- 1Department of Oral Physiology, School of Dentistry and Institute of Oral Bioscience, Jeonbuk National University, Jeonju, South Korea
- 2Department of Obstetrics and Gynecology, Jeonbuk National University Medical School, Institute of Clinical Medicine of Jeonbuk National University-Biomedical Research Institute for Medical Sciences, Jeonbuk National University Hospital, Jeonju, South Korea
It has been reported that reactive oxygen species (ROS) derived from oxygen molecule reduction can interfere with the cross-talk between the hypothalamic-pituitary-gonadal (HPG) axis and other endocrine axes, thus affecting fertility. Furthermore, ROS have been linked to GnRH receptor signaling in gonadotropes involved in gonadotropin release. There has been evidence that ROS can interfere with the HPG axis and gonadotropin release at various levels. However, the direct effect of ROS on gonadotropin-releasing hormone (GnRH) neuron remains unclear. Thus, the objective of this study was to determine the effect of hydrogen peroxide (H2O2), an ROS source, on GnRH neuronal excitabilities in transgenic GnRH-green fluorescent protein-tagged mice using the whole-cell patch-clamp electrophysiology. In adults, H2O2 at high concentrations (mM level) hyperpolarized most GnRH neurons tested, whereas low concentrations (pM to μM) caused slight depolarization. In immature GnRH neurons, H2O2 exposure induced excitation. The sensitivity of GnRH neurons to H2O2 was increased with postnatal development. The effect of H2O2 on adult female GnRH neurons was found to be estrous cycle-dependent. Hyperpolarization mediated by H2O2 persisted in the presence of tetrodotoxin, a voltage-gated Na+ channel blocker, and amino-acids receptor blocking cocktail containing blockers for the ionotropic glutamate receptors, glycine receptors, and GABAA receptors, indicating that H2O2 could act on GnRH neurons directly. Furthermore, glibenclamide, an ATP-sensitive K+ (KATP) channel blocker, completely blocked H2O2-mediated hyperpolarization. Increasing endogenous H2O2 by inhibiting glutathione peroxidase decreased spontaneous activities of most GnRH neurons. We conclude that ROS can act as signaling molecules for regulating GnRH neuron’s excitability and that adult GnRH neurons are sensitive to increased ROS concentration. Results of this study demonstrate that ROS have direct modulatory effects on the HPG axis at the hypothalamic level to regulate GnRH neuron’s excitabilities.
Introduction
Reactive oxygen species (ROS) are chemically reactive molecules or free radicals formed when oxygen molecules are reduced. Mitochondria are primary cellular organelles responsible for the production of a large amount of ROS in cells (1, 2). External sources including pollution, radiation, physical stress, alcohol abuse, cigarette smoking and vaping, drug abuse, obesity, malnutrition, lifestyle modification, and endocrine-disrupting chemicals can intensify ROS production in cells (3, 4). At the cellular level, ROS at low concentrations operate as signaling molecules (5). However, excessive levels of ROS cause oxidative stress and cell death (6). Numerous enzymatic and non-enzymatic antioxidant systems can counteract increasing concentration of ROS in cells. Enzymes such as glutathione peroxidase (GPx), superoxide dismutase, and catalase (CAT) play an enzymatic role in the degradation of ROS, while scavengers such as vitamin C, vitamin E, glutathione, carotenoids, and ubiquinone play a non-enzymatic role in the detoxification of free radicals (7, 8).
Gonadotropin-releasing hormone (GnRH) neurons are key regulators of the hypothalamic-pituitary-gonadal (HPG) axis. They play a pivotal role in the regulation of fertility via release of gonadotropins in mammals (9). It has been shown that ROS produced by endogenous and exogenous sources can impair reproductive function, decrease gonadal hormones, and interfere with cross-talk between the HPG axis and other endocrine axes, eventually affecting fertility (3). Furthermore, ROS are connected to GnRH receptor signaling involved in gonadotropin release of gonadotropes (10). In contrast, endogenous gonadal hormones strongly influence ROS generation in brain mitochondria (11). An external source of ROS has now emerged as a leading cause of reproductive issues such as infertility and pregnancy complications (3, 12, 13).
ROS in the brain can act as potent signaling molecules at physiological concentration. Neurons can sense, convert, and transmit ROS into relevant intracellular signals and regulate peripheral tissue activities via the autonomous nervous system (14). New evidence has suggested that ROS play a signaling role in regulating hypothalamus activity. For example, ROS in the hypothalamus can regulate energy homeostasis (15) and maintain body fluid dynamics (16). ROS can also affect functions of hypothalamic neurons such as neuropeptide-Y (NPY)/agouti-related protein (AgRP) neurons, pro-opiomelanocortin (POMC)/cocaine-and-amphetamine responsive transcript (CART) neurons, and paraventricular nucleus (PVN) (17, 18). Hormones, peptides, neurotransmitters, and nutrients can also affect the release of ROS in the hypothalamus (14).
Studies mentioned above have shown that ROS can inhibit gonadotropin release at several levels of the HPG axis. However, the mechanism underlying how ROS impact GnRH neuronal activities remains unknown. Among various ROS, hydrogen peroxide (H2O2) is the most stable and long-lived ROS as it has a cellular half-life of 1 ms compared to other ROS such as superoxide anion radicals (1 μs), and hydroxyl radicals (1 ns) (19–21). Furthermore, Ledo et al. reported that the extracellular H2O2 in brain slices and in vivo has a half-life of 2.5 and 2.2 s respectively (22). Additionally, H2O2 is a highly diffusible and less toxic ROS that has emerged as a neuromodulator and an intercellular signaling molecule in the brain (19, 22). H2O2 perfusion on brain slices can influence neuronal excitabilities (18, 23–25), synaptic activity, and neurotransmitter release (26, 27). Thus, the objective of this study was to investigate the effect of membrane diffusible extracellular ROS source H2O2 on excitabilities of GnRH neurons in hypothalamic preoptic area (hPOA) brain slices using a whole-cell patch-clamp approach.
Materials and methods
Animals
C57BL/6 GnRH-green fluorescent protein-tagged (GnRH-GFP) mice (28) housed under stable room temperature (23-26 °C) and an automatic 12:12-h light-dark cycle (lights on at 07:00 h) with ad libitum access to food and water were sacrificed for the experiment. All animal care conditions and experimental procedures were in accordance with the Institutional Animal Care and Use Committee of Jeonbuk National University (CBNU 2020-0122). Estrous cycle stage of female mice was assessed by vaginal smear examination.
Preparation of brain slices
Coronal brain slices were prepared using the same procedure as described in a previous study (29). In brief, mice were beheaded between 10:00 and 12:00 p.m. UTC+09:00 (Universal Time Coordinated). Their brains were swiftly removed and immersed in ice-cold artificial cerebrospinal fluid (ACSF) containing 126 mM NaCl, 2.5 mM KCl, 2.4 mM CaCl2, 1.2 mM MgCl2, 11 mM D-glucose, 1.4 mM NaH2PO4, and 25 mM NaHCO3 (pH value of 7.3 to 7.4 was maintained when bubbled with 95% O2 and 5% CO2). Coronal brain slices (180-270 μm thick) containing the preoptic hypothalamic area were prepared using a vibratome (VT1200S, Leica biosystem, Wetzlar, Germany) in ice-cold ACSF. For recovery, the brain slices were stored in oxygenated ACSF at room temperature for at least 1hour before being transferred to the recording chamber.
Electrophysiology
Before electrophysiological recording, brain slices were transferred to the recording chamber mounted on an upright microscope (BX51W1; Olympus, Tokyo, Japan). They were, entirely submerged, and continuously perfused (4~5 mL/min) with oxygenated ACSF. The view of the coronal slice was displayed on a video monitor. The hPOA region was identified under X10 objective magnification. Fluorescent GnRH neurons were identified under X40 objective magnification via brief fluorescence illumination. Identified GnRH neurons were patched under Nomarski differential interference contrast optics. Thin-wall borosilicate glass capillaries (PG52151-4, WPI, Sarasota, FL, USA) were pulled on a Flaming/Brown puller (P-97; Sutter Instruments Co., Novate, CA USA) to fabricate patch pipette. These pipettes typically displayed a tip resistance of 4 to 6 MΩ when filled with pipette solution filtered through a disposable 0.22-µM filter. The loaded pipette solution was composed of 140 mM KCl, 1mM CaCl2, 1 mM MgCl2, 10 mM HEPES, 10 mM EGTA, and 4 mM Mg-ATP (pH 7.3 with KOH). Pipette offset was set to zero before a high-resistance seal (“gigaseal”) was achieved. After a giga seal was achieved between the pipette and the neuronal membrane, negative pressure by a short suction pulse was applied to make the whole cell.
Whole-cell recorded signals were amplified with an Axopatch 200B (Molecular Devices, San Jose, CA, USA) and filtered at 1 kHz with a Bessel filter before digitizing at a rate of 1 kHz. Membrane potential changes were sampled using a Digidata 1440A interface (Molecular Devices, San Jose, CA, USA). Signals were recorded and analyzed using an Axon pClamp 10.6 data acquisition program (Molecular Devices, San Jose, CA, USA). Neurons that showed changes in membrane potential of more than 2 mV after being exposed to H2O2 were considered to have responded. All recordings were made at room temperature.
