- 1Institute of Biomedicine, Research Centre for Integrative Physiology and Pharmacology, University of Turku, Turku, Finland
- 2Department of Pediatrics, Turku University Hospital, Turku, Finland
- 3Centre for Population Health Research, University of Turku and Turku University Hospital, Turku, Finland
The retinoblastoma (RB) protein family members (pRB, p107 and p130) are key regulators of cell cycle progression, but also play crucial roles in apoptosis, and stem cell self-renewal and differentiation. RB proteins exert their effects through binding to E2F transcription factors, which are essential developmental and physiological regulators of tissue and organ homeostasis. According to the canonical view, phosphorylation of RB results in release of E2Fs and induction of genes needed for progress of the cell cycle. However, there are eight members in the E2F transcription factor family with both activator (E2F1-3a) and repressor (E2F3b–E2F8) roles, highlighting the functional diversity of RB-E2F pathway. In this review article we summarize the data showing that RB-E2F interaction is a key cell-autonomous mechanism responsible for establishment and maintenance of lifelong male fertility. We also review the expression pattern of RB proteins and E2F transcription factors in the testis and male germ cells. The available evidence supports that RB and E2F family members are widely and dynamically expressed in the testis, and they are known to have versatile roles during spermatogenesis. Knowledge of the function and significance of RB-E2F interplay for testicular development and spermatogenesis comes primarily from gene knock-out (KO) studies. Several studies conducted in Sertoli cell-specific pRB-KO mice have demonstrated that pRB-mediated inhibition of E2F3 is essential for Sertoli cell functional maturation and cell cycle exit, highlighting that RB-E2F interaction in Sertoli cells is paramount to male fertility. Similarly, ablation of either pRB or E2F1 in the germline results in progressive testicular atrophy due to germline stem cell (GSC) depletion, emphasizing the importance of proper RB-E2F interplay for germline maintenance and lifelong sperm production. In summary, while balanced RB-E2F interplay is essential for cell-autonomous maintenance of GSCs and, the pRB-E2F3 system in Sertoli cells is critical for providing GSC niche thus laying the basis for spermatogenesis.
RB-E2F Pathway
Strict regulation of the cell cycle is critical during testicular development and steady-state spermatogenesis. The mechanisms that define whether a cell stays in the G1 state or transits to S phase or G0 are ultimately responsible for normal development of any tissue and its function under homeostasis. The G1/S transition is controlled by the interaction between retinoblastoma (RB) tumor suppressor proteins and E2F transcription factors. In G1 RB family proteins form complexes with E2Fs and various chromatin modifiers to repress E2F activity on the promoters of the genes that are needed to enter the S phase. The function of RB is thus growth-inhibitory and misregulation of RB-E2F interplay is one of the hallmarks of cancer (1). The RB-dependent repression on E2F-driven transcription is relieved upon phosphorylation of RB by cyclin-dependent kinases (CDKs) resulting in cellular growth, DNA synthesis and advancement of the cell cycle (Figure 1). It is considered that many different stimuli that affect cell fate decisions are channeled through CDKs to control the phosphorylation status of RB to ultimately control the progress or arrest of the cell cycle at G1 (2).
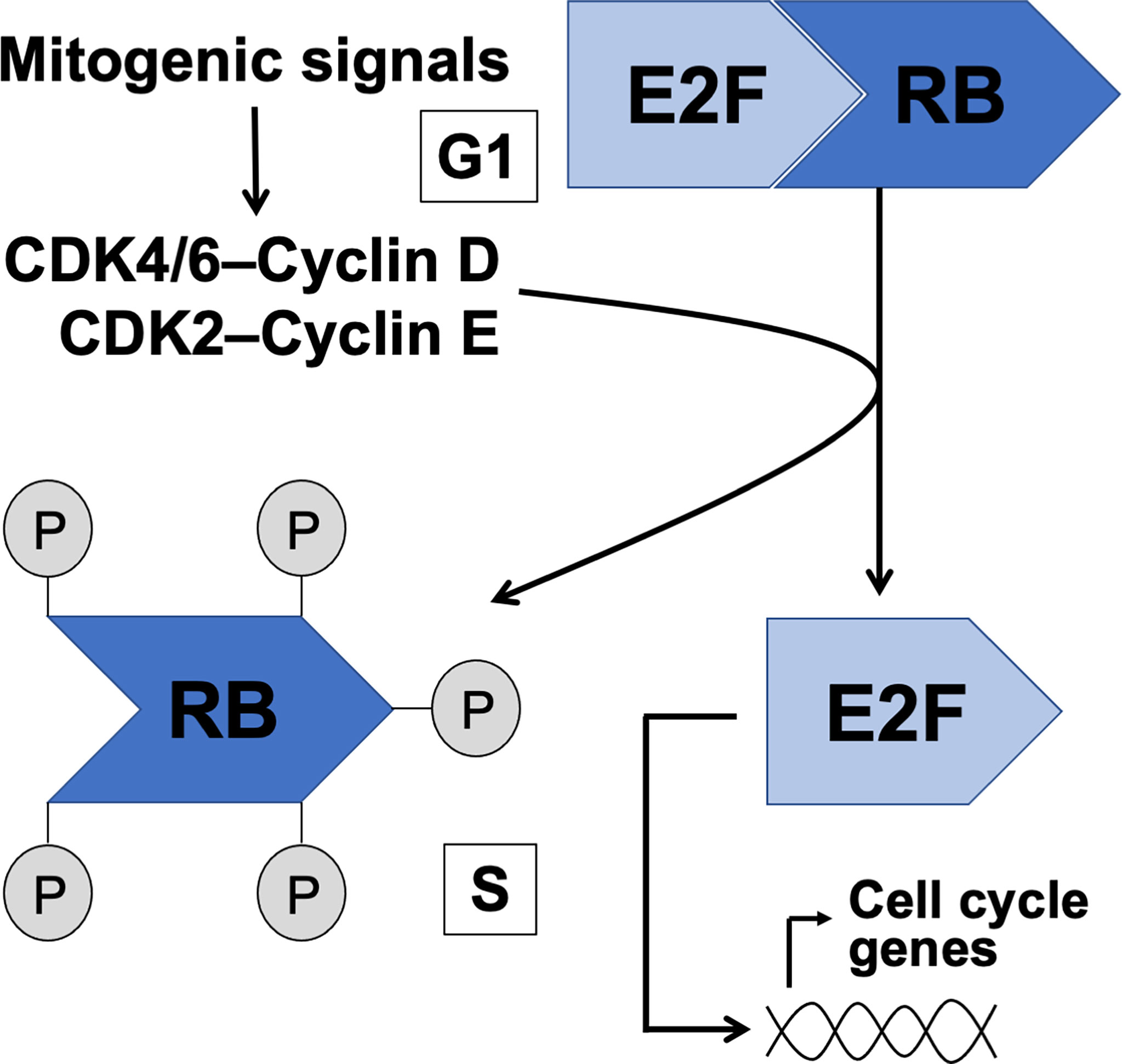
Figure 1 The canonical RB-E2F pathway in control of G1 to S phase transition. Intra- and extracellular mitogenic signals are considered to converge on CDK/cyclin activation resulting in phosphorylation of RB, release of E2F and transcription of S phase genes.
Efficient entry in the S phase depends on numerous phosphorylation events on RB resulting in the dissociation of the RB-E2F complex and activation of E2F transcription factors. While numerous different kinases have a capacity to phosphorylate RB (3), the role of CDK4/6 and CDK2 in G1/S transition is probably the most critical (4). The transcriptional targets of E2F include cyclin E, Cdk1, DNA polymerase-alpha and E2fs themselves (5). While the molecular control of CDK-RB-E2F pathway has been much studied and relatively well understood, the versatility in RB and E2F protein families brings a layer of complexity to the big picture.
The retinoblastoma protein family consists of three proteins (pRB, p107 and p130; also known as RB1, Retinoblastoma-like 1 and Retinoblastoma-like 2 (RBL1-2), respectively) that share significant homology, yet only pRb is found frequently mutated in cancer (6). While some degree of functional redundancy in the RB protein family is apparent - especially between p107 and p130, as observed in mouse knock-out studies - the data support an indispensable role for pRB in development and oncogenesis [reviewed in (7)]. Yet, in a physiological context evaluation of such aspects is often irrelevant due to cell-type dependent expression patterns, as we will later see also in the testis. Thus far eight different E2f genes (E2f1-8) are known, giving rise to nine distinct E2F proteins. E2F1, E2F2 and E2F3A are generally considered as transcriptional activators, whereas E2F3B and E2F4-8 have been assigned transcriptional repressor functions. However, at least in some developmental contexts E2Fs display functional plasticity and may interconvert between repressor and activator functions (8–12). While activator E2Fs are generally considered to function in cell proliferation, repressor E2Fs are involved in cell cycle exit and differentiation (13). However, such clear-cut functional dichotomy in a physiological context barely exists, and the downstream effects of E2F activation are context-dependent. A notable feature in the E2F protein family is functional redundancy, and in many cases two or more E2f genes need to be ablated in order to achieve a phenotypic change (14).
