- Laboratorio RAMSES, IRCCS Istituto Ortopedico Rizzoli, Bologna, Italy
The progressive decline of bone mass and the deterioration of bone microarchitecture are hallmarks of the bone aging. The resulting increase in bone fragility is the leading cause of bone fractures, a major cause of disability. As the frontline pharmacological treatments for osteoporosis suffer from low patients’ adherence and occasional side effects, the importance of diet regimens for the prevention of excessive bone fragility has been increasingly recognized. Indeed, certain diet components have been already associated to a reduced fracture risk. Organosulfur compounds are a broad class of molecules containing sulfur. Among them, several molecules of potential therapeutic interest are found in edible plants belonging to the Allium and Brassica botanical genera. Polysulfides derived from Alliaceae and isothiocyanates derived from Brassicaceae hold remarkable nutraceutical potential as anti-inflammatory, antioxidants, vasorelaxant and hypolipemic. Some of these effects are linked to the ability to release the gasotrasmitter hydrogen sulfide (H2S). Recent preclinical studies have investigated the effect of organosulfur compounds in bone wasting and metabolic bone diseases, revealing a strong potential to preserve skeletal health by exerting cytoprotection and stimulating the bone forming activity by osteoblasts and attenuating bone resorption by osteoclasts. This review is intended for revising evidence from preclinical and epidemiological studies on the skeletal effects of organosulfur molecules of dietary origin, with emphasis on the direct regulation of bone cells by plant-derived polysulfides, glucosinolates and isothiocyanates. Moreover, we highlight the potential molecular mechanisms underlying the biological role of these compounds and revise the importance of the so-called ‘H2S-system’ on the regulation of bone homeostasis.
Highlights
A literature search was conducted using MEDLINE database. Relevant pre-clinical and clinical studies were selected using a combination of keywords including bone, diet and/or organosulfur compounds, Allium, Brassicaceae, alliin, allicin, garlic, ajoene, diallyl trisulfide, diallyl disulfide, S-allylcysteine, diallyl sulfide, glucosinolate, thiosulfinate, sulforaphane, broccoli, methyl sulfide, isothiocyanates. Additional studies were identified by an extensive manual search of bibliographic references in original papers and reviews. Abstracts and non-English papers were not included. This study selected a total of in vitro studies (10 Alliaceae, 9 Brassicaceae); in vivo studies (17 Alliaceae, 11 Brassicaceae) and population-based studies (4 Alliaceae, 1 Brassicaceae).
Introduction
Osteoporosis (OP) is a chronic metabolic bone disease characterized by the deterioration of bone microarchitecture and a reduction in bone mass, leading to decreased bone strength and increased risk of bone fracture (1). Approximately 6 % of men and 21 % of women aged 50–84 years are diagnosed with OP and the number of fragility fractures in Europe has increased from 3.1 to nearly 4.3 million in 20 years since year 2000 (2); due to the strong correlation with the ageing of the population, the prevalence of OP is projected to further increase over the next decades (3).
At the bone tissue level, OP is characterized by increased bone porosity which results from the loss of balance between bone formation and bone resorption as aging, disuse, inflammatory diseases, hormonal imbalance or the effect of glucocorticoids impair the ability of osteoblast to keep up with the pace of bone resorption by the osteoclasts (4). Importantly, aging is associated with a decreased number of osteoprogenitor cells, inhibited proliferation, decreased mineralizing capacity, and a shift of osteogenic differentiation toward adipogenesis in senescent mesenchymal stromal cells (MSCs) (5–7).
Pharmacotherapy helps patients to prevent the occurrence or recurrence of fragility fractures and to manage symptoms. However, drugs are mostly used in patients who already show severe bone loss, and the existence of side effects, although very limited in prevalence, often leads to low patient’s adherence to anti-OP drugs (8, 9). In this context, non-pharmacological strategies aimed at preventing excessive bone loss hold relevance given that OP remains in most cases a subclinical condition until fracture occurs.
One safe way to prevent bone loss and reduce the risk of bone fracture is to positively impact bone mass through healthy lifestyles and nutrition (10, 11). In particular, the importance of defining specific diet regimens for the prevention of excessive bone fragility has been increasingly recognized (12–15). Adherence to Mediterranean diet lowered hip fracture risk (16) and certain micronutrients contained in fruit and vegetables contributed to delay bone fragility in ageing and to decrease the incidence of bone fractures (17–20). Moreover, a dietary pattern consisting of a high consumption of fruits, vegetables and seafood, has been shown to be directly associated with increased bone mineral density (BMD), independent of dietary calcium intake (21, 22).
Phytochemicals are defined as the chemical bioactive components of nutrient plants that may provide desirable health benefits beyond basic nutrition to reduce the risk of major chronic diseases. They include several classes of compounds: terpenoids, polyphenols, alkaloids, organosulfur compounds (OSCs) and phytosterols (23). Concerning OSCs, much of the research on their health benefits has been in the areas of cardiovascular diseases, cancer and neurological disorders (24–26). However, a growing body of scientific evidence supports the idea that dietary OSCs may play an important role for skeletal health by favoring bone anabolism, inhibiting bone catabolism, and preventing pathological bone loss.
This manuscript intends to provide an up-to-date review of the current evidence from preclinical (both in vitro and in vivo) and clinical studies on the skeletal effects of OSCs of dietary origin, discussing the chemical nature, the mechanism of action and the potential role of hydrogen sulfide (H2S) in their biological action. A specific focus is given to the pair glucoraphanin (GRA)-sulforaphane (SFN) as a paradigm of OSCs-H2S system in bone tissue. Finally, implications and future challenges in the field will be discussed considering the potential translation of OSCs-containing dietary components to clinical studies.
Dietary sources and chemical nature of OSCs
Naturally derived OSCs are a broad class of molecules containing sulfur, predominantly found in edible plants belonging to the Allium and Brassica (also known as cruciferous vegetables) genera. These plants have been widely used throughout the centuries either as vegetables for culinary purposes as well as in folk and traditional medicine, given their renowned medicinal properties and therapeutic effects. Allium genus consists of more than 600 species which are among the oldest cultivated vegetables used as food and still represent one of the main components of the Mediterranean diet (27). Brassica genus consists of 37 species; among them, several species are known for their nutritional and therapeutic properties (28, 29). A partial list of edible plants belonging to the Allium and Brassica genera, and their main content in OSCs, is reported in Table 1.
In Allium, over half of the total sulfur content within the mature garlic bulb is found in the form of S-alk(en)yl cysteine sulfoxides (ASCOs) (69), non-protein sulfur amino acids which are converted to their respective thiosulfinates or propanethial-S-oxide upon tissue damage (70).
The synthesis of ASCOs in Allium species starts with the transformation of γ-glutamyl peptides (such as γ-l-glutamyl-S-methyl-L-cysteine) into sulfur-containing γ-glutamyl-S-alk(en)yl-cysteines such as γ-glutamyl-S-methyl-cysteines, γ-glutamyl-S-allyl-cysteine, γ-glutamyl-propenyl-l-cysteine sulfoxide (PeCSO). These are further deglutamylated and S-oxygenated to yield S-alk(en)yl-l-cysteine sulfoxides (71, 72). These reactions are catalyzed by γ-glutamyl transpeptidase, l-glutaminases, and oxidase in the cytoplasm of plant cells. The intact garlic bulbs contain alliin, γ-glutamyl-S-allyl-l-cysteine (GSAC), methiin, S-trans-1-propenyl-l-cysteine sulfoxide, S-2-carboxypropylglutathione, S-allylcysteine (SAC) (37).
When the bulbs are cut, crushed, chopped or chewed, the enzyme alliinase (a vacuolar lyase) is released from vacuoles and catalyzes the formation of sulfenic acids from l-cysteine sulfoxides: S-allyl-l-cysteine sulfoxide (alliin); S-methyl-l-cysteine sulfoxide (methiin); S-propyl-l-cysteine sulfoxide (propiin); S-trans-1-propenyl-l-cysteine sulfoxide (isoallin) (71, 72). Sulfenic acids spontaneously react with each other to form unstable compounds called thiosulfinates (69): eg. alliin is converted into allicin (alkenyl alkene thiosulfinate - diallyl thiosulfinate). Allicin immediately decomposes into allyl sulfide (AS), diallyl disulfide (DADS), diallyl trisulfide (DATS), diallyl tetrasulfide, dipropyl disulfide (DPDS), ajoenes, and vinyldithiins (72). The direct catabolism of γ-glutamylcysteine by γ-glutamyltranspeptidase leads to the formation of SAC and S-allylmercaptocysteine (SAMC). Allicin can react with glutathione and l-cysteine to produce S-allylmercaptoglutathione (SAMG) and SAMC, respectively (69, 72).
Among Allium, the most common ASCOs are alliin, methiin, propiin and isoalliin (70, 73, 74). However, they are differentially expressed in specific edible plants. The most abundant in garlic is alliin; in onion isoalliin, methiin, propiin are predominantly detected.
In Brassica vegetables two different kinds of OSCs are present: methiin, mainly known from Allium vegetables, and glucosinolates (S-β-thioglucoside N-hydroxhysulfates, GLS). Methiin is metabolized to (+)-S-alk(en)yl-l-cysteine sulfoxides which can degrade to volatile organosulfur compounds (VOSCs) such as S-methyl methane thiosulfinate, which is converted to dimethyl trisulfide and dimethyl disulfide.
GLS are sulfur-based compounds that consist of β-thioglycoside N-hydroxysulfates with various side chains and a sulfur-linked β-d-glycopyranose moiety. A very different profile of GLS may be found in different Brassica extracts (75). Natural isothiocyanates (ITCs) are bioactive OSCs derived from the hydrolysis of GLS by the enzyme myrosinase. In plant cells, GLS are physically separated from myrosinases and come in contact only upon tissue damage or crushing. Importantly, myrosinase is not expressed by mammalian cells; however, a small proportion is converted in the mouth by action of plant myrosinase released by chewing (76); moreover, the gut microbiota is entailed with myrosinase activity and constitutes the major site in humans where GLS are hydrolyzes to ITCs (77). While GLS are chemically stable and are characterized by a relatively long half-life, ITCs are highly reactive and short-lived in vivo (75, 78).
Effect of OSCs on bone tissue: Preclinical evidence
The effect of OSCs in bone tissue has been investigated in several preclinical models, revealing a strong potential to preserve skeletal health by stimulating the bone forming activity of osteoblasts and inhibiting the bone resorbing activity of osteoclasts, two of the key processes of bone remodeling (79).
Figures 1, 2 provide a graphical summary, respectively, of the main biological processes and molecular targets regulated by OSCs within MSCs/osteoblasts and monocytes/osteoclasts. A detailed description of these mechanisms is provided in the next paragraphs.
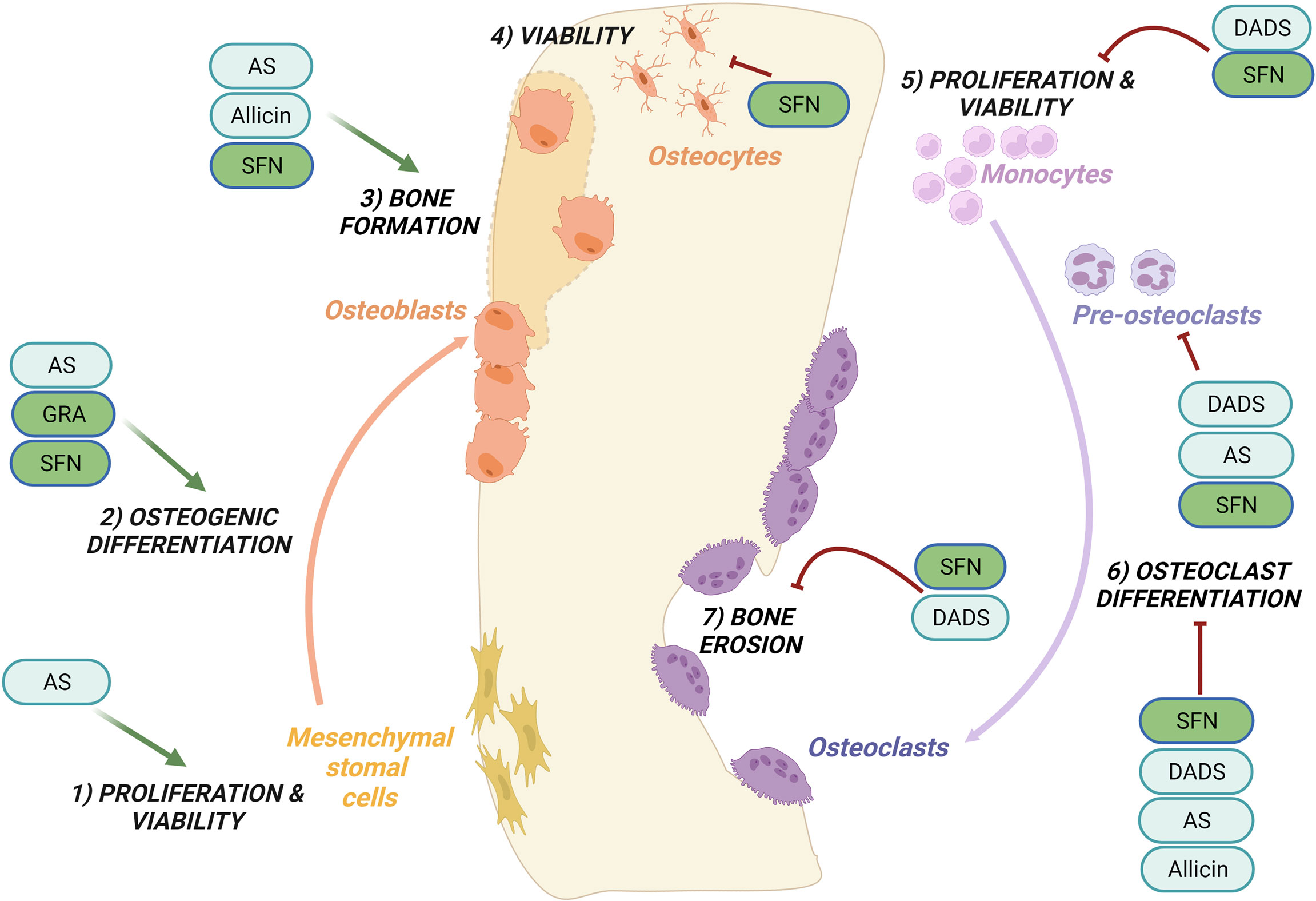
Figure 1 Regulation of bone remodeling processes by purified OSCs molecules. Bone remodeling is governed by the balance between bone formation by the osteoblasts (left side) and bone erosion by the osteoclasts (right side). Ancillary processes are shown. OSCs specifically regulate the following processes: promote cells proliferation and viability of mesenchymal stromal cells (1) while inhibit the proliferation and viability of monocytes (5); promote the osteogenic differentiation (2) and bone formation (3); inhibit at different stages osteoclast differentiation (6) and reduce bone erosion (7); inhibit the viability of osteocytes (4). Among the OSCs which modulate bone processes are allicin, allyl sulfide (AS), sulforaphane (SFN), glucoraphanin (GRA), diallyl sulfide (DADS). See the text for details.
