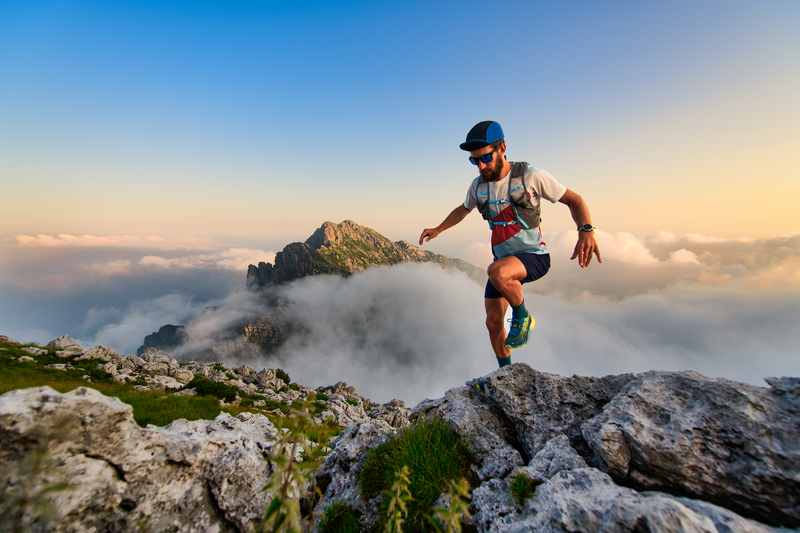
95% of researchers rate our articles as excellent or good
Learn more about the work of our research integrity team to safeguard the quality of each article we publish.
Find out more
REVIEW article
Front. Endocrinol. , 19 July 2022
Sec. Gut Endocrinology
Volume 13 - 2022 | https://doi.org/10.3389/fendo.2022.933110
This article is part of the Research Topic The Role of Bile Acid (BA) and Related Metabolites and Hormone Abnormalities in Metabolic Diseases View all 12 articles
A correction has been applied to this article in:
Corrigendum: Bone marrow mesenchymal stem cells in premature ovarian failure: Mechanisms and prospects
Polycystic ovary syndrome (PCOS) is a common disease, affecting 8%–13% of the females of reproductive age, thereby compromising their fertility and long-term health. However, the pathogenesis of PCOS is still unclear. It is not only a reproductive endocrine disease, dominated by hyperandrogenemia, but also is accompanied by different degrees of metabolic abnormalities and insulin resistance. With a deeper understanding of its pathogenesis, more small metabolic molecules, such as bile acids, amino acids, and short-chain fatty acids, have been reported to be involved in the pathological process of PCOS. Recently, the critical role of gut microbiota in metabolism has been focused on. The gut microbiota-related metabolic pathways can significantly affect inflammation levels, insulin signaling, glucose metabolism, lipid metabolism, and hormonal secretions. Although the abnormalities in gut microbiota and metabolites might not be the initial factors of PCOS, they may have a significant role in the pathological process of PCOS. The dysbiosis of gut microbiota and disturbance of gut metabolites can affect the progression of PCOS. Meanwhile, PCOS itself can adversely affect the function of gut, thereby contributing to the aggravation of the disease. Inhibiting this vicious cycle might alleviate the symptoms of PCOS. However, the role of gut microbiota in PCOS has not been fully explored yet. This review aims to summarize the potential effects and modulative mechanisms of the gut metabolites on PCOS and suggests its potential intervention targets, thus providing more possible treatment options for PCOS in the future.
Polycystic ovary syndrome (PCOS), characterized by oligo-ovulation or anovulation, hyperandrogenemia, and polycystic ovarian morphology, is a common disorder of the reproductive endocrine system, affecting 8%–13% of the women of reproductive age as well as impairing their fertility and long-term health (1, 2). Women with PCOS have a higher risk of infertility and pregnancy complications, accompanied by subsequent complications, such as obesity, type 2 diabetes, non-alcoholic fatty liver disease (NAFLD) (3), cardiovascular disease (4), endometrial cancer, and osteoporosis (5). All these complications have a far-reaching impact on the physical and mental health of women (6).
Until now, the specific etiology and pathophysiology of PCOS remain unclear. PCOS might be a polygenic heritable condition, which is affected by a variety of acquired variables (7). Hyperandrogenemia is generally regarded as the core part of PCOS, causing reproductive disorders, insulin resistance (IR), and metabolic imbalances, such as glucose and lipid metabolic imbalance (8). In particular, the IR and compensatory hyperinsulinemia might cause abnormality in the sex hormone levels, chronic inflammation, and metabolic disorders, thereby contributing to follicular dysplasia (9). These pathological factors create a vicious cycle, which increases the obstacles to PCOS treatment.
With a deeper understanding of gut biology, the potential role of the gut in PCOS has become the center of attention. Gut microbiota, also known as the “second genome” of the host, can affect the metabolism and immune response of the host by interacting with the external environment (8). Alpha-diversity (α-diversity) is regarded as an indicator of ecosystem health, representing the number of species present in the given community, whereas beta-diversity (β-diversity) denotes the similarity of a community or individual sample with another community or individual sample, respectively (10). As compared to the normal group, the dysbiosis of gut microbiota in the PCOS women showed lower α- and β-diversities, decreased relative abundance of Bifidobcterium, and increased relative abundances of Bacteroides, Parabacteroides, and Clostridium (11–13). Furthermore, the dehydroepiandrosterone (DHEA)–induced PCOS rats showed the dysbiosis of gut microbiota, and transferring this microbiota to healthy rats could induce the PCOS-like metabolic and endocrinal dysfunctions, indicating that the gut might be a novel therapeutic target for the treatment of PCOS (14).
Recently, studies on gut metabolites have emphasized the importance of the gut in maintaining general homeostasis. The gut metabolites, such as bile acids (BAs), amino acids, and short-chain fatty acids (SCFAs), are greatly involved in modulating the integrity of the gut barrier, thereby maintaining the internal environment and homeostasis. A disturbance in gut metabolites might increase the gut permeability, leading to the leakage of lipopolysaccharides (LPSs) and endotoxemia, which might disturb the endocrine system, immune system, insulin signaling, glucose metabolism, lipid metabolism (8), and gut microbiota (15). Furthermore, the SCFAs, BAs, and branched-chain amino acids (BCAAs) can directly regulate the secretion and sensitivity of pancreatic insulin in the target organs through endocrine signaling. While circulating through the portal venous system, these metabolites reach the liver to regulate lipid metabolism and oxidation. Moreover, these metabolites also take part in neuronal homeostasis by modulating the integrity of the blood–brain barrier (16). The gut–brain peptides, which can be affected by gut metabolites, might communicate with the brain, thereby influencing appetite and energy maintenance as well as increasing the secretion of luteinizing hormone (LH) (17).
Interestingly, the activity and contents of gut metabolites can be regulated by the gut microbiota (18, 19). The correlations between gut metabolites and gut microbiota have been demonstrated in numerous metabolic diseases, such as obesity, type 2 diabetes, NAFLD, and cardiovascular diseases (20, 21). Qiao and colleagues also demonstrated that an increase in the relative abundance of Bacteroides in patients with PCOS was related to the disturbance in gut metabolites, which might have a potential pathological role in PCOS (13, 22).
These studies indicate that, in PCOS, the gut microbiota and related metabolites might be affected. They both are closely linked to the insulin signaling pathway, steroid hormone levels, glucose metabolism, lipid metabolism, and immunological homeostasis, all of which are greatly involved in the pathogenesis of PCOS (16, 17). However, understanding the mechanism of interactions between gut metabolites and PCOS is still unclear. This review aims to summarize the existing studies and demonstrates the interaction between PCOS and gut microbiota-related metabolites, which might help in developing novel treatments for PCOS.
BAs are the key metabolites, which include primary BAs and secondary BAs. In humans, cholic acid (CA) and chenodeoxycholic acid (CDCA) are the most common primary BAs. Under normal physiological conditions, these primary BAs are synthesized from cholesterol in the pericentral hepatocytes through “classical” (neutral) and “alternative” (acidic) pathways (23, 24). The classical pathway favors the biosynthesis of CA and CDCA (25), whereas the alternative pathway only favors the biosynthesis of CDCA. After the primary BAs are modified and transported by various enzymes and transporters, they are conjugated with taurine or glycine and secreted into the bile, which are then released into the small intestine and aid in lipid digestion (26). In the ileocecum, the gut microbiota and bile salt hydrolase (BSH) convert the conjugated BAs to free BAs. Following the modifications, such as the removal, oxidation, or epimerization of the nuclear hydroxyl by the host or gut microbiota, the free primary BAs are converted into secondary BAs (25, 27). The secondary BAs play a critical role in regulating glucose metabolism, insulin signaling, lipid metabolism, and inflammation. In the distal ileum, most of the secreted molecules (95%) are reabsorbed through apical sodium-dependent BA transporter (ASBT) and are ultimately transported into the liver through the portal vein system. This phenomenon is known as enterohepatic circulation. Meanwhile, the remaining secreted molecules are excreted in feces (15, 25). In the ileum, BAs facilitate the secretion of fibroblast growth factor 19 (FGF19) in humans or FGF15 in mice by activating the farnesoid X receptor (FXR). FGF19 further represses the BA synthesis as negative feedback when circulated to the liver (26).
