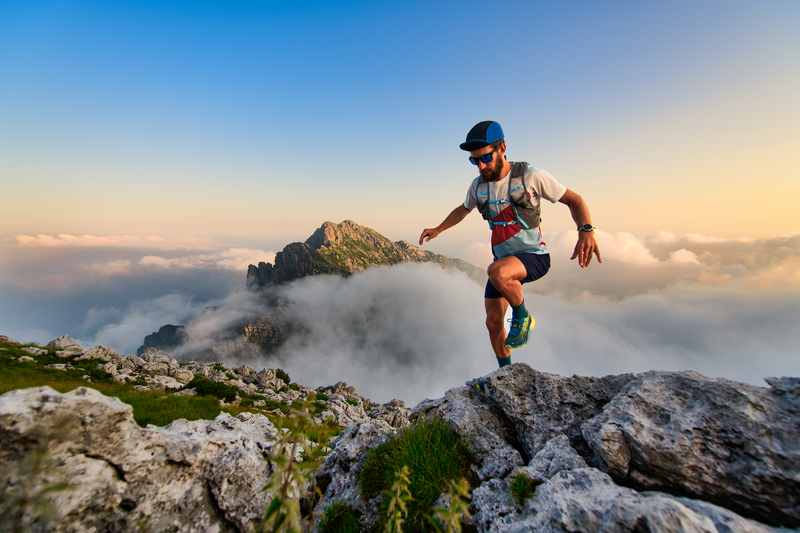
95% of researchers rate our articles as excellent or good
Learn more about the work of our research integrity team to safeguard the quality of each article we publish.
Find out more
ORIGINAL RESEARCH article
Front. Endocrinol. , 28 July 2022
Sec. Developmental Endocrinology
Volume 13 - 2022 | https://doi.org/10.3389/fendo.2022.908240
This article is part of the Research Topic Maternal-Fetal Interface: New Insight in Placenta Research View all 18 articles
Maternal hypothyroidism is associated with fetal growth restriction, placental dysfunction, and reduced kisspeptin/Kiss1R at the maternal-fetal interface. Kisspeptin affects trophoblastic migration and has antioxidant and immunomodulatory activities. This study aimed to evaluate the therapeutic potential of kisspeptin in the fetal-placental dysfunction of hypothyroid Wistar rats. Hypothyroidism was induced by daily administration of propylthiouracil. Kisspeptin-10 (Kp-10) treatment was performed every other day or daily beginning on day 8 of gestation. Feto-placental development, placental histomorphometry, and expression levels of growth factors (VEGF, PLGF, IGF1, IGF2, and GLUT1), hormonal (Dio2) and inflammatory mediators (TNFα, IL10, and IL6), markers of hypoxia (HIF1α) and oxidative damage (8-OHdG), antioxidant enzymes (SOD1, Cat, and GPx1), and endoplasmic reticulum stress mediators (ATF4, GRP78, and CHOP) were evaluated on day 18 of gestation. Daily treatment with Kp-10 increased free T3 and T4 levels and improved fetal weight. Both treatments reestablished the glycogen cell population in the junctional zone. Daily treatment with Kp-10 increased the gene expression levels of Plgf, Igf1, and Glut1 in the placenta of hypothyroid animals, in addition to blocking the increase in 8-OHdG and increasing protein and/or mRNA expression levels of SOD1, Cat, and GPx1. Daily treatment with Kp-10 did not alter the higher protein expression levels of VEGF, HIF1α, IL10, GRP78, and CHOP caused by hypothyroidism in the junctional zone compared to control, nor the lower expression of Dio2 caused by hypothyroidism. However, in the labyrinth zone, this treatment restored the expression of VEGF and IL10 and reduced the GRP78 and CHOP immunostaining. These findings demonstrate that daily treatment with Kp-10 improves fetal development and placental morphology in hypothyroid rats, blocks placental oxidative damage, and increases the expression of growth factors and antioxidant enzymes in the placenta.
Kisspeptin, encoded by the gene Kiss1, was first purified from human placenta (1, 2). Soon after kisspeptin was discovered, it was recognized as essential for fertility, as it regulates gonadotropin-releasing hormone (GnRH) secretion by the hypothalamus through the G protein-coupled receptor GPR54 (Kiss1R). Therefore, failures in the kisspeptin/Kiss1R signaling system result in hypogonadotropic hypogonadism (3, 4). In addition to its hypothalamic action, however, the kisspeptin/Kiss1R system is expressed in a variety of tissues, including the placenta (5, 6), although studies on the action of kisspeptin on the placenta are still scarce (7–9).
In humans, circulating levels of kisspeptin are low in most physiological conditions, except in the final third of gestation, when these levels increase almost 10, 000 times, after which they quickly return to basal level after delivery (10–12). This suggests that the placenta is one of the main sources of systemic kisspeptin. Studies have shown that kisspeptin influences the adhesion and implantation of blastocysts and the decidualization and migration of trophoblasts, and regulates the immunological profile and uterine natural killer cells (uNKs) (5, 7–9, 11–17), suggesting that faults in its expression in the maternal-fetal interface may be involved in placental disorders (12, 18).
Gestational diseases such as preeclampsia, gestational hypertension, miscarriage, gestational diabetes, and obesity have altered plasma and/or placental levels of kisspeptin (17–30). Therefore, its plasma profile can be assessed to predict gestational success (12, 18). We have recently demonstrated that rats with maternal hypothyroidism, another important gestational disease, also exhibit decidual and placental reduction of the kisspeptin/Kiss1R system (31).
Women with maternal hypothyroidism are more likely to suffer miscarriage and intrauterine growth restriction (Silva et al., 2012; Silva, Ocarino, and Serakides, 2018). Moreover, plasma levels of triiodothyronine (T3) and thyroxine (T4) are low in patients with preeclampsia (32, 33). Studies in rats have also shown that hypothyroidism reduces intrauterine trophoblast migration and proliferation, increases placental apoptosis, compromises placental morphogenesis and vascularization, causes oxidative and reticular stress, and alters the immune profile and uNK cell population at the maternal-fetal interface (34–40). However, the role of kisspeptin in these placental changes is still unknown.
Although T4 replacement is the first choice in patients with hypothyroidism, some patients are refractory to T4 replacement (41) and need other therapeutic alternatives when they need to treat a medical condition, such as infertility problems. In this sense, exogenous kisspeptin has been shown to restore ovarian function in hypothyroid rats (42). Furthermore, studies have shown that kisspeptin administration has an immunomodulatory effect on gestation (15) and an antioxidative effect on the ovary (43), liver (44), and testicle (45), and blocks the occurrence of reticular stress in hypothalamic GT1-7 cell line (46). Therefore, this study aimed to evaluate the therapeutic potential of kisspeptin in placental dysfunction caused by maternal hypothyroidism. We demonstrate that daily kisspeptin treatment improves development of the fetus and placenta in hypothyroid rats, increases the expression of growth factors and antioxidant enzymes by the placenta, and positively modulates oxidative stress, reticular stress and immune mediators, thus characterizing the therapeutic potential of kisspeptin in a gestational disease for the first time.
Adult Wistar rats (200-250 grams) were kept in plastic boxes with controlled temperature (22 ± 2°C) and brightness (12 h light/12 h dark), and water and feed ad libitum. The animals were equally distributed into euthyroid (control) (n=13), hypothyroid (n=15), and hypothyroid groups treated with kisspeptin-10 (Kp10) every other day (KpT1; n=15) or every day (KpT2; n=15). Hypothyroidism was induced by administering 6-propyl-2-thiouracil (PTU) (4 mg/Kg/day) through an orogastric tube every day, starting five days before mating, while the control group received water as a placebo (35, 36). All experimental procedures were approved by the Ethics Committee on the Use of Animals of Santa Cruz State University (UESC) (Protocol 036/16).
Five days after the start of treatment with PTU, five animals from each group were euthanized by decapitation for blood collection and dosage of free T4 to confirm the induction of hypothyroidism (Control, 1.20 ± 0.05 µg/dL; Hypothyroid, 0.47 ± 0.09 µg/dL; KpT1, 0.52 ± 0.06 µg/dL; KpT2, 0,42 ± 0.08 µg/dL (P<0.01)). Vaginal cytology was performed in the remaining rats, and the animals in proestrus were housed with fertile adult males for mating. The presence of spermatozoa in the vaginal cytology the next morning confirmed copulation and was defined as day 0 of gestation (0 GD). All animals from control group were mated and became pregnant, while from hypothyroid groups (Hypothyoid; KpT1; KpT2) around 86.6% of the females became pregnant.
The hypothyroid animals treated with Kp10 were distributed into two groups, one treated every other day (KpT1) and one treated daily with Kp10 (KpT2). Treatment was initiated on the 8th GD (8 µg/Kg/day) (Cat. No. 4243, Tocris Bioscience, Bristol, UK), intraperitoneally, and was maintained until the day of euthanasia. Kp10 treatment was initiated on the 8th GD so that it would not influence embryo implantation. The animals in the control and hypothyroid groups received sterile water as a placebo.
The animals were euthanized by decapitation on the 18th GD and blood was collected from the neck into tubes with heparin for dosage of free T3 and T4. The blood was centrifuged at 3000 rpm for 20 min and the plasma was obtained and stored at -20°C.
