- 1Department of Endocrinology, National Health Commission (NHC) Key Laboratory of Endocrinology, Chinese Academy of Medical Sciences, Peking Union Medical College Hospital, Peking Union Medical College, Beijing, China
- 2Department of Medical Research Center, Chinese Academy of Medical Sciences, Peking Union Medical College Hospital, Peking Union Medical College, Beijing, China
The current research and existing facts indicate that type 2 diabetes mellitus (T2DM) is characterized by gut microbiota dysbiosis and disturbed microbial metabolites. Oral glucose-lowering drugs are reported with pleiotropic beneficial effects, including not only a decrease in glucose level but also weight loss, antihypertension, anti-inflammation, and cardiovascular protection, but the underlying mechanisms are still not clear. Evidence can be found showing that oral glucose-lowering drugs might modify the gut microbiome and thereby alter gastrointestinal metabolites to improve host health. Although the connections among gut microbial communities, microbial metabolites, and T2DM are complex, figuring out how antidiabetic agents shape the gut microbiome is vital for optimizing the treatment, meaningful for the instruction for probiotic therapy and gut microbiota transplantation in T2DM. In this review, we focused on the literatures in gut microbiota and its metabolite profile alterations beneficial from oral antidiabetic drugs, trying to provide implications for future study in the developing field of these drugs, such as combination therapies, pre- and probiotics intervention in T2DM, and subjects with pregestational diabetes and gestational diabetes mellitus.
Introduction
The International Diabetes Federation Diabetes Atlas 10th edition shows a continued global increase in diabetes prevalence, estimating that 537 million adults are living with diabetes worldwide, most of which is type 2 diabetes mellitus (T2DM) (1). T2DM is a metabolic disorder with multiple pathogenic factors, including genetic elements, sedentary behaviors, and overeating (2). Once without effective treatment, it might lead to a composite of microvascular or macrovascular complications, for instance chronic kidney disease, diabetic eye disease, and cardiovascular disease (CVD) (3). Differing from insulin-dependent type 1 diabetes mellitus, T2DM is closely interrelated with insulin resistance (IR) and strongly intertwined with obesity, non-alcoholic fatty liver disease, and metabolic syndrome (4). Nowadays, more than 10 types of medicines are approved by the USA Food and Drug Administration for the glycemic treatment (5). Thousands of clinical trials and basic research are proceeding worldwide for diabetes pharmacotherapy, including looking for potential intervention targets (6). In addition to the reduction in HbA1c, results from a vast number of clinical and experimental studies have shown the potential effects of glucose-lowering drugs, such as weight reduction, cardiovascular safety, and lipid-lowering and antihypertensive effects; however, the mechanisms behind these benefits need to be further revealed (5, 6).
Gut microbiota has become a hot topic in metabolic disorders in the past decade, including T2DM (7–9). Accumulating evidence confirms that gut microbiota has emerged as a large complex ecological community and a vital regulator of host physical condition, via microbial metabolites and host interactions (10, 11). Among 100 trillion of microorganisms, which is 10 times the number of human body cells, including bacteria, fungi, viruses, and protozoa, the bacterial component is characterized as the most well-investigated group (11, 12) and will be the chief spotlight of this review. There are nearly 500–1,000 species of bacteria within the gastrointestinal tract and more than 90% of the total community are Firmicutes and Bacteroidetes at the phylum level, followed by Proteobacteria, Actinobacteria, Verrucomicrobiota, Fusobacteria, Cyanobacteria, and Tenericutes (11, 12). The gut microbiota homeostasis is preserved with control of pathogenic microbe growth and protection of beneficial microbes (11, 13). The gut microbiome is considered as a modifiable “new organ” that plays a crucial role in shaping the metabolic and immunological functions of T2DM (14). Although with wide interindividual variation, once the gut microbiota composition was destroyed, an imbalanced gut microbiome community leads to an abnormal production of metabolites, lipid and carbohydrate metabolism disturbance, IR, oxidative stress, and low-grade chronic inflammatory state in T2DM (7, 8, 15–18).
Therefore, understanding how antidiabetes agents influence the gut microbiome might be of importance for optimizing T2DM treatment. Microbiota and host metabolism might deliver promising and novel constructive aspects of commonly used oral antidiabetic drugs (19). In addition, fecal microbiota transplantation (FMT) has become a promising strategy for patients with T2DM (20, 21). In this review, we focus on the literatures in gut microbiota and metabolite profile alterations beneficial from oral antidiabetic drugs in diabetes and metabolic disorder state, in both basic research and clinical studies. We aim to figure out the similarities and differences in the literatures of gut microbiota and the metabolite-related effect of oral antidiabetic drugs, in order to deliver some leads for future studies in these developing fields of these drugs and T2DM treatment.
Gut Microbiota and Metabolites Altered in T2DM
Although the definite microbial signatures linked to T2DM have not been discovered yet, a large number of studies have found that gut microbiota dysbiosis in T2DM is highly associated with specific intestinal microbial taxa or certain enrichment of gene functional pathways (22–28). In a metagenome-wide association study from 345 Chinese individuals, T2DM-related gut flora dysbiosis was characterized by a decreased abundance in a cluster of butyrate-producing bacteria, such as Roseburia intestinalis, Faecalibacterium prausnitzii, Clostridiales sp. SS3/4, and Eubacterium rectale, and an increased abundance of opportunistic pathogens, such as Bacteroides caccae, Escherichia coli, and some Clostridium species (Clostridium symbiosum, Clostridium bolteae, Clostridium hathewayi, and Clostridium ramosum) (22). Another large-scale metagenome analysis study which recruited a population of 145 70-year-old European women with metagenomic profiles showed increases in the abundance of four Lactobacillus species (including Lactobacillus gasseri), Streptococcus mutans, and Clostridium hathewayi and decreases in the abundance of five Clostridium species (including Clostridium beijerinckii, Clostridium botulinum), Roseburia_272, and Bacteroides intestinalis in the T2DM group (23). Due to the difference in genetic inheritance, diet, and lifestyle factors, the connections among gut microbial communities, microbial metabolites, and T2DM are intricate. Despite the obvious discrepancy in metagenomic clusters between these two populations, the similar microbial functions enriched in T2DM included an increased level in lipid or glucose metabolism-related membrane transport and oxidative stress resistance and a decreased level in metabolism of vitamins and cofactors, butyrate production, and cell motility (22, 23). To recognize the core gut microbial features of T2DM, a machine learning framework totally recruited more than 9,000 people revealed that a microbiome risk score including 14 microbial features was positively associated with risk of T2DM and the future glucose increment after adjustment for traditional risk factors (such as age, sex, parental history of diabetes, body mass index, systolic blood pressure, and triglycerides) (28). In the meantime, a downward trend of butyrate-producing genus (Roseburia spp.) and a rising trend of chronic inflammation-associated genus (f:lactobacillaceae) were confirmed in this interpretable machine learning framework (28). Among a substantial body of experimental and clinical research, the genera of Bifidobacterium, Akkermansia, Bacteroides, Roseburia, and Faecalibacterium were inversely correlated with T2DM, while the genera of Ruminococcus, Blautia, Lactobacillus, and Fusobacterium were positively correlated with T2DM (8, 22, 23).
Although the underlying mechanism between complex gut microbiota and T2DM is still unclear, evidence has shown that a variety of metabolites derived from gut flora, including short-chain fatty acids (SCFAs), glycolipid lipopolysaccharides (LPS), bile acids (BAs), trimethylamine-N-oxide (TMAO), indole derivatives, amino acids, vitamins, and one-carbon metabolites, interacted with the host as signaling molecules and were further involved in the pathophysiological process of metabolic diseases (29–40) (Figure 1). SCFAs (including butyrate, acetate, and propionate) are the major microbial metabolites produced by dietary fiber fermentation within the intestinal lumen (41). SCFAs were found reduced in T2DM in both clinical and experimental research (42–45). By activation of specific G protein-coupled receptor 41 and 43 (GPR41 and GPR43), SCFAs could stimulate the secretion of peptide tyrosine-tyrosine (PYY) and glucagon-like peptide-1 (GLP-1) from intestinal enteroendocrine L cells (39, 46). PPY is an important neuroendocrine hormone, regulating food intake and energy balance; reduced secretion of GLP-1 in T2DM leads to a reduction of insulin and thus impaired glucose and energy metabolism (47). Besides, SCFAs have been identified as vital mediators in maintaining intestinal immunity and systemic inflammation through upregulating anti-inflammatory regulatory T cells, inhibition of histone deacetylase, and further inhibition of inflammatory signaling pathways and proinflammatory cytokines, such as nuclear factor-kappaB (NF-κb) and tumor necrosis factor alpha (TNF-α) (37, 48).
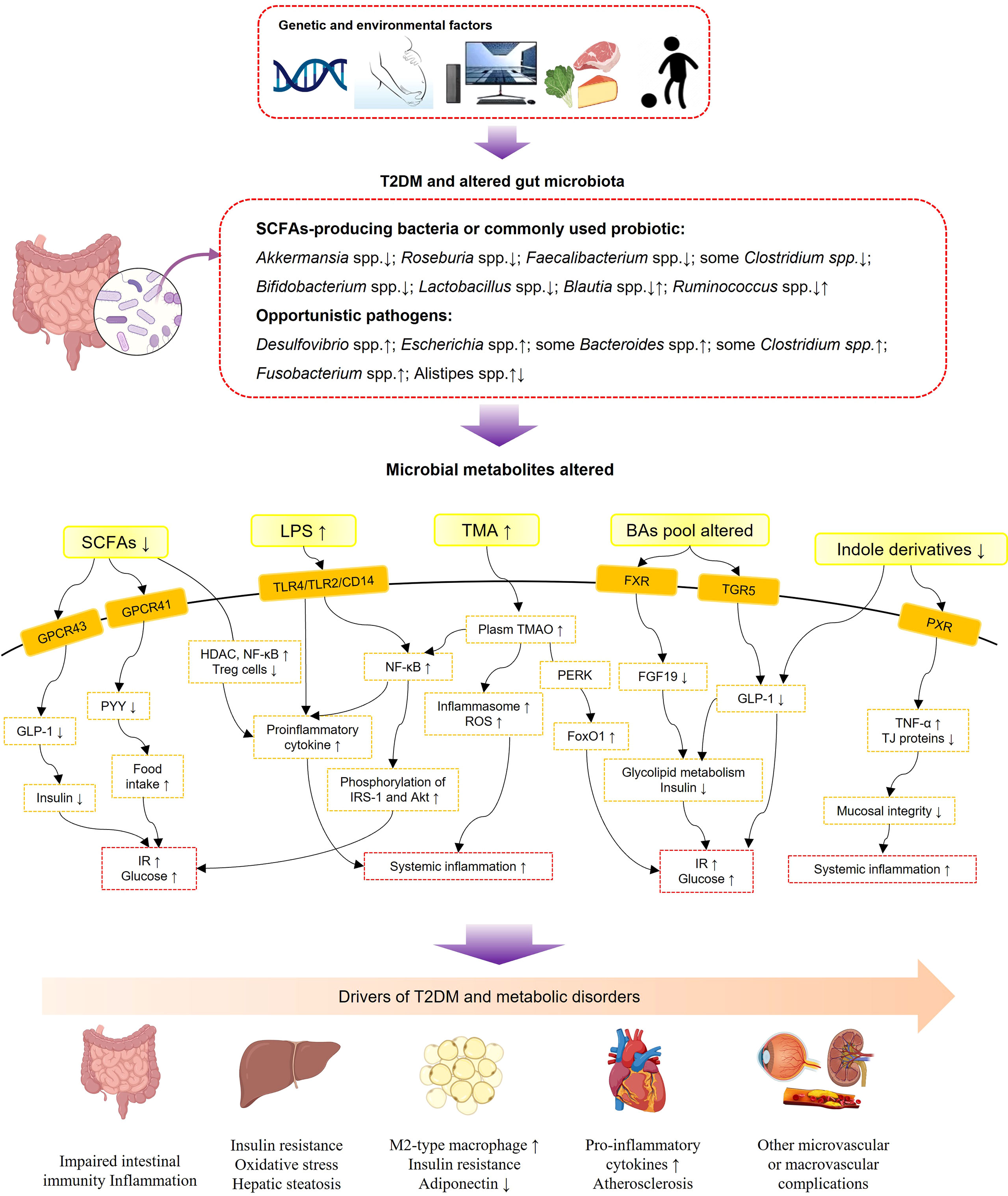
Figure 1 Schematic view of gut microbiota, microbial metabolites, and T2DM-associated metabolic disorders. SCFAs, short-chain fatty acids; LPS, lipopolysaccharides; TMA, trimethylamine; TMAO, trimethylamine-N-oxide; BAs, bile acids; GPCR43, G-protein-coupled receptor 43; GPCR41, G-protein-coupled receptor 41; TLR4, toll-like receptor 4; TLR2, toll-like receptor 4; CD14, cluster of differentiation 14; FXR, farnesoid X receptor; TGR5, Takeda G protein-coupled receptor 5; PXR, pregnane X receptor; GLP-1, glucagon-likepeptide-1; PYY, peptide tyrosine-tyrosine; HDAC, histone deacetylases; NF-κB, nuclear factor-kappaB; IRS-1, insulin receptor substrate-1; ROS, reactive oxygen species; PERK, protein kinase-like ER kinase; FoxO1, forkhead box-O1; FGF19, fibroblast growth factor 19; TNF-α, tumor necrosis factor alpha; TJ proteins, tight-junction proteins; IR, insulin resistance.