The above process relies heavily on gut microbiota. The gut microbiota can affect the production of BAs by regulating the liver enzymes, such as 7a hydroxylase and sterol-27-hydroxylase, especially CDCA in humans (28). BSH has been widely detected in rodent and human gut microbiota, such as Clostridium spp (29).. The diversity of secondary BAs is greatly affected by the species differences in gut microbiota (23). The mouse models in the absence of gut microbiota demonstrate that almost all the BAs were primary BAs, indicating the importance of gut microbiota in the production of free BAs (15, 30). The gut microbiota could affect the ileum mucosa and ASBT to regulate the reabsorption of BA in rodents (28). Moreover, the gut microbiota partially inhibits the BA biosynthesis through the FXR-dependent mechanism (31–33). The BAs shape the structure of gut microbiota and exert antibacterial effects by selectively promoting the growth of BA-synthesizing bacteria, thereby showing a bidirectional communication between the gut microbiota and BAs (15, 33).
One of the most important functions of BAs is their participation in lipid emulsification and solubilization. They convert fat into fat droplets, which can be digested by trypsin and absorbed by gut mucosa, thereby assisting the absorption of dietary fat, which is critically important for lipid metabolism (34). Certain essential vitamins, such as vitamins A and D, are non-polar lipids, which can only be absorbed if bound to micelles in the presence of BAs (25, 35). Whenever the concentration of cholate is lower, cholesterol absorption is inhibited (25).
The BAs modulate metabolic homeostasis by stimulating the receptors, such as G protein receptor 5 (TGR5) and FXR. TGR5 is widely distributed in a variety of animal tissues, such as fat, central nervous system, liver, and gut and participates in regulating insulin signaling, glucose metabolism, and energy expenditure in brown adipose tissue and muscle (36). The intestinal hormone glucagon-like peptide 1 (GLP-1) and peptide YY (PYY) are simulated by TGR5 (37). In the murine brain, BAs could activate TGR5, causing the central anorexigenic actions to control the appetite (38). FXR is another BAs receptor, which is found in white adipose tissue, liver, gut, immune cells, and other tissues (39). The BAs, in combination with FXR, can induce FGF15 and/or FGF19, which might regulate glucose tolerance and normal glycemia by reducing hepatic gluconeogenesis. A reduction in the number of activated FXR might reduce the secretion of FGF15 and/or FGF19. This might result in the increase of hepatic gluconeogenesis, deposition of hepatic lipid, and disruption of glucose homeostasis in adipocytes and the decrease of insulin production in pancreatic cells (26, 32). Moreover, in the cardiac and visceral fat cells, tauroursodeoxycholic acid (TUDCA) could reduce endoplasmic reticulum stress, thereby preventing obesity and inflammation (40). Therefore, reduction in the TUDCA might result in the diminished suppression of abdominal and visceral fat inflammation, aggravating the IR and metabolic disorders (8, 40).
However, the current studies on the link between BAs and metabolic syndrome include a few individuals in the BAs pool. The levels of fasting circulating total BAs were higher among the populations with mild IR and obesity (25). However, the changes in the levels of fasting circulating BAs in disease states as well as the role of each BA in metabolic diseases are needed to be investigated.
Recently, the crosstalk between SCFAs and gut has been focused on. The SCFAs originated from the microbiota-accessible carbohydrates (MACs) in the colon (41), which are fermented from the dietary fibers and resistant starch ferment. They mainly consist of acetic, propionic, butyric, valeric, and caproic acids, which are biosynthesized in various pathways, such as the Wood–Ljungdahl pathway, aided by the different classes of gut microbes (42). The exact contents and relative proportion of each type of SCFA might differ based on the diet, composition of the microbiota, and gut transit time (43, 44). When the BCAAs, including valine, isoleucine, and leucine, escape digestion in the upper gut, they might be fermented into branched-chain fatty acids (43, 44). Furthermore, the SCFAs are taken up by colonocytes via passive diffusion or active transport (42). A part of the unmetabolized SCFAs are transported into the liver through the portal system and serve as substrates for the energy metabolism and anabolic processes, thereby playing a prominent role in the inhibition of glycolysis, stimulation of lipogenesis and gluconeogenesis, and regulation of mitochondrial energy production (45).
SCFAs are important for balancing metabolism and energy. They are taken up by colon cells after binding to G protein–coupled receptors (GPCRs), which are also known as free fatty acid receptors (FFARs) and are present on the enteroendocrine cells of the gastrointestinal mucosa (46), thereby stimulating the secretion of intestinal hormones, such as GLP-1, PYY, gamma-aminobutyric acid (GABA), and serotonin (5-HT) (46). The intestinal hormones aid in reducing the production of hepatic glucose, enhancing the absorption of peripheral glucose, and suppressing the appetite (47). Moreover, the SCFAs can also stimulate leptin secretion in adipocytes and insulin secretion in the pancreatic cells (48). The circulating SCFAs can activate the burning of brown adipose tissue, thereby increasing energy consumption and preventing weight gain (43, 49). In addition, the SCFAs also improve insulin sensitivity in the muscle and liver tissues (47).
In contrast to hepatic gluconeogenesis, intestinal gluconeogenesis (IGN) is beneficial for controlling the glucose level by reducing food intake and hepatic glucose output (47, 49). In a study based on mouse models, butyrate could directly promote the IGN expression in enterocytes in a Cyclic Adenosine Monophosphate (cAMP)-dependent manner, whereas propionate could increase the IGN expression by binding to FFAR3 in the portal nerve, thereby initiating the portal-hypothalamic crosstalk, improving the insulin sensitivity and glucose tolerance, and lowering the fat mass (47).
Recently, studies have demonstrated that SCFAs could affect the host’s immune system. SCFAs could affect the hematopoietic progenitors in the murine bone marrow, implying that they were important for the development of innate and adaptive immune systems (50). Moreover, they exerted a systematic anti-inflammatory effect in mice by affecting the peripheral DCs and T cells (51). In particular, the SCFAs increased the number of T-regulatory (Treg) cells, induced the differentiation of Treg cells, and regulated the production of interleukin, thereby minimizing the oxidative stress and protecting pancreatic cells (52–54). In a murine model of gout, SCFAs could bind to the GPCR43 in the central nervous system and act on microglia to regulate host immunity (55). Furthermore, they strengthened the integrity of the blood–brain barrier and regulated the levels of neuronal factors and neurogenesis to relieve the neural and central inflammation (52).
Moreover, SCFAs can also increase the expression of intestinal epithelial tight junction protein and decrease the death of intestinal epithelial cells (IEC), thereby promoting gut mucosal immunity and barrier integrity (56, 57). Once the intestinal mucosal barrier is disrupted, LPS enters the blood circulation, resulting in a persistent inflammation, which is correlated with IR (51).
The SCFAs, when reaching the brain, can alter the integrity of the blood–brain barrier by increasing the expression of tight junction proteins in the blood–brain barrier and regulating the state of neural and central inflammation (41, 52). SCFAs also affect the function of glial cells and neurogenesis in order to maintain neuronal homeostasis (41).
Amino acids, consisting of essential and non-essential amino acids, are life-supporting molecules, which provide raw materials for protein synthesis. The food amino acids are primarily absorbed in the small intestine via the concentrative amino acid transporters. A small number of amino acids are also absorbed by the large intestine, whereas the remaining are excreted in the feces (58, 59). Then, the amino acids are released primarily through passive efflux across the basolateral membrane, which is mediated by a group of transporters (59, 60). When released into the bloodstream, they are transported into the cells via the corresponding secondary active transporters, which are also called functional transporters (61). Simultaneously, an increase in the cytoplasmic amino acid pool activates the amino acid metabolism, forcing the excess amino acids to be catabolized via oxidation, hydroxylation, and other processes (62). The majority of amino acids are metabolized and restored in the liver (58). However, they may also be stored in extrahepatic tissues, such as muscle, brown fat, kidneys, liver, and heart tissues (62); this storage in extrahepatic tissues is regulated by the insulin-mediated signaling in the hypothalamus (63).
In addition to synthesizing proteins, amino acids are also involved in glycolysis and mitochondrial metabolism through the tricarboxylic acid (TCA) cycle and oxidative phosphorylation and modulate the cellular activities, such as lipid and glucose metabolism (64). By acting on the IR substrates (IRSs), the amino acids can affect insulin signaling (65). Furthermore, recent studies have demonstrated that amino acids are the potential precursors of the brain neurotransmitter, impacting habits (66). Furthermore, amino acids participate in ATP generation, nucleotide synthesis, and redox balance, which support the growth, proliferation, and effector function of immune cells (64, 67).
To date, significant differences have been reported between the gut microbiota and metabolites in patients with PCOS as compared to the control group. PCOS, as a multi-system endocrine disease, has a negative impact on the function and composition of gut microbiota and metabolites.
