- Pôle de chirurgie expérimentale et transplantation, Université catholique de Louvain, Brussels, Belgium
The potential use of porcine islets for transplantation in humans has triggered interest in understanding porcine islet physiology. However, the number of studies dedicated to this topic has remained limited, as most islet physiologists prefer to use the less time-consuming rodent model or the more clinically relevant human islet. An often-overlooked aspect of pig islet physiology is its alpha cell activity and regulation of its glucagon secretion. In vitro islet perifusion is a reliable method to study the dynamics of hormone secretion in response to different stimuli. We thus used this method to quantify and study glucagon secretion from pig islets. Pancreatic islets were isolated from 20 neonatal (14 to 21-day old) and 5 adult (>2 years) pigs and cultured in appropriate media. Islet perifusion experiments were performed 8 to 10 days post-isolation for neonatal islets and 1 to 2 days post-isolation for adult islets. Insulin and glucagon were quantified in perifusion effluent fractions as well as in islet extracts by RIA. Increasing glucose concentration from 1 mM to 15 mM markedly inhibited glucagon secretion independently of animal age. Interestingly, the effect of high glucose was more drastic on glucagon secretion compared to its effect on insulin secretion. In vivo, glucose injection during IVGTT initiated a quick (2-10 minutes) 3-fold decrease of plasmatic glucagon whereas the increase of plasmatic insulin took 20 minutes to become significant. These results suggest that regulation of glucagon secretion significantly contributes to glucose homeostasis in pigs and might compensate for the mild changes in insulin secretion in response to changes in glucose concentration.
Introduction
Pancreatic islets adapt their hormone output to surrounding glucose concentration through stimulus-secretion coupling mechanisms that translate glucose and other nutrient metabolism into increasing or decreasing secretory rates of the two major pancreatic hormones, insulin and glucagon. Beta-cells have been thoroughly studied for years and pathways by which glucose controls insulin secretion are mostly well-understood: glucose accelerates beta-cell metabolism resulting in an increase of cytosolic ATP/ADP ratio leading to closure of ATP-dependent potassium channels, membrane depolarization and opening of voltage-gated calcium channels which then triggers insulin granule exocytosis that can be further modulated by yet unknown metabolic signals resulting in amplification of glucose-induced insulin secretion thought to account for as much as 50% of total insulin response (1, 2). The situation is less clear in the case of alpha-cells as two major hypothesis have been debated (3): in a beta-cell-like model, glucose metabolism causes closure of ATP-dependent potassium channels but fails to sufficiently depolarize the membrane to open L-type calcium channels (4, 5) while inactivating voltage-dependent Na+ channels and therefore reducing Ca2+ entry via P/Q calcium channels due to diminished amplitude of action potentials (6, 7). This glucose-induced fall in [Ca2+]i then suppresses glucagon secretion (8). Another model proposes ER store-operated calcium entry and subsequent membrane depolarization followed by further calcium entry via voltage-gated calcium channels as the mechanism by which low glucose stimulates glucagon secretion (9, 10). All of the above-mentioned studies utilized mostly rodent and sometimes human islet cells to explore these stimulus-secretion coupling mechanisms. In porcine islets, glucose has been shown to trigger biphasic insulin secretion with a major difference in the amplitude of the response as we (11) and others (12, 13) have reported a modest 2-4-fold increase of insulin secretion (11) upon stimulation with 15 mM glucose compared to a 10-fold increase at least following similar stimulation of human islets (14). Even less is known of the regulation of glucagon secretion from porcine islets with one study reporting very low basal and arginine-stimulated glucagon secretion from adult pig islets (15) while other studies focusing on porcine islet function have simply overlooked this aspect of their physiology. In the present study, we used in vitro dynamic perifusion of isolated islets as well as in vivo glucose tolerance test to characterize glucagon secretion from neonatal and adult porcine islets since they currently represent one of the most realistically relevant options for islet replacement therapy in the clinic (16).
Materials and methods
Islet isolation
All experiments were conducted in accordance with the local ethical committee and with EU Directive 2010/63/EU for animal experiments. Outbred pigs (Belgian landrace; 200-300 kg) were provided by Rattlerow-Seghers genetics (Ooigem, Belgium). Piglets (Belgian landrace; 3-8 kg) were directly delivered to the islet isolation facility in Brussels. Adult pigs were housed in the A. de Marbaix center (Louvain-la-Neuve, Belgium). Pancreatic islet isolation was carried out as previously described (11). Briefly, collagenase V from Sigma (Darmstadt, Germany) was used for piglet pancreas digestion and collagenase NB8 from Serva (Heidelberg, Germany) was used for adult pancreas digestion. Neonatal pig islets (NPI) were cultured in HAM-F10 culture medium supplemented with 0.5% BSA, 50 µM IBMX (3-isobutyl-1-methylxanthine), 10 mM glucose, 2 mM glutamine, 10 mM nicotinamide, 1% penicillin and 1% streptomycin during a period of 8 days to allow maturation of islet cells and removal of exocrine tissue as previously described (17). Adult porcine islets (API) were purified using a discontinuous Ficoll gradient before culture in RPMI 1640 supplemented with 10% heat-inactivated FBS, 1% penicillin, 1% streptomycin, 5 mM glutamine and 5 mM glucose for 12-24 hours before islet perifusion.
