promotes them (158). Moreover, by a negative feedback mechanism, high cortisol levels inhibit CRH, AVP, and ACTH expression, whereas low cortisol levels act in opposite manner. This mechanism is altered by physical and psychological stress factors. The physiological role of cortisol is extremely well-structured, which involves not only energetic metabolism (breakdown of carbohydrates and inhibition of gluconeogenesis) but also immune processes and, above all, cardiac function (159–161).
Interestingly, in recent years, many researchers have correlated cardiac dysfunction to the circulating level of growth differentiation factor 15 (GDF15), a peptide hormone member of the transforming growth factor β superfamily, which is expressed in several tissues, from kidney to adipose tissue (162). Tsai et al. have observed that in normal state, GDF15 is expressed at a low concentration with a diurnal variation that is not directly related to meals (163), but as demonstrated by Zhao et al., circadian expression of GDF15 is correlated with inhibition of REV-ERBs (164). In pathological conditions, mainly characterized by inflammation state and mitochondrial dysfunction, GDF15 expression is overexpressed. High levels of GDF15 have been detected in obese subjects and in patients inflicted with different cardiovascular diseases (162, 165, 166). Accumulating data suggest a crucial role of GDF15 on the control of food intake. The first evidence of the possible correlation between weight and GDF15 has been obtained by studying individuals with advanced prostate cancer: in these subjects, high circulating GDF15 levels correlated with weight loss (167). In rats fed a high-fat diet, GDF15 treatment suppresses food intake, enhances body weight loss, and consequently ameliorates cardiometabolic condition (168). Accumulating data indicate that GDF15 modifies appetite through multiple systemic mechanisms, including changes in food preferences, gastric emptying, and nausea, but at cellular level, GDF15 action is exclusively mediated by GDNF family receptor α-like (GFRAL) (162, 169–173). Indeed, in GFRAL knockout mice, anorectic effects of GDF15 are inhibited; GFRAL−/− mice are hyperphagic under stressed conditions, refractory to the effects of recombinant human GDF15 on body weight, food intake, and glucose parameters and are resistant to chemotherapy-induced anorexia (171, 174). It is important to note that GFRAL is expressed in hindbrain neurons, specifically in the nucleus of the solitary tract and the area postrema (173–175). As demonstrated by Hsu et al., the parabrachial nucleus and the central amygdala, which are circuits that regulate food intake and body weight under stressed conditions, are the primary neural circuits that respond to GFRAL activation (176). Thus, it is possible to speculate that GDF15 is a stress biomarker. In line with this hypothesis, epidemiological data obtained by studying patients affected by chronic cardiac pathologies, i.e., hypertrophy and endothelial dysfunction, have demonstrated that a high level of GFD15 is a crucial biomarker associated with unfavorable prognosis (165, 166). In mouse models of ischaemia–reperfusion injury, GDF15 deficiency correlated with a major damage and cardiomyocyte apoptosis, while treatment with GDF15 counteracts ischaemia–reperfusion injury (177–179). This positive action of GDF15 treatment in obese and in ischemia-reperfused mice suggest that high levels of GDF15 play a compensatory role as recently suggested by Townsend et al., who demonstrated that the increase in hepatic GDF15 is associated with the energy stress response controlled by AMPK (180). Additionally, Patel et al. demonstrated that different nutritional stress conditions, such as prolonged high-fat diet or an amino acid imbalanced diet, induce a significant increase in circulating GDF15 levels (174). Recently, Miyake et al. observed that in adipocytes, GDF15 action is correlated with the eIF2α phosphorylation-dependent integrated stress response (ISR), a signaling pathway involved in the maintenance of cellular homeostasis exposed to different stresses (181). Finally, several data have shown that GDF15 are involved in mitochondrial and endoplasmic reticulum stress conditions in non-alcoholic steatohepatitis (182).
Like an orchestra, this complex system of communication can be out of tune: circadian misalignment is an important risk factor for the development of cardiometabolic pathologies (Figure 1).
As mentioned in Introduction, different causes induce a disharmony between central clock and peripheral clocks. Shift work, jetlag, and social jetlag are probably the most studied external factors able to disturb circadian rhythmicity (1, 7, 20, 22, 24, 26).
In addition, eating contributes to circadian disturbances (5). Yasumoto et al. have fed mice during their inactive phase, i.e., daytime for nocturnal animals like mice (daytime feeding with high-fat/high-sucrose diet, DF), and have observed desynchronization of peripheral clocks and, above all, obesity onset. Moreover, mice were affected by hepatic fat accumulation and impaired leptin signaling (183). Leptin signaling appears crucial in circadian misalignment induced by DF: Oishi et al. have shown that DF-induced metabolic damages were abolished in mice expressing mutations in leptin receptor (db/db mice) (184). In addition, not only the adipose tissue and liver are targets of DF, but the skeletal muscle is also injured: Abe et al. revealed that DF reduced skeletal muscle mass in mice (185).
Inappropriate eating behaviors disrupt cardiometabolic homeostasis also in humans. As previously reported, shifts workers are more predisposed to obesity, diabetes, and cardiovascular diseases than day workers (186). Additionally, data obtained by several population-cohort study have proven how a higher energy intake during evening/night increases the risk of metabolic syndrome and cardiovascular disease (187–191). During the coronavirus disease 2019 (COVID-19) pandemic, young people have accentuated this tendency to consume sweet foods/snacks in the evening hours. Furthermore, adolescents were exposed to lengthier screen time and have shown an inadequate sleeping pattern (192, 193). Woo et al. have identified that these changes in lifestyle behaviors are associated with weight gain and with worsening of some cardiometabolic markers, including triglycerides and leptin, in a cohort of children and adolescents with overweight and obesity (194).
In addition, subjects affected by night eating syndrome (evening hyperphagia/nocturnal ingestions) usually are characterized by circadian phase delays of leptin, cortisol, and melatonin. These abnormalities are associated with a higher risk of obesity and consequently of cardiovascular disease (195, 196). It is important to note that these patients prefer eating fast food and sugar-sweetened beverages (196).
In fact, not only the time of eating but also the composition of meal is involved in cardiometabolic damages (5, 197–199). In the past decades, studies performed using animal models have revealed that a high-fat diet alters the expression and cycling of circadian clock genes and clock-controlled genes involved in metabolic metabolism in different murine tissues, including the hypothalamus, liver, and adipose tissue (200–202).
In addition, the National Health and Nutrition Examination Survey 2003–2016 (NHANES) study, which included a total of 27,911 participants, showed that an excessive intake of low-quality carbohydrates and animal protein at dinner was significantly connected with higher cardiovascular disease risk (203, 204). Accumulating data have corroborated these observations in humans (205), and recently, Kessler et al. have demonstrated that a diet characterized by fat-rich meals until 13:30 and carbohydrate-rich meals between 16:30 and 22:00 worsens metabolic condition in subjects with impaired glucose metabolism (206).
Finally, it is important to note that dietary cues are bidirectionally correlated with gut microbiota, including bacteria, yeasts, and viruses. Firmicutes and Bacteroidetes species represent 90% of the gut microbiota even if Actinobacteria, Proteobacteria, Fusobacteria, and Verrucomicrobia bacteria are presented. As demonstrated by a large amount of data, unbalanced intestinal ecosystem, in particular the ratio between Firmicutes and Bacteroidetes, is related to obesity (207). Microbial components, primarily lipopolysaccharide, and specific microbial metabolites, short-chain fatty acids (SCFAs), and unconjugated bile acids, influence the expression of gene clocks in peripheral tissues and, above all, in intestinal epithelial cell (208, 209). Tahara et al. have demonstrated that oral administration of mixed SCFAs and lactate positively influences PER2 in peripheral tissues (210). Mukherji et al., after disrupting murine microbiota by antibiotics, have observed an overproduction of corticosterone, synthetized by intestinal epithelial cells, and an impaired expression of circadian genes. But above all, Mukherji et al. have determined that after a month, mice developed prediabetic syndrome (211). At the same time, feeding/starvation rhythm and food composition alter gut composition and consequently induced desynchronization (212). Mice fed a normal diet are characterized by cyclical fluctuation in gut microbial composition: Firmicutes species reach their abundant peak during dark/active phase, whereas Bacteroidetes and Verrucomicrobia species have an opposite cycle with peak during light/rest phase (213, 214). Conversely, mice fed a high-fat diet destroys microbial diurnal cyclical fluctuation, causing a drastic reduction in the diversity and variability of microbial communities. A high-fat diet modifies gut microbiota by enhancing Firmicutes species, which express more enzymes involved in carbohydrates and lipids metabolism (212, 213). In addition, a high-fat diet is associated with the production of microbially derived metabolites, especially short-chain fatty acids, that are able to increase the expression of BMAL1 gene in the hypothalamus, as also demonstrated by Leone et al. (215).
However, meal timing and composition is not only a problem for cardiometabolic homeostasis but also a fundamental solution. In fact, nutrition could be a crucial metronome that is able to lead circadian re-synchronization (Figure 1). Observational data have revealed that meal regularity and, in particular, high energy intake in the morning is associated with wellbeing outcomes (216). It is important to note that food timing also influences the metabolic state of obese subjects: as observed by Mazri et al., early temporal patterns of energy and macronutrient intake characterized obesity with healthy metabolic status (217). In diabetic patients, morning distribution of food intake, especially a carbohydrate-rich breakfast, accelerates weight loss, reduces appetite and craving, and thus improves metabolic parameters, such as postprandial glycemia and glycated hemoglobin (218–220). Moreover, Jakubowicz et al., after a nutrition study performed in 193 obese healthy patients, suggested that a high energy breakfast, primarily composed by carbohydrates and proteins, could prevent obesity relapse by decreasing hunger/craving and influencing ghrelin signaling. Molecular studies have highlighted that the absence of breakfast is associated with an inhibition of clock genes expression, in particular BMAL1 and PER genes, whereas a high-energy breakfast has an opposite effect (221, 222).
In the last decades, several researchers have studied in animal models the effects of time-restricted feeding (TRF), called time-restricted energy (TRE) if referring to humans, as therapeutic treatment aimed to re-synchronize and consequently prevent cardiometabolic damage. This daily nutritional intervention is characterized by the restriction of food consumption to certain hours, while the daily fasting period lasts >12 h without modifying nutrient quality or quantity (223, 224).
Using different animal models, several studies were performed to investigate TRF action. Usually, these experiments were designed to compare the metabolic state of mice having access to food (chow or high fat) ad libitum with mice having access to food restricted to 8–10 h. Chaix et al. studied TRF action on a particular knockout mouse, lacking whole-body CRY gene and BMAL1 and REV-ERBα/β in the liver. In both animal models, TRF diet protected mice from excessive weight gain and metabolic diseases. Moreover, TRF significantly reduced hepatic lipid accumulation and improved antioxidant cellular defenses. It is important to underline that these results indicate that TRF action is independent by clock gene expression (225). Accumulating data have revealed that also in mice fed a high-fat diet, TRF has shown beneficial effects counteracting excessive weight gain and protecting from metabolic diseases. TFR has improved nutrient utilization and energy expenditure, restoring expression oscillations of the circadian clock genes and daily rhythms of ghrelin and corticosterone (226, 227). In addition, TRF has improved murine gut microbiota, re-establishing cyclical variation in many families of bacteria including Lactobacillus family (228). In addition, in Drosophila melanogaster, this dietary approach ameliorates cardiometabolic state attenuating age-related cardiac deterioration (229) and enhances muscle performance by decreasing intramuscular fat deposits in obese model of this insect (230).
