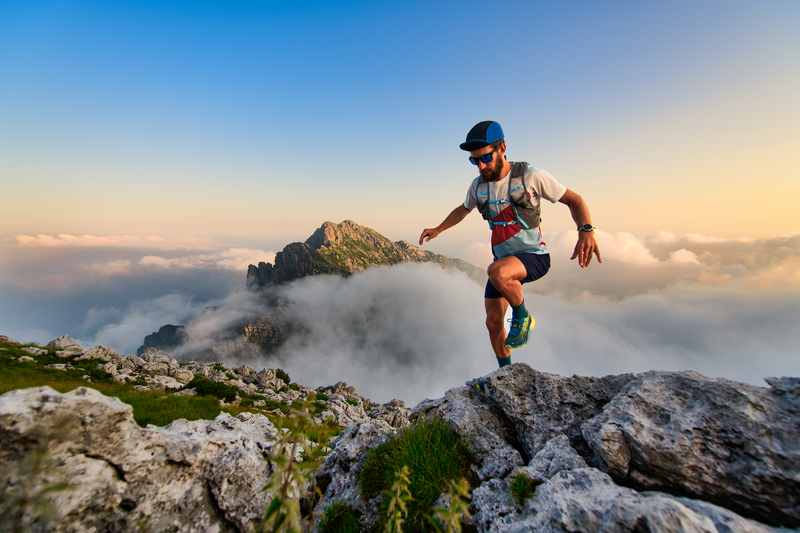
95% of researchers rate our articles as excellent or good
Learn more about the work of our research integrity team to safeguard the quality of each article we publish.
Find out more
REVIEW article
Front. Endocrinol. , 05 August 2022
Sec. Diabetes: Molecular Mechanisms
Volume 13 - 2022 | https://doi.org/10.3389/fendo.2022.950798
This article is part of the Research Topic Novel Insights into the Pathophysiology of Diabetes-related Complications: Implications for Improved Therapeutic Strategies View all 11 articles
Diabetic wound is one of the complications of diabetes and is not easy to heal. It often evolves into chronic ulcers, and severe patients will face amputation. Compared with normal wounds, diabetic wounds have an increased proportion of pro-inflammatory cytokines that are detrimental to the normal healing response. The burden of this disease on patients and healthcare providers is overwhelming, and practical solutions for managing and treating diabetic wounds are urgently needed. Pyroptosis, an inflammatory type of programmed cell death, is usually triggered by the inflammasome. The pyroptosis-driven cell death process is primarily mediated by the traditional signaling pathway caused by caspase -1 and the non-classical signaling pathways induced by caspase -4/5/11. Growing evidence that pyroptosis promotes diabetic complications, including diabetic wounds. In addition, inflammation is thought to be detrimental to wound healing. It is worth noting that the activation of the NLRP3 inflammasome plays a crucial role in the recovery of diabetic wounds. This review has described the mechanisms of pyroptosis-related signaling pathways and their impact on diabetic wounds. It has discussed new theories and approaches to promote diabetic wound healing, as well as some potential compounds targeting pyroptosis and inflammasome signaling pathways that could be new approaches to treating diabetic wounds.
Diabetes is a metabolic disease caused by a variety of etiologies, and the number of people suffering from type 2 diabetes mellitus (T2DM) is growing every year (1). By 2045, the number of people with diabetes is expected to exceed 700 million (7.8% of the global population) (2). Diabetes has over 100 complications, making it the most well-known disease. These complications include cardiovascular disease, peripheral neuropathy, chronic renal failure, stroke, and diabetic wounds or ulcers (3). Diabetic wounds are a condition that affects about 20% of patients with diabetes (4). Diabetic wounds are characterized by impaired healing responses, prolonged inflammation, and reduced epithelialization kinetics in diabetic patients (5).
Wound healing is a physiological response to structural tissue injury, which includes skin damage. Wound healing is a multi-stage process that includes hemostasis, inflammation, proliferation, and remodeling (6). Diabetic wounds heal slower than normal wounds due to the production of pro-inflammatory mediators, ischemia induced by microvascular problems, particular metabolic deficiencies, and decreased production of healing-related components, among other causes (7). As a result, diabetic wounds have a longer course and more complex mechanisms than normal wounds, affecting patients’ morbidity, mortality, and quality of life significantly (8).
Pyroptosis, an type of programmed cell death, is usually associated with inflammatory responses (9). According to new research, cell death pathways include apoptosis, necroptosis, autophagy, ferroptosis, cuproptosis, pyroptosis, and necrosis, and the regulators and effectors of these pathways remain promising therapeutic targets (10). When germs, pathogens, or endotoxins stimulate cells, the caspase family becomes active, triggering pyroptosis, also known as inflammatory cell death. As a result of this process, cell swelling, cell membrane pore formation, cell membrane rupture, inflammasome activation, and finally the release of cell contents and inflammatory mediators all occur, culminating in severe inflammatory responses (11, 12).
In conclusion, this review summarizes the research progress on the relationship between pyroptosis and diabetic wounds. The primary purpose of this review is to clarify the mechanism of pyroptosis-related signaling pathways and their impact on diabetic wound, explore new therapeutic approaches and identify potential therapeutic targets.
Cell death dependent on caspase-1 was originally called apoptosis (13). In 2000, Cookson introduced the term “pyroptosis”. Pyroptosis differs from apoptosis in the morphological features and inflammatory nature of cell death (14, 15). Pyroptosis is involved in the pathogenesis of various diseases, such as tumors, cardiovascular diseases, COVID-19, diabetes and its complications. The mechanism of pyroptosis is regulated by many protein or protein complexes, such as caspase, inflammasome, and Gasdermin. Typically, caspases exist in an inactive precursor form, a pro-caspase. After activation by the inflammasome, caspases cleave Gasdermin and generate a hydrophilic C-terminal domain and a lipophilic N-terminal domain. The N-terminal lipophilic domain oligomerizes and binds to the cell membrane to form a pyroptotic pore, ultimately triggering pyroptosis.
Caspases, a group of proteases with similar structures present in the cytoplasm, are known to drive apoptosis or pyroptosis and play a key role in programmed cell death and inflammation (16). Mammalian caspases fall into two broad categories: apoptotic and inflammatory caspases. Among them, caspase-1, -4, -5, -11, and -12 belong to the family of inflammatory caspases, which are closely related to pyroptosis (17). According to the latest research, the signaling pathways of pyroptosis can be split into four kinds based on different activation modalities: canonical inflammasome signaling pathways (caspase-1), non-canonical inflammasome signaling pathways (caspase-4/5/11), and pyroptotic pathways depending on caspase-3 and caspase-8 (18–20).
The inflammasome is a multi-protein complex assembled by intracellular pattern recognition receptors (PRRs) and is an essential part of the innate immune system (21, 22). Damage-associated molecular patterns (DAMPs), endogenous molecules released by the body’s cell death, namely endogenous danger signals, originate from immune cells activated by damaged or necrotic tissue. Pathogen-associated molecular patterns (PAMPs) are ligand receptors that PRRs recognize and bind, mainly referring to some highly conserved molecular structures shared on the surface of pathogenic microorganisms, such as lipopolysaccharide of G-bacteria. Generally, both PAMPs and DAMPs induce inflammasome activation via PRRs. Inflammasomes can recognize PAMPs or DAMPs and recruit and activate the pro-inflammatory protease caspase-1, thereby inducing cell death under pathological conditions of inflammation and stress. It is known that this process can promote the maturation and secretion of pro-IL-1β and pro-IL-18 during innate immune defense (23–25).
Current research indicates that there are five common inflammasomes, including NLRP1, NLRP3, NLRC4, Pyrin, and AIM2 (26). The NLRP3 inflammasome, in particular, has been widely investigated and is involved in a number of diseases, including type 2 diabetes and diabetic wounds (22, 27). NLRP3 may promote interaction with the pyrin domain (PYD) in ASC in response to immune activators (e.g., PAMPs, DAMPs), other exogenous invaders, or environmental stimuli. Subsequently, the caspase recruitment domain (CARD) of ASC binds to the CARD domain on pro-caspase-1 to generate the NLRP3 inflammasome. The creation of this complex causes pro-caspase-1 to self-cleave, resulting in an active caspase-1 p10/p20 tetramer, and the maturation of the pro-inflammatory cytokines IL-1β and IL-18 from their immature “pro” versions.
Activation of the NLRP3 inflammasome appears to proceed in two steps. The first step involves priming or initiation signals, and many PAMPs or DAMPs are recognized, leading to the activation of NF-κB-mediated signaling that upregulates the transcription of inflammasome-related components, including inactive NLRP3, pro-IL-1β, and pro -IL-18. The second step is the oligomerization of NLRP3 and the subsequent assembly of NLRP3, ASC, and pro-caspase-1 into a complex (28). Based on the available evidence and research results, pro-caspase-1 conversion to caspase-1 is accompanied by the release of mature IL-1β and IL-18. Furthermore, triggering PAMPs and DAMPs causes the creation of ROS, which promotes the assembly and activation of the NLRP3 inflammasome (Figure 1). Therefore, as the center of the inflammatory response, the NLRP3 inflammasome could be a potential therapeutic target for inflammatory illnesses. However, non-canonical inflammasome activation pathways mediated by mouse caspase-11 or human caspase-4/5 are independent of inflammasome activation. Pyroptosis was also successfully induced in response to intracellular lipopolysaccharide (LPS) detection (29). ASC (also known as PYCARD) is a protein containing pyran and CARD domains that aid in inflammasome assembly (30).