In vitro insulin and glucagon secretion assays
Secretory function of isolated neonatal and adult islets was studied by dynamic islet perifusion experiments. the working medium was a bicarbonate-buffered solution containing 120 mM NaCl, 4.8 mM KCl, 2.5 mM CaCl2, 1.2 mM MgCl2, 24 mM NaHCO3, 1 mg/mL BSA and varying concentrations of glucose and test substances as indicated in the figures. Batches of 200-1000 IEQ were placed in perifusion chambers, covered with 8 µm cellulose filters and sealed. Test solutions kept at 37°C and gassed continuously to stabilize pH around 7.2 were pumped at a flow rate of 1 mL/min. Effluent fractions were collected at 2-minute intervals and saved for insulin and glucagon assays using radioimmunoassay (RIA) kits (Millipore, Billerica, MA, USA). At the end of the experiments, islets were recovered and their insulin and glucagon content was determined after extraction in acid-ethanol (75% ethanol, 180 mM HCl from Merck).
Intravenous glucose tolerance test
To study in vivo secretion of insulin and glucagon in adult pigs, we performed glucose tolerance tests by administering an intravenous glucose bolus (0.5 g/kg bodyweight) in an auricular vein. Blood samples were taken before and at 1, 3, 5, 10, 20, 30, 60 and 90 minutes after glucose injection using a catheter placed on the other ear. Blood glucose was immediately measured using Aviva nano glucometer (Accu-Chek) and serum samples were prepared and stored at -80°C for subsequent insulin and glucagon assays.
Results
Glucose inhibits glucagon and stimulates insulin secretion from porcine islets