According to studies, sex hormones have a substantial impact on the composition of the gut microbiota. They affect the composition of gut microbiota in a sex-specific manner after puberty (10, 68). As a result, the gut microbiota in females has higher α-diversity but significantly lower abundances of Bacteroides species, including Prevotella and Bacteroides thetaiotomicron, as compared to that of males. The studies of rodents and other species have also shown similar results, but the outcomes vary across the studies (68, 69). Meanwhile, the dysbiosis of gut microbiota in the PCOS women was characterized by the lower α-diversity, decreased relative abundance of Bifidobacterium, increased relative abundance of Bacteroides, and changes in the β-diversity as compared to the control group (11–13). This showed that the gut microbiota of the PCOS women altered when compared to that of the men. Although different conclusions have been presented, the accumulating data confess that hyperandrogenism might affect the gut microbiota of patients with PCOS by affecting the gut function and regulating the activity of β-glucuronidase and its substrate levels, such as bilirubin, neurotransmitters, and hormones, which are present in the liver (10, 11, 70). Because the gut metabolites are closely related to the gut microbiota, the changes in gut microbiota in response to hormones might also change the gut metabolites. For example, Sherman et al. revealed that the prenatal androgens were linked to the changes in the abundance of gut microbiota involved in the production of SCFAs in the rat (71). In a nutshell, the disruption of sex hormones in PCOS affects the composition of gut metabolites and microbiota.
The dysbiosis of gut microbiota is correlated with the phenotype of PCOS. There are differences in the gut microbiota of non-obese and obese individuals with PCOS (17). The abundance of clostridium cluster XVII increased in the non-obese patients with PCOS, whereas that of Clostridium sensustricto and Roseburia decreased (72). Liu et al. reported that the relative abundances of gut microbiota, including Bacteroides, Escherichia/Shigella, and Streptococcus increased, whereas those of Akkermansia and Ruminococcaceae decreased in the patients with PCOS, which were correlated with body mass index (BMI) (17). It has also been reported that obese women with PCOS tend to have lower α-diversity and biodiversity of the gut microbiota as compared to the women with normal BMI (69). Furthermore, according to Li and colleagues, obesity was associated with the altered BA metabolism caused by the dysbiosis of gut microbiota (21). Because adiposity is a source of sex steroids, it might affect the composition of gut microbiota and gut metabolites by affecting the production of sex hormones (73). In addition, obesity might contribute to the development of a chronic inflammatory state, which might alter the gut permeability and microbiota, thereby affecting the function and composition of gut metabolites (21, 74).
Among the women with IR, the relative abundance of Bacteroidaceae increased, whereas that of Prevotellaceae decreased as compared to the PCOS women without IR (71). The IR-induced gut dysbiosis could result in the accumulation of BCAAs (13, 36, 75, 76). On the other hand, the TCA cycle was significantly inhibited by IR, resulting in decreased BCAA clearance (77). Furthermore, IR and compensatory hyperinsulinism contributed to hyperandrogenism, thereby disrupting the gut dysbiosis and metabolites (8, 10).
Numerous patients with PCOS have bad habits, such as an adoration of sweets, a love of fat, an absence of dietary fiber, and little exercise, which affects gut health (18, 78). A high-fat diet (HFD) was linked to an increase in the pro-inflammatory microbiota, such as Clostridiales, Bacteroides, and Enterobacteriales, and a decrease in the anti-inflammatory microbiota, such as Lactobacillus, in the rat (75). The high levels of glucose, fructose, and sucrose could increase the relative abundance of Bifidobacteria while suppressing that of Bacteroides (75). The lack of dietary fiber might result in a decrease in the production of SCFAs. All these could affect the biosynthesis of gut metabolites.
Exercise can enhance gut health by increasing the diversity of gut microbiota and balancing the beneficial and pathogenic bacterial communities (79). Specifically, exercise increases the ratio of butyrate-producing bacteria, such as Roseburia hominis, thereby increasing the concentration of butyrate (80, 81). Moreover, exercise can reduce the contact time between feces and the gastrointestinal mucus layer by enhancing gastrointestinal motility to benefit gut health (79, 80). It also boosts the production of key antioxidant enzymes and anti-inflammatory cytokines in the intestinal lymphocytes, thereby reducing intestinal inflammation (76, 77). A reduction in exercise might disrupt the gut metabolites (43, 44, 79).
In a nutshell, the dysbiosis of gut microbiota is caused by unhealthy habits, hyperandrogenism, obesity, hyperinsulinism, and disturbances in the glucose and lipid metabolism in PCOS, leading to increased gut permeability, exaggerated dysbiosis, and altered gut metabolites (82, 83).
The BA metabolism is a key metabolic pathway affected by the changes in gut microbiota in patients with PCOS. Zhang and colleagues demonstrated that an increase in the circulating conjugated primary BAs was positively correlated with hyperandrogenism in women with PCOS (84). In both the stool and serum, the levels of secondary BAs, such as glycodeoxycholic acid (GDCA) and TUDCA, were lower in the PCOS group as compared to those in the control group and were correlated with the disturbance of gut microbiota (13).
The findings from PCOS rats revealed that UDCA administration could improve ovarian morphology and decrease the total testosterone and insulin levels. However, the lipid parameters, E1, E2, glucose, and homeostatic model assessment for IR were comparable between the groups (85).
Moreover, BAs could also regulate the performance of gut immune cells. Both the protein and mRNA levels of Interleukin-22 (IL-22) in the cultured group 3 innate lymphoid cells (ILCs) were greatly stimulated in the presence of TUDCA or GDCA, which was also confirmed in mouse models, showing that TUDCA or GDCA therapy could enhance the mRNA levels of gut IL-22 and alleviate the disease symptoms (13). These beneficial effects of BAs on IR and ovarian function in PCOS mice were reversed by knocking out the IL-22 receptor gene (13). The IL-22 levels in serum and follicle fluid of patients with PCOS decreased, whereas the IL-22 administration could improve IR, ovarian dysfunction, dysbiosis of gut microbiota, and prenatal Müllerian hormone in the DHEA-induced PCOS mice (86). Therefore, it could be proposed that the regulatory effects of BAs on PCOS were at least partially mediated by IL-22 (13).
IL-22 has diverse benefits, such as improving insulin sensitivity and regulating the lipid metabolism in the liver and adipose tissues. IL-22 can promote the proliferation of IEC and the production of antimicrobial peptides and mucins in IEC (13, 51). Therefore, a reduction in the IL-22 level might further disrupt the integrity of the gut barrier and microbiome hemostasis, thereby aggravating the endotoxemia, chronic inflammation state, and particularly the IR (13, 87, 88). Recently, Qi et al. reported that IL-22 could reverse the disturbed menstrual cycle in PCOS mice by relieving the inflammatory state in ovarian granulosa cells, further indicating the possible role of IL-22 in the PCOS intervention (86).
SCFAs are essential elements in maintaining the homeostasis of gut microbiota and regulating the intestinal mucosal barrier as an important energy source for the gut microbiota and IEC (51, 89). For instance, butyrate regulates the utilization of intestinal oxygen, thereby regulating the proportion of aerobic and anaerobic gut microbiota. Therefore, a reduction in the SCFA levels might cause gut dysbiosis in PCOS (90) and disrupt the intestinal mucosal barrier to exacerbate the chronic inflammatory state (51).
In addition to affecting the gut health and microbiota, the SCFAs also exert various physiological effects through IL-22. SCFAs could promote the IL-22 production in CD4+ T cells and ILCs by binding to the histone deacetylase inhibitors and GPCRs (89). The IL-22 could maintain metabolic homeostasis, which was disturbed by SCFAs reduction (89), thereby producing a synergistic effect with BA–IL-22. In addition, studies reported that the secretion of gastrointestinal hormones in patients with PCOS was disturbed, such as a decrease in the GLP-1 level (91). SCFAs could stimulate the secretion of gastrointestinal hormones, such as GLP-1, PYY, GABA, and 5-HT, thereby reversing their decreased levels in the patients with PCOS, maintaining insulin homeostasis, and suppressing the appetite (46).
In addition to their effects on the gut, SCFAs also exert peripheral effects. SCFAs could promote the IGN gene expression in enterocytes through the portal-hypothalamic circuit, thereby maintaining food intake and hepatic glucose output in mice (49). The decrease in the SCFA levels can boost insulin secretion from the pancreatic cells via GPCRs, improve insulin sensitivity, increase the energy expenditure in brown adipose tissue, and upregulate the antilipolytic activity of glucose transporter type 4, thereby further aggravating the PCOS (41, 45, 47).
Moreover, Lin et al. discovered that the absorption of SCFAs decreased in the PCOS rats. They demonstrated that the fecal SCFA concentrations increased and were positively correlated with the tumor necrosis factor and IL-6 levels (92). Enhancing the SCFA absorption could improve the integrity of the intestinal mucosal barrier and inhibit intestinal and parenteral inflammation (92).
Therefore, SCFAs are critically important for maintaining glucose and insulin homeostasis and ameliorating chronic inflammation throughout the body. The supplementation of SCFAs or enhancing their beneficial effects, such as activating the relevant receptors, might be helpful in the PCOS treatment.