Chemicals
Chemicals including hydrogen peroxide (H2O2), picrotoxin, strychnine hydrochloride (strychnine), glibenclamide, tetraethylammonium chloride (TEA), barium chloride (BaCl2), mercaptosuccinic acid (MCS), 3-amino-1,2,4-triazole (ATZ), and ACSF compositions were purchased from Sigma-Aldrich (St. Louis, MO, USA), except for CNQX disodium salt (CNQX), DL-AP5 (AP5), and tetrodotoxin citrate (TTX) which was bought from Tocris Bioscience (Avonmouth, Bristol, UK). Stocks were diluted (usually 1,000-fold) in ACSF to desired final concentrations before bath application. H2O2 of desired concentration was freshly prepared from stock by dripping directly to ACSF immediately before bath application.
Data and statistical analysis
For statistical analysis, Student’s t-test and one-way ANOVA post-hoc Scheffe test were used to compare means of two and more than two experimental groups, respectively. All statistical analyses were performed using Origin 8 software (OriginLab Corp, Northampton, MA, USA). All numerical values are expressed as mean ± standard error of the mean. Results with p-value < 0.05 are considered to be statistically significant. Traces were plotted using Origin 8 software (OriginLab Corp, Northampton, MA, USA). Action potential firings were analyzed using a Mini-Analysis software (ver. 6.0.7; Synaptosoft Inc., Decatur, GA, USA).
Results
Hydrogen peroxide exposure induces variegated response in GnRH neurons
We used whole-cell current-clamp recordings to investigate the influence of H2O2 on membrane excitability in GnRH neurons and found that superfusion with 1 mM H2O2 elicited a variety of responses in adult GnRH neurons, including membrane hyperpolarization, depolarization, and no response as shown in Figure 1. Bath treatment with 1 mM H2O2 for 3 to 5 minutes produced responses in 70% of adult GnRH neurons, while 30% of adult GnRH neurons were unresponsive to H2O2 (Figure 1A). Among responding neurons, 10% generated an average membrane depolarization of 4.60 ± 0.65 mV (n = 15; Figure 1B) while 60% of neurons induced an average membrane hyperpolarization of -14.6 ± 0.81 mV (n = 82; Figure 1C). Depolarized neurons showed a minor increase in spontaneous action potential firing in addition to membrane potential change. In contrast, hyperpolarized neurons showed partial and/or full cessation of spontaneous action potential firing. These alterations were reversed after more than 15-20 minutes of H2O2 washout.
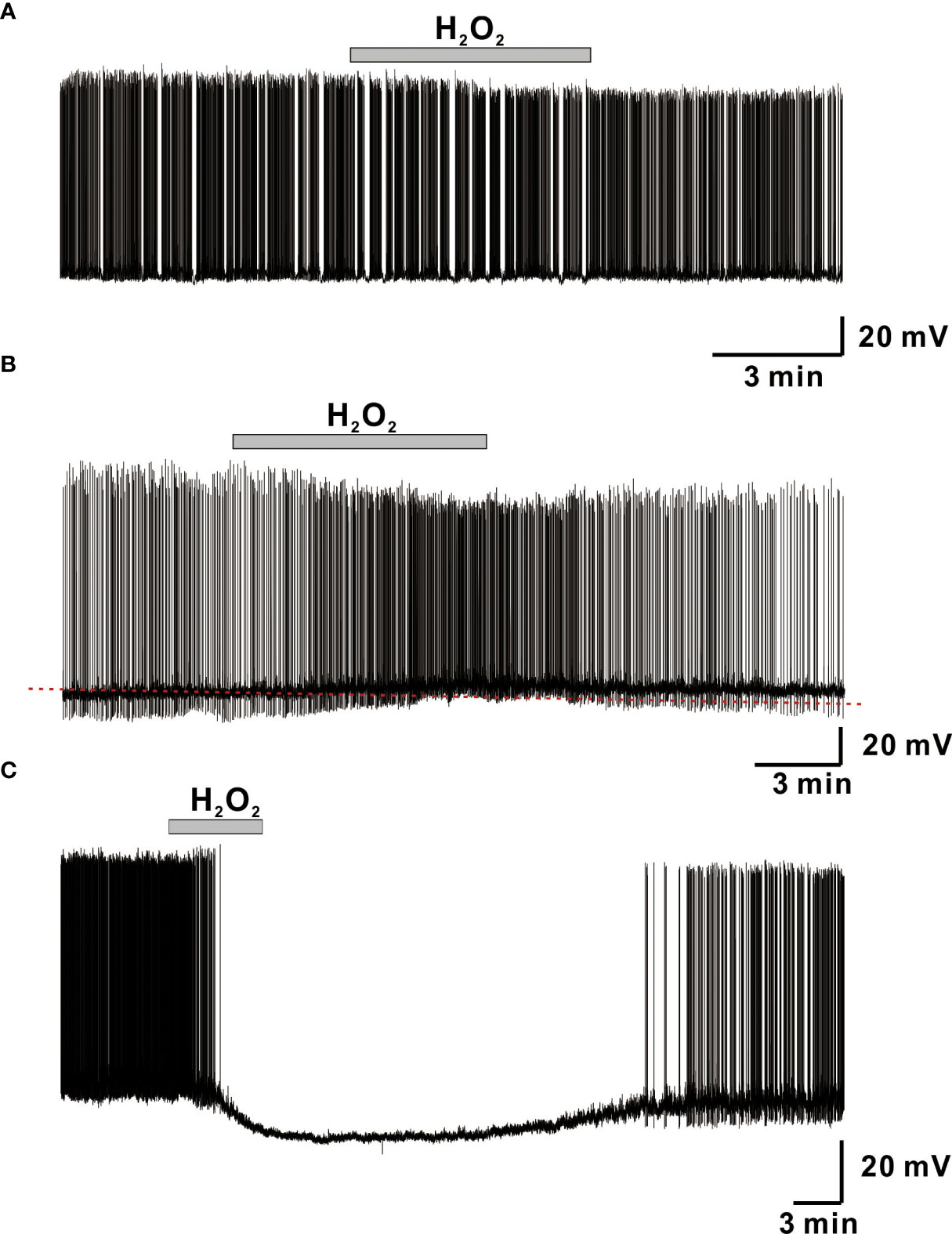
Figure 1 H2O2 induces variegated responses of adult male GnRH neurons. (A–C) Representative voltage traces from GnRH neurons showing no response, membrane depolarization, and membrane hyperpolarization, respectively, upon perfusion with 1 mM H2O2.
According to previous studies, oxidative stress vulnerability increases with age, with adults being more vulnerable and juveniles being partially resistant (30, 31). In the present study, effects of 1 mM H2O2 on GnRH neurons were studied in three groups according to age: juvenile, 8 to 25 postnatal days (PND); peripubertal, 26 to 45 PND; and adults, more than 60 PND. In contrast with its hyperpolarization effect on most adult GnRH neurons, H2O2 depolarized most GnRH neurons 67% (8/12) in juveniles. On the other hand, H2O2 exposure elicited similar percentages of responses, 46% (5/11) for depolarization and 36% (4/11) for hyperpolarization in peripubertal mice as shown in Figures 2A, B. Furthermore, there was no significant difference in mean depolarization between juvenile and peripubertal. Similarly, GnRH neurons from both adult females and males responded equally to H2O2 exposure (females; 69%, 24/35: males; 69%, 73/106). In addition, the mean values for induced hyperpolarization (male; -14.9 ± 0.84 mV, n = 65: female; -12.5 ± 1.50 mV, n = 17, p > 0.05; unpaired t-test) and depolarization (male; 3.98 ± 0.46 mV, n = 8: female; 5.32 ± 1.3 mV, n = 7, p > 0.05; unpaired t-test) were not significantly different between adult females and males GnRH neurons as shown in Figure 2A. Similarly, there was no significant difference in the mean hyperpolarization among estrous phases in female mice (estrous; -11.1 ± 2.11mV, n = 5: diestrous; -15.4 ± 0.96 mV, n = 5: proestrous; -11.4 ± 3.23 mV, n = 7, p > 0.05; one-way ANOVA, Figure 2C). However, female GnRH neurons demonstrated estrous cycle-dependent variation in response percentage to H2O2 exposure. During H2O2 exposure, 100% of GnRH neurons from proestrous mice showed hyperpolarization, whereas only 45% of GnRH neurons from estrous mice responded to H2O2 with hyperpolarization. Similarly, 70% of GnRH neurons from diestrous mice responded to H2O2 treatment, accounting 30% for hyperpolarization and 40% for depolarization, as shown in Figure 2D.