At necropsy, the entire genital system was collected. Subsequently, the uterus containing the placenta and fetuses, and the uterus with placenta were weighed, as were the fetuses, individually. Hysterectometric (maternal weight without the gravid uterus) maternal weight gain was also evaluated. The weight of amniotic fluid was estimated by subtracting the weight of the uterus and placenta with the fetuses from the weight of the fetuses and the weight of the uterus and placenta without the fetuses. The number of fetuses and the number of sites with resorption or fetal death were also counted. Fragments of the central region of the placenta measuring 2 mm in diameter were dissected and removed from two placental sites/animal and separately immersed in TRIzol®, followed by freezing in liquid nitrogen and storing at -80°C for quantitative real-time reverse transcriptase-polymerase chain reaction (qRT-PCR) analyses. The remaining discs (placenta+basal decidua+metrial gland) were fixed in 4% paraformaldehyde at 4°C for 24 hours and processed using the paraffin embedding technique for histomorphometry and immunohistochemistry analysis. The tissues were dehydrated in a serial solution of 70% to 100% alcohol, with subsequent xylene diaphanization and paraffin impregnation and embedding. Microtomy on histological slides was used to obtain 4-µm tissue sections for histomorphometry evaluation. Silane-coated polarized slides (StarFrost Polycat, Germany) were used for immunohistochemistry.
Dosage of Free T3 and T4 was performed using enzyme-linked immunosorbent assay (ELISA) kits according to the manufacturer’s instructions (IMMULITE, Siemens Medical Solutions Diagnostics, Malvern, PA, USA).
The brain, heart, liver, lungs, and kidneys of each fetus were dissected and weighed, and the relative weight of the organs in relation to fetal weight was obtained. After weighing the fetal organs, the brain to liver ratio, an indicator of asymmetric fetal growth restriction, was calculated (47).
For analysis of fetal weight distribution and risk of fetal growth restriction, fetal weight histograms were constructed for each group with the individual fetal weight and non-linear regression was performed according to Dilworth et al. (48). The 5th percentile weight was calculated as: (-Z score x SD) + mean, assuming that Z score = 1.645, SD = standard deviation, mean = mean of control group.
Histomorphometry analysis of the placenta was performed on 4 µm histological sections stained with hematoxylin and eosin. All evaluations were performed blindly by two evaluators and without knowledge of the experimental groups. The quantitative evaluation was performed on 7-8 placental discs/group, and a histological section/placental disc was obtained from the center of the tissue with maternal central blood vessel to ensure the histological sections were uniform. Images of each placental disc were captured using a Leica S9i stereo microscope, and the thickness of each placental layer (junctional zone and labyrinth zone) was assessed in 10 random regions and averaged per placental site. The analyses were performed using Image Pro Plus® version 4.5 software and the values were transformed into millimeters using a micrometer scale.
In the junctional zone, the proportion of area occupied by glycogen cells, spongiotrophoblasts, and trophoblastic giant cells per field was evaluated by selecting 5 random fields with the 20x lens. In the labyrinth zone, the proportion of area occupied by maternal vascular sinus, fetal capillaries, and fetal mesenchyme/trophoblastic cells per field was evaluated by selecting 10 random fields of the labyrinth with the 40x lens. The images were captured on a Leica DM2500 photon microscope and quantification was performed using a graticule of 99 (junctional zone) and 100 (labyrinth zone) points with Image Pro Plus® software version 4.5 (35).
Histological sections of the placental discs were submitted to immunohistochemistry analysis using the antibodies anti-8-OHdG (sc-393871), anti-HIF1α (sc-13515), anti-SOD1 (sc-101523), anti-catalase (sc-271803), anti-GPx 1/2 (sc-133160), anti-GRP78 (sc-13539), anti-CHOP (sc-71136), anti-ATF4 (sc-390063), anti-TNFα (sc-52746), anti-IL-10 (sc-365858), and anti-VEGF (sc-152), from Santa Cruz Biotechnology, CA, USA. All antibodies used in this study were validated by the manufacturer.
The streptavidin-biotin-peroxidase staining technique (Novolink Polymer Detection Systems, Leica Biosystems Inc., Buffalo Grove, IL, USA) was used and antigen retrieval was performed with heat in a water bath at 98°C using citric acid solution at pH 6.0. The slides were incubated in a humid chamber for 18 or 40 hours with the primary antibody (Supplementary Figure 1) and for 30 minutes in the blocking stages of endogenous peroxidase, serum blocking, and streptavidin peroxidase. The chromogen was diaminobenzidine (EnVision FLEX DAB+ Chromogen, Agilent Technologies, Inc., Santa Clara, CA. USA). The sections were counterstained with Harris hematoxylin. The negative control was obtained by replacing the primary antibody with phosphate buffered saline (PBS) (36).
A descriptive and quantitative evaluation of the immunohistochemistry expression of HIF1α, 8-OHdG, SOD1, Catalase, GPx1/2, GRP78, CHOP, ATF4, VEGF, TNFα, and IL-10 was performed in the junctional zone and labyrinth zone layers of the placenta. A quantitative evaluation was performed randomly on six placental discs/group. Images of 5 random fields in each region of the placental disc were obtained with a Leica DMI 300B photon microscope (Leica Microsystems, Germany) with the 40x lens. The immunolabeling area was determined using WCIF ImageJ® software (Media Cybernetics Manufacturing, Rockville, MD, USA). The images were subjected to color deconvolution and thresholding. Data from each placental disc were archived, analyzed, and expressed as immunolabeling area in pixels (36).
For the qRT-PCR technique, total RNA from the placenta was extracted using TRIzol® according to the manufacturer’s instructions (Invitrogen, Life Technologies, Carlsbad, CA, USA). Subsequently, 1 µg of RNA was used for the reverse transcription reactions with the GoTaq® qPCR and RT-qPCR Systems kit (A6010, PROMEGA). The transcripts of the target genes were quantified by qPCR using SYBR Green in the Applied Biosystems® 7500 Real-Time PCR System. For the reactions, 1.5 μL of cDNA, 100 nM of each primer, and 12.5 μL of the GoTaq® qPCR Master Mix 2X reagent was used in a final volume of 20 μL of reaction. As a negative control, the DNA amplification mix was used, in which the cDNA sample was replaced by water. The amplifications were performed under the following conditions: enzyme activation at 95°C for 2 min, 40 cycles of denaturation at 95°C for 15 s, and annealing/extension at 60°C for 60 s. To evaluate the linearity and efficiency of qPCR amplification, standard curves of all transcripts were generated using serial dilutions of the cDNA, followed by an evaluation of the melting curve of the amplification products. The primers for Sod1, Catalase, Gpx1, Hif1α, Grp78, Chop, Il-10, Tnf, Il-6, Dio2, Igf1, Igf2, Glut1, Vegf, and Plgf were delineated based on Rattus norvegicus mRNA sequence (Table 1). Gene expression was calculated by the 2-ΔΔCT method, where the results obtained for each group were quantitatively compared after normalization based on the expression of Polr2a Rattus norvegicus (36, 49).
The differences of means among of the groups were determined by performing ANOVA followed by the Student-Newman-Keuls (SNK) test. The data were tested for normality (Shapiro-Wilk) and homoscedasticity (Brown-Forsythe) of the residuals, and for those that did not meet the assumptions, even after logarithmic transformation, the non-parametric Kruskal-Wallis test followed by Dunn’s test were used. Generalized linear mixed-model analysis followed by the Tukey test was used to evaluate the fetal and fetal organs’ weight and liver/brain ratio (50). Parametric data were represented by mean ± standard error of the mean (SEM), while non-parametric data were represented by median and interquartile range. The analyses were performed using GraphPad Prism 9.0.0 and R version 4.2.0 software, and differences where P<0.05 were considered significant.
Because fetal growth restriction in hypothyroid rats is associated with reduced Kiss1 and Kiss1R expression at the maternal-fetal interface (31), the effect of two treatment protocols with Kp-10 was evaluated every other day (KpT1) and daily (KpT2), starting on the 8th GD, on the maternal and fetal parameters of hypothyroid rats. The evaluation of maternal and placental parameters revealed that daily treatment with Kp-10 increased hysterectometric maternal weight gain during gestation when compared to the hypothyroid and KpT1 groups (Figure 1A; P<0.05), which had lower gestational weight gain compared to the control (P<0.001). No significant difference was observed between the hypothyroid and KpT1 groups for hysterectometric maternal weight gain (P>0.05). Interestingly, there was an increase in plasma free T3 and T4 levels in the group treated daily with Kp10 compared to the hypothyroid and KpT1 groups (Figures 1B, C; P<0.0001; P<0.05) which had lower free T3 and T4 levels compared to the control (P<0.001). Furthermore, the KpT1 and KpT2 treatments did not increase the uteroplacental weight or the amniotic fluid of the hypothyroid animals (P>0.05), which showed a reduction compared to the control group (P<0.05) (Figures 1E, F).
Figure 1 Maternal and fetal parameters of control, hypothyroid, and kisspeptin-10-treated rats on the 18th GD. (A) Hysterectometric maternal weight gain (mean ± SEM; n = 8). (B) Free T3 (mean ± SEM; n = 4-5). (C) Free T4 (mean ± SEM; n = 4-5). (D) Representative image of fetuses and placentas from each experimental group … (E–F) Uteroplacental (E) and amniotic fluid (F) weight (mean ± SEM; n = 8). (G) Number of viable fetuses/litter (mean ± SEM; n = 8). (H) Percentage of fetal death (median, interquatile range; n = 8). (I) Fetal weight (mean ± SEM; n = 63-76). (J) Relative frequency distribution curve of fetal weight. (K) Weight of fetal organs (liver, brain, heart, lung, kidney) (mean ± SEM; n = 15-30). (L) Brain/liver ratio (mean ± SEM; n = 15-30). Significant differences were determined by ANOVA post hoc SNK except for fetal weight and fetal organs’ weight, which were determined by Generalized linear mixed-model analysis followed by the Tukey test, and fetal death which was determined by Dunn’s post hoc Kruskal-Wallis test, *P<0.05, **P<0.01, ***P<0.001, ****P<0.0001. KpT1, yes/no day treatment with Kp10; KpT2, daily treatment with Kp10; GD, gestational day.