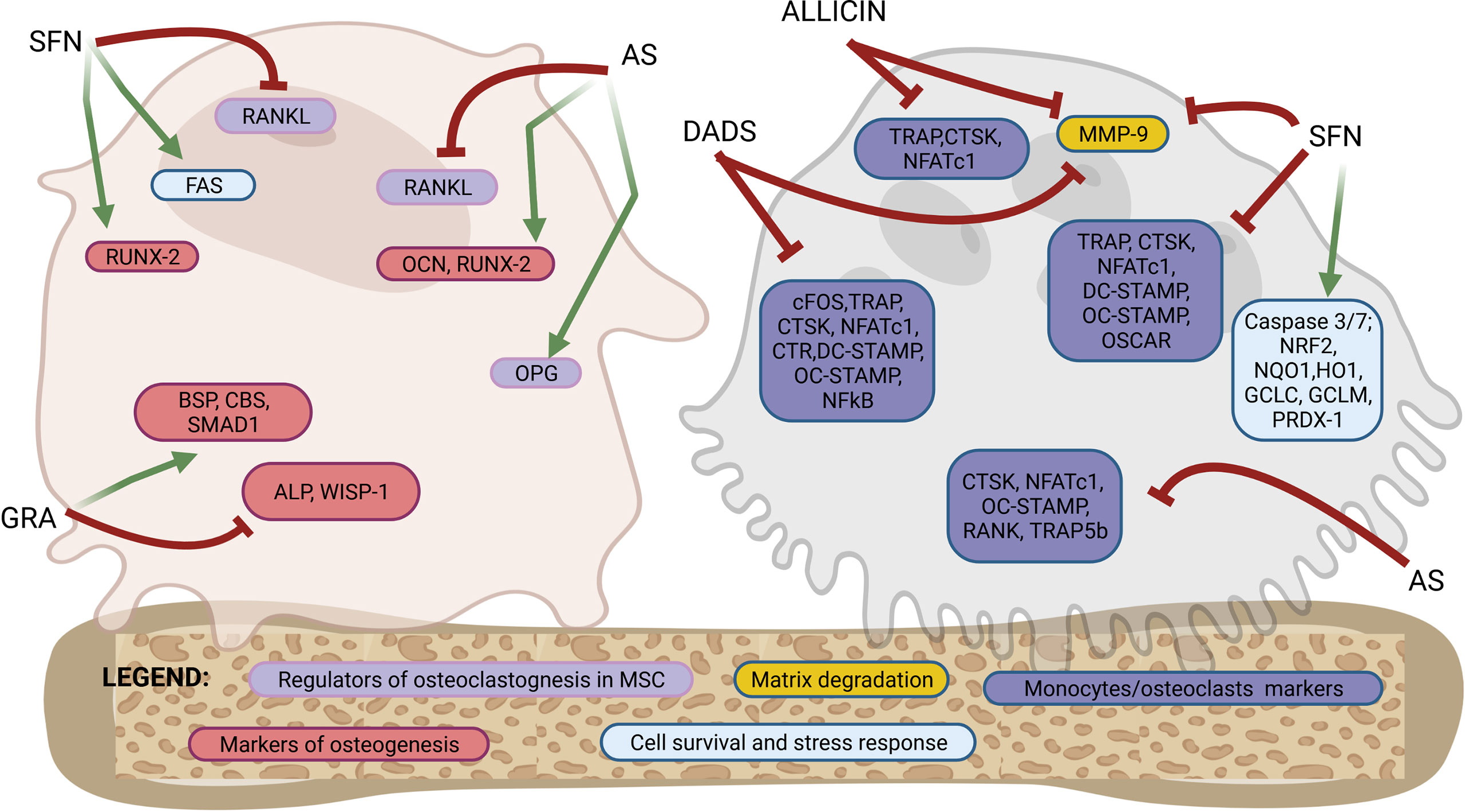
Figure 2 Molecular targets of purified OSCs molecules in bone cells. Osteoblastogenesis and osteoclastogenesis are the two key processes of bone remodeling and are regulated by a tightly organized activation of specific molecular targets. This figure shows a schematic representation of a mesenchymal stromal cells/osteoblast and a monocyte/osteoclast to highlight the specific molecular targets regulated by OSCs at different stages of differentiation from precursors to fully differentiated cells. Among the OSCs which drives the modulation of specific molecular targets are allicin, allyl sulfide (AS), sulforaphane (SFN), glucoraphanin (GRA) and diallyl sulfide (DADS). The overall effects are an activation of osteogenic differentiation in mesenchymal stromal cells and both a direct and indirect inhibition of osteoclast differentiation. Follows a list of the molecular targets shown in the figure. Markers of osteoblastogenesis: osteocalcin (OCN), runt-related transcription factor 2 (RUNX-2), alkaline phosphatase (ALP), WNT1-inducible-signaling pathway protein 1 (WISP-1), bone sialoprotein (BSP), cystathionine-β-synthase (CBS), SMAD family member 1 (SMAD-1). Markers of regulators of osteoclastogenesis produced by mesenchymal stromal cells or osteoblasts: receptor activator of nuclear factor-κB ligand (RANKL), osteoprotegerin (OPG). Marker of cells survival and stress response: FAS, caspase 3/7, nuclear factor erythroid-derived 2-related factor 2 (NRF2), NAD(P)H: quinone oxidoreductase 1 (NQO1), heme oxygenase-1 (HO1), glutamate cysteine ligase catalytic subunit (GCLC), glutamate-cysteine ligase modifier subunit (GCLM), peroxiredoxin 1 (PRDX-1). Markers of osteoclasts: nuclear factor of activated T-cells cytoplasmic 1 (NFATc1), cathepsin K (CTSK), receptor activator of NF-KB (RANK), osteoclast stimulatory transmembrane protein (OC-STAMP), dendritic cell specific transmembrane protein (DC-STAMP), osteoclasts-specific activating receptor (OSCAR), tartrate-resistant acid phosphatase (TRAP), calcitonin receptor (CTR), c-fos, tartrate-resistant acid phosphatase 5b (TRAP-5b), matrix metallopeptidase 9 (MMP-9). See the text for details.
Tables 2–5 summarize data from preclinical studies showing an effect of extracts rich in OSCs or individual OSCs molecules derived from Allium (Tables 2, 3) and Brassica vegetables (Tables 4, 5).
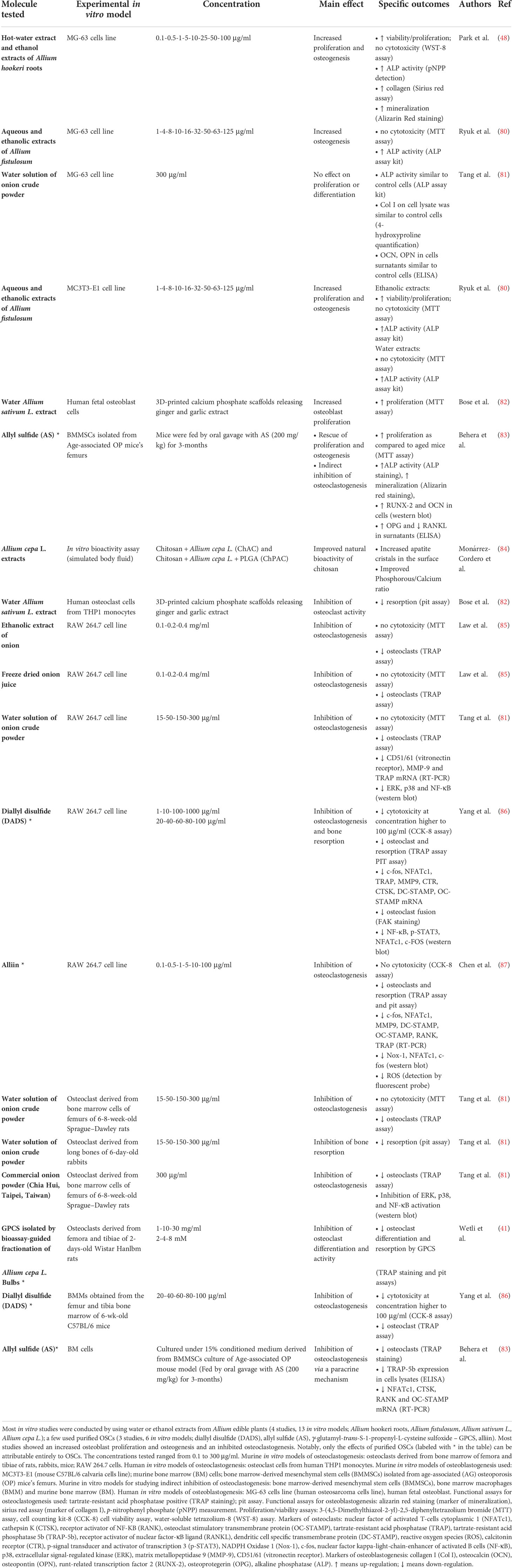
Table 2 Alliaceae-derived OSCs: effects on in vitro models of osteoclastogenesis and osteoblastogenesis.
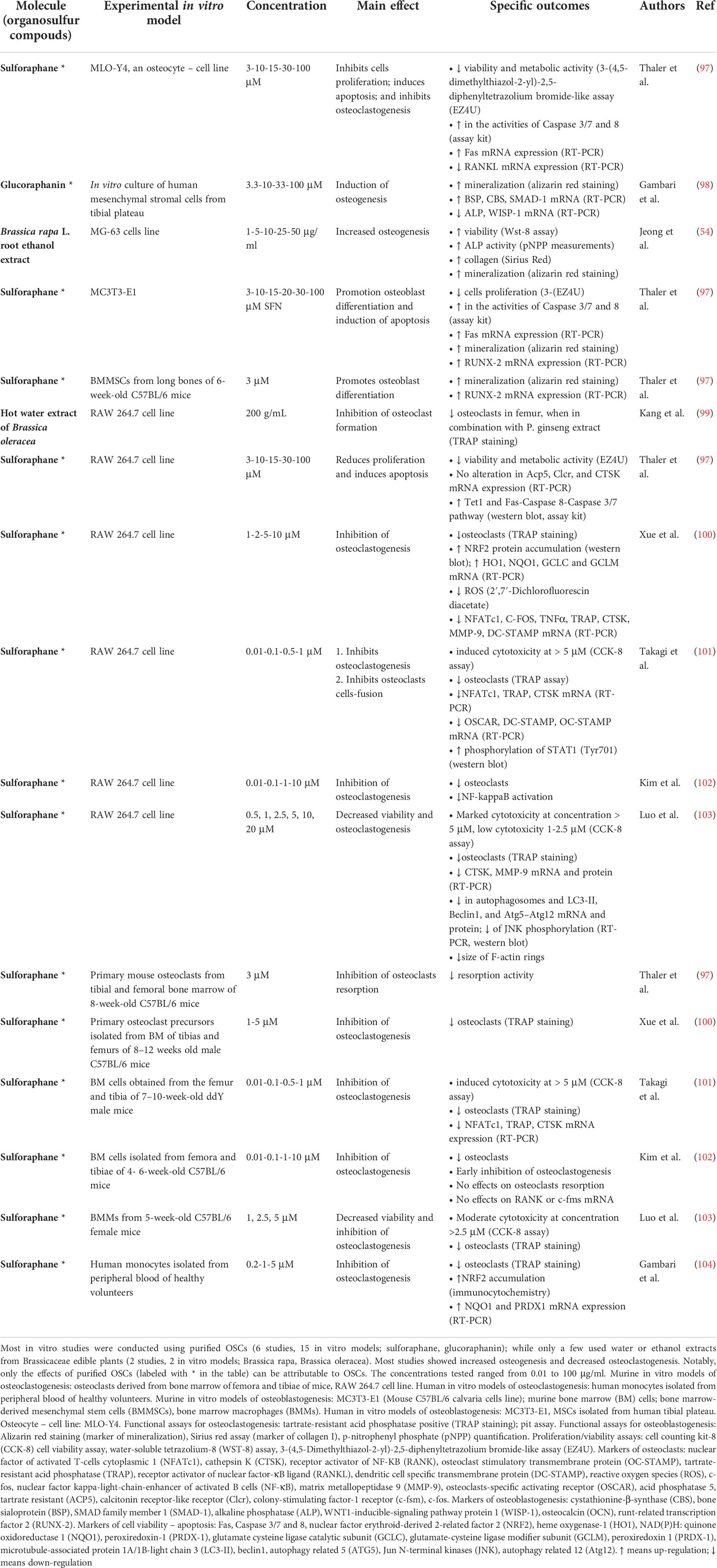
Table 4 Brassicaceae-derived OSCs: effects on in vitro models of osteoclastogenesis and osteoblastogenesis.
Importantly, while data obtained from studies on purified molecules (labeled with * in the tables) clearly attest to the effectiveness of individual OSCs, the effect of OSCs-rich extracts may result from the combined action of other phytochemicals contained in the extracts. Indeed, Allium species contains polyphenols, flavonoids, flavanols, anthocyanins, tannins, ascorbic acid, saponins and fructans (109–111); Brassica species contains ascorbic acid, phenolics, carotenoids, terpenes, phytoalexins and alkaloids (29, 112).
Regulation of osteogenesis and bone formation
Osteoblasts, the bone forming cells, regulate bone homeostasis by synthesizing a wide variety of extracellular protein of bone matrix. They differentiate from MSCs through the osteogenic differentiation process which is regulated by an orchestrated activation of several pathways. The master regulator of osteogenic differentiation is runt-related transcription factor 2 (RUNX-2), which is expressed in the early stages of differentiation and is at the intersection of several signaling pathways among which growth hormone-janus Kinase 2 (GH-JAK2), bone morphogenetic protein-SMAD (BMP-SMAD), canonical Wingless/Integrated (Wnt) and Notch signaling (113, 114). Among the genes targeted by RUNX-2 are osteocalcin (OCN), collagen I (Col I), bone sialoprotein (BSP), osteopontin (OPN), alkaline phosphatase (ALP). BSP, OPN and ALP are correlated to matrix mineralization; Coll I and OCN are among the major components of bone matrix. Wnts-β-catenin signal activates osteogenic target genes such as distal-less homeobox 5 (Dlx5) and osterix (Osx) (115) and suppresses the transcription of adipogenic transcription factors such as peroxisome proliferator-activated receptor-γ (PPAR-γ) (116). SMAD family number 1 (SMAD-1) is a critical immediate downstream mediator of BMP receptor transduction (117). Among downstream targets of canonical Wnt and BMP signaling is WNT1-inducible signaling pathway protein 1 (WISP-1), which is involved in the positive regulation of osteogenesis and negative regulation of adipogenesis (118). Interestingly, the expression of H2S generating enzymes, cystathionine-β-synthase (CBS) and cystathionine-γ-lyase (CSE), was found to be transcriptionally up-regulated during osteogenesis and to correlate with the biosynthesis of mineral matrix (119), thus suggesting a role for endogenous H2S in osteogenic differentiation. Osteogenic differentiation is associated to increased ALP activity and mineralization in vitro and increased BMD in vivo. Osteoblast finally differentiate toward osteocytes, multifunctional bone cells that are embedded in mineralized bone matrix. Osteocytes act as orchestrators of bone remodeling, through regulation of both osteoclast and osteoblast activity; as regulators of phosphate metabolism and calcium availability, by functioning as an endocrine cell; as mechanosensory cells (120). Key factors produced by osteocytes are sclerostin (a negative regulator of bone mass), FGF-23 (a regulator of phosphate metabolism), and the key regulator of osteoclast differentiation receptor activator of nuclear factor κβ ligand (RANKL), also produced by osteoblasts and MSCs (120, 121).