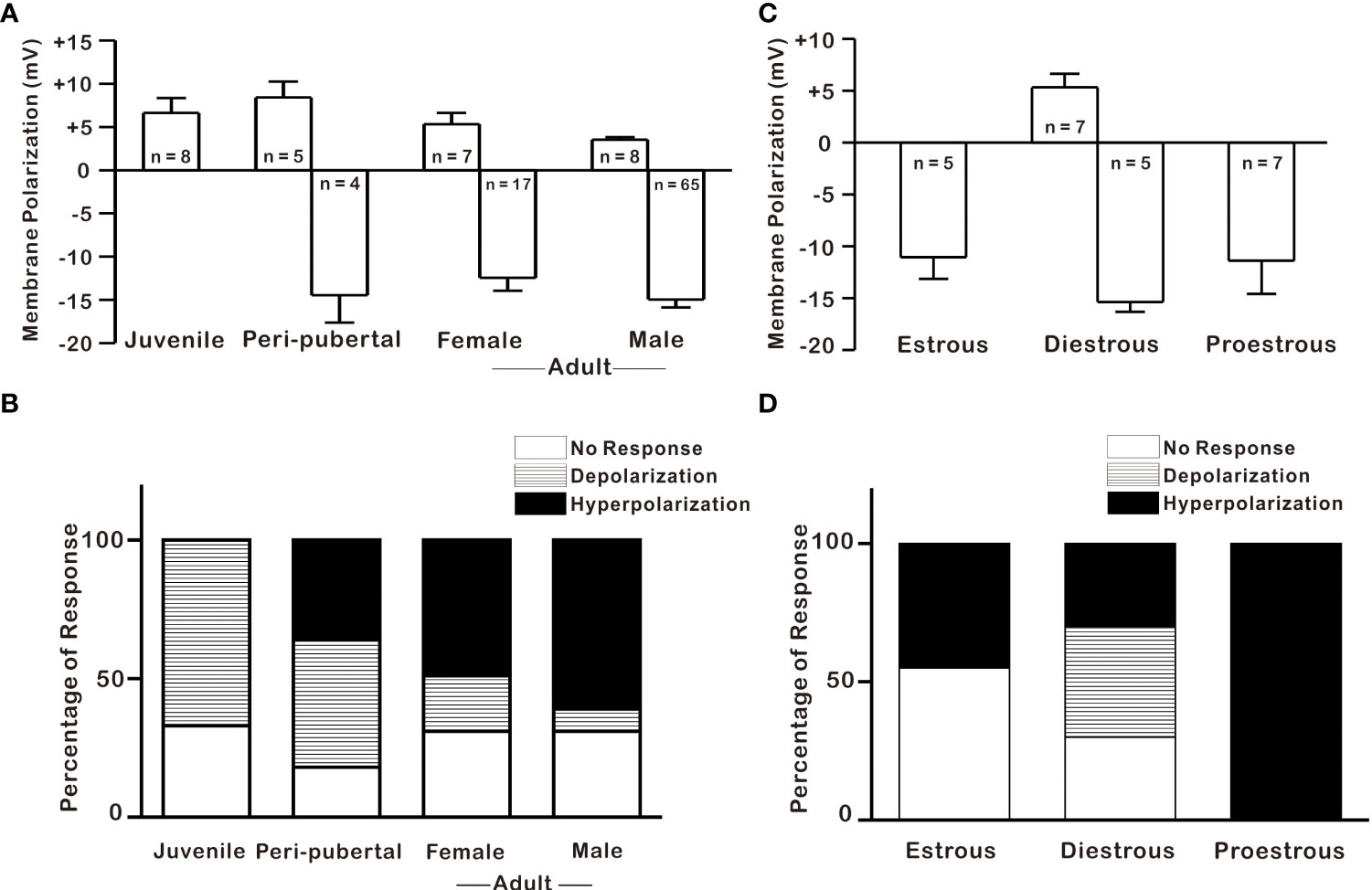
Figure 2 H2O2 effect on GnRH neurons across postnatal development and estrous cycle. (A–C) Histograms depicting H2O2-induced membrane polarization in GnRH neurons throughout the postnatal development and estrous cycle, respectively (p > 0.05; one-way ANOVA). (B–D) Histograms showing percentages of variegated responses of GnRH neurons by H2O2 exposure across postnatal development and at various estrous cycle stages in adult females, respectively.
Response of adult GnRH neurons to H2O2 exposure is concentration-dependent
After discovering that adult GnRH neurons were susceptible to 1 mM H2O2, we conducted a dose-dependent experiment in adult male GnRH neurons with low and high concentrations of H2O2. As demonstrated in Figure 3A, low concentrations of H2O2 caused minor membrane depolarization, whereas high concentrations of H2O2 caused membrane potential to become more hyperpolarized. Low concentrations of H2O2 (100 pm, 100 nM, and 10 μM) exhibited depolarization in the majority of GnRH neurons, corresponding to 80% (8/10), 43% (3/7), and 75% (3/4), respectively. In contrast, high concentrations of H2O2 (0.3, 1 and 3 mM) induced hyperpolarization in majority of GnRH neurons, corresponding to 69% (9/13), 61% (65/106), and 72% (13/18), respectively. However, 100 μM H2O2 induced depolarization in one of the fourteen neurons tested accounting 7% as shown in Figure 3B.
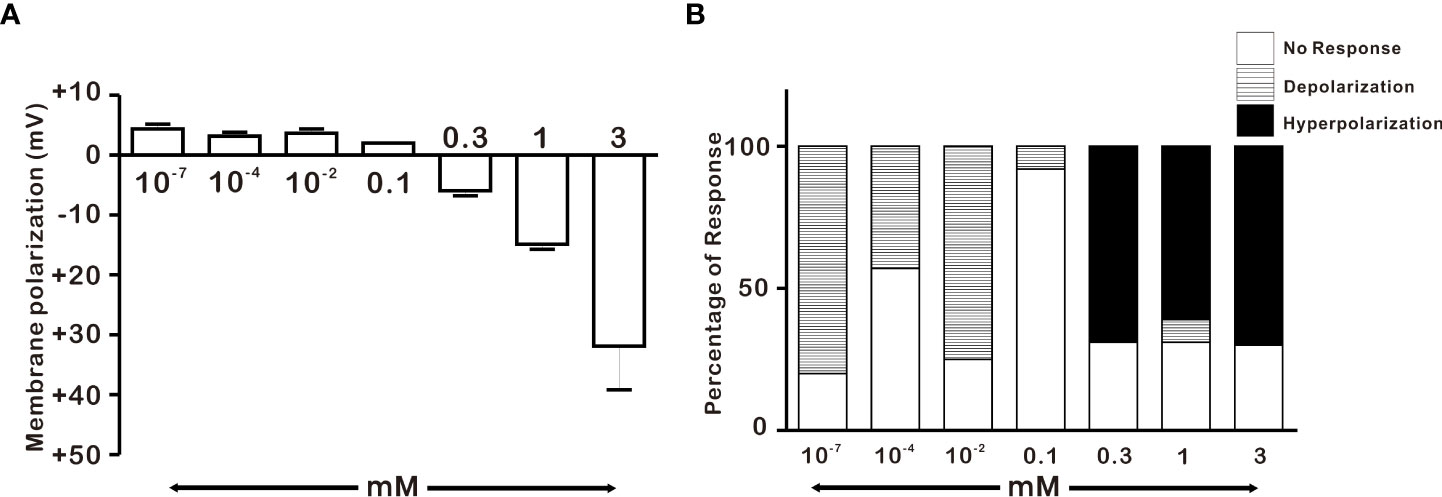
Figure 3 Concentration-dependent effect of H2O2 on GnRH membrane potential under whole-cell current clamp. (A) Histograms depicting H2O2-induced membrane polarization in response to various concentrations of H2O2 on GnRH neurons of adult males (one-way ANOVA post-hoc Scheffe test) (B) Histograms depicting percentage of variegated responses induced by various concentrations of H2O2 on GnRH neurons of adult males.
H2O2 acts on GnRH neurons postsynaptically
Hyperpolarization of GnRH neurons induced by 1 mM H2O2 recovered almost completely after more than 15 to 20 minutes of washout. Therefore, we determined whether H2O2 elicited repeatable responses of GnRH neurons. To access this, H2O2 was consecutively applied after the washout of the first application. On repeat application, H2O2 induced hyperpolarization with comparable amplitude to that of the first application. The mean hyperpolarization induced by H2O2 on the first application (-18.0 ± 4.84 mV, n = 8) was similar to that induced on the second application (-18.4 ± 4.8 mV, n = 8, p > 0.05; Figure 4A). Further, we aimed to examine whether H2O2 could act on GnRH neurons directly. For this, the hyperpolarization induced on bath application of H2O2 was recorded in the presence of TTX (0.5 µM), a sodium channel blocker known to block action potential-dependent transmission. Action potentials were promptly suppressed when recorded in the presence of TTX. However, the hyperpolarizing effect of H2O2 on GnRH neurons persisted. Average responses generated by H2O2 alone (-16.8 ± 2.2 mV, n = 8) and in the presence of TTX (-13.6 ± 1.7 mV, n = 8, p > 0.05; Figure 4B) were not significantly different.