Figure 1 NLRP3 inflammasome signaling pathway. It is known that the activation of NLRP3 is divided into two key steps: priming and activation. Signal 1 (priming; left): When pathogens and their products, injury, stress, and other signals stimulate cells, TLR, TNFR, and other receptors can be activated. NF-κB is activated through different pathways, which in turn promotes NLRP3, pro-IL-1β, and pro-IL-18. Provide a material basis for the activation and function of NLRP3 inflammasome. Signal 2 (activation; right): Multiple upstream signaling events are activated by PAMPs and DAMPs. These include K+ efflux, reactive oxygen species (ROS) production, etc. Inflammasome formation activates caspase-1, which cleaves pro-IL-1β and pro-IL-18. Gasdermin is also cleaved and inserted into membranes, forming pores and inducing pyroptosis.
The six members of the gasdermins are Gasdermin A–E and DFNB59 (31). Except for DFNB59, other components have been reported to be associated with the pyroptotic process. A peptide linker connects an N-terminal domain (effector domain that creates the transmembrane pore) to a C-terminal domain (with auto-inhibitory effects). Among gasdermins, gasdermin D(GSDMD) and gasdermin E(GSDME) are the most well-characterized in terms of activation and function (32). Gasdermin family members are mainly expressed in skin, gastrointestinal tract, and immune cells to actively eliminate infected cells through pyroptosis (33, 34). GSDMD is a substrate of caspase-1, a component of the inflammasome responsible for executing pyroptosis and secreting mature IL-1β (35). When caspase cleaves the gasdermin-N domain free, it binds to the lipids on the cell membrane and encourages the creation of pores, which causes the membrane to rapidly lose its integrity and allow the contents of the cell to flow out. Pore-forming activity and pyroptosis are therefore reduced in the absence of GSDMD activation (36). Of course, two critical steps in pyroptosis are inflammasome activation and GSDMD cleavage. Recent studies have found that disruption of mitochondrial membrane potential (MMP) and reactive oxygen species (ROS) production is commonly associated with macrophage pyroptosis (37).
In most cases, the formation of the inflammasome requires pattern recognition receptors (PRRs) as sensors, the adaptor protein ASC (CARD-containing apoptosis-associated speck-like protein) and caspase1 (38). The assembly of inflammasomes triggers the hydrolysis of inactive pro-caspase-1 to active caspase-1, which converts the cytokines pro-IL-1β and pro-IL-18 into mature and bioactive IL-1β and IL-18, respectively (39, 40). Caspase-1 cleaves GSDMD, which is involved in membrane pore formation. Membrane pores cause the production of inflammatory molecules, including IL-1β and IL-18, which causes the cells to expand and eventually develop into pyroptosis (Figure 2).
Figure 2 Molecular mechanism of pyroptosis. In the canonical inflammasome signaling pathway, PAMPs, DAMPs, and extracellular ATP are stimulated by intracellular signaling molecules and assembled with pro-caspase-1 and ASC to form inflammasomes and activated caspase-1. N-GSDMD perforates cell membranes by forming non-selective pores. In addition, IL-1β and IL-18 were secreted in the pores formed by N-GSDMD. In the non-canonical inflammasome signaling pathway, intracytoplasmic LPS activates caspase-4/5/11, which triggers pyroptosis by cleaving GSDMD. However, oxPAPC competes with LPS for binding to caspase-4, thereby inhibiting pyroptosis. Cleavage of GSDMD leads to K+ efflux that ultimately mediates the assembly of the NLRP3 inflammasome. Moreover, cleavage of GSDMD leads to cleavage of pro-IL-1β and pro-IL-18. In the caspase-3-mediated pathway, active caspase-3 cleaves GSDME to form N-GSDME, inducing pyroptosis. In the caspase-8-mediated pathway, inhibition of TAK1 induces the activation of caspase-8, which cleaves GSDMD, leading to pyroptosis. In addition, under hypoxia, TNF-α activates caspase-8 after apoptosis to pyroptosis, which can regulate the transcription of GSDMC.
Many multifactorial diseases, such as gouty arthritis, atherosclerosis, and type 2 diabetes, are exacerbated by NLRP3-mediated inflammation. PAMPs/DAMPs that activate NLRP3 include hyperglycemia, fatty acids, protein aggregates, and extracellular ATP, among many others (41, 42). The mechanism of activation of the NLRP3 inflammasome is clearly described in paragraph 2 (Figure 1). The inflammasome effector cytokines IL-1β and IL-18, due to NLRP3 activation, are major effector molecules that exacerbate these diseases. As a result, maintaining optimal cellular homeostasis and health requires fine-tuning NLRP3 inflammasome activity (43).
Mouse caspase-11 and its human orthologs caspase-4 and -5 are involved in non-canonical inflammasome signaling, and investigations of mouse caspase-11 have led to various findings concerning their immunological role (44). The TLR4/MD2 complex identified the pathogen-associated molecular pattern to activate inflammatory responses as lipopolysaccharide (LPS), a significant component of Gram-negative bacteria’s outer membrane (45). Myeloid differentiation factor 2 (MD2) is a key mediating protein required for the dimerization/activation of TLR4 (Toll-like receptor 4). LPS activates pro-caspase-4/5/11, and pro-caspase-4/5/11 initiates pyroptosis-mediated cell death (46). Caspase-4/5/11 binds to LPS and cleaves the 53-kDa precursor form of the GSDMD protein molecule, resulting in the formation of the N-terminus of the mature GSDMD p30 fragment. Thus, the formation of pores in the cell membrane results in the release of IL-1β and IL-18 from the cells and induction of pyroptosis (47, 48). Caspase-4/5/11 can also stimulate NLRP3-mediated caspase-1 initiation and IL-1β/IL-18 via GSDMD cleavage. GSDMD has been identified as an important downstream component of both canonical and non-canonical inflammasome pathways involved in pyroptosis (49, 50) (Figure 2).
Caspase-3, an apoptosis-executing protein, is the main protein responsible for the cleavage and activation of GSDME. In addition to GSDMD, GSDME also plays a crucial role in pyroptosis (51). Caspase-3 is activated by the mitochondrial and death receptor pathways and cleaves GSDME to form GSDME-N fragments, which lead to plasma membrane pore formation, cell swelling, and pyroptosis (52). GSDME protein molecules can be cleaved into N-terminal and C-terminal fragments by caspase-3. GSDME-N components are equivalent in function and purpose to GSDMD-N components (53). GSDME directly induces tumor cell pyroptosis through Caspase-3 and indirectly acts on T lymphocytes through Granzyme B, acting as a tumor suppressor gene (54). Furthermore, when chemotherapeutic drugs activate Caspase-3, primary human cells exhibit GSDME-dependent pyroptosis, providing new insights into cancer chemotherapy (54). These findings indicate that different caspase substrates, rather than activated caspases, influence the type of cell death induced.
Recent research has revealed unexpected roles for caspase-8’s enzymatic activity and scaffold function in inflammasome activation and pyroptosis induction (55). The activation of the ASC-caspase-1 inflammasome is caused by the production of catalytically inactive caspase-8, leading to GSDMD-mediated pyroptosis (56). In the presence of inhibitors, activation of caspase-8 cleaves GSDMD leading to pyroptosis. More research is needed to see if caspase-8 can directly cleave GSDMD or if other intermediary substrates, other than caspase-1/11, are necessary to produce the pore-forming p30 subunit (57). However, pure active caspase-8 has been demonstrated to cleave recombinant mouse GSDMD creating p30 pore-forming fragments (58). Following caspase-8 activation in tumor cells, GSDMC has been found to mediate tumor necrosis. The pyrogenic cell death mediated by GSDMC/caspase-8 provides essential insights into the pyroptotic pathway in cancer cells (59). Based on the current research results, the mechanism of pyroptosis induced by caspase-8 is not clear enough, and the research on its mechanism is a direction worthy of attention in the future.
Acute wound healing includes four stages: hemostasis, inflammation, proliferation, and remodeling (60). Excessive or long-term inflammation is one of the main characteristics of chronic wounds, since this condition negatively affects wound healing and leaves scars behind (61). The NLRP3 inflammasome is expressed in epithelial tissues, such as skin. As the first line of defense against external threats, it can participate in the skin’s innate immunity (62, 63).
The role of the NLRP3 inflammasome in the early stages of cutaneous wound healing is increasingly being investigated. Results of studies have demonstrated the effect of mulberry leaf and fruit extract (MLFE) on skin wound healing and the involvement of the NLRP3 inflammasome (64). Concurrently, research has revealed the function of the NLRP3 inflammasome in the proliferative and remodeling phases of wound healing (65). A combination of mulberry leaf and fruit extract (MLFE) provided better anti-obesity and anti-inflammatory benefits than mulberry leaf extract alone. Under obese conditions, the expression of the NLRP3 inflammasome and its associated markers (pro-caspase-1, IL-1β-precursor and IL-1β-mature) is higher than basal levels. Among them, the NLRP3 inflammasome is inhibited during the inflammatory phase of skin wound healing (66). The results showed that the addition of MLFE reduced body fat mass, fasting blood glucose levels, blood lipid levels, and hepatotoxicity. Therefore, body fat mass and fasting blood glucose may be potential indicators of delayed wound healing in obese patients. Although the exact activator or mechanism of the NLRP3 inflammasome is not known, it can be determined that MLFE normalizes the levels of the NLRP3 inflammasome and suppresses skin inflammatory responses during the early stages of wound healing in obesity (67). Deletion of the NLRP3 inflammasome will result in a decrease in proinflammatory cytokines such as IL-1β and TNF-α and delayed angiogenesis (68). MLFE may have potential therapeutic value in treating obesity and obesity-related complications, and NLRP3 might be a promising target in the fight against obesity wounds since it may promote the early healing of wounds. However, this study focused on detecting NLRP3-related proteins and ignored the close relationship between the inflammasome and pyroptosis. The article only detects pro-caspase-1 is not enough. If the expression of GSDMD and caspase-1 protein is detected, it is enough to show that it is related to pyroptosis, which may be another way to explore.