Recently, the correlations between BCAAs and metabolic dysbiosis have been focused on. The human body cannot synthesize BCAAs, which are essential amino acids; therefore, they must be absorbed from the digestion of food (65). By phosphorylating the IRS-1 and IRS-2 at serine or damaging the mitochondrial function in the β-pancreatic cells, excessive BCAAs could aggravate IR in rodents with PCOS (65, 88, 93). Furthermore, BCAAs might induce the expression of proinflammatory genes to deteriorate chronic inflammation, thereby developing IR (65).
As compared to the SCFAs and BAs, the current studies on intestinal amino acids are limited. Moreover, the conclusions are not completely consistent due to the inter- and intra-species differences and experimental conditions. The correlations between amino acids and PCOS are still unclear. However, it could be concluded that downregulating the excessive BCAAs or blocking their associated binding sites might further ameliorate IR in PCOS. Whether and how BCAAs can be applied for the prediction and treatment of PCOS are worth exploring.
Currently, the primary goal of PCOS treatment is to alleviate its symptoms, such as hyperandrogenism, IR, oligo- or anovulation, and infertility. For example, letrozole aids in developing the dominant follicles, whereas metformin is typically for the treatment of metabolic symptoms and IR, possibly restoring their ovulation (94). These treatment strategies can only provide temporary relief from the symptoms or achieve a short-term goal. Therefore, the fundamental and permanent treatment of the pathological processes in PCOS is worth exploring.
As stated above, the PCOS-related hyperandrogenism, IR, obesity, metabolic disturbance, unhealthy diet, and other factors could disrupt the gut microbiota and metabolites, which, in turn, deteriorated the pathological process of PCOS, forming a vicious cycle (95) (Figure 1).
Figure 1 Crosstalk between PCOS and gut metabolites. PCOS, polycystic ovary syndrome; BAs, bile acids; SCFAs, short-chain fatty acids; BCAAs, branched-chain amino acids; IGN, intestinal gluconeogenesis; LPS, lipopolysaccharide; GLP-1, glucagon-like peptide 1; PYY, peptide YY; 5-HT, 5-hydroxytryptamine; LH, luteinizing hormone; SHBG, sex hormone–binding globulin. PCOS disturbs intestinal microbial homeostasis and metabolites, which may be linked to the insulin signaling pathway, steroid hormone levels, glucose metabolism, lipid metabolism, and immunological homeostasis etc., all of which are involved in PCOS pathogenesis, thus forming a vicious cycle.
Interestingly, the changes in gut metabolites could predict PCOS (23, 96) and might even be associated with the different clinical phenotypes (17). The gut metabolites could be more precise predictors than the gut microbiota due to the susceptibility of gut microbiota to a variety of factors, such as environmental contamination and abrupt changes in diet.
The therapies, targeting the gut homeostasis to break the vicious circle between hyperandrogenism and metabolic abnormalities, can be the tipping point for the treatment of PCOS. For example, adopting a healthier lifestyle; supplementing the specific BAs (TDUCA and GDCA), SCFAs, and IL-22; regulating the metabolism of amino acids; and blocking the BCAA targets could be beneficial for the treatment of PCOS (89, 95). It has been reported that the IL-22 levels in patients with PCOS were significantly lower than those in the normal group (22). Therefore, the IL-22 supplementation could be an effective treatment option for PCOS. Studies on the PCOS mice confirmed that the intraperitoneal injection of IL-22 could improve endocrine and metabolic disorders (13). However, there were certain side effects, such as liposarcoma (97). On the other hand, there is limited clinical evidence, supporting the efficiency and safety of IL-22 in humans. Thus, safer therapies are needed to be developed as soon as possible.
The probiotics (or synbiotics) supplementation and fecal microbiota transplantation (FMT) might have a significant impact in this regard. Studies indicated that the administration of probiotics (or synbiotics) for 8–12 weeks could lower serum levels of glucose, insulin, triglycerides (TGs), very low–density lipoprotein, and cholesterol while improving the IR, lipid metabolic disturbance, and inflammatory state. It could also effectively lower the body weight and BMI of patients with PCOS (98–100). Nevertheless, a meta-analysis showed that the effects of probiotics (or synbiotics) supplementation on LDL, weight, BMI, and IR were not significant (101). However, probiotics had certain effects on regulating the metabolisms of glucose, insulin, and lipids, which could lower the serum levels of glucose, insulin, and TG while increasing HDL (101). Another study indicated that the supplementation of probiotics (or synbiotics) improved androgen metabolism without having any other therapeutic effect (102). The administration of different probiotic species and doses to the patients with varied PCOS phenotypes might explain the differences in these outcomes. In rodents, FMT could treat PCOS by restoring the composition of gut microbiota and improving the sex hormone balance and ovarian function (103). However, currently, there are limited applications of FMT in the treatment of PCOS. Therefore, the use of FMT in humans is yet to be determined.
The results showed that the supplementation of insulin-enriched synbiotic yogurt to the PCOS mice could decrease the body weight gain, improve estrus cycles and ovary morphology, and reduce the levels of LH while increasing those of follicle-stimulating hormone and IL-22 in serum. At the genus level, the synbiotic yogurt increased the relative abundances of Lactobacillus, Bifidobacterium, and Akkermansia (104).
Traditional Chinese medicines (TCMs) are vast treasure, which need to be explored. The TCMs, including flavonoids, polysaccharides, saponins, and other compounds, might possess tremendous prospects for stimulating the growth of a particular gut microbial species, increasing the production of beneficial SCFAs and BAs, and suppressing the growth of pathogenic bacteria and BCAA products (94, 105, 106). Berberine, a powerful natural product, which is used for the treatment of metabolic syndrome, could lower the abundance of BCAA-producing bacteria and aberrant blood BCAA levels in the HFD-induced rodents, thereby improving the glucose metabolism, lipid metabolism, and IR in the PCOS rodents (107, 108). Studies on the rodents demonstrated that baicalin could boost the SCFA generation and alter the BA metabolism by modifying the immunology and gut microbiota, as well as affecting the liver–gut axis by regulating the BA-FXR/TGR5 signaling pathway (109). Ginseng polysaccharides and ginsenosides could also boost the growth of Lactobacillus spp. and Bacteroides spp. in the rat. These two were the most significantly boosted probiotics, restoring the balance of gut microbiota and thereby regulating intestinal metabolism (110). Thus, the TCM and natural products might affect the gut immunity, barrier, and gut microbiota to modulate the local metabolisms. Although studies have revealed that some TCM products have low bioavailability, it is remarkable that gut microbes can transform them into components, which can be absorbed more easily, thereby improving their efficiency and indicating the positive interaction between TCM and gut microbiota (94, 111–113).
Adopting a healthier lifestyle might also improve PCOS, especially the exercise and diet, which act as the modulators of gut microbiota. As mentioned before, bad habits such as an adoration of sweets, a love of fat, an absence of dietary fiber, and little exercise, might disrupt gut health. As compared to the Western diet (enriched in animal protein and fat and low in fiber), gluten-free diet, vegetarian diets (high in fermentable plant-based foods), etc., the Mediterranean diet is more recommended for the patients with PCOS (114). Numerous human or rodent studies have demonstrated that the Western diet could significantly decrease the abundance of total and beneficial bacteria species, including Bifidobacterium and Eubacterium (115). The beneficial bacterial populations, such as Bifidobacterium and Lactobacillus, decreased, whereas those of potentially harmful bacteria increased in the human who consumed a gluten-free diet (116). The results of various studies on vegetarian diets are contradictory. In general, the Mediterranean diet is characterized as a healthy and balanced diet, which can improve obesity, lipid profile, and inflammation (114, 117). Specifically, the assorted fruits, vegetables, nuts, legumes, and cereals are recommended, whereas the intake of red meat, processed meat, and sweets should be limited (114). Exercise can enhance gut health by increasing the diversity of gut microbiota and balancing the beneficial and pathogenic bacterial communities, and a minimum of 150-min exercise of moderate intensity per week is necessary for patients with PCOS (1).
The studies on gut health and PCOS are still in the initial stages, which limit the scope of this review. On one hand, this review mainly focused on the interactions of PCOS with the SCFAs, BAs, and amino acids. Nevertheless, there might be additional metabolic loops closely associated with the PCOS, such as carnitine metabolism (118). The amino acids, other than BCAAs, are also needed to be thoroughly investigated in the future. On the other hand, there might be some changes in the synthesis and transit of gastrointestinal metabolites between species. Therefore, the findings from animal studies remain to be validated in humans.
MZ and YS contributed to the conception of this review. RH wrote the manuscript. RH, MZ, and YS revised the manuscript. RH and YH designed and illustrated the figures. YH, FZ, FL, ZL, and YG performed the literature search and interpretation. MZ, RH, YH, FZ, FL, ZL, YG, HD, WM, KS, and YS reviewed the manuscript. All authors approved the submission.
The authors declare that the research was conducted in the absence of any commercial or financial relationships that could be construed as a potential conflict of interest.
All claims expressed in this article are solely those of the authors and do not necessarily represent those of their affiliated organizations, or those of the publisher, the editors and the reviewers. Any product that may be evaluated in this article, or claim that may be made by its manufacturer, is not guaranteed or endorsed by the publisher.