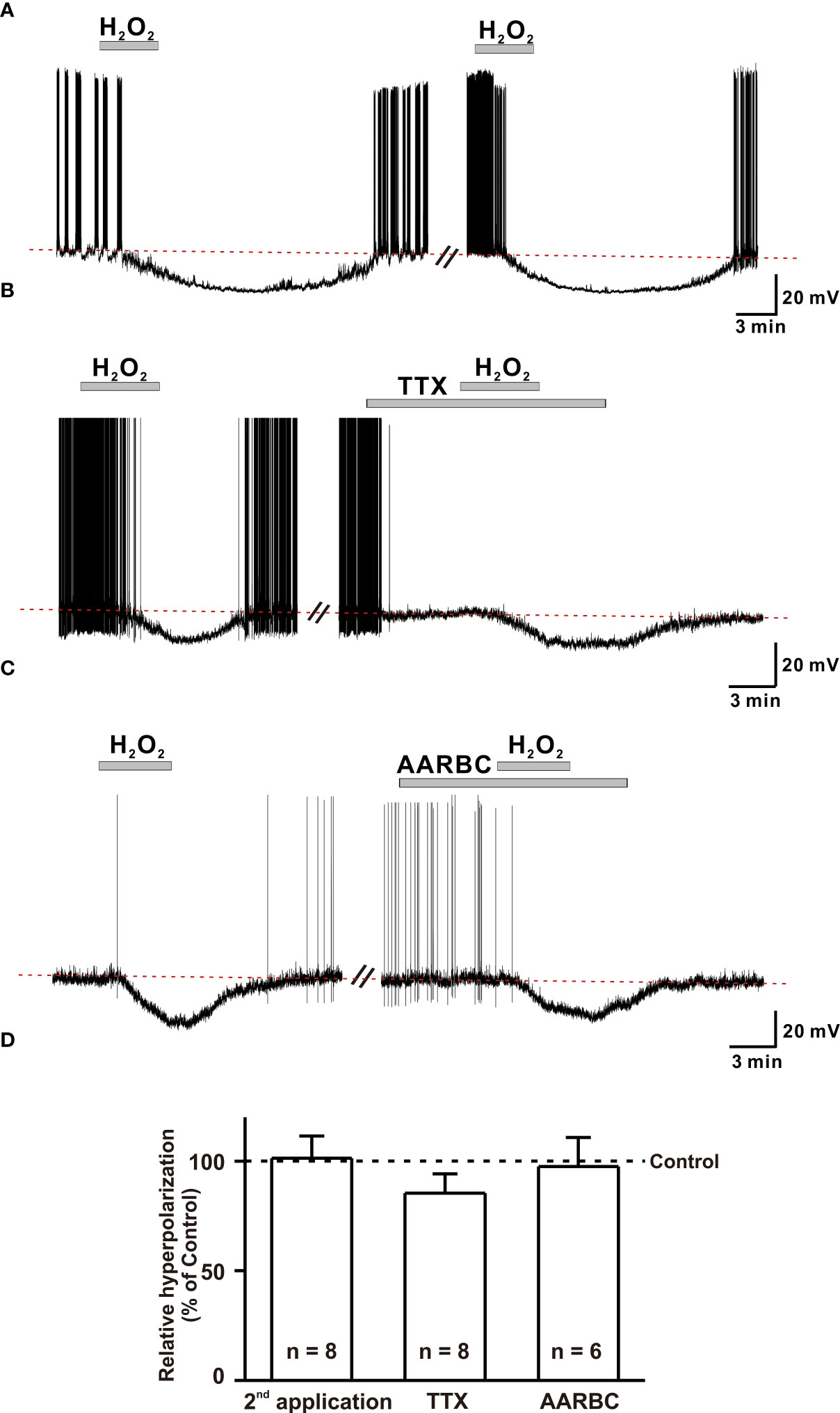
Figure 4 H2O2 acts on GnRH neurons post-synaptically. (A) A representative trace showing repeatable hyperpolarization induced by 1 mM H2O2 under a whole-cell current clamp. (B, C) Representative traces showing persistence of H2O2 induced hyperpolarization response in the presence of (TTX, 0.5 μM), the voltage-sensitive Na+ channel blocker and amino-acid receptor blocking cock-tail (AARBC), respectively. (D) A bar diagram showing mean relative values of hyperpolarization induced by 1 mM H2O2 on 2nd application, in the presence of TTX, and in the presence of AARBC (p > 0.05; paired t-test).
Next, to assess the possible involvement of both pre- and post-synaptic GABA, glycine, and glutamate receptors in H2O2 mediated actions of GnRH neurons, H2O2-induced hyperpolarization was recorded in the presence of an amino acid receptor blocker cocktail (AARBC) containing picrotoxin (50 µM), AP5 (20 µM), CNQX (10 µM), and strychnine (2 µM). Under these circumstances, H2O2 still induced hyperpolarization of GnRH neurons. The average hyperpolarization induced by H2O2 alone was -17.0 ± 1.95 mV (n = 6), which was not significantly different from that induced by H2O2 in the presence of AARBC (-16.5 ± 2.57 mV, n = 6, p > 0.05; Figure 4C). As shown in Figure 4D, the average relative percentage of H2O2-induced hyperpolarization on the second application, TTX and AARBC compared to respective control were 101.3 ± 10.1% (n = 8, p > 0.05), 85.3 ± 8.9% (n = 8, p > 0.05), and 97.5 ± 13.3% (n = 6, p > 0.05), respectively. These findings imply that H2O2 directly acts on postsynaptic GnRH neurons to induce hyperpolarization effect.
H2O2-mediated hyperpolarization is due to activation of KATP channels
When exposed to exogenous H2O2, hyperpolarization and reduced excitation are hypothesized to be caused by the activation of potassium channels in various neuronal cells (18, 23). As a result, we examined hyperpolarization caused by H2O2 exposure in the presence of potassium channel blockers such as TEA, BaCl2, and glibenclamide. Blocker concentrations utilized in this study have been shown to be able to inhibit potassium channels in brain slices (32–34). To confirm the involvement of potassium channels in the hyperpolarizing effect of H2O2, the response elicited by H2O2 was examined in the presence of non-specific K+ channel blocker, TEA. The hyperpolarizing impact of H2O2 was maintained even in the presence of TEA (Figure 5A).
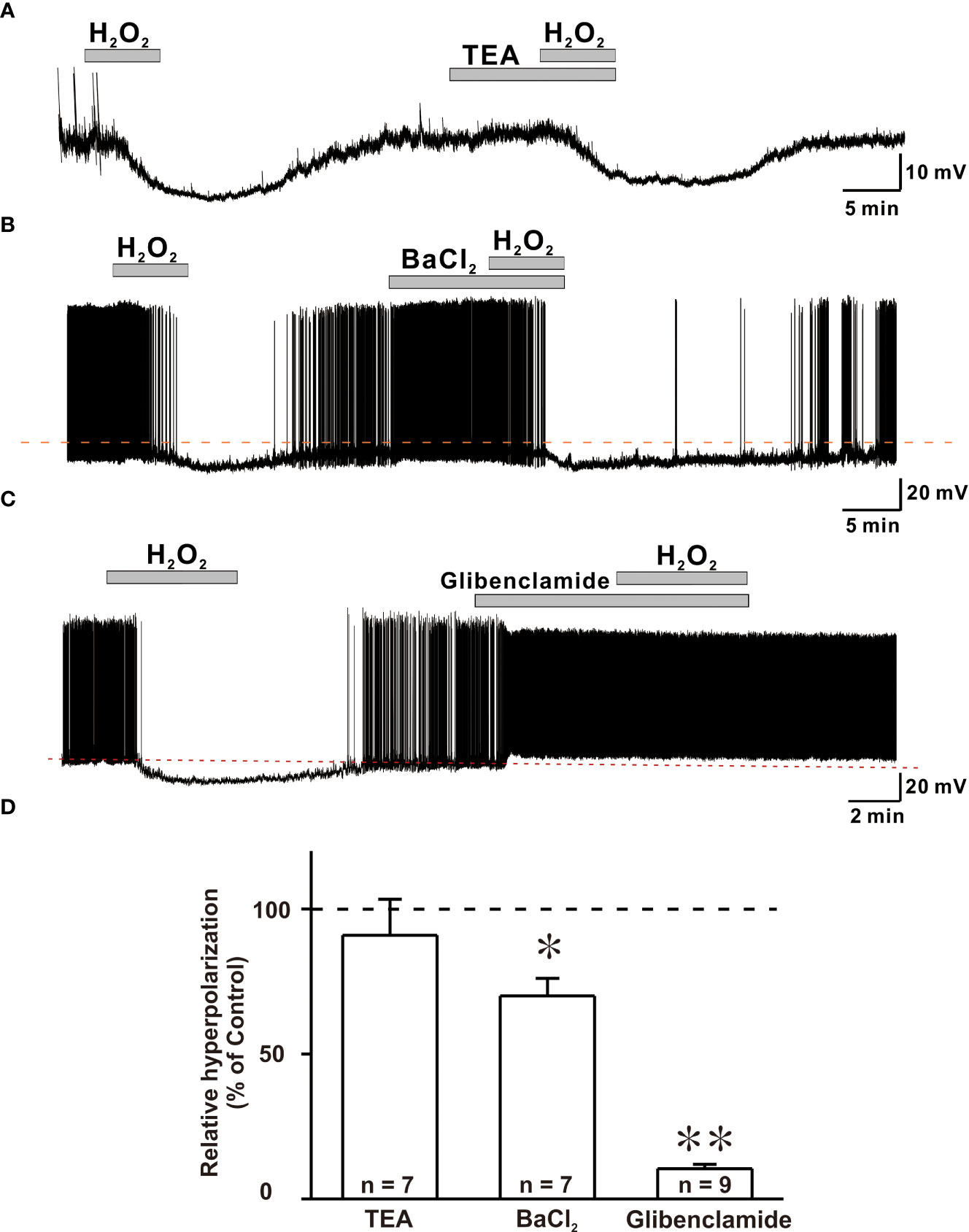
Figure 5 ATP-sensitive potassium channels (KATP) are susceptible to H2O2-induced hyperpolarization in GnRH neurons. (A, B) Representative traces showing persistence of H2O2-induced hyperpolarization response in the presence of TEA and BaCl2, respectively. (C) A representative trace showing complete blockade of hyperpolarization induced by 1mM H2O2 by KATP channel blocker glibenclamide under whole-cell current clamp. (D) A bar diagram depicting mean relative values of hyperpolarization caused by 1 mM H2O2 in the presence of various potassium channel blockers (TEA: n = 7, no significant; BaCl2: n = 7, *p < 0.05; glibenclamide: n = 9, **p < 0.01, paired t-test).