DNA nanomaterials with distinctive spatial configurations are known as tetrahedral framework nucleic acids (TFNAs) (69). TFNAs have excellent biosafety with anti-inflammatory, antioxidant, anti-fibrotic, angiogenic, and skin wound healing activities with little toxicity (70). In vitro and in vivo research have uncovered that TFNAs increase corneal transparency, speed wound reepithelialization, and play a positive role in corneal epithelial wound healing (71). TFNAs are not only beneficial for corneal wound healing but also for skin wound healing. The results of the study showed that treatment with TFNAs accelerated the healing process of skin wounds and reduced scarring. This is the first report that nanophase materials with nucleic acid biological properties can accelerate wound healing and reduce scarring, suggesting that TFNA can be used to promote skin tissue regeneration (72).
In addition, studies have shown that TFNA can promote diabetic wound healing by accelerating processes such as angiogenesis, epithelialization, and collagen deposition. Through their antioxidant activity via the PI3K/Akt/Nrf2/HO-1 signaling pathway, TFNAs can protect endothelial cell function, reduce inflammation, and prevent oxidative damage. The PI3K/Akt/Nrf2/HO-1 signaling pathway is regulated by metformin and plays a key role in metformin-induced osteogenesis. Therefore, the use of TFNAs could help diabetic wounds recover faster (73). As various local or systemic diseases promote skin inflammation, fibrosis (the result of a dysregulated tissue repair response) begins to dominate the repair process when the intensity or duration of skin damage exceeds the ability of the tissue to repair. As a result, medicines that substantially prevent skin fibrosis while also reducing immunogenicity, inflammation, apoptosis, and pyroptosis are required. TFNA inhibits the pyroptotic pathway and reduces inflammatory cytokine levels and skin collagen content in studies. Both NLRP3 inflammasome and pro-caspase-1 levels were down-regulated after TFNA treatment, indicating that the inflammasome was reduced and the active form of caspase-1 was reduced, resulting in a subsequent down-regulation of N-terminal GSDMD levels. The results showed that TFNA has anti-inflammatory and anti-fibrotic abilities without cytotoxicity (74). In this study, proteins related to pyroptosis and inflammasome signaling pathways were detected, including pro-caspase-1, caspase-1, NLRP3, GSDMD, etc. In a nutshell, TFNAs have important research significance for skin wound healing and have been confirmed to be closely related to pyroptosis and inflammasome pathways.
The research progress of bioactive glass (BG) in soft tissue repair is relatively rapid, especially in wound healing. The findings suggest that BG may accelerate wound closure, granulation development, collagen deposition, and angiogenesis (75). The method used in this study is that BG inhibits endothelial cell pyroptosis and promotes wound healing by regulating the Cx43/ROS signaling pathway. Therefore, BG inhibits the activation of caspase-1 by the NLRP3 inflammasome, attenuate the perforation activity of GSDMD, and ultimately inhibit the pyroptosis of endothelial cells (76). In addition, BG inhibits the production of ROS while regulating the expression of connexin 43 (Cx43) (77). Subsequently, BG promotes the formation of blood vessels resulting in accelerated wound healing. The study also made further proofs showed that BG could reduce the expression of Cx43 and the level of ROS, which strongly suggested that BG could inhibit the pyroptosis of endothelial cells (78). It has been shown that inhibiting pyroptosis enhances angiogenesis in many animal models, implying that BG also enhances angiogenesis. It can be concluded that BG can promote wound healing by impeding pyroptosis through the Cx43/ROS signaling pathway. Even though this study did not directly regulate pyroptosis and inflammasome signaling pathways, it did confirm their close relationship. It inspires us is that fewer drugs and methods inhibit pyroptosis, but it can be achieved by modulating other signaling pathways.
While the wound healing process is complex and dynamic, the pyroptosis and inflammasome pathways also have complex connections. There is currently a lack of studies on the crosstalk between multiple signaling pathways in wound healing or on pyroptosis and changes in the NLRP3 inflammasome at different phases of wound healing. Therefore, further in-depth study of other factors in the wound healing process will also provide us with new insights into the mechanism of wound healing. New treatment methods such as new carriers and new Chinese herbal extracts have been evaluated and will be used in the clinic in the future. How to optimize individualized treatment strategies while improving chronic inflammatory and pyroptotic states during wound healing needs to be considered.
Diabetic wound pathogenesis is complicated and involves numerous pathways. There is some evidence that suggests that the local hyperglycemic environment is the main factor leading to diabetic wounds, but recent research shows that factors such as oxidative stress damage, accumulation of advanced glycation end products (AGEs), and chronic inflammation are closely related to diabetic wounds (79, 80). Persistent inflammatory activation is the leading cause of chronic refractory diabetic wound, and an essential factor leading to diabetic foot ulcer (DFU), gangrene, amputations, and even the root cause of prolonged hospital stays and increased wound management costs (81).
Targeting the NLRP3 inflammasome, which plays a role in the pathophysiology of numerous inflammatory disorders, could be a potential target for enhancing diabetic wound healing. Neutrophils release extracellular traps (NETs) to defend against pathogens that induce tissue damage (82). NETs have been detected in diabetic wounds and have been associated with impaired healing processes, but the mechanism by which NETs pause wound healing and their role in promoting inflammatory dysregulation remain unclear (83). Overproduced NETs in diabetic wounds trigger NLRP3 inflammasome activation and IL-1β release in macrophages (84). Meanwhile, NETs up-regulates the levels of NLRP3 and pro-IL-1β through the TLR-4/TLR-9/NF-κB signaling pathway, triggering the production of ROS, and activating the NLRP3 inflammasome (85). Furthermore, in a diabetic rat model, NET digestion by DNase I reduced NLRP3 inflammasome activation, altered immune cell infiltration, and expedited wound healing (86).
In recent years, most studies have focused on the role of the inflammasome, especially NLRP3 and pyroptosis, on wound healing. The NLRP3 inflammasome has been characterized in a corneal epithelial wear model in which wound healing and non-regeneration of diabetic corneal wounds have been studied. Recent studies suggest that the NLRP3 inflammasome-mediated inflammation and pyroptosis contribute to the pathogenesis of diabetic keratopathy (DK) (87). NLRP3 is necessary for corneal wound healing and nerve regeneration in physiological circumstances (88). In diabetics, however, prolonged activation of the NLRP3 inflammasome causes corneal wound healing to be delayed and nerve regeneration to be hindered. In addition, inhibiting the AGEs/ROS/NLRP3 inflammasome axis genetically and pharmacologically greatly accelerates diabetic corneal epithelial wound closure and nerve regeneration (87). The study highlighted that ocular surface damage in diabetic mice might be related to ROS/NLRP3/Caspase-1/IL-1β signaling pathway (89, 90). Activation of the NLRP3 inflammasome by high glucose-induced P2X7R (purinergic ligand-gated ion channel 7 receptor) affects the pathogenesis of diabetic retinopathy (DR) (91). Therefore, it is known that NLRP3 inflammasome and pyroptosis play critical roles in DK and DR. In addition, NLRP3 and pyroptosis also have significant effects on diabetic wounds (Table 1).
Table 1 Compounds or Molecules Inhibiting the Pyroptosis Signaling Pathway for the Treatment and Management of Diabetic Wound.
Glyburide is a commonly used sulfonylurea drug to treat type 2 diabetes (92). The capacity to suppress the NLRP3 inflammasome through a mechanism different from its ability to enhance insulin release from pancreatic beta-cells was recently discovered, and glyburide improves wound healing in diabetic mice (98). Cryopyrin/NALP3/NLRP3 is an essential component of the inflammasome triggered by PAMPs, DAMPs, and crystalline substances (99). Glyburide is the first chemical able to block PAMPs, DAMPs, and crystal-induced IL-1β production by acting upstream of cryoproteins. Inflammasome activity was persistent in macrophages (mø) isolated from diabetes and db/db mice wounds, which was associated with low expression levels of endogenous inflammasome inhibitors. Because a wound-conditioned medium activates caspase-1 and stimulates the production of IL-1β and IL-18 in cultured cells through a ROS-mediated route, soluble components in these wound biochemical conditions are sufficient to activate the inflammasome (100). Inhibiting inflammasome activity in wounds of db/db mice by topical application of pharmacological inhibitors improves wound healing. This treatment shifts from a pro-inflammatory state to a pre-healing state and increases pre-healing growth factor levels.
Bacillus subtilis is a probiotic that modulates immune responses and reshapes the gut flora (101). Bacillus subtilis (WB800N) has the ability to activate TLRs (Toll-like receptors) and enhance immune responses (102). In recent years, there has been evidence that diabetic wounds are associated with gut microbiota. Studies have shown that amoxicillin can reduce the alpha and beta diversity of the intestinal microbiota in mice, leading to intestinal microbiota disturbances, thereby alleviating diabetic wounds (103). It is also significant that Bacillus subtilis (WB800N) can relieve diabetic wounds by regulating Toll-like receptor-2 (TLR2) (93). TLR2 is a major innate immune response factor, and of immune response activation benefits diabetic wound healing (104). The results showed that Bacillus subtilis (WB800N) could increase the expression of TLR2, NLRP3, ASC and Caspase-1 in diabetic wound mice. However, the TLR2 antagonist SsnB could reduce the expression of TLR2, NLRP3, ASC and Caspase-1 in diabetic wound mice. NLRP3/ASC is required for Caspase-1 activation and pro-IL-1β cleavage to generate mature IL-1β. In conclusion, Bacillus subtilis (WB800N) promotes inflammatory response in diabetic wound mice by activating TLR2. This study only explored the NLRP3 inflammasome signaling pathway but lacked the detection of pyroptosis-related proteins. The authors believe that Bacillus subtilis (WB800N) promotes cell apoptosis, but it is actually more likely to be pyroptosis, which is worth exploring in the future.