LPSs, the main compounds of gram-negative bacterial membranes, are known as potent stimulators of inflammation (49). Evidence shows that T2DM subjects possess a high enrichment of gram-negative bacteria, particularly those belonging to Proteobacteria at the phylum level (50). Notably, the Bacteroidetes phylum also belongs to a large part of gram-negative bacteria, but a decreased abundance of Bacteroidetes was found in obesity and diabetes conditions (24, 51–54). This contradiction might be explained by the fact that the LPS produced by the Bacteroidetes phylum has a lower endotoxic activity than other gram-negative bacteria such as the Proteobacteria phylum (55). Subsequently, a high concentration of LPS produced within the gut (metabolic endotoxemia) might lead to chronic low-grade inflammation in diabetic subjects through upregulating inflammatory signaling pathways and proinflammatory cytokine secretion (56, 57). LPSs produced by gut bacteria might damage the intestinal barrier leading to a “leaky gut” syndrome, for instance, a weakened tight junction and reduced gut secretory immunoglobulin A (58). Besides, LPSs have been confirmed to result in IR due to increased IRS-1 and Akt phosphorylation (59) (Figure 1).
Originally synthesized from cholesterol in the liver, BAs were revealed to have a reciprocal interaction with gut microbiota via the gut-to-liver axis (40). Primary BAs are converted into secondary BAs by gut microbiota (40). BAs are important signaling mediators regulating energy metabolism and systematic inflammation via the nuclear farnesoid X receptor (FXR) and Takeda G protein-coupled receptor 5 (TGR5) (40). In subjects with diabetes and metabolic diseases, BAs’ pool composition altered (60). The altered proportion of FXR antagonistic BAs leads to an altered expression of fibroblast growth factor 19 (FGF19), which were both vital molecules for BAs and glycolipid metabolism (60). Activation of TGR5 by secondary BAs stimulates GLP-1 secretion from L cells to increase insulin secretion and glucose tolerance (61). Evidence shows that modifications of the BA pool presented a beneficial effect in bariatric surgery and antidiabetic treatment (62–64).
TMAO is predominantly generated from dietary choline, which is transformed to trimethylamine in the gut and then oxidized in the liver (31). Elevated plasma concentrations of TMAO were reported positively related with metabolic dysfunction, such as insulin resistance, CVDs, and T2DM (31, 34, 65), and various bacteria (such as Clostridium hathewayi, Escherichia fergusonii, Providencia alcalifaciens, and Providencia rustigianii) have been recognized as contributing to the production of TMAO (66). TMAO was found to play a proinflammatory role by activating the nucleotide-binding oligomerization domain-like receptor family pyrin domain-containing 3 inflammasome, accelerating reactive oxygen species generation and various proinflammatory cytokines (67). In addition, evidence in experimental research shows that TMAO promoted metabolic dysfunction by directly binding and activating protein kinase-like ER kinase, a key sensor of intracellular stress, and then enhanced transcription activity of forkhead box-O1 in the liver (31).
Indole derivatives are produced from tryptophan by the gut microflora (33). In the recent years, indole derivatives have exhibited anti-inflammatory and antidiabetic effects (68). Evidence shows that indole derivatives were able to stimulate the secretion of GLP-1 from L cells (32). Various indole derivatives have been synthesized to investigate their bioactivities and biological functions (68). Microbe-specific indoles, such as indole 3-propionic acid, were found to regulate mucosal integrity through activating the xenobiotic sensor, pregnane X receptor, to downregulate enterocyte TNF-α expression and upregulate junctional protein expression (36). In addition to the abovementioned metabolites, vitamins and cofactors produced by probiotics, such as Bifidobacterium and Lactobacillus, yield greater health benefits on patients with T2DM and metabolic diseases (69). Amino acids synthesized by the gastrointestinal microbiota were also vital factors to energy metabolism and glucose homeostasis (70). For instance, Prevotella copri and Bacteroides vulgatus were discovered as the main species mediating the association between biosynthesis of branched-chain amino acids (BCAAs) and IR, and Prevotella copri could induce IR, aggravate glucose intolerance, and increase circulating BCAAs levels (70).
Overall, a vast body of human studies and plentiful animal studies have suggested that T2DM was characterized by gut microbiota dysbiosis and alterations of gut microbiota-derived metabolites, which are important contributors to the pathological injury of T2DM.
The Effects of Oral Antidiabetic Drugs on Gut Microbiota and Microbial Metabolites
Metformin
Metformin can alleviate patients’ hyperglycemia mainly by significant suppression of glucose production in the liver (71). Activation of the master cellular energy sensor AMP-activated protein kinase (AMPK) is well documented in the mechanism of metformin but may not interpret for its complex beneficial effects (72–75). In fact, metformin was found to modify the intestinal flora community in T2DM in a vast body of clinical research and experimental animal studies (76–80) (summarized in Table 1).
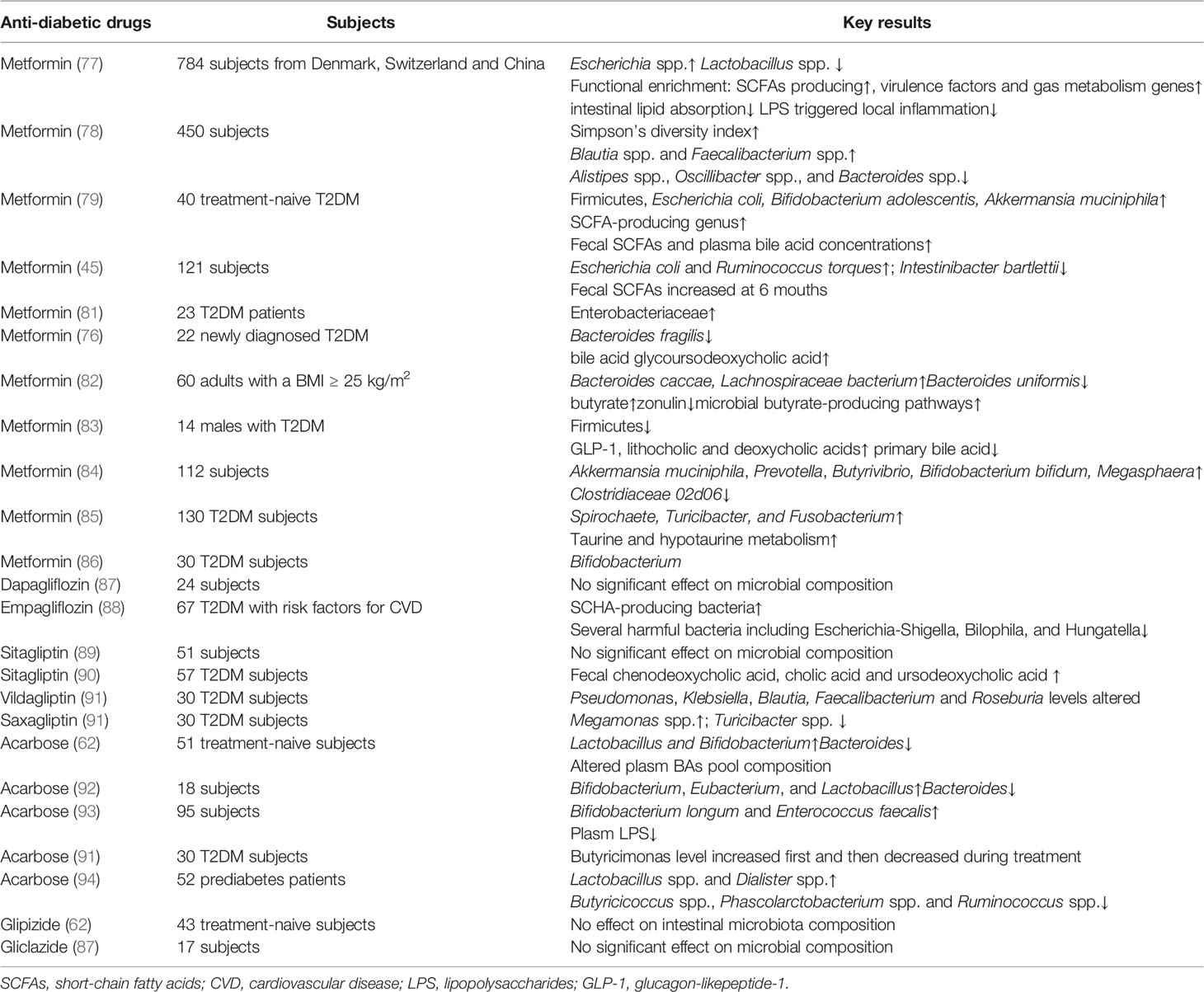
Table 1 Clinical research exploring the effects of oral anti-diabetic drugs on gut microbiota in T2DM.
Metagenomics combined with targeted metabolomic data in a randomized, placebo-controlled, double-blind study showed that metformin strongly altered the gut microbiome and its function in individuals with treatment-naive T2DM (79). Subsequently, the authors transplanted fecal samples from three donors (treatment-naive condition compared with 4-month metformin-treated condition) into germ-free mice and observed that glucose tolerance was improved in mice that received 4-month metformin-treated fecal samples, indicating a direct beneficial effect on glucose homeostasis (79). This effect might be mediated by increased SCFA-producing bacteria and the abundance of Akkermansia muciniphila, enriched pathways of the metabolism of vitamins and cofactors, and metalloproteins or metal transporters (79). In line with this research, a large study aimed at disentangling metformin treatment signatures in T2DM recruited 784 subjects from Denmark, Switzerland, and China and illustrated that metformin treatment significantly increased the abundance of Escherichia spp. and reduced that of Intestinibacter spp. The functional enrichment analyses demonstrated that SCFA-producing pathways and enrichment of virulence factors and gas metabolism genes were significantly enhanced, while intestinal lipid absorption and LPS-triggered intestinal inflammation were reduced (77). A randomized clinical trial which recruited 450 T2DM subjects uncovered that metformin altered the gut microbiota composition, increased the beneficial bacteria, such as Blautia and Faecalibacterium, and inhibited potential pathogen-like microbiota, for example, Oscillibacter, Alistipes, and Bacteroides (78). As summarized in Table 1, most clinical studies revealed that microbes mediated the therapeutic effects of metformin chiefly through improvement in SCFA production, BA pool composition alteration, or reduction in LPS production.
In addition to clinical studies on T2DM patients, a clinical trial which recruited 20 healthy Korean participants found that metformin treatment altered the abundances of Clostridium, Escherichia, Intestinibacter, and Romboutsia, and the relative abundances of metabolites changed including carbohydrate, fatty acid, and amino acid metabolism (95). In experimental animal models, treatment with metformin was revealed to increase SCFA production, to reduce circulation LPS, to inhibit intestinal proinflammatory signaling activities, which was in line with clinical studies (80, 96, 97) (Figure 2). The activation of SCFA receptors, GPR41 and GPR43, stimulated the secretion of PYY and GLP-1, inhibiting appetite and improving insulin secretion. At the same time, increased-circulation SCFAs are responsible for improving energy metabolism, suppressing fat accumulation and insulin signaling in adipose tissue, and regulating the intestinal immunity and systemic inflammation (38, 39, 98). Accompanied by decreased LPS produced in the gut, metformin intervention increased goblet cell mass, mucin production, and tight-junction (ZO1 and occludin) proteins in obese gut, thereby relieving intestinal inflammation, decreasing leaky gut, and repairing the intestinal barrier structure (80, 96). In addition, the metabolic benefits of metformin might also be mediated by gut microbiota and bile acid homeostasis (76). Evidence shows that Bacteroides fragilis was decreased in samples from newly diagnosed T2DM patients after metformin treatment for 3 days, meanwhile the BA pool was altered (76). Bile acid glycoursodeoxycholic acid was increased, accompanied by inhibition of intestinal FXR signaling and decreased serum FGF19 levels (76). Reduced circulating FGF19 was found in subjects with metabolic disorders and hepatic steatosis, and FGF19 analogues have been identified as promising therapeutic methods in metabolic improvement (60). However, research associated with FGF19 was inconsistent, and the underlying mechanism still needs further research. Among the numerous gut flora altered during the metformin treatment in both clinical and experimental studies, Akkermansia muciniphila, a mucin-degrading bacterium, is related to healthy intestinal mucosa (79, 84, 96, 99). Furthermore, oral administration of Akkermansia muciniphila to high-fat diet-induced mice without metformin treatment significantly improved glucose homeostasis and reduced visceral adipose tissue inflammation by inducing Tregs, indicating the promising treatment value of Akkermansia spp. for T2DM (99).