Next, hyperpolarization induced by H2O2 exposure was recorded in the presence of BaCl2, a broad-spectrum potassium channel blocker. In the presence of BaCl2, the hyperpolarization induced by H2O2 was partially suppressed (Figure 5B). Next, glibenclamide, KATP channel blocker, was coapplied with H2O2. After treatment with glibenclamide, five of nine GnRH neurons depolarized with increased firing frequency. Glibenclamide also prevented H2O2-elicited hyperpolarization of all neurons examined (Figure 5C). As shown in Figure 5D, average relative hyperpolarization percentages induced by H2O2 in the presence of TEA, BaCl2 and glibenclamide compared to those by H2O2 alone were 91.0 ± 12.4% (n = 7, p > 0.05), 70.0 ± 6.04% (n = 7, **p < 0.01), and 10.5 ± 1.52% (n = 9, ***p < 0.001), respectively. These findings imply a complete involvement of KATP channels in H2O2-mediated hyperpolarization of GnRH neurons.
Role of endogenous H2O2 in regulating excitability of GnRH neurons
In this study, exogenous H2O2 was identified as a possible regulator of GnRH neuron activity, influencing membrane potential and spontaneous firing activities. Next, we determined whether elevation in endogenously produced H2O2 could affect the activity of these cells. Recent studies have shown that endogenous H2O2 amplification can regulate neuronal excitability in distinct neuronal populations (23, 35).To explore the influence of endogenous H2O2 on GnRH neurons excitability, ATZ (1 mM), a CAT inhibitor, and MCS (1 mM), a GPx inhibitor, were bath applied. ATZ and MCS have been shown to increase the production of intracellular H2O2 in cells (23, 35). Using ATZ, we first examined the effect of CAT inhibition on GnRH neuronal activity. Except for one neuron that displayed depolarization of 19.7 mV, bath administration of 1mM ATZ had no significant effect on membrane potential or spontaneous activity of all GnRH neurons examined (Figure 6A). The frequency of spontaneous firing under ATZ treatment remained considerably unaltered compared to that of the control as shown in Figure 6B (Control: 1.68 ± 0.229, ATZ: 1.63 ± 0.22; n = 9; p > 0.05). Inhibiting GPx with MCS resulted in a partial cessation of spontaneous activity in most (13/17) GnRH neurons and a complete blockade in four neurons. In the presence of MCS, the spontaneous firing activity of GnRH neurons decreased from 1.90 ± 0.32 Hz to 0.80 ± 0.23 Hz (n = 17; p < 0.05; Figures 6C, D), with an average decrease of 66.2 ± 5.2%. In addition, MCS exposure resulted in membrane response in 9 of 17 GnRH neurons tested. Among them, seven neurons displayed a slight depolarization (3.75 ± 0.43 mV, n = 7), whereas the remaining two exhibited hyperpolarization of -3.70 ± 0.67 mV. All changes were reversible upon washout of MCS with ACSF.
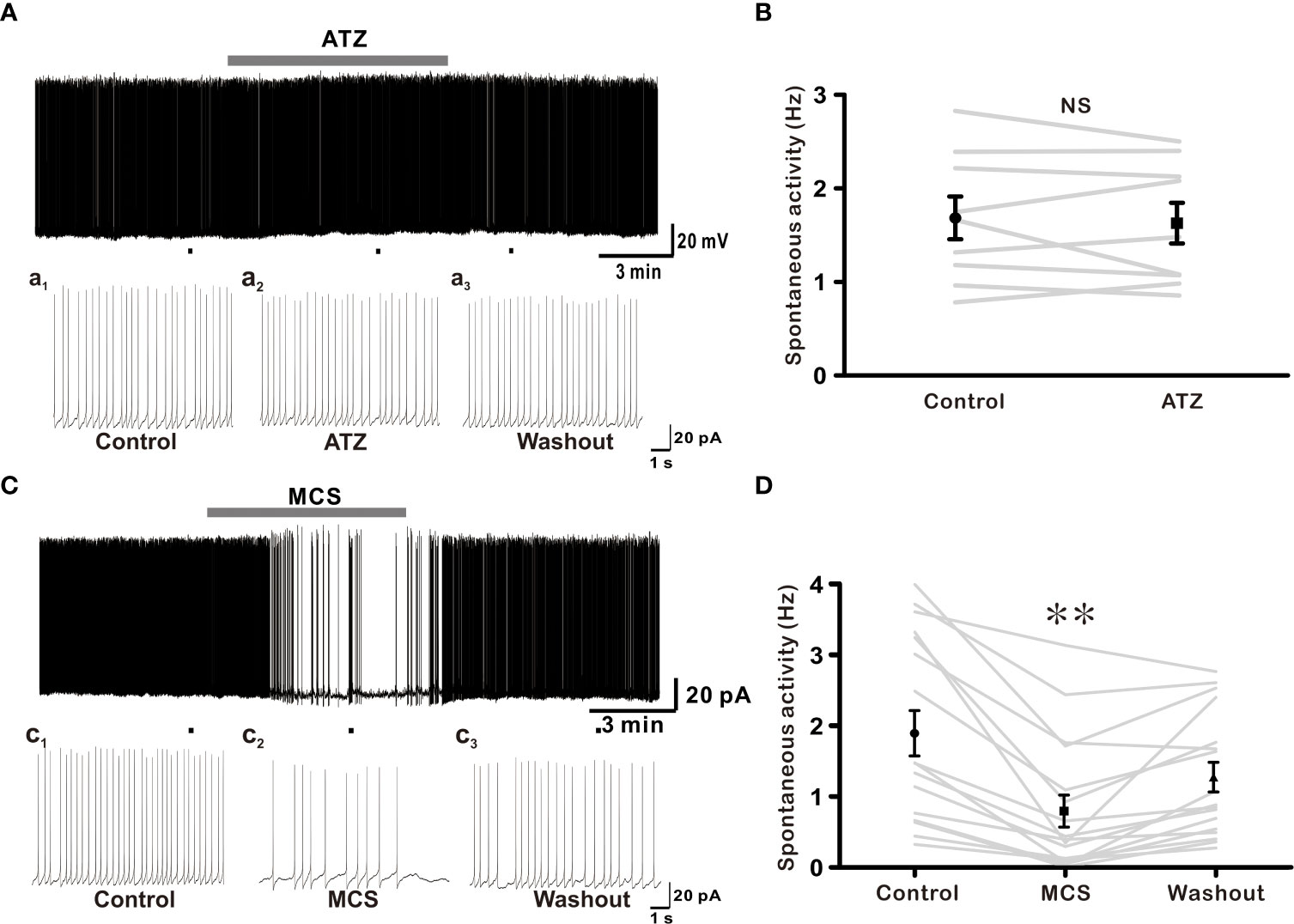
Figure 6 Glutathione peroxidase (GPx) inhibition suppresses excitability of GnRH neurons. (A) A representative current-clamp trace showing no effect of 1 mM ATZ (catalase inhibitor) on GnRH neurons. (C) A typical current-clamp trace showing a decrease of spontaneous activity of GnRH neurons after perfusion with 1 mM MCS, a GPx inhibitor. (B, D) Before and after plot showing effects of ATZ and MCS on mean spontaneous firing of GnRH neurons, respectively (**p < 0.01; one-way ANOVA).
Discussion
For the first time, this study shows that the majority of adult GnRH neurons are vulnerable to oxidative stress. This study aimed to determine the role of ROS H2O2 in modulating the GnRH neuronal activity. Our electrophysiological data demonstrated that exogenous H2O2 elicited post-synaptic inhibition of activities of most adult GnRH neurons via activation of KATP channels. Furthermore, immature GnRH neurons, unlike adult GnRH neurons, exhibited excitation upon H2O2 exposure. The vulnerability of GnRH neurons to H2O2 increased with postnatal development. H2O2 sensitivity to adult GnRH neurons was found to be highly dependent on H2O2 concentration and the estrous cycle of females. In addition, inhibiting GPx caused GnRH neurons to lose their spontaneous activity.