Skin infections and the spread of Staphylococcus aureus (S. aureus) in mice are limited by perforin-2, an innate immune molecule against intracellular bacteria (105). The study showed the accumulation of S. aureus within the epidermal cells of DFU without clinical signs of infection due to significant inhibition of perforin-2 (106). Evidence from studies shows that S. aureus within the epidermis of DFU triggers AIM2 inflammasome activation and pyroptosis. From the results, increased induction of AIM2 inflammasome, ASC-pyroptosome, and IL-1β was found in non-healing DFU (107). The correlation of AIM2 with healing outcomes suggests that AIM2 has a central function in regulating the inflammatory response to DFU. There is evidence that the increase in IL-1β involved in pyroptosis is accompanied by an increase in the AIM2 inflammasome, resulting in the oligomerization of ASCs into pyroptosomes which then triggers the activation of pro-caspase-1 resulting in the cleavage of porins such as Gasdermin D, and the lysis of cells involved in inflammation (108). In patients with DFU, the inhibition of perforin-2, intracellular accumulation of S. aureus, and related blepharoptosis lead to the inhibition of wound healing and the persistence of inflammation (94). Intracellular S. aureus accumulates in the DFU epidermis as a result of perforin-2 suppression, triggering activation of the AIM2 inflammasome, which results in caspase-1-mediated IL-1β activation and proteolysis of the pore-forming gasdermin D processing. As a consequence of this cascade, holes are formed in the plasma membrane, opening up a pathway for pyroptosis and for the release of intracellular components. As a result, inflammatory mediators and accumulated intracellular S. aureus are released, leading to chronic inflammation and direct inhibition of wound healing. Further, it was determined that gasdermin D, one of the substrates of caspase-1, is also cleaved and activated by DFU (109). This study is the first to demonstrate that intracellular S. aureus can inhibit perforin-2 in DFU. Pyroptosis is the predominant form of cell death in DFU, but the possibility of other forms of cell death such as necroptosis, ferroptosis, and cuproptosis remains to be tested.
A growing body of research suggests that Bletilla striata polysaccharide (BSP), the main active ingredient in Bletilla striata, promotes normal or diabetic wound healing (110). This is due to the fact that BSP improves diabetic wound healing by infiltrating fibroblasts and enhancing collagen synthesis in the skin wound tissue. Furthermore, BSP is suitable for medical applications, including wound dressings, hydrogels, tissue engineering scaffolds, and drug delivery vehicles (111–113). This study has demonstrated that the therapeutic effect of BSP on DFU is mediated by inhibiting HG-induced NLRP3 inflammasome activation in macrophages, which increases insulin sensitivity in endothelial cells (95). The results showed that the expressions of TXNIP, NLRP3, pro-caspase-1, cleaved-caspase-1, pro-IL-1β and cleaved-IL-1β were increased in diabetic skin wounds. However, BSP treatment resulted in decreased levels of some proteins, such as pro-caspase-1, cleaved-caspase-1, pro-IL-1β, and cleaved-IL-1β. In addition, the sensitivity of BSP to insulin was improved.
BSP has a protective effect on macrophages and can significantly reduce the amount of ROS produced by HG, thereby preventing macrophages from being induced by HG. In addition to this, IL-1β secretion and NLRP3 inflammasome activation were also inhibited (114, 115). Additionally, BSP is thought to play an important role in preventing HG-induced endothelial cell inactivation and ROS homeostasis imbalance (116). The results showed that BSP more effectively protected BMDMs (Bone marrow-derived macrophages) from the HG-induced ROS production, inhibited NLRP3 inflammasome activation and reduced IL-1β secretion. It also reduced the abnormal production of ROS in CMECs (cardiac microvascular endothelial cells) while maintaining cell viability (114). Macrophage infiltration and angiogenesis in DFU are inhibited by IL-1β, TNF-α, and monocyte chemoattractant protein 1, which ultimately interfere with wound healing (117). This study showed that the dosing regimen of BSP affects the local production of TNF-α and IL-1β (especially in skin tissue), excluding serum levels.
BSP reduced macrophage infiltration and increased angiogenesis in cutaneous wound tissue. These results are in line with increased TNF-α and IL-1β levels in skin wound tissue. The potential of BSP to improve diabetic wound healing may be due to its inhibition of NLRP3 inflammasome activation in macrophages. Although the study did not explicitly mention that BSP inhibits the pyroptotic pathway, it is closely related to NLRP3. In addition to promoting the maturation and release of IL-1β, the over-activation of the NLRP3 inflammasome also leads to the over-activation of pyroptosis. In future research, we can consider detecting the expression of ASC-pyroptosome and GSDMD and explore the molecular mechanism of the pyroptosis and inflammasome pathway.
Paeoniflorin is one of the main active components in Paeonia alba Radix, with antioxidant and anti-inflammatory effects (118). The use of paeoniflorin can alleviate diabetic nephropathy by inhibiting the release of inflammatory cytokines and chemokines (TNF-α, IL-1β, and MCP-1) through toll-like receptor 2 (TLR2) inactivation (119). In recent years, traditional Chinese medicine has been recommended as adjuvant therapy for DFU patients (120). Herbal products containing phenolic compounds, terpenoids, or glycosides have positively affected managing diabetic complications (121). Activation of NLRP3 inflammasome in diabetic wounds can maintain inflammation and delay the wound healing process, so inhibiting the activation of NLRP3 inflammasome and the production of IL-1β can effectively promote the healing of diabetic wounds. Paeoniflorin treatment reduced inflammatory cells and decreased the expression levels of NLRP3 and cleaved-caspase-1. In addition, paeoniflorin significantly down-regulated IL-1β, IL-18 and TNF-α levels in DFU.
Paeoniflorin efficiently suppressed NLRP3 and NF-B-mediated DFU inflammation by inhibiting CXCR2, according to in vitro findings (122). CXCR2 is a neutrophil receptor that is activated by chemokines like CXCL1 and CXCL2. The activation of the NF-κB pathway in response to IL-1β drives the production of these chemokines. Additionally, studies have shown that TNF-α can regulate the chemokine network in inflammation-related diseases through the NF-κB signaling pathway (123, 124). CXCR2 has critical functions in neutrophil activation and recruitment at inflammatory sites, which provides a reference for positioning CXCR2 as a drug target for many inflammatory diseases. In HG-treated HaCaT cells, blockade of CXCR2 blunts NLPR3/ASC inflammasome activation. In conclusion, paeoniflorin inhibits the formation of NLRP3/ASC/caspase-1 inflammasome and the NF-κB transcription by blocking CXCR2, inhibiting the release of pro-inflammatory cytokines, and promoting the healing of diabetic wounds (96). Although the current evidence is insufficient and further exploration is needed, studies have shown that paeoniflorin is a potential drug for the treatment of DFU.
Heparan sulfate (HS) is a structural element of tissue scaffold and regulates activities of locally synthesized proteolytic enzymes, morphogens, chemokines and growth factors (125, 126). Morphogens are proteins that encode transcription factors, receptors and regulate translation. HS was shown to improve the healing of diabetic wounds in rats by reducing the inflammatory response (127). DAMPs are increased during diabetes to act as activators of the NLRP3 inflammasome and these activators promote inflammasome assembly leading to insulin resistance and organ dysfunction (128). Based on the existing results, it is speculated that HS may promote wound healing by reducing neutrophil infiltration and accumulation of macrophages (129). Moreover, diabetic wounds are more prone to inflammation than different types of wounds. Cleaved-IL-1β and IL-18 play key roles in wound inflammation through interactions with pro-inflammatory cytokines. Research evidence indicates that antagonists of cleaved-IL-1β or IL-18 may be used to treat inflammatory diseases such as gout, arthritis, or arthritic pain. Among them, TNF-α is a representative pro-inflammatory factor, and high levels of TNF-α can amplify and prolong the inflammatory response. The study found that HS significantly reduced Cleaved-IL-1β, IL-18 and TNF-α levels in diabetic rats (130). In addition, it may be possible for HS to work as an anti-inflammatory mechanism by inhibiting the NLRP3 inflammasome activity, so that controlling the level of NLRP3 inflammasome activity can be a method for treating diabetic wounds (97). In the process of wound healing, HS can promote wound healing in diabetic rats. The activation of Cleaved-IL-1β, IL-18 and TNF-α was decreased, and the expressions of NLRP3 and ASC were decreased in the HS group. HS inhibits the inflammatory response and promotes wound healing during diabetic wound healing by down-regulating NLRP3 inflammasome and Cleaved-IL-1β. Therefore, reducing the production of inflammatory factors and the reduction of neutrophil infiltration in the diabetic wound will improve the diabetic wound environment and ultimately shorten the wound healing time (Figure 3).
Figure 3 The therapeutic significance of potential molecules or materials for diabetic wound by inhibiting pyroptosis and inflammasome NLRP3 and AIM2.
The NLRP3 inflammasome plays an essential physiological role in skin wound healing. Therefore, targeting NLRP3 inflammasome activity and its effectors may be an effective therapeutic strategy to reduce chronic inflammation and promote healing in diabetic wounds. During wound healing, the transient activity of the inflammasome promotes the propagation of wound inflammation and is critical for both epidermis and dermis healing (131). Among the strategies for inhibiting pyroptosis and the NLRP3 inflammasome signaling pathway, drugs such as glyburide and metformin are the closest to clinical translation. In published studies, a variety of compounds can be used to directly or indirectly inhibit NLRP3 inflammasome activity in diabetic wounds. Improves diabetic wound healing by affecting pyroptosis and NLRP3 inflammasome or upstream and downstream signaling. Most researchers are now keen to inhibit the inflammasome pathway to improve diabetic wounds. However, it is often overlooked that inflammation and pyroptosis are closely related. The research horizon should be broadened, and more attention should be paid to pyroptosis while detecting the inflammasome pathway, which may lead to surprising results.