Most studies investigating OSCs extracts focused on a commonly used human osteoblastic model, the human osteosarcoma cell line (MG-63 cells). They showed increased cell proliferation and increased osteogenesis/mineralization by Allium Hookeri roots treatments (48); increased osteogenesis by Allium fistulosum (80) and Brassica Rapa L. (Jeong); while no effect on proliferation and differentiation was shown by treatment with water solution of onion crude powder (81). However, MG-63 cells are osteoblasts derived from osteosarcoma, a malignant bone tumors, thus are not fully representative of physiological osteoblasts (122). Increased cells proliferation by Allium genus was also shown by ginger and garlic extracts released by 3D-printed calcium phosphate scaffolds on human fetal osteoblast cells (82); increased osteogenesis by Allium fistulosum was also shown in the mouse C57BL/6 osteoblastic calvaria cell line (MC3T3-E1) (80). Up to date no studies on primary cultures of human MSCs have been performed with extracts derived from Alliaceae or Brassicaceae.
Treatment with Alliaceae extracts improved bone formation in normal control rats (41, 48, 88) and mitigated the bone loss due to several pathological conditions among which osteoporosis (47, 80, 94). Similarly, extracts from Brassicaceae induced bone formation in control rats (54) and prevented bone loss in several models of osteoporosis (59, 99, 106–108). Interestingly, treatment with Lepidium sativum resulted in improved fracture healing (28, 123).
Notably, several studies focused on purified OSCs molecules, revealing a specific effect of OSCs on proliferation, osteogenic differentiation, and bone formation. Behera et al. showed increased proliferation, ALP activity and mineralization in murine MSCs derived from femur bone marrow (BMMSCs) upon allyl sulfide stimulation, with a mechanism implicating increased RUNX-2 and OCN expression (83). Thaler et al. demonstrated increased mineralization in mouse MSCs and in an ex vivo culture of calvariae explants treated with SFN (97); at the molecular level, SFN induced up-regulation of RUNX-2 in mouse MSCs (97). Gambari et al. showed increased mineralization and BSP, CBS and SMAD-1 mRNA up-regulation by GRA administration in primary human MSCs (98). Finally, with regards to osteocyte regulation, Thaler et al. showed that SFN inhibited proliferation in murine osteocyte-like cell line (MLO-Y4) (97).
Purified OSCs have also been tested in in vivo models of bone loss or osteolysis, showing beneficial effects on preserving bone mass. Oral administration of allyl sulfide in an age-associated osteoporosis mouse model resulted in increased bone density at X-ray analysis and increased serum levels of procollagen 1 intact N-terminal propeptide (P1NP; a marker of bone formation) (83). Similarly, intragastric administration of allicin increased BMD, as detected by dual energy X-ray absorptiometry, and bone strength, as measured by three-point bending assay, in a model of aging osteoporotic rats (96). Intraperitoneal administration of allicin prevented the bone loss in a mice model of lead-induced bone loss (osteoporosis induced by a toxic heavy metal), as measured by increased BMD, trabecular number (Tb.N), trabecular thickness (Tb.Th) and decreased trabecular space (Tb.Sp), quantified using micro-CT analysis (95). Finally, SFN showed to be protective against bone loss in different in vivo models. Intraperitoneal injection of SFN in lipopolysaccharide (LPS)-induced erosion of the mice calvaria bone induced increased trabecular bone volume (BV/TV), increased Tb.N and decreased Tb.Sp, as measured by micro-CT analysis (103); moreover, intraperitoneal injection of SFN in a mice model of ovariectomy-induced bone loss stimulated trabecular bone formation, increased Tb.N and decreased Tb.Sp (97); finally, the oral administration of SFN-01 (a stabilized form of SFN) in a mice model of osteoarthritis, resulted in increased trabecular bone volume and serum P1NP (105).
Regulation of osteoclastogenesis and bone resorption
Osteoclasts are bone-resorbing cells which arise from immature monocytes and mature tissue macrophages (124). Osteoclasts differentiation stems from the signaling triggered by two critical cytokines produced by MSCs, osteoblasts and osteocytes: macrophage colony-stimulating factor (M-CSF) and RANKL binding, respectively, to the receptors colony-stimulating factor-1 receptor (c-fms) and receptor activator of nuclear factor κ B (RANK) (125, 126). RANKL signaling activation induces various intracellular signal transduction cascades such as tumor necrosis factor receptor-associated factor 6 (TRAF-6), NADPH oxidase 1 (NOX-1), RAC family small GTPase 1 (RAC1), nuclear factor kappa-light-chain-enhancer of activated B cells (NF-κB), and nuclear factor-activated T cells c1 (NFATc1), c-fos (127–129). Other receptors involved in osteoclastogenesis are calcitonin receptor (CTR), ITAM bearing Fc receptor standard g chain (FcRγ), osteoclasts-specific activating receptor (OSCAR) (126, 130); key signaling is mediated by mitogen-activated protein kinases (MAPK), and includes extracellular signal-regulated kinase (ERK), c-Jun N-terminal kinase (JNK), and p38 activation. Moreover, critical to osteoclast differentiation and function are: intracellular reactive oxygen species (ROS) generation, which act as key signaling molecules (82, 88, 94); osteoclast fusion mediated among other factors, by the fusogenic molecules osteoclasts-stimulatory transmembrane protein (OC-STAMP) and dendritic cell-specific transmembrane protein (DC-STAMP) (126, 131, 132); and expression of specific enzymes such as tartrate-resistant acid phosphatase (TRAP), cathepsin K (CTSK) (126, 130), tartrate-resistant acid phosphatase 5b (TRAP5b) (83) and matrix metallopeptidase 9 (MMP-9).
Extracts from both Allium and Brassica species were shown to attenuate osteoclast differentiation in vitro in the murine macrophage cell line, RAW 264.7. In particular, extracts of onion (85), freeze dried onion juice (85), solution of onion crude powder (81) inhibited osteoclastogenesis, as measured by TRAP staining in vitro. A similar effect was achieved by an extract of Brassica oleracea but only in combination with extract from Panax ginseng (99). Using human THP1 monocytes, Bose et al. showed that ginger and garlic extracts reduce the frequency and the size of resorption pits carved by osteoclasts (82); inhibition of osteoclast number was found also by onion and commercial onion extracts in rat and rabbit osteoclasts (81). Notably, Wetli et al. demonstrated that onion extract reduced rat osteoclast differentiation and were able to isolate a specific sulfoxide component of onion powder, γ-glutamyl-trans-S-1-propenyl-l-cysteine sulfoxide (GPCS), which the authors found to be the key responsible of this biological activity (41).
In vivo administration of extracts rich in OSCs decreased osteoclastogenesis and bone erosion in rodent model of osteoporosis; Huang et al. showed that ovariectomized rats fed with different concentrations of onion extracts (up to 14% wt/wt in the diet powder) were partly protected against loss of bone mass and bone material properties (94); moreover, histomorphometry revealed that treatment with onion extracts was associated with a lower number of osteoclasts in vivo (94). Similar findings were reported by Kang et al. using ovariectomized mice fed with a combination of extracts obtained from Panax ginseng and Brassica oleracea (99). Furthermore, Abdallah HM et al. reported that ovariectomized rats treated with extracts of Lepidium sativum were partly protected against osteoporosis and showed a sharply decreased RANKL/osteoprotegerin (OPG) ratio in femur bones (59).
Studies that used purified OSCs molecules further supported efficacy and specificity. Yang et al. demonstrated a dose-dependent inhibition of osteoclast differentiation and a decreased bone resorption by mature osteoclasts upon treatment with DADS (86). Monocytes proliferation and viability was inhibited by SFN (97).
Luo et al. (103) and Xue et al. (100) showed that SFN inhibits osteoclast differentiation in RAW 264.7 murine macrophagic cell line; Takagi et al. (101) and Kim et al. (102) showed similar findings in murine BM cells and so did Gambari et al. (104) in a model of osteoclast derived from human monocytes. Moreover, Chen et al. reported the inhibition of osteoclast differentiation by alliin in RAW 264.7 via scavenging of ROS signaling (87).
Mechanisms of regulation of osteoclastic differentiation by OSCs involved different molecular targets. Li et al. reported that the anti-osteoclastogenic activity of allicin in mice is associated to the activation of the SIRT1/FOXO1 pathway and ROS scavenging (95). Similarly, one key mechanism of action of SFN is the activation of the master regulator of the antioxidant defense system, nuclear factor erythroid-derived 2-related factor 2 (NRF2), and its downstream target antioxidant and detoxifying enzymes (133), which is known to actively inhibit mouse osteoclasts differentiation in vitro (104, 134). SFN modifies sulfhydryl groups in kelch-like erythroid-cell-derived protein with CNC homology (ECH)-associated protein (KEAP-1), causing KEAP-1 dislocation, NRF2 stabilization and nuclear translocation (135); moreover, SFN regulates NRF2 expression via epigenetic mechanisms (136). Coherently, SFN was shown to increase NRF2 protein accumulation in RAW 264.7 murine cell line, to increase the expression of some NRF2-mediated antioxidant genes (heme oxygenase-1, HO1; NAD(P)H: quinone oxidoreductase 1, NQO1; glutamate cysteine ligase catalytic subunit, GCLC; ligase modifier subunit, GCLM) and decrease intracellular ROS production, and the overall number of osteoclasts as shown by Xue et al. (100). Similarly, SFN was shown to inhibit the osteoclast differentiation of human monocytes while increasing NRF2 nuclear translocation and protein expression of NRF2-mediated antioxidant genes (NQO1; Peroxiredoxin 1, PRDX-1), as published by Gambari et al. (104). Finally, SFN induces Caspase 8 and 3/7, thus inducing apoptosis in a RAW 264.7 murine cell line as shown by Thaler et al. (97).
Moreover, downregulation of the key transcription factor NFATc1 is implicated in several studies showing inhibition of osteoclast development: Yang et al. reported a dose-dependent down-regulation of NFATc1 in a RAW 264.7 murine cell line after DADS treatment (86); Xue et al. (100) and Takagi et al. (101), respectively, reported similar findings in RAW 264.7 murine cell line and in murine BM cells after SFN treatment; Behera et al. in murine BM cells after allyl sulfide treatment (83). The inhibition of other key transcription factor c-Fos and Nf-kB was shown by Yang et al. in a RAW 264.7 murine cell line after DADS treatment (86).
Several other proteins implicated in the adhesion an (83, 95, 100, 137), in RAW264.7 cells and murine BM and are detailed in Tables 2, 4.
OSCs can modulate the expression of osteoclasts-specific activating receptors, necessary for the co-stimulatory signaling with immunoreceptors and prevented osteoclast fusion by inhibiting fusogenic molecules. Takagi et al. showed in RAW 264.7 murine cell line that OSCAR is inhibited by SFN (101). DC-STAMP was found inhibited in RAW 264.7 murine cell line after SFN treatment as shown by Takagi et al. (101) and by Xue et al. (100) and after DADS treatment as shown by Yang et al. (86). OC-STAMP was found inhibited in RAW 264.7 murine cell line after SFN treatment as shown by Takagi et al. (101).
Finally, OSCs compounds were shown to inhibit osteoclast differentiation via a paracrine mechanism, acting on osteoclasts-supporting cells. Thaler et al. showed that RANKL was inhibited by SFN in a murine osteocytes cell line (MLO-Y4) (97). Behera et al. showed that RANKL was inhibited while OPG was increased in supernatants of murine MSCs cells culture treated with allyl sulfide (83); and that treatment with this conditioned medium inhibited the expression of RANK and osteoclast differentiation of murine bone marrow (BM) cells (83).
Only a few in vivo studies used purified OSCs to investigate bone metabolism. In a mice model of lead-induced bone loss, intraperitoneal injection of allicin alleviates bone loss by preventing oxidative stress and osteoclastogenesis by modulating SIRT1/FOXO1 pathway (95). SFN treatment in a mouse calvaria model treated with LPS decreased the number of osteoclasts (103). Treatment of Lepidium sativum in a rat model of ovariectomy-induced osteoporosis improved mechanical properties of femurs while decreasing TRAP, serum type I collagen breakdown product (CTX-I), RANKL (59) and the number of osteoclasts (107).
H2S release from OSCs as a potential mechanism of bioactivity in bone
H2S is a pleiotropic molecule which provides numerous health benefits by improving hypertension and cardiometabolic disorders (138) (139), relieving pain (140, 141), and increasing insulin sensitivity (142); protecting against neurological diseases including Alzheimer disease (143). Moreover, H2S is critically involved in the extension of lifespan provided by caloric restriction (144, 145). Supraphysiological levels of H2S may be generated in certain pathological conditions and lead to toxicity, inducing inflammation or tissue damage (146).
The intriguing overlap between biological effects attributed to some Allium and Brassica species and those exhibited by the gasotransmitter H2S prompted several researchers to verify the H2S releasing capacity of those molecules. Recently, the ability of releasing H2S was found as a distinctive feature of several OSCs, and a plausible mechanism for their biological effects across different organs and tissues was described. The biological relevance of H2S release by OSCs was first demonstrated by Benavides et al. in the context of a study on the vasoactivity of garlic. The authors showed that garlic polysulfides DATS and DADS, the downstream metabolites of alliin, released H2S in red blood cells; importantly, pre-treating the cells with the thiol-blocking reagent iodoacetamide inhibited the release of H2S, thereby demonstrating that the mechanism by which polysulfides release H2S is dependent on intracellular thiols, such as glutathione (GSH) (147). Chemically, this reaction involves a nucleophilic substitution from thiol at the α carbon of the H2S-donor moiety and a subsequent release of H2S (148). This mechanism is biologically relevant as the relaxation induced by both garlic extract and DADS on isolated rat aortic rings strongly correlated to the amount of H2S released. In the wake of this work, Citi et al. first revealed that a similar mechanism accounts for the ability of several Brassicaceae-derived ITCs to release pharmacologically relevant concentrations of H2S in an l-cysteine dependent manner (149): allyl isothiocyanate (AITC), 4-hydroxybenzyl isothiocyanate (HBITC), benzyl isothiocyanate (BITC), erucin (ER), SFN (149, 150). The same group reported that H2S-release is associated with the in vivo anti-hypertensive, hypoglycemic, pain-relieving, and anti-inflammatory effects of OSCs derived from the Brassicacea Eruca Sativa (138, 151–153). Interestingly, Lucarini et al. first demonstrated that GRA, a GLS, can release H2S in aqueous solution independent of myrosinase, but the chemical mechanism underlying this phenomenon is still unclear (150). Whether other Alliaceae or Brassicaceae-derived OSCs releases H2S is still unknown.
Figure 3 summarizes the known reactions leading to H2S release from polysulfides, GLS or ITCs.