Islet hormone secretion was tested after 8 days of culture in a maturation medium for neonatal islets and after 24-48 hours for adult islets. As shown in Table 1, islet glucagon content decreased from 817 pg/IEQ at neonatal age (2-3 weeks) to 471 pg/IEQ at adult age (>18 months). In parallel, insulin content increased from 113 µU/IEQ to 723 µU/IEQ at neonatal and adult age respectively. Using dynamic islet perifusion, we studied glucagon and insulin secretion in response to changes in glucose concentration. At low glucose (1 mM; G1), glucagon secretion was stimulated in both neonatal (2.6 pg/IEQ/min; Figure 1A) and adult (1.5 pg/IEQ/min; Figure 1B) islets. Basal insulin secretion was lower in neonatal islets compared to adult islets, 2.10-3 µU/IEQ/min vs. 4.10-2 µU/IEQ/min respectively. Upon stimulation with 15 mM glucose (G15), glucagon secretion dropped 6-fold in neonatal islets and only 2-fold in adult islets. In parallel, insulin secretion increased similarly in both neonatal and adult islets (2.6- and 2.3-fold respectively; Figures 1A, B). Interestingly, inhibition of glucagon secretion occurred after 4 minutes of glucose stimulation whereas the increase of insulin secretion took 6 minutes to become measurable as shown in Figure 1.
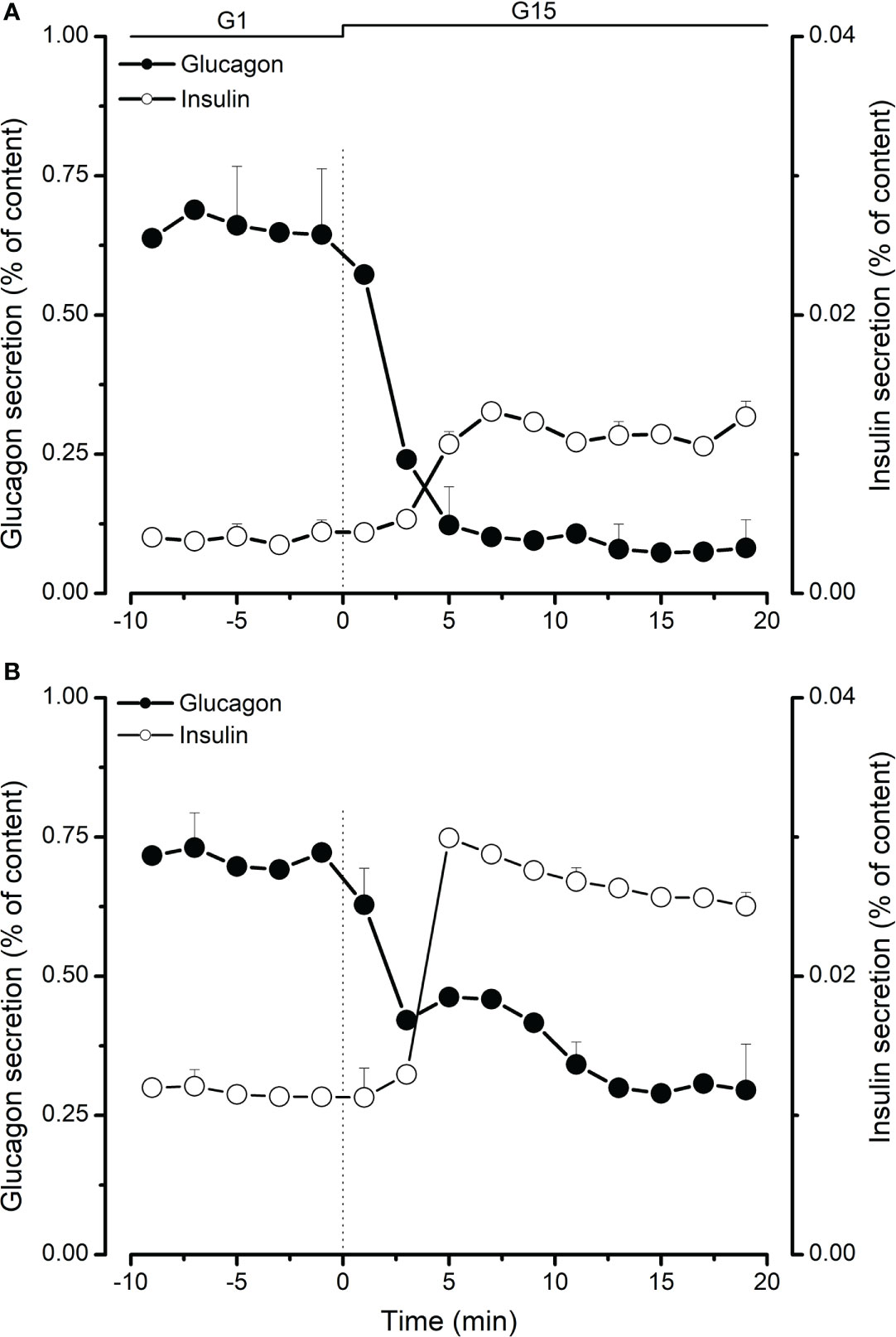
Figure 1 Comparison of glucagon (●) and insulin (○) secretion from neonatal (A) and adult (B) perifused porcine islets. Islets in 1 mM glucose (G1) were stimulated with 15 mM glucose (G15) for 20 minutes. Glucagon and insulin secretion was measured in effluent fractions and expressed as percentage of total glucagon or insulin islet content. Values are means ± SEM from n=4-8 different preparations from each age group.
Role of ATP-dependent potassium channels in regulation of glucagon secretion
In a second set of experiments, we tested the effect of tolbutamide that mimics the action of glucose on ATP-dependent potassium channels by binding their SUR subunit. In the presence of high glucose (15 mM), 500 µM tolbutamide had no effect on glucagon secretion in both neonatal and adult islets (Figure 2A). Membrane depolarization using 30 mM KCl (K30) significantly increased glucagon secretion in the presence of high glucose. As shown in Figure 2A, the effect of K30 was significantly higher on neonatal islets compared to its effect on islets from adult pigs. Addition of ATP-dependent channel opener, diazoxide (50 µM) significantly inhibited low glucose-induced glucagon secretion and this inhibitory effect was partially reversed by tolbutamide (Figure 2B).
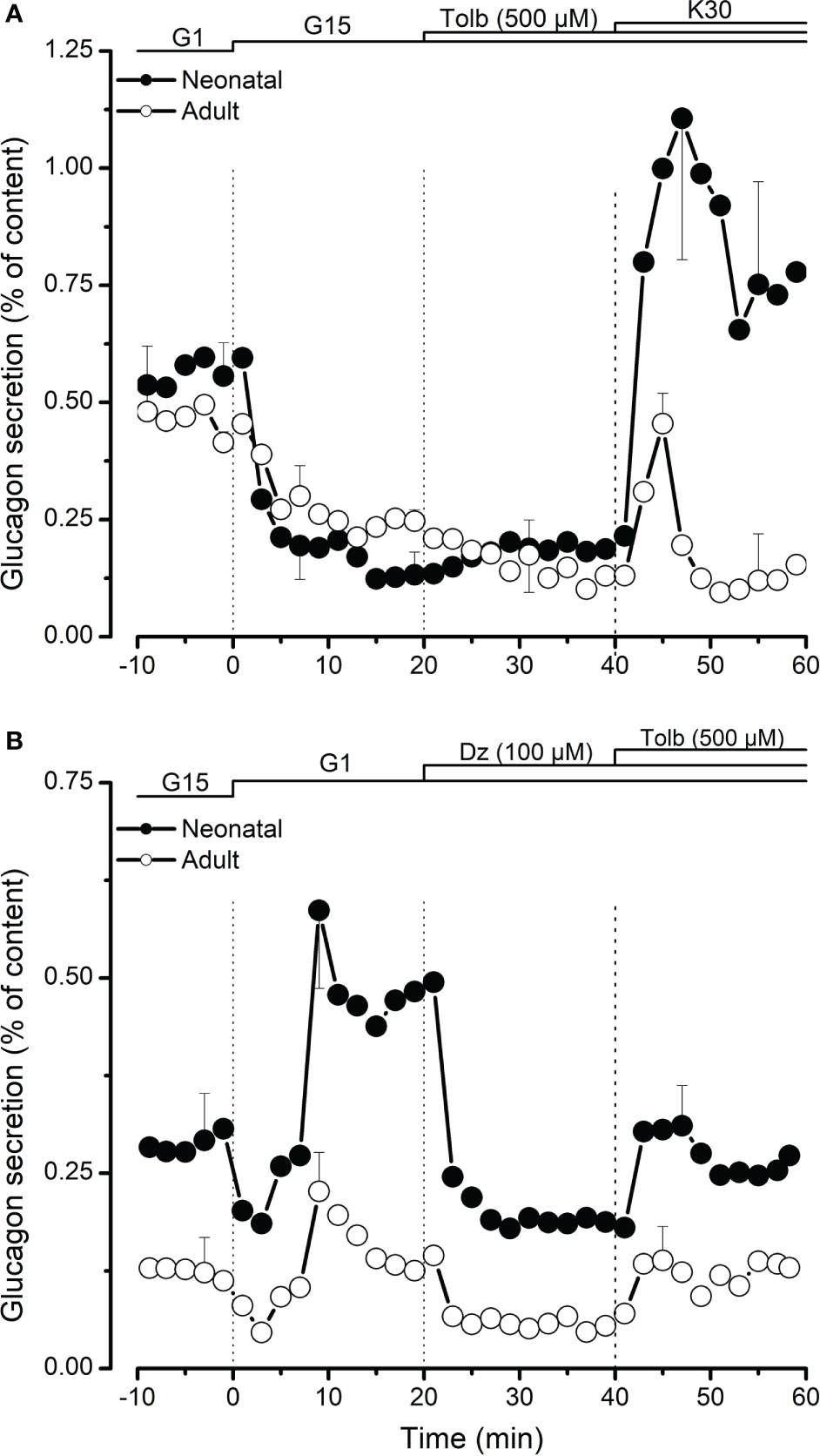
Figure 2 Role of K-ATP channels in regulation of glucagon secretion from neonatal (●) and adult (○) porcine islets. In (A), the effect of K-ATP channel blocker, tolbutamide (Tolb, 500 µM) was studied in the presence of high glucose (15 mM, G15). 30 mM KCl (K30) was added at the end to test the effect of membrane depolarization on glucagon secretion. In (B), the effects of tolbutamide and K-ATP channel activator, diazoxide (Dz, 100 µM) were studied in the presence of low glucose (1 mM, G1). Values are means ± SEM from n=3 different preparations from each age group.
Effect of cAMP on glucagon secretion
We used froskolin (1 µM) to activate c-AMP-dependent secretion pathways in porcine islet cells. As shown in Figure 3A, increasing cystosolic cAMP greatly increased stimulated glucagon secretion from neonatal islets and slightly slowed down the inhibitory effect of high glucose. This was also observed, although to a lesser extent, with adult islets (Figure 3B).
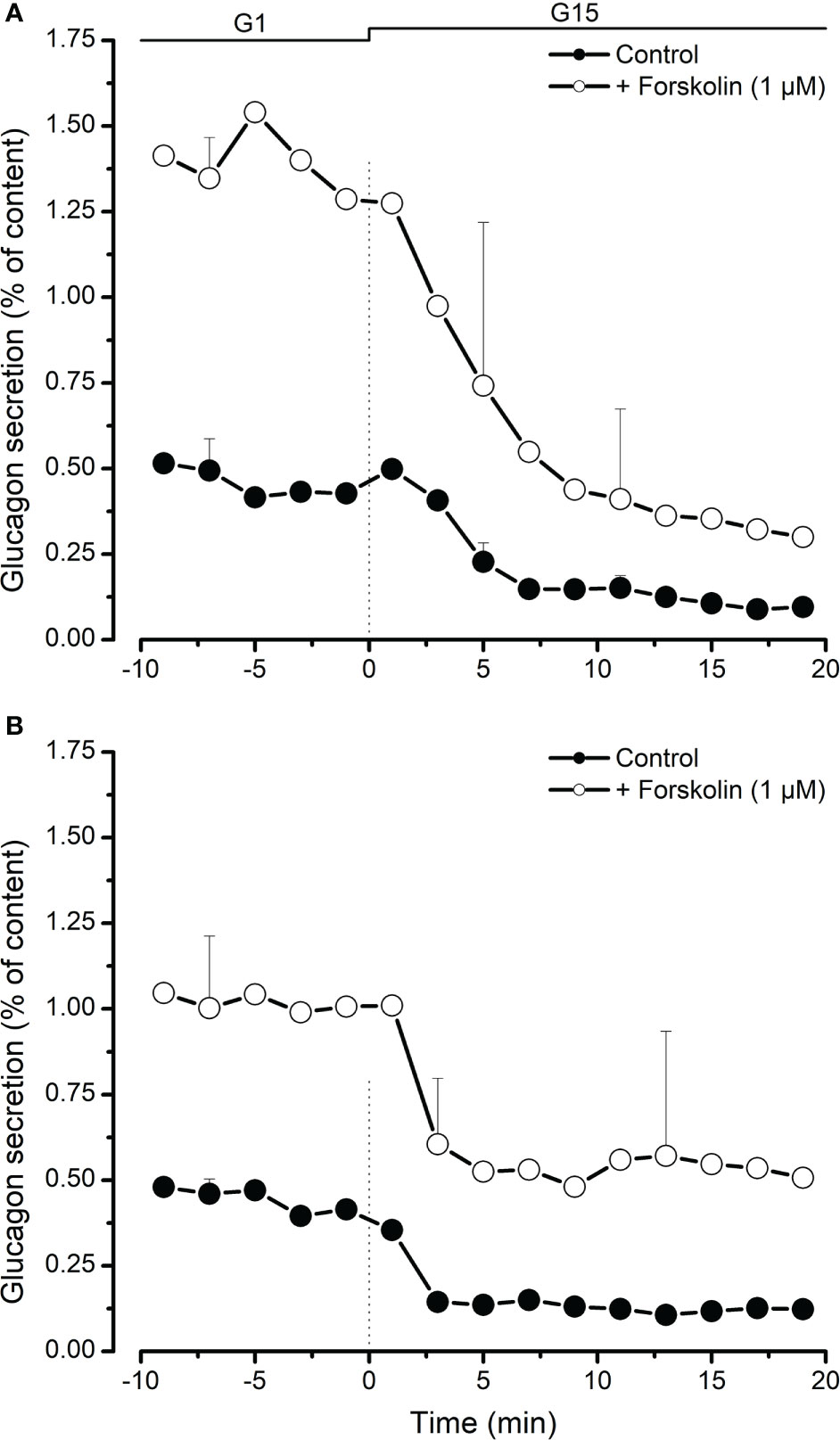
Figure 3 Inhibition of glucagon secretion by high glucose (15 mM, G15) from neonatal (A) and adult (B) porcine islets in the presence (○) or absence (●) of forskolin (1 µM, adenylyl cyckase activator causing an increase of cytosolic cAMP). Values are means ± SEM from n=3 different preparations from each age group.