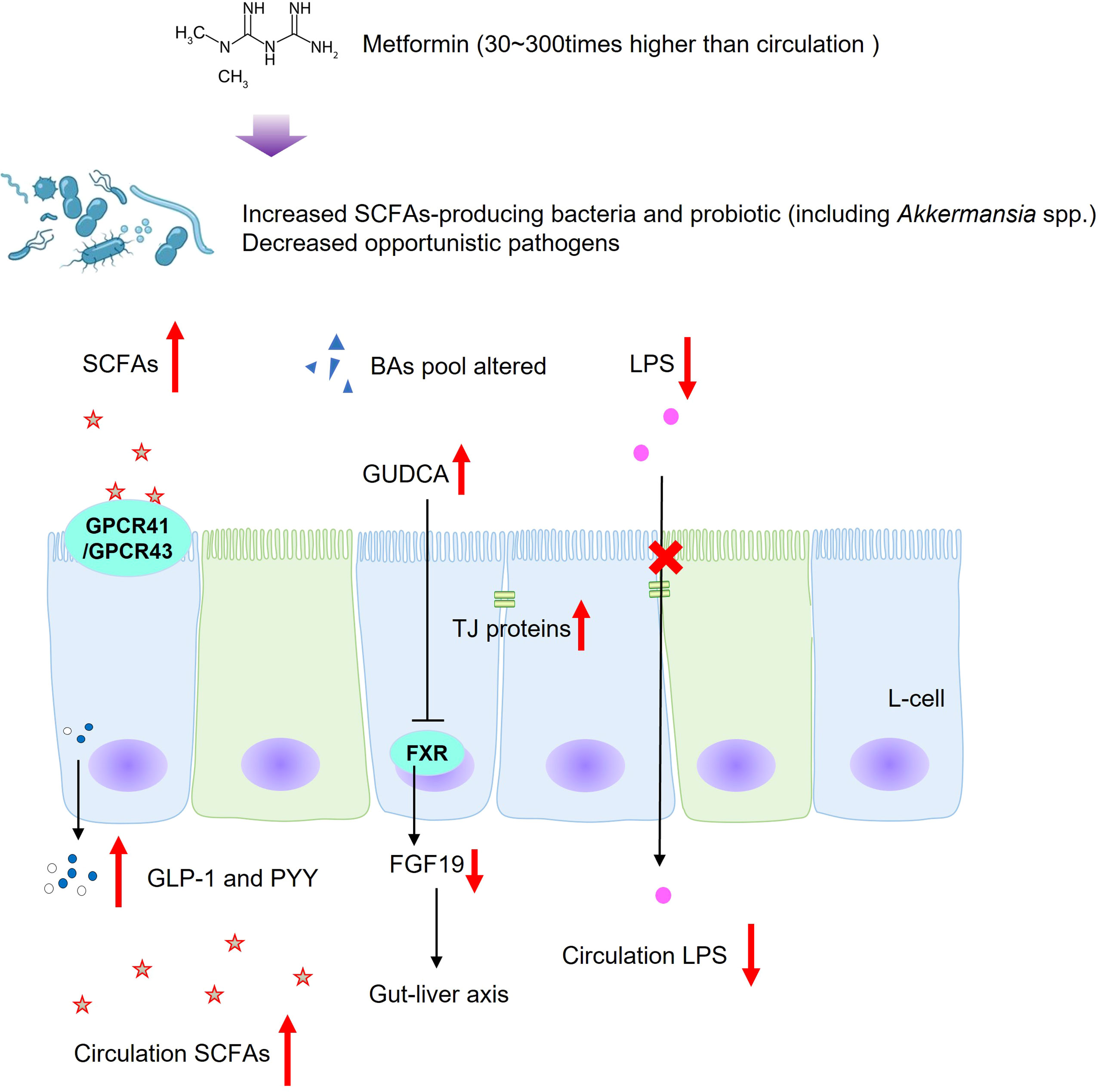
Figure 2 Possible regulatory mechanisms of metformin on gut microbiota and microbial metabolites in T2DM. SCFAs, short-chain fatty acids; BAs, bile acids; LPS, lipopolysaccharides; GUDCA, glycoursodeoxycholic acid; GPCR43, G-protein-coupled receptor 43; GPCR41, G-protein-coupled receptor 41; FXR, farnesoid X receptor; TGR5, Takeda G protein-coupled receptor 5; GLP-1, glucagon-likepeptide-1; PYY, peptide; tyrosine-tyrosine; FGF19, fibroblast growth factor 19; TJ proteins, tight-junction proteins.
In brief, in addition to activation of the master cellular energy sensor AMPK (74), metformin might act partly through gut microbiota and its metabolites to improve metabolic health. Notably, the metformin concentration in the gastrointestinal lumen is 30–300 times higher than in the circulation (100). High concentrations of metformin in the gastrointestinal lumen can increase glucose uptake and inhibit mitochondrial oxidative phosphorylation in enterocytes then accelerate glucose utilization through glycolysis and overproduction of lactate, the reason why metformin might contribute to gastrointestinal intolerance in a minority of people (71, 101, 102). Previous studies also hint that overproduction of lactate might also be microbially mediated (71, 103). Therefore, the potential mechanisms and contradiction of gastrointestinal intolerance and gut microbiota-related benefits need further investigation.
SGLT2 Inhibitors
Sodium-glucose cotransporter 2 (SGLT2) inhibitors improve glycemic control by increasing renal glucose excretion, accompanied by pleiotropic non-glycemic properties, such as reductions in body weight and cardiovascular and renal protection effects (104–107). However, the underlying mechanism of the pleiotropic benefits was still not clear. Evidence shows that the protective effect might be explained for increased ketone body production in CVD, a clear fuel to improve the cardiac function of the energy-starved myocardium (108). As an orally ingested antidiabetic agent, experimental animal studies have found that SGLT2 inhibitor intervention slightly altered the microbiota composition in experimental animal studies (109–111) (summarized in Table 2).
Dapagliflozin treatment showed minor beneficial alterations of gut microbiota in T2DM mice, a trend for decreased Oscillospira spp. and Firmicutes/Bacteroidetes ratios and increased Akkermansia muciniphila in the treatment group (109). In the butyrate-supplemented diet-fed db/db mice, the dapagliflozin-treated mice were also characterized by a decreased trend in Firmicutes/Bacteroidetes ratios, as well as a decreased trend in Adlercreutzia spp. and Alistipes spp. and an increased trend in Streptococcus spp (111).. In addition to slight alterations in gut microbiota, SGLT2 inhibitor intervention significantly improved intestinal SCFA production in animal models (110, 113). However, the results were inconsistent, and dapagliflozin treatment was found to have no beneficial effects on gut bacteria in diabetic rats (112). Only two clinical studies explored the alteration of fecal microbiome with SGLT2 inhibitor treatment (87, 88). Seventy-six treatment-naive T2DM with risk factors for CVD were included in a randomized, open-label, two-arm clinical trial (88). After a 3-month intervention, empagliflozin improved glucose metabolism and reduced CVD-related risks, while it significantly altered the gut microbiota, including an increase in SCFA-producing bacteria and a reduction in several harmful bacteria such as Escherichia–Shigella, Bilophila, and Hungatella (88). However, another clinical study found no significant effect on microbial alpha diversity or composition (87). It might be due to the fact that all of the subjects included had already been treated with metformin, which might have overshadowed the possible impact of dapagliflozin on the gut microbiome (87). Experimental studies found that dapagliflozin increased the abundance of Desulfovibrionaceae, which was increased in the fecal microbiota of animal models with metabolic disorders (114, 115), while metformin reduced Desulfovibrionaceae, suggesting that the combination drug therapy of dapagliflozin and metformin might have complementary actions on the gut microbiota in diabetes (112). Given all this, the pleiotropic beneficial effects of the SGLT2 inhibitor might be slightly mediated by gut microbiota or not be mediated by gut microbiota, and the potential mechanism of the pleiotropic beneficial effects of SGLT2 inhibitors need to be further uncovered (116).
Thiazolidinedione Insulin Sensitizers
Thiazolidinedione (TZD) drugs are effective oral agents for T2DM in improving insulin sensitivity (117). TZDs are ligands of peroxisome proliferator-activated receptor gamma (PPAR-γ), leading to the activation of various pathways related to glycemic homeostasis and lipid metabolism (117, 118). The expression of PPAR-γ is abundant in the intestinal tract; thus, it is possible that PPAR-γ agonists straightly impact on gut microbiome homeostasis to improve energy metabolism (119, 120). However, only a few experimental animal studies explored whether TZD treatment can modify gut microbiota homeostasis (119, 121, 122). In a high-fructose-fed mouse model, pioglitazone partly altered gut microbiota and relieved the intestinal inflammation and epithelial barrier impairment, such as preventing the increment of the pathogenic bacteria Deferribacteraceae (Mucispirillum) (121). In diabetic mice, treatment with rosiglitazone promoted insulin sensitivity without modifying the composition of gut flora but improved the gene expression related to lipid and carbohydrate metabolism as well as immune regulation in the ileum and colon (119). Another experimental study discovered that microbial metabolites, for example, hippurate and indole-3-ethanol, were decreased by pioglitazone intervention in iNOS knockout mice (122). These experiment research suggested that TZDs might have mild protective effects on gut microbiota, mainly focused on lipid and carbohydrate metabolism and inflammation. However, no clinical study focused on gut microbiota and microbial metabolites alterations with TZDs treatment in T2DM subjects; further research is still needed.
Dipeptidyl Peptidase-4 Inhibitors
Dipeptidyl peptidase-4 (DPP-4) inhibitors inhibit the degradation of glucagon-like peptide-1 (GLP-1) and glucose-dependent insulinotropic polypeptide to stimulate insulin secretion, reserve β-cell function, and maintain glucose homeostasis (123). A series of experimental studies have shown that DPP-4 inhibitors might be able to improve energy metabolism through shaping the gut microbial composition and increasing fecal SCFAs (124–127) (summarized in Table 3). In high-fat diet-induced obesity mice, DPP-4 inhibitors exerted an important impact on gut microbial composition and fecal metabolites, particularly the increased abundance of Bacteroidetes (124). Researchers then transplanted the fecal microbiota of DPP-4 inhibitor-treated patients to germ-free mice and observed an improved glucose intolerance (124). Compared with that in GLP-1 receptor agonist liraglutide-treated mice, the gut microbiota differed substantially in mice treated with DPP-4 inhibitors, indicating that the hypoglycemic mechanism of DPP-4 inhibitors on gut microbiota is at least not primarily by GLP-1 and the other potential benefit of DPP-4 inhibitors needs further research (124, 128). In addition to increment of SCFA-producing flora, DPP-4 inhibitors were found to reduce Toll-like receptor ligands and improve the production of antimicrobial peptides, exerting immunomodulatory and anti-inflammatory effects and maintaining intestinal homeostasis in obese mice, as well as cross talk with the liver and the whole-host health (126, 127). Some studies exhibited a decreased trend in the Firmicutes/Bacteroidetes ratio with treatment of DPP-4 inhibitors (124, 125, 127), while one experimental animal study found an enlarged abundance of Firmicutes and increased ratios of Firmicutes/Bacteroidetes (94). Although the relation between metabolic disorders and the Firmicutes/Bacteroidetes ratio is currently contradictory, more literatures considered it as a characteristic of obesity and T2DM (55).
There existed a few clinical studies that explored the gut flora modifying the effect of DPP-4 inhibitors (89–91). However, in a clinical study which included 51 T2DM patients, the advantageous effect of sitagliptin on glucose control, weight loss, and BA metabolism was not related to alterations in the gut microbiota (89, 90). No significant effect on microbial composition was found, which is possibly due to the fact that these subjects previously used metformin or sulphonylureas as hypoglycemic therapies, and it might have covered the possible effects of DPP-4 inhibitors (89, 124). Another clinical study which included 90 T2DM subjects found that both vildagliptin and saxagliptin altered the composition of gut microbiota, respectively (91). Thus, the microbiota-shaping effects of DPP-4 inhibitors in clinical studies and its additional hypoglycemic mechanism need further investigation.
α-Glucosidase Inhibitors
α-Glucosidase inhibitors are antidiabetic drugs, including acarbose, miglitol, and voglibose, which delay the absorption of carbohydrates in the intestinal tract to inhibit the rise in postprandial plasma glucose concentration (131). α-Glucosidase inhibitors are inhibitors of both human and bacterial α-glucosidases, and because of its high intestinal drug concentration, α-glucosidase usually has noticeable impacts on the intestinal flora (132, 133). Large amounts of research revealed that α-glucosidase inhibitors could shape the composition of the gut microbiome in both animal studies and clinical studies (62, 92–94, 134). Evidence shows that acarbose modulated the gut microbiota and corresponding shaped fecal and plasma BA composition, which may improve host energy metabolism (62, 135). A clinical study which recruited 51 treatment-naive T2DM patients showed that a three-mouth treatment with acarbose increased Lactobacillus and Bifidobacterium abundances and reduced Bacteroides abundances, along with altered plasm BA pool composition (62). Another clinical study which included 95 T2DM patients found that acarbose treatment improved the abundance of Enterococcus faecalis and Bifidobacterium longum, along with the reduction of plasma inflammatory factors, such as prothrombin activator inhibitor-1 and LPS levels (93). As summarized in Table 4, intervention with α-glucosidase inhibitors in experimental animal studies also confirmed significant impacts on gut microbiota and relevant metabolites. In addition to their glucose-lowering and energy metabolism-improving effects, α-glucosidase inhibitors were found to reverse joint inflammation on collagen-induced arthritis mice and the underlying mechanism might be due to the alteration of host–commensal interactions, which have been confirmed to be correlated with rheumatoid arthritis, such as several butyrate-producing species, Lactobacillus spp. and Oscillospira spp (48, 138, 141).. These results suggested a promising prospective of α-glucosidase inhibitors due to its potential antiarthritis effect mediated by the gut microbiome (134, 138).