Interestingly, results obtained by human trials are encouraging (231). Meta-analysis performed by Moon et al. have shown how TRE positively influences body weight in overweight or obese patients, reducing fat mass and preserving fat-free mass, and ameliorates metabolic parameters, including blood pressure, fasting glucose concentration, and cholesterol profiles (232). Clinical trials performed by Sutton et al. corroborates TRE-induced cardiometabolic improvement even if, in this cohort of men with prediabetes, TRE did not modify weight (233). Similarly, Jones et al. obtained comparable results in healthy men treated with TRE for 2 weeks: they enhanced whole-body insulin sensitivity and skeletal muscle glucose and branched-chain amino acids uptake without modifying body weight (234).
Taken together, data obtained by human studies point out that TRE has several beneficial impacts on the cardiometabolic state in healthy and obese patients (235). Moreover, in obese patients TRE seems to promote body composition remodeling, emphasizing food timing–circadian cycle interconnection. It is important to note that results obtained by human trials have shown that TRE is safe and well-tolerated with good adherence (235).
Additionally, nutraceutical compounds or functional foods could represent an effective strategy to normalize circadian rhythm. The action of polyphenols, in particular resveratrol, in cardiometabolic pathologies are well investigated by in vitro and in vivo models (236–238). Moreover, resveratrol and other polyphenols are able to modify gut composition, ameliorating metabolic abnormalities, including liver steatosis and insulin resistance (239, 240). But above all, resveratrol is able to activate SIRT1, an important mediator of circadian molecular pathway, as previous described (241, 242). Based on these data, researchers have tested the possible action of resveratrol, and in general polyphenols, on re-synchronization (243). As previously reported, these studies have been performed in vitro or using different animal models; not rarely, resveratrol is tested in association with other nutraceuticals. For these reasons, many aspects correlated to therapeutic use of resveratrol as chronobiotic agent are not completed clarified, i.e., molecular mechanism on circadian genes or the effective dose. In any case, the preliminary data are more promising. Li et al. observed that resveratrol mitigates impaired intracellular lipid metabolism in a BMAL1-dependent manner in hepatocytes (244). In addition, Sun et al. investigated the effects of resveratrol supplementation on high-fat-diet-induced disorders in mice, observing a significative decrease in body weight and a rhythmic restoration of fasting blood glucose and leptin associated with different expression pattern of CLOCK, BMAL1, and PER2 genes (245). Similar results have been obtained by Koh et al., who treated jetlagged mice with pterostilbene and resveratrol. In these animals, the combination of pterostilbene and resveratrol improved gut diversity (246).
Considering the relationship between gut and circadian rhythms, recently, several authors have begun to examine prebiotic supplementation as dietary approach for mitigating circadian misalignment. In obese mice exposed to weekly shifted light–dark cycle, β-glucan and inulin supplementation have restored the expression and phase of circadian-clock genes (247). Comparable effects have been achieved using oat β-glucan, which is able to improve microbial gut diversity and to counteract body weight gain and leptin signaling alteration restoring glucose tolerance (248). Obviously, there are other nutraceutical compounds that could influence circadian machine regulating intestinal flora and bioactive molecules secretion (249). Recently, Huang et al. proposed several nutraceutical molecules as chronobiotics (250): first data are promising but, as previously reported, were mainly obtained in vitro and in animal models, and therefore, it will be necessary to perform robust human studies especially in order to evaluate the bioavailability of these compounds, for example, resveratrol and other polyphenols characterized by low bioavailability. Moreover, it will be important to design closer investigations for specific diseases, such as different cardiovascular pathologies, to better understand extensive therapeutic action of different nutraceutical supplements.
Growing evidence demonstrates that circadian rhythm is a crucial aspect to ensure cardiometabolic health. Circadian rhythm is a complex network able to interconnect different signaling: from photic zeitgeber to food, exercise, and social behaviors. Bioactive compounds secreted by tissues are mainly responsible for daily inter-organ communication. However, as an orchestra, this system can be out of tune leading to obesity and metabolic and cardiovascular diseases development. Eating, mainly altered meal timing and composition, causes circadian dissonance. Recently, however, interesting studies seem to suggest that eating could play as a chrono-regulator promoting re-synchronizing circadian rhythm. Data obtained mainly using in vitro and animal models indicate that high-calorie breakfast associated with reduced food intake at dinner or supplementation with nutritional compounds could regulate the expression of circadian clock transcription factor or the composition of gut microbiota. But above all, TRE could counteract circadian misalignment beneficially influencing cardiometabolic state. The results obtained from the first human trials are encouraging and support the hypothesis that specific nutritional treatments could positively influence circadian misalignment: high-energy breakfast and TRE have beneficial effects and, above all, are well accepted by patients.
Nevertheless, considering the short duration of the clinical trials and/or the small sample size of enrolled subjects, further studies will be indispensable to well establish the clinical efficacy of different nutritional interventions and to standardize the treatment protocols. To this regard, clinical trials based on the use of mobile app should be encouraged considering the rapid development of e-health in nutrition clinical practice.
Even if many aspects of the relationship between circadian rhythm, secreted biomolecules, and nutrition have yet to be elucidated, the translation of ancient maxim, “Eat breakfast like a king, lunch like a prince and dinner like a pauper,” in daily nutritional therapy could represent an additional medical intervention in the management of cardiometabolic pathologies.
PS and IT designed the article. PS wrote the manuscript. AF and LL revised the manuscript. All authors contributed to the article and approved the submitted and the published version.
This work has been supported by Ministry of Health—Ricerca Corrente—IRCCS MultiMedica.
The authors declare that the research was conducted in the absence of any commercial or financial relationships that could be construed as a potential conflict of interest.
All claims expressed in this article are solely those of the authors and do not necessarily represent those of their affiliated organizations, or those of the publisher, the editors and the reviewers. Any product that may be evaluated in this article, or claim that may be made by its manufacturer, is not guaranteed or endorsed by the publisher.
SCN, suprachiasmatic nucleus; TTFLs, transcription–translation feedback loops; CLOCK, circadian locomotor output cycles kaput; BMAL1, brain and muscle ARNT-like 1; PER1–3, period circadian regulators 1–3; CYR1/2, cryptochrome circadian regulators 1 and 2; REV-ERBs, nuclear receptors reverse erythroblastoma-erbα/β; ROR, retinoic-acid-related orphan; NAD+, oxidized form of adenine dinucleotide; NADH, reduced form of adenine dinucleotide; FAA, food anticipatory activity; FEOs, food-entrainable oscillators; ARC, arcuate nucleus; PVN, periventricular nucleus; VTA, ventral tegmental area; NAc, nucleus accumbens; POMC/CART, POMC-/cocaine-amphetamine-related transcript (CART); NPY/AgRP, neuropeptide Y/agounti-related peptide; α-MSH, alpha-melanocyte stimulating hormone; MC4R, melanocortin 4 receptor; TNFα, tumor necrosis factor-α; GHSR, growth hormone secretagogue receptor; GIP, duodenal glucose-dependent insulinotropic polypeptide; GLP-1, glucagon-like peptide-1; PPG, preproglucagon-neurons; FGF-21, fibroblast growth factor 21; PPARα, peroxisome proliferator-activated receptor α; KLB, β-Klotho co-receptor; VMH, ventromedial hypothalamus; AVP, vasopressin; ACTH, adrenocorticotrophic hormone; CRH, corticotropin-releasing hormone; GDF15, growth differentiation factor 15; GFRAL, GDNF family receptor α-like; SCFAs, short-chain fatty acids; TRF, time-restricted feeding; TRE, time-restricted energy.