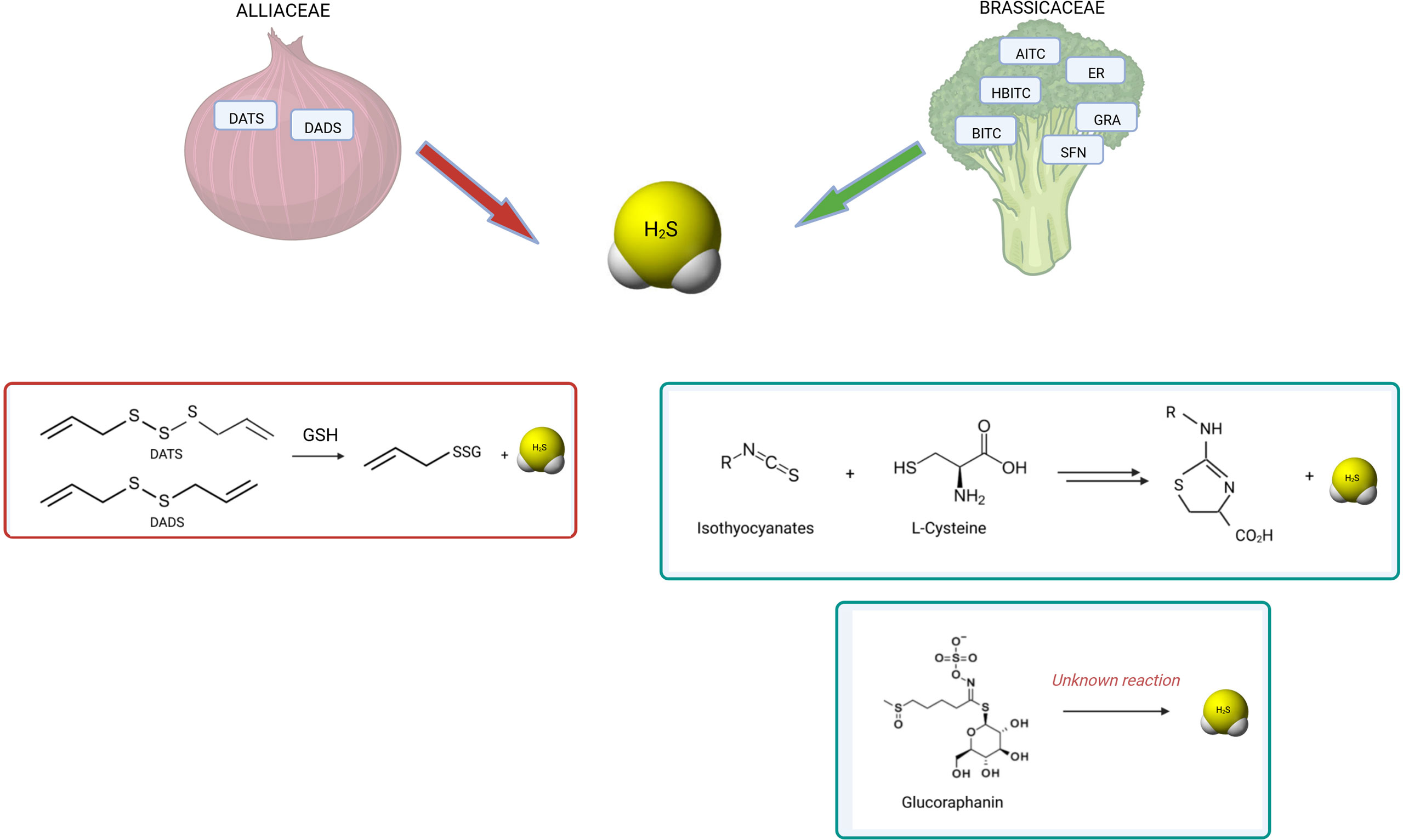
Figure 3 H2S release by OSCs derived from Alliaceae and Brassicaceae. The known reactions occurring for H2S release by polysulfides and isothiocyanates are shown. Among garlic-derived polysulfides, diallyl disulfide (DADS) and diallyl trisulfide (DATS) have been shown to release H2S by reaction with glutathione (GSH) by polarographic H2S sensor (154) (147) (148). Among glucosinolates, GRA has been found to release H2S by amperometric approach (149). Similarly, several isothiocyanates showed H2S-releasing activity: allyl isothiocyanate (AITC), 4-hydroxybenzyl isothiocyanate (HBITC), benzyl isothiocyanate (BITC), erucin (ER), sulforaphane (SFN) (149) (150). While the mechanism of release is unknown for glucosinolates, the mechanism of release by isothiocyanates is dependent on L-cysteine reaction (155). Moreover, different OSCs have different kinetics of H2S release.
This mechanism holds important implications for bone. Recent findings by our group and others demonstrated that H2S plays an important role in the regulation of bone cell differentiation and function. In vitro, H2S-donors promote osteogenic differentiation and stimulate mineralization by increasing calcium intake (156) and the expression of genes directly involved in the biosynthesis of hydroxyapatite, such BSP (157). Furthermore, the expression of the enzymes CBS and CSE, which are responsible for endogenous H2S production, steadily increased during osteogenic differentiation and correlated to mineral apposition (119). Moreover, H2S-donors inhibit osteoclast maturation and resorption by activating the antioxidant response elicited by the NRF2 transcription factor (104, 158). Further attesting to the relevance of H2S in bone homeostasis, evidence from several in vivo preclinical models showed that the depletion of H2S levels is associated with loss of bone mass; similar findings were reported in ovariectomized mice (157), in H2S-deficient CBS+/− mice (156), in glucocorticoids-induced osteoporosis (159). Interestingly, when animals were treated with pharmacological H2S-donors to normalize the plasma level of H2S, bone loss was prevented or reversed (156, 157). The ability of H2S to stimulate bone formation appears to be maintained across various conditions, even unrelated to systemic or genetic disfunctions: for example, the exogenous administration of H2S by means of the pharmacological donor GYY4137 was effective to attenuate the bone loss induced by modelled microgravity (160) and to promote osteogenesis in a model of distraction osteogenesis (161).
Overall, these data demonstrate that H2S regulates osteogenesis and bone formation in both healthy and pathological conditions.
Therefore, H2S release by OSCs could account, at least in part, for their biological properties. However, up to date no clinical or preclinical in vivo studies have investigated the effect of OSCs by correlating their bioactivity to the H2S levels.
The GRA/SFN system: A case-model for OSCs bioactivity based on H2S release
GRA is a glucosinolate abundant in aerial portions, developing florets (flower buds), sprouts, seeds and mature plants of cabbage, broccoli, cauliflower, kale and Brussels sprouts (77). GRA conversion to SFN, an ITC, requires the enzyme myrosinase, an intracellular thioglucosidase, which catalyzes its hydrolysis to an unstable aglucone that spontaneously rearranges to give rise to a range of products, including SFN. SFN is the progenitor of a family of compounds widely studied in the literature mostly due to their antioxidant and anticancer properties. In mammalians, GRA conversion to SFN is primarily mediated by bacterial microflora of the gastrointestinal tract; while a small proportion is generated in the mouth by plant myrosinase when released by plants after chewing. Our current knowledge on the bioavailability and the rate of conversion of GSL into ITCs are largely based on studies on the GRA/SFN system.
Although most of GRA introduced with diet undergoes hydrolysis in the gut by microbial thioglucosidases, a fraction of GRA (around 10-15%) is absorbed directly in the stomach and in the small intestine, before the catabolic breakdown to SFN is triggered by gut microbiota (77, 162).
Gastric acidity appears to attenuate GSL bioavailability (163). However, GRA is not destroyed by digestive enzymes during passage through the digestive tract and is able to reach the rat cecum intact, when is hydrolyzed to SFN which is able to cross the cecal enterocyte for systemic absorption and enterohepatic circulation (164, 165). Conversion of GRA to bioactive SFN by the rat cecal microbiota requires four or more days after broccoli consumption and is reversible (166); however, recent randomized clinical trials have ascertained that upon ingestion of GRA-enriched soups, increased SFN levels were detectable as early as 30’ in plasma and 1h in the urine of patients (162). Attesting the tissue systemic absorption of SFN and ITCs in general, they have been detected in both plasma and synovial fluid of osteoarthritis patients undergoing consumption of GLS-rich diets for 2 weeks (167). On the other hand, the direct delivery of SFN from foods is possible and was demonstrated in recent clinical studies (168, 169) where SFN was shown to be readily bioavailable (170); however, SFN is unstable, requires storage at freezing temperature, and SFN-enriched extracts are difficult to prepare and very expensive (163).
Although most of the research on the biological effects of SFN is focused on cancer because of its effect on cell cycle and apoptosis (171–173), it also regulates bone cells: in vitro, SFN inhibits monocyte cell proliferation and osteoclast differentiation in multiple ways, detailed above (100–104), while increases mineralization in mouse MSCs and in an ex vivo culture of calvariae explants (97). Notably, in one in vivo study the administration of SFN for 5 weeks to normal and ovariectomized mice lead to an approximate 20% increase in bone mass (97), shifting the balance of bone homeostasis and favoring bone acquisition and/or mitigation of bone resorption.
Of note, our group recently demonstrated that GRA obtained from Tuscan black kale promotes osteogenesis in human MSCs, independent of SFN, and this effect is associated to the release of H2S and an increased H2S uptake inside the cells (98). This is relatively unexpected as GLS have been considered for many years a relatively inert precursor of reactive derivatives ITCs. Although the chemistry underlying this phenomenon is still unclear and will require further investigation, this finding suggests that GLS may exert inherent biological activity based on their capacity to release H2S.
As the hydrolytic product of GRA, SFN, had been already shown to inhibit the activity of osteoclast in bone, it can be suggested that the ‘GRA-SFN system’ exerts a beneficial effect on bone both at level of GLS and of its cognate ITC. The routes of absorption of GRA and SFN as well as the proposed mode of action on bone cells is summarized in Figure 4.
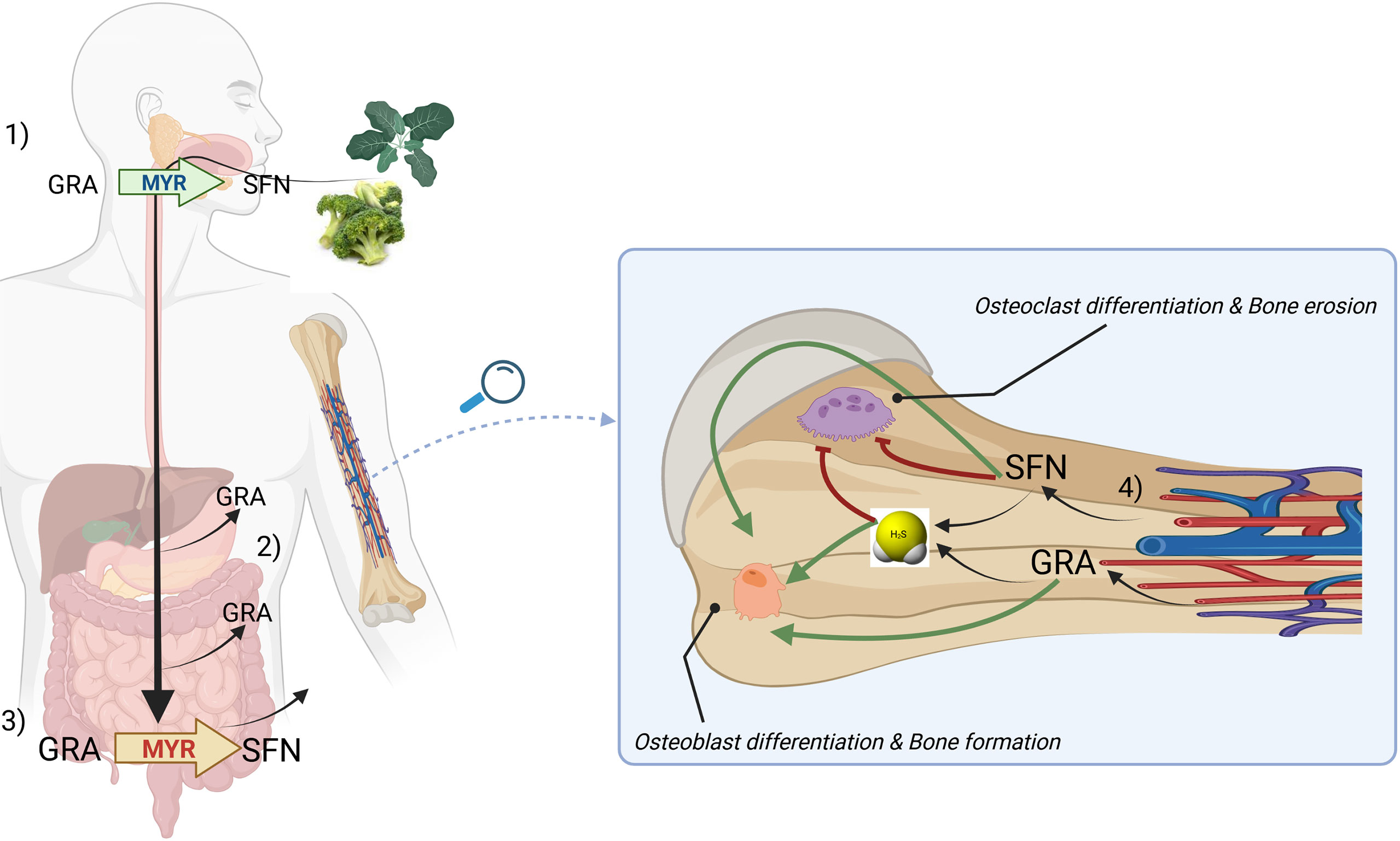
Figure 4 A general model describing the routes of absorption of GRA and SFN and a proposed mechanism of action on bone cells based on H2S-release. Briefly, upon chewing of plants belonging to Brassica genus, myrosinase (MYR, green) is released and can convert glucoraphanin (GRA) to sulforaphane (SFN) (1). GRA can be adsorbed in the stomach or in the small intestine (2). Microbacterial thioglucosidases (MYR, red) converts GRA to SFN which is further adsorbed in large quantities (3). SFN and GRA are released by circulation in bone tissue where can release H2S and exert anabolic and anticatabolic properties on bone cells (4). The mechanism by which H2S can be directly released from GRA has not been clarified yet.
Clinical studies
OSCs and chronic diseases
Despite this review focuses primarily on the skeletal effects of OSCs, much of the clinical research on the health benefits of OSCs is aimed at metabolic or cardiovascular disease and cancer.
Vegetables or extracts rich in OSCs improved dyslipidemia, insulin resistance, hypertension and cardiovascular risk linked to atherosclerotic plaques in human studies.
Among interventional, randomized clinical trials, Jeon et al. evidenced that ethanol extracts from Brassica rapa, administrated as a part of the diet of overweight human for 10 weeks, induce a significant increase in the HLDL-cholesterol concentration and a significant reduction in the total cholesterol/HDL-cholesterol ratio, free fatty acid, and adipsin levels (174). A randomized double-blind trial, performed by Bahadoran et. al., investigated the effects of broccoli sprouts powder containing high concentration of SFN for four weeks in type 2 diabetic patients and showed that broccoli sprouts improve insulin resistance by decreasing serum insulin concentration and ‘homeostatic model assessment for insulin resistance’ (HOMA-IR) score (175).
In a prospective cohort study on Australian women aged 70 years and older, without clinical atherosclerotic vascular disease (ASVD) or diabetes mellitus at baseline, Blekkenhorst et al. investigated the occurrence of ASVD‐related deaths during 15 years of follow‐up and correlated it with several dietary intake, through a multivariable‐adjusted model. Among the nutrients tested, intakes of cruciferous and Allium vegetables were inversely associated with ASVD mortality supporting the evidence that the effect of increased intake of cruciferous and Allium vegetables lowered cardiovascular disease risk (176).
In cancer, treatment with OSCs-rich food showed promising results as chemopreventive.