Thanks to BioRender. The figure is created with BioRender.com.
1. Teede HJ, Misso ML, Costello MF, Dokras A, Laven J, Moran L, et al. Recommendations From the International Evidence-Based Guideline for the Assessment and Management of Polycystic Ovary Syndrome. Fertil Steril (2018) 110(3):364–79. doi: 10.1016/j.fertnstert.2018.05.004
2. Liao B, Qiao J, Pang Y. Central Regulation of Pcos: Abnormal Neuronal-Reproductive-Metabolic Circuits in Pcos Pathophysiology. Front Endocrinol (Lausanne) (2021) 12:667422. doi: 10.3389/fendo.2021.667422
3. Azziz R, Carmina E, Chen Z, Dunaif A, Laven JS, Legro RS, et al. Polycystic Ovary Syndrome. Nat Rev Dis Primers (2016) 2:16057. doi: 10.1038/nrdp.2016.57
4. Lizneva D, Suturina L, Walker W, Brakta S, Gavrilova-Jordan L, Azziz R. Criteria, Prevalence, and Phenotypes of Polycystic Ovary Syndrome. Fertil Steril (2016) 106(1):6–15. doi: 10.1016/j.fertnstert.2016.05.003
5. Rosenfield RL. The Diagnosis of Polycystic Ovary Syndrome in Adolescents. Pediatrics (2015) 136(6):1154–65. doi: 10.1542/peds.2015-1430
6. Han Q, Wang J, Li W, Chen ZJ, Du Y. Androgen-Induced Gut Dysbiosis Disrupts Glucolipid Metabolism and Endocrinal Functions in Polycystic Ovary Syndrome. Microbiome (2021) 9(1):101. doi: 10.1186/s40168-021-01046-5
7. De Leo V, Musacchio MC, Cappelli V, Massaro MG, Morgante G, Petraglia F. Genetic, Hormonal and Metabolic Aspects of Pcos: An Update. Reprod Biol Endocrinol (2016) 14(1):38. doi: 10.1186/s12958-016-0173-x
8. Giampaolino P, Foreste V, Di Filippo C, Gallo A, Mercorio A, Serafino P, et al. Microbiome and Pcos: State-Of-Art and Future Aspects. Int J Mol Sci (2021) 22(4):2048. doi: 10.3390/ijms22042048
9. Rajska A, Buszewska-Forajta M, Rachoń D, Markuszewski MJ. Metabolomic Insight Into Polycystic Ovary Syndrome-An Overview. Int J Mol Sci (2020) 21(14):4835. doi: 10.3390/ijms21144853
10. Thackray VG. Sex, Microbes, and Polycystic Ovary Syndrome. Trends Endocrinol Metab (2019) 30(1):54–65. doi: 10.1016/j.tem.2018.11.001
11. Insenser M, Murri M, Del Campo R, Martínez-García M, Fernández-Durán E, Escobar-Morreale HF. Gut Microbiota and the Polycystic Ovary Syndrome: Influence of Sex, Sex Hormones, and Obesity. J Clin Endocrinol Metab (2018) 103(7):2552–62. doi: 10.1210/jc.2017-02799
12. Zhang J, Sun Z, Jiang S, Bai X, Ma C, Peng Q, et al. Probiotic Bifidobacterium Lactis V9 Regulates the Secretion of Sex Hormones in Polycystic Ovary Syndrome Patients Through the Gut-Brain Axis. mSystems (2019) 4(2):e00017-19. doi: 10.1128/mSystems.00017-19
13. Qi X, Yun C, Sun L, Xia J, Wu Q, Wang Y, et al. Gut Microbiota-Bile Acid-Interleukin-22 Axis Orchestrates Polycystic Ovary Syndrome. Nat Med (2019) 25(8):1225–33. doi: 10.1038/s41591-019-0509-0
14. Garcha D, Walker SP, MacDonald TM, Hyett J, Jellins J, Myers J, et al. Circulating Syndecan-1 Is Reduced in Pregnancies With Poor Fetal Growth and Its Secretion Regulated by Matrix Metalloproteinases and the Mitochondria. Sci Rep (2021) 11(1):16595. doi: 10.1038/s41598-021-96077-1
15. Wahlström A, Sayin SI, Marschall HU, Bäckhed F. Intestinal Crosstalk Between Bile Acids and Microbiota and Its Impact on Host Metabolism. Cell Metab (2016) 24(1):41–50. doi: 10.1016/j.cmet.2016.05.005
16. Wang L, Zhou J, Gober HJ, Leung WT, Huang Z, Pan X, et al. Alterations in the Intestinal Microbiome Associated With Pcos Affect the Clinical Phenotype. BioMed Pharmacother (2021) 133:110958. doi: 10.1016/j.biopha.2020.110958
17. Liu R, Zhang C, Shi Y, Zhang F, Li L, Wang X, et al. Dysbiosis of Gut Microbiota Associated With Clinical Parameters in Polycystic Ovary Syndrome. Front Microbiol (2017) 8:324. doi: 10.3389/fmicb.2017.00324
18. Zheng YH, Xu Y, Ma HX, Liang CJ, Yang T. Effect of High-Fat Diet on the Intestinal Flora in Letrozole-Induced Polycystic Ovary Syndrome Rats. Evid Based Complement Alternat Med (2021) 2021:6674965. doi: 10.1155/2021/6674965
19. Wang T, Sha L, Li Y, Zhu L, Wang Z, Li K, et al. Dietary α-Linolenic Acid-Rich Flaxseed Oil Exerts Beneficial Effects on Polycystic Ovary Syndrome Through Sex Steroid Hormones-Microbiota-Inflammation Axis in Rats. Front Endocrinol (Lausanne) (2020) 11:284. doi: 10.3389/fendo.2020.00284
20. Zhang C, Fang R, Lu X, Zhang Y, Yang M, Su Y, et al. Lactobacillus Reuteri J1 Prevents Obesity by Altering the Gut Microbiota and Regulating Bile Acid Metabolism in Obese Mice. Food Funct (2022) 13(12):6688–6701. doi: 10.1039/d1fo04387k
21. Li R, Andreu-Sánchez S, Kuipers F, Fu J. Gut Microbiome and Bile Acids in Obesity-Related Diseases. Best Pract Res Clin Endocrinol Metab (2021) 35(3):101493. doi: 10.1016/j.beem.2021.101493
22. Qi X, Nie Q, Pang Y, Qiao J. IL-22 and Its Interaction With Amino Acid and Glycolipid Metabolite in Polycystic Ovary Syndrome (Pcos) Patients. Chin Med J (2021). doi: 10.1097/cm9.0000000000001915
23. Li J, Dawson PA. Animal Models to Study Bile Acid Metabolism. Biochim Biophys Acta Mol Basis Dis (2019) 1865(5):895–911. doi: 10.1016/j.bbadis.2018.05.011
24. Russell DW. The Enzymes, Regulation, and Genetics of Bile Acid Synthesis. Annu Rev Biochem (2003) 72:137–74. doi: 10.1146/annurev.biochem.72.121801.161712
25. McGlone ER, Bloom SR. Bile Acids and the Metabolic Syndrome. Ann Clin Biochem (2019) 56(3):326–37. doi: 10.1177/0004563218817798
26. Liu H, Hu C, Zhang X, Jia W. Role of Gut Microbiota, Bile Acids and Their Cross-Talk in the Effects of Bariatric Surgery on Obesity and Type 2 Diabetes. J Diabetes Investig (2018) 9(1):13–20. doi: 10.1111/jdi.12687
27. Ridlon JM, Harris SC, Bhowmik S, Kang DJ, Hylemon PB. Consequences of Bile Salt Biotransformations by Intestinal Bacteria. Gut Microbes (2016) 7(1):22–39. doi: 10.1080/19490976.2015.1127483
28. Sayin SI, Wahlström A, Felin J, Jäntti S, Marschall HU, Bamberg K, et al. Gut Microbiota Regulates Bile Acid Metabolism by Reducing the Levels of Tauro-Beta-Muricholic Acid, a Naturally Occurring Fxr Antagonist. Cell Metab (2013) 17(2):225–35. doi: 10.1016/j.cmet.2013.01.003
29. Dawson PA, Karpen SJ. Intestinal Transport and Metabolism of Bile Acids. J Lipid Res (2015) 56(6):1085–99. doi: 10.1194/jlr.R054114
30. Selwyn FP, Csanaky IL, Zhang Y, Klaassen CD. Importance of Large Intestine in Regulating Bile Acids and Glucagon-Like Peptide-1 in Germ-Free Mice. Drug Metab Dispos (2015) 43(10):1544–56. doi: 10.1124/dmd.115.065276
31. Schmitt J, Kong B, Stieger B, Tschopp O, Schultze SM, Rau M, et al. Protective Effects of Farnesoid X Receptor (Fxr) on Hepatic Lipid Accumulation Are Mediated by Hepatic Fxr and Independent of Intestinal Fgf15 Signal. Liver Int (2015) 35(4):1133–44. doi: 10.1111/liv.12456
32. Jiang C, Xie C, Li F, Zhang L, Nichols RG, Krausz KW, et al. Intestinal Farnesoid X Receptor Signaling Promotes Nonalcoholic Fatty Liver Disease. J Clin Invest (2015) 125(1):386–402. doi: 10.1172/jci76738