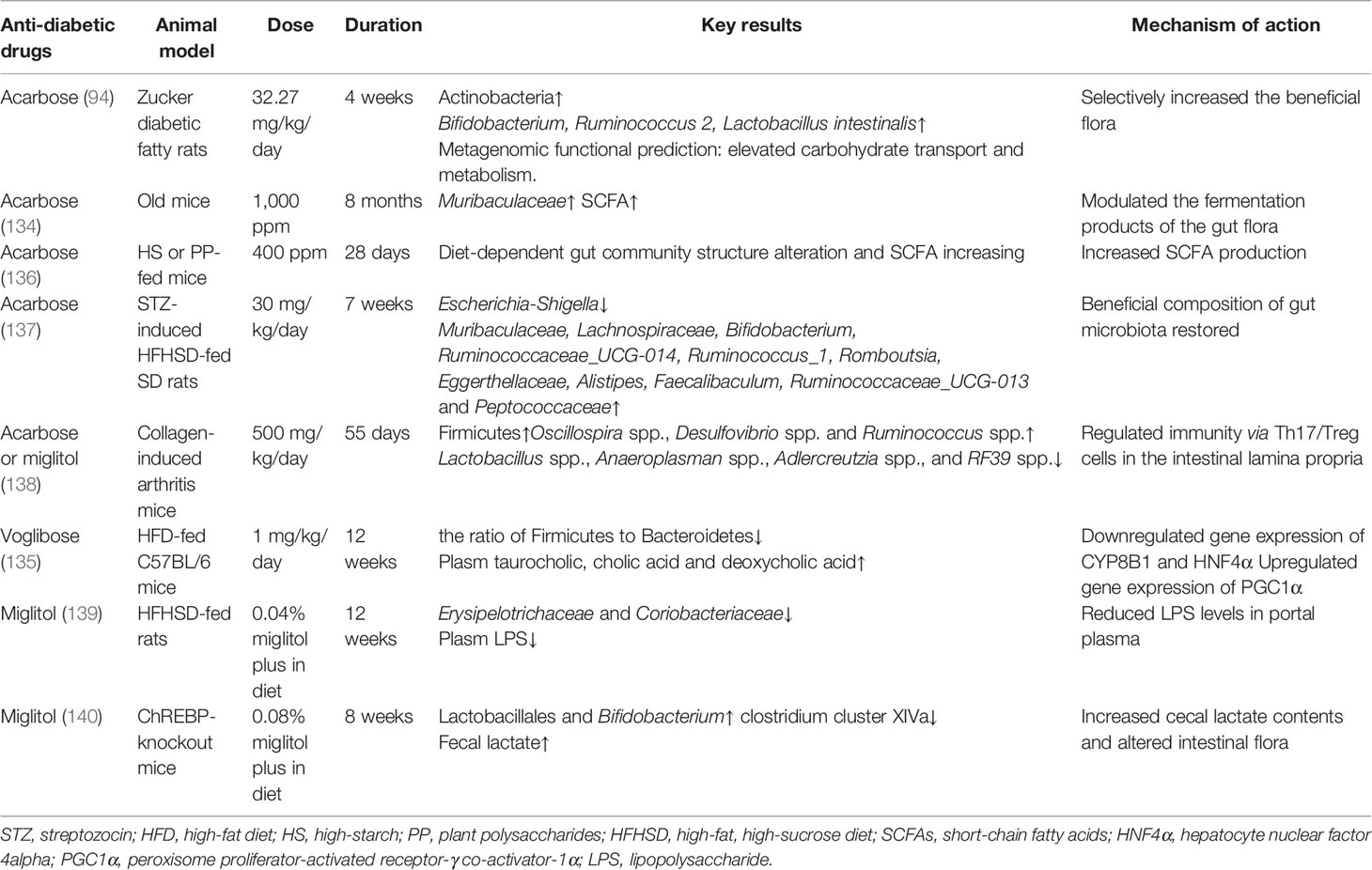
Table 4 Experimental animal studies analyzing the effects of α-glucosidase inhibitors on gut microbiota.
In fact, over 95% of the acarbose dose was not absorbed in the gut, coupled with its feature to inhibit microbial α-glucosidases, and subjects’ treatment response to acarbose is dependent on several factors, such as dietary intake, genetic factor, and microbiota composition before treatment (also named enterotypes) (62, 91–93, 136, 142, 143). The acarbose-shaped gut microbial composition might be related to the dietary intake in a small Japanese population with T2DM (92). Moreover, hierarchical clustering showed that the habitual dietary intake of sucrose, fat, and carbohydrate was associated with three distinct microbial clusters, and even the abundance alteration of Faecalibacterium was positively related to dietary rice intake but negatively related to bread intake (92). A previous study also found that patients with a gut flora driven by Bacteroides displayed more beneficial modifications in gut microbiota, plasma BA composition, and more metabolic metabolism enhancement after acarbose treatment than those with Prevotella (62). In addition, researchers revealed that acarbose resistance has spread in certain host gut microbiomes, which contributed an emerging layer to the multifaceted network of carbohydrate-mediated cross talk among various human microbiomes (132, 144). Besides, in antibiotic pretreatment mice, whose gut microbial enzyme activities have been weakened, the metabolism of voglibose was reduced and more significantly glucose-lowering effects were presented (143). In brief, from currently clinical and experimental studies, α-glucosidase inhibitors have obvious effects on gut microbiota and its effects significantly depend on host diet and the original composition of the gut microbiome.
Other Oral Glucose-Lowering Agents
Other less researched oral antidiabetes medications, such as sulfonylurea and glinide insulin secretagogues, have been noticed to cross talk with probiotic bacteria or microbial metabolic profiles (145–147). Nevertheless, two clinical studies which were designed to assess the effects of sulfonylureas on gut microbiota in T2DM subjects found no beneficial impacts on gut microbiota composition even in treatment-naive subjects, but with enhanced glycemic control (62, 87). At the same time, acarbose showed beneficial effects on the composition of the gut microbiome, suggesting that the detected metabolic modifications of sulfonylureas might not be intermediated by their impacts on the gut microbiota (62, 87). Recently, a few newly invented oral anti-glucose agents were discovered and used in clinical application, such as chiglitazar and imeglimin (148, 149). Activating as a peroxisome proliferator-activated receptor pan-agonist for glucose control, chiglitazar was found to improve insulin sensitivity and lipid homeostasis and reduce circulating levels of inflammatory parameters (150, 151). Imeglimin was confirmed to have the effects of modulating mitochondrial bioenergetics, enhancing mitochondrial function, improving insulin sensitivity, and preserving β-cell function (152–154). However, the associations of these anti-glucose agents and gut microbiota composition were still lacking.
In addition, newly identified exciting targets, including glucokinase activators and G-protein-coupled receptor 40 agonists, have also been researched, although not clinically usable (155, 156). Therefore, with the development of novel glucose-lowering agents, further research is still needed to uncover the complex interaction among gut microbiota, glucose-lowering agents, and the microbial-host metabolic cross talk.
Conclusions and Future Prospects
Antidiabetic agents modify the gut flora and thereby alter gastrointestinal and plasma metabolite profiles, further improving metabolic health. Knowledge and studies so far indicate that oral antidiabetes drugs, including metformin, DPP-4 inhibitors, and α-glucosidase inhibitors, have obvious effects on gut microbiota and microbial metabolites, while SGLT2 inhibitors and TZDs have slighter effects (62, 77, 87, 89). Even if the definite microbial signatures linked to certain antidiabetic agents have not been discovered yet, understanding how antidiabetes drugs influence the gut microbiome might be vital for identifying their potential mechanisms and optimizing their treatment. Although different hypoglycemic drugs shape gut microbiota differently, they have been confirmed to have some similar effects in regulating microbiota and metabolites. Among various microbiota and metabolites derived from gut flora, metformin, SGLT2 inhibitors, DPP-4 inhibitors, and α-glucosidase inhibitors have been demonstrated to have similar effects on increased SCFA-producing bacteria and SCFA production, which may partly explain their beneficial effects in the regulation of insulin sensitivity enhancement, energy metabolism, and systemic inflammation (77, 78, 110, 124, 134). Notably, among various SCFA-producing bacteria, Akkermansia muciniphila has been proven increased particularly during the metformin treatment in both clinical and experimental studies, which also related to healthy intestinal mucosa and anti-inflammatory action (79, 84, 96, 99). In addition, alteration of the BA pool was commonly displayed in both metformin and α-glucosidase inhibitors, corresponding with decreased Bacteroides fragilis in metformin-treated individuals and increased Lactobacillus and Bifidobacterium abundances and reduced Bacteroides abundances in α-glucosidase inhibitor-treated individuals, respectively (62, 76). In addition, reduction of opportunistic pathogen and attenuated intestinal inflammation could be seen in intervention research on metformin, DPP-4 inhibitors, and α-glucosidase inhibitors (77, 126, 138). Therefore, manipulation of gut microflora composition could be a potential and promising target to improve metabolic outcomes in subjects with T2DM. The microbiota–host cross talk might convey novel and potential ideas of generally used oral glucose-lowering drugs.
Firstly, combination therapy might have additional benefits, due to the fact that different antidiabetes drugs shape gut microbiota with distinct effects (62, 94, 112), For example, dapagliflozin increased the abundance of Desulfovibrionaceae in a T2DM rat model, which is an unfriendly sulfate-reducing bacteria in the gut, while metformin reduced it on the contrary, revealing a rationality and complementary action of combined pharmacotherapy between dapagliflozin and metformin (112). However, the definite combination effects of metformin and SGLT2 inhibitors need further investigation. T2DM is a chronic disease with progressive features and possible complex complications; a satisfactory treatment effect is hard to achieve with monotherapy. Besides metformin and SGLT2 inhibitor combined treatment, combination therapies, such as metformin with pioglitazone or metformin with DPP-4 inhibitors, might exhibit a synergetic role in gut microbiome benefits (157, 158). Further investigations in both experimental and clinical are needed to figure out the combined pharmacotherapy effects on gut microbiota.
Secondly, pre- and probiotics could be a promising treatment for T2DM in the modulation of gut microbiota (159). For example, Actinoplanes spp. and Lactobacillus spp. have been definitively demonstrated to effectively inhibit the alpha-glucosidase activity to reduce glucose levels (160, 161). The combination of hypoglycemic agents and certain probiotics or prebiotics may further enhance the glucose-lowering effects (82, 162). Prebiotics, such as inulin and galacto-oligosaccharide, could be fermented by the gut flora, leading to modulation of intestinal microbiota and the production of various microbial metabolites including SCFAs (163–165). Besides, evidence shows that combination of metformin and gastrointestinal microbiome modulator (consisting of inulin, beta-glucan, and polyphenols) treatment significantly relieved metformin tolerance than the placebo combination (166). Notably, for patients with pregestational diabetes and gestational diabetes mellitus (GDM), the dominating pharmacotherapy is insulin, while only metformin and glyburide are used in some countries (167, 168). Other oral hypoglycemic agents are limited in these patients. Hyperglycemia during pregnancy is associated with significantly increased maternal and fetal metabolic disturbance and morbidity (167). Therefore, dietary modification and physical activity are particularly important for glycemia control (167). A systematic review and meta-analysis revealed that probiotic supplementation in GDM could significantly reduce homeostasis model assessment of the insulin resistance index with no adverse effects reported (169). Evidence shows that inulin-type fructan supplementary improved glucose and lipid metabolism in HFD-induced GDM mouse models associated with gut flora modification (170). Our research team found that maternal inulin treatment improved glucose metabolism in adult male offspring via regulation of the hepatic long non-coding RNA profile (164). However, results are inconsistent showing that probiotics, including Lactobacillus rhamnosus and Bifidobacterium animalis subspecies lactis, did not prevent GDM in overweight and obese pregnant women (171). Thus, more clinical studies are needed to verify these results and explore the ideal bacterial composition of pre- and probiotics that might positively alter glucose metabolism in GDM or pregestational diabetes.
Thirdly, FMT from normal glucose tolerance or antidiabetes treatment subjects to mice revealed a significant improvement in gut microbiota composition, glucose homeostasis, and metabolic health (17, 124, 172). Despite that this promising treatment was still in its infancy (173–175), FMT combined with antidiabetes drugs might bring novel interventions and perspectives in T2DM management. The effects and mechanisms underneath these potential treatment schedules are still unclear, and it is vital to further develop meaningful and applicable interventions combined with intestinal microbiota in the future study.
Author Contributions
DW: writing—original draft preparation. JL, LZ, QZ, ML, and XX: writing—review and editing. XX: supervision. XX and QZ: funding acquisition. All authors contributed to the article and approved the submitted version.
Funding
This work was supported by the grants from the National Natural Science Foundation of China (No. 82170854, 81870579, 81870545, 81570715, 81170736), Beijing Natural Science Foundation (7202163), Beijing Municipal Science & Technology Commission (Z201100005520011), CAMS Innovation Fund for Medical Sciences (CIFMS2021-1-I2M-002).
Conflict of Interest
The authors declare that the research was conducted in the absence of any commercial or financial relationships that could be construed as a potential conflict of interest.
Publisher’s Note
All claims expressed in this article are solely those of the authors and do not necessarily represent those of their affiliated organizations, or those of the publisher, the editors and the reviewers. Any product that may be evaluated in this article, or claim that may be made by its manufacturer, is not guaranteed or endorsed by the publisher.