In recent years, numerous studies have demonstrated that pyroptosis plays a vital role in developing diabetes and its complications. This article has reviewed the impact and role of pyroptosis in diabetic wound healing and the inflammasome is a crucial player in pyroptosis. Danger signals or stimuli cause the activation of caspase-1/4/5/11/3/8 and release IL-1β, IL-18 and other inflammatory factors, resulting in cell pyroptosis. Pyroptosis inhibits wound healing and prolongs the inflammatory response in diabetic wounds, and multiple studies have demonstrated that inhibition of pyroptosis improves wound healing. This review has described some potential drugs and molecules that may be helpful to targets for managing and treating diabetic wounds in the future.
At present, there are few studies on pyroptosis in diabetic wounds, and extensive research is needed to deeply analyze and elucidate the mechanisms and pathophysiological roles of pyroptosis and inflammasome in diabetic wounds. Furthermore, unlike common cell death mechanisms, ferroptosis and cuproptosis have become research hotspots in recent years. Among the forms of cell death in wound healing or diabetic wound healing, the possibility of other forms of cell death (such as necroptosis, ferroptosis, and cuproptosis) remains to be confirmed and is an area worthy of future attention and research. A more in-depth examination of the various modes of cell death could provide new insights into the pathogenesis and development of diabetic wounds.
XM was responsible for the literature review and writing. XW, WH and YL were responsible for proofreading. XN and FW were responsible for correction. All authors contributed to the article and approved the submitted version.
This work was supported by the National Natural Science Foundation of China (82160770, 81960741, 82060687), the Guizhou Provincial Natural Science Foundation (QKH-J-2020-1Z070), Outstanding Young Scientific and Technological Talents Project of Guizhou Province (2021-5639), Dendrobium Specialized Class Project of Guizhou Province (QSKH-2019003).
The authors declare that the research was conducted in the absence of any commercial or financial relationships that could be construed as a potential conflict of interest.
All claims expressed in this article are solely those of the authors and do not necessarily represent those of their affiliated organizations, or those of the publisher, the editors and the reviewers. Any product that may be evaluated in this article, or claim that may be made by its manufacturer, is not guaranteed or endorsed by the publisher.
1. IDF releases report of global survey on access to medicines and supplies for people with diabetes. Diabetes Res Clin Pract (2017) 129:224–5. doi: 10.1016/j.diabres.2017.06.001
2. Saeedi P, Petersohn I, Salpea P, Malanda B, Karuranga S, Unwin N, et al. Global and regional diabetes prevalence estimates for 2019 and projections for 2030 and 2045: Results from the international diabetes federation diabetes atlas, 9th edition. Diabetes Res Clin Pract (2019) 157:107843. doi: 10.1016/j.diabres.2019.107843
3. Nathan DM. Long-term complications of diabetes mellitus. N Engl J Med (1993) 328:1676–85. doi: 10.1056/NEJM199306103282306
4. Siegel KR, Ali MK, Zhou X, Ng BP, Jawanda S, Proia K, et al. Cost-effectiveness of interventions to manage diabetes: Has the evidence changed since 2008? Diabetes Care (2020) 43:1557–92. doi: 10.2337/dci20-0017
5. Alavi A, Sibbald RG, Mayer D, Goodman L, Botros M, Armstrong DG, et al. Diabetic foot ulcers: Part i. pathophysiology and prevention. J Am Acad Dermatol (2014) 70:e1–18:1. doi: 10.1016/j.jaad.2013.06.055
6. Guo S, DiPietro LA. Factors affecting wound healing. J Dent Res (2010) 89:219–29. doi: 10.1177/0022034509359125
7. Burgess JL, Wyant WA, Abdo Abujamra B, Kirsner RS, Jozic I. Diabetic wound-healing science. Med (Kaunas) (2021) 57:1072. doi: 10.3390/medicina57101072
8. Sanapalli BKR, Yele V, Singh MK, Thaggikuppe Krishnamurthy P, Karri VVSR. Preclinical models of diabetic wound healing: A critical review. Biomed Pharmacother (2021) 142:111946. doi: 10.1016/j.biopha.2021.111946
9. Bergsbaken T, Fink SL, Cookson BT. Pyroptosis: host cell death and inflammation. Nat Rev Microbiol (2009) 7:99–109. doi: 10.1038/nrmicro2070
10. Moujalled D, Strasser A, Liddell JR. Molecular mechanisms of cell death in neurological diseases. Cell Death Differ (2021) 28:2029–44. doi: 10.1038/s41418-021-00814-y
11. Man SM, Karki R, Kanneganti T-D. Molecular mechanisms and functions of pyroptosis, inflammatory caspases and inflammasomes in infectious diseases. Immunol Rev (2017) 277:61–75. doi: 10.1111/imr.12534
12. Song H, Yang B, Li Y, Qian A, Kang Y, Shan X. Focus on the mechanisms and functions of pyroptosis, inflammasomes, and inflammatory caspases in infectious diseases. Oxid Med Cell Longevity (2022) 2022:1–21. doi: 10.1155/2022/2501279
13. Hilbi H, Moss JE, Hersh D, Chen Y, Arondel J, Banerjee S, et al. Shigella-induced apoptosis is dependent on caspase-1 which binds to IpaB. J Biol Chem (1998) 273:32895–900. doi: 10.1074/jbc.273.49.32895
14. Brennan MA, Cookson BT. Salmonella induces macrophage death by caspase-1-dependent necrosis. Mol Microbiol (2000) 38:31–40. doi: 10.1046/j.1365-2958.2000.02103.x
15. Cookson BT, Brennan MA. Pro-inflammatory programmed cell death. Trends Microbiol (2001) 9:113–4. doi: 10.1016/S0966-842X(00)01936-3
16. Kesavardhana S, Malireddi RKS, Kanneganti T-D. Caspases in cell death, inflammation, and gasdermin-induced pyroptosis. Annu Rev Immunol (2020) 38:567–95. doi: 10.1146/annurev-immunol-073119-095439
17. Man SM, Kanneganti T-D. Converging roles of caspases in inflammasome activation, cell death and innate immunity. Nat Rev Immunol (2016) 16:7–21. doi: 10.1038/nri.2015.7
18. Mandal R, Barrón JC, Kostova I, Becker S, Strebhardt K. Caspase-8: The double-edged sword. Biochim Biophys Acta Rev Cancer (2020) 1873:188357. doi: 10.1016/j.bbcan.2020.188357
19. Jiang M, Qi L, Li L, Li Y. The caspase-3/GSDME signal pathway as a switch between apoptosis and pyroptosis in cancer. Cell Death Discovery (2020) 6:112. doi: 10.1038/s41420-020-00349-0
20. Jorgensen I, Rayamajhi M, Miao EA. Programmed cell death as a defence against infection. Nat Rev Immunol (2017) 17:151–64. doi: 10.1038/nri.2016.147
21. Broz P, Dixit VM. Inflammasomes: mechanism of assembly, regulation and signalling. Nat Rev Immunol (2016) 16:407–20. doi: 10.1038/nri.2016.58
22. Guo H, Callaway JB, Ting JP-Y. Inflammasomes: mechanism of action, role in disease, and therapeutics. Nat Med (2015) 21:677–87. doi: 10.1038/nm.3893
23. Qiu Z, He Y, Ming H, Lei S, Leng Y, Xia Z-Y. Lipopolysaccharide (LPS) aggravates high glucose- and Hypoxia/Reoxygenation-induced injury through activating ROS-dependent NLRP3 inflammasome-mediated pyroptosis in H9C2 cardiomyocytes. J Diabetes Res (2019) 2019:8151836. doi: 10.1155/2019/8151836
24. Huang Y, Xu W, Zhou R. NLRP3 inflammasome activation and cell death. Cell Mol Immunol (2021) 18:2114–27. doi: 10.1038/s41423-021-00740-6
25. Kovacs SB, Miao EA. Gasdermins: Effectors of pyroptosis. Trends Cell Biol (2017) 27:673–84. doi: 10.1016/j.tcb.2017.05.005
26. Li Y, Huang H, Liu B, Zhang Y, Pan X, Yu X-Y, et al. Inflammasomes as therapeutic targets in human diseases. Signal Transduct Target Ther (2021) 6:247. doi: 10.1038/s41392-021-00650-z
27. Anand PK. Lipids, inflammasomes, metabolism, and disease. Immunol Rev (2020) 297:108–22. doi: 10.1111/imr.12891
28. Ozaki E, Campbell M, Doyle SL. Targeting the NLRP3 inflammasome in chronic inflammatory diseases: current perspectives. J Inflammation Res (2015) 8:15–27. doi: 10.2147/JIR.S51250
29. Kayagaki N, Wong MT, Stowe IB, Ramani SR, Gonzalez LC, Akashi-Takamura S, et al. Noncanonical inflammasome activation by intracellular LPS independent of TLR4. Science (2013) 341:1246–9. doi: 10.1126/science.1240248