The hypothalamus is a predominant brain area that receives integrated information from multiple sources, including hormones, neurotransmitters, and metabolites, to regulate homeostasis, energy metabolism, and hormone release (14, 36). Furthermore, the hypothalamus is highly susceptible to oxidative stress. In addition, NADPH oxidase, a neuronal enzyme that produces ROS, is found in the hypothalamus, especially in the arcuate nucleus (ARC), ventromedial (VMN), and PVN regions (14, 17). The ARC, PVN, and VMN are known to contain neuromodulators that affect fertility (37). NPY/AgRP and POMC/CART neurons in the ARC project directly onto GnRH neuron cell bodies and nerve terminals (38, 39). Neuropeptides released by these neurons can influence GnRH neuron activity (40, 41). Furthermore, cellular activity of the NPY/AgRP and POMC/CART neuronal population is directly controlled by intracellular ROS (17). In the case of GnRH neurons, ROS H2O2 appeared to influence neuronal activity across postnatal development in a concentration-dependent and estrous-cycle-dependent manner.
Our findings, revealed that 1 mM H2O2 inhibited adult GnRH neurons, consistent with previous studies on dopamine neurons (23), PVN (18), substantia nigra pars reticulate (SNr) GABAergic neurons (35), and intrinsic cardiac ganglia neurons (42). Most studies using adult experimental animals have shown that H2O2 can inhibit neuronal excitability (18, 23, 35, 42). However, unlike adults, most immature GnRH neurons were stimulated by the same concentration of H2O2. According to previous studies, oxidative stress vulnerability increase with age, with young rats being more resistant to ROS than adults (30). Furthermore, H2O2 has both excitatory and inhibitory effects on neuronal excitability depending on neuronal population and brain location (43).
In the present study, the responsiveness of adult female GnRH neurons to H2O2 exposure varied throughout the estrous cycle. Circulating gonadal hormones, which fluctuate during estrous phases (44), can significantly impact GnRH neuronal excitability (45). Some studies show that proestrus mice had higher GnRH neuronal activity than mice in other estrous phases (46, 47). On the other hand, Piet et al. have reported less GnRH neuronal activity in proestrus mice than in mice at diestrus stage (48). According to previous studies, estradiol appears to have a positive feedback effect on GnRH neuronal activity in proestrus mice (49), and a neuroprotective effect against oxidative stress (50). We found that GnRH neurons in proestrus mice were more vulnerable to oxidative stress than those in estrous and diestrous stages. There is no information on how circulating steroid hormones influence GnRH neurons during oxidative stress. This requires further investigation.
In mature GnRH neurons, H2O2 mainly caused hyperpolarization and action potential suppression. Such H2O2-mediated response was retained in the presence of voltage-gated Na+ channel blocker TTX and AARBC, indicating a post-synaptic effect of H2O2 on GnRH neurons. H2O2 has been previously shown to have a similar post-synaptic effect (18). Studies have shown that H2O2 can induce membrane potential depolarization and hyperpolarization via different mechanisms. H2O2 can activate transient receptor potential channels (35, 51) or inhibit inward-rectifying K+ channels to induce depolarization (52). Opening of KATP channels leads to hyperpolarization (18, 23, 35). Activation of barium-sensitive potassium channels by H2O2 exposure has also been reported in a few studies (53). Similar to other studies, we observed the involvement of KATP and Ba2+ sensitive potassium channel in the hyperpolarization of GnRH neurons induced by H2O2.
The potassium channel plays a role in hormone and neurotransmitter release (54). Identifying signaling molecules that affect K+ channels in GnRH neurons is of particular interest nowadays. Studies have shown that GnRH neurons are susceptible to metabolic stress, which activates KATP channels. Functional KATP channel subunits have been detected in GnRH neurons (55). When the ATP/ADP ratio falls, KATP channels, which govern resting membrane properties of neurons, will open, causing cells to hyperpolarize and provide neuroprotection (56). Aside from neuroprotection, KATP channels are involved in glucose homeostasis in the hypothalamus, including GnRH neurons (55, 57). Recently, H2O2 has been identified as a signaling molecule for KATP channel activation (23, 35). Furthermore, inhibiting GPx and CAT of antioxidant systems can increase endogenous H2O2 in midbrain dopamine neurons (23) and SNr GABAergic neurons (35), resulting in KATP channel activation.
GPx and CAT are two major enzymes involved in H2O2 detoxification. Therefore, antioxidant enzymes inhibitors ATZ and MCS were used to determine the effect of endogenous H2O2 on GnRH neuronal excitability in the present study. ATZ is a CAT inhibitor that elevates endogenous H2O2 (58). It has a similar effect as exogenous H2O2 on midbrain dopamine neurons (23). However, ATZ showed no effect on GnRH neuron excitability. On the other hand, inhibition of GPx, another antioxidant enzyme, caused GnRH neurons to lose their spontaneous activity. Avshalumov et al. have reported a similar result. They showed that MCS treatment caused most dopamine neurons in the midbrain to hyperpolarize and lose their spontaneous activity (23). CAT and GPx are endogenous antioxidant-active enzymes responsible for the enzymatic clearance of H2O2, changing H2O2 into H2O and O2 molecules (18, 59). GPx is a crucial enzyme in the cytosol that plays an important role in the host’s defense against oxidative stress (60). Its principal antioxidant enzyme activity is to protect neurons against H2O2 toxicity (61). CAT is predominantly found in peroxisomes while GPx is distributed in the cytosol and mitochondria (61). Inhibiting GPx may cause H2O2 to accumulate in the cytosol, hence regulating neuronal excitability.
GnRH neurons not only can respond to hormonal, neurotransmitter, and neuropeptide inputs, but also can react directly to metabolic signals (55, 62, 63). The generation of reactive oxygen species is commonly linked to metabolic signals. In aging and pathologic situations, impairment in the antioxidant defense system becomes more noticeable, resulting in increased ROS generation (64, 65). The interaction between energy metabolism and ROS becomes more evident during aging, increasing the risk of age-related illnesses (66). Female reproductive disorders such as endometriosis, polycystic ovary syndrome, preeclampsia, and recurrent pregnancy loss can result from a pro-oxidant/antioxidant imbalance (12). Similarly, oxidative stress can affect sperm function in males, resulting in infertility (67). We demonstrated that H2O2 inhibited the majority of adult GnRH from both sex, which could reinforce the preexisting hypothesis about oxidative stress is linked to infertility. Furthermore, the direct impact of H2O2 on GnRH neuronal excitability via ion-channel mechanism could explain the cause of ROS disruption in the crosstalk of the HPG axis with another endocrine axis at hypothalamic levels and ROS-induced hormonal imbalance that leads to infertility.
In conclusion, current findings indicate that H2O2 can regulate KATP channels in adult GnRH neurons. Potassium channels can influence hormone and neurotransmitter release. Thus, oxidative stress regulating KATP channels in hypothalamic GnRH neurons could modulate pulsatile release of gonadotropins, impacting the reproductive axis.
Data availability statement
The raw data supporting the conclusions of this article will be made available by the authors, without undue reservation.
Ethics statement
The animal study was reviewed and approved by Institutional Animal Care and Use Committee of Jeonbuk National University (CBNU 2020-0122).
Author contributions
SR performed the experiments, analyzed the data, and wrote the draft. SJ contributed to reviewing and editing the draft. DC and SH conceptualized and design the study and completed the manuscript. All authors contributed to the article and approved the submitted version.
Funding
This research was supported by the National Research Foundation of Korea (NRF) grant funded by the Korean government (MSIT) (2021R1F1A1045406) and (2021R1F1A1046123). The funders had no role in the design, analysis, or writing of this article.
Conflict of interest
The authors declare that the research was conducted in the absence of any commercial or financial relationships that could be construed as a potential conflict of interest.
Publisher’s note
All claims expressed in this article are solely those of the authors and do not necessarily represent those of their affiliated organizations, or those of the publisher, the editors and the reviewers. Any product that may be evaluated in this article, or claim that may be made by its manufacturer, is not guaranteed or endorsed by the publisher.