References
1. Sun H, Saeedi P, Karuranga S, Pinkepank M, Ogurtsova K, Duncan BB, et al. IDF Diabetes Atlas: Global, Regional and Country-Level Diabetes Prevalence Estimates for 2021 and Projections for 2045. Diabetes Res Clin Pract (2022) 183:109119. doi: 10.1016/j.diabres.2021.109119
2. Stumvoll M, Goldstein BJ, van Haeften TW. Type 2 Diabetes: Principles of Pathogenesis and Therapy. Lancet (2005) 365(9467):1333–46. doi: 10.1016/s0140-6736(05)61032-x
3. Gregg EW, Sattar N, Ali MK. The Changing Face of Diabetes Complications. Lancet Diabetes Endocrinol (2016) 4(6):537–47. doi: 10.1016/s2213-8587(16)30010-9
4. Ferguson D, Finck BN. Emerging Therapeutic Approaches for the Treatment of NAFLD and Type 2 Diabetes Mellitus. Nat Rev Endocrinol (2021) 17(8):484–95. doi: 10.1038/s41574-021-00507-z
5. Draznin B, Aroda VR, Bakris G, Benson G, Brown FM, Freeman R, et al.9. Pharmacologic Approaches to Glycemic Treatment: Standards of Medical Care in Diabetes-2021. Diabetes Care (2021) 44(Suppl 1):S111–s124. doi: 10.2337/dc21-S009
6. Perreault L, Skyler JS, Rosenstock J. Novel Therapies With Precision Mechanisms for Type 2 Diabetes Mellitus. Nat Rev Endocrinol (2021) 17(6):364–77. doi: 10.1038/s41574-021-00489-y
7. Bordalo Tonucci L, Dos Santos KM, De Luces Fortes Ferreira CL, Ribeiro SM, De Oliveira LL, Martino HS. Gut Microbiota and Probiotics: Focus on Diabetes Mellitus. Crit Rev Food Sci Nutr (2017) 57(11):2296–309. doi: 10.1080/10408398.2014.934438
8. Gurung M, Li Z, You H, Rodrigues R, Jump DB, Morgun A, et al. Role of Gut Microbiota in Type 2 Diabetes Pathophysiology. EBioMedicine (2020) 51:102590. doi: 10.1016/j.ebiom.2019.11.051
9. Turnbaugh PJ, Ley RE, Hamady M, Fraser-Liggett CM, Knight R, Gordon JI. The Human Microbiome Project. Nature (2007) 449(7164):804–10. doi: 10.1038/nature06244
10. Canfora EE, Meex RCR, Venema K, Blaak EE. Gut Microbial Metabolites in Obesity, NAFLD and T2DM. Nat Rev Endocrinol (2019) 15(5):261–73. doi: 10.1038/s41574-019-0156-z
11. Lozupone CA, Stombaugh JI, Gordon JI, Jansson JK, Knight R. Diversity, Stability and Resilience of the Human Gut Microbiota. Nature (2012) 489(7415):220–30. doi: 10.1038/nature11550
12. Sommer F, Bäckhed F. The Gut Microbiota–Masters of Host Development and Physiology. Nat Rev Microbiol (2013) 11(4):227–38. doi: 10.1038/nrmicro2974
13. Jandhyala SM, Talukdar R, Subramanyam C, Vuyyuru H, Sasikala M, Nageshwar Reddy D. Role of the Normal Gut Microbiota. World J Gastroenterol (2015) 21(29):8787–803. doi: 10.3748/wjg.v21.i29.8787
14. Tilg H, Moschen AR. Microbiota and Diabetes: An Evolving Relationship. Gut (2014) 63(9):1513–21. doi: 10.1136/gutjnl-2014-306928
15. Zhao L, Zhang F, Ding X, Wu G, Lam YY, Wang X, et al. Gut Bacteria Selectively Promoted by Dietary Fibers Alleviate Type 2 Diabetes. Science (2018) 359(6380):1151–6. doi: 10.1126/science.aao5774
16. Jia J, Dou P, Gao M, Kong X, Li C, Liu Z, et al. Assessment of Causal Direction Between Gut Microbiota-Dependent Metabolites and Cardiometabolic Health: A Bidirectional Mendelian Randomization Analysis. Diabetes (2019) 68(9):1747–55. doi: 10.2337/db19-0153
17. Zhang PP, Li LL, Han X, Li QW, Zhang XH, Liu JJ, et al. Fecal Microbiota Transplantation Improves Metabolism and Gut Microbiome Composition in Db/Db Mice. Acta Pharmacol Sin (2020) 41(5):678–85. doi: 10.1038/s41401-019-0330-9
18. Vangipurapu J, Fernandes Silva L, Kuulasmaa T, Smith U, Laakso M. Microbiota-Related Metabolites and the Risk of Type 2 Diabetes. Diabetes Care (2020) 43(6):1319–25. doi: 10.2337/dc19-2533
19. Hu N, Zhang Q, Wang H, Yang X, Jiang Y, Chen R, et al. Comparative Evaluation of the Effect of Metformin and Insulin on Gut Microbiota and Metabolome Profiles of Type 2 Diabetic Rats Induced by the Combination of Streptozotocin and High-Fat Diet. Front Pharmacol (2021) 12:794103. doi: 10.3389/fphar.2021.794103
20. Ng SC, Xu Z, Mak JWY, Yang K, Liu Q, Zuo T, et al. Microbiota Engraftment After Faecal Microbiota Transplantation in Obese Subjects With Type 2 Diabetes: A 24-Week, Double-Blind, Randomised Controlled Trial. Gut (2022) 71(4):716–23. doi: 10.1136/gutjnl-2020-323617
21. Hartstra AV, Bouter KE, Bäckhed F, Nieuwdorp M. Insights Into the Role of the Microbiome in Obesity and Type 2 Diabetes. Diabetes Care (2015) 38(1):159–65. doi: 10.2337/dc14-0769
22. Qin J, Li Y, Cai Z, Li S, Zhu J, Zhang F, et al. A Metagenome-Wide Association Study of Gut Microbiota in Type 2 Diabetes. Nature (2012) 490(7418):55–60. doi: 10.1038/nature11450
23. Karlsson FH, Tremaroli V, Nookaew I, Bergström G, Behre CJ, Fagerberg B, et al. Gut Metagenome in European Women With Normal, Impaired and Diabetic Glucose Control. Nature (2013) 498(7452):99–103. doi: 10.1038/nature12198
24. Medina-Vera I, Sanchez-Tapia M, Noriega-López L, Granados-Portillo O, Guevara-Cruz M, Flores-López A, et al. A Dietary Intervention With Functional Foods Reduces Metabolic Endotoxaemia and Attenuates Biochemical Abnormalities by Modifying Faecal Microbiota in People With Type 2 Diabetes. Diabetes Metab (2019) 45(2):122–31. doi: 10.1016/j.diabet.2018.09.004
25. Org E, Blum Y, Kasela S, Mehrabian M, Kuusisto J, Kangas AJ, et al. Relationships Between Gut Microbiota, Plasma Metabolites, and Metabolic Syndrome Traits in the METSIM Cohort. Genome Biol (2017) 18(1):70. doi: 10.1186/s13059-017-1194-2
26. Li SC, Xiao Y, Wu RT, Xie D, Zhao HH, Shen GY, et al. Comparative Analysis of Type 2 Diabetes-Associated Gut Microbiota Between Han and Mongolian People. J Microbiol (2021) 59(7):693–701. doi: 10.1007/s12275-021-0454-8
27. Wu H, Tremaroli V, Schmidt C, Lundqvist A, Olsson LM, Krämer M, et al. The Gut Microbiota in Prediabetes and Diabetes: A Population-Based Cross-Sectional Study. Cell Metab (2020) 32(3):379–390.e3. doi: 10.1016/j.cmet.2020.06.011
28. Gou W, Ling CW, He Y, Jiang Z, Fu Y, Xu F, et al. Interpretable Machine Learning Framework Reveals Robust Gut Microbiome Features Associated With Type 2 Diabetes. Diabetes Care (2021) 44(2):358–66. doi: 10.2337/dc20-1536
29. Agus A, Clément K, Sokol H. Gut Microbiota-Derived Metabolites as Central Regulators in Metabolic Disorders. Gut (2021) 70(6):1174–82. doi: 10.1136/gutjnl-2020-323071
30. Krautkramer KA, Fan J, Bäckhed F. Gut Microbial Metabolites as Multi-Kingdom Intermediates. Nat Rev Microbiol (2021) 19(2):77–94. doi: 10.1038/s41579-020-0438-4
31. Chen S, Henderson A, Petriello MC, Romano KA, Gearing M, Miao J, et al. Trimethylamine N-Oxide Binds and Activates PERK to Promote Metabolic Dysfunction. Cell Metab (2019) 30(6):1141–1151.e5. doi: 10.1016/j.cmet.2019.08.021
32. Chimerel C, Emery E, Summers DK, Keyser U, Gribble FM, Reimann F. Bacterial Metabolite Indole Modulates Incretin Secretion From Intestinal Enteroendocrine L Cells. Cell Rep (2014) 9(4):1202–8. doi: 10.1016/j.celrep.2014.10.032
33. Galligan JJ. Beneficial Actions of Microbiota-Derived Tryptophan Metabolites. Neurogastroenterol Motil (2018) 30(2):e13283. doi: 10.1111/nmo.13283
34. Heianza Y, Ma W, Manson JE, Rexrode KM, Qi L. Gut Microbiota Metabolites and Risk of Major Adverse Cardiovascular Disease Events and Death: A Systematic Review and Meta-Analysis of Prospective Studies. J Am Heart Assoc (2017) 6(7):e004947. doi: 10.1161/jaha.116.004947
35. Saad MJ, Santos A, Prada PO. Linking Gut Microbiota and Inflammation to Obesity and Insulin Resistance. Physiol (Bethesda) (2016) 31(4):283–93. doi: 10.1152/physiol.00041.2015
36. Venkatesh M, Mukherjee S, Wang H, Li H, Sun K, Benechet AP, et al. Symbiotic Bacterial Metabolites Regulate Gastrointestinal Barrier Function via the Xenobiotic Sensor PXR and Toll-Like Receptor 4. Immunity (2014) 41(2):296–310. doi: 10.1016/j.immuni.2014.06.014
37. Yang W, Yu T, Huang X, Bilotta AJ, Xu L, Lu Y, et al. Intestinal Microbiota-Derived Short-Chain Fatty Acids Regulation of Immune Cell IL-22 Production and Gut Immunity. Nat Commun (2020) 11(1):4457. doi: 10.1038/s41467-020-18262-6
38. Ratajczak W, Rył A, Mizerski A, Walczakiewicz K, Sipak O, Laszczyńska M. Immunomodulatory Potential of Gut Microbiome-Derived Short-Chain Fatty Acids (SCFAs). Acta Biochim Pol (2019) 66(1):1–12. doi: 10.18388/abp.2018_2648
39. Kimura I, Ozawa K, Inoue D, Imamura T, Kimura K, Maeda T, et al. The Gut Microbiota Suppresses Insulin-Mediated Fat Accumulation via the Short-Chain Fatty Acid Receptor GPR43. Nat Commun (2013) 4:1829. doi: 10.1038/ncomms2852
40. Chávez-Talavera O, Tailleux A, Lefebvre P, Staels B. Bile Acid Control of Metabolism and Inflammation in Obesity, Type 2 Diabetes, Dyslipidemia, and Nonalcoholic Fatty Liver Disease. Gastroenterology (2017) 152(7):1679–1694.e3. doi: 10.1053/j.gastro.2017.01.055
41. Dalile B, Van Oudenhove L, Vervliet B, Verbeke K. The Role of Short-Chain Fatty Acids in Microbiota-Gut-Brain Communication. Nat Rev Gastroenterol Hepatol (2019) 16(8):461–78. doi: 10.1038/s41575-019-0157-3
42. Zhang Y, Peng Y, Zhao L, Zhou G, Li X. Regulating the Gut Microbiota and SCFAs in the Faeces of T2DM Rats Should be One of Antidiabetic Mechanisms of Mogrosides in the Fruits of Siraitia Grosvenorii. J Ethnopharmacol (2021) 274:114033. doi: 10.1016/j.jep.2021.114033
43. Yamaguchi Y, Adachi K, Sugiyama T, Shimozato A, Ebi M, Ogasawara N, et al. Association of Intestinal Microbiota With Metabolic Markers and Dietary Habits in Patients With Type 2 Diabetes. Digestion (2016) 94(2):66–72. doi: 10.1159/000447690
44. Ballan R, Saad SMI. Characteristics of the Gut Microbiota and Potential Effects of Probiotic Supplements in Individuals With Type 2 Diabetes Mellitus. Foods (2021) 10(11):2528. doi: 10.3390/foods10112528
45. Mueller NT, Differding MK, Zhang M, Maruthur NM, Juraschek SP, Miller ER, et al. Metformin Affects Gut Microbiome Composition and Function and Circulating Short-Chain Fatty Acids: A Randomized Trial. Diabetes Care (2021) 44(7):1462–71. doi: 10.2337/dc20-2257
46. Martin-Gallausiaux C, Marinelli L, Blottière HM, Larraufie P, Lapaque N. SCFA: Mechanisms and Functional Importance in the Gut. Proc Nutr Soc (2021) 80(1):37–49. doi: 10.1017/s0029665120006916
47. Yao Y, Yan L, Chen H, Wu N, Wang W, Wang D. Cyclocarya Paliurus Polysaccharides Alleviate Type 2 Diabetic Symptoms by Modulating Gut Microbiota and Short-Chain Fatty Acids. Phytomedicine (2020) 77:153268. doi: 10.1016/j.phymed.2020.153268
48. Arpaia N, Campbell C, Fan X, Dikiy S, van der Veeken J, deRoos P, et al. Metabolites Produced by Commensal Bacteria Promote Peripheral Regulatory T-Cell Generation. Nature (2013) 504(7480):451–5. doi: 10.1038/nature12726