RB family proteins are so called pocket proteins that interact widely with different proteins (>300 proteins have been identified as possible binding partners), not just with E2Fs (15, 16). Within the E2F family they show preferences in terms of interaction partners, and while E2F1-3 predominantly associate with pRB, E2F4-5 preferentially interact with p107 or p130 (7). Conversely, E2F6 is an RB-independent transcriptional repressor and instead forms a complex with Polycomb group proteins (17), and E2F7/8-driven transcriptional repression is also independent from CDK-mediated phosphorylation of RBs (18). Notably, the function of RBs goes beyond gatekeeping G1/S transition and they play critical roles in quiescent, senescent and differentiating cells by maintaining G0/G1. Among E2Fs the interaction of E2F1 with pRB is considered unique and even hyperphosphorylation of pRB does not fully preclude this interaction (19, 20). Conspicuously, E2F1 is also the most extensively studied of the E2F family members and future investigations will be required to elucidate the nuances in tissue-, cell- and context-dependent action of different E2Fs.
In addition to its canonical role in control of the cell cycle and proliferation, the RB-E2F pathway has also been implicated in regulation of heterochromatin and chromosome stability, and apoptosis (2), all of which are very relevant to spermatogenesis. A number of studies have shown that increased E2F activity due to RB loss-of-function has an adverse effect on chromosome stability and causes aneuploidy (2, 21). One of the underlying mechanism may be deregulation of the pericentric heterochromatin, an essential chromosomal region for proper segregation during mitosis and meiosis (22, 23). Considering the role of activator E2Fs in cell cycle progression, it is somewhat counterintuitive that increased E2F activity is also able to induce apoptosis via DNA damage signaling pathways. According to a model proposed by Dick & Rubin (2013) this involves extensive post-transcriptional modifications in both RB and E2F1 allowing expression of pro-apoptotic genes, but repression of E2F-dependent cell cycle genes (2). All these different functional aspects of RB-E2F pathway make it a key regulator of testicular development and physiology, as will be highlighted in this article.
Dynamic Expression of RBs and E2Fs in Rodent Testis
mRNA Expression in Mouse Spermatogenic Cells
Application of single-cell RNA-sequencing technology (scRNA-seq) for the analysis of gene expression during spermatogenesis has provided a powerful tool to better understand its molecular regulation and identify the genes/pathways involved in it in various organisms (24–32). We took advantage of a previously published adult mouse testis scRNA-seq dataset (26) and Loupe Cell Browser v6.0.0 from 10x Genomics to visualize the distribution of RB and E2F family mRNAs along the entire spermatogenic trajectory (Figure 2), or specifically in spermatogonia (Figure 3) and round spermatids (Figure 4). We chose the dataset of Hermann et al. (2018) because it is the first comprehensive scRNA-seq analysis of adult mouse spermatogenic cells distinguishing 11 cell types (26) and enabling feasible analysis of their gene expression signature. For this analysis spermatogonia were further divided into clusters (1-13; Figure 3), with clusters 1, 3, 5 and 10 representing undifferentiated spermatogonia as defined by expression of Gfra1 (33, 34) and Eomes (35, 36), and clusters 2, 3, 6, 8, 11 and 13 representing differentiating spermatogonia with characteristic expression for Kit (37, 38), Stra8 (39, 40) and Sycp3 (41, 42). Round spermatids were divided into early, mid and late steps (Figure 4) based on expression of Speer4e, Catsper1 and Tnp1 (26). Besides the entire spermatogenic trajectory (Figure 2), we decided to focus on GSCs/spermatogonia (Figure 3) because they are the foundation of spermatogenesis, and round spermatids (Figure 4) because p130, E2f4 and E2f5 display intriguing expression patterns in these cells. It should be noted that the data presented in Figures 2–4 are from a previously published dataset (26) and their reliability have not been validated with independent approaches.
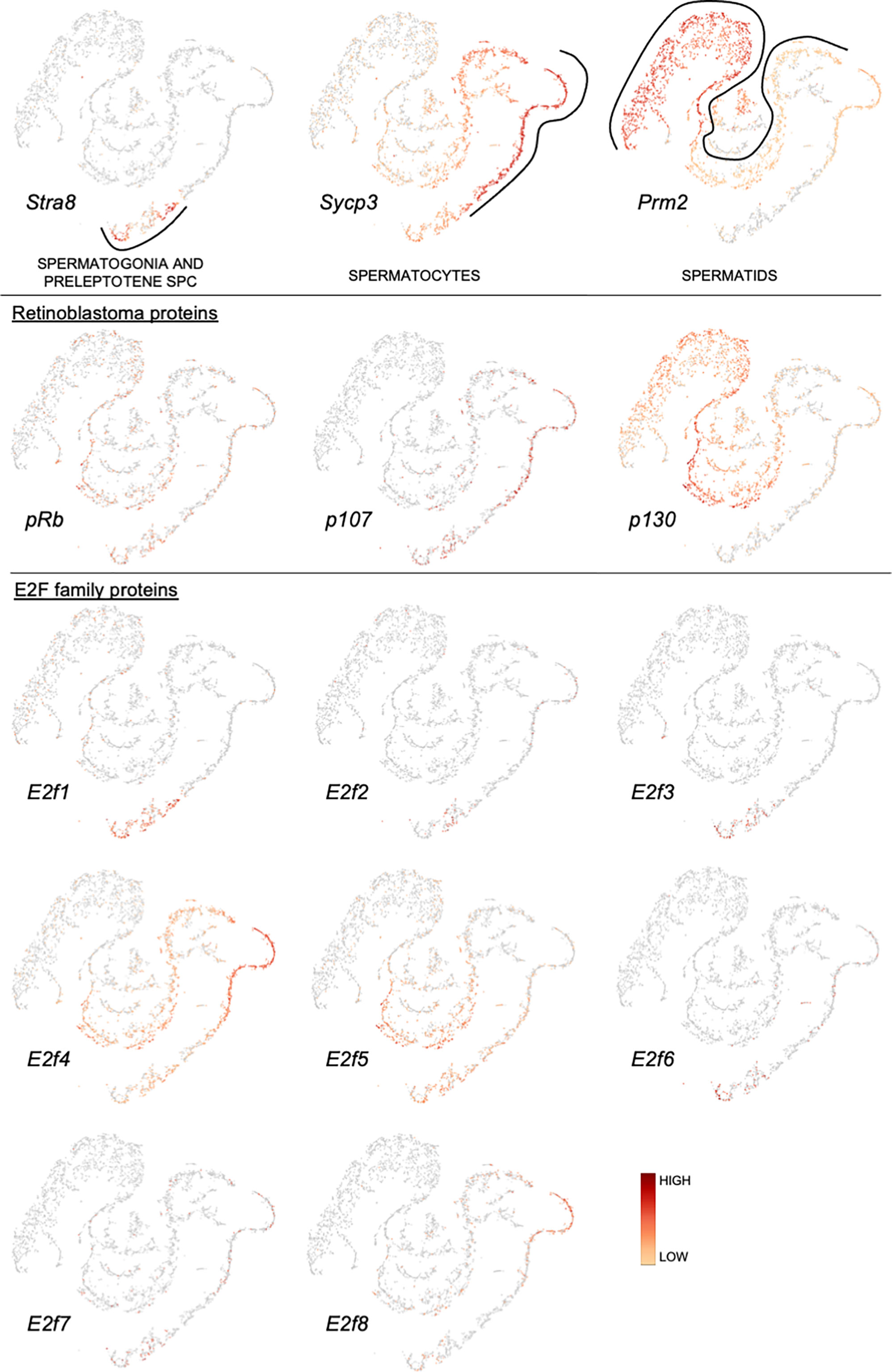
Figure 2 Expression of retinoblastoma protein family and E2F transcription factor family mRNAs along a pseudotime trajectory of adult mouse spermatogenic cells. The data is extracted from Hermann et al. 2018 (26) using Loupe Cell Browser v6.0.0 from 10x Genomics. Stra8, Sycp3 and Prm2 are included to denote spermatogonia/preleptotene spermatocytes, spermatocytes and spermatids, respectively.