In vivo response to hyperglycemic stimulation
We performed intravenous glucose tolerance test in 4 adult pigs. Mean fasting glycaemia was 125 mg/dl. Following glucose injection, the rise in glycaemia was detectable already after 2 minutes, and then it gradually decreased and returned to starting values after 90 minutes (Figure 4A). Plasma insulin measurement showed a slower response that culminated in a 2-fold increase 20 minutes after glucose injection (Figure 4B). In parallel, glucagon concentration dropped 3-fold within 3-5 minutes (Figure 4C).
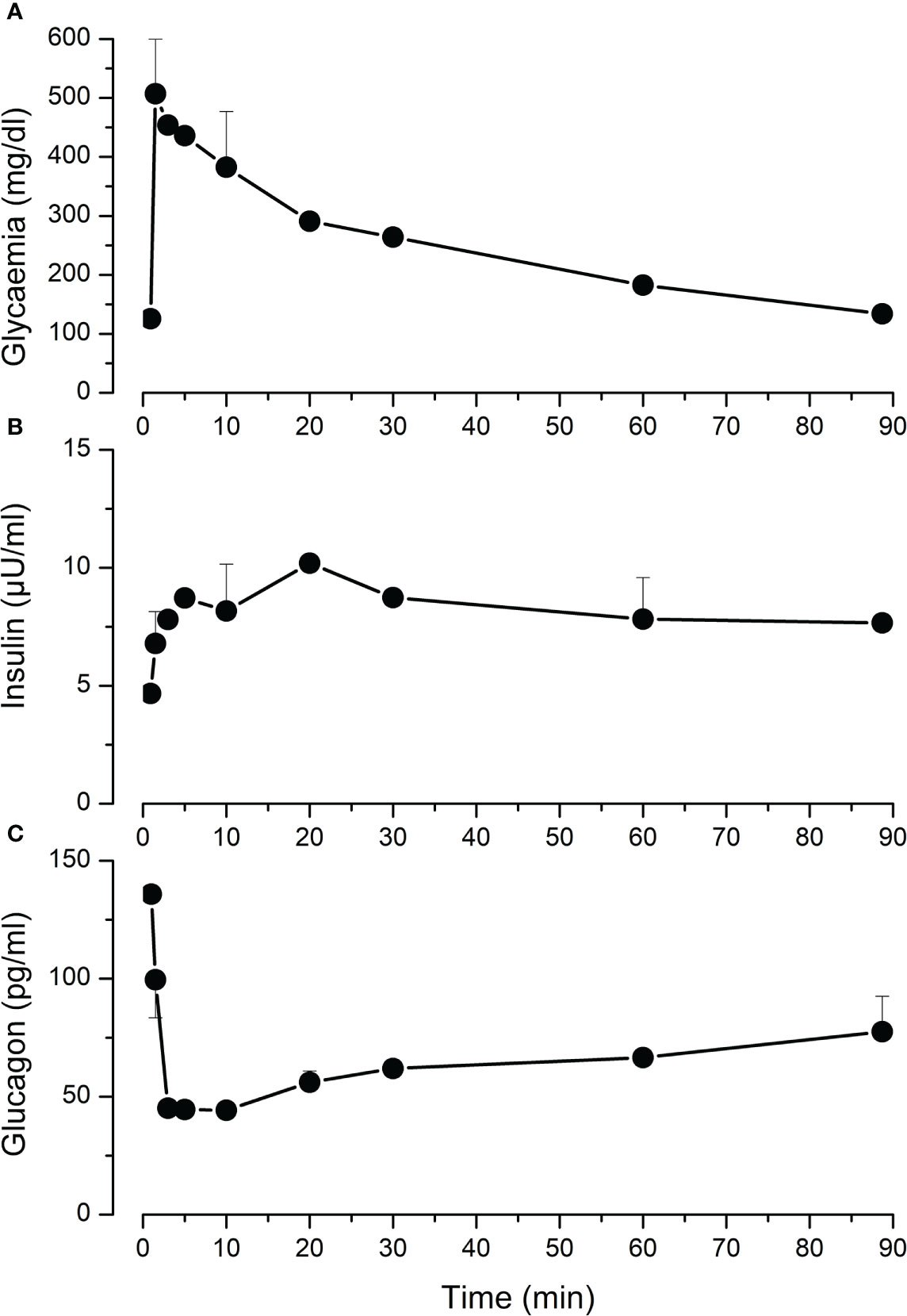
Figure 4 Intravenous glucose tolerance test (IVGGT) in adult pigs. Sedated fasting animals received an intravenous glucose bolus (0.5 g/Kg) at t=0. Blood samples were taken before and during 90 minutes after the injection to measure glycaemia (A), insulin (B) and glucagon (C). Values are means ± SEM from n=4 adult pigs.