A placebo double-blind randomized controlled trial on men scheduled for prostate biopsy and treated with broccoli sprout extract (BSE) supplementation (providing SFN and myrosinase) for 4.4 wk, performed by Zhang et. al., showed that BSE supplementation correlated with changes in gene expression but not with other prostate cancer immunohistochemistry biomarkers (173). In a double-blind placebo randomized clinical trial in patients with colorectal adenomas-precancerous lesions of the large bowel treated with aged garlic extract (AGE), Tanaka et al. demonstrated that AGE significantly reduced the size and number of colon adenomas in patients after 12 month (25). Several epidemiological studies showed that SFN consumption has been reported to be associated with a lower risk of cancer development (breast, lung, stomach, esophagus, mammary glands, gastric, colorectal, prostate, skin, head and neck, and liver) (172). In a large cohort study Millen et al. correlated the presence of adenoma with food intake of several fruit and vegetables, as assessed by a food-frequency questionnaire, and showed that onions and garlic were significantly related to lower risk of adenoma (177). Notably, a randomized double-blinded intervention study, performed by Traka et. al., showed that consuming GRA-rich broccoli for 12 months reduced the risk of prostate cancer progression (178). In particular, patients administrated with a weekly portion of soup made from a standard broccoli or 2 experimental broccoli genotypes with enhanced concentrations of GRA, showed dose-dependent attenuated activation of gene expression associated to oncogenic pathways in transperineal biopsies; and an inverse association between consumption of cruciferous vegetables and cancer progression was observed (178).
Overall, these studies highlighted the significant role of diet administration of OSCs in several chronic diseases and substantiate the relevance of creating specific dietary regimen for their prevention.
OSCs in the prevention of bone loss and skeletal frailty
A few clinical trials or population-based studies have revealed positive relationships between the consumption of vegetables, bone density, muscle strength and fractures in women/men, as summarized in Table 6.
Matheson et al. used a food frequency questionnaire added to the Nutritional Health and Nutrition Examination Survey (2003–2004) to examine the correlation between habitual consumption of onion over the past 12 months to BMD (N unweighted =507; N weighed =35.7 million). They found that higher consumption of onion increased the BMD by 5% (179). Law et al. administrated onion juice to healthy men and women and post-menopausal women for 8 weeks and investigated the association with bone BMD; the results found that the BMD of 3 postmenopausal women was mildly improved at the end of the treatment (85).
In an intriguing study, Blekkenhorst et al. used a food frequency questionnaire to examine the associations of vegetable and fruit intakes, separately, and specific types of vegetables and fruits with fracture-related hospitalizations in a prospective cohort of elderly women (mean age ≥ 70; n=1468); the authors found that the consumption of vegetable, but not fruit, is associated to a lower incidence of fracture; of note, the habitual consumption of cruciferous vegetables and Allium vegetables was significantly inversely associated with all fractures (18); importantly, these results were adjusted for energy intake and physical activity.
In musculoskeletal ageing, sarcopenia and declining physical activity are often associated with osteoporosis as the clinical hallmarks of frailty (180).
Interestingly, a prospective cohort study performed on elderly women (mean age ≥ 70; n=1429) investigated the correlation between vegetable consumption and incident falls-related hospitalization over a time-period of 14 years. The authors found that hospitalizations were lower in participants consuming more vegetables, but the consumption of cruciferous vegetables was most strongly associated with lower falls-related hospitalization (181) and was associated with increased muscle strength.
Finally, cross-sectional study, by Gu et. al., demonstrated a positive correlation between raw garlic consumption, assessed using a food frequency questionnaire, and handgrip strength in both males and females (182). The results were adjusted for age, body mass index, smoking status, alcohol-consumption status, education levels, employment status, household income, family history of diseases (cardiovascular disease, hypertension, hyperlipidemia, and diabetes), metabolic syndromes, physical activity, total energy intake, dietary pattern, onion intake. Although this study did not directly assess indexes of bone quantity, it supports an overall protective effect of OSCs-rich vegetables on the musculoskeletal system (181).
Perspectives and challenges
The present literature revision stems from the increasing appreciation of the link between dietary habits, and particularly the use of phytochemicals, and bone health. We show that a growing body of evidence supports a beneficial effect of dietary OSCs on skeletal health. Of note, although a few population-based studies offer interesting clues on the clinical relevance of OSCs-rich vegetables for the prevention of bone fragility (18, 85, 179, 183), no clinical studies have been performed yet to specifically address the potential protective role of OSCs against osteoporosis or bone fractures; this goal would require a study design including a controlled intake of OSCs-rich nutrients for long time-periods and/or the evaluation of purified OSCs molecules.
The ability of OSCs to work a as dietary source of the bioactive molecule H2S provide interesting future perspectives. OSCs-rich vegetables appear as the ideal candidate for clinical investigations on whether nutrients rich in sulfur can affect the pool of circulating reactive sulfur species (RSS), which include H2S; this may have a broad implication for the prevention of those pathologies, sometimes referred to as ‘H2S-poor diseases’, where the onset of the disease was associated to a lower systemic concentration of RSS compared to healthy controls. Increasing systemic RSS levels may also have important implication for bone-wasting diseases such as osteoporosis: indeed, animal studies have established that the bone loss associated to estrogen deficiency or to corticosteroid therapy is associated to a low systemic level of H2S (157, 159). However, these preclinical data still await confirmation in observational clinical studies in humans. To obtain reliable data on this topic, it will be critical to include in the study design a robust analytical methodology to quantitatively measure the different sulfur species in human serum or plasma since they may hold different importance in different pathologies (184, 185) and the high reactivity of these gaseous molecules implies a complex chemistry (186).
Further investigations may be addressed to the evaluation of the effect of these compounds on the gut-bone axis. OSCs show a considerable ability to modulate the gut microbiome and its secondary metabolites (187–190) and to mitigate the gut-based inflammatory response; given the paramount importance of metabolites and cytokines originated from the gut on the regulation on bone metabolism (191), it is conceivable that dietary OSCs may modulate the bone-bioactive components of the microbiota.
In the end, it is apparent that members of the OSCs family of phytochemicals affect bone homeostasis in several ways and may provide new insights into the potential bone health benefits of plant-derived food and leading to a more effective prevention of osteoporosis by non-pharmacological tools. This review may be useful to fuel clinical trials that may use a robust set of outcome measurements, aiming at assessing both bone quantity and bone quality before and after specific nutrition protocols; correlation between nutrients intake, H2S blood levels and bone status would help to define preventive/clinical dietary protocols for patients with an increased risk of bone fragility.
Author contributions
FG and LG contributed to the conception and design of the review. FG ad LG wrote the first draft of the manuscript. All authors contributed to manuscript revision, read, and approved the submitted version.
Conflict of interest
The authors declare that the research was conducted in the absence of any commercial or financial relationships that could be construed as a potential conflict of interest.
Publisher’s note
All claims expressed in this article are solely those of the authors and do not necessarily represent those of their affiliated organizations, or those of the publisher, the editors and the reviewers. Any product that may be evaluated in this article, or claim that may be made by its manufacturer, is not guaranteed or endorsed by the publisher.
Glossary
References
1. Clynes MA, Harvey NC, Curtis EM, Fuggle NR, Dennison EM, Cooper C. The epidemiology of osteoporosis. Br Med Bull (2020) 133(1):105–17. doi: 10.1093/bmb/ldaa005
2. Hernlund E, Svedbom A, Ivergard M, Compston J, Cooper C, Stenmark J, et al. Osteoporosis in the European union: medical management, epidemiology and economic burden. a report prepared in collaboration with the international osteoporosis foundation (IOF) and the European federation of pharmaceutical industry associations (EFPIA). Arch Osteoporos. (2013) 8:136. doi: 10.1007/s11657-013-0136-1
3. Kanis JA, Norton N, Harvey NC, Jacobson T, Johansson H, Lorentzon M, et al. SCOPE 2021: a new scorecard for osteoporosis in Europe. Arch Osteoporos. (2021) 16(1):82. doi: 10.1007/s11657-020-00871-9
4. Zaidi M. Skeletal remodeling in health and disease. Nat Med (2007) 13(7):791–801. doi: 10.1038/nm1593
5. Meunier P, Aaron J, Edouard C, Vignon G. Osteoporosis and the replacement of cell populations of the marrow by adipose tissue. a quantitative study of 84 iliac bone biopsies. Clin Orthop Relat Res (1971) 80:147–54. doi: 10.1097/00003086-197110000-00021
6. Verma S, Rajaratnam JH, Denton J, Hoyland JA, Byers RJ. Adipocytic proportion of bone marrow is inversely related to bone formation in osteoporosis. J Clin Pathol (2002) 55(9):693–8. doi: 10.1136/jcp.55.9.693
7. Infante A, Rodriguez CI. Osteogenesis and aging: lessons from mesenchymal stem cells. Stem Cell Res Ther (2018) 9(1):244. doi: 10.1186/s13287-018-0995-x
8. Papaioannou A, Kennedy CC, Dolovich L, Lau E, Adachi JD. Patient adherence to osteoporosis medications: problems, consequences and management strategies. Drugs Aging. (2007) 24(1):37–55. doi: 10.2165/00002512-200724010-00003
9. Siris ES, Harris ST, Rosen CJ, Barr CE, Arvesen JN, Abbott TA, et al. Adherence to bisphosphonate therapy and fracture rates in osteoporotic women: relationship to vertebral and nonvertebral fractures from 2 US claims databases. Mayo Clin Proc (2006) 81(8):1013–22. doi: 10.4065/81.8.1013
10. Willems HME, van den Heuvel E, Schoemaker RJW, Klein-Nulend J, Bakker AD. Diet and exercise: a match made in bone. Curr Osteoporos Rep (2017) 15(6):555–63. doi: 10.1007/s11914-017-0406-8
11. Karpouzos A, Diamantis E, Farmaki P, Savvanis S, Troupis T. Nutritional aspects of bone health and fracture healing. J Osteoporos. (2017) 2017:4218472. doi: 10.1155/2017/4218472
12. Munoz-Garach A, Garcia-Fontana B, Munoz-Torres M. Nutrients and dietary patterns related to osteoporosis. Nutrients (2020) 12(7):1986 . doi: 10.3390/nu12071986
13. Rizzoli R. Dairy products and bone health. Aging Clin Exp Res (2022) 34(1):9–24. doi: 10.1007/s40520-021-01970-4
14. Rizzoli R, Biver E, Brennan-Speranza TC. Nutritional intake and bone health. Lancet Diabetes Endocrinol (2021) 9(9):606–21. doi: 10.1016/S2213-8587(21)00119-4
15. Warensjo Lemming E, Byberg L. Is a healthy diet also suitable for the prevention of fragility fractures? Nutrients (2020) 12(9):2642. doi: 10.3390/nu12092642
16. Benetou V, Orfanos P, Feskanich D, Michaelsson K, Pettersson-Kymmer U, Byberg L, et al. Mediterranean Diet and hip fracture incidence among older adults: the CHANCES project. Osteoporos Int (2018) 29(7):1591–9. doi: 10.1007/s00198-018-4517-6
17. Benetou V, Orfanos P, Pettersson-Kymmer U, Bergstrom U, Svensson O, Johansson I, et al. Mediterranean Diet and incidence of hip fractures in a European cohort. Osteoporos Int (2013) 24(5):1587–98. doi: 10.1007/s00198-012-2187-3
18. Blekkenhorst LC, Hodgson JM, Lewis JR, Devine A, Woodman RJ, Lim WH, et al. Vegetable and Fruit Intake and Fracture-Related Hospitalisations: A Prospective Study of Older Women. (2017) 9(5):11. doi: 10.3390/nu9050511
19. Byberg L, Bellavia A, Orsini N, Wolk A, Michaelsson K. Fruit and vegetable intake and risk of hip fracture: a cohort study of Swedish men and women. J Bone Miner Res (2015) 30(6):976–84. doi: 10.1002/jbmr.2384
20. Tucker KL, Chen H, Hannan MT, Cupples LA, Wilson PW, Felson D, et al. Bone mineral density and dietary patterns in older adults: the framingham osteoporosis study. Am J Clin Nutr (2002) 76(1):245–52. doi: 10.1093/ajcn/76.1.245
21. de Haas SCM, de Jonge EAL, Voortman T, Graaff JS, Franco OH, Ikram MA, et al. Dietary patterns and changes in frailty status: the Rotterdam study. Eur J Nutr (2018) 57(7):2365–75. doi: 10.1007/s00394-017-1509-9
22. de Jonge EAL, Rivadeneira F, Erler NS, Hofman A, Uitterlinden AG, Franco OH, et al. Dietary patterns in an elderly population and their relation with bone mineral density: the Rotterdam study. Eur J Nutr (2018) 57(1):61–73. doi: 10.1007/s00394-016-1297-7
23. Somani SJ, Modi KP, Majumdar AS, Sadarani BN. Phytochemicals and their potential usefulness in inflammatory bowel disease. Phytother Res (2015) 29(3):339–50. doi: 10.1002/ptr.5271
24. Mathew BC, Prasad NV, Prabodh R. Cholesterol-lowering effect of organosulphur compounds from garlic: a possible mechanism of action. (KUMJ) (2004) 2(2):100–2.