49. Simpson BW, Trent MS. Pushing the Envelope: LPS Modifications and Their Consequences. Nat Rev Microbiol (2019) 17(7):403–16. doi: 10.1038/s41579-019-0201-x
50. Larsen N, Vogensen FK, van den Berg FW, Nielsen DS, Andreasen AS, Pedersen BK, et al. Gut Microbiota in Human Adults With Type 2 Diabetes Differs From non-Diabetic Adults. PloS One (2010) 5(2):e9085. doi: 10.1371/journal.pone.0009085
51. Ley RE, Turnbaugh PJ, Klein S, Gordon JI. Microbial Ecology: Human Gut Microbes Associated With Obesity. Nature (2006) 444(7122):1022–3. doi: 10.1038/4441022a
52. Denou E, Marcinko K, Surette MG, Steinberg GR, Schertzer JD. High-Intensity Exercise Training Increases the Diversity and Metabolic Capacity of the Mouse Distal Gut Microbiota During Diet-Induced Obesity. Am J Physiol Endocrinol Metab (2016) 310(11):E982–93. doi: 10.1152/ajpendo.00537.2015
53. Huang L, Thonusin C, Chattipakorn N, Chattipakorn SC. Impacts of Gut Microbiota on Gestational Diabetes Mellitus: A Comprehensive Review. Eur J Nutr (2021) 60(5):2343–60. doi: 10.1007/s00394-021-02483-6
54. Chen B, Wang Z, Wang J, Su X, Yang J, Zhang Q, et al. The Oral Microbiome Profile and Biomarker in Chinese Type 2 Diabetes Mellitus Patients. Endocrine (2020) 68(3):564–72. doi: 10.1007/s12020-020-02269-6
55. Magne F, Gotteland M, Gauthier L, Zazueta A, Pesoa S, Navarrete P, et al. The Firmicutes/Bacteroidetes Ratio: A Relevant Marker of Gut Dysbiosis in Obese Patients? Nutrients (2020) 12(5):1474. doi: 10.3390/nu12051474
56. Gomes JMG, Costa JA, Alfenas RCG. Metabolic Endotoxemia and Diabetes Mellitus: A Systematic Review. Metabolism (2017) 68:133–44. doi: 10.1016/j.metabol.2016.12.009
57. Fang WY, Tseng YT, Lee TY, Fu YC, Chang WH, Lo WW, et al. Triptolide Prevents LPS-Induced Skeletal Muscle Atrophy via Inhibiting NF-κb/TNF-α and Regulating Protein Synthesis/Degradation Pathway. Br J Pharmacol (2021) 178(15):2998–3016. doi: 10.1111/bph.15472
58. Zhang H, Qi C, Zhao Y, Lu M, Li X, Zhou J, et al. Depletion of Gut Secretory Immunoglobulin A Coated Lactobacillus Reuteri is Associated With Gestational Diabetes Mellitus-Related Intestinal Mucosal Barrier Damage. Food Funct (2021) 12(21):10783–94. doi: 10.1039/d1fo02517a
59. Carvalho BM, Guadagnini D, Tsukumo DML, Schenka AA, Latuf-Filho P, Vassallo J, et al. Modulation of Gut Microbiota by Antibiotics Improves Insulin Signalling in High-Fat Fed Mice. Diabetologia (2012) 55(10):2823–34. doi: 10.1007/s00125-012-2648-4
60. Jiao N, Baker SS, Chapa-Rodriguez A, Liu W, Nugent CA, Tsompana M, et al. Suppressed Hepatic Bile Acid Signalling Despite Elevated Production of Primary and Secondary Bile Acids in NAFLD. Gut (2018) 67(10):1881–91. doi: 10.1136/gutjnl-2017-314307
61. Thomas C, Gioiello A, Noriega L, Strehle A, Oury J, Rizzo G, et al. TGR5-Mediated Bile Acid Sensing Controls Glucose Homeostasis. Cell Metab (2009) 10(3):167–77. doi: 10.1016/j.cmet.2009.08.001
62. Gu Y, Wang X, Li J, Zhang Y, Zhong H, Liu R, et al. Analyses of Gut Microbiota and Plasma Bile Acids Enable Stratification of Patients for Antidiabetic Treatment. Nat Commun (2017) 8(1):1785. doi: 10.1038/s41467-017-01682-2
63. Li W, Liu R, Li X, Tao B, Zhai N, Wang X, et al. Saxagliptin Alters Bile Acid Profiles and Yields Metabolic Benefits in Drug-Naïve Overweight or Obese Type 2 Diabetes Patient. J Diabetes (2019) 11(12):982–92. doi: 10.1111/1753-0407.12956
64. Haal S, Guman MSS, Boerlage TCC, Acherman YIZ, de Brauw LM, Bruin S, et al. Ursodeoxycholic Acid for the Prevention of Symptomatic Gallstone Disease After Bariatric Surgery (UPGRADE): A Multicentre, Double-Blind, Randomised, Placebo-Controlled Superiority Trial. Lancet Gastroenterol Hepatol (2021) 6(12):993–1001. doi: 10.1016/s2468-1253(21)00301-0
65. Velasquez MT, Ramezani A, Manal A, Raj DS. Trimethylamine N-Oxide: The Good, the Bad and the Unknown. Toxins (Basel) (2016) 8(11):326. doi: 10.3390/toxins8110326
66. Romano KA, Vivas EI, Amador-Noguez D, Rey FE. Intestinal Microbiota Composition Modulates Choline Bioavailability From Diet and Accumulation of the Proatherogenic Metabolite Trimethylamine-N-Oxide. mBio (2015) 6(2):e02481. doi: 10.1128/mBio.02481-14
67. Chen ML, Zhu XH, Ran L, Lang HD, Yi L, Mi MT. Trimethylamine-N-Oxide Induces Vascular Inflammation by Activating the NLRP3 Inflammasome Through the SIRT3-SOD2-mtROS Signaling Pathway. J Am Heart Assoc (2017) 6(9):e006347. doi: 10.1161/jaha.117.006347
68. Zhu Y, Zhao J, Luo L, Gao Y, Bao H, Li P, et al. Research Progress of Indole Compounds With Potential Antidiabetic Activity. Eur J Med Chem (2021) 223:113665. doi: 10.1016/j.ejmech.2021.113665
69. Abboud M, Rizk R, AlAnouti F, Papandreou D, Haidar S, Mahboub N. The Health Effects of Vitamin D and Probiotic Co-Supplementation: A Systematic Review of Randomized Controlled Trials. Nutrients (2020) 13(1):111. doi: 10.3390/nu13010111
70. Pedersen HK, Gudmundsdottir V, Nielsen HB, Hyotylainen T, Nielsen T, Jensen BA, et al. Human Gut Microbes Impact Host Serum Metabolome and Insulin Sensitivity. Nature (2016) 535(7612):376–81. doi: 10.1038/nature18646
71. He L. Metformin and Systemic Metabolism. Trends Pharmacol Sci (2020) 41(11):868–81. doi: 10.1016/j.tips.2020.09.001
72. Lv Z, Guo Y. Metformin and Its Benefits for Various Diseases. Front Endocrinol (Lausanne) (2020) 11:191. doi: 10.3389/fendo.2020.00191
73. Wang Y, Xu W, Yan Z, Zhao W, Mi J, Li J, et al. Metformin Induces Autophagy and G0/G1 Phase Cell Cycle Arrest in Myeloma by Targeting the AMPK/mTORC1 and Mtorc2 Pathways. J Exp Clin Cancer Res (2018) 37(1):63. doi: 10.1186/s13046-018-0731-5
74. Foretz M, Guigas B, Viollet B. Understanding the Glucoregulatory Mechanisms of Metformin in Type 2 Diabetes Mellitus. Nat Rev Endocrinol (2019) 15(10):569–89. doi: 10.1038/s41574-019-0242-2
75. Madiraju AK, Qiu Y, Perry RJ, Rahimi Y, Zhang XM, Zhang D, et al. Metformin Inhibits Gluconeogenesis via a Redox-Dependent Mechanism In Vivo. Nat Med (2018) 24(9):1384–94. doi: 10.1038/s41591-018-0125-4
76. Sun L, Xie C, Wang G, Wu Y, Wu Q, Wang X, et al. Gut Microbiota and Intestinal FXR Mediate the Clinical Benefits of Metformin. Nat Med (2018) 24(12):1919–29. doi: 10.1038/s41591-018-0222-4
77. Forslund K, Hildebrand F, Nielsen T, Falony G, Le Chatelier E, Sunagawa S, et al. Disentangling Type 2 Diabetes and Metformin Treatment Signatures in the Human Gut Microbiota. Nature (2015) 528(7581):262–6. doi: 10.1038/nature15766
78. Tong X, Xu J, Lian F, Yu X, Zhao Y, Xu L, et al. Structural Alteration of Gut Microbiota During the Amelioration of Human Type 2 Diabetes With Hyperlipidemia by Metformin and a Traditional Chinese Herbal Formula: A Multicenter, Randomized, Open Label Clinical Trial. mBio (2018) 9(3):e02392–17. doi: 10.1128/mBio.02392-17
79. Wu H, Esteve E, Tremaroli V, Khan MT, Caesar R, Mannerås-Holm L, et al. Metformin Alters the Gut Microbiome of Individuals With Treatment-Naive Type 2 Diabetes, Contributing to the Therapeutic Effects of the Drug. Nat Med (2017) 23(7):850–8. doi: 10.1038/nm.4345
80. Ahmadi S, Razazan A, Nagpal R, Jain S, Wang B, Mishra SP, et al. Metformin Reduces Aging-Related Leaky Gut and Improves Cognitive Function by Beneficially Modulating Gut Microbiome/Goblet Cell/Mucin Axis. J Gerontol A Biol Sci Med Sci (2020) 75(7):e9–e21. doi: 10.1093/gerona/glaa056
81. Huang F, Nilholm C, Roth B, Linninge C, Höglund P, Nyman M, et al. Anthropometric and Metabolic Improvements in Human Type 2 Diabetes After Introduction of an Okinawan-Based Nordic Diet are Not Associated With Changes in Microbial Diversity or SCFA Concentrations. Int J Food Sci Nutr (2018) 69(6):729–40. doi: 10.1080/09637486.2017.1408059
82. Palacios T, Vitetta L, Coulson S, Madigan CD, Lam YY, Manuel R, et al. Targeting the Intestinal Microbiota to Prevent Type 2 Diabetes and Enhance the Effect of Metformin on Glycaemia: A Randomised Controlled Pilot Study. Nutrients (2020) 12(7):2041. doi: 10.3390/nu12072041
83. Napolitano A, Miller S, Nicholls AW, Baker D, Van Horn S, Thomas E, et al. Novel Gut-Based Pharmacology of Metformin in Patients With Type 2 Diabetes Mellitus. PloS One (2014) 9(7):e100778. doi: 10.1371/journal.pone.0100778
84. de la Cuesta-Zuluaga J, Mueller NT, Corrales-Agudelo V, Velásquez-Mejía EP, Carmona JA, Abad JM, et al. Metformin Is Associated With Higher Relative Abundance of Mucin-Degrading Akkermansia Muciniphila and Several Short-Chain Fatty Acid-Producing Microbiota in the Gut. Diabetes Care (2017) 40(1):54–62. doi: 10.2337/dc16-1324
85. Zhang F, Wang M, Yang J, Xu Q, Liang C, Chen B, et al. Response of Gut Microbiota in Type 2 Diabetes to Hypoglycemic Agents. Endocrine (2019) 66(3):485–93. doi: 10.1007/s12020-019-02041-5
86. Barengolts E, Green SJ, Eisenberg Y, Akbar A, Reddivari B, Layden BT, et al. Gut microbiota varies by opioid use, circulating leptin and oxytocin in African American men with diabetes and high burden of chronic disease. PloS one (2018) 13(3):e0194171. doi: 10.1371/journal.pone.0194171
87. van Bommel EJM, Herrema H, Davids M, Kramer MHH, Nieuwdorp M, van Raalte DH. Effects of 12-Week Treatment With Dapagliflozin and Gliclazide on Faecal Microbiome: Results of a Double-Blind Randomized Trial in Patients With Type 2 Diabetes. Diabetes Metab (2020) 46(2):164–8. doi: 10.1016/j.diabet.2019.11.005
88. Deng X, Zhang C, Wang P, Wei W, Shi X, Wang P, et al. Cardiovascular Benefits of Empagliflozin are Associated With Gut Microbiota and Plasma Metabolites in Type 2 Diabetes. J Clin Endocrinol Metab (2022) 107(7):1888–96. doi: 10.1210/clinem/dgac210
89. Smits MM, Fluitman KS, Herrema H, Davids M, Kramer MHH, Groen AK, et al. Liraglutide and Sitagliptin Have No Effect on Intestinal Microbiota Composition: A 12-Week Randomized Placebo-Controlled Trial in Adults With Type 2 Diabetes. Diabetes Metab (2021) 47(5):101223. doi: 10.1016/j.diabet.2021.101223
90. Smits MM, Tonneijck L, Muskiet MH, Hoekstra T, Kramer MH, Diamant M, et al. Biliary Effects of Liraglutide and Sitagliptin, a 12-Week Randomized Placebo-Controlled Trial in Type 2 Diabetes Patients. Diabetes Obes Metab (2016) 18(12):1217–25. doi: 10.1111/dom.12748
91. Wang Z, Wang J, Hu J, Chen Y, Dong B, Wang Y. A Comparative Study of Acarbose, Vildagliptin and Saxagliptin Intended for Better Efficacy and Safety on Type 2 Diabetes Mellitus Treatment. Life Sci (2021) 274:119069. doi: 10.1016/j.lfs.2021.119069