In the evaluation of fetal parameters, although a significant difference was not observed in the number of viable fetuses between the experimental groups (Figure 1G), the KpT1 and KpT2 treatments did not present any differences in the percentage of fetal death when compared to the hypothyroid group (P>0.05), which showed a higher percentage of fetal death when compared to the control (Figure 1H; P<0.05). The KpT1 and KpT2 treatments were also unable of restore the reduction of fetal weight cause by hypothyroidism when compared to the control (Figures 1D, I; P<0.001; P<0.05). However, in assessing the relative frequency of fetal weight distribution, both the KpT2 (87.30%) and control (95.05%) groups had a greater distribution than 5th percentile (1.278g) (dashed line), while most fetuses in the hypothyroid (61.49%) and KpT1 (78.58%) groups was below the 5th, with a shift to the left of the distribution curve (Figure 1J).
The KpT2 treatment did not restore the lower heart and lung weights exhibited by the fetuses of hypothyroid animals (P>0.05), which had reduced weights when compared to the control (Figure 1K; P<0.001; P<0.01). However, the fetuses in the KpT1 group had lower liver weight when compared to the control and KpT2 groups (P<0.001), as well as lower heart and lung weight in relation to the control and hypothyroid groups. Regarding kidney weight, the fetuses of the KpT1 group also showed a reduction compared to the control (P<0.01), hypothyroid (P<0.001), and KpT2 (P<0.01) groups. No significant difference was observed for fetal brain weight and the brain/liver ratio between the experimental groups (Figures 1K, L; P>0.05). Together, these data demonstrate that daily treatment with Kp10 was not able to increase fetal weight, but improved the “growth restricted” condition demonstrated by the fetal weight distribution curve. This was unlike the KpT1 treatment, which did not increase fetal weight, kept the greater part of the fetal weight below the 5th percentile of the control group, and had negative effects on fetal organogenesis.
After observing that daily treatment with Kp-10 improved fetal development in hypothyroid rats, it was necessary to assess whether this effect could result from improved placental morphology (Figures 2A–D). No difference was observed in the thickness of the junctional zone between the experimental groups, while the hypothyroid and KpT1 groups showed reduced thickness of the labyrinth zone when compared to the control group (Figure 2E; P<0.05). In the cellularity evaluation of the junctional zone, the placenta of hypothyroid animals had a higher percentage of area occupied by glycogen cells and fewer spongiotrophoblasts when compared to the control group (P<0.05). The treatments with KpT1 and KpT2 were able to restore the population of glycogen cells and/or spongiotrophoblasts in the placenta of hypothyroid animals, resembling that of the control animals (Figure 2F; P>0.05). A significant reduction was also observed in the giant cells from junctional zone in the KpT1 and KpT2 groups compared to the control (P<0.01;P<0.05) and to the hypothyroid group (Figure 2F; P<0.01;P<0.05). In the evaluation of the labyrinth zone, no significant difference was observed in the regions evaluated (fetal capillary, maternal vascular sinus and fetal mesenchyme/trophoblastic cells) between the groups (Figure 2G; P>0.05).
Figure 2 Histomorphometry evaluation of the placenta of control, hypothyroid, and kisspeptin-10 treated-rats on the 18th GD. (A–D) Illustrative photomicrographs of the maternal-fetal interface (A) and placental layers (B), giant cells; (C), spongiotrophoblast; (D), labyrinth zone) (Hematoxylin and eosin staining; Bar = 500μm (A); 50μm (B–D). (E) Thickness of the junctional zone (giant cells+spongiotrophoblast) and labyrinth zone (mean ± SEM; n = 8). (F) Percentage of area occupied by glycogen cells, spongiotrophoblasts, and giant cells in the junctional zone (mean ± SEM; n = 8). (G) Percentage of area occupied by maternal vascular sinus, fetal capillaries, and fetal mesenchyme/trophoblast in the labyrinth zone (mean ± SEM; n = 8). Significant differences were determined by ANOVA post hoc SNK, *P<0.05, **P<0.01. MT, mesometrial triangle; BD, basal decidua; JZ, junctional zone; LZ, labyrinth zone; CV, central vessel; SP, spongiotrophoblast; Arrow, glycogen cells; Arrowhead, fetal capillaries; Asterisks, maternal vascular sinus; KpT1, yes/no day treatment with Kp-10; KpT2, daily treatment with Kp-10; GD, gestational day.
The macroscopic evaluations of fetal-placental development and histological evaluations of the placenta showed that daily treatment with Kp10 had a better effect on fetal and placental development of hypothyroid rats, particularly by improving fetal weight distribution and junctional zone cellularity, while the group KpT1 presented negative effects on fetal organogenesis. For this reason, the placental expression of growth (VEGF, PLGF, IGF1, IGF2) and hormonal (Dio2) factors, and transporters (GLUT1) was evaluated in the KpT2 group in relation to the control and hypothyroid groups, as the altered expression of these factors is associated with altered fetal-placental growth (51–54).
Immunolabeling for VEGF was cytoplasmic and heterogeneous in trophoblasts in the junctional and labyrinth zones, regardless of the experimental group. However, the analysis of the labeled area revealed that the hypothyroid and KpT2 groups had higher expression of VEGF in the junctional zone when compared to the control group (Figures 3A–C, G). This was also observed in the placental gene transcript expression for Vegf, which had higher expression in the hypothyroid and KpT2 groups compared to the control group (Figure 3H; P<0.05). In the labyrinth zone, on the other hand, KpT2 treatment reduced VEGF immunolabeling compared to the hypothyroid group (P<0.001), equaling the control group (Figures 3D–G; P>0.05). Regarding the other placental growth and transport factors, daily treatment with Kp10 significantly increased mRNA expression for Plgf, Igf1, and Glut1 in the placenta when compared to the control and hypothyroid groups (Figure 3H; P<0.05; P<0.01). For Igf2, no difference was observed between the groups (P>0.05), while Dio2 had lower expression in the hypothyroid and KpT2 groups compared to the control group (Figure 3H; P<0.01; P<0.01).
Figure 3 Expression of VEGF, PIGF, IGF1, IGF2, GLUT1 and DIO2 in the placenta of control, hypothyroid, and kisspeptin-10-treated rats on the 18th GD. (A–F) Photomicrographs of immunohistochemical expression of VEGF in the junctional zone (A–C) and labyrinth zone (D–F) (Streptavidin-biotin-peroxidase; Harris hematoxylin; Bar = 50 µm). (G) Immunolabeling area, in pixels, of VEGF expression in the junctional zone and labyrinth zone on the 18 GD (mean ± SEM; n = 8). (H) Relative gene expression of Vegf, Pigf, Igf1, Igf2, Glut1, and Dio2 in the placenta (mean ± SEM; n = 8). Significant differences were determined ANOVA post hoc SNK, *P<0.05, **P<0.01, ***P<0.001, ****P<0.0001. KpT2, daily treatment with Kp10; GD, gestational day.
Since fetal-placental development is influenced by immune mediators produced by the placenta, and considering that previous studies have shown that maternal hypothyroidism affects placental TNFα, MIF, and NOS2 expression in rats (36), the effect of daily treatment with Kp-10 on placental TNFα, IL-10, and IL-6 expression was evaluated in hypothyroid rats. TNFα and IL-10 immunolabeling was cytoplasmic in trophoblastic cells of the junctional and labyrinth zones, and was heterogeneous for TNFα and homogeneous for IL-10 (Figures 4A, C). Immunolabeling for TNFα was more intense in the junctional zone of the Kp10 group than in the control and hypothyroid groups (Figure 4A), as confirmed by the immunolabeling area analysis (Figure 4B; P<0.05). In the labyrinth zone, hypothyroid rats showed higher TNFα expression compared to the control (P<0.05), while the Kp10 treatment was similar to the hypothyroid group (P>0.05). No significant differences in mRNA expression for Tnf were observed between the groups (Figure 4E; P>0.05).
Figure 4 Expression of TNFα, IL-10, and IL-6 in the placenta of control, hypothyroid, and kisspeptin-10-treated rats on the 18th GD. (A) Photomicrographs of immunohistochemical expression of TNFα in the junctional zone and labyrinth zone (Streptavidin-biotin-peroxidase; Harris hematoxylin; Bar = 50 µm). (B) Immunolabeling area, in pixels, of TNFα expression in the junctional zone and labyrinth zone (mean ± SEM; n = 8). (C) Photomicrographs of immunohistochemical expression of IL-10 in the junctional zone and labyrinth zone (Streptavidin-biotin-peroxidase; Harris hematoxylin; Bar = 50 µm). (D) Immunolabeling area, in pixels, of IL-10 expression in the junctional zone and labyrinth zone (mean ± SEM; n = 8). (E) Relative gene expression of Tnf, Il10, and Il6 in the placenta (mean ± SEM; n = 8). Significant differences were determined by ANOVA post hoc SNK, *P<0.05, **P<0.01. KpT2, daily treatment with Kp10; GD, gestational day.
For IL-10, both the hypothyroid and KpT2 groups showed higher immunolabeling in the junctional zone compared to the control (Figure 4C), as confirmed by the immunolabeling area analysis (Figure 4D; P<0.05; P<0.01). In the labyrinth zone, however, while hypothyroidism also increased the IL-10 labeling area when compared to the control (P<0.05), Kp10 treatment reduced IL-10 immunolabeling, matching it to that of the control group (Figure 4D; P>0.05). As observed in the immunohistochemistry, hypothyroidism increased mRNA expression for Il10 compared to the control (P<0.05), while daily treatment with Kp10 showed no significant differences compared to the control and hypothyroid groups (Figure 4E; P>0.05). There was no difference in the mRNA expression for Il6 between the groups (Figure 4E).