33. Parséus A, Sommer N, Sommer F, Caesar R, Molinaro A, Ståhlman M, et al. Microbiota-Induced Obesity Requires Farnesoid X Receptor. Gut (2017) 66(3):429–37. doi: 10.1136/gutjnl-2015-310283
34. Saeed A, Hoekstra M, Hoeke MO, Heegsma J, Faber KN. The Interrelationship Between Bile Acid and Vitamin a Homeostasis. Biochim Biophys Acta Mol Cell Biol Lipids (2017) 1862(5):496–512. doi: 10.1016/j.bbalip.2017.01.007
35. Charach G, Argov O, Geiger K, Charach L, Rogowski O, Grosskopf I. Diminished Bile Acids Excretion is a Risk Factor for Coronary Artery Disease: 20-Year Follow Up and Long-Term Outcome. Ther Adv Gastroenterol (2018) 11:1756283x17743420. doi: 10.1177/1756283x17743420
36. Qi X, Yun C, Pang Y, Qiao J. The Impact of the Gut Microbiota on the Reproductive and Metabolic Endocrine System. Gut Microbes (2021) 13(1):1–21. doi: 10.1080/19490976.2021.1894070
37. Kuhre RE, Wewer Albrechtsen NJ, Larsen O, Jepsen SL, Balk-Møller E, Andersen DB, et al. Bile Acids are Important Direct and Indirect Regulators of the Secretion of Appetite- and Metabolism-Regulating Hormones From the Gut and Pancreas. Mol Metab (2018) 11:84–95. doi: 10.1016/j.molmet.2018.03.007
38. Perino A, Velazquez-Villegas LA, Bresciani N, Sun Y, Huang Q, Fenelon VS, et al. Central Anorexigenic Actions of Bile Acids Are Mediated by Tgr5. Nat Metab (2021) 3(5):595–603. doi: 10.1038/s42255-021-00398-4
39. Alemi F, Kwon E, Poole DP, Lieu T, Lyo V, Cattaruzza F, et al. The Tgr5 Receptor Mediates Bile Acid-Induced Itch and Analgesia. J Clin Invest (2013) 123(4):1513–30. doi: 10.1172/jci64551
40. Xie Y, He Y, Cai Z, Cai J, Xi M, Zhang Y, et al. Tauroursodeoxycholic Acid Inhibits Endoplasmic Reticulum Stress, Blocks Mitochondrial Permeability Transition Pore Opening, and Suppresses Reperfusion Injury Through Gsk-3ß in Cardiac H9c2 Cells. Am J Transl Res (2016) 8(11):4586–97.
41. Silva YP, Bernardi A, Frozza RL. The Role of Short-Chain Fatty Acids From Gut Microbiota in Gut-Brain Communication. Front Endocrinol (Lausanne) (2020) 11:25. doi: 10.3389/fendo.2020.00025
42. Koh A, De Vadder F, Kovatcheva-Datchary P, Bäckhed F. From Dietary Fiber to Host Physiology: Short-Chain Fatty Acids as Key Bacterial Metabolites. Cell (2016) 165(6):1332–45. doi: 10.1016/j.cell.2016.05.041
43. Hernández MAG, Canfora EE, Jocken JWE, Blaak EE. The Short-Chain Fatty Acid Acetate in Body Weight Control and Insulin Sensitivity. Nutrients (2019) 11(8):1943. doi: 10.3390/nu11081943
44. Portincasa P, Bonfrate L, Vacca M, De Angelis M, Farella I, Lanza E, et al. Gut Microbiota and Short Chain Fatty Acids: Implications in Glucose Homeostasis. Int J Mol Sci (2022) 23(3):1105. doi: 10.3390/ijms23031105
45. Schönfeld P, Wojtczak L. Short- and Medium-Chain Fatty Acids in Energy Metabolism: The Cellular Perspective. J Lipid Res (2016) 57(6):943–54. doi: 10.1194/jlr.R067629
46. Bolognini D, Tobin AB, Milligan G, Moss CE. The Pharmacology and Function of Receptors for Short-Chain Fatty Acids. Mol Pharmacol (2016) 89(3):388–98. doi: 10.1124/mol.115.102301
47. Kim YA, Keogh JB, Clifton PM. Probiotics, Prebiotics, Synbiotics and Insulin Sensitivity. Nutr Res Rev (2018) 31(1):35–51. doi: 10.1017/S095442241700018X
48. Puddu A, Sanguineti R, Montecucco F, Viviani GL. Evidence for the Gut Microbiota Short-Chain Fatty Acids as Key Pathophysiological Molecules Improving Diabetes. Mediators Inflamm (2014) 2014:162021. doi: 10.1155/2014/162021
49. De Vadder F, Kovatcheva-Datchary P, Goncalves D, Vinera J, Zitoun C, Duchampt A, et al. Microbiota-Generated Metabolites Promote Metabolic Benefits via Gut-Brain Neural Circuits. Cell (2014) 156(1-2):84–96. doi: 10.1016/j.cell.2013.12.016
50. Dang AT, Marsland BJ. Microbes, Metabolites, and the Gut-Lung Axis. Mucosal Immunol (2019) 12(4):843–50. doi: 10.1038/s41385-019-0160-6
51. Rooks MG, Garrett WS. Gut Microbiota, Metabolites and Host Immunity. Nat Rev Immunol (2016) 16(6):341–52. doi: 10.1038/nri.2016.42
52. Kim CH, Park J, Kim M. Gut Microbiota-Derived Short-Chain Fatty Acids, T Cells, and Inflammation. Immune Netw (2014) 14(6):277–88. doi: 10.4110/in.2014.14.6.277
53. Nowarski R, Jackson R, Gagliani N, de Zoete MR, Palm NW, Bailis W, et al. Epithelial Il-18 Equilibrium Controls Barrier Function in Colitis. Cell (2015) 163(6):1444–56. doi: 10.1016/j.cell.2015.10.072
54. Smith PM, Howitt MR, Panikov N, Michaud M, Gallini CA, Bohlooly YM, et al. The Microbial Metabolites, Short-Chain Fatty Acids, Regulate Colonic Treg Cell Homeostasis. Science (2013) 341(6145):569–73. doi: 10.1126/science.1241165
55. Vieira AT, Macia L, Galvão I, Martins FS, Canesso MC, Amaral FA, et al. A Role for Gut Microbiota and the Metabolite-Sensing Receptor Gpr43 in a Murine Model of Gout. Arthritis Rheumatol (2015) 67(6):1646–56. doi: 10.1002/art.39107
56. Mathewson ND, Jenq R, Mathew AV, Koenigsknecht M, Hanash A, Toubai T, et al. Gut Microbiome-Derived Metabolites Modulate Intestinal Epithelial Cell Damage and Mitigate Graft-Versus-Host Disease. Nat Immunol (2016) 17(5):505–13. doi: 10.1038/ni.3400
57. Stilling RM, van de Wouw M, Clarke G, Stanton C, Dinan TG, Cryan JF. The Neuropharmacology of Butyrate: The Bread and Butter of the Microbiota-Gut-Brain Axis? Neurochem Int (2016) 99:110–32. doi: 10.1016/j.neuint.2016.06.011
58. Bröer S, Gauthier-Coles G. Amino Acid Homeostasis in Mammalian Cells With a Focus on Amino Acid Transport. J Nutr (2022) 152(1):16–28. doi: 10.1093/jn/nxab342
59. Bröer S, Fairweather SJ. Amino Acid Transport Across the Mammalian Intestine. Compr Physiol (2018) 9(1):343–73. doi: 10.1002/cphy.c170041
60. Yan R, Zhao X, Lei J, Zhou Q. Structure of the Human Lat1-4f2hc Heteromeric Amino Acid Transporter Complex. Nature (2019) 568(7750):127–30. doi: 10.1038/s41586-019-1011-z
61. Gauthier-Coles G, Vennitti J, Zhang Z, Comb WC, Xing S, Javed K, et al. Quantitative Modelling of Amino Acid Transport and Homeostasis in Mammalian Cells. Nat Commun (2021) 12(1):5282. doi: 10.1038/s41467-021-25563-x
62. Neinast MD, Jang C, Hui S, Murashige DS, Chu Q, Morscher RJ, et al. Quantitative Analysis of the Whole-Body Metabolic Fate of Branched-Chain Amino Acids. Cell Metab (2019) 29(2):417–29.e4. doi: 10.1016/j.cmet.2018.10.013
63. Shin AC, Fasshauer M, Filatova N, Grundell LA, Zielinski E, Zhou JY, et al. Brain Insulin Lowers Circulating Bcaa Levels by Inducing Hepatic Bcaa Catabolism. Cell Metab (2014) 20(5):898–909. doi: 10.1016/j.cmet.2014.09.003
64. Kelly B, Pearce EL. Amino Assets: How Amino Acids Support Immunity. Cell Metab (2020) 32(2):154–75. doi: 10.1016/j.cmet.2020.06.010
65. Chen W, Pang Y. Metabolic Syndrome and Pcos: Pathogenesis and the Role of Metabolites. Metabolites (2021) 11(12):869. doi: 10.3390/metabo11120869
66. Usuda K, Kawase T, Shigeno Y, Fukuzawa S, Fujii K, Zhang H, et al. Hippocampal Metabolism of Amino Acids by L-Amino Acid Oxidase is Involved in Fear Learning and Memory. Sci Rep (2018) 8(1):11073. doi: 10.1038/s41598-018-28885-x