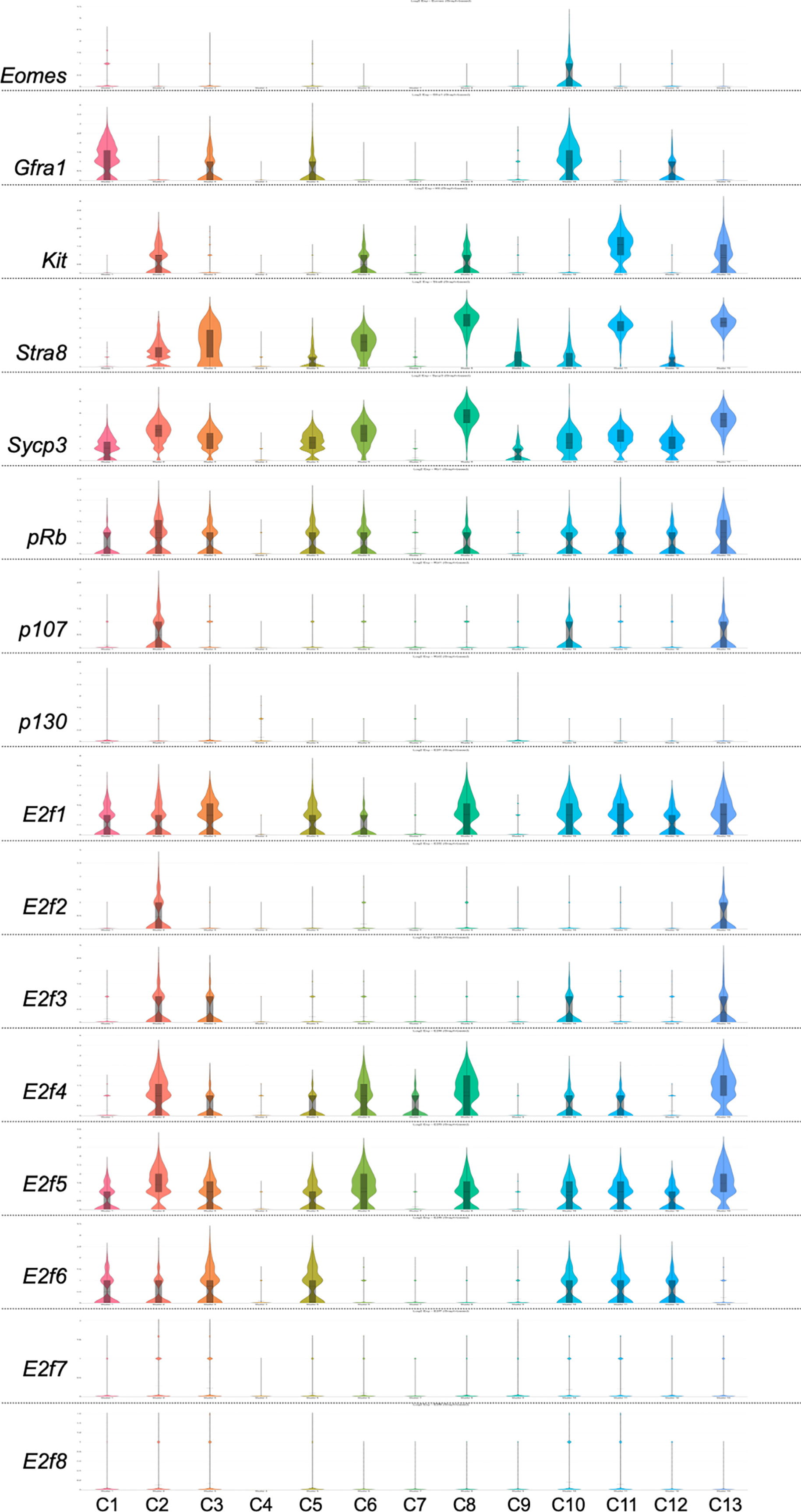
Figure 3 Violin plots displaying the expression of Eomes, Gfra1, Kit, Stra8, Sycp3, pRb, p107, p130 and E2f1-E2f8 in spermatogonial cells of an adult mouse. The data is extracted from Hermann et al. 2018 (26) using Loupe Cell Browser v6.0.0 from 10x Genomics. Spermatogonia are divided into clusters 1-13 (C1-13).
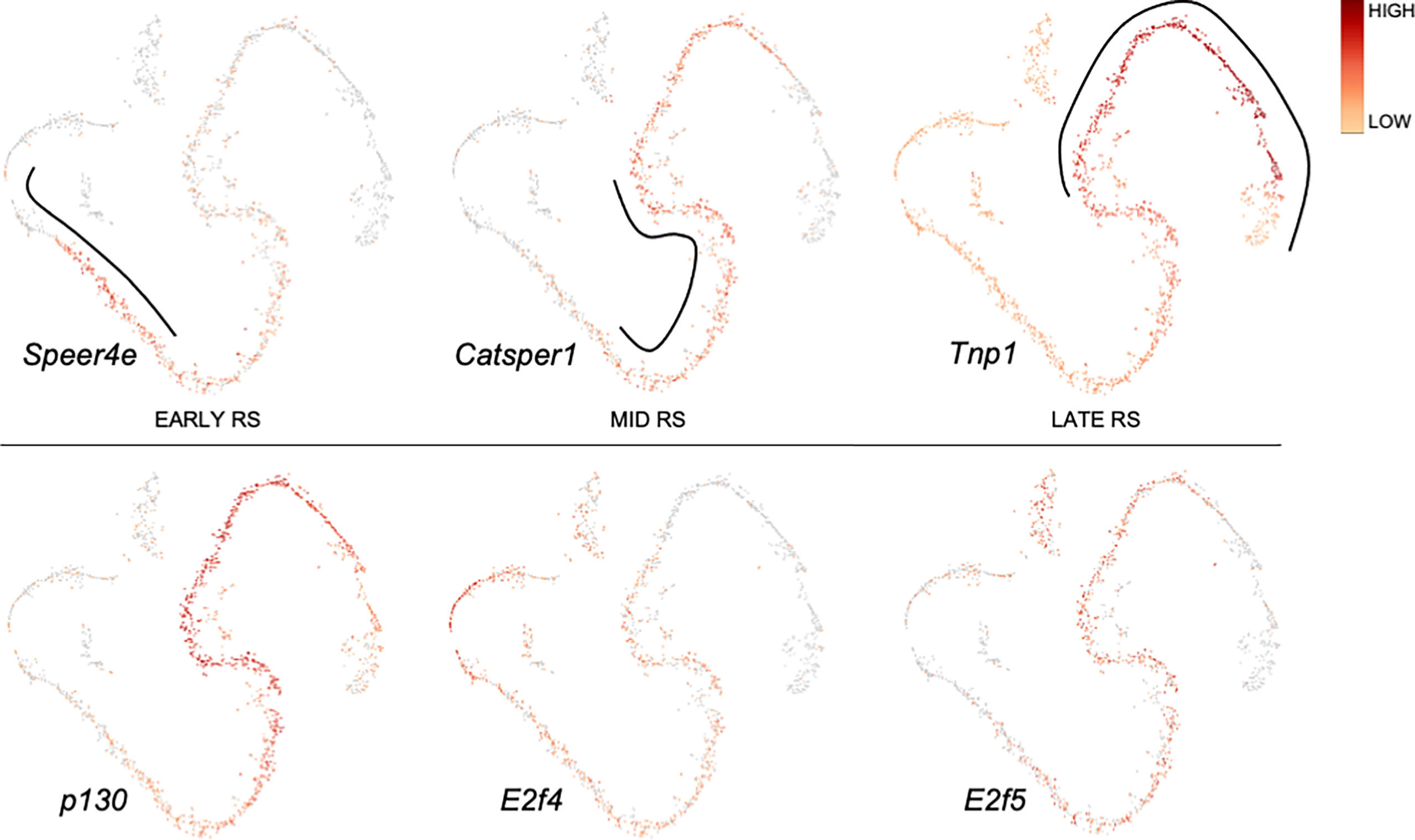
Figure 4 Expression of p130, E2f4 and E2f5 along a pseudotime trajectory of sorted mouse round spermatids. The data is extracted from Hermann et al. 2018 (26) using Loupe Cell Browser v6.0.0 from 10x Genomics. Expression for Speer4e, Catsper1 and Tnp1 are included to demarcate early, mid and late round spermatids, respectively.
pRB (Rb1)
pRB is expressed rather uniformly throughout spermatogenic differentiation (Figure 2), and also in most cell clusters within the spermatogonial compartment (Figure 3).
p107 (Rbl1)
p107 is expressed in spermatocytes, and to a lesser extent in early spermatids (Figure 2) and spermatogonia (Figure 3).
p130 (Rbl2)
p130 is expressed in spermatids (Figure 2) with the highest levels in mid-to-late round spermatids (Figure 4).