25. Tanaka S, Haruma K, Yoshihara M, Kajiyama G, Kira K, Amagase H, et al. Aged garlic extract has potential suppressive effect on colorectal adenomas in humans. J Nutr (2006) 136(3 Suppl):821S–6S. doi: 10.1093/jn/136.3.821S
26. Panjwani AA, Liu H, Fahey JW. Crucifers and related vegetables and supplements for neurologic disorders: what is the evidence? Curr Opin Clin Nutr Metab Care (2018) 21(6):451–7. doi: 10.1097/MCO.0000000000000511
27. Del Chierico F, Vernocchi P, Dallapiccola B, Putignani L. Mediterranean Diet and health: food effects on gut microbiota and disease control. Int J Mol Sci (2014) 15(7):11678–99. doi: 10.3390/ijms150711678
28. Dixit V Jr Iii, Kumar I, Palandurkar K, Giri R, Giri K. Lepidium sativum: Bone healer in traditional medicine, an experimental validation study in rats. J Family Med Prim Care (2020) 9(2):812–8. doi: 10.4103/jfmpc.jfmpc_761_19
29. Ramirez D, Abellan-Victorio A, Beretta V, Camargo A, Moreno DA. Functional ingredients from brassicaceae species: Overview and perspectives. Int J Mol Sci (2020) 21(6):1998. doi: 10.3390/ijms21061998
30. Iberl B, Winkler G, Muller B, Knobloch K. Quantitative determination of allicin and alliin from garlic by HPLC*. Planta Med (1990) 56(3):320–6. doi: 10.1055/s-2006-960969
31. Bose S, Laha B, Banerjee S. Quantification of allicin by high performance liquid chromatography-ultraviolet analysis with effect of post-ultrasonic sound and microwave radiation on fresh garlic cloves. Pharmacogn Mag. (2014) 10(Suppl 2):S288–93. doi: 10.4103/0973-1296.133279
32. Iberl B, Winkler G, Knobloch K. Products of allicin transformation: Ajoenes and dithiins, characterization and their determination by HPLC*. Planta Med (1990) 56(2):202–11. doi: 10.1055/s-2006-960926
33. Egen-Schwind C, Eckard R, Jekat FW, Winterhoff H. Pharmacokinetics of vinyldithiins, transformation products of allicin. Planta Med (1992) 58(1):8–13. doi: 10.1055/s-2006-961379
34. Lawson LD, Wang ZJ, Hughes BG. Identification and HPLC quantitation of the sulfides and dialk(en)yl thiosulfinates in commercial garlic products. Planta Med (1991) 57(4):363–70. doi: 10.1055/s-2006-960119
35. Rabinkov A, Miron T, Mirelman D, Wilchek M, Glozman S, Yavin E, et al. S-allylmercaptoglutathione: the reaction product of allicin with glutathione possesses SH-modifying and antioxidant properties. Biochim Biophys Acta (2000) 1499(1-2):144–53. doi: 10.1016/S0167-4889(00)00119-1
36. Bastaki SMA, Ojha S, Kalasz H, Adeghate E. Chemical constituents and medicinal properties of allium species. Mol Cell Biochem (2021) 476(12):4301–21. doi: 10.1007/s11010-021-04213-2
37. Iciek M, Kwiecien I, Wlodek L. Biological properties of garlic and garlic-derived organosulfur compounds. Environ Mol Mutagen (2009) 50(3):247–65. doi: 10.1002/em.20474
38. Rauf A, Abu-Izneid T, Thiruvengadam M, Imran M, Olatunde A, Shariati MA, et al. Garlic (Allium sativum l.): Its chemistry, nutritional composition, toxicity and anticancer properties. Curr Top Med Chem (2021) 22(11):957–72. doi: 10.2174/1568026621666211105094939
39. Cascajosa-Lira A, Prieto Ortega AI, Guzman-Guillen R, Catunescu GM, de la Torre JM, Guillamon E, et al. Simultaneous determination of allium compounds (Propyl propane thiosulfonate and thiosulfinate) in animal feed using UPLC-MS/MS. Food Chem Toxicol (2021) 157:112619. doi: 10.1016/j.fct.2021.112619
40. Kim S, Lee S, Shin D, Yoo M. Change in organosulfur compounds in onion (Allium cepa l.) during heat treatment. Food Sci Biotechnol (2016) 25(1):115–9. doi: 10.1007/s10068-016-0017-7
41. Wetli HA, Brenneisen R, Tschudi I, Langos M, Bigler P, Sprang T, et al. A gamma-glutamyl peptide isolated from onion (Allium cepa l.) by bioassay-guided fractionation inhibits resorption activity of osteoclasts. J Agric Food Chem (2005) 53(9):3408–14. doi: 10.1021/jf040457i
42. Nohara T, Fujiwara Y, Kudo R, Yamaguchi K, Ikeda T, Murakami K, et al. Isolation and characterization of new onionins A2 and A3 from allium cepa, and of onionins A1, A2, and A3 from allium fistulosum. Chem Pharm Bull (Tokyo). (2014) 62(11):1141–5. doi: 10.1248/cpb.c14-00461
43. Ueda Y, Tsubuku T, Miyajima R. Composition of sulfur-containing components in onion and their flavor characters. Biosci Biotechnol Biochem (1994) 58(1):108–10. doi: 10.1271/bbb.58.108
44. Wang H, Li J, Wang Z, Zhang X, Ni Y. Modified method for rapid quantitation of s-alk(en)yl-L-cysteine sulfoxide in yellow onions (Allium cepa l.). J Agric Food Chem (2007) 55(14):5429–35. doi: 10.1021/jf070298d
45. Fernandes S, Gois A, Mendes F, Perestrelo R, Medina S, Camara JS. Typicality assessment of onions (Allium cepa) from different geographical regions based on the volatile signature and chemometric tools. Foods (2020) 9(3):375. doi: 10.3390/foods9030375
46. Tigu AB, Moldovan CS, Toma VA, Farcas AD, Mot AC, Jurj A, et al. Phytochemical analysis and In vitro effects of allium fistulosum l. and allium sativum l. extracts on human normal and tumor cell lines: A comparative study. Molecules (2021) 26(3):574. doi: 10.3390/molecules26030574
47. Yang HJ, Kim MJ, Qiu JY, Zhang T, Wu X, Jang DJ, et al. Rice porridge containing welsh onion root water extract alleviates osteoarthritis-related pain behaviors, glucose levels, and bone metabolism in osteoarthritis-induced ovariectomized rats. Nutrients (2019) 11(7):1503. doi: 10.3390/nu11071503
48. Park H, Jeong J, Hyun H, Kim J, Kim H, Oh HI, et al. Effects of a hot-water extract of allium hookeri roots on bone formation in human osteoblast-like MG-63 cells In vitro and in rats in vivo. Planta Med (2016) 82(16):1410–5. doi: 10.1055/s-0042-108733
49. Kim S, Kim DB, Lee S, Park J, Shin D, Yoo M. Profiling of organosulphur compounds using HPLC-PDA and GC/MS system and antioxidant activities in hooker chive (Allium hookeri). Nat Prod Res (2016) 30(24):2798–804. doi: 10.1080/14786419.2016.1164700
50. Jang JY, Lee MJ, You BR, Jin JS, Lee SH, Yun YR, et al. Allium hookeri root extract exerts anti-inflammatory effects by nuclear factor-kappaB down-regulation in lipopolysaccharide-induced RAW264.7 cells. BMC Complement Altern Med (2017) 17(1):126. doi: 10.1186/s12906-017-1633-3
51. Kim HJ, Lee SH, Lee SH, Lee J, Kim H, Chang GT, et al. Longitudinal bone growth stimulating effect of allium macrostemon in adolescent female rats. Molecules (2020) 25(22):5449. doi: 10.3390/molecules25225449
52. Bernaert N, Goetghebeur L, De Clercq H, De Loose M, Daeseleire E, Van Pamel E, et al. Influence of cultivar and harvest time on the amounts of isoalliin and methiin in leek (Allium ampeloprasum var. porrum). J Agric Food Chem (2012) 60(44):10910–9. doi: 10.1021/jf302132a
53. Kubec R, Dadakova E. Chromatographic methods for determination of s-substituted cysteine derivatives–a comparative study. J Chromatogr A. (2009) 1216(41):6957–63. doi: 10.1016/j.chroma.2009.08.032
54. Jeong J, Park H, Hyun H, Kim J, Kim H, Oh HI, et al. Effects of glucosinolates from turnip (Brassica rapa l.) root on bone formation by human osteoblast-like MG-63 cells and in normal young rats. Phytother Res (2015) 29(6):902–9. doi: 10.1002/ptr.5331
55. Barbieri GPR, Maggio A, De Pascale S, Fogliano V. Glucosinolates profile of brassica rapa l. subsp. sylvestris l. janch. var. esculenta hort. Food Chem (2008) 107(4):1687–91. doi: 10.1016/j.foodchem.2007.09.054
56. Cramer JM, Teran-Garcia M, Jeffery EH. Enhancing sulforaphane absorption and excretion in healthy men through the combined consumption of fresh broccoli sprouts and a glucoraphanin-rich powder. Br J Nutr (2012) 107(9):1333–8. doi: 10.1017/S0007114511004429
57. Liang H, Li C, Yuan Q, Vriesekoop F. Separation and purification of sulforaphane from broccoli seeds by solid phase extraction and preparative high-performance liquid chromatography. J Agric Food Chem (2007) 55(20):8047–53. doi: 10.1021/jf0706833
58. Hashem FA, Motawea H, El-Shabrawy AE, Shaker K, El-Sherbini S. Myrosinase hydrolysates of brassica oleraceae l. var. italica reduce the risk of colon cancer. Phytother Res (2012) 26(5):743–7. doi: 10.1002/ptr.3591
59. Abdallah HM, Farag MA, Algandaby MM, Nasrullah MZ, Abdel-Naim AB, Eid BG, et al. Osteoprotective activity and metabolite fingerprint via UPLC/MS and GC/MS of lepidium sativum in ovariectomized rats. Nutrients (2020) 12(7):2075. doi: 10.3390/nu12072075
60. Robin AH, Yi GE, Laila R, Yang K, Park JI, Kim HR, et al. Expression profiling of glucosinolate biosynthetic genes in brassica oleracea l. var. capitata inbred lines reveals their association with glucosinolate content. Molecules (2016) 21(6):787. doi: 10.3390/molecules21060787
61. Soengas P, Velasco P, Fernandez JC, Cartea ME. New vegetable brassica foods: A promising source of bioactive compounds. Foods (2021) 10(12):2911. doi: 10.3390/foods10122911
62. Bell L, Oruna-Concha MJ, Wagstaff C. Identification and quantification of glucosinolate and flavonol compounds in rocket salad (Eruca sativa, eruca vesicaria and diplotaxis tenuifolia) by LC-MS: highlighting the potential for improving nutritional value of rocket crops. Food Chem (2015) 172:852–61. doi: 10.1016/j.foodchem.2014.09.116
63. Gerendas J, Breuning S, Stahl T, Mersch-Sundermann V, Muhling KH. Isothiocyanate concentration in kohlrabi (Brassica oleracea l. var. gongylodes) plants as influenced by sulfur and nitrogen supply. J Agric Food Chem (2008) 56(18):8334–42. doi: 10.1021/jf800399x
64. Marcinkowska M, Frank S, Steinhaus M, Jelen HH. Key odorants of raw and cooked green kohlrabi (Brassica oleracea var. gongylodes l.). J Agric Food Chem (2021) 69(41):12270–7. doi: 10.1021/acs.jafc.1c04339
65. Manivannan A, Kim JH, Kim DS, Lee ES, Lee HE. Deciphering the nutraceutical potential of raphanus sativus-a comprehensive overview. Nutrients (2019) 11(2):402. doi: 10.3390/nu11020402
66. Giorgetti L, Giorgi G, Cherubini E, Gervasi PG, Della Croce CM, Longo V, et al. Screening and identification of major phytochemical compounds in seeds, sprouts and leaves of Tuscan black kale brassica oleracea (L.) ssp acephala (DC) var. sabellica l. Nat Prod Res (2018) 32(14):1617–26. doi: 10.1080/14786419.2017.1392953
67. Liu Z, Hirani AH, McVetty PB, Daayf F, Quiros CF, Li G. Reducing progoitrin and enriching glucoraphanin in brassica napus seeds through silencing of the GSL-ALK gene family. Plant Mol Biol (2012) 79(1-2):179–89. doi: 10.1007/s11103-012-9905-2
68. Ku KM, Kim MJ, Jeffery EH, Kang YH, Juvik JA. Profiles of glucosinolates, their hydrolysis products, and quinone reductase inducing activity from 39 arugula (Eruca sativa mill.) accessions. J Agric Food Chem (2016) 64(34):6524–32. doi: 10.1021/acs.jafc.6b02750
69. Rektorisova M, Hrbek V, Jiru M, Ovesna J, Hajslova J. Variability in s-Alk(en)yl-L-Cysteine sulfoxides in garlic within a seven-month period determined by a liquid chromatography - tandem mass spectrometry method. Plant Foods Hum Nutr (2020) 75(3):376–82. doi: 10.1007/s11130-020-00817-z
70. Rose P, Whiteman M, Moore PK, Zhu YZ. Bioactive s-alk(en)yl cysteine sulfoxide metabolites in the genus allium: the chemistry of potential therapeutic agents. Nat Prod Rep (2005) 22(3):351–68. doi: 10.1039/b417639c
71. Kodera Y, Kurita M, Nakamoto M, Matsutomo T. Chemistry of aged garlic: Diversity of constituents in aged garlic extract and their production mechanisms via the combination of chemical and enzymatic reactions. Exp Ther Med (2020) 19(2):1574–84. doi: 10.3892/etm.2019.8393
72. Yoshimoto N, Saito K. S-alk(en)ylcysteine sulfoxides in the genus allium: proposed biosynthesis, chemical conversion, and bioactivities. J Exp Bot (2019) 70(16):4123–37. doi: 10.1093/jxb/erz243
73. Block E. Recent results in the organosulfur and organoselenium chemistry of genus allium and brassica plants. relevance for cancer prevention. Adv Exp Med Biol (1996) 401:155–69. doi: 10.1007/978-1-4613-0399-2_13
74. Calvey EM, White KD, Matusik JE, Sha D, Block E. Allium chemistry: identification of organosulfur compounds in ramp (Allium tricoccum) homogenates. Phytochemistry (1998) 49(2):359–64. doi: 10.1016/S0031-9422(98)00191-5
75. Prieto MA, Lopez CJ, Simal-Gandara J. Glucosinolates: Molecular structure, breakdown, genetic, bioavailability, properties and healthy and adverse effects. Adv Food Nutr Res (2019) 90:305–50. doi: 10.1016/bs.afnr.2019.02.008
76. Shekarri Q, Dekker M. A physiological-based model for simulating the bioavailability and kinetics of sulforaphane from broccoli products. Foods (2021) 10(11):2761. doi: 10.3390/foods10112761
77. Angelino DJ E. Glucosinolate hydrolysis and bioavailability of resulting isothiocyanates: Focus on glucoraphanin. J Funct Foods (2014) 7:67–76. doi: 10.1016/j.jff.2013.09.029
78. Shapiro TA, Fahey JW, Wade KL, Stephenson KK, Talalay P. Human metabolism and excretion of cancer chemoprotective glucosinolates and isothiocyanates of cruciferous vegetables. Cancer Epidemiol Biomarkers Prev (1998) 7(12):1091–100.
79. Datta HK, Ng WF, Walker JA, Tuck SP, Varanasi SS. The cell biology of bone metabolism. J Clin Pathol (2008) 61(5):577–87. doi: 10.1136/jcp.2007.048868
80. Ryuk JA. Effect of allium fistulosum extracts on the stimulation of longitudinal bone growth in animal modeling diet-induced calcium and vitamin d deficiencies. Appl Sci (2021) 11(17):7786. doi: 10.3390/app11177786
81. Tang CH, Huang TH, Chang CS, Fu WM, Yang RS. Water solution of onion crude powder inhibits RANKL-induced osteoclastogenesis through ERK, p38 and NF-kappaB pathways. Osteoporos Int (2009) 20(1):93–103. doi: 10.1007/s00198-008-0630-2
82. Bose S, Banerjee D, Vu AA. Ginger and garlic extracts enhance osteogenesis in 3D printed calcium phosphate bone scaffolds with bimodal pore distribution. ACS Appl Mater Interfaces (2022) 4(11):12964–75. doi: 10.1021/acsami.1c19617
83. Behera J, Ison J, Rai H, Tyagi N. Allyl sulfide promotes osteoblast differentiation and bone density via reducing mitochondrial DNA release mediated Kdm6b/H3K27me3 epigenetic mechanism. Biochem Biophys Res Commun (2021) 543:87–94. doi: 10.1016/j.bbrc.2021.01.016
84. Monarrez-Cordero BE, Rodriguez-Gonzalez CA, Valencia-Gomez LE, Hernandez-Paz JF, Martel-Estrada SA, Camacho-Montes H, et al. The effect of allium cepa extract on the chitosan/PLGA scaffolds bioactivity. J Appl Biomater Funct Mater (2021) 19:2280800021989701. doi: 10.1177/2280800021989701
85. Law YY, Chiu HF, Lee HH, Shen YC, Venkatakrishnan K, Wang CK. Consumption of onion juice modulates oxidative stress and attenuates the risk of bone disorders in middle-aged and post-menopausal healthy subjects. Food Funct (2016) 7(2):902–12. doi: 10.1039/C5FO01251A
86. Yang J, Tang R, Yi J, Chen Y, Li X, Yu T, et al. Diallyl disulfide alleviates inflammatory osteolysis by suppressing osteoclastogenesis via NF-kappaB-NFATc1 signal pathway. FASEB J (2019) 33(6):7261–73. doi: 10.1096/fj.201802172R
87. Chen Y, Sun J, Dou C, Li N, Kang F, Wang Y, et al. Alliin attenuated RANKL-induced osteoclastogenesis by scavenging reactive oxygen species through inhibiting Nox1. Int J Mol Sci (2016) 17(9):1516. doi: 10.3390/ijms17091516
88. Weisstaub AR, Salinas MV, Correa MJ, Barchuk M, Berg G, Zuleta A. Effects of the intake of white wheat bread added with garlic and resistant starch: action on calcium bioavailability and metabolic parameters of growing wistar rats. Food Funct (2018) 9(11):5707–14. doi: 10.1039/C8FO01407H
89. El-Sayyad HI, Abou-El-Naga AM, Gadallah AA, Bakr IH. Protective effects of allium sativum against defects of hypercholesterolemia on pregnant rats and their offspring. Int J Clin Exp Med (2010) 3(2):152–63.