67. Miyajima M. Amino Acids: Key Sources for Immunometabolites and Immunotransmitters. Int Immunol (2020) 32(7):435–46. doi: 10.1093/intimm/dxaa019
68. Markle JG, Frank DN, Mortin-Toth S, Robertson CE, Feazel LM, Rolle-Kampczyk U, et al. Sex Differences in the Gut Microbiome Drive Hormone-Dependent Regulation of Autoimmunity. Science (2013) 339(6123):1084–8. doi: 10.1126/science.1233521
69. Dominianni C, Sinha R, Goedert JJ, Pei Z, Yang L, Hayes RB, et al. Sex, Body Mass Index, and Dietary Fiber Intake Influence the Human Gut Microbiome. PloS One (2015) 10(4):e0124599. doi: 10.1371/journal.pone.0124599
70. Torres PJ, Siakowska M, Banaszewska B, Pawelczyk L, Duleba AJ, Kelley ST, et al. Gut Microbial Diversity in Women With Polycystic Ovary Syndrome Correlates With Hyperandrogenism. J Clin Endocrinol Metab (2018) 103(4):1502–11. doi: 10.1210/jc.2017-02153
71. Sherman SB, Sarsour N, Salehi M, Schroering A, Mell B, Joe B, et al. Prenatal Androgen Exposure Causes Hypertension and Gut Microbiota Dysbiosis. Gut Microbes (2018) 9(5):400–21. doi: 10.1080/19490976.2018.1441664
72. Mammadova G, Ozkul C, Yilmaz Isikhan S, Acikgoz A, Yildiz BO. Characterization of Gut Microbiota in Polycystic Ovary Syndrome: Findings From a Lean Population. Eur J Clin Invest (2021) 51(4):e13417. doi: 10.1111/eci.13417
73. Bulun SE, Chen D, Moy I, Brooks DC, Zhao H. Aromatase, Breast Cancer and Obesity: A Complex Interaction. Trends Endocrinol Metab (2012) 23(2):83–9. doi: 10.1016/j.tem.2011.10.003
74. Cani PD, Bibiloni R, Knauf C, Waget A, Neyrinck AM, Delzenne NM, et al. Changes in Gut Microbiota Control Metabolic Endotoxemia-Induced Inflammation in High-Fat Diet-Induced Obesity and Diabetes in Mice. Diabetes (2008) 57(6):1470–81. doi: 10.2337/db07-1403
75. Zheng Y, Yu J, Liang C, Li S, Wen X, Li Y. Characterization on Gut Microbiome of Pcos Rats and Its Further Design by Shifts in High-Fat Diet and Dihydrotestosterone Induction in Pcos Rats. Bioprocess Biosyst Eng (2021) 44(5):953–64. doi: 10.1007/s00449-020-02320-w
76. Pedersen HK, Gudmundsdottir V, Nielsen HB, Hyotylainen T, Nielsen T, Jensen BA, et al. Human Gut Microbes Impact Host Serum Metabolome and Insulin Sensitivity. Nature (2016) 535(7612):376–81. doi: 10.1038/nature18646
77. Sun Z, Chang HM, Wang A, Song J, Zhang X, Guo J, et al. Identification of Potential Metabolic Biomarkers of Polycystic Ovary Syndrome in Follicular Fluid by Swath Mass Spectrometry. Reprod Biol Endocrinol (2019) 17(1):45. doi: 10.1186/s12958-019-0490-y
78. Halama A, Aye MM, Dargham SR, Kulinski M, Suhre K, Atkin SL. Metabolomics of Dynamic Changes in Insulin Resistance Before and After Exercise in Pcos. Front Endocrinol (Lausanne) (2019) 10:116. doi: 10.3389/fendo.2019.00116
79. Gubert C, Kong G, Renoir T, Hannan AJ. Exercise, Diet and Stress as Modulators of Gut Microbiota: Implications for Neurodegenerative Diseases. Neurobiol Dis (2020) 134:104621. doi: 10.1016/j.nbd.2019.104621
80. Allen JM, Mailing LJ, Cohrs J, Salmonson C, Fryer JD, Nehra V, et al. Exercise Training-Induced Modification of the Gut Microbiota Persists After Microbiota Colonization and Attenuates the Response to Chemically-Induced Colitis in Gnotobiotic Mice. Gut Microbes (2018) 9(2):115–30. doi: 10.1080/19490976.2017.1372077
81. Allen JM, Mailing LJ, Niemiro GM, Moore R, Cook MD, White BA, et al. Exercise Alters Gut Microbiota Composition and Function in Lean and Obese Humans. Med Sci Sports Exerc (2018) 50(4):747–57. doi: 10.1249/mss.0000000000001495
82. Nakajima M, Arimatsu K, Kato T, Matsuda Y, Minagawa T, Takahashi N, et al. Oral Administration of P. Gingivalis Induces Dysbiosis of Gut Microbiota and Impaired Barrier Function Leading to Dissemination of Enterobacteria to the Liver. PloS One (2015) 10(7):e0134234. doi: 10.1371/journal.pone.0134234
83. Muccioli GG, Naslain D, Bäckhed F, Reigstad CS, Lambert DM, Delzenne NM, et al. The Endocannabinoid System Links Gut Microbiota to Adipogenesis. Mol Syst Biol (2010) 6:392. doi: 10.1038/msb.2010.46
84. Zhang B, Shen S, Gu T, Hong T, Liu J, Sun J, et al. Increased Circulating Conjugated Primary Bile Acids Are Associated With Hyperandrogenism in Women With Polycystic Ovary Syndrome. J Steroid Biochem Mol Biol (2019) 189:171–5. doi: 10.1016/j.jsbmb.2019.03.005
85. Gozukara I, Dokuyucu R, Özgür T, Özcan O, Pınar N, Kurt RK, et al. Histopathologic and Metabolic Effect of Ursodeoxycholic Acid Treatment on Pcos Rat Model. Gynecol Endocrinol (2016) 32(6):492–7. doi: 10.3109/09513590.2015.1134478
86. Qi X, Yun C, Liao B, Qiao J, Pang Y. The Therapeutic Effect of Interleukin-22 in High Androgen-Induced Polycystic Ovary Syndrome. J Endocrinol (2020) 245(2):281–9. doi: 10.1530/joe-19-0589
87. Bartelt A, Heeren J. Adipose Tissue Browning and Metabolic Health. Nat Rev Endocrinol (2014) 10(1):24–36. doi: 10.1038/nrendo.2013.204
88. Lynch CJ, Adams SH. Branched-Chain Amino Acids in Metabolic Signalling and Insulin Resistance. Nat Rev Endocrinol (2014) 10(12):723–36. doi: 10.1038/nrendo.2014.171
89. Yang W, Yu T, Huang X, Bilotta AJ, Xu L, Lu Y, et al. Intestinal Microbiota-Derived Short-Chain Fatty Acids Regulation of Immune Cell Il-22 Production and Gut Immunity. Nat Commun (2020) 11(1):4457. doi: 10.1038/s41467-020-18262-6
90. Litvak Y, Byndloss MX, Bäumler AJ. Colonocyte Metabolism Shapes the Gut Microbiota. Science (2018) 362(6418):eaat9076. doi: 10.1126/science.aat9076
91. Aydin K, Arusoglu G, Koksal G, Cinar N, Aksoy DY, Yildiz BO. Fasting and Post-Prandial Glucagon Like Peptide 1 and Oral Contraception in Polycystic Ovary Syndrome. Clin Endocrinol (Oxf) (2014) 81(4):588–92. doi: 10.1111/cen.12468
92. Lin W, Wen L, Wen J, Xiang G. Effects of Sleeve Gastrectomy on Fecal Gut Microbiota and Short-Chain Fatty Acid Content in a Rat Model of Polycystic Ovary Syndrome. Front Endocrinol (Lausanne) (2021) 12:747888. doi: 10.3389/fendo.2021.747888
93. Newgard CB. Interplay Between Lipids and Branched-Chain Amino Acids in Development of Insulin Resistance. Cell Metab (2012) 15(5):606–14. doi: 10.1016/j.cmet.2012.01.024
94. Feng W, Ao H, Peng C, Yan D. Gut Microbiota, a New Frontier to Understand Traditional Chinese Medicines. Pharmacol Res (2019) 142:176–91. doi: 10.1016/j.phrs.2019.02.024
95. Agus A, Clement K, Sokol H. Gut Microbiota-Derived Metabolites as Central Regulators in Metabolic Disorders. Gut (2021) 70(6):1174–82. doi: 10.1136/gutjnl-2020-323071
96. Ye Z, Zhang C, Wang S, Zhang Y, Li R, Zhao Y, et al. Amino Acid Signatures in Relation to Polycystic Ovary Syndrome and Increased Risk of Different Metabolic Disturbances. Reprod BioMed Online (2021) 44(4):737–746. doi: 10.1016/j.rbmo.2021.11.012
97. Wang Z, Yang L, Jiang Y, Ling ZQ, Li Z, Cheng Y, et al. High Fat Diet Induces Formation of Spontaneous Liposarcoma in Mouse Adipose Tissue With Overexpression of Interleukin 22. PloS One (2011) 6(8):e23737. doi: 10.1371/journal.pone.0023737