E2f1
E2f1 is expressed in spermatogonia and early spermatocytes (Figure 2), which is also supported by RNA in situ results (43). E2f1 expression is rather uniform in all spermatogonial clusters (Figure 3) from GSCs (Eomes/Gfra1+; clusters 1 and 10) to differentiating spermatogonia (Kit/Sycp3/Stra8+; clusters 2, 3, 6, 8, 11 and 13).
E2f2
There are very few cells expressing E2f2 along the spermatogenic trajectory (Figure 2).
E2f3
E2f3 is expressed in a limited fashion in spermatogonia and preleptotene spermatocytes (Figure 2).
E2f4
E2f4 is expressed in spermatogonia, spermatocytes and round spermatids (Figure 2). In spermatogonia (Figure 3) the highest E2f4 levels are seen in clusters 2, 6, 8 and 13 (Kit/Sycp3/Stra8+) corresponding to differentiating spermatogonia. In round spermatids E2f4 levels are highest in early and mid steps (Figure 4).
E2f5
E2f5 closely follows the expression pattern of E2f4 but is clearly less expressed in spermatocytes and early round spermatids (Figure 2). Within the round spermatid population the highest levels are seen in mid to late steps (Figure 4). Expression in spermatogonial clusters is rather uniform but with highest levels in differentiating spermatogonia (Figure 3).
E2f6
E2f6 expression is restricted to spermatogonia and only sporadically detected elsewhere (Figure 2). Within the spermatogonial compartment E2f6 is enriched in clusters that are also positive for Gfra1 (1, 3, 5 and 10) and thus potential GSCs (Figure 3).
E2f7-8
Spermatogenic expression for E2f7 and E2f8 is low. Late spermatocytes and/or early round spermatids show limited E2f8 expression (Figure 2).
Protein Expression in Rodent Testis
The protein expression of all RB and most E2F family members have been studied in a handful of articles. The expression pattern for each protein is summarized in Figure 5.
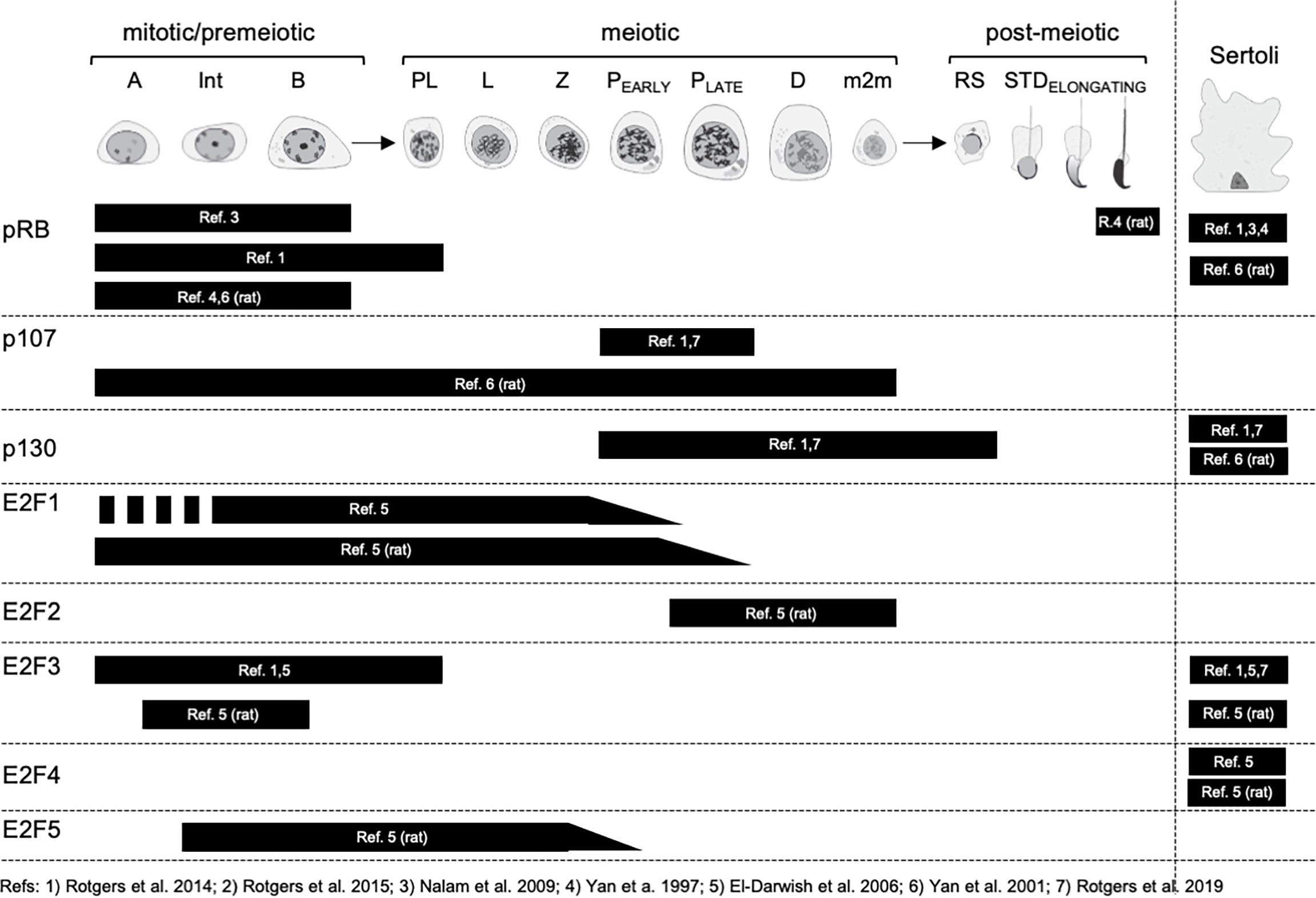
Figure 5 Expression of RB and E2F family proteins in spermatogenic cells and Sertoli cells. Mitotic/premeiotic germ cells are divided into type A (A), type Intermediate (Int) and type B (B) spermatogonia. Meiotic germ cells are presented as preleptotene (Pl), leptotene (L), zygotene (Z), early and late pachytene (P) and diplotene (D) spermatocytes, and secondary spermatocytes, m2m. Post-meiotic germ cells are round spermatids (RS) and elongating spermatids (STD).
pRB (RB1)
In the mouse testis Nalam et al. (2009) reported the staining of pRB in Sertoli cells and spermatogonia (44), while Rotgers et al. (2014) observed the same but extended the staining to preleptotene spermatocytes (45). In the rat testis Yan et al. (2001) confirmed pRB staining in Sertoli cells and spermatogonia but also showed that the highest levels of Sertoli cell pRB in seminiferous epithelial stages VII-VIII (46). The protein expression of pRB in spermatogenic cells is to some extent in conflict with mRNA level findings (Figure 2), and suggests that pRB mRNA is not translated to protein in post-mitotic male germ cells.
p107 (RBL1)
Rotgers et al. (2014 & 2019) reported p107 staining solely in pachytene spermatocytes in adult WT mouse (45), which is in agreement with single-cell RNA-sequencing data (Figure 2). In the rat testis p107 was localized to spermatogonia and spermatocytes (46).
p130 (RBL2)
In the mouse testis Sertoli cells were found positive for p130 (45, 47). Interestingly, the authors did not mention a weak staining for p130 in spermatocytes and round spermatids that would also be supported by enrichment of p130 mRNA in these germ cells (Figure 2). In the rat testis p130 protein expression is seen in Sertoli cells, Leydig cells and peritubular myoid cells, and excluded from differentiating germ cells (46).
E2F1
The expression of E2F1 in the mouse testis has been reported only in one study. El-Darwish et al. (2006) showed the expression in Intermediate to type B spermatogonia and in leptotene to early pachytene spermatocytes (48). The highest levels of E2F1 were observed in stages IX-XI, i.e leptotene and zygotene spermatocytes. These findings align with mRNA expression analysis, although leave the protein expression in type A spermatogonia elusive. Based on functional analyses (discussed later in the text) it is probable that E2F1 is expressed at the protein level already in type A spermatogonia. In the rat testis the E2F1 staining differs slightly with type A spermatogonia already expressing it, and the expression extending to mid-to-late pachytene spermatocytes (48).
E2F2
El-Darwish et al. (2006) also studied the localization of E2F2 in rodent testis. In the rat testis E2F2 is most highly expressed in pachytene and diplotene spermatocytes of stages VII-XIII, but also in secondary spermatocytes (48). In the mouse the results show abundant E2F2 staining in nearly all mouse testicular cell types, excluding condensing spermatids. Compared to the very restricted pattern of expression in the rat and the scanty mRNA expression in mouse (Figure 2) the results concerning the staining in the mouse would require further investigation and validation.