References
1. Beckhauser TF, Francis-Oliveira J, De Pasquale R. Reactive oxygen species: physiological and physiopathological effects on synaptic plasticity: supplementary issue: brain plasticity and repair. J Exp Neurosci (2016) 10:23–48. doi: 10.4137/JEN.S39887
2. Park A, Lee HI, Semjid D, Kim DK, Chun SW. Dual effect of exogenous nitric oxide on neuronal excitability in rat substantia gelatinosa neurons. Neural Plast (2014) 2014:628531. doi: 10.1155/2014/628531
3. Darbandi M, Darbandi S, Agarwal A, Sengupta P, Durairajanayagam D, Henkel R, et al. Reactive oxygen species and male reproductive hormones. Reprod Biol Endocrinol (2018) 16(1):87. doi: 10.1186/s12958-018-0406-2
4. Phaniendra A, Jestadi DB, Periyasamy L. Free radicals: properties, sources, targets, and their implication in various diseases. Indian J Clin Biochem (2015) 30(1):11–26. doi: 10.1007/s12291-014-0446-0
5. Checa J, Aran JM. Reactive oxygen species: Drivers of physiological and pathological processes. J Inflammation Res (2020) 13:1057–73. doi: 10.2147/JIR.S275595
6. Chen Y, McMillan-Ward E, Kong J, Israels S, Gibson S. Oxidative stress induces autophagic cell death independent of apoptosis in transformed and cancer cells. Cell Death Differ (2008) 15(1):171–82. doi: 10.1038/sj.cdd.4402233
7. Kurutas EB. The importance of antioxidants which play the role in cellular response against oxidative/nitrosative stress: current state. Nutr J (2016) 15(1):71. doi: 10.1186/s12937-016-0186-5
8. Birben E, Sahiner UM, Sackesen C, Erzurum S, Kalayci O. Oxidative stress and antioxidant defense. World Allergy Organ J (2012) 5(1):9–19. doi: 10.1097/WOX.0b013e3182439613
9. Marques P, Skorupskaite K, George JT, Anderson RA. Physiology of GnRH and gonadotropin secretion (2000) MDText.com, Inc.Available at: http://www.ncbi.nlm.nih.gov/pubmed/25905297.
10. Terasaka T, Adakama ME, Li S, Kim T, Terasaka E, Li D. Et.al. reactive oxygen species link gonadotropin-releasing hormone receptor signaling cascades in the gonadotrope. Front Endocrinol (2017) 8:286. doi: 10.3389/fendo.2017.00286
11. Razmara A, Duckles SP, Krause DN, Procaccio V. Estrogen suppresses brain mitochondrial oxidative stress in female and male rats. Brain Res (2007) 1176:71–81. doi: 10.1016/j.brainres.2007.08.036
12. Agarwal A, Aponte-Mellado A, Premkumar BJ, Shaman A, Gupta S. The effects of oxidative stress on female reproduction: a review. Reprod Biol Endocrinol (2012) 10(1):1–31. doi: 10.1186/1477-7827-10-49
13. Barati E, Nikzad H, Karimian M. Oxidative stress and male infertility: Current knowledge of pathophysiology and role of antioxidant therapy in disease management. Cell Mol Life Sci (2020) 77(1):93–113. doi: 10.1007/s00018-019-03253-8
14. Drougard A, Fournel A, Valet P, Knauf C. Impact of hypothalamic reactive oxygen species in the regulation of energy metabolism and food intake. Front Neurosci (2015) 9:56. doi: 10.3389/fnins.2015.00056
15. Benani A, Troy S, Carmona MC, Fioramonti X, Lorsignol A, Leloup C, et al. Role for mitochondrial reactive oxygen species in brain lipid sensing: redox regulation of food intake. Diabetes (2007) 56(1):152–60. doi: 10.2337/db06-0440
16. St-Louis R, Parmentier C, Raison D, Grange-Messent V, Hardin-Pouzet H. Reactive oxygen species are required for the hypothalamic osmoregulatory response. Endocrinology (2012) 153(3):1317–29. doi: 10.1210/en.2011-1350
17. Gyengesi E, Paxinos G B, Andrews Z. Oxidative stress in the hypothalamus: the importance of calcium signaling and mitochondrial ROS in body weight regulation. Curr Neuropharmacol (2012) 10(4):344–53. doi: 10.2174/157015912804143496
18. Dantzler HA, Matott MP, Martinez D, Kline DD. Hydrogen peroxide inhibits neurons in the paraventricular nucleus of the hypothalamus via potassium channel activation. Am J Physiol Regul Integr Comp Physiol (2019) 317(1):R121–33. doi: 10.1152/ajpregu.00054.2019
19. Giniatullin A, Giniatullin R. Dual action of hydrogen peroxide on synaptic transmission at the frog neuromuscular junction. J Physiol (2003) 552(1):283–93. doi: 10.1113/jphysiol.2003.050690
20. Chen X, Song M, Zang B, Zang Y. Reactive oxygen species regulate T cell immune response in the tumor microenvironment. Oxid Med Cell Longev (2016) 2016:1580967. doi: 10.1155/2016/1580967
21. D'Autréaux B, Toledano MB. ROS as signalling molecules: mechanisms that generate specificity in ROS homeostasis. Nat Rev Mol Cell Biol (2007) 8(10):813–24. doi: 10.1038/nrm2256
22. Ledo A, Fernandes E, Salvador A, Laranjinha J, Barbosa R. In vivo hydrogen peroxide diffusivity in brain tissue supports volume signalling activity. Redox Biol (2022) 50:102250. doi: 10.1016/j.redox.2022.102250
23. Avshalumov MV, Chen BT, Koós T, Tepper JM, Rice ME. Endogenous hydrogen peroxide regulates the excitability of midbrain dopamine neurons via ATP-sensitive potassium channels. J Neurosci (2005) 25(17):4222–31. doi: 10.1523/JNEUROSCI.4701-04.2005
24. Ohashi M, Hirano T, Watanabe K, Shoji H, Ohashi N, Baba H, et al. Hydrogen peroxide modulates neuronal excitability and membrane properties in ventral horn neurons of the rat spinal cord. Neuroscience (2016) 331:206–20. doi: 10.1016/j.neuroscience.2016.06.033
25. Bal R, Ozturk G, Etem EO, Him A, Cengiz N, Kuloglu T, et al. Modulation of excitability of stellate neurons in the ventral cochlear nucleus of mice by ATP-sensitive potassium channels. J Membr Biol (2018) 251(1):163–78. doi: 10.1007/s00232-017-0011-x
26. Takahashi A, Mikami M, Yang J. Hydrogen peroxide increases GABAergic mIPSC through presynaptic release of calcium from IP3 receptor-sensitive stores in spinal cord substantia gelatinosa neurons. Eur J Neurosci (2007) 25(3):705–16. doi: 10.1111/j.1460-9568.2007.05323.x
27. Ohashi M, Hirano T, Watanabe K, Katsumi K, Ohashi N, Baba H, et al. Hydrogen peroxide modulates synaptic transmission in ventral horn neurons of the rat spinal cord. J Physiol (2016) 594(1):115–34. doi: 10.1113/JP271449
28. Spergel DJ, Krüth U, Hanley DF, Sprengel R, Seeburg PH. GABA-and glutamate-activated channels in green fluorescent protein-tagged gonadotropin-releasing hormone neurons in transgenic mice. J Neurosci (1999) 19(6):2037–50. doi: 10.1523/JNEUROSCI.19-06-02037.1999
29. Rijal S, Cho DH, Park SA, Jang SH, Ábrahám IM, Han SK. Melatonin suppresses the kainate receptor-mediated excitation on gonadotropin-releasing hormone neurons in female and Male prepubertal mice. Int J Mol Sci (2020) 21(17):5991. doi: 10.3390/ijms21175991
30. Carrascal L, Gorton E, Pardillo-Díaz R, Perez-García P, Gómez-Oliva R, Castro C, et al. Age-dependent vulnerability to oxidative stress of postnatal rat pyramidal motor cortex neurons. Antioxidants (2020) 9(12):1307. doi: 10.3390/antiox9121307
31. Pardillo-Díaz R, Carrascal L, Ayala A, Nunez-Abades P. Oxidative stress induced by cumene hydroperoxide evokes changes in neuronal excitability of rat motor cortex neurons. Neuroscience (2015) 289:85–98. doi: 10.1016/j.neuroscience.2014.12.055
32. D'Ambrosio R, Gordon DS, Winn HR. Differential role of KIR channel and Na+/K+-pump in the regulation of extracellular k+ in rat hippocampus. J Neurophysiol (2002) 87(1):87–102. doi: 10.1152/jn.00240.2001
33. Fujimura N, Tanaka E, Yamamoto S, Shigemori M, Higashi H. Contribution of ATP-sensitive potassium channels to hypoxic hyperpolarization in rat hippocampal CA1 neurons. vitro J Neurophysiol (1997) 77(1):378–85. doi: 10.1152/jn.1997.77.1.378
34. Bhattarai JP, Roa J, Herbison AE, Han SK. Serotonin acts through 5-HT1 and 5-HT2 receptors to exert biphasic actions on GnRH neuron excitability in the mouse. Endocrinology (2014) 155(2):513–24. doi: 10.1210/en.2013-1692
35. Lee CR, Witkovsky P, Rice ME. Regulation of substantia nigra pars reticulata GABAergic neuron activity by H2O2via flufenamic acid-sensitive channels and KATP channels. Front Syst Neurosci (2011) 5:14. doi: 10.3389/fnsys.2011.00014