92. Takewaki F, Nakajima H, Takewaki D, Hashimoto Y, Majima S, Okada H, et al. Habitual Dietary Intake Affects the Altered Pattern of Gut Microbiome by Acarbose in Patients With Type 2 Diabetes. Nutrients (2021) 13(6):2107. doi: 10.3390/nu13062107
93. Su B, Liu H, Li J, Sunli Y, Liu B, Liu D, et al. Acarbose Treatment Affects the Serum Levels of Inflammatory Cytokines and the Gut Content of Bifidobacteria in Chinese Patients With Type 2 Diabetes Mellitus. J Diabetes (2015) 7(5):729–39. doi: 10.1111/1753-0407.12232
94. Zhang M, Feng R, Yang M, Qian C, Wang Z, Liu W, et al. Effects of Metformin, Acarbose, and Sitagliptin Monotherapy on Gut Microbiota in Zucker Diabetic Fatty Rats. BMJ Open Diabetes Res Care (2019) 7(1):e000717. doi: 10.1136/bmjdrc-2019-000717
95. Lee Y, Kim AH, Kim E, Lee S, Yu KS, Jang IJ, et al. Changes in the Gut Microbiome Influence the Hypoglycemic Effect of Metformin Through the Altered Metabolism of Branched-Chain and Nonessential Amino Acids. Diabetes Res Clin Pract (2021) 178:108985. doi: 10.1016/j.diabres.2021.108985
96. Zhang W, Xu JH, Yu T, Chen QK. Effects of Berberine and Metformin on Intestinal Inflammation and Gut Microbiome Composition in Db/Db Mice. BioMed Pharmacother (2019) 118:109131. doi: 10.1016/j.biopha.2019.109131
97. Liu Z, Liao W, Zhang Z, Sun R, Luo Y, Chen Q, et al. Metformin Affects Gut Microbiota Composition and Diversity Associated With Amelioration of Dextran Sulfate Sodium-Induced Colitis in Mice. Front Pharmacol (2021) 12:640347. doi: 10.3389/fphar.2021.640347
98. Schönfeld P, Wojtczak L. Short- and Medium-Chain Fatty Acids in Energy Metabolism: The Cellular Perspective. J Lipid Res (2016) 57(6):943–54. doi: 10.1194/jlr.R067629
99. Shin NR, Lee JC, Lee HY, Kim MS, Whon TW, Lee MS, et al. An Increase in the Akkermansia Spp. Population Induced by Metformin Treatment Improves Glucose Homeostasis in Diet-Induced Obese Mice. Gut (2014) 63(5):727–35. doi: 10.1136/gutjnl-2012-303839
100. McCreight LJ, Bailey CJ, Pearson ER. Metformin and the Gastrointestinal Tract. Diabetologia (2016) 59(3):426–35. doi: 10.1007/s00125-015-3844-9
101. Bennis Y, Bodeau S, Batteux B, Gras-Champel V, Masmoudi K, Maizel J, et al. A Study of Associations Between Plasma Metformin Concentration, Lactic Acidosis, and Mortality in an Emergency Hospitalization Context. Crit Care Med (2020) 48(12):e1194–202. doi: 10.1097/ccm.0000000000004589
102. Lazarus B, Wu A, Shin JI, Sang Y, Alexander GC, Secora A, et al. Association of Metformin Use With Risk of Lactic Acidosis Across the Range of Kidney Function: A Community-Based Cohort Study. JAMA Intern Med (2018) 178(7):903–10. doi: 10.1001/jamainternmed.2018.0292
103. Bryrup T, Thomsen CW, Kern T, Allin KH, Brandslund I, Jørgensen NR, et al. Metformin-Induced Changes of the Gut Microbiota in Healthy Young Men: Results of a non-Blinded, One-Armed Intervention Study. Diabetologia (2019) 62(6):1024–35. doi: 10.1007/s00125-019-4848-7
104. Cowie MR, Fisher M. SGLT2 Inhibitors: Mechanisms of Cardiovascular Benefit Beyond Glycaemic Control. Nat Rev Cardiol (2020) 17(12):761–72. doi: 10.1038/s41569-020-0406-8
105. Wiviott SD, Raz I, Bonaca MP, Mosenzon O, Kato ET, Cahn A, et al. Dapagliflozin and Cardiovascular Outcomes in Type 2 Diabetes. N Engl J Med (2019) 380(4):347–57. doi: 10.1056/NEJMoa1812389
106. Perkovic V, Jardine MJ, Neal B, Bompoint S, Heerspink HJL, Charytan DM, et al. Canagliflozin and Renal Outcomes in Type 2 Diabetes and Nephropathy. N Engl J Med (2019) 380(24):2295–306. doi: 10.1056/NEJMoa1811744
107. Thirunavukarasu S, Jex N, Chowdhary A, Hassan IU, Straw S, Craven TP, et al. Empagliflozin Treatment Is Associated With Improvements in Cardiac Energetics and Function and Reductions in Myocardial Cellular Volume in Patients With Type 2 Diabetes. Diabetes (2021) 70(12):2810–22. doi: 10.2337/db21-0270
108. Yurista SR, Chong CR, Badimon JJ, Kelly DP, de Boer RA, Westenbrink BD. Therapeutic Potential of Ketone Bodies for Patients With Cardiovascular Disease: JACC State-Of-the-Art Review. J Am Coll Cardiol (2021) 77(13):1660–9. doi: 10.1016/j.jacc.2020.12.065
109. Lee DM, Battson ML, Jarrell DK, Hou S, Ecton KE, Weir TL, et al. SGLT2 Inhibition via Dapagliflozin Improves Generalized Vascular Dysfunction and Alters the Gut Microbiota in Type 2 Diabetic Mice. Cardiovasc Diabetol (2018) 17(1):62. doi: 10.1186/s12933-018-0708-x
110. Li L, Xu S, Guo T, Gong S, Zhang C. Effect of Dapagliflozin on Intestinal Flora in MafA-Deficient Mice. Curr Pharm Des (2018) 24(27):3223–31. doi: 10.2174/1381612824666180912143434
111. Oh TJ, Sul WJ, Oh HN, Lee YK, Lim HL, Choi SH, et al. Butyrate Attenuated Fat Gain Through Gut Microbiota Modulation in Db/Db Mice Following Dapagliflozin Treatment. Sci Rep (2019) 9(1):20300. doi: 10.1038/s41598-019-56684-5
112. Yang M, Shi FH, Liu W, Zhang MC, Feng RL, Qian C, et al. Dapagliflozin Modulates the Fecal Microbiota in a Type 2 Diabetic Rat Model. Front Endocrinol (Lausanne) (2020) 11:635. doi: 10.3389/fendo.2020.00635
113. Mishima E, Fukuda S, Kanemitsu Y, Saigusa D, Mukawa C, Asaji K, et al. Canagliflozin Reduces Plasma Uremic Toxins and Alters the Intestinal Microbiota Composition in a Chronic Kidney Disease Mouse Model. Am J Physiol Renal Physiol (2018) 315(4):F824–f833. doi: 10.1152/ajprenal.00314.2017
114. Zhang Q, Yu H, Xiao X, Hu L, Xin F, Yu X. Inulin-Type Fructan Improves Diabetic Phenotype and Gut Microbiota Profiles in Rats. PeerJ (2018) 6:e4446. doi: 10.7717/peerj.4446
115. Just S, Mondot S, Ecker J, Wegner K, Rath E, Gau L, et al. The Gut Microbiota Drives the Impact of Bile Acids and Fat Source in Diet on Mouse Metabolism. Microbiome (2018) 6(1):134. doi: 10.1186/s40168-018-0510-8
116. Osataphan S, Macchi C, Singhal G, Chimene-Weiss J, Sales V, Kozuka C, et al. SGLT2 Inhibition Reprograms Systemic Metabolism via FGF21-Dependent and -Independent Mechanisms. JCI Insight (2019) 4(5):e123130. doi: 10.1172/jci.insight.123130
117. Ahmadian M, Suh JM, Hah N, Liddle C, Atkins AR, Downes M, et al. Pparγ Signaling and Metabolism: The Good, the Bad and the Future. Nat Med (2013) 19(5):557–66. doi: 10.1038/nm.3159
118. Yang H, Suh DH, Kim DH, Jung ES, Liu KH, Lee CH, et al. Metabolomic and Lipidomic Analysis of the Effect of Pioglitazone on Hepatic Steatosis in a Rat Model of Obese Type 2 Diabetes. Br J Pharmacol (2018) 175(17):3610–25. doi: 10.1111/bph.14434
119. Madsen MSA, Grønlund RV, Eid J, Christensen-Dalsgaard M, Sommer M, Rigbolt K, et al. Characterization of Local Gut Microbiome and Intestinal Transcriptome Responses to Rosiglitazone Treatment in Diabetic Db/Db Mice. BioMed Pharmacother (2021) 133:110966. doi: 10.1016/j.biopha.2020.110966
120. Lefebvre M, Paulweber B, Fajas L, Woods J, McCrary C, Colombel JF, et al. Peroxisome Proliferator-Activated Receptor Gamma is Induced During Differentiation of Colon Epithelium Cells. J Endocrinol (1999) 162(3):331–40. doi: 10.1677/joe.0.1620331
121. Li JM, Yu R, Zhang LP, Wen SY, Wang SJ, Zhang XY, et al. Dietary Fructose-Induced Gut Dysbiosis Promotes Mouse Hippocampal Neuroinflammation: A Benefit of Short-Chain Fatty Acids. Microbiome (2019) 7(1):98. doi: 10.1186/s40168-019-0713-7
122. Aggarwal H, Pathak P, Kumar Y, Jagavelu K, Dikshit M. Modulation of Insulin Resistance, Dyslipidemia and Serum Metabolome in iNOS Knockout Mice Following Treatment With Nitrite, Metformin, Pioglitazone, and a Combination of Ampicillin and Neomycin. Int J Mol Sci (2021) 23(1):195. doi: 10.3390/ijms23010195
123. Thornberry NA, Gallwitz B. Mechanism of Action of Inhibitors of Dipeptidyl-Peptidase-4 (DPP-4). Best Pract Res Clin Endocrinol Metab (2009) 23(4):479–86. doi: 10.1016/j.beem.2009.03.004
124. Liao X, Song L, Zeng B, Liu B, Qiu Y, Qu H, et al. Alteration of Gut Microbiota Induced by DPP-4i Treatment Improves Glucose Homeostasis. EBioMedicine (2019) 44:665–74. doi: 10.1016/j.ebiom.2019.03.057
125. Ryan PM, Patterson E, Carafa I, Mandal R, Wishart DS, Dinan TG, et al. Metformin and Dipeptidyl Peptidase-4 Inhibitor Differentially Modulate the Intestinal Microbiota and Plasma Metabolome of Metabolically Dysfunctional Mice. Can J Diabetes (2020) 44(2):146–155.e2. doi: 10.1016/j.jcjd.2019.05.008
126. Silva-Veiga FM, Miranda CS, Martins FF, Daleprane JB, Mandarim-de-Lacerda CA, Souza-Mello V. Gut-Liver Axis Modulation in Fructose-Fed Mice: A Role for PPAR-Alpha and Linagliptin. J Endocrinol (2020) 247(1):11–24. doi: 10.1530/joe-20-0139
127. Olivares M, Neyrinck AM, Pötgens SA, Beaumont M, Salazar N, Cani PD, et al. The DPP-4 Inhibitor Vildagliptin Impacts the Gut Microbiota and Prevents Disruption of Intestinal Homeostasis Induced by a Western Diet in Mice. Diabetologia (2018) 61(8):1838–48. doi: 10.1007/s00125-018-4647-6
128. Wang L, Li P, Tang Z, Yan X, Feng B. Structural Modulation of the Gut Microbiota and the Relationship With Body Weight: Compared Evaluation of Liraglutide and Saxagliptin Treatment. Sci Rep (2016) 6:33251. doi: 10.1038/srep33251
129. Zhang Q, Xiao X, Li M, Yu M, Ping F, Zheng J, et al. Vildagliptin Increases Butyrate-Producing Bacteria in the Gut of Diabetic Rats. PloS One (2017) 12(10):e0184735. doi: 10.1371/journal.pone.0184735
130. Yan X, Feng B, Li P, Tang Z, Wang L. Microflora Disturbance During Progression of Glucose Intolerance and Effect of Sitagliptin: An Animal Study. J Diabetes Res (2016) 2016:2093171. doi: 10.1155/2016/2093171
131. Dirir AM, Daou M, Yousef AF, Yousef LF. A Review of Alpha-Glucosidase Inhibitors From Plants as Potential Candidates for the Treatment of Type-2 Diabetes. Phytochem Rev (2021), 1–31. doi: 10.1007/s11101-021-09773-1
132. Balaich J, Estrella M, Wu G, Jeffrey PD, Biswas A, Zhao L, et al. The Human Microbiome Encodes Resistance to the Antidiabetic Drug Acarbose. Nature (2021) 600(7887):110–5. doi: 10.1038/s41586-021-04091-0
133. Tan K, Tesar C, Wilton R, Jedrzejczak RP, Joachimiak A. Interaction of Antidiabetic α-Glucosidase Inhibitors and Gut Bacteria α-Glucosidase. Protein Sci (2018) 27(8):1498–508. doi: 10.1002/pro.3444
134. Smith BJ, Miller RA, Ericsson AC, Harrison DC, Strong R, Schmidt TM. Changes in the Gut Microbiome and Fermentation Products Concurrent With Enhanced Longevity in Acarbose-Treated Mice. BMC Microbiol (2019) 19(1):130. doi: 10.1186/s12866-019-1494-7
135. Do HJ, Lee YS, Ha MJ, Cho Y, Yi H, Hwang YJ, et al. Beneficial Effects of Voglibose Administration on Body Weight and Lipid Metabolism via Gastrointestinal Bile Acid Modification. Endocr J (2016) 63(8):691–702. doi: 10.1507/endocrj.EJ15-0747