Discussion
Interest in porcine islets as a potential cell source for islet replacement therapy was sparked by several of their characteristics including physiological compatibility of porcine insulin in humans, availability of theoretically unlimited donor supply and possibility of genetic modifications of donor pigs to render their cells less immunogenic (16, 18, 19), exclude potential xeno-pathogens (20, 21) or even increase their insulin secretion (11). A number of studies have investigated porcine islet physiology both in vitro and in vivo all agreeing on the low insulin response of neonatal and even adult islets to stimulation with glucose or other secretagogues (11–13, 22). Much less documented is the regulation of glucagon secretion from porcine islets. Our results show that, similarly to rodent (23) and human (24) islets, high concentration of glucose inhibits glucagon secretion from both neonatal and adult islets. In rodent islets, full ATP-dependent potassium channel closure initiated by tolbutamide has been shown to stimulate glucagon secretion in the presence of inhibitory glucose levels (23, 25). In human islets, tolbutamide (100-500 µM), exerts its glucagonostatic effect independently of paracrine activity via intrinsic depolarization of alpha-cell membrane and reduction of calcium entry (24, 26). The situation differs in porcine islets since we did not observe any effect of tolbutamide on glucagon secretion when it was already inhibited by glucose. Only membrane depolarization with 30 mM KCl succeeded in stimulating glucagon secretion despite the presence of high glucose. As expected, high concentration of diazoxide (100 µM) inhibited glucagon secretion and this inhibition was partially lifted by tolbutamide in the presence of low glucose. This might reflect membrane depolarization by tolbutamide independently of its effect on action potential amplitude since 100 µM diazoxide completely abolish action potential firing (6). Increasing cytosolic cAMP is known to amplify glucose-stimulated insulin secretion from porcine islets (11, 12). Here, we show that forskolin increases low glucose-stimulated glucagon secretion and slightly reduces the inhibitory effect of high glucose on glucagon. This observation is similar to what has been previously reported in mouse and human islets (27, 28) but differs from other experiments using human islets where GLP-1 and forskolin both induced a 2-3-fold inhibition of glucagon secretion in the presence of 1 mM glucose (29). Compared to their adult counterparts, neonatal porcine islets had greater glucagon content and were more responsive to glucose or tolbutamide stimulation, membrane depolarization and cAMP-dependent amplification even after secretion was reported to total islet glucagon content. Moreover, the effects of these substances on glucagon secretion was more drastic than their effects on insulin secretion especially in neonatal islets suggesting an important role of glucagon in porcine islet physiology. This is supported by our observation that during IVGTT, there was a very rapid 3-fold drop in plasmatic glucagon whereas a 2-fold increase of insulin concentration took 20 minutes to occur. Similarly, a clinical study in healthy individuals showed in vivo stimulation of glucagon secretion under hypoglycaemic conditions and inhibition in normoglycaemia or following infusion of tolbutamide. In contrast, the effect of glucose on in vivo insulin secretion estimated from plasma C-peptide is more drastic and rapid in humans (30). Differences in pancreatic hormone secretion profiles from neonatal and adult porcine islets reflect maturation of islet cells (31) as previously shown in human islets (32) where basal insulin secretion was high and glucose responsiveness required presence of cAMP-raising agent forskolin. The prominent role of glucagon in neonatal islet physiology might reflect the yet immature state of glucose metabolism-secretion coupling in young beta cells and their reliance on glucagon-dependent cAMP signaling to stimulate insulin secretion in response to glucose (33). In conclusion, our study, the first to investigate regulation of glucagon secretion from porcine islets, highlights the necessity for a better understanding of pig islet physiology as their peculiar secretory characteristics will greatly affect the outcome of their transplantation to human patients.
Data availability statement
The raw data supporting the conclusions of this article will be made available by the authors, without undue reservation.
Ethics statement
The animal study was reviewed and approved by Commission d’Ethique pour l’Expérimentation Animale, Secteur des Sciences de la Santé, Université catholique de Louvain.
Author contributions
NM designed the study, performed experiments, analyzed data and wrote the manuscript. DX performed experiments. PG designed the study and wrote the manuscript. All authors contributed to the article and approved the submitted version.