Since oxidative stress is associated with placental dysfunction (55–57), and maternal hypothyroidism has been recently reported to cause oxidative damage in rat placenta (40), it was important to evaluate whether daily treatment with Kp10 can reverse or reduce this oxidative stress caused by hypothyroidism. To this end, the gene and/or protein expression profile of HIF1α and 8-Hydroxy-2′-deoxyguanosine (8-OHdG), biomarkers of hypoxia and oxidative DNA damage, respectively (58–60), were analyzed.
HIF1α and 8-OHdG immunolabeling were cytoplasmic and heterogeneous in trophoblastic cells of the junctional and labyrinth zones, regardless of the experimental group (Figures 5A, B). In the HIF1α immunolabeling analysis, both the hypothyroid and Kp10-treated groups showed a larger immunolabeling area in the junctional zone when compared to the control (Figures 5C, P<0.05). In the labyrinth zone, in contrast, no significant differences in immunolabeling were observed between the groups (P>0.05). However, in gene evaluation, similar to the result of junctional zone immunolabeling, the hypothyroid and Kp10-treated groups showed higher placental mRNA expression of Hif1α compared to the control (Figure 5E; P<0.05).
Figure 5 Expression of HIF1α and 8-OHdG in the placenta of control, hypothyroid, and kisspeptin-10-treated rats on the 18th GD. (A) Photomicrographs of immunohistochemical expression of HIF1α in the junctional zone and labyrinth zone (Streptavidin-biotin-peroxidase; Harris hematoxylin; Bar = 50 µm). (B) Immunolabeling area, in pixels, of HIF1α expression in the junctional zone and labyrinth zone (mean ± SEM; n = 8). (C) Immunolabeling area, in pixels, of HIF1α expression in the junctional zone and labyrinth zone (mean ± SEM; n = 8). (D) Relative gene expression of Hif1α in the placenta (mean ± SEM; n = 8). Significant differences were determined by ANOVA post hoc SNK, *P<0.05, **P<0.01. KpT2, daily treatment with Kp10; GD, gestational day.
Regarding 8-OHdG immunolabeling, Kp10 treatment reduced the greater immunolabeling in the junctional zone caused by hypothyroidism (P<0.05), equaling the control (Figure 5D; P >0.05). No difference was observed in 8-OHdG expression in the labyrinth zone between the groups (P>0.05).
Since studies have already demonstrated that exogenous administration of kisspeptin exhibits antioxidant action in the ovary (43), liver (44), and testicle (45), the placental profile of SOD1, catalase, and GPx1/2 was evaluated for the animals in the present study. The immunostaining of SOD1, catalase, and GPx1/2 was cytoplasmic and heterogeneous in trophoblast cells of the junctional and labyrinth zones, and the expression of catalase was weaker in the junctional zone when compared to that of SOD1 and GPx1/2 (Figures 6A, C and E). Treatment with Kp10 significantly increased the expression of SOD1 and catalase in the junctional zone when compared to the control and hypothyroid groups (Figures 6B, D; P<0.01, P<0.0001). In the labyrinth zone, while the KpT2 group showed reduced SOD1 immunolabeling compared to the control (P<0.05), Kp10 treatment increased catalase expression when compared to the hypothyroid group (Figures 6B, D; P<0.05). No significant difference was observed in the GPx1/2 immunolabeling between groups in both the junctional zone and labyrinth zone (Figure 6F; P>0.05). However, in the gene transcript expression analysis, Gpx1, Sod1, and Cat showed higher mRNA expression in the Kp10-treated group when compared to the control and hypothyroid groups (Figure 6G).
Figure 6 Expression of SOD1, catalase, and GPx1/2 in the placenta of control, hypothyroid, and kisspeptin-10-treated rats on the 18th GD. (A) Photomicrographs of immunohistochemical expression of SOD1 in the junctional zone and labyrinth zone (Streptavidin-biotin-peroxidase; Harris hematoxylin; Bar = 50 µm). (B) Immunolabeling area, in pixels, of SOD1 expression in the junctional zone and labyrinth zone (mean ± SEM; n = 8). (C) Photomicrographs of immunohistochemical expression of catalase in the junctional zone and labyrinth zone (Streptavidin-biotin-peroxidase; Harris hematoxylin; Bar = 50 µm). (D) Immunolabeling area, in pixels, of catalase expression in the junctional zone and labyrinth zone (mean ± SEM; n = 8). (E) Photomicrographs of the immunohistochemical expression of GPx1/2 in the junctional zone and labyrinth zone (Streptavidin-biotin-peroxidase; Harris hematoxylin; Bar = 50 µm). (F) Immunolabeling area, in pixels, of GPx1/2 expression in the junctional zone and labyrinth zone (mean ± SEM; n = 8). (G) Relative gene expression of Sod1, catalase, and Gpx1 in the placenta (mean ± SEM; n = 8). Significant differences were determined by ANOVA post hoc SNK, *P<0.05, **P<0.01, ***P<0.001, ****P<0.0001. KpT2, daily treatment with Kp10; GD, gestational day.
Since maternal hypothyroidism causes reticular stress in rat placenta (40), and in vitro and in vivo studies have demonstrated that Kp-10 administration blocks the occurrence of reticular stress in hypothalamic neuronal cells exposed to androgen (46), it was evaluated whether Kp-10 treatment would affect the expression of GRP78, CHOP and ATF4 in the placenta of rats with hypothyroidism. Immunolabeling of these three mediators was cytoplasmic and heterogeneous in the junctional and labyrinth zones, and expression in the junctional zone was usually in isolated spongiotrophoblast cells or in glycogen cells (Figures 7A, C and E). According to the immunolabeling area analysis, the hypothyroid group showed higher immunolabeling of GRP78 and CHOP in the junctional zone when compared to the control (Figures 7B, D; P<0.05), while the KpT2 group showed no significant difference compared to the hypothyroid group for CHOP. However, with respect to GRP78, Kp-10 treatment increased immunolabeling in the junctional zone when compared to the control and hypothyroid groups (Figure 7B; P<0.05; P<0.01). In contrast, in the labyrinth zone, KpT2 treatment reduced the immunolabeled area of both GRP78 and CHOP relative to the hypothyroid group (Figures 7B, D; P<0.05; P<0.01). For ATF4, no differences in the immunolabeling area were observed between the groups (Figures 7F; P>0.05).
Figure 7 Expression of GRP78, CHOP, and ATF4 in the placenta of control, hypothyroid, and kisspeptin-10-treated rats on the 18th GD. (A) Photomicrographs of immunohistochemical expression of GRP78 in the junctional zone and labyrinth zone (Streptavidin-biotin-peroxidase; Harris hematoxylin; Bar = 50 µm). (B) Immunolabeling area, in pixels, of GRP78 expression in the junctional zone and labyrinth zone (mean ± SEM; n = 8). (C) Photomicrographs of immunohistochemical expression of CHOP in the junctional zone and labyrinth zone (Streptavidin-biotin-peroxidase; Harris hematoxylin; Bar = 50 µm). (D) Immunolabeling area, in pixels, of CHOP expression in the junctional zone and labyrinth zone (mean ± SEM; n = 8). (E) Photomicrographs of immunohistochemical expression of ATF4 in the junctional zone and labyrinth zone (Streptavidin-biotin-peroxidase; Harris hematoxylin; Bar = 50 µm). (F) Immunolabeling area, in pixels, of ATF4 expression in the junctional zone and labyrinth zone (mean ± SEM; n = 8). (G) Relative gene expression of Grp78 and Chop in the placenta (mean ± SEM; n = 8). Significant differences were determined by ANOVA post hoc SNK, *P<0.05, **P<0.01, ****P<0.0001. KpT2, daily treatment with Kp10; GD, gestational day.
In the gene transcript analysis, treatment with Kp-10 increased the placental expression of Grp78 compared to the control and hypothyroid groups (P<0.0001), as observed in junctional zone immunolabeling, while an increase in Chop gene expression was also observed relative to the hypothyroid group (Figure 7G; P<0.05). Taken together, these results demonstrate that daily treatment with Kp10 differentially affects the expression of reticular stress mediators in the placenta of hypothyroid rats since it maintains high expression in the junctional zone and reduces immunolabeling in the labyrinth zone.
Although the kisspeptin/Kiss1R system is mainly known for its action on the hypothalamic-pituitary-gonadal axis, stimulating the secretion of GnRH/LH, studies have shown that kisspeptin has peripheral action in the genital tract (5, 6). and gestational dysfunctions such as preeclampsia, spontaneous abortion, gestational diabetes, obesity, and even maternal hypothyroidism, are associated with alterations in the serum and/or placental profile of kisspeptin (12, 17, 20, 26–28, 30, 31). The results of the present study demonstrated that daily kisspeptin treatment was able to improve fetal development and placental morphology in hypothyroid rats. This improvement in fetal-placental development was associated with both increased expression of growth and transport factors in the placenta (Plgf, Igf1, and Glut1), and with blockage of placental oxidative damage and increased antioxidant enzymes (SOD1, catalase, GPx1).
Daily treatment with Kp-10 from the 8th GD not only increased maternal weight gain and plasma free T3 and T4 levels of hypothyroid rats, but also improved fetal weight distribution, which was not observed in the treatment performed every other day. These results reaffirm the need for precise control of kisspeptin plasma levels during pregnancy, since only daily treatment with Kp-10 had a positive effect on maternal weight gain, fetal weight distribution and on free T3 and T4 levels. This is the first in vivo study to demonstrate that kisspeptin can modulate the Corroborating our study, Radwańska and Kosior-Korzecka (61) demonstrated that in vitro treatment of ovine pituitary cells with 10−11 to 10−8 M of Kp10 increased TSH secretion. However, despite the increase in plasma levels of free T3 and T4 caused by the daily treatment with Kp-10, it is suggested that the local availability of T3 in the placenta has not changed, since the treatment with Kp-10 did not change the lower placental expression of Dio2 caused by hypothyroidism, the enzyme responsible for the intracellular availability of T3 (62). This reduction of Dio2 in the placenta of the hypothyroid rats may be associated with fetoplacental dysfunction presented by these animals, since Dio2 is involved in proliferation, differentiation and trophoblastic migration and low placental expression was associated early recurrent miscarriage (54).