90. Mukherjee M, Das AS, Mitra S, Mitra C. Prevention of bone loss by oil extract of garlic (Allium sativum linn.) in an ovariectomized rat model of osteoporosis. Phytother Res (2004) 18(5):389–94. doi: 10.1002/ptr.1448
91. Mukherjee M, Das AS, Das D, Mukherjee S, Mitra S, Mitra C. Effects of garlic oil on postmenopausal osteoporosis using ovariectomized rats: comparison with the effects of lovastatin and 17beta-estradiol. Phytother Res (2006) 20(1):21–7. doi: 10.1002/ptr.1795
92. Mukherjee M, Das AS, Das D, Mukherjee S, Mitra S, Mitra C. Role of oil extract of garlic (Allium sativum linn.) on intestinal transference of calcium and its possible correlation with preservation of skeletal health in an ovariectomized rat model of osteoporosis. Phytother Res (2006) 20(5):408–15. doi: 10.1002/ptr.1888
93. Mukherjee M, Das AS, Das D, Mukherjee S, Mitra S, Mitra C. Role of peritoneal macrophages and lymphocytes in the development of hypogonadal osteoporosis in an ovariectomized rat model: possible phytoestrogenic efficacy of oil extract of garlic to preserve skeletal health. Phytother Res (2007) 21(11):1045–54. doi: 10.1002/ptr.2209
94. Huang TH, Muhlbauer RC, Tang CH, Chen HI, Chang GL, Huang YW, et al. Onion decreases the ovariectomy-induced osteopenia in young adult rats. Bone (2008) 42(6):1154–63. doi: 10.1016/j.bone.2008.01.032
95. Li D, Liang H, Li Y, Zhang J, Qiao L, Luo H. Allicin alleviates lead-induced bone loss by preventing oxidative stress and osteoclastogenesis Via SIRT1/FOXO1 pathway in mice. Biol Trace Elem Res (2021) 199(1):237–43. doi: 10.1007/s12011-020-02136-5
96. Liu Y, You M, Shen J, Xu Y, Li L, Wang D, et al. Allicin reversed the process of frailty in aging Male Fischer 344 rats with osteoporosis. J Gerontol A Biol Sci Med Sci (2020) 75(5):821–5. doi: 10.1093/gerona/glz205
97. Thaler R, Maurizi A, Roschger P, Sturmlechner I, Khani F, Spitzer S, et al. Anabolic and antiresorptive modulation of bone homeostasis by the epigenetic modulator sulforaphane, a naturally occurring isothiocyanate. J Biol Chem (2016) 291(13):6754–71. doi: 10.1074/jbc.M115.678235
98. Gambari L, Barone M, Amore E, Grigolo B, Filardo G, Iori R, et al. Glucoraphanin increases intracellular hydrogen sulfide (H2S) levels and stimulates osteogenic differentiation in human mesenchymal stromal cell. Nutrients (2022) 14(3):435. doi: 10.3390/nu14030435
99. Kang IS, Agidigbi TS, Kwon YM, Kim DG, Kim RI, In G, et al. Effect of Co-administration of panax ginseng and brassica oleracea on postmenopausal osteoporosis in ovariectomized mice. Nutrients (2020) 12(8):2415. doi: 10.3390/nu12082415
100. Xue P, Hu X, Powers J, Nay N, Chang E, Kwon J, et al. CDDO-me, sulforaphane and tBHQ attenuate the RANKL-induced osteoclast differentiation via activating the NRF2-mediated antioxidant response. Biochem Biophys Res Commun (2019) 511(3):637–43. doi: 10.1016/j.bbrc.2019.02.095
101. Takagi T, Inoue H, Takahashi N, Katsumata-Tsuboi R, Uehara M. Sulforaphane inhibits osteoclast differentiation by suppressing the cell-cell fusion molecules DC-STAMP and OC-STAMP. Biochem Biophys Res Commun (2017) 483(1):718–24. doi: 10.1016/j.bbrc.2016.12.075
102. Kim SJ, Kang SY, Shin HH, Choi HS. Sulforaphane inhibits osteoclastogenesis by inhibiting nuclear factor-kappaB. Mol Cells (2005) 20(3):364–70.
103. Luo T, Fu X, Liu Y, Ji Y, Shang Z. Sulforaphane inhibits osteoclastogenesis via suppression of the autophagic pathway. Molecules (2021) 26(2):34. doi: 10.3390/molecules26020347
104. Gambari L, Lisignoli G, Cattini L, Manferdini C, Facchini A, Grassi F. Sodium hydrosulfide inhibits the differentiation of osteoclast progenitor cells via NRF2-dependent mechanism. Pharmacol Res (2014) 87:99–112. doi: 10.1016/j.phrs.2014.06.014
105. Javaheri B, Poulet B, Aljazzar A, de Souza R, Piles M, Hopkinson M, et al. Stable sulforaphane protects against gait anomalies and modifies bone microarchitecture in the spontaneous STR/Ort model of osteoarthritis. Bone (2017) 103:308–17. doi: 10.1016/j.bone.2017.07.028
106. Elshal MF, Almalki AL, Hussein HK, Khan JA. Synergistic antiosteoporotic effect of lepidium sativum and alendronate in glucocorticoid-induced osteoporosis in wistar rats. Afr J Tradit Complement Altern Med (2013) 10(5):267–73. doi: 10.4314/ajtcam.v10i5.8
107. El-Haroun H. Comparative study on the possible protective effect of lepidium sativum versus teriparatide in induced osteoporosis in adult Male Guinea pigs. Egiptian J Hystol (2020) 43(3):931–47. doi: 10.21608/ejh.2020.18855.1193
108. Zhang Y, Yu L, Ao M, Jin W. Effect of ethanol extract of lepidium meyenii walp. on osteoporosis in ovariectomized rat. J Ethnopharmacol (2006) 105(1-2):274–9. doi: 10.1016/j.jep.2005.12.013
109. Gorinstein S, Leontowicz H, Leontowicz M, Namiesnik J, Najman K, Drzewiecki J, et al. Comparison of the main bioactive compounds and antioxidant activities in garlic and white and red onions after treatment protocols. J Agric Food Chem (2008) 56(12):4418–26. doi: 10.1021/jf800038h
110. Kothari D, Lee WD, Niu KM, Kim SK. The genus allium as poultry feed additive: A review. Anim (Basel). (2019) 9(12):1032. doi: 10.3390/ani9121032
111. Sobolewska D, Michalska K, Podolak I, Grabowska K. Steroidal saponins from the genus allium. Phytochem Rev (2016) 15:1–35. doi: 10.1007/s11101-014-9381-1
112. Raiola A, Errico A, Petruk G, Monti DM, Barone A, Rigano MM. Bioactive compounds in brassicaceae vegetables with a role in the prevention of chronic diseases. Molecules (2017) 23(1):15. doi: 10.3390/molecules23010015
113. Darvin P, Joung YH, Yang YM. JAK2-STAT5B pathway and osteoblast differentiation. JAKSTAT (2013) 2(4):e24931. doi: 10.4161/jkst.24931
114. Lin GL, Hankenson KD. Integration of BMP, wnt, and notch signaling pathways in osteoblast differentiation. J Cell Biochem (2011) 112(12):3491–501. doi: 10.1002/jcb.23287
115. Kang S, Bennett CN, Gerin I, Rapp LA, Hankenson KD, Macdougald OA. Wnt signaling stimulates osteoblastogenesis of mesenchymal precursors by suppressing CCAAT/enhancer-binding protein alpha and peroxisome proliferator-activated receptor gamma. J Biol Chem (2007) 282(19):14515–24. doi: 10.1074/jbc.M700030200
116. Jeon MJ, Kim JA, Kwon SH, Kim SW, Park KS, Park SW, et al. Activation of peroxisome proliferator-activated receptor-gamma inhibits the Runx2-mediated transcription of osteocalcin in osteoblasts. J Biol Chem (2003) 278(26):23270–7. doi: 10.1074/jbc.M211610200
117. Wang M, Jin H, Tang D, Huang S, Zuscik MJ, Chen D. Smad1 plays an essential role in bone development and postnatal bone formation. Osteoarthr Cartil (2011) 19(6):751–62. doi: 10.1016/j.joca.2011.03.004
118. Meyers CA, Xu J, Asatrian G, Ding C, Shen J, Broderick K, et al. WISP-1 drives bone formation at the expense of fat formation in human perivascular stem cells. Sci Rep (2018) 8(1):15618. doi: 10.1038/s41598-018-34143-x
119. Gambari L, Lisignoli G, Gabusi E, Manferdini C, Paolella F, Piacentini A, et al. Distinctive expression pattern of cystathionine-beta-synthase and cystathionine-gamma-lyase identifies mesenchymal stromal cells transition to mineralizing osteoblasts. J Cell Physiol (2017) 232(12):3574–85. doi: 10.1002/jcp.25825
120. Robling AG, Bonewald LF. The osteocyte: New insights. Annu Rev Physiol (2020) 82:485–506. doi: 10.1146/annurev-physiol-021119-034332
121. Kitaura H, Marahleh A, Ohori F, Noguchi T, Shen WR, Qi J, et al. Osteocyte-related cytokines regulate osteoclast formation and bone resorption. Int J Mol Sci (2020) 21(14):5169. doi: 10.3390/ijms21145169
122. Pautke C, Schieker M, Tischer T, Kolk A, Neth P, Mutschler W, et al. Characterization of osteosarcoma cell lines MG-63, saos-2 and U-2 OS in comparison to human osteoblasts. Anticancer Res (2004) 24(6):3743–8.
123. Juma A. The effects of lepidium sativum seeds on fracture-induced healing in rabbits. MedGenMed (2007) 9(2):23. doi: 10.1016/j.jep.2005.12.013
124. Boyce BF. Advances in the regulation of osteoclasts and osteoclast functions. J Dent Res (2013) 92(10):860–7. doi: 10.1177/0022034513500306
125. Kim JM, Lin C, Stavre Z, Greenblatt MB, Shim JH. Osteoblast-osteoclast communication and bone homeostasis. Cells (2020) 9(9). doi: 10.3390/cells9092073
126. Gambari L, Grassi F, Roseti L, Grigolo B, Desando G. Learning from monocyte-macrophage fusion and multinucleation: Potential therapeutic targets for osteoporosis and rheumatoid arthritis. Int J Mol Sci (2020) 21(17):6001. doi: 10.3390/ijms21176001
127. Kim JH, Kim N. Regulation of NFATc1 in osteoclast differentiation. J Bone Metab (2014) 21(4):233–41. doi: 10.11005/jbm.2014.21.4.233
128. Lee NK, Choi YG, Baik JY, Han SY, Jeong DW, Bae YS, et al. A crucial role for reactive oxygen species in RANKL-induced osteoclast differentiation. Blood (2005) 106(3):852–9. doi: 10.1182/blood-2004-09-3662
129. Youn MY, Takada I, Imai Y, Yasuda H, Kato S. Transcriptionally active nuclei are selective in mature multinucleated osteoclasts. Genes Cells (2010) 15(10):1025–35. doi: 10.1111/j.1365-2443.2010.01441.x
130. Costa AG, Cusano NE, Silva BC, Cremers S, Bilezikian JP. Cathepsin K: its skeletal actions and role as a therapeutic target in osteoporosis. Nat Rev Rheumatol (2011) 7(8):447–56. doi: 10.1038/nrrheum.2011.77
131. Miyamoto K, Ninomiya K, Sonoda KH, Miyauchi Y, Hoshi H, Iwasaki R, et al. MCP-1 expressed by osteoclasts stimulates osteoclastogenesis in an autocrine/paracrine manner. Biochem Biophys Res Commun (2009) 383(3):373–7. doi: 10.1016/j.bbrc.2009.04.020
132. Witwicka H, Hwang SY, Reyes-Gutierrez P, Jia H, Odgren PE, Donahue LR, et al. Studies of OC-STAMP in osteoclast fusion: A new knockout mouse model, rescue of cell fusion, and transmembrane topology. PloS One (2015) 10(6):e0128275. doi: 10.1371/journal.pone.0128275
133. Kensler TW, Wakabayashi N, Biswal S. Cell survival responses to environmental stresses via the Keap1-Nrf2-ARE pathway. Annu Rev Pharmacol Toxicol (2007) 47:89–116. doi: 10.1146/annurev.pharmtox.46.120604.141046
134. Kanzaki H, Shinohara F, Kajiya M, Kodama T. The Keap1/Nrf2 protein axis plays a role in osteoclast differentiation by regulating intracellular reactive oxygen species signaling. J Biol Chem (2013) 288(32):23009–20. doi: 10.1074/jbc.M113.478545
135. Dinkova-Kostova AT, Holtzclaw WD, Cole RN, Itoh K, Wakabayashi N, Katoh Y, et al. Direct evidence that sulfhydryl groups of Keap1 are the sensors regulating induction of phase 2 enzymes that protect against carcinogens and oxidants. Proc Natl Acad Sci U S A. (2002) 99(18):11908–13. doi: 10.1073/pnas.172398899
136. Bhattacharjee S, Dashwood RH. Epigenetic regulation of NRF2/KEAP1 by phytochemicals. Antioxid (Basel). (2020) 9(9):865. doi: 10.3390/antiox9090865
137. Yang H, Qin J, Wang X, Ei-Shora HM, Yu B. Production of plant-derived anticancer precursor glucoraphanin in chromosomally engineered escherichia coli. Microbiol Res (2020) 238:126484. doi: 10.1016/j.micres.2020.126484
138. Martelli A, Piragine E, Citi V, Testai L, Pagnotta E, Ugolini L, et al. Erucin exhibits vasorelaxing effects and antihypertensive activity by H2 s-releasing properties. Br J Pharmacol (2020) 177(4):824–35. doi: 10.1111/bph.14645
139. Yang G, Wu L, Jiang B, Yang W, Qi J, Cao K, et al. H2S as a physiologic vasorelaxant: hypertension in mice with deletion of cystathionine gamma-lyase. Science (2008) 322(5901):587–90. doi: 10.1126/science.1162667
140. Di Cesare Mannelli L, Lucarini E, Micheli L, Mosca I, Ambrosino P, Soldovieri MV, et al. Effects of natural and synthetic isothiocyanate-based H2S-releasers against chemotherapy-induced neuropathic pain: Role of Kv7 potassium channels. Neuropharmacology (2017) 121:49–59. doi: 10.1016/j.neuropharm.2017.04.029
141. Kida K, Marutani E, Nguyen RK, Ichinose F. Inhaled hydrogen sulfide prevents neuropathic pain after peripheral nerve injury in mice. Nitric Oxide (2015) 46:87–92. doi: 10.1016/j.niox.2014.11.014
142. Xue R, Hao DD, Sun JP, Li WW, Zhao MM, Li XH, et al. Hydrogen sulfide treatment promotes glucose uptake by increasing insulin receptor sensitivity and ameliorates kidney lesions in type 2 diabetes. Antioxid Redox Signal (2013) 19(1):5–23. doi: 10.1089/ars.2012.5024
143. Giovinazzo D, Bursac B, Sbodio JI, Nalluru S, Vignane T, Snowman AM, et al. Hydrogen sulfide is neuroprotective in alzheimer's disease by sulfhydrating GSK3beta and inhibiting tau hyperphosphorylation. Proc Natl Acad Sci U.S.A. (2021) 118(4):e2017225118. doi: 10.1073/pnas.2017225118