30. Masumoto J, Taniguchi S, Ayukawa K, Sarvotham H, Kishino T, Niikawa N, et al. ASC, a novel 22-kDa protein, aggregates during apoptosis of human promyelocytic leukemia HL-60 cells. J Biol Chem (1999) 274:33835–8. doi: 10.1074/jbc.274.48.33835
31. Shi J, Gao W, Shao F. Pyroptosis: Gasdermin-mediated programmed necrotic cell death. Trends Biochem Sci (2017) 42:245–54. doi: 10.1016/j.tibs.2016.10.004
32. Be B, Esparza AN, Zhu H, Wang S. Gasdermin d in pyroptosis. Acta Pharm Sin B (2021) 11(9):2768–82. doi: 10.1016/j.apsb.2021.02.006
33. Feng S, Fox D, Man SM. Mechanisms of gasdermin family members in inflammasome signaling and cell death. J Mol Biol (2018) 430:3068–80. doi: 10.1016/j.jmb.2018.07.002
34. Shi J, Zhao Y, Wang K, Shi X, Wang Y, Huang H, et al. Cleavage of GSDMD by inflammatory caspases determines pyroptotic cell death. Nature (2015) 526:660–5. doi: 10.1038/nature15514
35. He W, Wan H, Hu L, Chen P, Wang X, Huang Z, et al. Gasdermin d is an executor of pyroptosis and required for interleukin-1β secretion. Cell Res (2015) 25:1285–98. doi: 10.1038/cr.2015.139
36. Kuang S, Zheng J, Yang H, Li S, Duan S, Shen Y, et al. Structure insight of GSDMD reveals the basis of GSDMD autoinhibition in cell pyroptosis. Proc Natl Acad Sci USA (2017) 114:10642–7. doi: 10.1073/pnas.1708194114
37. Wang Y, Shi P, Chen Q, Huang Z, Zou D, Zhang J, et al. Mitochondrial ROS promote macrophage pyroptosis by inducing GSDMD oxidation. J Mol Cell Biol (2019) 11:1069–82. doi: 10.1093/jmcb/mjz020
38. He Y, Hara H, Núñez G. Mechanism and regulation of NLRP3 inflammasome activation. Trends Biochem Sci (2016) 41:1012–21. doi: 10.1016/j.tibs.2016.09.002
39. Martinon F, Burns K, Tschopp J. The inflammasome: a molecular platform triggering activation of inflammatory caspases and processing of proIL-beta. Mol Cell (2002) 10:417–26. doi: 10.1016/s1097-2765(02)00599-3
40. Wang L, Manji GA, Grenier JM, Al-Garawi A, Merriam S, Lora JM, et al. PYPAF7, a novel PYRIN-containing Apaf1-like protein that regulates activation of NF-kappa b and caspase-1-dependent cytokine processing. J Biol Chem (2002) 277:29874–80. doi: 10.1074/jbc.M203915200
41. Jo E-K, Kim JK, Shin D-M, Sasakawa C. Molecular mechanisms regulating NLRP3 inflammasome activation. Cell Mol Immunol (2016) 13:148–59. doi: 10.1038/cmi.2015.95
42. Elliott EI, Sutterwala FS. Monocytes take their own path to IL-1β. Immunity (2016) 44:713–5. doi: 10.1016/j.immuni.2016.03.015
43. Zheng M, Kanneganti T-D. The regulation of the ZBP1-NLRP3 inflammasome and its implications in pyroptosis, apoptosis, and necroptosis (PANoptosis). Immunol Rev (2020) 297:26–38. doi: 10.1111/imr.12909
44. Zhao Y, Shi J, Shao F. Inflammatory caspases: Activation and cleavage of gasdermin-d In vitro and during pyroptosis. Methods Mol Biol (2018) 1714:131–48. doi: 10.1007/978-1-4939-7519-8_9
45. An J, Kim SH, Hwang D, Lee KE, Kim MJ, Yang EG, et al. Caspase-4 disaggregates lipopolysaccharide micelles via LPS-CARD interaction. Sci Rep (2019) 9:826. doi: 10.1038/s41598-018-36811-4
46. Kayagaki N, Stowe IB, Lee BL, O’Rourke K, Anderson K, Warming S, et al. Caspase-11 cleaves gasdermin d for non-canonical inflammasome signalling. Nature (2015) 526:666–71. doi: 10.1038/nature15541
47. Yang J, Zhao Y, Shao F. Non-canonical activation of inflammatory caspases by cytosolic LPS in innate immunity. Curr Opin Immunol (2015) 32:78–83. doi: 10.1016/j.coi.2015.01.007
48. Linder A, Hornung V. Irgm2 and gate-16 put a break on caspase-11 activation. EMBO Rep (2020) 21:e51787. doi: 10.15252/embr.202051787
49. Chen S, Jin P, Chen H, Wu D, Li S, Zhang Y, et al. Dual function of a turbot inflammatory caspase in mediating both canonical and non-canonical inflammasome activation. Dev Comp Immunol (2021) 121:104078. doi: 10.1016/j.dci.2021.104078
50. Man SM, Karki R, Sasai M, Place DE, Kesavardhana S, Temirov J, et al. IRGB10 liberates bacterial ligands for sensing by the AIM2 and caspase-11-NLRP3 inflammasomes. Cell (2016) 167:382–396.e17. doi: 10.1016/j.cell.2016.09.012
51. Shen X, Wang H, Weng C, Jiang H, Chen J. Caspase 3/GSDME-dependent pyroptosis contributes to chemotherapy drug-induced nephrotoxicity. Cell Death Dis (2021) 12:186. doi: 10.1038/s41419-021-03458-5
52. Rogers C, Fernandes-Alnemri T, Mayes L, Alnemri D, Cingolani G, Alnemri ES. Cleavage of DFNA5 by caspase-3 during apoptosis mediates progression to secondary necrotic/pyroptotic cell death. Nat Commun (2017) 8:14128. doi: 10.1038/ncomms14128
53. Rogers C, Erkes DA, Nardone A, Aplin AE, Fernandes-Alnemri T, Alnemri ES. Gasdermin pores permeabilize mitochondria to augment caspase-3 activation during apoptosis and inflammasome activation. Nat Commun (2019) 10:1689. doi: 10.1038/s41467-019-09397-2
54. Wang Y, Gao W, Shi X, Ding J, Liu W, He H, et al. Chemotherapy drugs induce pyroptosis through caspase-3 cleavage of a gasdermin. Nature (2017) 547:99–103. doi: 10.1038/nature22393
55. Fritsch M, Günther SD, Schwarzer R, Albert M-C, Schorn F, Werthenbach JP, et al. Caspase-8 is the molecular switch for apoptosis, necroptosis and pyroptosis. Nature (2019) 575:683–7. doi: 10.1038/s41586-019-1770-6
56. Schwarzer R, Laurien L, Pasparakis M. New insights into the regulation of apoptosis, necroptosis, and pyroptosis by receptor interacting protein kinase 1 and caspase-8. Curr Opin Cell Biol (2020) 63:186–93. doi: 10.1016/j.ceb.2020.02.004
57. Sarhan J, Liu BC, Muendlein HI, Li P, Nilson R, Tang AY, et al. Caspase-8 induces cleavage of gasdermin d to elicit pyroptosis during yersinia infection. Proc Natl Acad Sci USA (2018) 115:E10888–97. doi: 10.1073/pnas.1809548115
58. Orning P, Weng D, Starheim K, Ratner D, Best Z, Lee B, et al. Pathogen blockade of TAK1 triggers caspase-8-dependent cleavage of gasdermin d and cell death. Science (2018) 362:1064–9. doi: 10.1126/science.aau2818
59. Hou J, Zhao R, Xia W, Chang C-W, You Y, Hsu J-M, et al. PD-L1-mediated gasdermin c expression switches apoptosis to pyroptosis in cancer cells and facilitates tumour necrosis. Nat Cell Biol (2020) 22:1264–75. doi: 10.1038/s41556-020-0575-z
60. Mirza RE, Koh TJ. Contributions of cell subsets to cytokine production during normal and impaired wound healing. Cytokine (2015) 71:409–12. doi: 10.1016/j.cyto.2014.09.005
61. Thomay AA, Daley JM, Sabo E, Worth PJ, Shelton LJ, Harty MW, et al. Disruption of interleukin-1 signaling improves the quality of wound healing. Am J Pathol (2009) 174:2129–36. doi: 10.2353/ajpath.2009.080765
62. Feldmeyer L, Werner S, French LE, Beer H-D. Interleukin-1, inflammasomes and the skin. Eur J Cell Biol (2010) 89:638–44. doi: 10.1016/j.ejcb.2010.04.008
63. Faustin B, Reed JC. Sunburned skin activates inflammasomes. Trends Cell Biol (2008) 18:4–8. doi: 10.1016/j.tcb.2007.10.004
64. Eo H, Lim Y. Combined mulberry leaf and fruit extract improved early stage of cutaneous wound healing in high-fat diet-induced obese mice. J Med Food (2016) 19:161–9. doi: 10.1089/jmf.2015.3510
65. Bitto A, Altavilla D, Pizzino G, Irrera N, Pallio G, Colonna MR, et al. Inhibition of inflammasome activation improves the impaired pattern of healing in genetically diabetic mice. Br J Pharmacol (2014) 171:2300–7. doi: 10.1111/bph.12557
66. Artlett CM. Inflammasomes in wound healing and fibrosis. J Pathol (2013) 229:157–67. doi: 10.1002/path.4116
67. Chen F, Wei G, Xu J, Ma X, Wang Q. Naringin ameliorates the high glucose-induced rat mesangial cell inflammatory reaction by modulating the NLRP3 inflammasome. BMC Complement Altern Med (2018) 18:192. doi: 10.1186/s12906-018-2257-y
68. Weinheimer-Haus EM, Mirza RE, Koh TJ. Nod-like receptor protein-3 inflammasome plays an important role during early stages of wound healing. PloS One (2015) 10:e0119106. doi: 10.1371/journal.pone.0119106