The increase in the maternal weight gain and improviment fetal weight distribution of hypothyroid rats may be associated not only with increased levels of free T3 and T4 but also with the action of kisspeptin on maternal pancreatic function, as exogenous kisspeptin increases in vivo and in vitro insulin secretion by pancreatic β-cells in rats, mice, humans, and non-human primates (63–67), and pharmacological blockade or in vivo genetic ablation of the Kiss1R receptor in β-cells of pregnant mice results in glucose intolerance and impaired insulin secretion (68).
For fetal weight distribution, most fetuses (61.49%) of the hypothyroid group were below the 5th percentile of the control group, indicating the growth restriction (48, 50, 69, 70). Once the reduced body weight was accompanied by reduced fetal organ weight (heart and lung), prioritizing the brain development, we suggest the growth restriction caused by hypothyroidism was asymmetric, even though was no difference in the liver/brain ratio (48, 50, 71). Daily treatment with Kp-10 improved fetal weight distribution once most of the fetal weight (87.30%) were above the 5th percentile of the control group. This outcome in terms of fetal development is very important, as fetal growth restriction is associated with an increased risk of cardiovascular and metabolic diseases in postnatal life (51, 72).
However, optimal fetal development depends not only on maternal and fetal metabolism but also on adequate placental function (32). In the present study, daily treatment with Kp10 also improved the placental morphology of hypothyroid rats by restoring the proportion of trophoblastic cells forming the junctional zone. Previous studies have also demonstrated altered trophoblast population in the junctional zone of hypothyroid rats (35). The cells in the junctional zone are mainly responsible for the synthesis and secretion of peptides and hormones by the placenta, which are fundamental for controlling maternal and fetal metabolism (73).
In addition to improving placental morphology, daily treatment with Kp-10 increased mRNA expression for Plgf, Igf1, and Glut1 in the placenta. These factors are decisive for proper fetal growth and placental development (32, 51, 74, 75). PLGF is responsible for placental vascular bed maturation (76), and low plasma PLGF levels are associated with fetal growth restriction, HELLP syndrome, preeclampsia, and gestational hypertension (74). Therefore, plasma PLGF is considered an important predictor of fetal development (74). Reduced placental expression of IGF1 also compromises fetal and placental development (75), while GLUT1 is an important placental glucose transporter. The expression of GLUT1 is reduced when the placental expression of IGF1 fails (77, 78).Therefore, in the present study, restored fetal weight distribution caused by Kp-10 treatment may have also resulted from increased placental IGF1/GLUT1 signaling, as kisspeptin stimulates the release of insulin (63–68).
However, in relation to VEGF, treatment with Kp-10 did not reverse the increase in placental gene and protein expression caused by hypothyroidism, particularly in the junctional zone. In addition to the critical role of VEGF in the process of placental angiogenesis, especially in early to mid-gestation (79), it is an indicator of hypoxia since low oxygen levels stimulate its expression (80). In this sense, higher gene expression of VEGF in the placenta of hypothyroid and Kp-10-treated animals, and the higher immunolabeling in the junctional zone, suggest a low oxygen tension environment in the junctional zone of these animals. This hypothesis is supported by the higher gene and protein expression of HIF1α, especially in the junctional zone, observed in the hypothyroid and Kp-10-treated animals. HIF1α is expressed under hypoxic conditions and performs its functions in vascular permeability and cell survival by signaling via VEGF (81). Furthermore, studies have already demonstrated increased expression of HIF1α and VEGF in preeclamptic placentas, which are known to present a hypoxic environment (81–83). However, when evaluating the labyrinth zone, Kp10 treatment reduced the increase in VEGF immunolabeling caused by hypothyroidism, matching its expression to that of the control animals. These data reinforce the importance of immunohistochemistry analysis to discriminate and adequately evaluate the protein expression profile of the mediators evaluated in each region of the placenta.
Regarding TNFα, Kp10 treatment increased immunolabeling in the junctional zone, while in the labyrinth zone, hypothyroid animals showed higher expression compared to the control animals. In vitro studies have shown that TNFα secreted by decidual macrophages is recognized by TNF-R1 receptors in the trophoblasts and activates the extrinsic apoptosis pathway (84), while, according to a recent study, kisspeptin increases cell apoptosis (85). Therefore, Kp-10 treatment may have increased activation of placental apoptosis via TNFα signaling, although studies are needed to confirm this hypothesis. However, increased TNFα also occurs with increasing insulin resistance (86). Thus, another possibility to explain its higher expression in the junctional zone of Kp-10-treated animals may be the increase in insulin that occurs after kisspeptin administration (63–68).
As observed for TNFα, IL-10 showed higher expression in the junctional zone of hypothyroid and Kp-10-treated animals compared to the control, as well as higher gene expression in the placenta of hypothyroid animals. This increase in IL-10 may be a reflection of increased TNFα expression, as IL-10 attenuates the effects of pro-inflammatory cytokines at the maternal-fetal interface (38, 87) and TNFα stimulates the in vitro secretion of IL-10 in first-trimester trophoblastic villi (88). However, Kp-10 treatment reduced the higher IL-10 immunolabeling in the labyrinth zone caused by hypothyroidism, matching it to that of the control. Further studies are needed to elucidate the reduction of IL10 and VEGF in the labyrinth zone of the hypothyroid rats caused by Kp10 treatment.
As previously described, the increased mRNA and immunolabeling of HIF1α in the junctional zone of hypothyroid and Kp-10-treated animals signals low oxygen tension and suggests the occurrence of oxidative stress in the placenta of hypothyroid rats, which was recently confirmed in a previous study (40). Activation of HIF1α in the placenta of rats also favors the reduction of labyrinth zone thickness (89), thus corroborating the histomorphometry results of the present study. Moreover, Kp-10 treatment blocked the increase in placental immunolabeling of 8-OHdG caused by hypothyroidism. 8-OHdG is a biomarker of oxidative stress that signals DNA damage resulting from oxidative stress and lipid peroxidation (90), and studies have shown increased placental expression in preeclampsia, gestational diabetes mellitus, and maternal smoking (60, 90, 91). Thus, the results of the present study suggest that although Kp-10 treatment was not able to protect against placental hypoxia caused by maternal hypothyroidism, it was effective in protecting against the occurrence of placental oxidative damage.
Daily treatment with Kp-10 also increased the gene and/or protein expression of SOD1, catalase, and GPx1 in the placenta of hypothyroid rats. This suggests an antioxidant function of kisspeptin at the maternal-fetal interface. These results are in line with those of previous studies conducted on the ovary (43), liver (44), and testicle (45), that demonstrated increased antioxidant enzymes after kisspeptin treatment. Thus, together, these data suggest that in the condition of placental oxidative stress caused by hypothyroidism, treatment with Kp-10 can improve placental antioxidant defenses, inhibit oxidative damage, and, consequently, improve fetus and placenta development.
Furthermore, hypothyroidism increased immunolabeling in the junctional zone of endoplasmic reticulum stress mediators, GRP78, and CHOP, another pathophysiological process involved in placental dysfunction in preeclamptic women (92–94) and that has been recently demonstrated in the placenta of hypothyroid rats (40). Interestingly, although treatment with Kp-10 was not able to reverse the expression of these mediators in the junctional zone of hypothyroid rats, including with increased placental gene expression of Grp78 and Chop when compared to the hypothyroid group, Kp-10 treatment reduced GRP78 and CHOP immunolabeling in the labyrinth zone of hypothyroid animals. This suggests attenuation of reticular stress in this placental layer after Kp-10 treatment. Furthermore, a recent study demonstrated in vitro in hypothalamic GT1-7 cells, that silencing of Kiss1 or inhibition of Kiss1r with the antagonist Kp234 resulted in endoplasmic reticulum stress, while treatment with Kp-10 blocked its activation (46). Since maternal hypothyroidism in rats reduces placental and decidual expression of the Kiss/Kiss1r system (31), this may also be one of the reasons for the activation of reticular stress in the placenta of these animals (40).
The findings of this study demonstrated that daily treatment with Kp-10 in hypothyroid pregnant rats was able to improve fetus and placenta development and the plasma free T3 and T4 levels. This improvement was associated with not only increased expression of placental growth factors and antioxidant enzymes, but also with blockage of oxidative damage and positive modulation of reticular stress mediators, IL-10, and VEGF in the labyrinth zone. This is the first study to use kisspeptin as a therapeutic tool in a gestational disease.
The raw data supporting the conclusions of this article will be made available by the authors, without undue reservation.
The animal study was reviewed and approved by Ethics Committee on the Use of Animals of Santa Cruz State University (UESC) (Protocol 036/16).
BS and JS conceived and designed the study. BS, JA, EB, and LS conducted sample collection. BS and EB conducted the evaluation of maternal and fetus data. BS conducted histopathological preparation and analysis. BS, JA, LS, ES, LM, IM, and JS performed immunohistochemistry analysis. BS, JA, LS, and JS performed qRT-PCR. BS and JS analyzed data. JS, ML, RES, and RS provided reagents. BS and JS wrote the paper. All authors read and approved the manuscript. All authors contributed to the article and approved the submitted version.
This work was supported by Conselho Nacional de Desenvolvimento Cientifico e Tecnologico (CNPq), Universidade Estadual de Santa Cruz (UESC) and Coordenaçao de Aperfeiçoamento de Pessoal de Nivel Superior (Capes).