1. Patke A, Young MW, Axelrod S. Molecular mechanisms and physiological importance of circadian rhythms. Nat Rev Mol Cell Biol (2020) 21(2):67–84. doi: 10.1038/s41580-019-0179-2
2. Woelfle MA, Ouyang Y, Phanvijhitsiri K, Johnson CH. The adaptive value of circadian clocks: an experimental assessment in cyanobacteria. Curr Biol (2004) 14(16):1481–6. doi: 10.1016/j.cub.2004.08.023
3. Young MW, Kay SA. Time zones: a comparative genetics of circadian clocks. Nat Rev Genet (2001) 2(9):702–15. doi: 10.1038/35088576
4. Ashton A, Foster RG, Jagannath A. Photic entrainment of the circadian system. Int J Mol Sci (2022) 23(2):729. doi: 10.3390/ijms23020729
5. Boege HL, Bhatti MZ, St-Onge MP. Circadian rhythms and meal timing: impact on energy balance and body weight. Curr Opin Biotechnol (2021) 70:1–6. doi: 10.1016/j.copbio.2020.08.009
6. Dollet L, Zierath JR. Interplay between diet, exercise and the molecular circadian clock in orchestrating metabolic adaptations of adipose tissue. J Physiol (2019) 597(6):1439–50. doi: 10.1113/JP276488
7. Fernandes P, Pereira LM, Horta NAC, Cardoso TSR, Coimbra CC, Szawka RE, et al. Social interaction masking contributes to changes in the activity of the suprachiasmatic nucleus and impacts on circadian rhythms. Physiol Behav (2021) 237:113420. doi: 10.1016/j.physbeh.2021.113420
8. Ono D, Yamanaka A. Hypothalamic regulation of the sleep/wake cycle. Neurosci Res (2017) 118:74–81. doi: 10.1016/j.neures.2017.03.013
9. Montaruli A, Castelli L, Mulè A, Scurati R, Esposito F, Galasso L, et al. Biological rhythm and chronotype: New perspectives in health. Biomolecules (2021) 11(4):487. doi: 10.3390/biom11040487
10. Monk TH, Buysse DJ, Potts JM, DeGrazia JM, Kupfer DJ. Morningness-eveningness and lifestyle regularity. Chronobiol Int (2004) 21(3):435–43. doi: 10.1081/cbi-120038614
11. Cox RC, Olatunji BO. Eveningness predicts negative affect following sleep restriction. Behav Ther (2021) 52(4):797–805. doi: 10.1016/j.beth.2020.10.004
12. Bakotic M, Radosevic-Vidacek B, Koscec Bjelajac A. Morningness-eveningness and daytime functioning in university students: the mediating role of sleep characteristics. J Sleep Res (2017) 26(2):210–8. doi: 10.1111/jsr.12467
13. Lenneis A, Vainik U, Teder-Laving M, Ausmees L, Lemola S, Allik J, et al. Personality traits relate to chronotype at both the phenotypic and genetic level. J Pers. (2021) 89(6):1206–22. doi: 10.1111/jopy.12645
14. Kim KM, Han SM, Heo K, Kim WJ, Chu MK. Sex differences in the association between chronotype and risk of depression. Sci Rep (2020) 10(1):18512. doi: 10.1038/s41598-020-75724-z
15. Höller Y, Gudjónsdottir BE, Valgeirsdóttir SK, Heimisson GT. The effect of age and chronotype on seasonality, sleep problems, and mood. Psychiatry Res (2021) 297:113722. doi: 10.1016/j.psychres.2021.113722
16. Díaz-Morales JF, Parra-Robledo Z. Age and sex differences in Morningness/Eveningness along the life span: A cross-sectional study in Spain. J Genet Psychol (2018) 179(2):71–84. doi: 10.1080/00221325.2018.1424706
17. Randler C. Age and gender differences in morningness-eveningness during adolescence. J Genet Psychol (2011) 172(3):302–8. doi: 10.1080/00221325.2010.535225
18. Meliska CJ, Martínez LF, López AM, Sorenson DL, Nowakowski S, Parry BL. Relationship of morningness-eveningness questionnaire score to melatonin and sleep timing, body mass index and atypical depressive symptoms in peri- and post-menopausal women. Psychiatry Res (2011) 188(1):88–95. doi: 10.1016/j.psychres.2010.12.010
19. Baker FC, Lampio L, Saaresranta T, Polo-Kantola P. Sleep and sleep disorders in the menopausal transition. Sleep Med Clin (2018) 13(3):443–56. doi: 10.1016/j.jsmc.2018.04.011
20. Wickwire EM, Geiger-Brown J, Scharf SM, Drake CL. Shift work and shift work sleep disorder: Clinical and organizational perspectives. Chest. (2017) 151(5):1156–72. doi: 10.1016/j.chest.2016.12.007
21. Aguilar-Carrasco MT, Domínguez-Amarillo S, Acosta I, Sendra JJ. Indoor lighting design for healthier workplaces: natural and electric light assessment for suitable circadian stimulus. Opt Express. (2021) 29(19):29899–917. doi: 10.1364/OE.430747
22. Bae SA, Fang MZ, Rustgi V, Zarbl H, Androulakis IP. At The interface of lifestyle, behavior, and circadian rhythms: Metabolic implications. Front Nutr (2019) 6:132. doi: 10.3389/fnut.2019.00132
23. Cabeza de Baca T, Chayama KL, Redline S, Slopen N, Matsushita F, Prather AA, et al. Sleep debt: the impact of weekday sleep deprivation on cardiovascular health in older women. Sleep (2019) 42(10):zsz149. doi: 10.1093/sleep/zsz149
24. Touitou Y, Touitou D, Reinberg A. Disruption of adolescents' circadian clock: The vicious circle of media use, exposure to light at night, sleep loss and risk behaviors. J Physiol Paris (2016) 110(4 Pt B):467–79. doi: 10.1016/j.jphysparis.2017.05.001
25. Lin CY, Imani V, Griffiths MD, Broström A, Nygårdh A, Demetrovics Z, et al. Temporal associations between morningness/eveningness, problematic social media use, psychological distress and daytime sleepiness: Mediated roles of sleep quality and insomnia among young adults. J Sleep Res (2021) 30(1):e13076. doi: 10.1111/jsr.13076
26. Wittmann M, Dinich J, Merrow M, Roenneberg T. Social jetlag: misalignment of biological and social time. Chronobiol Int (2006) 23(1-2):497–509. doi: 10.1080/07420520500545979
27. Poggiogalle E, Jamshed H, Peterson CM. Circadian regulation of glucose, lipid, and energy metabolism in humans. Metabolism. (2018) 84:11–27. doi: 10.1016/j.metabol.2017.11.017
28. Chellappa SL, Vujovic N, Williams JS, Scheer FAJL. Impact of circadian disruption on cardiovascular function and disease. Trends Endocrinol Metab (2019) 30(10):767–79. doi: 10.1016/j.tem.2019.07.008
29. Lemmer B, Oster H. The role of circadian rhythms in the hypertension of diabetes mellitus and the metabolic syndrome. Curr Hypertens Rep (2018) 20(5):43. doi: 10.1007/s11906-018-0843-5
30. Li Y, Ma J, Yao K, Su W, Tan B, Wu X, et al. Circadian rhythms and obesity: Timekeeping governs lipid metabolism. J Pineal Res (2020) 69(3):e12682. doi: 10.1111/jpi.12682
31. Rácz B, Dušková M, Stárka L, Hainer V, Kunešová M. Links between the circadian rhythm, obesity and the microbiome. Physiol Res (2018) 67(Suppl 3):S409–20. doi: 10.33549/physiolres.934020
32. Schibler U, Gotic I, Saini C, Gos P, Curie T, Emmenegger Y, et al. Clock-talk: Interactions between central and peripheral circadian oscillators in mammals. Cold Spring Harb Symp Quant Biol (2015) 80:223–32. doi: 10.1101/sqb.2015.80.027490
33. Kolbe I, Brehm N, Oster H. Interplay of central and peripheral circadian clocks in energy metabolism regulation. J Neuroendocrinol. (2019) 31(5):e12659. doi: 10.1111/jne.12659
34. Mieda M. The central circadian clock of the suprachiasmatic nucleus as an ensemble of multiple oscillatory neurons. Neurosci Res (2020) 156:24–31. doi: 10.1016/j.neures.2019.08.003
35. Hattar S, Liao HW, Takao M, Berson DM, Yau KW. Melanopsin-containing retinal ganglion cells: architecture, projections, and intrinsic photosensitivity. Science. (2002) 295(5557):1065–70. doi: 10.1126/science.1069609
36. van Diepen HC, Ramkisoensing A, Peirson SN, Foster RG, Meijer JH. Irradiance encoding in the suprachiasmatic nuclei by rod and cone photoreceptors. FASEB J (2013) 27(10):4204–12. doi: 10.1096/fj.13-233098
37. van Diepen HC, Schoonderwoerd RA, Ramkisoensing A, Janse JAM, Hattar S, Meijer JH. Distinct contribution of cone photoreceptor subtypes to the mammalian biological clock. Proc Natl Acad Sci U S A. (2021) 118(22):e2024500118. doi: 10.1073/pnas.2024500118
38. Drouyer E, Rieux C, Hut RA, Cooper HM. Responses of suprachiasmatic nucleus neurons to light and dark adaptation: relative contributions of melanopsin and rod-cone inputs. J Neurosci (2007) 27(36):9623–31. doi: 10.1523/JNEUROSCI.1391-07.2007
39. Van Drunen R, Eckel-Mahan K. Circadian rhythms of the hypothalamus: From function to physiology. Clocks Sleep. (2021) 3(1):189–226. doi: 10.3390/clockssleep3010012
40. Chrobok L, Northeast RC, Myung J, Cunningham PS, Petit C, Piggins HD. Timekeeping in the hindbrain: a multi-oscillatory circadian centre in the mouse dorsal vagal complex. Commun Biol (2020) 3(1):225. doi: 10.1038/s42003-020-0960-y
41. Prayag AS, Najjar RP, Gronfier C. Melatonin suppression is exquisitely sensitive to light and primarily driven by melanopsin in humans. J Pineal Res (2019) 66(4):e12562. doi: 10.1111/jpi.12562
42. Mouland JW, Martial F, Watson A, Lucas RJ, Brown TM. Cones support alignment to an inconsistent world by suppressing mouse circadian responses to the blue colors associated with twilight. Curr Biol (2019) 29(24):4260–4267.e4. doi: 10.1016/j.cub.2019.10.028
43. Schoonderwoerd RA, de Rover M, Janse JAM, Hirschler L, Willemse CR, Scholten L, et al. The photobiology of the human circadian clock. Proc Natl Acad Sci U S A. (2022) 119(13):e2118803119. doi: 10.1073/pnas.2118803119
44. Brainard GC, Hanifin JP, Warfield B, Stone MK, James ME, Ayers M, et al. Short-wavelength enrichment of polychromatic light enhances human melatonin suppression potency. J Pineal Res (2015) 58(3):352–61. doi: 10.1111/jpi.12221
45. Prayag AS, Münch M, Aeschbach D, Chellappa SL, Gronfier C. Light modulation of human clocks, wake, and sleep. Clocks Sleep. (2019) 1(1):193–208. doi: 10.3390/clockssleep1010017
46. Wahl S, Engelhardt M, Schaupp P, Lappe C, Ivanov IV. The inner clock-blue light sets the human rhythm. J Biophotonics. (2019) 12(12):e201900102. doi: 10.1002/jbio.201900102
47. Tamaru T, Takamatsu K. Circadian modification network of a core clock driver BMAL1 to harmonize physiology from brain to peripheral tissues. Neurochem Int (2018) 119:11–6. doi: 10.1016/j.neuint.2017.12.013
48. Gekakis N, Staknis D, Nguyen HB, Davis FC, Wilsbacher LD, King DP, et al. Role of the CLOCK protein in the mammalian circadian mechanism. Science. (1998) 280(5369):1564–9. doi: 10.1126/science.280.5369.1564
49. Ray S, Valekunja UK, Stangherlin A, Howell SA, Snijders AP, Damodaran G, et al. Circadian rhythms in the absence of the clock gene Bmal1. Science. (2020) 367(6479):800–6. doi: 10.1126/science.aaw7365
50. Takahashi JS. Transcriptional architecture of the mammalian circadian clock. Nat Rev Genet (2017) 18(3):164–79. doi: 10.1038/nrg.2016.150
51. Trott AJ, Menet JS. Regulation of circadian clock transcriptional output by CLOCK:BMAL1. PloS Genet (2018) 14(1):e1007156. doi: 10.1371/journal.pgen.1007156
52. Isobe Y, Hida H, Nishino H. Circadian rhythm of metabolic oscillation in suprachiasmatic nucleus depends on the mitochondrial oxidation state, reflected by cytochrome c oxidase and lactate dehydrogenase. J Neurosci Res (2011) 89(6):929–35. doi: 10.1002/jnr.22609
53. Nakahata Y, Sahar S, Astarita G, Kaluzova M, Sassone-Corsi P. Circadian control of the NAD+ salvage pathway by CLOCK-SIRT1. Science. (2009) 324(5927):654–7. doi: 10.1126/science.1170803
54. Liu J, Zhou B, Yan M, Huang R, Wang Y, He Z, et al. CLOCK and BMAL1 regulate muscle insulin sensitivity via SIRT1 in Male mice. Endocrinology. (2016) 157(6):2259–69. doi: 10.1210/en.2015-2027
55. Liu Z, Qian M, Tang X, Hu W, Sun S, Li G, et al. SIRT7 couples light-driven body temperature cues to hepatic circadian phase coherence and gluconeogenesis. Nat Metab (2019) 1(11):1141–56. doi: 10.1038/s42255-019-0136-6
56. Duez H, Staels B. The nuclear receptors rev-erbs and RORs integrate circadian rhythms and metabolism. Diabetes Vasc Dis Res (2008) 5(2):82–8. doi: 10.3132/dvdr.2008.0014
57. Guillaumond F, Dardente H, Giguère V, Cermakian N. Differential control of Bmal1 circadian transcription by REV-ERB and ROR nuclear receptors. J Biol Rhythms. (2005) 20(5):391–403. doi: 10.1177/0748730405277232
58. Mavroudis PD, DuBois DC, Almon RR, Jusko WJ. Daily variation of gene expression in diverse rat tissues. PloS One (2018) 13(5):e0197258. doi: 10.1371/journal.pone.0197258
59. Guan D, Lazar M. Circadian regulation of gene expression and metabolism in the liver. Semin Liver Dis (2022) 1131–121. doi: 10.1055/a-1792-4240.