69. Li Y, Tang Y, Shi S, Gao S, Wang Y, Xiao D, et al. Tetrahedral framework nucleic acids ameliorate insulin resistance in type 2 diabetes mellitus via the PI3K/Akt pathway. ACS Appl Mater Interfaces (2021) 13:40354–64. doi: 10.1021/acsami.1c11468
70. Chen Y, Shi S, Li B, Lan T, Yuan K, Yuan J, et al. Therapeutic effects of self-assembled tetrahedral framework nucleic acids on liver regeneration in acute liver failure. ACS Appl Mater Interfaces (2022) 14:13136–46. doi: 10.1021/acsami.2c02523
71. Liu N, Zhang X, Li N, Zhou M, Zhang T, Li S, et al. Tetrahedral framework nucleic acids promote corneal epithelial wound healing in vitro and in vivo. Small (2019) 15:e1901907. doi: 10.1002/smll.201901907
72. Zhu J, Zhang M, Gao Y, Qin X, Zhang T, Cui W, et al. Tetrahedral framework nucleic acids promote scarless healing of cutaneous wounds via the AKT-signaling pathway. Signal Transduct Target Ther (2020) 5:120. doi: 10.1038/s41392-020-0173-3
73. Lin S, Zhang Q, Li S, Zhang T, Wang L, Qin X, et al. Antioxidative and angiogenesis-promoting effects of tetrahedral framework nucleic acids in diabetic wound healing with activation of the Akt/Nrf2/HO-1 pathway. ACS Appl Mater Interfaces (2020) 12:11397–408. doi: 10.1021/acsami.0c00874
74. Jiang Y, Li S, Zhang T, Zhang M, Chen Y, Wu Y, et al. Tetrahedral framework nucleic acids inhibit skin fibrosis via the pyroptosis pathway. ACS Appl Mater Interfaces (2022) 14(13):15069–79. doi: 10.1021/acsami.2c02877
75. Zhou Y, Gao L, Peng J, Xing M, Han Y, Wang X, et al. Bioglass activated albumin hydrogels for wound healing. Adv Healthc Mater (2018) 7:e1800144. doi: 10.1002/adhm.201800144
76. Dai W, Wang X, Teng H, Li C, Wang B, Wang J. Celastrol inhibits microglial pyroptosis and attenuates inflammatory reaction in acute spinal cord injury rats. Int Immunopharmacol (2019) 66:215–23. doi: 10.1016/j.intimp.2018.11.029
77. Ma J-W, Ji D-D, Li Q-Q, Zhang T, Luo L. Inhibition of connexin 43 attenuates oxidative stress and apoptosis in human umbilical vein endothelial cells. BMC Pulm Med (2020) 20:19. doi: 10.1186/s12890-019-1036-y
78. Zhang K, Chai B, Ji H, Chen L, Ma Y, Zhu L, et al. Bioglass promotes wound healing by inhibiting endothelial cell pyroptosis through regulation of the connexin 43/reactive oxygen species (ROS) signaling pathway. Lab Invest (2022) 102:90–101. doi: 10.1038/s41374-021-00675-6
79. Aitcheson SM, Frentiu FD, Hurn SE, Edwards K, Murray RZ. Skin wound healing: Normal macrophage function and macrophage dysfunction in diabetic wounds. Molecules (2021) 26:4917. doi: 10.3390/molecules26164917
80. Louiselle AE, Niemiec SM, Zgheib C, Liechty KW. Macrophage polarization and diabetic wound healing. Transl Res (2021) 236:109–16. doi: 10.1016/j.trsl.2021.05.006
81. Geng K, Ma X, Jiang Z, Huang W, Gao C, Pu Y, et al. Innate immunity in diabetic wound healing: Focus on the mastermind hidden in chronic inflammatory. Front Pharmacol (2021) 12:653940. doi: 10.3389/fphar.2021.653940
82. Hu Q, Shi H, Zeng T, Liu H, Su Y, Cheng X, et al. Increased neutrophil extracellular traps activate NLRP3 and inflammatory macrophages in adult-onset still’s disease. Arthritis Res Ther (2019) 21:9. doi: 10.1186/s13075-018-1800-z
83. Lee MKS, Sreejit G, Nagareddy PR, Murphy AJ. Attack of the NETs! NETosis primes IL-1β-mediated inflammation in diabetic foot ulcers. Clin Sci (Lond) (2020) 134:1399–401. doi: 10.1042/CS20200240
84. He S, Li L, Chen H, Hu X, Wang W, Zhang H, et al. PRRSV infection induces gasdermin d-driven pyroptosis of porcine alveolar macrophages through NLRP3 inflammasome activation. J Virol (2022) 96(14):e0212721. doi: 10.1128/jvi.02127-21
85. Wong SL, Demers M, Martinod K, Gallant M, Wang Y, Goldfine AB, et al. Diabetes primes neutrophils to undergo NETosis, which impairs wound healing. Nat Med (2015) 21:815–9. doi: 10.1038/nm.3887
86. Liu D, Yang P, Gao M, Yu T, Shi Y, Zhang M, et al. NLRP3 activation induced by neutrophil extracellular traps sustains inflammatory response in the diabetic wound. Clin Sci (2019) 133:565–82. doi: 10.1042/CS20180600
87. Wan L, Bai X, Zhou Q, Chen C, Wang H, Liu T, et al. The advanced glycation end-products (AGEs)/ROS/NLRP3 inflammasome axis contributes to delayed diabetic corneal wound healing and nerve regeneration. Int J Biol Sci (2022) 18:809–25. doi: 10.7150/ijbs.63219
88. Gu C, Draga D, Zhou C, Su T, Zou C, Gu Q, et al. miR-590-3p inhibits pyroptosis in diabetic retinopathy by targeting NLRP1 and inactivating the NOX4 signaling pathway. Invest Ophthalmol Vis Sci (2019) 60:4215–23. doi: 10.1167/iovs.19-27825
89. Liu X, Liu H, Lu X, Zhao S. N-acetylcysteine alleviates ocular surface damage in STZ-induced diabetic mice by inhibiting the ROS/NLRP3/Caspase-1/IL-1β signaling pathway. Exp Eye Res (2021) 209:108654. doi: 10.1016/j.exer.2021.108654
90. Liu H, Xu R, Kong Q, Liu J, Yu Z, Zhao C. Downregulated NLRP3 and NLRP1 inflammasomes signaling pathways in the development and progression of type 1 diabetes mellitus. BioMed Pharmacother (2017) 94:619–26. doi: 10.1016/j.biopha.2017.07.102
91. Yang K, Liu J, Zhang X, Ren Z, Gao L, Wang Y, et al. H3 relaxin alleviates migration, apoptosis and pyroptosis through P2X7R-mediated nucleotide binding oligomerization domain-like receptor protein 3 inflammasome activation in retinopathy induced by hyperglycemia. Front Pharmacol (2020) 11:603689. doi: 10.3389/fphar.2020.603689
92. Lamkanfi M, Mueller JL, Vitari AC, Misaghi S, Fedorova A, Deshayes K, et al. Glyburide inhibits the Cryopyrin/Nalp3 inflammasome. J Cell Biol (2009) 187:61–70. doi: 10.1083/jcb.200903124
93. Mi J, Xie C, Zeng L, Zhu Z, Chen N, He Q, et al. Bacillus subtilis WB800N alleviates diabetic wounds in mice by regulating gut microbiota homeostasis and TLR2. J Appl Microbiol (2022). doi: 10.1111/jam.15547
94. Pastar I, Sawaya AP, Marjanovic J, Burgess JL, Strbo N, Rivas KE, et al. Intracellular Staphylococcus aureus triggers pyroptosis and contributes to inhibition of healing due to perforin-2 suppression. J Clin Invest (2021) 131:e133727. doi: 10.1172/JCI133727
95. Zhao Y, Wang Q, Yan S, Zhou J, Huang L, Zhu H, et al. Bletilla striata polysaccharide promotes diabetic wound healing through inhibition of the NLRP3 inflammasome. Front Pharmacol (2021) 12:659215. doi: 10.3389/fphar.2021.659215
96. Sun X, Wang X, Zhao Z, Chen J, Li C, Zhao G. Paeoniflorin inhibited nod-like receptor protein-3 inflammasome and NF-kappa b-mediated inflammatory reactions in diabetic foot ulcer by inhibiting the chemokine receptor CXCR2. Drug Dev Res (2021) 82:404–11. doi: 10.1002/ddr.21763
97. Wang T, Zhao J, Zhang J, Mei J, Shao M, Pan Y, et al. Heparan sulfate inhibits inflammation and improves wound healing by downregulating the NLR family pyrin domain containing 3 (NLRP3) inflammasome in diabetic rats. J Diabetes (2018) 10:556–63. doi: 10.1111/1753-0407.12630
98. Mirza RE, Fang MM, Weinheimer-Haus EM, Ennis WJ, Koh TJ. Sustained inflammasome activity in macrophages impairs wound healing in type 2 diabetic humans and mice. Diabetes (2014) 63:1103–14. doi: 10.2337/db13-0927
99. Sutterwala FS, Ogura Y, Szczepanik M, Lara-Tejero M, Lichtenberger GS, Grant EP, et al. Critical role for NALP3/CIAS1/Cryopyrin in innate and adaptive immunity through its regulation of caspase-1. Immunity (2006) 24. doi: 10.1016/j.immuni.2006.02.004
100. Tschopp J, Schroder K. NLRP3 inflammasome activation: The convergence of multiple signalling pathways on ROS production? Nat Rev Immunol (2010) 10:210–5. doi: 10.1038/nri2725
101. Earl AM, Losick R, Kolter R. Ecology and genomics of bacillus subtilis. Trends Microbiol (2008) 16:269–75. doi: 10.1016/j.tim.2008.03.004
102. Yang Y, Jing Y, Yang J, Yang Q. Effects of intranasal administration with bacillus subtilis on immune cells in the nasal mucosa and tonsils of piglets. Exp Ther Med (2018) 15:5189–98. doi: 10.3892/etm.2018.6093