144. Hine C, Harputlugil E, Zhang Y, Ruckenstuhl C, Lee BC, Brace L, et al. Endogenous hydrogen sulfide production is essential for dietary restriction benefits. Cell (2015) 160(1-2):132–44. doi: 10.1016/j.cell.2014.11.048
145. Hine C, Mitchell JR. Calorie restriction and methionine restriction in control of endogenous hydrogen sulfide production by the transsulfuration pathway. Exp Gerontol. (2015) 68:26–32. doi: 10.1016/j.exger.2014.12.010
146. Whiteman M, Winyard PG. Hydrogen sulfide and inflammation: the good, the bad, the ugly and the promising. Expert Rev Clin Pharmacol (2011) 4(1):13–32. doi: 10.1586/ecp.10.134
147. Benavides GA, Squadrito GL, Mills RW, Patel HD, Isbell TS, Patel RP, et al. Hydrogen sulfide mediates the vasoactivity of garlic. Proc Natl Acad Sci U S A. (2007) 104(46):17977–82. doi: 10.1073/pnas.0705710104
148. Liang D, Wu H, Wong MW, Huang D. Diallyl trisulfide is a fast H2S donor, but diallyl disulfide is a slow one: The reaction pathways and intermediates of glutathione with polysulfides. Org Lett (2015) 17(17):4196–9. doi: 10.1021/acs.orglett.5b01962
149. Citi V, Martelli A, Testai L, Marino A, Breschi MC, Calderone V. Hydrogen sulfide releasing capacity of natural isothiocyanates: is it a reliable explanation for the multiple biological effects of brassicaceae? Planta Med (2014) 80(8-9):610–3. doi: 10.1055/s-0034-1368591
150. Lucarini E, Micheli L, Trallori E, Citi V, Martelli A, Testai L, et al. Effect of glucoraphanin and sulforaphane against chemotherapy-induced neuropathic pain: Kv7 potassium channels modulation by H2 s release in vivo. Phytother Res (2018) 32(11):2226–34. doi: 10.1002/ptr.6159
151. Lucarini E, Pagnotta E, Micheli L, Parisio C, Testai L, Martelli A, et al. Eruca sativa meal against diabetic neuropathic pain: An H2S-mediated effect of glucoerucin. Molecules (2019) 24(16):3006. doi: 10.3390/molecules24163006
152. Martelli A, Piragine E, Gorica E, Citi V, Testai L, Pagnotta E, et al. The H2S-donor erucin exhibits protective effects against vascular inflammation in human endothelial and smooth muscle cells. Antioxid (Basel) (2021) 10(6):961. doi: 10.3390/antiox10060961
153. Piragine E, Flori L, Di Cesare Mannelli L, Ghelardini C, Pagnotta E, Matteo R, et al. Eruca sativa mill. seed extract promotes anti-obesity and hypoglycemic effects in mice fed with a high-fat diet. Phytother Res (2021) 35(4):1983–90. doi: 10.1002/ptr.6941
154. Cai YR, Hu CH. Computational study of H2S release in reactions of diallyl polysulfides with thiols. J Phys Chem B (2017) 121(26):6359–66. doi: 10.1021/acs.jpcb.7b03683
155. Lin Y, Yang X, Lu Y, Liang D, Huang D. Isothiocyanates as H2S donors triggered by cysteine: Reaction mechanism and structure and activity relationship. Org Lett (2019) 21(15):5977–80. doi: 10.1021/acs.orglett.9b02117
156. Liu Y, Yang R, Liu X, Zhou Y, Qu C, Kikuiri T, et al. Hydrogen sulfide maintains mesenchymal stem cell function and bone homeostasis via regulation of Ca(2+) channel sulfhydration. Cell Stem Cell (2014) 15(1):66–78. doi: 10.1016/j.stem.2014.03.005
157. Grassi F, Tyagi AM, Calvert JW, Gambari L, Walker LD, Yu M, et al. Hydrogen sulfide is a novel regulator of bone formation implicated in the bone loss induced by estrogen deficiency. J Bone Miner Res (2016) 31(5):949–63. doi: 10.1002/jbmr.2757
158. Lee SK, Chung JH, Choi SC, Auh QS, Lee YM, Lee SI, et al. Sodium hydrogen sulfide inhibits nicotine and lipopolysaccharide-induced osteoclastic differentiation and reversed osteoblastic differentiation in human periodontal ligament cells. J Cell Biochem (2013) 114(5):1183–93. doi: 10.1002/jcb.24461
159. Ma J, Shi C, Liu Z, Han B, Guo L, Zhu L, et al. Hydrogen sulfide is a novel regulator implicated in glucocorticoids-inhibited bone formation. Aging (Albany NY). (2019) 11(18):7537–52. doi: 10.18632/aging.102269
160. Zheng W, Li X, Zhang T, Wang J. Biological mechanisms and clinical efficacy of sulforaphane for mental disorders. Gen Psychiatr (2022) 35(2):e100700. doi: 10.1136/gpsych-2021-100700
161. Jiang X, Chen Y, Lu K, Zhang H, Fan X. GYY4137 promotes bone formation in a rabbit distraction osteogenesis model: a preliminary report. J Oral Maxillofac Surg (2015) 73(4):732.e1–6. doi: 10.1016/j.joms.2014.11.012
162. Sivapalan T, Melchini A, Saha S, Needs PW, Traka MH, Tapp H, et al. Bioavailability of glucoraphanin and sulforaphane from high-glucoraphanin broccoli. Mol Nutr Food Res (2018) 62(18):e1700911. doi: 10.1002/mnfr.201700911
163. Fahey JW, Wade KL, Stephenson KK, Panjwani AA, Liu H, Cornblatt G, et al. Bioavailability of sulforaphane following ingestion of glucoraphanin-rich broccoli sprout and seed extracts with active myrosinase: A pilot study of the effects of proton pump inhibitor administration. Nutrients (2019) 11(7):1489. doi: 10.3390/nu11071489
164. Lai RH, Miller MJ, Jeffery E. Glucoraphanin hydrolysis by microbiota in the rat cecum results in sulforaphane absorption. Food Funct (2010) 1(2):161–6. doi: 10.1039/c0fo00110d
165. Bheemreddy RM, Jeffery EH. The metabolic fate of purified glucoraphanin in F344 rats. J Agric Food Chem (2007) 55(8):2861–6. doi: 10.1021/jf0633544
166. Liu X, Wang Y, Hoeflinger JL, Neme BP, Jeffery EH, Miller MJ. Dietary broccoli alters rat cecal microbiota to improve glucoraphanin hydrolysis to bioactive isothiocyanates. Nutrients (2017) 9(3):262. doi: 10.3390/nu9030262
167. Davidson R, Gardner S, Jupp O, Bullough A, Butters S, Watts L, et al. Isothiocyanates are detected in human synovial fluid following broccoli consumption and can affect the tissues of the knee joint. Sci Rep (2017) 7(1):3398. doi: 10.1038/s41598-017-03629-5
168. Oliviero T, Lamers S, Capuano E, Dekker M, Verkerk R. Bioavailability of isothiocyanates from broccoli sprouts in protein, lipid, and fiber gels. Mol Nutr Food Res (2018) 62(18):e1700837. doi: 10.1002/mnfr.201700837
169. Dinkova-Kostova AT, Fahey JW, Kostov RV, Kensler TW. KEAP1 and done? targeting the NRF2 pathway with sulforaphane. Trends Food Sci Technol (2017) 69(Pt B):257–69. doi: 10.1016/j.tifs.2017.02.002
170. Fahey JW, Wade KL, Wehage SL, Holtzclaw WD, Liu H, Talalay P, et al. Stabilized sulforaphane for clinical use: Phytochemical delivery efficiency. Mol Nutr Food Res (2017) 61(4). doi: 10.1002/mnfr.201600766
171. Gasparello J, Gambari L, Papi C, Rozzi A, Manicardi A, Corradini R, et al. High levels of apoptosis are induced in the human colon cancer HT-29 cell line by Co-administration of sulforaphane and a peptide nucleic acid targeting miR-15b-5p. Nucleic Acid Ther (2020) 30(3):164–74. doi: 10.1089/nat.2019.0825
172. Ullah MF. Sulforaphane (SFN): An isothiocyanate in a cancer chemoprevention paradigm. Medicines (Basel). (2015) 2(3):141–56. doi: 10.3390/medicines2030141
173. Zhang Z, Garzotto M, Davis EW 2nd, Mori M, Stoller WA, Farris PE, et al. Sulforaphane bioavailability and chemopreventive activity in men presenting for biopsy of the prostate gland: A randomized controlled trial. Nutr Cancer. (2020) 72(1):74–87. doi: 10.1080/01635581.2019.1619783
174. Jeon SM, Kim JE, Shin SK, Kwon EY, Jung UJ, Baek NI, et al. Randomized double-blind placebo-controlled trial of powdered brassica rapa ethanol extract on alteration of body composition and plasma lipid and adipocytokine profiles in overweight subjects. J Med Food (2013) 16(2):133–8. doi: 10.1089/jmf.2012.2249
175. Bahadoran Z, Tohidi M, Nazeri P, Mehran M, Azizi F, Mirmiran P. Effect of broccoli sprouts on insulin resistance in type 2 diabetic patients: a randomized double-blind clinical trial. Int J Food Sci Nutr (2012) 63(7):767–71. doi: 10.3109/09637486.2012.665043
176. Blekkenhorst LC, Bondonno CP, Lewis JR, Devine A, Zhu K, Lim WH, et al. Cruciferous and allium vegetable intakes are inversely associated with 15-year atherosclerotic vascular disease deaths in older adult women. J Am Heart Assoc (2017) 6(10):e006558. doi: 10.1161/JAHA.117.006558
177. Millen AE, Subar AF, Graubard BI, Peters U, Hayes RB, Weissfeld JL, et al. Fruit and vegetable intake and prevalence of colorectal adenoma in a cancer screening trial. Am J Clin Nutr (2007) 86(6):1754–64. doi: 10.1093/ajcn/86.5.1754
178. Traka MH, Melchini A, Coode-Bate J, Al Kadhi O, Saha S, Defernez M, et al. Transcriptional changes in prostate of men on active surveillance after a 12-mo glucoraphanin-rich broccoli intervention-results from the effect of sulforaphane on prostate CAncer PrEvention (ESCAPE) randomized controlled trial. Am J Clin Nutr (2019) 109(4):1133–44. doi: 10.1093/ajcn/nqz012
179. Matheson EM, Mainous AG 3rd, Carnemolla MA. The association between onion consumption and bone density in perimenopausal and postmenopausal non-Hispanic white women 50 years and older. Menopause (2009) 16(4):756–9. doi: 10.1097/gme.0b013e31819581a5
180. Greco EA, Pietschmann P, Migliaccio S. Osteoporosis and sarcopenia increase frailty syndrome in the elderly. Front Endocrinol (Lausanne). (2019) 10:255. doi: 10.3389/fendo.2019.00255
181. Sim M, Blekkenhorst LC, Lewis JR, Bondonno CP, Devine A, Zhu K, et al. Vegetable and fruit intake and injurious falls risk in older women: a prospective cohort study. Br J Nutr (2018) 120(8):925–34. doi: 10.1017/S0007114518002155
182. Gu Y, Zhang S, Wang J, Chi VTQ, Zhang Q, Liu L, et al. Relationship between consumption of raw garlic and handgrip strength in a large-scale adult population. Clin Nutr (2020) 39(4):1234–41. doi: 10.1016/j.clnu.2019.05.015
183. Sim M, Blekkenhorst LC, Lewis JR, Bondonno CP, Devine A, Zhu K, et al. Vegetable diversity, injurious falls, and fracture risk in older women: A prospective cohort study. Nutrients (2018) 10(8):1081. doi: 10.3390/nu10081081
184. Zuhra K, Tome CS, Forte E, Vicente JB, Giuffre A. The multifaceted roles of sulfane sulfur species in cancer-associated processes. Biochim Biophys Acta Bioenerg. (2021) 1862(2):148338. doi: 10.1016/j.bbabio.2020.148338
185. Kolluru GK, Shen X, Kevil CG. Reactive sulfur species: A new redox player in cardiovascular pathophysiology. Arterioscler Thromb Vasc Biol (2020) 40(4):874–84. doi: 10.1161/ATVBAHA.120.314084
186. Roda B, Zhang N, Gambari L, Grigolo B, Eller-Vainicher C, Gennari L, et al. Optimization of a monobromobimane (MBB) derivatization and RP-HPLC-FLD detection method for sulfur species measurement in human serum after sulfur inhalation treatment. Antioxid (Basel) (2022) 11(5):939. doi: 10.3390/antiox11050939
187. Chen K, Nakasone Y, Xie K, Sakao K, Hou DX. Modulation of allicin-free garlic on gut microbiome. Molecules (2020) 25(3):682. doi: 10.3390/molecules25030682
188. Chen K, Xie K, Liu Z, Nakasone Y, Sakao K, Hossain A, et al. Preventive effects and mechanisms of garlic on dyslipidemia and gut microbiome dysbiosis. Nutrients (2019) 11(6):1225. doi: 10.3390/nu11061225
189. Keirns BH, Lucas EA, Smith BJ. Phytochemicals affect T helper 17 and T regulatory cells and gut integrity: implications on the gut-bone axis. Nutr Res (2020) 83:30–48. doi: 10.1016/j.nutres.2020.08.006
190. Lee SH, Bang S, Jang HH, Lee EB, Kim BS, Kim SH, et al. Effects of allium hookeri on gut microbiome related to growth performance in young broiler chickens. PloS One (2020) 15(1):e0226833. doi: 10.1371/journal.pone.0226833
Keywords: organosulfur compounds (OSCs), osteoporosis, hydrogen sulfide (H2S), Brassicaceae, Allium, glucosinolates, isothiocyanates, polysulfides
Citation: Gambari L, Grigolo B and Grassi F (2022) Dietary organosulfur compounds: Emerging players in the regulation of bone homeostasis by plant-derived molecules. Front. Endocrinol. 13:937956. doi: 10.3389/fendo.2022.937956
Received: 06 May 2022; Accepted: 23 August 2022;
Published: 15 September 2022.
Edited by:
Simona Bertoli, University of Milan, ItalyReviewed by:
Franziska S. Hanschen, Leibniz Institute of Vegetable and Ornamental Crops, GermanyHanyao Huang, Sichuan University, China
Copyright © 2022 Gambari, Grigolo and Grassi. This is an open-access article distributed under the terms of the Creative Commons Attribution License (CC BY). The use, distribution or reproduction in other forums is permitted, provided the original author(s) and the copyright owner(s) are credited and that the original publication in this journal is cited, in accordance with accepted academic practice. No use, distribution or reproduction is permitted which does not comply with these terms.
*Correspondence: Laura Gambari, bGF1cmEuZ2FtYmFyaUBpb3IuaXQ=; Francesco Grassi, ZnJhbmNlc2NvLmdyYXNzaUBpb3IuaXQ=