The authors thank Ivo Arouca (Universidade Estadual de Santa Cruz, Ilheus, Brazil) by the technical support provided.
The authors declare that the research was conducted in the absence of any commercial or financial relationships that could be construed as a potential conflict of interest.
All claims expressed in this article are solely those of the authors and do not necessarily represent those of their affiliated organizations, or those of the publisher, the editors and the reviewers. Any product that may be evaluated in this article, or claim that may be made by its manufacturer, is not guaranteed or endorsed by the publisher.
The Supplementary Material for this article can be found online at: https://www.frontiersin.org/articles/10.3389/fendo.2022.908240/full#supplementary-material
Supplementary Figure 1 | Immunohistochemistry. (A–B) Representative images of the junctional zone (A) and the labyrinth zone (B) in the negative control. (C) Antibodies used with their respective dilutions and incubation and revelation times.
1. Kotani M, Detheux M, Vandenbogaerde A, Communi D, Vanderwinden J-M, Le Poul E, et al. The metastasis suppressor gene KiSS-1 encodes kisspeptins, the natural ligands of the orphan G protein-coupled receptor GPR54. Cell Mol Life Sci (2019) 76:3479–96. doi: 10.1074/jbc.M104847200
2. Ohtaki T, Shintani Y, Honda S, Matsumoto H, Hori A, Kanehashi K, et al. Metastasis suppressor gene KiSS-1 encodes peptide ligand of a G-protein-coupled receptor. Nature (2001) 411:613–7. doi: 10.1038/35079135
3. de Roux N, Genin E, Carel J-C, Matsuda F, Chaussain J-L, Milgrom E. Hypogonadotropic hypogonadism due to loss of function of the KiSS1-derived peptide receptor GPR54. Proc Natl Acad Sci (2003) 100:10972–6. doi: 10.1073/pnas.1834399100
4. Seminara SB, Messager S, Chatzidaki EE, Thresher RR, Acierno JS, Shagoury JK, et al. The GPR54 gene as a regulator of puberty. N Engl J Med (2003) 349:1614–27. doi: 10.1056/NEJMoa035322
5. Cao Y, Li Z, Jiang W, Ling Y, Kuang H. Reproductive functions of Kisspeptin/KISS1R systems in the periphery. Reprod Biol Endocrinol (2019) 17:1–9. doi: 10.1186/s12958-019-0511-x
6. D’Occhio MJ, Campanile G, Baruselli PS, D’Occhio MJ, Campanile G, Baruselli PS. Peripheral action of kisspeptin at reproductive tissues–role in ovarian function and embryo implantation and relevance to assisted reproductive technology in livestock: a review. Biol Reprod (2020) 103:1157–70. doi: 10.1093/biolre/ioaa135
7. Hu K-LL, Chang H-MM, Zhao H-CC, Yu Y, Li R, Qiao J. Potential roles for the kisspeptin/kisspeptin receptor system in implantation and placentation. Hum Reprod Update (2019) 25:326–43. doi: 10.1093/humupd/dmy046
8. Fang L, Gao Y, Wang Z, Li Y, Yan Y, Wu Z, et al. EGF stimulates human trophoblast cell invasion by downregulating ID3-mediated KISS1 expression. Cell Commun Signal (2021) 19:1–13. doi: 10.1186/s12964-021-00783-2
9. Fang L, Yan Y, Gao Y, Wu Z, Wang Z, Yang S, et al. TGF-β1 inhibits human trophoblast cell invasion by upregulating kisspeptin expression through ERK1 / 2 but not SMAD signaling pathway. Reprod Biol Endocrinol (2022) 20:1–12. doi: 10.1186/s12958-022-00902-9
10. Harter CJLL, Kavanagh GS, Smith JT. The role of kisspeptin neurons in reproduction and metabolism. J Endocrinol (2018) 238:R173–83. doi: 10.1530/JOE-18-0108
11. Hiden U, Bilban M, Knöfler M, Desoye G. Kisspeptins and the placenta: Regulation of trophoblast invasion. Rev Endocr Metab Disord (2007) 8:31–9. doi: 10.1007/s11154-007-9030-8
12. Hu K-LL, Zhao H, Yu Y, Li R. Kisspeptin as a potential biomarker throughout pregnancy. Eur J Obstet Gynecol Reprod Biol (2019) 240:261–6. doi: 10.1016/j.ejogrb.2019.07.016
13. Bilban M, Ghaffari-Tabrizi N, Hintermann E, Bauer S, Molzer S, Zoratti C, et al. Kisspeptin-10, a KiSS-1/metastin-derived decapeptide, is a physiological invasion inhibitor of primary human trophoblasts. J Cell Sci (2004) 117:1319–28. doi: 10.1242/jcs.00971
14. Fayazi M, Calder M, Bhattacharya M, Vilos GA, Power S, Babwah AV. The pregnant mouse uterus exhibits a functional kisspeptin/KISS1R signaling system on the day of embryo implantation. Reprod Biol Endocrinol (2015) 13:1–6. doi: 10.1186/s12958-015-0105-1
15. Gorbunova OL, Shirshev SV. The role of kisspeptin in immune tolerance formation during pregnancy. Dokl Biol Sci (2014) 457:258–60. doi: 10.1134/S0012496614040085
16. Zhang P, Tang M, Zhong T, Lin Y, Zong T, Zhong C, et al. Expression and function of kisspeptin during mouse decidualization. PloS One (2014) 9:1–8. doi: 10.1371/journal.pone.0097647
17. Park DW, Lee SK, Hong SR, Han AR, Kwak-Kim J, Yang KM. Expression of kisspeptin and its receptor GPR54 in the first trimester trophoblast of women with recurrent pregnancy loss. Am J Reprod Immunol (2012) 67:132–9. doi: 10.1111/j.1600-0897.2011.01073.x
18. Sullivan-Pyke C, Haisenleder DJ, Senapati S, Nicolais O, Eisenberg E, Sammel MD, et al. Kisspeptin as a new serum biomarker to discriminate miscarriage from viable intrauterine pregnancy. Fertil Steril (2018) 109:137–141.e2. doi: 10.1016/j.fertnstert.2017.09.029
19. Wu S, Zhang H, Tian J, Liu L, Dong Y, Mao T. Expression of kisspeptin/GPR54 and PIBF/PR in the first trimester trophoblast and decidua of women with recurrent spontaneous abortion. Pathol Res Pract (2014) 210:47–54. doi: 10.1016/j.prp.2013.09.017
20. Logie JJ, Denison FC, Riley SC, Ramaesh T, Forbes S, Norman JE, et al. Evaluation of kisspeptin levels in obese pregnancy as a biomarker for pre-eclampsia. Clin Endocrinol (Oxf) (2012) 76:887–93. doi: 10.1111/j.1365-2265.2011.04317.x
21. Kołodziejski PA, Pruszyńska-Oszmałek E, Korek E, Sassek M, Szczepankiewicz D, Kaczmarek P, et al. Serum levels of spexin and kisspeptin negatively correlate with obesity and insulin resistance in women. Physiol Res (2018) 67:45–56. doi: 10.33549/physiolres.933467
22. Pérez-López FR, López-Baena MT, Varikasuvu SR, Ruiz-Román R, Fuentes-Carrasco M, Savirón-Cornudella R. Preeclampsia and gestational hypertension are associated to low maternal circulating kisspeptin levels: a systematic review and meta-analysis. Gynecol Endocrinol (2021) 37:1055–62. doi: 10.1080/09513590.2021.2004396
23. Adali E, Kurdoglu Z, Kurdoglu M, Kamaci M, Kolusari A, Yildizhan R. Metastin levels in pregnancies complicated by pre-eclampsia and their relation with disease severity. J Matern Neonatal Med (2012) 25:2671–5. doi: 10.3109/14767058.2012.708369
24. Armstrong RA, Reynolds RM, Leask R, Shearing CH, Calder AA, Riley SC. Decreased serum levels of kisspeptin in early pregnancy are associated with intra-uterine growth restriction and pre-eclampsia. Prenat Diagn (2009) 29:982–5. doi: 10.1002/pd.2328
25. Cartwright JE, Williams PJ. Altered placental expression of kisspeptin and its receptor in pre-eclampsia. J Endocrinol (2012) 214:79–85. doi: 10.1530/JOE-12-0091
26. Ćetković A, Miljic D, Ljubić A, Patterson M, Ghatei M, Stamenkoví J, et al. Plasma kisspeptin levels in pregnancies with diabetes and hypertensive disease as a potential marker of placental dysfunction and adverse perinatal outcome. Endocr Res (2012) 37:78–88. doi: 10.3109/07435800.2011.639319
27. Colak E, Ozcimen EE, Erinanç OH, Tohma YA, Ceran MU. Is placental KISS-1 expression associated with first trimester abortion spontaneous? Obstet Gynecol Sci (2020) 63:490–6. doi: 10.5468/OGS.19242
28. Kapustin RV, Drobintseva AO, Alekseenkova EN, Onopriychuk AR, Arzhanova ON, Polyakova VO, et al. Placental protein expression of kisspeptin-1 (KISS1) and the kisspeptin-1 receptor (KISS1R) in pregnancy complicated by diabetes mellitus or preeclampsia. Arch Gynecol Obstet (2020) 301:437–45. doi: 10.1007/s00404-019-05408-1
29. Li L, Tian J, Zhou L, Wu S, Zhang S, Qi L, et al. Role of kisspeptin/GPR54 in the first trimester trophoblast of women with a history of recurrent spontaneous abortion. Int J Clin Exp Pathol (2017) 10:8161–73.