E2F3
The data concerning E2F3 expression in the mouse testis is contradictory. While the expression in Sertoli cells is supported by all available data (45, 47, 48), germ cell expression remains somewhat elusive. While El-Darwish et al. (2006) and Rotgers et al. (2014) reported E2F3 staining in spermatogonia and preleptotene spermatocytes (45, 48), which is compatible with mRNA expression (Figure 2), Rotgers et al. (2019) did not confirm spermatogonial staining with E2F3a-specific or pan-E2F3 antibodies in the adult mouse testis (47). Isoform unspecific E2F3 antibody, however, did stain spermatogonia in the juvenile testis. In the adult rat testis E2F3 expression was seen in Sertoli cells and spermatogonia (48).
E2F4
Strong E2F4 staining is seen in the nuclei of testicular somatic cells: Sertoli cells, Leydig cells and peritubular myoid cells both in mouse and rat (48). The authors speculate though, that only the Sertoli cell staining is specific because parallel staining of E2f4 KO mouse testis sections show that only Sertoli staining was absent. Interestingly, there is a granular staining for E2F4 from spermatocytes to elongated spermatids both in mouse and rat.
E2F5
E2F5 is expressed from type Intermediate spermatogonia to mid-Pachytene spermatocytes in the rat testis (48). To our knowledge E2F5 localization in the mouse testis has not been studied.
As far as we know there are no studies where the localization of E2F6, E2F7 or E2F8 in rodent testis would have been studied.
Functional Consequences of RB-E2F Pathway Loss-of-Function in the Testis
pRB Is Essential for Establishment and Maintenance of GSCs
The fact that pRB-null mice are embryonically lethal (49–51) has complicated the study of pRB's tissue-specific functions. In the testicular context, however, this limitation has been circumvented by creation of cell type-specific conditional KO mice (44, 45, 52–54) and ex vivo culture (55). Of note, p107- and p130-deficient mice are viable, healthy and fertile (56, 57). We will first focus on the effects of germ cell-specific ablation of pRB and then summarize the role of pRB in Sertoli cells. The available literature indicates that pRB is indispensable for formation and maintenance of germline stem cells (GSCs). Lifelong ability of sperm production depends on GSCs, whose cell fate decisions need to be tightly balanced in order to maintain high and continuous production of sperm throughout the reproductive life span. In theory, GSCs must undergo both self-renewal divisions to sustain the GSC pool and differentiation divisions to give rise to transit-amplifying progenitor spermatogonia that are destined to complete spermatogenesis. Mechanistically it is not entirely clear to what extent these cell fate decisions are cell-autonomous and how/when the molecular cues in the GSC niche microenvironment are incorporated into the regulation of these events. The stem cell capacity in the mouse testis is thought to reside in a subset of undifferentiated spermatogonia (Aundiff), although in regenerative conditions Aundiff display functional plasticity suggesting that the number of potential stem cells markedly exceeds that of steady-state stem cells (58, 59).
Given its role as the gatekeeper of G1/S transition, or quiescence vs. proliferation, pRB is understandably a critical regulator of GSCs. The evidence for this comes from various mouse models where pRB has been conditionally deleted from germ cells at different ages: prenatally from primordial germ cells (pRB-KOBlimp1) (53) or ED15.5-17 gonocytes (pRB-KODdx4) (52, 54), and postnatally from progenitor-Aundiff (pRB-KONgn3) (54) and progenitor/differentiating spermatogonia (pRB-KOStra8) (54). Contrary to its role in somatic stem cells where inactivation of RB family proteins often results in stem cell expansion, increased apoptosis, altered cell fate/differentiation defects, and initiation of cancer (60), pRB-deficient male GSCs have been reported to lose their capacity to self-renew, possibly explaining why no testicular tumors were observed (52, 54). Notably, the differentiation capacity of pRB-null germ cells was not affected but young adult males (until 2-3 months of age) were able to sire offspring. However, due to gradual depletion of GSCs they became infertile with age (52, 54).
Despite the fact that pRB is expressed very early in the germ lineage, its absence does not seem to have any effects on fetal germ cells, termed gonocytes (or prospermatogonia), before ED14.5 (53, 55) which is the time when WT gonocytes start to enter mitotic quiescence (61). Recently, Du et al. (2021) discovered that at ED16.5, when practically all control gonocytes had stopped proliferating, the majority of pRB-KOBlimp1 were still engaged in the cell cycle resulting in two-fold higher number of germ cells at the same time point. Subsequently, there is a massive wave of apoptosis in pRB-KOBlimp1 testes that ablates the germline by the time of onset of the first round of spermatogenesis (PND3.5-6.5) (53). Interestingly, in this time window the classical effects of pRB-deficiency are also recapitulated in the germline: mitotic overexpansion followed by increased apoptosis. It is not exactly clear what induces programmed cell death in practically all pRB-KOBlimp1 germ cells perinatally, but there are a number of factors that might contribute to it, including a failure of pRB-KOBlimp1 gonocytes to inhibit the onset of meiosis, a demarcating feature of all fetal male germ cells (53). It has been well documented that the gonocyte population experiences a wave of apoptosis between ED13.5 and ED17.5 (62, 63), which is considered to eradicate genetically or epigenetically defective male germ cells that are developmentally incompetent (64). Considering that the cellular functions of pRB are not limited to cell cycle regulation, it is likely that the reason for germline ablation in pRB-KOBlimp1 mice is due to failure of pRB-deficient gonocytes to pass this developmental quality control check-point.
Perinatal testicular development in pRB-KODdx4 mice (pRB deleted in ED15.5-17 gonocytes) is apparently normal although the number of spermatogonia in the pre/peripubertal testis is higher than in control mice (52). Despite a previously established role for pRB in control of gonocyte cell cycle exit before birth (55), this is not due to extended proliferation of gonocytes and pRB-KODdx4 mice are born with a normal number of germ cells (52), suggesting that conditional deletion of pRB at the time of gonocyte quiescence, does not disrupt this state. Notably, and in contrast to pRB-KOBlimp1 mice, the GSC pool is formed in pRB-KODdx4 mice because GFRa1-positive (GDNF family receptor alpha 1) cells are observed at the basement membrane of the seminiferous epithelium (52). These GSCs, however, show very limited, if any, self-renewal capacity (52, 54). Although it has not been studied whether the GSC niche forms properly in pRB-KODdx4 mice, it is conceivable that inability to self-renew is likely GSC-intrinsic rather than due to extrinsic factors, such as lack of GDNF (glial cell line-derived neurotrophic factor) (36, 65). Hu et al. (2013) also report that pRB-KODdx4 GSC exit from self-renewal is followed by expansion of progenitor-Aundiff population (52). This is supported by increased density of spermatogonia in peripubertal mice but suffers some limitations because the authors use PLZF (promyelocytic leukemia zinc finger) as a marker of Aundiff, while PLZF is also expressed in differentiating spermatogonia (66, 67). Nonetheless, these data support that pRB regulates the timely and stage-dependent cell cycle entry and exit in spermatogonia.
If pRB is ablated in progenitor-Aundiff or differentiating spermatogonia, as in pRB-KONgn3 and pRB-KOStra8 mice, there are no effects on male fertility, but the testes of respective mice are often cystic and have areas that are devoid of spermatogenic cells, and sometimes, as Yang et al. (2013) report, house cells with neoplastic features (54). These results suggest that the consequences of pRB-deficiency in differentiation-committed and differentiating germ cells and all subsequent spermatogenic cell types are significant but possibly compensated by other RB family members, as also suggested by Figure 2, given the continuation of qualitatively normal spermatogenesis in adult pRB-KONgn3 and pRB-KOStra8 mice.
To summarize, the spermatogenic phenotype that germ cell-specific pRB deletion inflicts highly depends on the developmental stage where the deletion has been induced. While pRB-KOBlimp1 mice are sterile, pRB-KODdx4 mice undergo 1-2 rounds of spermatogenesis, and both pRB-KONgn3 and pRB-KOStra8 mice display qualitatively normal spermatogenesis and are fertile (52–54). These data demonstrate that pRB is a critical regulator of formation and maintenance of GSCs but dispensable for spermatogenic differentiation of their progeny.