60. van der Spek R, Fliers E, la Fleur SE, Kalsbeek A. Daily gene expression rhythms in rat white adipose tissue do not differ between subcutaneous and intra-abdominal depots. Front Endocrinol (Lausanne). (2018) 9:206. doi: 10.3389/fendo.2018.00206
61. Taira A, Arita E, Matsumoto E, Oohira A, Iwase K, Hiwasa T, et al. Systemic oscillator-driven and nutrient-responsive hormonal regulation of daily expression rhythms for gluconeogenic enzyme genes in the mouse liver. Chronobiol Int (2019) 36(5):591–615. doi: 10.1080/07420528.2019.1570246
62. Brubaker PL, Martchenko A. Metabolic homeostasis: It's all in the timing. Endocrinology (2022) 163(1):bqab199. doi: 10.1210/endocr/bqab199
63. Richter CP. A behavioristic study of the activity of the rat. Comp Psychol Monogr (1922) 1, 2:56. doi: 10.5962/bhl.title.151527
64. Engin A. Circadian rhythms in diet-induced obesity. Adv Exp Med Biol (2017) 960:19–52. doi: 10.1007/978-3-319-48382-5_2
65. de Lartigue G, McDougle M. Dorsal striatum dopamine oscillations: Setting the pace of food anticipatory activity. Acta Physiol (Oxf). (2019) 225(1):e13152. doi: 10.1111/apha.13152
66. Wilcox AG, Bains RS, Williams D, Joynson E, Vizor L, Oliver PL, et al. Zfhx3-mediated genetic ablation of the SCN abolishes light entrainable circadian activity while sparing food anticipatory activity. iScience. (2021) 24(10):103142. doi: 10.1016/j.isci.2021.103142
67. Pendergast JS, Yamazaki S. The mysterious food-entrainable oscillator: Insights from mutant and engineered mouse models. J Biol Rhythms. (2018) 33(5):458–74. doi: 10.1177/0748730418789043
68. Schwartz MW, Woods SC, Porte D Jr, Seeley RJ, Baskin DG. Central nervous system control of food intake. Nature. (2000) 404(6778):661–71. doi: 10.1038/s41574-019-0210-x
69. Cifuentes L, Acosta A. Homeostatic regulation of food intake. Clin Res Hepatol Gastroenterol (2022) 46(2):101794. doi: 10.1016/j.clinre.2021.101794
70. Ulrich-Lai YM, Fulton S, Wilson M, Petrovich G, Rinaman L. Stress exposure, food intake and emotional state. Stress. (2015) 18(4):381–99. doi: 10.3109/10253890.2015.1062981
71. Gamble KL, Berry R, Frank SJ, Young ME. Circadian clock control of endocrine factors. Nat Rev Endocrinol (2014) 10(8):466–75. doi: 10.1038/nrendo.2014.78
72. Zhang J, Sun R, Jiang T, Yang G, Chen L. Circadian blood pressure rhythm in cardiovascular and renal health and disease. Biomolecules. (2021) 11(6):868. doi: 10.3390/biom11060868
73. Sinha M, Behera AK, Sinha R, Parganiha A, Pande B, Sharma R, et al. Circadian rhythmicity of heart rate variability and its impact on cardiac autonomic modulation in asthma. Chronobiol Int (2021) 38(11):1631–9. doi: 10.1080/07420528.2021.1938595
74. du Pré B, Van Veen T, Crnko S, Vos M, Deddens J, Doevendans P, et al. Variation within variation: Comparison of 24-h rhythm in rodent infarct size between ischemia reperfusion and permanent ligation. Int J Mol Sci (2017) 18(8):1670. doi: 10.3390/ijms18081670
75. Mahmoud KD, de Smet BJ, Zijlstra F, Rihal CS, Holmes DR Jr.Sudden cardiac death: epidemiology, circadian variation, and triggers. Curr Probl Cardiol (2011) 36(2):56–80. doi: 10.1016/j.cpcardiol.2011.01.002
76. Black N, D'Souza A, Wang Y, Piggins H, Dobrzynski H, Morris G, et al. Circadian rhythm of cardiac electrophysiology, arrhythmogenesis, and the underlying mechanisms. Heart Rhythm. (2019) 16(2):298–307. doi: 10.1016/j.hrthm.2018.08.026
77. McNamara P, Seo SB, Rudic RD, Sehgal A, Chakravarti D, FitzGerald GA. Regulation of CLOCK and MOP4 by nuclear hormone receptors in the vasculature: a humoral mechanism to reset a peripheral clock. Cell. (2001) 105(7):877–89. doi: 10.1016/s0092-8674(01)00401-9
78. Davidson AJ, London B, Block GD, Menaker M. Cardiovascular tissues contain independent circadian clocks. Clin Exp Hypertens (2005) 27(2-3):307–11. doi: 10.1081/CEH-48933
79. Durgan DJ, Hotze MA, Tomlin TM, Egbejimi O, Graveleau C, Abel ED, et al. The intrinsic circadian clock within the cardiomyocyte. Am J Physiol Heart Circ Physiol (2005) 289(4):H1530–41. doi: 10.1152/ajpheart.00406.2005
80. Dierickx P, Vermunt MW, Muraro MJ, Creyghton MP, Doevendans PA, van Oudenaarden A, et al. Circadian networks in human embryonic stem cell-derived cardiomyocytes. EMBO Rep (2017) 18(7):1199–212. doi: 10.15252/embr.201743897
81. Martino TA, Tata N, Belsham DD, Chalmers J, Straume M, Lee P, et al. Disturbed diurnal rhythm alters gene expression and exacerbates cardiovascular disease with rescue by resynchronization. Hypertension. (2007) 49(5):1104–13. doi: 10.1161/HYPERTENSIONAHA.106.083568
82. Podobed P, Pyle WG, Ackloo S, Alibhai FJ, Tsimakouridze EV, Ratcliffe WF, et al. The day/night proteome in the murine heart. Am J Physiol Regul Integr Comp Physiol (2014) 307(2):R121–37. doi: 10.1152/ajpregu.00011.2014
83. Bray MS, Shaw CA, Moore MW, Garcia RA, Zanquetta MM, Durgan DJ, et al. Disruption of the circadian clock within the cardiomyocyte influences myocardial contractile function, metabolism, and gene expression. Am J Physiol Heart Circ Physiol (2008) 294(2):H1036–47. doi: 10.1152/ajpheart.01291.2007
84. Qiu Z, Ming H, Lei S, Zhou B, Zhao B, Yu Y, et al. Roles of HDAC3-orchestrated circadian clock gene oscillations in diabetic rats following myocardial ischaemia/reperfusion injury. Cell Death Dis (2021) 12(1):43. doi: 10.1038/s41419-020-03295-y
85. Durgan DJ, Tsai JY, Grenett MH, Pat BM, Ratcliffe WF, Villegas-Montoya C, et al. Evidence suggesting that the cardiomyocyte circadian clock modulates responsiveness of the heart to hypertrophic stimuli in mice. Chronobiol Int (2011) 28(3):187–203. doi: 10.3109/07420528.2010.550406
86. Schroder EA, Burgess DE, Zhang X, Lefta M, Smith JL, Patwardhan A, et al. The cardiomyocyte molecular clock regulates the circadian expression of Kcnh2 and contributes to ventricular repolarization. Heart Rhythm. (2015) 12(6):1306–14. doi: 10.1016/j.hrthm.2015.02.019
87. Scheer FA, van Doornen LJ, Buijs RM. Light and diurnal cycle affect human heart rate: possible role for the circadian pacemaker. J Biol Rhythms. (1999) 14(3):202–12. doi: 10.1177/074873099129000614
88. Curtis AM, Cheng Y, Kapoor S, Reilly D, Price TS, Fitzgerald GA. Circadian variation of blood pressure and the vascular response to asynchronous stress. Proc Natl Acad Sci U S A. (2007) 104(9):3450–5. doi: 10.1073/pnas.0611680104
89. Sedova KA, Bernikova OG, Cuprova JI, Ivanova AD, Kutaeva GA, Pliss MG, et al. Association between antiarrhythmic, electrophysiological, and antioxidative effects of melatonin in Ischemia/Reperfusion. Int J Mol Sci (2019) 20(24):6331. doi: 10.3390/ijms20246331
90. Picó C, Palou M, Pomar CA, Rodríguez AM, Palou A. Leptin as a key regulator of the adipose organ. Rev Endocr Metab Disord (2022) 23(1):13–30. doi: 10.1007/s11154-021-09687-5
91. Templeman I, Smith HA, Walhin JP, Middleton B, Gonzalez JT, Karagounis LG, et al. Unacylated ghrelin, leptin, and appetite display diurnal rhythmicity in lean adults. J Appl Physiol (2021) 130(5):1534–43. doi: 10.1152/japplphysiol.00920.2020
92. Kalsbeek A, Fliers E, Romijn JA, La Fleur SE, Wortel J, Bakker O, et al. The suprachiasmatic nucleus generates the diurnal changes in plasma leptin levels. Endocrinology. (2001) 142(6):2677–85. doi: 10.1210/endo.142.6.8197
93. Luo S, Ezrokhi M, Cominos N, Tsai TH, Stoelzel CR, Trubitsyna Y, et al. Experimental dopaminergic neuron lesion at the area of the biological clock pacemaker, suprachiasmatic nuclei (SCN) induces metabolic syndrome in rats. Diabetol Metab Syndr (2021) 13(1):11. doi: 10.1186/s13098-021-00630-x
94. Montserrat-de la Paz S, Pérez-Pérez A, Vilariño-García T, Jiménez-Cortegana C, Muriana FJG, Millán-Linares MC, et al. Nutritional modulation of leptin expression and leptin action in obesity and obesity-associated complications. J Nutr Biochem (2021) 89:108561. doi: 10.1016/j.jnutbio.2020.108561
95. Picó C, Palou M. Leptin and metabolic programming. Nutrients. (2021) 14(1):114. doi: 10.3390/nu14010114
96. Vohra MS, Benchoula K, Serpell CJ, Hwa WE. AgRP/NPY and POMC neurons in the arcuate nucleus and their potential role in treatment of obesity. Eur J Pharmacol (2022) 915:174611. doi: 10.1016/j.ejphar.2021.174611
97. Singh U, Jiang J, Saito K, Toth BA, Dickey JE, Rodeghiero SR, et al. Neuroanatomical organization and functional roles of PVN MC4R pathways in physiological and behavioral regulations. Mol Metab (2022) 55:101401. doi: 10.1016/j.molmet.2021.101401
98. Domingos AI, Vaynshteyn J, Voss HU, Ren X, Gradinaru V, Zang F, et al. Leptin regulates the reward value of nutrient. Nat Neurosci (2011) 14(12):1562–8. doi: 10.1038/nn.2977
99. Omrani A, de Vrind VAJ, Lodder B, Stoltenborg I, Kooij K, Wolterink-Donselaar IG, et al. Identification of novel neurocircuitry through which leptin targets multiple inputs to the dopamine system to reduce food reward seeking. Biol Psychiatry (2021) 90(12):843–52. doi: 10.1016/j.biopsych.2021.02.017
100. Ollmann MM, Wilson BD, Yang YK, Kerns JA, Chen Y, Gantz I, et al. Antagonism of central melanocortin receptors in vitro and in vivo by agouti-related protein. Science. (1997) 278(5335):135–8. doi: 10.1126/science.278.5335.135