136. Baxter NT, Lesniak NA, Sinani H, Schloss PD, Koropatkin NM. The Glucoamylase Inhibitor Acarbose Has a Diet-Dependent and Reversible Effect on the Murine Gut Microbiome. mSphere (2019) 4(1):e00528–18. doi: 10.1128/mSphere.00528-18
137. Li ZR, Jia RB, Wu J, Lin L, Ou ZR, Liao B, et al. Sargassum Fusiforme Polysaccharide Partly Replaces Acarbose Against Type 2 Diabetes in Rats. Int J Biol Macromol (2021) 170:447–58. doi: 10.1016/j.ijbiomac.2020.12.126
138. Zhang L, Song P, Zhang X, Metea C, Schleisman M, Karstens L, et al. Alpha-Glucosidase Inhibitors Alter Gut Microbiota and Ameliorate Collagen-Induced Arthritis. Front Pharmacol (2019) 10:1684. doi: 10.3389/fphar.2019.01684
139. Kishida Y, Okubo H, Ohno H, Oki K, Yoneda M. Effect of Miglitol on the Suppression of Nonalcoholic Steatohepatitis Development and Improvement of the Gut Environment in a Rodent Model. J Gastroenterol (2017) 52(11):1180–91. doi: 10.1007/s00535-017-1331-4
140. Kato T, Iizuka K, Takao K, Horikawa Y, Kitamura T, Takeda J. ChREBP-Knockout Mice Show Sucrose Intolerance and Fructose Malabsorption. Nutrients (2018) 10(3):340. doi: 10.3390/nu10030340
141. Walters WA, Xu Z, Knight R. Meta-Analyses of Human Gut Microbes Associated With Obesity and IBD. FEBS Lett (2014) 588(22):4223–33. doi: 10.1016/j.febslet.2014.09.039
142. Smith BJ, Miller RA, Schmidt TM. Muribaculaceae Genomes Assembled From Metagenomes Suggest Genetic Drivers of Differential Response to Acarbose Treatment in Mice. mSphere (2021) 6(6):e0085121. doi: 10.1128/msphere.00851-21
143. Nepal MR, Kang MJ, Kim GH, Cha DH, Kim JH, Jeong TC. Role of Intestinal Microbiota in Metabolism of Voglibose In Vitro and In Vivo. Diabetes Metab J (2020) 44(6):908–18. doi: 10.4093/dmj.2019.0147
144. Patnode ML, Beller ZW, Han ND, Cheng J, Peters SL, Terrapon N, et al. Interspecies Competition Impacts Targeted Manipulation of Human Gut Bacteria by Fiber-Derived Glycans. Cell (2019) 179(1):59–73.e13. doi: 10.1016/j.cell.2019.08.011
145. Huo T, Xiong Z, Lu X, Cai S. Metabonomic Study of Biochemical Changes in Urinary of Type 2 Diabetes Mellitus Patients After the Treatment of Sulfonylurea Antidiabetic Drugs Based on Ultra-Performance Liquid Chromatography/Mass Spectrometry. BioMed Chromatogr (2015) 29(1):115–22. doi: 10.1002/bmc.3247
146. Đanić M, Pavlović N, Stanimirov B, Lazarević S, Vukmirović S, Al-Salami H, et al. PAMPA Model of Gliclazide Permeability: The Impact of Probiotic Bacteria and Bile Acids. Eur J Pharm Sci (2021) 158:105668. doi: 10.1016/j.ejps.2020.105668
147. Kondo Y, Hashimoto Y, Hamaguchi M, Ando S, Kaji A, Sakai R, et al. Unique Habitual Food Intakes in the Gut Microbiota Cluster Associated With Type 2 Diabetes Mellitus. Nutrients (2021) 13(11):3816. doi: 10.3390/nu13113816
148. Deeks ED. Chiglitazar: First Approval. Drugs (2022) 82(1):87–92. doi: 10.1007/s40265-021-01648-1
149. Hallakou-Bozec S, Vial G, Kergoat M, Fouqueray P, Bolze S, Borel AL, et al. Mechanism of Action of Imeglimin: A Novel Therapeutic Agent for Type 2 Diabetes. Diabetes Obes Metab (2021) 23(3):664–73. doi: 10.1111/dom.14277
150. Wang Y, Li H, Gao H, Xu X, Cai T, Wang H, et al. Effect of Chiglitazar and Sitagliptin on Glucose Variations, Insulin Resistance and Inflammatory-Related Biomarkers in Untreated Patients With Type 2 Diabetes. Diabetes Res Clin Pract (2022) 183:109171. doi: 10.1016/j.diabres.2021.109171
151. Li PP, Shan S, Chen YT, Ning ZQ, Sun SJ, Liu Q, et al. The PPARalpha/gamma Dual Agonist Chiglitazar Improves Insulin Resistance and Dyslipidemia in MSG Obese Rats. Br J Pharmacol (2006) 148(5):610–8. doi: 10.1038/sj.bjp.0706745
152. Dubourg J, Fouqueray P, Thang C, Grouin JM, Ueki K. Efficacy and Safety of Imeglimin Monotherapy Versus Placebo in Japanese Patients With Type 2 Diabetes (TIMES 1): A Double-Blind, Randomized, Placebo-Controlled, Parallel-Group, Multicenter Phase 3 Trial. Diabetes Care (2021) 44(4):952–9. doi: 10.2337/dc20-0763
153. Dubourg J, Fouqueray P, Quinslot D, Grouin JM, Kaku K. Long-Term Safety and Efficacy of Imeglimin as Monotherapy or in Combination With Existing Antidiabetic Agents in Japanese Patients With Type 2 Diabetes (TIMES 2): A 52-Week, Open-Label, Multicentre Phase 3 Trial. Diabetes Obes Metab (2022) 24(4):609–19. doi: 10.1111/dom.14613
154. Li J, Inoue R, Togashi Y, Okuyama T, Satoh A, Kyohara M, et al. Imeglimin Ameliorates β-Cell Apoptosis by Modulating the Endoplasmic Reticulum Homeostasis Pathway. Diabetes (2022) 71(3):424–39. doi: 10.2337/db21-0123
155. Toulis KA, Nirantharakumar K, Pourzitaki C, Barnett AH, Tahrani AA. Glucokinase Activators for Type 2 Diabetes: Challenges and Future Developments. Drugs (2020) 80(5):467–75. doi: 10.1007/s40265-020-01278-z
156. Chen X, Chen Z, Xu D, Lyu Y, Li Y, Li S, et al. De Novo Design of G Protein-Coupled Receptor 40 Peptide Agonists for Type 2 Diabetes Mellitus Based on Artificial Intelligence and Site-Directed Mutagenesis. Front Bioeng Biotechnol (2021) 9:694100. doi: 10.3389/fbioe.2021.694100
157. Scheen AJ. Could Metformin Modulate Cardiovascular Outcomes Differently With DPP-4 Inhibitors Compared With SGLT2 Inhibitors? Diabetes Metab (2021) 47(4):101209. doi: 10.1016/j.diabet.2020.11.001
158. Deeks ED, Scott LJ. Pioglitazone/Metformin. Drugs (2006) 66(14):1863–77; discussion 1878-80. doi: 10.2165/00003495-200666140-00007
159. Salgaço MK, Oliveira LGS, Costa GN, Bianchi F, Sivieri K. Relationship Between Gut Microbiota, Probiotics, and Type 2 Diabetes Mellitus. Appl Microbiol Biotechnol (2019) 103(23-24):9229–38. doi: 10.1007/s00253-019-10156-y
160. Schwientek P, Szczepanowski R, Rückert C, Kalinowski J, Klein A, Selber K, et al. The Complete Genome Sequence of the Acarbose Producer Actinoplanes Sp. SE50/110. BMC Genomics (2012) 13:112. doi: 10.1186/1471-2164-13-112
161. Panwar H, Calderwood D, Grant IR, Grover S, Green BD. Lactobacillus Strains Isolated From Infant Faeces Possess Potent Inhibitory Activity Against Intestinal Alpha- and Beta-Glucosidases Suggesting Anti-Diabetic Potential. Eur J Nutr (2014) 53(7):1465–74. doi: 10.1007/s00394-013-0649-9
162. Kattar SA, Jurjus R, Pinon A, Leger DY, Jurjus A, Boukarim C, et al. Metformin and Probiotics in the Crosstalk Between Colitis-Associated Colorectal Cancer and Diabetes in Mice. Cancers (Basel) (2020) 12(7):1857. doi: 10.3390/cancers12071857
163. Snelson M, de Pasquale C, Ekinci EI, Coughlan MT. Gut Microbiome, Prebiotics, Intestinal Permeability and Diabetes Complications. Best Pract Res Clin Endocrinol Metab (2021) 35(3):101507. doi: 10.1016/j.beem.2021.101507
164. Guo Y, Yu Y, Li H, Ding X, Li X, Jing X, et al. Inulin Supplementation Ameliorates Hyperuricemia and Modulates Gut Microbiota in Uox-Knockout Mice. Eur J Nutr (2021) 60(4):2217–30. doi: 10.1007/s00394-020-02414-x
165. Pedersen C, Gallagher E, Horton F, Ellis RJ, Ijaz UZ, Wu H, et al. Host-Microbiome Interactions in Human Type 2 Diabetes Following Prebiotic Fibre (Galacto-Oligosaccharide) Intake. Br J Nutr (2016) 116(11):1869–77. doi: 10.1017/s0007114516004086
166. Burton JH, Johnson M, Johnson J, Hsia DS, Greenway FL, Heiman ML. Addition of a Gastrointestinal Microbiome Modulator to Metformin Improves Metformin Tolerance and Fasting Glucose Levels. J Diabetes Sci Technol (2015) 9(4):808–14. doi: 10.1177/1932296815577425
167. McIntyre HD, Catalano P, Zhang C, Desoye G, Mathiesen ER, Damm P. Gestational Diabetes Mellitus. Nat Rev Dis Primers (2019) 5(1):47. doi: 10.1038/s41572-019-0098-8
168. Molina-Vega M, Picón-César MJ, Gutiérrez-Repiso C, Fernández-Valero A, Lima-Rubio F, González-Romero S, et al. Metformin Action Over Gut Microbiota is Related to Weight and Glycemic Control in Gestational Diabetes Mellitus: A Randomized Trial. BioMed Pharmacother (2022) 145:112465. doi: 10.1016/j.biopha.2021.112465
169. Taylor BL, Woodfall GE, Sheedy KE, O'Riley ML, Rainbow KA, Bramwell EL, et al. Effect of Probiotics on Metabolic Outcomes in Pregnant Women With Gestational Diabetes: A Systematic Review and Meta-Analysis of Randomized Controlled Trials. Nutrients (2017) 9(5):461. doi: 10.3390/nu9050461
170. Miao M, Wang Q, Wang X, Fan C, Luan T, Yan L, et al. The Protective Effects of Inulin-Type Fructans Against High-Fat/Sucrose Diet-Induced Gestational Diabetes Mice in Association With Gut Microbiota Regulation. Front Microbiol (2022) 13:832151. doi: 10.3389/fmicb.2022.832151
171. Callaway LK, McIntyre HD, Barrett HL, Foxcroft K, Tremellen A, Lingwood BE, et al. Probiotics for the Prevention of Gestational Diabetes Mellitus in Overweight and Obese Women: Findings From the SPRING Double-Blind Randomized Controlled Trial. Diabetes Care (2019) 42(3):364–71. doi: 10.2337/dc18-2248
172. Hanssen NMJ, de Vos WM, Nieuwdorp M. Fecal Microbiota Transplantation in Human Metabolic Diseases: From a Murky Past to a Bright Future? Cell Metab (2021) 33(6):1098–110. doi: 10.1016/j.cmet.2021.05.005
173. Su L, Hong Z, Zhou T, Jian Y, Xu M, Zhang X, et al. Health Improvements of Type 2 Diabetic Patients Through Diet and Diet Plus Fecal Microbiota Transplantation. Sci Rep (2022) 12(1):1152. doi: 10.1038/s41598-022-05127-9
174. Liu Y, Wang Y, Ni Y, Cheung CKY, Lam KSL, Wang Y, et al. Gut Microbiome Fermentation Determines the Efficacy of Exercise for Diabetes Prevention. Cell Metab (2020) 31(1):77–91.e5. doi: 10.1016/j.cmet.2019.11.001
Keywords: gut microbiota, microbial metabolites, T2DM, antidiabetic drugs, SCFA
Citation: Wang D, Liu J, Zhou L, Zhang Q, Li M and Xiao X (2022) Effects of Oral Glucose-Lowering Agents on Gut Microbiota and Microbial Metabolites. Front. Endocrinol. 13:905171. doi: 10.3389/fendo.2022.905171
Received: 26 March 2022; Accepted: 14 June 2022;
Published: 13 July 2022.
Edited by:
Alok Raghav, Ganesh Shankar Vidyarthi Memorial Medical College, IndiaReviewed by:
Yongbo Kang, Shanxi Medical University, ChinaKirti Amresh Gautam, G D Goenka University, India
Copyright © 2022 Wang, Liu, Zhou, Zhang, Li and Xiao. This is an open-access article distributed under the terms of the Creative Commons Attribution License (CC BY). The use, distribution or reproduction in other forums is permitted, provided the original author(s) and the copyright owner(s) are credited and that the original publication in this journal is cited, in accordance with accepted academic practice. No use, distribution or reproduction is permitted which does not comply with these terms.
*Correspondence: Xinhua Xiao, eGlhb3hoMjAxNEB2aXAuMTYzLmNvbQ==