Funding
This work was partially funded by the ‘‘Service public de Wallonie, SPW Economie, Emploi, Recherche’’ Grant ‘‘8318 SPW’’.
Conflict of interest
The authors declare that the research was conducted in the absence of any commercial or financial relationships that could be construed as a potential conflict of interest.
Publisher’s note
All claims expressed in this article are solely those of the authors and do not necessarily represent those of their affiliated organizations, or those of the publisher, the editors and the reviewers. Any product that may be evaluated in this article, or claim that may be made by its manufacturer, is not guaranteed or endorsed by the publisher.
References
1. Henquin JC. Triggering and amplifying pathways of regulation of insulin secretion by glucose. Diabetes (2000) 49:1751–60. doi: 10.2337/diabetes.49.11.1751
2. Henquin JC, Nenquin M, Ravier MA, Szollosi A. Shortcomings of current models of glucose-induced insulin secretion. Diabetes Obes Metab (2009) 11 (Suppl 4):168–79. doi: 10.1111/j.1463-1326.2009.01109.x
3. Gylfe E, Gilon P. Glucose regulation of glucagon secretion. Diabetes Res Clin Pract (2014) 103:1–10. doi: 10.1016/j.diabres.2013.11.019
4. Barg S, Galvanovskis J, Gopel SO, Rorsman P, Eliasson L. Tight coupling between electrical activity and exocytosis in mouse glucagon-secreting alpha-cells. Diabetes (2000) 49:1500–10. doi: 10.2337/diabetes.49.9.1500
5. Quoix N, Cheng-Xue R, Mattart L, Zeinoun Z, Guiot Y, Beauvois MC, et al. Glucose and pharmacological modulators of ATP-sensitive k+ channels control [Ca2+]c by different mechanisms in isolated mouse alpha-cells. Diabetes (2009) 58:412–21. doi: 10.2337/db07-1298
6. Zhang Q, Ramracheya R, Lahmann C, Tarasov A, Bengtsson M, Braha O, et al. Role of KATP channels in glucose-regulated glucagon secretion and impaired counterregulation in type 2 diabetes. Cell Metab (2013) 18:871–82. doi: 10.1016/j.cmet.2013.10.014
7. Zhang Q, Chibalina MV, Bengtsson M, Groschner LN, Ramracheya R, Rorsman NJ, et al. Na+ current properties in islet alpha- and beta-cells reflect cell-specific Scn3a and Scn9a expression. J Physiol (2014) 592:4677–96. doi: 10.1113/jphysiol.2014.274209
8. Zhang Q, Dou H, Rorsman P. 'Resistance is futile?' - paradoxical inhibitory effects of KATP channel closure in glucagon-secreting alpha-cells. J Physiol (2020) 598:4765–80. doi: 10.1113/JP279775
9. Liu YJ, Vieira E, Gylfe E. A store-operated mechanism determines the activity of the electrically excitable glucagon-secreting pancreatic alpha-cell. Cell Calcium (2004) 35:357–65. doi: 10.1016/j.ceca.2003.10.002
10. Vieira E, Salehi A, Gylfe E. Glucose inhibits glucagon secretion by a direct effect on mouse pancreatic alpha cells. Diabetologia (2007) 50:370–9. doi: 10.1007/s00125-006-0511-1
11. Mourad NI, Perota A, Xhema D, Galli C, Gianello P. Transgenic expression of glucagon-like peptide-1 (GLP-1) and activated muscarinic receptor (M3R) significantly improves pig islet secretory function. Cell Transplant (2017) 26:901–11. doi: 10.3727/096368916X693798
12. Dufrane D, Nenquin M, Henquin JC. Nutrient control of insulin secretion in perifused adult pig islets. Diabetes Metab (2007) 33:430–8. doi: 10.1016/j.diabet.2007.05.001
13. Mueller KR, Balamurugan AN, Cline GW, Pongratz RL, Hooper RL, Weegman BP, et al. Differences in glucose-stimulated insulin secretion in vitro of islets from human, nonhuman primate, and porcine origin. Xenotransplantation (2013) 20:75–81. doi: 10.1111/xen.12022
14. Henquin JC. Glucose-induced insulin secretion in isolated human islets: Does it truly reflect beta-cell function in vivo? Mol Metab (2021) 48:101212. doi: 10.1016/j.molmet.2021.101212
15. Bertuzzi F, Berra C, Socci C, Davalli AM, Calori G, Freschi M, et al. Glucagon improves insulin secretion from pig islets in vitro. J Endocrinol (1995) 147:87–93. doi: 10.1677/joe.0.1470087
16. Mourad NI, Gianello PR. Xenoislets: porcine pancreatic islets for the treatment of type I diabetes. Curr Opin Organ Transplant (2017) 22:529–34. doi: 10.1097/MOT.0000000000000464
17. Korbutt GS, Elliott JF, Ao Z, Smith DK, Warnock GL, Rajotte RV. Large Scale isolation, growth, and function of porcine neonatal islet cells. J Clin Invest (1996) 97:2119–29. doi: 10.1172/JCI118649
18. Phelps CJ, Koike C, Vaught TD, Boone J, Wells KD, Chen SH, et al. Production of alpha 1,3-galactosyltransferase-deficient pigs. Science (2003) 299:411–4. doi: 10.1126/science.1078942