101. Simonds SE, Pryor JT, Ravussin E, Greenway FL, Dileone R, Allen AM, et al. Leptin mediates the increase in blood pressure associated with obesity. Cell. (2014) 159(6):1404–16. doi: 10.1016/j.cell.2014.10.058
102. Fujita Y, Kouda K, Ohara K, Nakamura H, Iki M. Leptin mediates the relationship between fat mass and blood pressure: The hamamatsu school-based health study. Med (Baltimore). (2019) 98(12):e14934. doi: 10.1097/MD.0000000000014934
103. Han C, Wu W, Ale A, Kim MS, Cai D. Central leptin and tumor necrosis factor-α (TNFα) in diurnal control of blood pressure and hypertension. J Biol Chem (2016) 291(29):15131–42. doi: 10.1074/jbc.M116.730408
104. Deschaine SL, Leggio L. From "Hunger hormone" to "It's complicated": Ghrelin beyond feeding control. Physiol (Bethesda). (2022) 37(1):5–15. doi: 10.1152/physiol.00024.2021
105. Wang Y, Wu Q, Zhou Q, Chen Y, Lei X, Chen Y, et al. Circulating acyl and des-acyl ghrelin levels in obese adults: a systematic review and meta-analysis. Sci Rep (2022) 12(1):2679. doi: 10.1038/s41598-022-06636-3
106. Ringuet MT, Furness JB. Furness SGB. G protein-coupled receptor interactions and modification of signalling involving the ghrelin receptor, GHSR1a. J Neuroendocrinol. (2021) 9:e13077. doi: 10.1111/jne.13077
107. Willesen MG, Kristensen P, Rømer J. Co-Localization of growth hormone secretagogue receptor and NPY mRNA in the arcuate nucleus of the rat. Neuroendocrinology. (1999) 70(5):306–16. doi: 10.1159/000054491
108. Airapetov MI, Eresko SO, Lebedev AA, Bychkov ER, Shabanov PD. Expression of the growth hormone secretagogue receptor 1a (GHS-R1a) in the brain. Physiol Rep (2021) 9(21):e15113. doi: 10.14814/phy2.15113
109. Su M, Yan M, Gong Y. Ghrelin fiber projections from the hypothalamic arcuate nucleus into the dorsal vagal complex and the regulation of glycolipid metabolism. Neuropeptides. (2019) 78:101972. doi: 10.1016/j.npep.2019.101972
110. Qian J, Morris CJ, Caputo R, Garaulet M, Scheer FAJL. Ghrelin is impacted by the endogenous circadian system and by circadian misalignment in humans. Int J Obes (Lond). (2019) 43(8):1644–9. doi: 10.1038/s41366-018-0208-9
111. Nunez-Salces M, Li H, Feinle-Bisset C, Young RL, Page AJ. The regulation of gastric ghrelin secretion. Acta Physiol (Oxf). (2021) 231(3):e13588. doi: 10.1111/apha.13588
112. Lamont EW, Bruton J, Blum ID, Abizaid A. Ghrelin receptor-knockout mice display alterations in circadian rhythms of activity and feeding under constant lighting conditions. Eur J Neurosci (2014) 39(2):207–17. doi: 10.1111/ejn.12390
113. Sinha R, Gu P, Hart R, Guarnaccia JB. Food craving, cortisol and ghrelin responses in modeling highly palatable snack intake in the laboratory. Physiol Behav (2019) 208:112563. doi: 10.1016/j.physbeh.2019.112563
114. Nauck MA, Meier JJ. Incretin hormones: Their role in health and disease. Diabetes Obes Metab (2018) 20 Suppl 1:5–21. doi: 10.1111/dom.13129
115. Rehfeld JF. The origin and understanding of the incretin concept. Front Endocrinol (Lausanne). (2018) 9:387. doi: 10.3389/fendo.2018.00387
116. Dupre J, Ross SA, Watson D, Brown JC. Stimulation of insulin secretion by gastric inhibitory polypeptide in man. J Clin Endocrinol Metab (1973) 37(5):826–8. doi: 10.1210/jcem-37-5-826
117. Holst JJ, Orskov C, Nielsen OV, Schwartz TW. Truncated glucagon-like peptide I, an insulin-releasing hormone from the distal gut. FEBS Lett (1987) 211(2):169–74. doi: 10.1016/0014-5793(87)81430-8
118. Holst JJ.The incretin system in healthy humans: The role of GIP and GLP-1. Metabolism. (2019) 96:46–55. doi: 10.1016/j.metabol.2019.04.014
119. Orskov C, Wettergren A, Holst JJ. Secretion of the incretin hormones glucagon-like peptide-1 and gastric inhibitory polypeptide correlates with insulin secretion in normal man throughout the day. Scand J Gastroenterol (1996) 31(7):665–70. doi: 10.3109/00365529609009147
120. Salera M, Giacomoni P, Pironi L, Ustra C, Capelli M, Giorgi A, et al. Circadian rhythm of gastric inhibitory polypeptide (GIP) in man. Metabolism. (1983) 32(1):21–4. doi: 10.1016/0026-0495(83)90150-6
121. Elliott RM, Morgan LM, Tredger JA, Deacon S, Wright J, Marks V. Glucagon-like peptide-1 (7-36)amide and glucose-dependent insulinotropic polypeptide secretion in response to nutrient ingestion in man: acute post-prandial and 24-h secretion patterns. J Endocrinol (1993) 138(1):159–66. doi: 10.1677/joe.0.1380159
122. Martchenko SE, Prescott D, Martchenko A, Sweeney ME, Philpott DJ, Brubaker PL. Diurnal changes in the murine small intestine are disrupted by obesogenic Western diet feeding and microbial dysbiosis. Sci Rep (2021) 11(1):20571. doi: 10.1038/s41598-021-98986-7
123. Martchenko SE, Martchenko A, Cox BJ, Naismith K, Waller A, Gurges P, et al. Circadian GLP-1 secretion in mice is dependent on the intestinal microbiome for maintenance of diurnal metabolic homeostasis. Diabetes. (2020) 69(12):2589–602. doi: 10.2337/db20-0262
124. Grasset E, Puel A, Charpentier J, Klopp P, Christensen JE, Lelouvier B, et al. Gut microbiota dysbiosis of type 2 diabetic mice impairs the intestinal daily rhythms of GLP-1 sensitivity. Acta Diabetol (2022) 59(2):243–58. doi: 10.1007/s00592-021-01790-y
125. Diz-Chaves Y, Herrera-Pérez S, González-Matías LC, Lamas JA, Mallo F. Glucagon-like peptide-1 (GLP-1) in the integration of neural and endocrine responses to stress. Nutrients (2020) 12(11):3304. doi: 10.3390/nu12113304
126. Holt MK, Richards JE, Cook DR, Brierley DI, Williams DL, Reimann F, et al. Preproglucagon neurons in the nucleus of the solitary tract are the main source of brain GLP-1, mediate stress-induced hypophagia, and limit unusually Large intakes of food. Diabetes. (2019) 68(1):21–33. doi: 10.2337/db18-0729
127. Richard JE, Anderberg RH, Göteson A, Gribble FM, Reimann F, Skibicka KP. Activation of the GLP-1 receptors in the nucleus of the solitary tract reduces food reward behavior and targets the mesolimbic system. PloS One (2015) 10(3):e0119034. doi: 10.1371/journal.pone.0119034
128. Brierley DI, Holt MK, Singh A, de Araujo A, McDougle M, Vergara M, et al. Central and peripheral GLP-1 systems independently suppress eating. Nat Metab (2021) 3(2):258–73. doi: 10.1038/s42255-021-00344-4
129. Eren-Yazicioglu CY, Yigit A, Dogruoz RE, Yapici-Eser H. Can GLP-1 be a target for reward system related disorders? a qualitative synthesis and systematic review analysis of studies on palatable food, drugs of abuse, and alcohol. Front Behav Neurosci (2021) 14:614884. doi: 10.3389/fnbeh.2020.614884
130. Hernandez NS, Schmidt HD. Central GLP-1 receptors: Novel molecular targets for cocaine use disorder. Physiol Behav (2019) 206:93–105. doi: 10.1016/j.physbeh.2019.03.026
131. You ZB, Wang B, Gardner EL, Wise RA. Cocaine and cocaine expectancy increase growth hormone, ghrelin, GLP-1, IGF-1, adiponectin, and corticosterone while decreasing leptin, insulin, GIP, and prolactin. Pharmacol Biochem Behav (2019) 176:53–6. doi: 10.1016/j.pbb.2018.11.001
132. Schmidt HD, Mietlicki-Baase EG, Ige KY, Maurer JJ, Reiner DJ, Zimmer DJ, et al. Glucagon-like peptide-1 receptor activation in the ventral tegmental area decreases the reinforcing efficacy of cocaine. Neuropsychopharmacology. (2016) 41(7):1917–28. doi: 10.1038/npp.2015.362
133. Geng L, Lam KSL, Xu A. The therapeutic potential of FGF21 in metabolic diseases: from bench to clinic. Nat Rev Endocrinol (2020) 16(11):654–67. doi: 10.1038/s41574-020-0386-0
134. Flippo KH, Potthoff MJ. Metabolic messengers: FGF21. Nat Metab (2021) 3(3):309–17. doi: 10.1038/s42255-021-00354-2
135. Xie T, Leung PS. Fibroblast growth factor 21: a regulator of metabolic disease and health span. Am J Physiol Endocrinol Metab (2017) 313(3):E292–302. doi: 10.1152/ajpendo.00101.2017
136. Yu H, Xia F, Lam KS, Wang Y, Bao Y, Zhang J, et al. Circadian rhythm of circulating fibroblast growth factor 21 is related to diurnal changes in fatty acids in humans. Clin Chem (2011) 57(5):691–700. doi: 10.1373/clinchem.2010.155184
137. Wu CT, Chaffin AT, Ryan KK. Fibroblast growth factor 21 facilitates the homeostatic control of feeding behavior. J Clin Med (2022) 11(3):580. doi: 10.3390/jcm11030580
138. Liu X, Zhang Y, Ma C, Lin J, Du J. Alternate-day fasting alleviates high fat diet induced non-alcoholic fatty liver disease through controlling PPARα/Fgf21 signaling. Mol Biol Rep (2022) 49(4):3113–22. doi: 10.1007/s11033-022-07142-5
139. Inagaki T, Dutchak P, Zhao G, Ding X, Gautron L, Parameswara V, et al. Endocrine regulation of the fasting response by PPARalpha-mediated induction of fibroblast growth factor 21. Cell Metab (2007) 5(6):415–25. doi: 10.1016/j.cmet.2007.05.003
140. Montagner A, Polizzi A, Fouché E, Ducheix S, Lippi Y, Lasserre F, et al. Liver PPARα is crucial for whole-body fatty acid homeostasis and is protective against NAFLD. Gut. (2016) 65(7):1202–14. doi: 10.1136/gutjnl-2015-310798
141. Liu M, Cao H, Hou Y, Sun G, Li D, Wang W. Liver plays a major role in FGF-21 mediated glucose homeostasis. Cell Physiol Biochem (2018) 45(4):1423–33. doi: 10.1159/000487568