30. Matjila M, Millar R, van der Spuy Z, Katz A. Elevated placental expression at the maternal-fetal interface but diminished maternal circulatory kisspeptin in preeclamptic pregnancies. Pregnancy Hypertens (2016) 6:79–87. doi: 10.1016/j.preghy.2015.11.001
31. Santos BR, dos Anjos Cordeiro JM, Santos LC, de Oliveira LS, Mendonça LD, Santos EO, et al. Maternal hypothyroidism reduces the expression of the kisspeptin/Kiss1r system in the maternal-fetal interface of rats. Reprod Biol (2022) 22:16–20. doi: 10.1016/j.repbio.2022.100615
32. Newbern D, Freemark M. Placental hormones and the control of maternal metabolism and fetal growth. Curr Opin Endocrinol Diabetes Obes (2011) 18:409–16. doi: 10.1097/MED.0b013e32834c800d
33. Kurlak LO, Mistry HD, Kaptein E, Visser TJ, Broughton Pipkin F. Thyroid hormones and their placental deiodination in normal and pre-eclamptic pregnancy. Placenta (2013) 34:395–400. doi: 10.1016/j.placenta.2013.02.009
34. Silva JF, Ocarino NM, Serakides R. Thyroid hormones and female reproduction†. Biol Reprod (2018) 99:907–21. doi: 10.1093/biolre/ioy115
35. Silva JF, Vidigal PN, Galvão DD, Boeloni JN, Nunes PP, Ocarino NM, et al. Fetal growth restriction in hypothyroidism is associated with changes in proliferative activity, apoptosis and vascularisation of the placenta. Reprod Fertil Dev (2012) 24:923. doi: 10.1071/RD11219
36. Silva JF, Ocarino NM, Serakides R. Maternal thyroid dysfunction affects placental profile of inflammatory mediators and the intrauterine trophoblast migration kinetics. Reproduction (2014) 147:803–16. doi: 10.1530/REP-13-0374
37. Silva JF, Ocarino NM, Serakides R. Placental angiogenic and hormonal factors are affected by thyroid hormones in rats. Pathol Res Pract (2015) 211:226–34. doi: 10.1016/j.prp.2014.11.003
38. Silva JF, Ocarino NM, Serakides R. Spatiotemporal expression profile of proteases and immunological, angiogenic, hormonal and apoptotic mediators in rat placenta before and during intrauterine trophoblast migration. Reprod Fertil Dev (2017) 29:1774–86. doi: 10.1071/RD16280
39. Souza CA, Silva JF, Ocarino NM, Silva CLR, Gomes LA, Assunção GSM, et al. Efeito do hipotireoidismo materno na expressão espaço-temporal de mediadores imunológicos e população de células natural killers na decídua e na glândula metrial de ratas. Arq Bras Med Veterinária e Zootec (2020) 72:177–90. doi: 10.1590/1678-4162-10697
40. dos A Cordeiro JM, Santos BR, Santos LC, Santos EO, de Oliveira LS, Barbosa EM, et al. “Manganese porphyrin-based treatment blocks placental stress caused by maternal hypothyroidism and improves placental morphogenesis and fetal development in a rat experimental model.,”. In: Bronstein MD, editor. Archives of archives of and metabolism. São Paulo: Brazilian Society of Endocrinology and Metabolism (2021). p. S11.
41. Centanni M, Benvenga S, Sachmechi I. Diagnosis and management of treatment-refractory hypothyroidism: an expert consensus report. J Endocrinol Invest (2017) 40:1289–301. doi: 10.1007/s40618-017-0706-y
42. de Oliveira LS, da Silva TQM, Barbosa EM, dos A Cordeiro JM, Santos LC, Henriques PC, et al. “Kisspeptin treatment restores ovarian function in hypothyroid rats.,”. In: Armani ALC, de A Araújo EJ, editors. Annals of the III international symposium of experimental pathology. Londrina: UEL (2021). p. 42.
43. Aslan M, Erkanli Senturk G, Akkaya H, Sahin S, Yılmaz B. The effect of oxytocin and kisspeptin-10 in ovary and uterus of ischemia-reperfusion injured rats. Taiwan J Obstet Gynecol (2017) 56:456–62. doi: 10.1016/j.tjog.2016.12.018
44. Aydin M, Oktar S, Yonden Z, Ozturk OH, Yilmaz B. Direct and indirect effects of kisspeptin on liver oxidant and antioxidant systems in young male rats. Cell Biochem Funct (2010) 28:293–9. doi: 10.1002/cbf.1656
45. Akkaya H, Eyuboglu S, Erkanlı Senturk G, Yilmaz B. Investigation of the effects of kisspeptin-10 in methionine-induced lipid peroxidation in testicle tissue of young rats. J Biochem Mol Toxicol (2017) 31:1–7. doi: 10.1002/jbt.21881
46. Yuan C, Huang WQ, Guo JH, Liu XY, Yang JZ, Chen JJ, et al. Involvement of kisspeptin in androgen-induced hypothalamic endoplasmic reticulum stress and its rescuing effect in PCOS rats. Biochim Biophys Acta - Mol Basis Dis (2021) 1867:166242. doi: 10.1016/j.bbadis.2021.166242
47. Napso T, Hung Y-P, Davidge ST, Care AS, Sferruzzi-Perri AN. Advanced maternal age compromises fetal growth and induces sex-specific changes in placental phenotype in rats. Sci Rep (2019) 9:16916. doi: 10.1038/s41598-019-53199-x
48. Dilworth MR, Kusinski LC, Baker BC, Renshall LJ, Greenwood SL, Sibley CP, et al. Defining fetal growth restriction in mice: A standardized and clinically relevant approach. Placenta (2011) 32:914–6. doi: 10.1016/j.placenta.2011.08.007
49. Solano ME, Thiele K, Kowal MK, Arck PC. Identification of suitable reference genes in the mouse placenta. Placenta (2016) 39:7–15. doi: 10.1016/j.placenta.2015.12.017
50. Charnock JC, Dilworth MR, Aplin JD, Sibley CP, Westwood M, Crocker IP. The impact of a human IGF-II analog ([Leu27]IGF-II) on fetal growth in a mouse model of fetal growth restriction. Am J Physiol - Endocrinol Metab (2015) 310:E24–31. doi: 10.1152/ajpendo.00379.2015
51. Fowden AL, Forhead AJ, Coan PM, Burton GJ. The placenta and intrauterine programming. J Neuroendocrinol (2008) 20:439–50. doi: 10.1111/j.1365-2826.2008.01663.x
52. Vrachnis N, Kalampokas E, Sifakis S, Vitoratos N, Kalampokas T, Botsis D, et al. Placental growth factor (PlGF): A key to optimizing fetal growth. J Matern Neonatal Med (2013) 26:995–1002. doi: 10.3109/14767058.2013.766694
53. Balachandiran M, Bobby Z, Dorairajan G, Gladwin V, Vinayagam V, Packirisamy RM. Decreased maternal serum adiponectin and increased insulin-like growth factor-1 levels along with increased placental glucose transporter-1 expression in gestational diabetes mellitus: Possible role in fetal overgrowth: Regulation of placental GLUT-1 expr. Placenta (2021) 104:71–80. doi: 10.1016/j.placenta.2020.11.008
54. Adu-Gyamfi EA, Lamptey J, Chen X-M, Li F-F, Li C, Ruan L-L, et al. Iodothyronine deiodinase 2 (DiO2) regulates trophoblast cell line cycle, invasion and apoptosis; and its downregulation is associated with early recurrent miscarriage. Placenta (2021) 111:54–68. doi: 10.1016/j.placenta.2021.06.004
55. Burton GJ, Yung HW, Cindrova-Davies T, Charnock-Jones DS. Placental endoplasmic reticulum stress and oxidative stress in the pathophysiology of unexplained intrauterine growth restriction and early onset preeclampsia. Placenta (2009) 30:43–8. doi: 10.1016/j.placenta.2008.11.003
56. Yung HW, Hemberger M, Watson ED, Senner CE, Jones CP, Kaufman RJ, et al. Endoplasmic reticulum stress disrupts placental morphogenesis: Implications for human intrauterine growth restriction. J Pathol (2012) 228:554–64. doi: 10.1002/path.4068
57. Yung HW, Atkinson D, Campion-Smith T, Olovsson M, Charnock-Jones DS, Burton GJ. Differential activation of placental unfolded protein response pathways implies heterogeneity in causation of early- and late-onset pre-eclampsia. J Pathol (2014) 234:262–76. doi: 10.1002/path.4394
58. Rosario GX, Konno T, Soares MJ. Maternal hypoxia activates endovascular trophoblast cell invasion. Dev Biol (2008) 314:362–75. doi: 10.1016/j.ydbio.2007.12.007
59. Soares MJ, Iqbal K, Kozai K. Hypoxia and placental development. Birth defects Res (2017) 109:1309–29. doi: 10.1002/bdr2.1135
60. Urbaniak SK, Boguszewska K, Szewczuk M, Kázmierczak-Barańska J, Karwowski BT. 8-Oxo-7,8-dihydro-2′-deoxyguanosine (8-oxodG) and 8-hydroxy-2′-deoxyguanosine (8-OHdG) as a potential biomarker for gestational diabetes mellitus (GDM) development. Molecules (2020) 25:202. doi: 10.3390/molecules25010202
61. Radwańska P, Kosior-Korzecka U. Relationships between leptin, KiSS-1/GPR54 expression and TSH secretion from pituitary cells of pubertal ewes in vitro. Res Vet Sci (2016) 105:180–7. doi: 10.1016/j.rvsc.2016.02.014
62. Adu-Gyamfi EA, Wang Y-X, Ding Y-B. The interplay between thyroid hormones and the placenta: a comprehensive review†. Biol Reprod (2020) 102:8–17. doi: 10.1093/biolre/ioz182