RB-E2F3 in Sertoli Cell Maturation
In addition to germ cells, the developmental and functional consequences of pRB ablation have also been studied in Sertoli cells (44, 45). Sertoli cells are the only somatic cell type inside the seminiferous tubules and they are paramount to testicular development, function and spermatogenesis (68). It is considered that the number of Sertoli cells ultimately defines sperm production capacity because each Sertoli cell is able to support a finite number of spermatogenic cells (69). Therefore, any factors that impinge on Sertoli cell proliferation or apoptosis are a potential threat to male fertility. Sertoli cells are specified in the mouse XY gonad following the expression Sry (sex-determining region Y) and its most significant downstream target gene Sox9 (SRY-box 9) at ED10.5-11 (61). Sertoli cells then coordinate the differentiation of all other testicular cell types, including the germ cells. Sertoli cells undergo maturation in pubertal testis involving polarization, formation of the blood-testis barrier, a profound change in the transcriptome/proteome and exit from the cell cycle. Notably, Sertoli cells of an adult mouse do not proliferate but are in a seemingly terminally-differentiated state. However, under specific in vitro circumstances they are able to resume the cell cycle (70, 71). As discussed in detail below, similar to many other somatic cells, but not germ cells, Sertoli cells fail to enter a functionally mature mitotically-quiescent state if lacking pRB (44, 45, 52, 60).
The consequences of Sertoli cell-specific ablation of pRB have been explored in three studies, all of which relied on Cre expression under Anti-Müllerian hormone promoter (pRB-KOAmh) resulting in pRB loss of function from ED14.5 (44, 45, 47). The studies show congruent results: pRB-deficiency leads to rapid testicular atrophy and male infertility. However, while the gene expression is misregulated at least as early as in PND10 testis, testicular development and onset of spermatogenesis in juvenile mice appear unaffected in pRB-KOAmh mice, and until 6-weeks of age there are no obvious changes in testicular histology (44, 45). Subsequently, the changes in the phenotype are fast and the male mice become infertile by early adulthood. The testicular phenotype of pRB-KOAmh mice also includes Sertoli cell vacuolization, sloughing of Sertoli cells and immature germ cells from the seminiferous epithelium, increased germ cell apoptosis and Leydig cell hyperplasia (44, 45, 47). Interestingly, this might present an evolutionarily conserved function of RB proteins given a comparable phenotype in Drosophila following somatic deletion of Rbf (a pRB homolog) (72, 73).
There are a few remarkable changes in pRB-ablated Sertoli cell physiology that have a common nominator: inability to mature functionally. First of all, while WT Sertoli cells exit the cell cycle at around PND15, pRB-KOAmh Sertoli cells continue to proliferate at a constant rate at least until PND30 (45). This is followed by increased apoptosis probably due to activation of p53 pathway, thus precluding tumor formation (44). While the likely reason for spermatogenic failure in these mice is complex and multifactorial [loss of BTB (blood-testis barrier) integrity, apoptosis of pRB-deficient Sertoli cells to nurture and maintain spermatogenic cells etc.] it has not been carefully studied at which step spermatogenesis fails and whether functional GSCs were sustained in adult pRB-KOAmh mice. Considering the critical cell-autonomous role of pRB in GSCs, as described above, this is an interesting question and would deserve further investigation. Given the timeline of male infertility in these mice [2-3 months (44) and 3-6 months (45)] it is conceivable that GSCs are able to undergo at least some rounds of self-renewal in these conditions. This is also supported by qPCR data showing reduced but measurable levels of GSC-associated transcripts: Gfra1 and Lin28 (47). However, Pou5f1 (Oct4) expression is lost in pRB-KOAmh testis suggesting a loss of progenitor-Aundiff, where Pou5f1 expression is enriched (36).
Interestingly, according to Rotgers et al. (2014) the BTB first organizes normally in 6-week old pRB-KOAmh mice but then disintegrates (45). However, the data from Nalam et al. (2009) indicate that the BTB is not functional even at this early time point and allows the leakage of a tracer from the interstitium to the adluminal compartment of seminiferous tubules, an immune-privileged site in normal testis (44). These findings suggest that pRB is not developmentally needed for formation of the BTB but is indispensable for its integrity and maintenance, which is another sign of Sertoli cell dedifferentiation and immaturity in pRB-KOAmh mice. Interestingly, Sertoli cells deficient for pRB display seminiferous epithelial cyclic activity, as judged by fluctuation of androgen receptor (AR) staining intensity between seminiferous tubule cross-sections (47). AR is one of the proteins that are known to be expressed in Sertoli cells in a seminiferous epithelial stage-dependent manner with the highest levels in early-to-mid stages and lowest levels in late stages (74, 75).
In WT testis pRB interacts with E2F3, the only activator E2F that is expressed in Sertoli cells (45). E2f3 gene encodes two isoforms, E2f3a and E2f3b, both of which are expressed in the mouse testis. Interestingly, while Sertoli cells also express p130, Rotgers et al. (2014) do not confirm an interaction between E2F3 and p130 in WT testis. In pRB-KOAmh testis, however, E2F3 is found to form a complex with both p130 and p107, whose expression is induced in pRB-deficient Sertoli cells (45). This is in agreement with previous findings showing that when pRB is absent p107 and p130 also bind to activator E2Fs (E2F1-3), not just the repressors (E2F4-5) (76). While these findings imply that there might be a compensatory mechanism, the severity of the phenotype indicates that such a mechanism does not have any functional relevance. Already in their original paper Rotgers et al. (2014) provide data showing that impairment of spermatogenesis due to pRB-deficiency is attenuated by simultaneous silencing of E2f3 in vivo (45). These results were corroborated by a follow-up study where pRB-E2f3 compound-KOAmh mice were created (47). These mice display full spermatogenesis but interestingly the litter size is markedly reduced when compared to control. This might be due to disturbance of non-canonical, i.e. non-E2F-mediated, pRB functions in compound-KOAmh mice, including chromatin organization and nuclear architecture which are pronouncedly relevant in the spermatogenic context (77, 78). While spermatogenesis is qualitatively normal in pRB-KOAmh/E2f3-haploinsufficient mice, they display age-dependent testicular atrophy, and continuously cycling Sertoli cells, suggesting that E2f3 gene dosage is critical for spermatogenesis. Notably, adult Sertoli cells of pRB-E2f3 compound-KOAmh mice are non-proliferative and seem to be functionally comparable to their WT counterparts (47). In summary, these data show that spermatogenic failure, prolonged proliferation, increased apoptosis and dedifferentiation of Sertoli cells are mediated by adverse and non-restricted action of E2F3 in Sertoli cells of pRB-KOAmh mice, and highlights the role of pRB as a key regulator in life-long maintenance of the non-renewable Sertoli cell population.
Considering the spermatogenic rescue experiments by simultaneous deletion (47) or shRNA-mediated in vivo knock-down of E2f3 (45) on a pRB-KOAmh background, it is surprising that E2f3-KOAmh mice, where E2f3 has been deleted from fetal Sertoli cells, are fertile and show no signs of impaired spermatogenesis (47). The authors hypothesize that E2F4 might fully compensate for lack of E2F3 in these mice. While this might be possible there are, however, at least two obvious pitfalls in this interpretation. Firstly, E2F4 is considered a transcriptional repressor, whereas E2F3a isoform is classically considered a transcriptional activator. Secondly, E2F4 primarily associates with p107 and p130, not with pRB. Thus, further investigations are warranted to address this question. Results from these studies further support the concept that Sertoli cells are not terminally-differentiated but in a state of continuous cell cycle repression (79), and show that pRB is responsible for maintaining this non-proliferative state. These conclusions, however, suffer some limitations because pRB is ablated already in fetal Sertoli cells. An inducible pRB-deletion in adult Sertoli cells would be required to further elucidate this question. The developmental roles of pRB in the testis are summarized in Figure 6.