142. BonDurant LD, Ameka M, Naber MC, Markan KR, Idiga SO, Acevedo MR, et al. FGF21 regulates metabolism through adipose-dependent and -independent mechanisms. Cell Metab (2017) 25(4):935–944.e4. doi: 10.1016/j.cmet.2017.03.005
143. Yano K, Yamaguchi K, Seko Y, Okishio S, Ishiba H, Tochiki N, et al. Hepatocyte-specific fibroblast growth factor 21 overexpression ameliorates high-fat diet-induced obesity and liver steatosis in mice. Lab Invest. (2022) 102(3):281–9. doi: 10.1038/s41374-021-00680-9
144. Matsui S, Sasaki T, Kohno D, Yaku K, Inutsuka A, Yokota-Hashimoto H, et al. Neuronal SIRT1 regulates macronutrient-based diet selection through FGF21 and oxytocin signalling in mice. Nat Commun (2018) 9(1):4604. doi: 10.1038/s41467-018-07033-z
145. Jensen-Cody SO, Flippo KH, Claflin KE, Yavuz Y, Sapouckey SA, Walters GC, et al. FGF21 signals to glutamatergic neurons in the ventromedial hypothalamus to suppress carbohydrate intake. Cell Metab (2020) 32(2):273–286.e6. doi: 10.1016/j.cmet.2020.06.008
146. Bookout AL, de Groot MH, Owen BM, Lee S, Gautron L, Lawrence HL, et al. FGF21 regulates metabolism and circadian behavior by acting on the nervous system. Nat Med (2013) 19(9):1147–52. doi: 10.1038/nm.3249
147. Glavaš M, Gitlin-Domagalska A, Dębowski D, Ptaszyńska N, Łęgowska A, Rolka K. Vasopressin and its analogues: From natural hormones to multitasking peptides. Int J Mol Sci (2022) 23(6):3068. doi: 10.3390/ijms23063068
148. Maejima T, Tsuno Y, Miyazaki S, Tsuneoka Y, Hasegawa E, Islam MT, et al. GABA from vasopressin neurons regulates the time at which suprachiasmatic nucleus molecular clocks enable circadian behavior. Proc Natl Acad Sci U S A. (2021) 118(6):e2010168118. doi: 10.1073/pnas.2010168118
149. Mieda M. The network mechanism of the central circadian pacemaker of the SCN: Do AVP neurons play a more critical role than expected? Front Neurosci (2019) 13:139. doi: 10.3389/fnins.2019.00139
150. Shirai H, Oishi K, Ishida N. Bidirectional CLOCK/BMAL1-dependent circadian gene regulation by retinoic acid. vitro. Biochem Biophys Res Commun (2006) 351(2):387–91. doi: 10.1016/j.bbrc.2006.10.031
151. Jin X, Shearman LP, Weaver DR, Zylka MJ, de Vries GJ, Reppert SM. A molecular mechanism regulating rhythmic output from the suprachiasmatic circadian clock. Cell. (1999) 96(1):57–68. doi: 10.1016/s0092-8674(00)80959-9
152. Edwards MD, Brancaccio M, Chesham JE, Maywood ES, Hastings MH. Rhythmic expression of cryptochrome induces the circadian clock of arrhythmic suprachiasmatic nuclei through arginine vasopressin signaling. Proc Natl Acad Sci U S A. (2016) 113(10):2732–7. doi: 10.1073/pnas.1519044113
153. Gizowski C, Zaelzer C, Bourque CW. Clock-driven vasopressin neurotransmission mediates anticipatory thirst prior to sleep. Nature. (2016) 537(7622):685–8. doi: 10.1038/nature19756
154. Lightman SL, Birnie MT, Conway-Campbell BL. Dynamics of ACTH and cortisol secretion and implications for disease. Endocr Rev (2020) 41(3):bnaa002. doi: 10.1210/endrev/bnaa002
155. Law R, Clow A. Stress, the cortisol awakening response and cognitive function. Int Rev Neurobiol (2020) 150:187–217. doi: 10.1016/bs.irn.2020.01.001
156. Keller-Wood M. Hypothalamic-Pituitary–adrenal axis-feedback control. Compr Physiol (2015) 5(3):1161–82. doi: 10.1002/cphy.c140065
157. Gomez F, Chapleur M, Fernette B, Burlet C, Nicolas JP, Burlet A. Arginine vasopressin (AVP) depletion in neurons of the suprachiasmatic nuclei affects the AVP content of the paraventricular neurons and stimulates adrenocorticotrophic hormone release. J Neurosci Res (1997) 50(4):565–74. doi: 10.1002/(SICI)1097-4547(19971115)50:4<565::AID-JNR7>3.0.CO;2-C
158. Moreira AC, Antonini SR, de Castro M. Mechanisms in endocrinology: A sense of time of the glucocorticoid circadian clock: from the ontogeny to the diagnosis of cushing's syndrome. Euro J Endocrinol (2018) 179(1):R1–R18. doi: 10.1530/EJE-18-0102
159. Minnetti M, Hasenmajer V, Pofi R, Venneri MA, Alexandraki KI, Isidori AM. Fixing the broken clock in adrenal disorders: focus on glucocorticoids and chronotherapy. J Endocrinol (2020) 246(2):R13–31. doi: 10.1530/JOE-20-0066
160. Vgontzas AN, Fernandez-Mendoza J, Lenker KP, Basta M, Bixler EO, Chrousos GP. Hypothalamic-pituitary-adrenal (HPA) axis response to exogenous corticotropin-releasing hormone (CRH) is attenuated in men with chronic insomnia. J Sleep Res (2021) 25:e13526. doi: 10.1111/jsr.13526
161. Mohd Azmi NAS, Juliana N, Azmani S, Mohd Effendy N, Abu IF, Mohd Fahmi Teng NI, et al. Cortisol on circadian rhythm and its effect on cardiovascular system. Int J Environ Res Public Health (2021) 18(2):676. doi: 10.3390/ijerph18020676
162. Wang D, Day EA, Townsend LK, Djordjevic D, Jørgensen SB, Steinberg GR. GDF15: emerging biology and therapeutic applications for obesity and cardiometabolic disease. Nat Rev Endocrinol (2021) 17(10):592–607. doi: 10.1038/s41574-021-00529-7
163. Tsai VW, Macia L, Feinle-Bisset C, Manandhar R, Astrup A, Raben A, et al. Serum levels of human MIC-1/GDF15 vary in a diurnal pattern, do not display a profile suggestive of a satiety factor and are related to BMI. PloS One (2015) 10(7):e0133362. doi: 10.1371/journal.pone.0133362
164. Zhao L, Isayama K, Chen H, Yamauchi N, Shigeyoshi Y, Hashimoto S, et al. The nuclear receptor REV-ERBα represses the transcription of growth/differentiation factor 10 and 15 genes in rat endometrium stromal cells. Physiol Rep (2016) 4(2):e12663. doi: 10.14814/phy2.12663
165. Wollert KC, Kempf T, Wallentin L. Growth differentiation factor 15 as a biomarker in cardiovascular disease. Clin Chem (2017) 63(1):140–51. doi: 10.1373/clinchem.2016.255174
166. Wallentin L, Hijazi Z, Andersson U, Alexander JH, De Caterina R, Hanna M, et al. ARISTOTLE investigators. growth differentiation factor 15, a marker of oxidative stress and inflammation, for risk assessment in patients with atrial fibrillation: insights from the apixaban for reduction in stroke and other thromboembolic events in atrial fibrillation (ARISTOTLE) trial. Circulation. (2014) 130(21):1847–58. doi: 10.1161/CIRCULATIONAHA.114.011204
167. Brown DA, Moore J, Johnen H, Smeets TJ, Bauskin AR, Kuffner T, et al. Serum macrophage inhibitory cytokine 1 in rheumatoid arthritis: a potential marker of erosive joint destruction. Arthritis Rheumatol (2007) 56(3):753–64. doi: 10.1002/art.22410
168. Emmerson PJ, Wang F, Du Y, Liu Q, Pickard RT, Gonciarz MD, et al. The metabolic effects of GDF15 are mediated by the orphan receptor GFRAL. Nat Med (2017) 23(10):1215–9. doi: 10.1038/nm.4393(KO
169. Mullican SE, Lin-Schmidt X, Chin CN, Chavez JA, Furman JL, Armstrong AA, et al. GFRAL is the receptor for GDF15 and the ligand promotes weight loss in mice and nonhuman primates. Nat Med (2017) 23(10):1150–7. doi: 10.1038/nm.4392
170. Xiong Y, Walker K, Min X, Hale C, Tran T, Komorowski R, et al. Long-acting MIC-1/GDF15 molecules to treat obesity: Evidence from mice to monkeys. Sci Transl Med (2017) 9(412):eaan8732. doi: 10.1126/scitranslmed.aan8732
171. Johnen H, Lin S, Kuffner T, Brown DA, Tsai VW, Bauskin AR, et al. Tumor-induced anorexia and weight loss are mediated by the TGF-beta superfamily cytokine MIC-1. Nat Med (2007) 13(11):1333–40. doi: 10.1038/nm1677
172. Yang L, Chang CC, Sun Z, Madsen D, Zhu H, Padkjær SB, et al. GFRAL is the receptor for GDF15 and is required for the anti-obesity effects of the ligand. Nat Med (2017) 23(10):1158–66. doi: 10.1038/nm.4394
173. Sabatini PV, Frikke-Schmidt H, Arthurs J, Gordian D, Patel A, Rupp AC, et al. GFRAL-expressing neurons suppress food intake via aversive pathways. Proc Natl Acad Sci U.S.A. (2021) 118(8):e2021357118. doi: 10.1073/pnas.2021357118
174. Patel S, Alvarez-Guaita A, Melvin A, Rimmington D, Dattilo A, Miedzybrodzka EL, et al. GDF15 provides an endocrine signal of nutritional stress in mice and humans. Cell Metab (2019) 29(3):707–718.e8. doi: 10.1016/j.cmet.2018.12.016
175. Worth AA, Shoop R, Tye K, Feetham CH, D'Agostino G, Dodd GT, et al. The cytokine GDF15 signals through a population of brainstem cholecystokinin neurons to mediate anorectic signalling. Elife. (2020) 29:9:e55164. doi: 10.7554/eLife.55164
176. Hsu JY, Crawley S, Chen M, Ayupova DA, Lindhout DA, Higbee J, et al. Non-homeostatic body weight regulation through a brainstem-restricted receptor for GDF15. Nature. (2017) 550(7675):255–9. doi: 10.1038/nature24042
177. Heger J, Schiegnitz E, von Waldthausen D, Anwar MM, Piper HM, Euler G. Growth differentiation factor 15 acts anti-apoptotic and pro-hypertrophic in adult cardiomyocytes. J Cell Physiol (2010) 224(1):120–6. doi: 10.1002/jcp.22102
178. Kempf T, Eden M, Strelau J, Naguib M, Willenbockel C, Tongers J, et al. The transforming growth factor-beta superfamily member growth-differentiation factor-15 protects the heart from ischemia/reperfusion injury. Circ Res (2006) 98(3):351–60. doi: 10.1161/01.RES.0000202805.73038.48
179. Zhang Y, Moszczynski LA, Liu Q, Jiang J, Zhao D, Quan D, et al. Over-expression of growth differentiation factor 15 (GDF15) preventing cold ischemia reperfusion (I/R) injury in heart transplantation through Foxo3a signaling. Oncotarget. (2017) 8(22):36531–44. doi: 10.18632/oncotarget.16607