98. Ahmadi S, Jamilian M, Karamali M, Tajabadi-Ebrahimi M, Jafari P, Taghizadeh M, et al. Probiotic Supplementation and the Effects on Weight Loss, Glycaemia and Lipid Profiles in Women With Polycystic Ovary Syndrome: A Randomized, Double-Blind, Placebo-Controlled Trial. Hum Fertil (Camb) (2017) 20(4):254–61. doi: 10.1080/14647273.2017.1283446
99. Shoaei T, Heidari-Beni M, Tehrani HG, Feizi A, Esmaillzadeh A, Askari G. Effects of Probiotic Supplementation on Pancreatic β-Cell Function and C-Reactive Protein in Women With Polycystic Ovary Syndrome: A Randomized Double-Blind Placebo-Controlled Clinical Trial. Int J Prev Med (2015) 6:27. doi: 10.4103/2008-7802.153866
100. Jamilian M, Mansury S, Bahmani F, Heidar Z, Amirani E, Asemi Z. The Effects of Probiotic and Selenium Co-Supplementation on Parameters of Mental Health, Hormonal Profiles, and Biomarkers of Inflammation and Oxidative Stress in Women With Polycystic Ovary Syndrome. J Ovarian Res (2018) 11(1):80. doi: 10.1186/s13048-018-0457-1
101. Heshmati J, Farsi F, Yosaee S, Razavi M, Rezaeinejad M, Karimie E, et al. The Effects of Probiotics or Synbiotics Supplementation in Women With Polycystic Ovarian Syndrome: A Systematic Review and Meta-Analysis of Randomized Clinical Trials. Probiotics Antimicrob Proteins (2019) 11(4):1236–47. doi: 10.1007/s12602-018-9493-9
102. Shamasbi SG, Ghanbari-Homayi S, Mirghafourvand M. The Effect of Probiotics, Prebiotics, and Synbiotics on Hormonal and Inflammatory Indices in Women With Polycystic Ovary Syndrome: A Systematic Review and Meta-Analysis. Eur J Nutr (2020) 59(2):433–50. doi: 10.1007/s00394-019-02033-1
103. Guo Y, Qi Y, Yang X, Zhao L, Wen S, Liu Y, et al. Association Between Polycystic Ovary Syndrome and Gut Microbiota. PloS One (2016) 11(4):e0153196. doi: 10.1371/journal.pone.0153196
104. Li T, Zhang Y, Song J, Chen L, Du M, Mao X. Yogurt Enriched With Inulin Ameliorated Reproductive Functions and Regulated Gut Microbiota in Dehydroepiandrosterone-Induced Polycystic Ovary Syndrome Mice. Nutrients (2022) 14(2):279. doi: 10.3390/nu14020279
105. Wang HY, Qi LW, Wang CZ, Li P. Bioactivity Enhancement of Herbal Supplements by Intestinal Microbiota Focusing on Ginsenosides. Am J Chin Med (2011) 39(6):1103–15. doi: 10.1142/s0192415x11009433
106. Chang CJ, Lin CS, Lu CC, Martel J, Ko YF, Ojcius DM, et al. Ganoderma Lucidum Reduces Obesity in Mice by Modulating the Composition of the Gut Microbiota. Nat Commun (2015) 6:7489. doi: 10.1038/ncomms8489
107. Shen HR, Xu X, Ye D, Li XL. Berberine Improves the Symptoms of Dhea-Induced Pcos Rats by Regulating Gut Microbiotas and Metabolites. Gynecol Obstet Invest (2021) 86(4):388–97. doi: 10.1159/000518040
108. Yue SJ, Liu J, Wang AT, Meng XT, Yang ZR, Peng C, et al. Berberine Alleviates Insulin Resistance by Reducing Peripheral Branched-Chain Amino Acids. Am J Physiol Endocrinol Metab (2019) 316(1):E73–e85. doi: 10.1152/ajpendo.00256.2018
109. Hu Q, Zhang W, Wu Z, Tian X, Xiang J, Li L, et al. Baicalin and the Liver-Gut System: Pharmacological Bases Explaining Its Therapeutic Effects. Pharmacol Res (2021) 165:105444. doi: 10.1016/j.phrs.2021.105444
110. Zhou SS, Xu J, Zhu H, Wu J, Xu JD, Yan R, et al. Gut Microbiota-Involved Mechanisms in Enhancing Systemic Exposure of Ginsenosides by Coexisting Polysaccharides in Ginseng Decoction. Sci Rep (2016) 6:22474. doi: 10.1038/srep22474
111. Zheng HR, Chu Y, Zhou DZ, Ju AC, Li W, Li X, et al. Integrated Pharmacokinetics of Ginsenosides After Intravenous Administration of Yiqifumai Powder Injection in Rats With Chronic Heart Failure by Uflc-Ms/Ms. J Chromatogr B Analyt Technol BioMed Life Sci (2018) 1072:282–9. doi: 10.1016/j.jchromb.2017.10.056
112. Chen F, Wen Q, Jiang J, Li HL, Tan YF, Li YH, et al. Could the Gut Microbiota Reconcile the Oral Bioavailability Conundrum of Traditional Herbs? J Ethnopharmacol (2016) 179:253–64. doi: 10.1016/j.jep.2015.12.031
113. Feng R, Shou JW, Zhao ZX, He CY, Ma C, Huang M, et al. Transforming Berberine Into Its Intestine-Absorbable Form by the Gut Microbiota. Sci Rep (2015) 5:12155. doi: 10.1038/srep12155
114. Singh RK, Chang HW, Yan D, Lee KM, Ucmak D, Wong K, et al. Influence of Diet on the Gut Microbiome and Implications for Human Health. J Transl Med (2017) 15(1):73. doi: 10.1186/s12967-017-1175-y
115. Wu GD, Chen J, Hoffmann C, Bittinger K, Chen YY, Keilbaugh SA, et al. Linking Long-Term Dietary Patterns With Gut Microbial Enterotypes. Science (2011) 334(6052):105–8. doi: 10.1126/science.1208344
116. Sanz Y. Effects of a Gluten-Free Diet on Gut Microbiota and Immune Function in Healthy Adult Humans. Gut Microbes (2010) 1(3):135–7. doi: 10.4161/gmic.1.3.11868
117. Koloverou E, Panagiotakos DB, Pitsavos C, Chrysohoou C, Georgousopoulou EN, Grekas A, et al. Adherence to Mediterranean Diet and 10-Year Incidence (2002-2012) of Diabetes: Correlations With Inflammatory and Oxidative Stress Biomarkers in the Attica Cohort Study. Diabetes Metab Res Rev (2016) 32(1):73–81. doi: 10.1002/dmrr.2672
Keywords: polycystic ovary syndrome, gut metabolites, crosstalk, bile acids, short chain fatty acids, amino acids
Citation: Zhang M, Hu R, Huang Y, Zhou F, Li F, Liu Z, Geng Y, Dong H, Ma W, Song K and Song Y (2022) Present and Future: Crosstalks Between Polycystic Ovary Syndrome and Gut Metabolites Relating to Gut Microbiota. Front. Endocrinol. 13:933110. doi: 10.3389/fendo.2022.933110
Received: 30 April 2022; Accepted: 20 June 2022;
Published: 19 July 2022.
Edited by:
Yanli Pang, Peking University Third Hospital, ChinaReviewed by:
Rong Li, Peking University Third Hospital, ChinaCopyright © 2022 Zhang, Hu, Huang, Zhou, Li, Liu, Geng, Dong, Ma, Song and Song. This is an open-access article distributed under the terms of the Creative Commons Attribution License (CC BY). The use, distribution or reproduction in other forums is permitted, provided the original author(s) and the copyright owner(s) are credited and that the original publication in this journal is cited, in accordance with accepted academic practice. No use, distribution or reproduction is permitted which does not comply with these terms.
*Correspondence: Yufan Song, c29uZ3l1ZmFuMjNAMTYzLmNvbQ==
†These authors have contributed equally to this work and share first authorship
Disclaimer: All claims expressed in this article are solely those of the authors and do not necessarily represent those of their affiliated organizations, or those of the publisher, the editors and the reviewers. Any product that may be evaluated in this article or claim that may be made by its manufacturer is not guaranteed or endorsed by the publisher.
Research integrity at Frontiers
Learn more about the work of our research integrity team to safeguard the quality of each article we publish.