36. Cai D, Liu T. Hypothalamic inflammation: a double-edged sword to nutritional diseases. Ann N Y Acad Sci (2011) 1243(1):E1–E39. doi: 10.1111/j.1749-6632.2011.06388.x
37. Navarro VM. New insights into the control of pulsatile GnRH release: the role of Kiss1/neurokinin b neurons. Front Endocrinol (2012) 3:48. doi: 10.3389/fendo.2012.00048
38. Campbell RE, Cowley MA, Smith MS, Grove KL. Hypothalamic circuitry of neuropeptide y regulation of neuroendocrine function and food intake via the Y5 receptor subtype. Neuroendocrinology (2001) 74(2):106–19. doi: 10.1159/000054676
39. Zheng SX, Bosch MA, Rønnekleiv OK. μ-opioid receptor mRNA expression in identified hypothalamic neurons. J Comp Neurol (2005) 487(3):332–44. doi: 10.1002/cne.20557
40. Klenke U, Constantin S, Wray S. Neuropeptide y directly inhibits neuronal activity in a subpopulation of gonadotropin-releasing hormone-1 neurons via Y1 receptors. Endocrinology (2010) 151(6):2736–46. doi: 10.1210/en.2009-1198
41. Roa J, Herbison AE. Direct regulation of GnRH neuron excitability by arcuate nucleus POMC and NPY neuron neuropeptides in female mice. Endocrinology (2012) 153(11):5587–99. doi: 10.1210/en.2012-1470
42. Whyte K, Hogg RC, Dyavanapalli J, Harper A, Adams DJ. Reactive oxygen species modulate neuronal excitability in rat intrinsic cardiac ganglia. Auton Neurosci (2009) 150(1-2):45–52. doi: 10.1016/j.autneu.2009.04.005
43. Lee CR, Patel JC, O'Neill B, Rice ME. Inhibitory and excitatory neuromodulation by hydrogen peroxide: translating energetics to information. J Physiol (2015) 593(16):3431–46. doi: 10.1113/jphysiol.2014.273839
44. Bell MR. Comparing postnatal development of gonadal hormones and associated social behaviors in rats, mice, and humans. Endocrinology (2018) 159(7):2596–613. doi: 10.1210/en.2018-00220
45. Radovick S, Levine JE, Wolfe A. Estrogenic regulation of the GnRH neuron. Front Endocrin (2012) 3:52. doi: 10.3389/fendo.2012.00052
46. Silveira MA, Burger LL, DeFazio RA, Wagenmaker ER, Moenter SM. GnRH neuron activity and pituitary response in estradiol-induced vs proestrous luteinizing hormone surges in female mice. Endocrinology (2017) 158(2):356–66. doi: 10.1210/en.2016-1771
47. Farkas I, Vastagh C, Sárvári M, Liposits Z. Ghrelin decreases firing activity of gonadotropin-releasing hormone (GnRH) neurons in an estrous cycle and endocannabinoid signaling dependent manner. PloS One (2013) 8(10):e78178. doi: 10.1371/journal.pone.0078178
48. Piet R, Dunckley H, Lee K, Herbison AE. Vasoactive intestinal peptide excites GnRH neurons in male and female mice. Endocrinology (2016) 157(9):3621–30. doi: 10.1210/en.2016-1399
49. Herbison AE. Estrogen positive feedback to gonadotropin-releasing hormone (GnRH) neurons in the rodent: the case for the rostral periventricular area of the third ventricle (RP3V). Brain Res Rev (2008) 57(2):277–87. doi: 10.1016/j.brainresrev.2007.05.006
50. Ozacmak VH, Sayan H. The effects of 17β estradiol, 17α estradiol and progesterone on oxidative stress biomarkers in ovariectomized female rat brain subjected to global cerebral ischemic. Physiol Res (2009) 58(6):909–12. doi: 10.33549/physiolres.931647
51. Bao L, Avshalumov MV, Rice ME. Partial mitochondrial inhibition causes striatal dopamine release suppression and medium spiny neuron depolarization via H2O2 elevation, not ATP depletion. J Neurosci (2005) 25(43):10029–40. doi: 10.1523/JNEUROSCI.2652-05.2005
52. Bychkov R, Pieper K, Ried C, Milosheva M, Bychkov E, Luft FC, et al. Hydrogen peroxide, potassium currents, and membrane potential in human endothelial cells. Circulation (1999) 99(13):1719–25. doi: 10.1161/01.cir.99.13.1719
53. Ostrowski TD, Hasser EM, Heesch CM, Kline DD. H2O2 induces delayed hyperexcitability in nucleus tractus solitarii neurons. Neuroscience (2014) 262:53–69. doi: 10.1016/j.neuroscience.2013.12.055
54. Yamada M, Inanobe A, Kurachi Y. G Protein regulation of potassium ion channels. Pharmacol Rev (1998) 50(4):723–57.
55. Zhang C, Bosch MA, Levine JE, Rønnekleiv OK, Kelly MJ. Gonadotropin-releasing hormone neurons express KATP channels that are regulated by estrogen and responsive to glucose and metabolic inhibition. Neurosci (2007) 27(38):10153–64. doi: 10.1523/JNEUROSCI.1657-07.2007
56. Sun HS, Feng ZP. Neuroprotective role of ATP-sensitive potassium channels in cerebral ischemia. Acta Pharmacol Sin (2013) 34(1):24–32. doi: 10.1038/aps.2012.138
57. Miki T, Liss B, Minami K, Shiuchi T, Saraya A, Kashima Y, et al. ATP-sensitive k+ channels in the hypothalamus are essential for the maintenance of glucose homeostasis. Nat Neurosci (2001) 4(5):507–12. doi: 10.1038/87455
58. Zhang M, Qin DN, Suo YP, Su Q, Li HB, Miao YW, et al. Endogenous hydrogen peroxide in the hypothalamic paraventricular nucleus regulates neurohormonal excitation in high salt-induced hypertension. Toxicol Lett (2015) 235(3):206–15. doi: 10.1016/j.toxlet.2015.04.008
59. Leloup C, Magnan C, Benani A, Bonnet E, Alquier T, Offer G, et al. Mitochondrial reactive oxygen species are required for hypothalamic glucose sensing. Diabetes (2006) 55(7):2084–90. doi: 10.2337/db06-0086
60. Dede FÖ. Periodontal health and disease in glutathione peroxidase. In: Glutathione system and oxidative stress in health and disease London, United Kingdom: IntechOpen (2020), p. 83–96.
61. Desagher S, Glowinski J, Premont J. Astrocytes protect neurons from hydrogen peroxide toxicity. J Neurosci (1996) 16(8):2553–62. doi: 10.1523/JNEUROSCI.16-08-02553.1996
62. Spergel DJ. Modulation of gonadotropin-releasing hormone neuron activity and secretion in mice by non-peptide neurotransmitters, gasotransmitters, and gliotransmitters. Front Endocrinol (2019) 10:329. doi: 10.3389/fendo.2019.00329
63. Spergel DJ. Neuropeptidergic modulation of GnRH neuronal activity and GnRH secretion controlling reproduction: insights from recent mouse studies. Cell Tissue Res (2019) 375(1):179–91. doi: 10.1007/s00441-018-2893-z
64. Nita M, Grzybowski A. The role of the reactive oxygen species and oxidative stress in the pathomechanism of the age-related ocular diseases and other pathologies of the anterior and posterior eye segments in adults. Oxid Med Cell Longev (2016) 2016:3164734. doi: 10.1155/2016/3164734
65. Rybka J, Kupczyk D, Kędziora-Kornatowska K, Pawluk H, Czuczejko J, Szewczyk-Golec K, et al. Age-related changes in an antioxidant defense system in elderly patients with essential hypertension compared with healthy controls. Redox Rep (2011) 16(2):71–7. doi: 10.1179/174329211X13002357050897
66. Quijano C, Trujillo M, Castro L, Trostchansky A. Interplay between oxidant species and energy metabolism. Redox Biol (2016) 8:28–42. doi: 10.1016/j.redox.2015.11.010
Keywords: hydrogen peroxide, gonadotropin-releasing hormone neurons, hypothalamic-pituitary-gonadal axis, patch-clamp, KATP channels, reactive oxygen species
Citation: Rijal S, Jang SH, Cho DH and Han SK (2022) Hydrogen peroxide suppresses excitability of gonadotropin-releasing hormone neurons in adult mouse. Front. Endocrinol. 13:939699. doi: 10.3389/fendo.2022.939699
Received: 09 May 2022; Accepted: 14 October 2022;
Published: 28 October 2022.
Edited by:
Klaudia Barabas, University of Pécs, HungaryReviewed by:
Imre Farkas, Institute of Experimental Medicine (MTA), HungaryHonoo Satake, Suntory Foundation for Life Sciences, Japan
Copyright © 2022 Rijal, Jang, Cho and Han. This is an open-access article distributed under the terms of the Creative Commons Attribution License (CC BY). The use, distribution or reproduction in other forums is permitted, provided the original author(s) and the copyright owner(s) are credited and that the original publication in this journal is cited, in accordance with accepted academic practice. No use, distribution or reproduction is permitted which does not comply with these terms.
*Correspondence: Dong Hyu Cho, b2JneW4yMDAxQGpibnUuYWMua3I=; Seong Kyu Han, c2toYW5AamJudS5hYy5rcg==
†These authors have contributed equally to this work