19. Hawthorne WJ, Lew AM, Thomas HE. Genetic strategies to bring islet xenotransplantation to the clinic. Curr Opin Organ Transplant (2016) 21:476–83. doi: 10.1097/MOT.0000000000000353
20. Scobie L, Denner J, Schuurman HJ. Inactivation of porcine endogenous retrovirus in pigs using CRISPR-Cas9, editorial commentary. Xenotransplantation (2017) 24:6. doi: 10.1111/xen.12363
21. Niu D, Wei HJ, Lin L, George H, Wang T, Lee IH, et al. Inactivation of porcine endogenous retrovirus in pigs using CRISPR-Cas9. Science (2017) 357:1303–7. doi: 10.1126/science.aan4187
22. Smith KE, Purvis WG, Davis MA, Min CG, Cooksey AM, Weber CS, et al. In vitro characterization of neonatal, juvenile, and adult porcine islet oxygen demand, beta-cell function, and transcriptomes. Xenotransplantation (2018) 25:e12432. doi: 10.1111/xen.12432
23. Cheng-Xue R, Gomez-Ruiz A, Antoine N, Noel LA, Chae HY, Ravier MA, et al. Tolbutamide controls glucagon release from mouse islets differently than glucose: involvement of K(ATP) channels from both alpha-cells and delta-cells. Diabetes (2013) 62:1612–22. doi: 10.2337/db12-0347
24. Ramracheya R, Ward C, Shigeto M, Walker JN, Amisten S, Zhang Q, et al. Membrane potential-dependent inactivation of voltage-gated ion channels in alpha-cells inhibits glucagon secretion from human islets. Diabetes (2010) 59:2198–208. doi: 10.2337/db09-1505
25. Singh B, Khattab F, Chae H, Desmet L, Herrera PL, Gilon P. KATP channel blockers control glucagon secretion by distinct mechanisms: A direct stimulation of alpha-cells involving a [Ca(2+)]c rise and an indirect inhibition mediated by somatostatin. Mol Metab (2021) 53:101268. doi: 10.1016/j.molmet.2021.101268
26. MacDonald PE, De Marinis YZ, Ramracheya R, Salehi A, Ma X, Johnson PR, et al. A K ATP channel-dependent pathway within alpha cells regulates glucagon release from both rodent and human islets of langerhans. PloS Biol (2007) 5:e143. doi: 10.1371/journal.pbio.0050143
27. Edlund A, Pedersen MG, Lindqvist A, Wierup N, Flodstrom-Tullberg M, Eliasson L. CFTR is involved in the regulation of glucagon secretion in human and rodent alpha cells. Sci Rep (2017) 7:90. doi: 10.1038/s41598-017-00098-8
28. De Marinis YZ, Salehi A, Ward CE, Zhang Q, Abdulkader F, Bengtsson M, et al. GLP-1 inhibits and adrenaline stimulates glucagon release by differential modulation of n- and l-type Ca2+ channel-dependent exocytosis. Cell Metab (2010) 11:543–53. doi: 10.1016/j.cmet.2010.04.007
29. Ramracheya R, Chapman C, Chibalina M, Dou H, Miranda C, Gonzalez A, et al. GLP-1 suppresses glucagon secretion in human pancreatic alpha-cells by inhibition of P/Q-type Ca(2+) channels. Physiol Rep (2018) 6:e13852. doi: 10.14814/phy2.13852
30. Banarer S, McGregor VP, Cryer PE. Intraislet hyperinsulinemia prevents the glucagon response to hypoglycemia despite an intact autonomic response. Diabetes (2002) 51:958–65. doi: 10.2337/diabetes.51.4.958
31. Kim S, Whitener RL, Peiris H, Gu X, Chang CA, Lam JY, et al. Molecular and genetic regulation of pig pancreatic islet cell development. Dev (2020) 147(6). doi: 10.1242/dev.186213
32. Henquin JC, Nenquin M. Immaturity of insulin secretion by pancreatic islets isolated from one human neonate. J Diabetes Investig (2018) 9:270–3. doi: 10.1111/jdi.12701
Keywords: porcine islets, glucagon, insulin, perifusion, secretion
Citation: Mourad NI, Xhema D and Gianello P (2022) In vitro assessment of pancreatic hormone secretion from isolated porcine islets. Front. Endocrinol. 13:935060. doi: 10.3389/fendo.2022.935060
Received: 03 May 2022; Accepted: 25 July 2022;
Published: 11 August 2022.
Edited by:
Rita Bottino, Allegheny Health Network, United StatesReviewed by:
Beatriz Merino, University of Valladolid, SpainJian Ma, Harbin Medical University, China
Copyright © 2022 Mourad, Xhema and Gianello. This is an open-access article distributed under the terms of the Creative Commons Attribution License (CC BY). The use, distribution or reproduction in other forums is permitted, provided the original author(s) and the copyright owner(s) are credited and that the original publication in this journal is cited, in accordance with accepted academic practice. No use, distribution or reproduction is permitted which does not comply with these terms.
*Correspondence: Pierre Gianello, UGllcnJlLkdpYW5lbGxvQHVjbG91dmFpbi5iZQ==