180. Townsend LK, Weber AJ, Day EA, Shamshoum H, Shaw SJ, Perry CGR, et al. AMPK mediates energetic stress-induced liver GDF15. FASEB J (2021) 35(1):e21218. doi: 10.1096/fj.202000954R
181. Miyake M, Zhang J, Yasue A, Hisanaga S, Tsugawa K, Sakaue H, et al. Integrated stress response regulates GDF15 secretion from adipocytes, preferentially suppresses appetite for a high-fat diet and improves obesity. iScience. (2021) 24(12):103448. doi: 10.1016/j.isci.2021.103448
182. Kim KH, Lee MS. GDF15 as a central mediator for integrated stress response and a promising therapeutic molecule for metabolic disorders and NASH. Biochim Biophys Acta Gen Subj. (2021) 1865(3):129834. doi: 10.1016/j.bbagen.2020.129834
183. Yasumoto Y, Hashimoto C, Nakao R, Yamazaki H, Hiroyama H, Nemoto T, et al. Short-term feeding at the wrong time is sufficient to desynchronize peripheral clocks and induce obesity with hyperphagia, physical inactivity and metabolic disorders in mice. Metabolism. (2016) 65(5):714–27. doi: 10.1016/j.metabol.2016.02.003
184. Oishi K, Hashimoto C. Short-term time-restricted feeding during the resting phase is sufficient to induce leptin resistance that contributes to development of obesity and metabolic disorders in mice. Chronobiol Int (2018) 35(11):1576–94. doi: 10.1080/07420528.2018.1496927
185. Abe T, Kazama R, Okauchi H, Oishi K. Food deprivation during active phase induces skeletal muscle atrophy via IGF-1 reduction in mice. Arch Biochem Biophys (2019) 677:108160. doi: 10.1016/j.abb.2019.108160
186. Boivin DB, Boudreau P, Kosmadopoulos A. Disturbance of the circadian system in shift work and its health impact. J Biol Rhythms. (2022) 37(1):3–28. doi: 10.1177/07487304211064218
187. Chellappa SL, Qian J, Vujovic N, Morris CJ, Nedeltcheva A, Nguyen H, et al. Daytime eating prevents internal circadian misalignment and glucose intolerance in night work. Sci Adv (2021) 7(49):eabg9910. doi: 10.1126/sciadv.abg9910
188. Flanagan A, Bechtold DA, Pot GK, Johnston JD. Chrono-nutrition: From molecular and neuronal mechanisms to human epidemiology and timed feeding patterns. J Neurochem (2021) 157(1):53–72. doi: 10.1111/jnc.15246
189. Hermenegildo-López Y, Donat-Vargas C, Sandoval-Insausti H, Moreno-Franco B, Rodríguez-Ayala M, Rey-García J, et al. A higher intake of energy at dinner is associated with incident metabolic syndrome: A prospective cohort study in older adults. Nutrients (2021) 13(9):3035. doi: 10.3390/nu13093035
190. Hermenegildo Y, López-García E, García-Esquinas E, Pérez-Tasigchana RF, Rodríguez-Artalejo F, Guallar-Castillón P. Distribution of energy intake throughout the day and weight gain: a population-based cohort study in Spain. Br J Nutr (2016) 115(11):2003–10. doi: 10.1017/S0007114516000891
191. Pot GK, Hardy R, Stephen AM. Irregular consumption of energy intake in meals is associated with a higher cardiometabolic risk in adults of a British birth cohort. Int J Obes (Lond). (2014) 38(12):1518–24. doi: 10.1038/ijo.2014.51
192. Saals B, Boss HM, Pot GK. Young people and adolescents have more irregular meals during the COVID-19 pandemic: A nested case-control study on chrono-nutrition before and during the COVID-19 pandemic. Chronobiol Int (2022) 30:1–10. doi: 10.1080/07420528.2022.2054347
193. Teixeira MT, Vitorino RS, da Silva JH, Raposo LM, Aquino LA, Ribas SA. Eating habits of children and adolescents during the COVID-19 pandemic: The impact of social isolation. J Hum Nutr Diet. (2021) 34(4):670–8. doi: 10.1111/jhn.12901
194. Woo S, Yang H, Kim Y, Lim H, Song HJ, Park KH. Sedentary time and fast-food consumption associated with weight gain during COVID-19 lockdown in children and adolescents with overweight or obesity. J Korean Med Sci (2022) 37(12):e103. doi: 10.3346/jkms.2022.37.e103
195. Goel N, Stunkard AJ, Rogers NL, Van Dongen HP, Allison KC, O'Reardon JP, et al. Circadian rhythm profiles in women with night eating syndrome. J Biol Rhythms. (2009) 4(1):85–94. doi: 10.1177/0748730408328914
196. Lent MR, Atwood M, Bennett WL, Woolf TB, Martin L, Zhao D, et al. Night eating, weight, and health behaviors in adults participating in the Daily24 study. Eat Behav (2022) 45:101605. doi: 10.1016/j.eatbeh.2022.101605
197. Barrington WE, Beresford SAA. Eating occasions, obesity and related behaviors in working adults: Does it matter when you snack? Nutrients. (2019) 11(10):2320. doi: 10.3390/nu11102320
198. Wehrens SMT, Christou S, Isherwood C, Middleton B, Gibbs MA, Archer SN, et al. Meal timing regulates the human circadian system. Curr Biol (2017) 27(12):1768–1775.e3. doi: 10.1016/j.cub.2017.04.059
199. Basdeki ED, Koumi K, Tsirimiagkou C, Argyris A, Chrysostomou S, Sfikakis PP, et al. Late-night overeating or low-quality food choices late at night are associated with subclinical vascular damage in patients at increased cardiovascular risk. Nutrients. (2022) 14(3):470. doi: 10.3390/nu14030470
200. Kohsaka A, Laposky AD, Ramsey KM, Estrada C, Joshu C, Kobayashi Y, et al. High-fat diet disrupts behavioral and molecular circadian rhythms in mice. Cell Metab (2007) 6(5):414–21. doi: 10.1016/j.cmet.2007.09.006
201. Li D, Ikaga R, Ogawa H, Yamazaki T. Different expressions of clock genes in fatty liver induced by high-sucrose and high-fat diets. Chronobiol Int (2021) 38(5):762–78. doi: 10.1080/07420528.2021.1889579
202. Nayak N, Mishra M. High fat diet induced abnormalities in metabolism, growth, behavior, and circadian clock in drosophila melanogaster. Life Sci (2021) 281:119758. doi: 10.1016/j.lfs.2021.119758
203. Hou W, Gao J, Jiang W, Wei W, Wu H, Zhang Y, et al. Meal timing of subtypes of macronutrients consumption with cardiovascular diseases: NHANES, 2003 to 2016. J Clin Endocrinol Metab (2021) 106(7):e2480–90. doi: 10.1210/clinem/dgab288
204. Berryman CE, Lieberman HR, Fulgoni VL 3rd, Pasiakos SM. Greater protein intake at breakfast or as snacks and less at dinner is associated with cardiometabolic health in adults. Clin Nutr (2021) 40(6):4301–8. doi: 10.1016/j.clnu.2021.01.018
205. Zitting KM, Vetrivelan R, Yuan RK, Vujovic N, Wang W, Bandaru SS, et al. Chronic circadian disruption on a high-fat diet impairs glucose tolerance. Metabolism. (2022) 130:155158. doi: 10.1016/j.metabol.2022.155158
206. Kessler K, Hornemann S, Petzke KJ, Kemper M, Kramer A, Pfeiffer AF, et al. The effect of diurnal distribution of carbohydrates and fat on glycaemic control in humans: a randomized controlled trial. Sci Rep (2017) 7:44170. doi: 10.1038/srep44170
207. Stojanov S, Berlec A, Štrukelj B. The influence of probiotics on the Firmicutes/Bacteroidetes ratio in the treatment of obesity and inflammatory bowel disease. Microorganisms. (2020) 8(11):1715. doi: 10.3390/microorganisms8111715
208. Choi H, Rao MC, Chang EB. Gut microbiota as a transducer of dietary cues to regulate host circadian rhythms and metabolism. Nat Rev Gastroenterol Hepatol (2021) 18(10):679–89. doi: 10.1038/s41575-021-00452-2
209. Parkar SG, Kalsbeek A, Cheeseman JF. Potential role for the gut microbiota in modulating host circadian rhythms and metabolic health. Microorganisms. (2019) 7(2):41. doi: 10.3390/microorganisms7020041
210. Tahara Y, Yamazaki M, Sukigara H, Motohashi H, Sasaki H, Miyakawa H, et al. Gut microbiota-derived short chain fatty acids induce circadian clock entrainment in mouse peripheral tissue. Sci Rep (2018) 8(1):1395. doi: 10.1038/s41598-018-19836-7
211. Mukherji A, Kobiita A, Ye T, Chambon P. Homeostasis in intestinal epithelium is orchestrated by the circadian clock and microbiota cues transduced by TLRs. Cell. (2013) 153(4):812–27. doi: 10.1016/j.cell.2013.04.020
212. Teichman EM, O'Riordan KJ, Gahan CGM, Dinan TG, Cryan JF. When rhythms meet the blues: Circadian interactions with the microbiota-Gut-Brain axis. Cell Metab (2020) 31(3):448–71. doi: 10.1016/j.cmet.2020.02.008
213. Zarrinpar A, Chaix A, Yooseph S, Panda S. Diet and feeding pattern affect the diurnal dynamics of the gut microbiome. Cell Metab (2014) 20(6):1006–17. doi: 10.1016/j.cmet.2014.11.008
214. Thaiss CA, Zeevi D, Levy M, Zilberman-Schapira G, Suez J, Tengeler AC, et al. Transkingdom control of microbiota diurnal oscillations promotes metabolic homeostasis. Cell. (2014) 159(3):514–29. doi: 10.1016/j.cell.2014.09.048
215. Leone V, Gibbons SM, Martinez K, Hutchison AL, Huang EY, Cham CM, et al. Effects of diurnal variation of gut microbes and high-fat feeding on host circadian clock function and metabolism. Cell Host Microbe (2015) 17(5):681–9. doi: 10.1016/j.chom.2015.03.006
216. Smith HA, Betts JA. Nutrient timing and metabolic regulation. J Physiol (2022) 600(6):1299–312. doi: 10.1113/JP280756
217. Mazri FH, Manaf ZA, Shahar S, Mat Ludin AF, Karim NA, Hazwari NDD, et al. Do temporal eating patterns differ in healthy versus unhealthy Overweight/Obese individuals? Nutrients. (2021) 13(11):4121. doi: 10.3390/nu13114121
218. Jakubowicz D, Landau Z, Tsameret S, Wainstein J, Raz I, Ahren B, et al. Reduction in glycated hemoglobin and daily insulin dose alongside circadian clock upregulation in patients with type 2 diabetes consuming a three-meal diet: A randomized clinical trial. Diabetes Care (2019) 42(12):2171–80. doi: 10.2337/dc19-1142