103. Huon J-F, Montassier E, Leroy A-G, Grégoire M, Vibet M-A, Caillon J, et al. Phages versus antibiotics to treat infected diabetic wounds in a mouse model: a microbiological and microbiotic evaluation. mSystems (2020) 5:e00542–20. doi: 10.1128/mSystems.00542-20
104. Dasu MR, Thangappan RK, Bourgette A, DiPietro LA, Isseroff R, Jialal I. TLR2 expression and signaling-dependent inflammation impair wound healing in diabetic mice. Lab Invest (2010) 90:1628–36. doi: 10.1038/labinvest.2010.158
105. Ramirez HA, Pastar I, Jozic I, Stojadinovic O, Stone RC, Ojeh N, et al. Staphylococcus aureus triggers induction of miR-15B-5P to diminish DNA repair and deregulate inflammatory response in diabetic foot ulcers. J Invest Dermatol (2018) 138:1187–96. doi: 10.1016/j.jid.2017.11.038
106. Yuan L, Sun Y, Xu M, Zeng F, Xiong X. miR-203 acts as an inhibitor for epithelial-mesenchymal transition process in diabetic foot ulcers via targeting interleukin-8. Neuroimmunomodulation (2019) 26:239–49. doi: 10.1159/000503087
107. Miller LS, Pietras EM, Uricchio LH, Hirano K, Rao S, Lin H, et al. Inflammasome-mediated production of IL-1beta is required for neutrophil recruitment against Staphylococcus aureus in vivo. J Immunol (2007) 179:6933–42. doi: 10.4049/jimmunol.179.10.6933
108. Chen S, Li R, Cheng C, Xu J-Y, Jin C, Gao F, et al. Pseudomonas aeruginosa infection alters the macrophage phenotype switching process during wound healing in diabetic mice. Cell Biol Int (2018) 42:877–89. doi: 10.1002/cbin.10955
109. Jiang S, Zhang H, Li X, Yi B, Huang L, Hu Z, et al. Vitamin D/VDR attenuate cisplatin-induced AKI by down-regulating NLRP3/Caspase-1/GSDMD pyroptosis pathway. J Steroid Biochem Mol Biol (2021) 206:105789. doi: 10.1016/j.jsbmb.2020.105789
110. Zhang C, He Y, Chen Z, Shi J, Qu Y, Zhang J. Effect of polysaccharides from bletilla striata on the healing of dermal wounds in mice. Evid Based Complement Alternat Med (2019) 2019:9212314. doi: 10.1155/2019/9212314
111. He X, Wang X, Fang J, Zhao Z, Huang L, Guo H, et al. Bletilla striata: Medicinal uses, phytochemistry and pharmacological activities. J Ethnopharmacol (2017) 195:20–38. doi: 10.1016/j.jep.2016.11.026
112. Luo Y, Diao H, Xia S, Dong L, Chen J, Zhang J. A physiologically active polysaccharide hydrogel promotes wound healing. J BioMed Mater Res A (2010) 94:193–204. doi: 10.1002/jbm.a.32711
113. Ding L, Shan X, Zhao X, Zha H, Chen X, Wang J, et al. Spongy bilayer dressing composed of chitosan-Ag nanoparticles and chitosan-bletilla striata polysaccharide for wound healing applications. Carbohydr Polym (2017) 157:1538–47. doi: 10.1016/j.carbpol.2016.11.040
114. Zheng T, Wang Q, Dong Y, Ma W, Zhang Y, Zhao Y, et al. High glucose-aggravated hepatic insulin resistance: Role of the NLRP3 inflammasome in kupffer cells. Obes (Silver Spring) (2020) 28:1270–82. doi: 10.1002/oby.22821
115. Li Y, Xu S, Mihaylova MM, Zheng B, Hou X, Jiang B, et al. AMPK phosphorylates and inhibits SREBP activity to attenuate hepatic steatosis and atherosclerosis in diet-induced insulin-resistant mice. Cell Metab (2011) 13:376–88. doi: 10.1016/j.cmet.2011.03.009
116. Zheng T, Yang X, Li W, Wang Q, Chen L, Wu D, et al. Salidroside attenuates high-fat diet-induced nonalcoholic fatty liver disease via AMPK-dependent TXNIP/NLRP3 pathway. Oxid Med Cell Longev (2018) 2018:8597897. doi: 10.1155/2018/8597897
117. Dinh T, Tecilazich F, Kafanas A, Doupis J, Gnardellis C, Leal E, et al. Mechanisms involved in the development and healing of diabetic foot ulceration. Diabetes (2012) 61:2937–47. doi: 10.2337/db12-0227
118. Chen LC, Lee MH, Chou MH, Lin MF, Yang LL. Pharmacokinetic study of paeoniflorin in mice after oral administration of paeoniae radix extract. J Chromatogr B BioMed Sci Appl (1999) 735:33–40. doi: 10.1016/s0378-4347(99)00408-9
119. Shao Y-X, Xu X-X, Wang K, Qi X-M, Wu Y-G. Paeoniflorin attenuates incipient diabetic nephropathy in streptozotocin-induced mice by the suppression of the toll-like receptor-2 signaling pathway. Drug Des Devel Ther (2017) 11:3221–33. doi: 10.2147/DDDT.S149504
120. Kulprachakarn K, Ounjaijean S, Wungrath J, Mani R, Rerkasem K. Micronutrients and natural compounds status and their effects on wound healing in the diabetic foot ulcer. Int J Low Extrem Wounds (2017) 16:244–50. doi: 10.1177/1534734617737659
121. Mamun AA, Wu Y, Nasrin F, Akter A, Taniya MA, Munir F, et al. Role of pyroptosis in diabetes and its therapeutic implications. J Inflammation Res (2021) 14:2187–206. doi: 10.2147/JIR.S291453
122. Mirza RE, Fang MM, Ennis WJ, Koh TJ. Blocking interleukin-1β induces a healing-associated wound macrophage phenotype and improves healing in type 2 diabetes. Diabetes (2013) 62:2579–87. doi: 10.2337/db12-1450
123. Baggiolini M. Chemokines in pathology and medicine. J Intern Med (2001) 250:91–104. doi: 10.1046/j.1365-2796.2001.00867.x
124. Boro M, Balaji KN. CXCL1 and CXCL2 regulate NLRP3 inflammasome activation via G-Protein-Coupled receptor CXCR2. J Immunol (2017) 199:1660–71. doi: 10.4049/jimmunol.1700129
125. Chang Z, Meyer K, Rapraeger AC, Friedl A. Differential ability of heparan sulfate proteoglycans to assemble the fibroblast growth factor receptor complex in situ. FASEB J (2000) 14:137–44. doi: 10.1096/fasebj.14.1.137
126. Bosman FT, Stamenkovic I. Functional structure and composition of the extracellular matrix. J Pathol (2003) 200:423–8. doi: 10.1002/path.1437
127. Tong M, Tuk B, Shang P, Hekking IM, Fijneman EMG, Guijt M, et al. Diabetes-impaired wound healing is improved by matrix therapy with heparan sulfate glycosaminoglycan mimetic OTR4120 in rats. Diabetes (2012) 61:2633–41. doi: 10.2337/db11-1329
128. Eming SA, Krieg T, Davidson JM. Inflammation in wound repair: molecular and cellular mechanisms. J Invest Dermatol (2007) 127:514–25. doi: 10.1038/sj.jid.5700701
129. Tong M, Tuk B, Hekking IM, Pleumeekers MM, Boldewijn MB, Hovius SER, et al. Heparan sulfate glycosaminoglycan mimetic improves pressure ulcer healing in a rat model of cutaneous ischemia-reperfusion injury. Wound Repair Regener (2011) 19:505–14. doi: 10.1111/j.1524-475X.2011.00704.x
130. Luo B, Li B, Wang W, Liu X, Xia Y, Zhang C, et al. NLRP3 gene silencing ameliorates diabetic cardiomyopathy in a type 2 diabetes rat model. PloS One (2014) 9:e104771. doi: 10.1371/journal.pone.0104771
Keywords: pyroptosis, inflammasome, signaling pathways, NLRP3, diabetic wound
Citation: Mu X, Wu X, He W, Liu Y, Wu F and Nie X (2022) Pyroptosis and inflammasomes in diabetic wound healing. Front. Endocrinol. 13:950798. doi: 10.3389/fendo.2022.950798
Received: 23 May 2022; Accepted: 19 July 2022;
Published: 05 August 2022.
Edited by:
Jian Ma, Harbin Medical University, ChinaReviewed by:
Ziyue Li, Dana–Farber Cancer Institute, United StatesCopyright © 2022 Mu, Wu, He, Liu, Wu and Nie. This is an open-access article distributed under the terms of the Creative Commons Attribution License (CC BY). The use, distribution or reproduction in other forums is permitted, provided the original author(s) and the copyright owner(s) are credited and that the original publication in this journal is cited, in accordance with accepted academic practice. No use, distribution or reproduction is permitted which does not comply with these terms.
*Correspondence: Xuqiang Nie, bmlleHVxaWFuZ0AxMjYuY29t
†ORCID: Xuqiang Nie, orcid.org/0000-0002-6926-6515
Disclaimer: All claims expressed in this article are solely those of the authors and do not necessarily represent those of their affiliated organizations, or those of the publisher, the editors and the reviewers. Any product that may be evaluated in this article or claim that may be made by its manufacturer is not guaranteed or endorsed by the publisher.
Research integrity at Frontiers
Learn more about the work of our research integrity team to safeguard the quality of each article we publish.