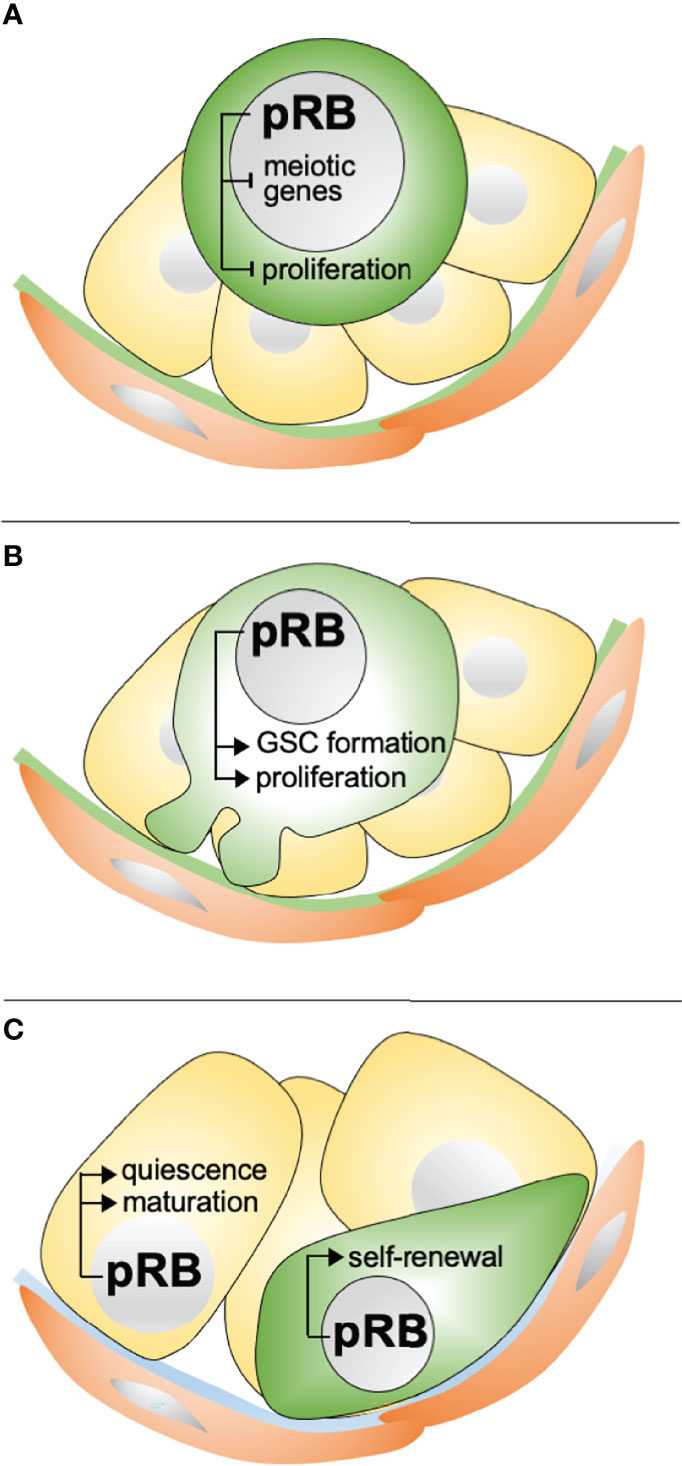
Figure 6 Known developmental roles of pRB in the testis. (A) In ED14.5-16.5 gonocytes, pRB is needed for cessation of proliferation and entry into a mitotically quiescent state. pRB likely also inhibits the expression of meiotic genes. (B) In the perinatal testis, pRB is needed for cell cycle re-entry and formation of the GSC pool. (C) In pubertal and adult testis, pRB is required for functional maturation of Sertoli cells, including their exit from the cell cycle, and self-renewal of GSCs.
pRB Is Not Involved in Testicular Tumorigenesis
To our knowledge, there are only very limited data available supporting a relationship between pRB-deficiency and increased tumorigenesis in the testis. Targeted deletion of pRB from prenatal germ cells results in germline ablation and infertility (44, 53, 54), and focal GCNIS-like (germ cell neoplasia in situ) like cells are only observed when pRB is deleted from progenitors and/or differentiating spermatogonia, arguably due to inability to commit to the spermatogenic lineage (54), an interpretation that might have some merit given that progenitor-Aundiff are enriched for pluripotency-associated transcripts (36). Ablation of pRB in Sertoli cells leads to continued engagement in the cell cycle but also increased apoptosis thus precluding tumor formation (44). Interestingly, Nalam et al. (2010) also studied the compound effects of pRB ablation on an inhibin-α-knockout (Inha KO) background (80). Inha KO mice are known to develop gonadotropin-dependent gonadal stromal tumors, which originate from Sertoli cells (81). Unexpectedly, the double-KO mice did not display exacerbation of the tumorigenic phenotype but Sertoli cell dysfunction took place earlier in these mice when compared to pRB-KOAmh mice (80). In summary, there are no strong indications that pRB would have tumor suppressor properties in the mouse testis.
E2F1 Has a Multifaceted Role in the Testis
Similar to other tissues and cellular contexts, E2F1's role in the testis has deserved most attention within the E2F family. E2F1 transcription factor is a critical regulator of the cell cycle, and a direct target of pRB. However, like all activator E2Fs, E2F1 in particular displays intriguing functional dichotomy and is able to induce both proliferation and apoptosis (13). This is apparent also in the testis. E2F1 has been associated with progressive degeneration of the seminiferous epithelium (43, 82–84), GSC maintenance (43, 84), apoptosis of germ cells (43, 85) and Sertoli cells (44), germ cell neoplasia in situ (86), testicular tumors (82), testicular descent in human (87, 88) and mouse (84), and human male infertility (87, 88).
Our understanding of E2F1's testicular function comes primarily from mouse work and use of E2f1tm1Meg mouse strain (89) (referred to E2f1-null hereafter). E2f1-null mice display characteristics of progressive testicular atrophy that is manifested in a background strain-dependent manner implying that genetic background of the mice has an impact on the time course and severity of the phenotype (43, 82–84, 89). Interestingly, on C57Bl/6 background the first signs of spermatogenic impairment can be observed as early as 20 days of age when the number of meiotic cells is reduced in E2f1-null mice when compared to control (43). E2F1 is an early regulator of the spermatogonial compartment and likely promotes spermatogonial apoptosis during the first wave of spermatogenesis (43). Somewhat counterintuitively, loss of E2F1 does not result in accumulation of spermatogonia during steady-state spermatogenesis but their gradual depletion and occurrence of tubules with Sertoli-cell-only phenotype (43, 82, 84). While the underlying mechanisms are not fully understood it is likely that GSCs in E2f1-null mice are lost via loss of self-renewal capacity and escape to differentiation. The phenotype thus shares remarkable but limited resemblance to pRB-KODdx4 mice (52). While E2f1-null males remain fertile for at least 5-6 months (43, 82, 84), pRB-KODdx4 mice become infertile by 2-3 months (52, 54). This is a significant difference because it shows that GSCs are able to self-renew in E2f1-null but not in pRB-KODdx4 mice.
While the exact explanation for the discrepancy in time course is yet to be discovered, there are a couple of likely explanations: compensation by other E2F activators and non-E2F-mediated effects of pRB (discussed above). Notably, apart from E2f1 no other E2fs were differentially expressed in E2f1-null testis casting doubts for compensation at the transcriptional level (43), yet leaving the door open for post-transcriptional mechanisms. Interestingly, when human E2F1 is overexpressed in the mouse testis, increased germ cell apoptosis is observed, accompanied with accumulation of undifferentiated spermatogonia (85), thus parallelling GDNF or NANOS2 overexpression phenotype (65, 90). Together these data demonstrate that E2F1 is a critical regulator of GSC maintenance and germ cell apoptosis. Although the definitive data are missing, as how E2F1 might be involved in GSC fate decisions, the literature provides some clues about the potential mechanisms. Transcriptomic changes in E2f1-null testis have been studied at 20 days of age by Rotgers et al. (2015) (43), and 3 and 7 months of age by Jorgez et al. (2021) (84). However, choice of the time point and method of study (microarray vs. qPCR) makes the results of these studies hard to compare. While the histological differences in a 20-day-old E2f1-null and WT mouse testis are relatively small making comparison between the genotypes feasible at this time point, the transcriptome at this age is different from adult testis with full spermatogenesis which complicates the comparison between the time points. Then again, the cellularity already in a 3-month-old and particularly in a 7-month-old E2f1-null testis is dramatically different from WT testis thereby complicating the analysis. For these reasons we will look at the findings separately.
Rotgers et al. (2015) identify a couple of E2F1 candidate target genes in a 20-day old mouse testis that, based on available studies, might be relevant for the development of the phenotype: Cnot1 (CCR4-NOT Transcription Complex Subunit 1) and Chd1 (Chromodomain Helicase DNA Binding Protein 1) both of which are downregulated in E2f1-null testes (43). CNOT1 is particularly interesting because it directly interacts with NANOS2, a key intrinsic regulator of the male germline (90, 91). NANOS2 is involved in both degradation and sequestration of specific mRNA molecules in ribonucleoprotein complexes, and its loss results in GSC depletion and rapid germline ablation (59, 90–93). CNOT1 mediates NANOS2 interaction with CCR4-NOT, a major cytoplasmic deadenylase that primes mRNAs for degradation (91, 93). Importantly, CCR4-NOT–CNOT1–NANOS2 interaction is critical for NANOS2 function and maintenance of the male germline (91), a plausible mechanism also explaining GSC depletion in E2f1-null mice. Another interesting E2F1-candidate gene discovered by the microarray analysis is Chd1. While its role in spermatogenesis has not been studied, two of its family members CHD4 and CHD5 are critical for sperm production, albeit at the opposite ends of this complex process. CHD4 has been shown to be highly expressed in GSCs where its involved in their maintenance and self-renewal (94), whereas CDH5 is required for spermiogenesis and especially for chromatin condensation in elongating spermatids (95, 96).