63. Bowe JE, King AJ, Kinsey-Jones JS, Foot VL, Li XF, O’Byrne KT, et al. Kisspeptin stimulation of insulin secretion: Mechanisms of action in mouse islets and rats. Diabetologia (2009) 52:855–62. doi: 10.1007/s00125-009-1283-1
64. Bowe JE, Foot VL, Amiel SA, Huang GC, Lamb M, Lakey J, et al. GPR54 peptide agonists stimulate insulin secretion from murine, porcine and human islets. Islets (2012) 4:20–3. doi: 10.4161/isl.18261
65. Izzi-Engbeaya C, Comninos AN, Clarke SA, Jomard A, Yang L, Jones S, et al. The effects of kisspeptin on β-cell function, serum metabolites and appetite in humans. Diabetes Obes Metab (2018) 20:2800–10. doi: 10.1111/dom.13460
66. Schwetz TA, Reissaus CA, Piston DW. Differential stimulation of insulin secretion by glp-1 and kisspeptin-10. PLoS One (2014) 9:1–10. doi: 10.1371/journal.pone.0113020
67. Wahab F, Riaz T, Shahab M. Study on the effect of peripheral kisspeptin administration on basal and glucose-induced insulin secretion under fed and fasting conditions in the adult male rhesus monkey (Macaca mulatta). Horm Metab Res (2011) 43:37–42. doi: 10.1055/s-0030-1268458
68. Bowe JE, Hill TG, Hunt KF, Smith LIF, Simpson SJS, Amiel SA, et al. A role for placental kisspeptin in β cell adaptation to pregnancy. JCI Insight (2019) 4:e124540. doi: 10.1172/jci.insight.124540
69. Kusinski LC, Stanley JL, Dilworth MR, Hirt CJ, Andersson IJ, Renshall LJ, et al. eNOS knockout mouse as a model of fetal growth restriction with an impaired uterine artery function and placental transport phenotype. Am J Physiol - Regul Integr Comp Physiol (2012) 303:R86–93. doi: 10.1152/ajpregu.00600.2011
70. Renshall LJ, Morgan HL, Moens H, Cansfield D, Finn-Sell SL, Tropea T, et al. Melatonin increases fetal weight in wild-type mice but not in mouse models of fetal growth restriction. Front Physiol (2018) 9:1141. doi: 10.3389/fphys.2018.01141
71. Shrivastava D, Master A. Fetal growth restriction. J Obstet Gynecol India (2020) 70:103–10. doi: 10.1007/s13224-019-01278-4
72. Burton GJ, Fowden AL, Thornburg KL. Placental origins of chronic disease. Physiol Rev (2016) 96:1509–65. doi: 10.1152/physrev.00029.2015
73. Silva JF, Serakides R. Intrauterine trophoblast migration: A comparative view of humans and rodents. Cell Adh Migr (2016) 10:88–110. doi: 10.1080/19336918.2015.1120397
74. Cowans NJ, Stamatopoulou A, Matwejew E, von Kaisenberg CS, Spencer K. First-trimester placental growth factor as a marker for hypertensive disorders and SGA. Prenat Diagn (2010) 30:565–70. doi: 10.1002/pd.2525
75. Sferruzzi-Perri AN, Sandovici I, Constancia M, Fowden AL. Placental phenotype and the insulin-like growth factors: resource allocation to fetal growth. J Physiol (2017) 595:5057–93. doi: 10.1113/JP273330
76. Torry DS, Ahn H, Barnes EL, Torry RJ. Placenta growth factor: Potential role in pregnancy. Am J Reprod Immunol (1999) 41:79–85. doi: 10.1111/j.1600-0897.1999.tb00078.x
77. Desoye G, Gauster M, Wadsack C. Placental transport in pregnancy pathologies. Am J Clin Nutr (2011) 94:1896–902. doi: 10.3945/ajcn.110.000851
78. Lüscher BP, Marini C, Joerger-Messerli MS, Huang X, Hediger MA, Albrecht C, et al. Placental glucose transporter (GLUT)-1 is down-regulated in preeclampsia. Placenta (2017) 55:94–9. doi: 10.1016/j.placenta.2017.04.023
79. Ahmed A, Dunk C, Ahmad S, Khaliq A. Regulation of placental vascular endothelial growth factor (VEGF) and placenta growth factor (PIGF) and soluble flt-1 by oxygen–a review. Placenta (2000) 21 Suppl:A:S16–24. doi: 10.1053/plac.1999.0524
80. Ferrara N. Vascular endothelial growth factor: Basic science and clinical progress. Endocr Rev (2004) 25:581–611. doi: 10.1210/er.2003-0027
81. Kaelin WG, Ratcliffe PJ. Oxygen sensing by metazoans: The central role of the HIF hydroxylase pathway. Mol Cell (2008) 30:393–402. doi: 10.1016/j.molcel.2008.04.009
82. Ali LE, Salih MM, Elhassan EM, Mohmmed AA, Adam I. Placental growth factor, vascular endothelial growth factor, and hypoxia-inducible factor-1α in the placentas of women with pre-eclampsia. J Matern Neonatal Med (2019) 32:2628–32. doi: 10.1080/14767058.2018.1443066
83. Sezer SD, Küçük M, Döger FK, Yüksel H, Odabaşı AR, Türkmen MK, et al. VEGF, PIGF and HIF-1α in placentas of early- and late-onset pre-eclamptic patients. Gynecol Endocrinol (2013) 29:797–800. doi: 10.3109/09513590.2013.801437
84. Kaufmann P, Black S, Huppertz B. Endovascular trophoblast invasion: Implications for the pathogenesis of intrauterine growth retardation and preeclampsia. Biol Reprod (2003) 69:1–7. doi: 10.1095/biolreprod.102.014977
85. Gorbunova OL, Shirshev SV. Role of kisspeptin in regulation of reproductive and immune reactions. Biochem (2020) 85:839–53. doi: 10.1134/S0006297920080015
86. Borst SE. The role of TNF-α in insulin resistance. Endocrine (2004) 23:177–82. doi: 10.1385/ENDO:23:2-3:177
87. Thaxton JE, Sharma S. Interleukin-10: A multi-faceted agent of pregnancy. Am J Reprod Immunol (2010) 63:482–91. doi: 10.1111/j.1600-0897.2010.00810.x
88. Siwetz M, Blaschitz A, El-Heliebi A, Hiden U, Desoye G, Huppertz B, et al. TNF-α alters the inflammatory secretion profile of human first trimester placenta. Lab Investig (2016) 96:428–38. doi: 10.1038/labinvest.2015.159
89. Soares MJ, Chakraborty D, Kubota K, Renaud SJ, Karim Rumi MA, Rumi MAK. Adaptive mechanisms controlling uterine spiral artery remodeling during the establishment of pregnancy. Int J Dev Biol (2014) 58:247–59. doi: 10.1387/ijdb.140083ms
90. Fukushima K, Murata M, Tsukimori K, Eisuke K, Wake N. 8-Hydroxy-2-deoxyguanosine staining in placenta is associated with maternal serum uric acid levels and gestational age at diagnosis in pre-eclampsia. Am J Hypertens (2011) 24:829–34. doi: 10.1038/ajh.2011.40
91. Kumar SN, Bastia B, Borgohain D, Agrawal U, Raisuddin S, Jain AK. Structural changes, increased hypoxia, and oxidative DNA damage in placenta due to maternal smokeless tobacco use. Birth Defects Res (2021) 113:1198–214. doi: 10.1002/bdr2.1941
92. Walter P, Ron D. The unfolded protein response: From stress pathway to homeostatic regulation. Science (2011) 334:1081–6. doi: 10.1126/science.1209038
93. wa Yung H, Alnæs-Katjavivi P, Jones CJPP, El-Bacha T, Golic M, Staff A-CC, et al. Placental endoplasmic reticulum stress in gestational diabetes: the potential for therapeutic intervention with chemical chaperones and antioxidants. Diabetologia (2016) 59:2240–50. doi: 10.1007/s00125-016-4040-2
Keywords: kisspeptin, trophoblast, thyroid, cell stress, inflammation, fetal development, rat
Citation: Santos BR, dos Anjos Cordeiro JM, Santos LC, Barbosa EM, Mendonça LD, Santos EO, de Macedo IO, de Lavor MSL, Szawka RE, Serakides R and Silva JF (2022) Kisspeptin treatment improves fetal-placental development and blocks placental oxidative damage caused by maternal hypothyroidism in an experimental rat model. Front. Endocrinol. 13:908240. doi: 10.3389/fendo.2022.908240
Received: 30 March 2022; Accepted: 04 July 2022;
Published: 28 July 2022.
Edited by:
Reinaldo Marín, Instituto Venezolano de Investigaciones Científicas (IVIC), VenezuelaReviewed by:
Jorge Lopez-Tello, University of Cambridge, United KingdomCopyright © 2022 Santos, dos Anjos Cordeiro, Santos, Barbosa, Mendonça, Santos, de Macedo, de Lavor, Szawka, Serakides and Silva. This is an open-access article distributed under the terms of the Creative Commons Attribution License (CC BY). The use, distribution or reproduction in other forums is permitted, provided the original author(s) and the copyright owner(s) are credited and that the original publication in this journal is cited, in accordance with accepted academic practice. No use, distribution or reproduction is permitted which does not comply with these terms.
*Correspondence: Juneo Freitas Silva, amZzaWx2YUB1ZXNjLmJy
Disclaimer: All claims expressed in this article are solely those of the authors and do not necessarily represent those of their affiliated organizations, or those of the publisher, the editors and the reviewers. Any product that may be evaluated in this article or claim that may be made by its manufacturer is not guaranteed or endorsed by the publisher.
Research integrity at Frontiers
Learn more about the work of our research integrity team to safeguard the quality of each article we publish.