219. Jamshed H, Beyl RA, Della Manna DL, Yang ES, Ravussin E, Peterson CM. Early time-restricted feeding improves 24-hour glucose levels and affects markers of the circadian clock, aging, and autophagy in humans. Nutrients. (2019) 11(6):1234. doi: 10.3390/nu11061234
220. Li QM, Wu CK, Ma PC, Cui H, Li RN, Hong C, et al. Breakfast consumption frequency is associated with dyslipidemia: a retrospective cohort study of a working population. Lipids Health Dis (2022) 21(1):33. doi: 10.1186/s12944-022-01641-x
221. Jakubowicz D, Froy O, Wainstein J, Boaz M. Meal timing and composition influence ghrelin levels, appetite scores and weight loss maintenance in overweight and obese adults. Steroids. (2012) 77(4):323–31. doi: 10.1016/j.steroids.2011.12.006
222. Jakubowicz D, Wainstein J, Landau Z, Raz I, Ahren B, Chapnik N, et al. Influences of breakfast on clock gene expression and postprandial glycemia in healthy individuals and individuals with diabetes: A randomized clinical trial. Diabetes Care (2017) 40(11):1573–9. doi: 10.2337/dc16-2753
223. Longo VD, Panda S. Fasting, circadian rhythms, and time-restricted feeding in healthy lifespan. Cell Metab (2016) 23(6):1048–59. doi: 10.1016/j.cmet.2016.06.001
224. Malinowski B, Zalewska K, Węsierska A, Sokołowska MM, Socha M, Liczner G, et al. Intermittent fasting in cardiovascular disorders-an overview. Nutrients. (2019) 11(3):673. doi: 10.3390/nu11030673
225. Chaix A, Lin T, Le HD, Chang MW, Panda S. Time-restricted feeding prevents obesity and metabolic syndrome in mice lacking a circadian clock. Cell Metab (2019) 29(2):303–319.e4. doi: 10.1016/j.cmet.2018.08.004
226. Hatori M, Vollmers C, Zarrinpar A, DiTacchio L, Bushong EA, Gill S, et al. Time-restricted feeding without reducing caloric intake prevents metabolic diseases in mice fed a high-fat diet. Cell Metab (2012) 15(6):848–60. doi: 10.1016/j.cmet.2012.04.019
227. Sherman H, Genzer Y, Cohen R, Chapnik N, Madar Z, Froy O. Timed high-fat diet resets circadian metabolism and prevents obesity. FASEB J (2012) 26(8):3493–502. doi: 10.1096/fj.12-208868
228. Zarrinpar A, Chaix A, Yooseph S, Panda S. Diet and feeding pattern affect the diurnal dynamics of the gut microbiome. Cell Metab (2014) 20(6):1006–17. doi: 10.1016/j.cmet.2014.11.008
229. Gill S, Le HD, Melkani GC, Panda S. Time-restricted feeding attenuates age-related cardiac decline in drosophila. Science. (2015) 347(6227):1265–9. doi: 10.1126/science.1256682
230. Villanueva JE, Livelo C, Trujillo AS, Chandran S, Woodworth B, Andrade L, et al. Time-restricted feeding restores muscle function in drosophila models of obesity and circadian-rhythm disruption. Nat Commun (2019) 10(1):2700. doi: 10.1038/s41467-019-10563-9
231. Melkani GC, Panda S. Time-restricted feeding for prevention and treatment of cardiometabolic disorders. J Physiol (2017) 595(12):3691–700. doi: 10.1113/JP273094
232. Moon S, Kang J, Kim SH, Chung HS, Kim YJ, Yu JM, et al. Beneficial effects of time-restricted eating on metabolic diseases: A systemic review and meta-analysis. Nutrients. (2020) 12(5):1267. doi: 10.3390/nu12051267
233. Sutton EF, Beyl R, Early KS, Cefalu WT, Ravussin E, Peterson CM. Early time-restricted feeding improves insulin sensitivity, blood pressure, and oxidative stress even without weight loss in men with prediabetes. Cell Metab (2018) 27(6):1212–1221.e3. doi: 10.1016/j.cmet.2018.04.010
234. Jones R, Pabla P, Mallinson J, Nixon A, Taylor T, Bennett A, et al. Two weeks of early time-restricted feeding (eTRF) improves skeletal muscle insulin and anabolic sensitivity in healthy men. Am J Clin Nutr (2020) 112(4):1015–28. doi: 10.1093/ajcn/nqaa192
235. Adafer R, Messaadi W, Meddahi M, Patey A, Haderbache A, Bayen S, et al. Food timing, circadian rhythm and chrononutrition: A systematic review of time-restricted eating's effects on human health. Nutrients. (2020) 12(12):3770. doi: 10.3390/nu12123770
236. Senesi P, Ferrulli A, Luzi L, Terruzzi I. Diabetes mellitus and cardiovascular diseases: Nutraceutical interventions related to caloric restriction. Int J Mol Sci (2021) 22(15):7772. doi: 10.3390/ijms22157772
237. Santana TM, Ogawa LY, Rogero MM, Barroso LP, Alves de Castro I. Effect of resveratrol supplementation on biomarkers associated with atherosclerosis in humans. Complement Ther Clin Pract (2022) 46:101491. doi: 10.1016/j.ctcp.2021.101491
238. Shahwan M, Alhumaydhi F, Ashraf GM, Hasan PMZ, Shamsi A. Role of polyphenols in combating type 2 diabetes and insulin resistance. Int J Biol Macromol. (2022) 206:567–79. doi: 10.1016/j.ijbiomac.2022.03.004
239. Ding S, Jiang H, Fang J, Liu G. Regulatory effect of resveratrol on inflammation induced by lipopolysaccharides via reprograming intestinal microbes and ameliorating serum metabolism profiles. Front Immunol (2021) 12:777159. doi: 10.3389/fimmu.2021.777159
240. Du F, Huang R, Lin D, Wang Y, Yang X, Huang X, et al. Resveratrol improves liver steatosis and insulin resistance in non-alcoholic fatty liver disease in association with the gut microbiota. Front Microbiol (2021) 12:611323. doi: 10.3389/fmicb.2021.611323
241. Sarubbo F, Esteban S, Miralles A, Moranta D. Effects of resveratrol and other polyphenols on Sirt1: Relevance to brain function during aging. Curr Neuropharmacol. (2018) 16(2):126–36. doi: 10.2174/1570159X15666170703113212
242. Chen Y, Zhang H, Ji S, Jia P, Chen Y, Li Y, et al. Resveratrol and its derivative pterostilbene attenuate oxidative stress-induced intestinal injury by improving mitochondrial redox homeostasis and function via SIRT1 signaling. Free Radic Biol Med (2021) 177:1–14. doi: 10.1016/j.freeradbiomed.2021.10.011
243. Man AWC, Xia N, Daiber A, Li H. The roles of gut microbiota and circadian rhythm in the cardiovascular protective effects of polyphenols. Br J Pharmacol (2020) 177(6):1278–93. doi: 10.1111/bph.14850
244. Li J, Wei L, Zhao C, Li J, Liu Z, Zhang M, et al. Resveratrol maintains lipid metabolism homeostasis via one of the mechanisms associated with the key circadian regulator Bmal1. Molecules. (2019) 24(16):2916. doi: 10.3390/molecules24162916
245. Sun L, Wang Y, Song Y, Cheng XR, Xia S, Rahman MR, et al. Resveratrol restores the circadian rhythmic disorder of lipid metabolism induced by high-fat diet in mice. Biochem Biophys Res Commun (2015) 458(1):86–91. doi: 10.1016/j.bbrc.2015.01.072
246. Koh YC, Lee PS, Kuo YL, Nagabhushanam K, Ho CT, Pan MH. Dietary pterostilbene and resveratrol modulate the gut microbiota influenced by circadian rhythm dysregulation. Mol Nutr Food Res (2021) 65(21):e2100434. doi: 10.1002/mnfr.202100434
247. Cheng WY, Lam KL, Pik-Shan Kong A, Chi-Keung Cheung P. Prebiotic supplementation (beta-glucan and inulin) attenuates circadian misalignment induced by shifted light-dark cycle in mice by modulating circadian gene expression. Food Res Int (2020) 137:109437. doi: 10.1016/j.foodres.2020
248. Cheng WY, Lam KL, Li X, Kong AP, Cheung PC. Circadian disruption-induced metabolic syndrome in mice is ameliorated by oat β-glucan mediated by gut microbiota. Carbohydr Polym. (2021) 267:118216. doi: 10.1016/j.carbpol.2021.118216
249. Bladé C, Aragonès G, Arola-Arnal A, Muguerza B, Bravo FI, Salvadó MJ, et al. Proanthocyanidins in health and disease. Biofactors. (2016) 42(1):5–12. doi: 10.1002/biof.1249
Keywords: Circadian disruption, cardiac clock, microbiota, food intake, time restricted feeding
Citation: Senesi P, Ferrulli A, Luzi L and Terruzzi I (2022) Chrono-communication and cardiometabolic health: The intrinsic relationship and therapeutic nutritional promises. Front. Endocrinol. 13:975509. doi: 10.3389/fendo.2022.975509
Received: 22 June 2022; Accepted: 23 August 2022;
Published: 13 September 2022.
Edited by:
Esmail Lutfi, Norwegian Institute of Food, Fisheries and Aquaculture Research (Nofima), NorwayReviewed by:
María Jesús Delgado, Complutense University of Madrid, SpainCopyright © 2022 Senesi, Ferrulli, Luzi and Terruzzi. This is an open-access article distributed under the terms of the Creative Commons Attribution License (CC BY). The use, distribution or reproduction in other forums is permitted, provided the original author(s) and the copyright owner(s) are credited and that the original publication in this journal is cited, in accordance with accepted academic practice. No use, distribution or reproduction is permitted which does not comply with these terms.
*Correspondence: Ileana Terruzzi, aWxlYW5hLnRlcnJ1enppQHVuaW1pLml0
†ORCID: Pamela Senesi, orcid.org/0000-0003-0304-1564
Anna Ferrulli, orcid.org/0000-0002-8518-5547
Livio Luzi, orcid.org/0000-0003-3183-0552
Ileana Terruzzi, orcid.org/0000-0002-8663-4033
Disclaimer: All claims expressed in this article are solely those of the authors and do not necessarily represent those of their affiliated organizations, or those of the publisher, the editors and the reviewers. Any product that may be evaluated in this article or claim that may be made by its manufacturer is not guaranteed or endorsed by the publisher.
Research integrity at Frontiers
Learn more about the work of our research integrity team to safeguard the quality of each article we publish.