Jorgez et al. (2021) studied the expression of select 66 mRNAs by qPCR and subsequent proteomic analyses (84). They found changes in the expression of cyclin genes and other E2F1 cell cycle targets. Interestingly, Wnt signaling pathway genes were differentially expressed between the genotypes, and all studied Wnt ligands were found generally upregulated, especially WNT4. Wnt signaling has been thought to prime GSCs for differentiation and promote exit from the self-renewing state (97–101). Boyer et al. (2012) reported that treatment of cultured GSCs with WNT4 reduces their stemness both in vitro and after transplantation (100). These data would support a model where GSCs in E2f1-null testis are subjected to differentiation-priming environment at the expense of self-renewal, thus resulting in their depletion over time. To explore this possibility Jorgez et al. (2021) generated a compound E2f1-null/Wnt4-null mouse line, where Wnt4 was conditionally deleted from Stra8-expressing germ cells, that is progenitor-Aundiff/differentiating spermatogonia. Remarkably, the spermatogenic capacity is qualitatively restored in these mice and they display nearly normal fertility parameters, demonstrating that many of the adverse effects of E2F1-deficiency can be overcome by simultaneous removal of WNT4. While the above findings provide a mechanism for GSC loss in E2f1-null mice, further studies are needed to address how the RB-E2F pathway is integrated in the control of GSC fate in WT mice, and how is it mechanistically linked with GDNF signaling (36, 65, 102) and mTOR (mammalian target of rapamycin) pathway (67, 103, 104) to balance self-renewal and differentiation of GSCs.
A typical feature of spermatogenic impairment when E2F1 is either deleted or over-expressed is spermatocyte apoptosis (43, 82, 85). While this leads to subfertility, E2f1-null male mice are able to sire viable offspring because some spermatocytes manage to avoid cell death. The reason for E2f1-null spermatocyte apoptosis is not known but there is data to support that DNA damage is not the underlying cause (43). Further studies are needed to unveil what induces spermatocyte apoptosis if E2F1 is either absent or overexpressed. Considering the expression pattern of E2F1 (highly expressed in premeiotic cells and early spermatocytes; Figures 2, 5) and its role as a transcriptional activator, it is conceivable that the expression of E2F1-target genes needs to be delicately balanced for successful meiosis. It is likely that spermatocyte apoptosis is induced in a cell-intrinsic manner, also considering the fact that despite being expressed in peritubular cells and in the testicular interstitium, E2F1-deficiency was not found to affect testicular somatic cells or gonadotropin or androgen levels (43, 84). However, albeit not known to express E2F1, the Sertoli cells of E2f1-null mice display some transcriptomic changes when compared to controls. Surprisingly, while some of the changes may be explained by a change in cellularity in E2f1-null vs. control mice testis (43), misregulation of genes involved in tight and adherens junctions were shown to have functional consequences and the blood-testis barrier of 3-month-old E2f1-null mice was found leaky (84).
Table 1 provides a summary of the spermatogenic phenotypes of different KO mouse strains where one or two genes encoding proteins of RB-E2F pathway has been deleted.
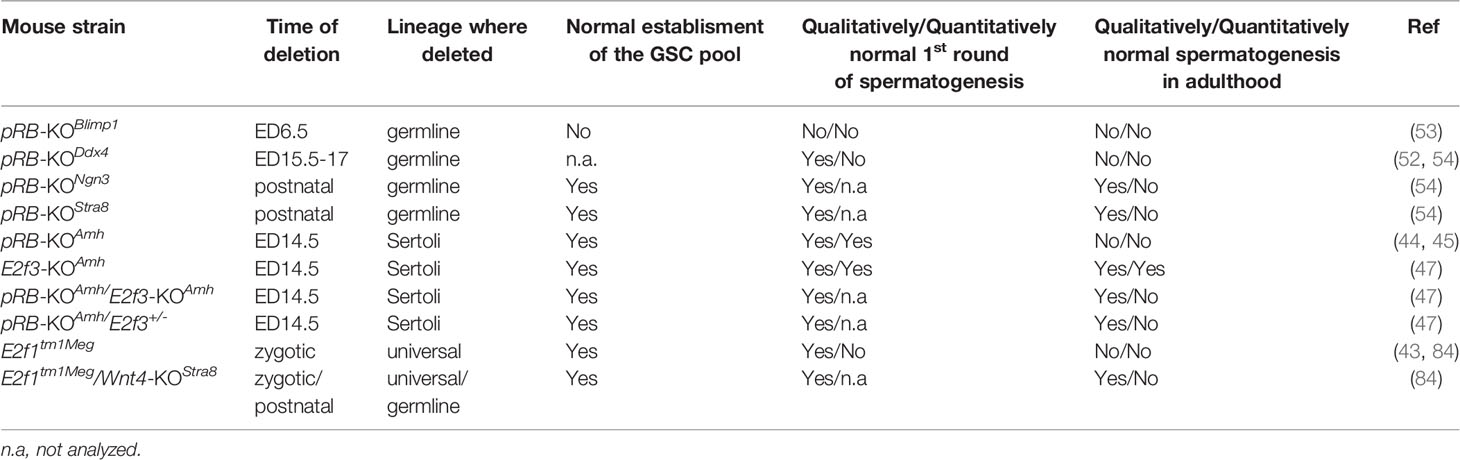
Table 1 Summary of spermatogenic phenotypes in different mouse strains with a deletion in gene(s) encoding RB-E2F pathway proteins.
Repressor E2Fs E2F4 and E2F5 Are Needed for the Development of Male Reproductive Tract
Compared to activator E2Fs, the functions of repressor E2Fs have been less investigated. While both E2f4 and E2f5-deficient mice are viable, they have a shortened lifespan (105–107). E2f4-mutants display developmental defects in multiple tissues and have a high early postnatal lethality due to susceptibility to infections (106, 107). E2f4-deficient male mice were found subfertile/infertile although the histology of male reproductive organs was reported normal (106, 107), and the underlying basis for this observation therefore remains elusive. There is a lack of knowledge concerning fertility of E2f5-mutant mice. However, e2f5 mutation in the zebrafish results in male infertility due to a spermatogenic arrest in prophase I, and subsequent apoptosis of spermatocytes (108). Rotgers et al. (2014, 2019) report a clear decrease in E2f4 and E2f5 expression in pRB-KOAmh mouse testis which is, however, likely due to lack of meiotic and postmeiotic germ cells (cf. Figure 2). Interestingly, E2F4 and E2F5 have been shown to display redundant roles in controlling the development of the male reproductive tract. E2f4-deficiency within the efferent ducts on a E2f5 heterozygous background leads to a loss of multiciliated cells from the efferent ducts, dilation of the seminiferous tubules and the rete testis, and infertility.
The other repressor E2Fs have been even less studied in the testis. E2f6-deficient mice are born at an expected Mendelian frequency, are viable and fertile, and grow and develop normally (109). Pohlers et al. (2005) have reported that E2F6 is needed to suppress the expression of germline genes in somatic tissues (109). However, the significance or functions of E2F6 in the germline cells are not known but mRNA level analysis (Figure 2) suggests they might be subtle. No fertility problems have been reported in mice deficient for E2f7 or E2f8, whereas the double-null mice are early embryonically lethal precluding any analysis on germline effects (110).
Concluding Remarks
Considering its role as the gatekeeper of cell cycle, it is no wonder that the RB-E2F pathway is critically important for development and function of the testis. Both the germline and the somatic Sertoli cells depend on proper regulation of its activity during formation of the testis and under steady-state spermatogenesis. Continuous production of sperm relies on lifelong maintenance of GSCs and functionally mature Sertoli cells, two fundamental outcomes of normal function of RB-E2F pathway, highlighting its importance for male fertility. Despite the fact that the functional consequences of pRB, E2F1 and E2F3-deficiency in the testis have been relatively well characterized, further studies are warranted to elucidate how RB-E2F is integrated into the regulation of the germline at the mechanistic level plus shed light on the diversity of RB-E2F signaling beyond pRB and E2F1 and E2F3. Notably, despite relatively high level of mRNA expression in germ cells, the spermatogenic functions of p107, p130, E2F4 and E2F5 are virtually undefined. Examining their roles would probably require creation of compound inducible KO mouse models to circumvent functional redundancy within both the RB and E2F families, and for targeted analyses of spermatogenic functions. Their temporally restricted patterns of expression along the spermatogenic trajectory are intriguing and worthy of deeper investigation.
Author Contributions
J-AM and JT: Conception of the article, writing and editing. All authors contributed to the article and approved the submitted version.
Conflict of Interest
The authors declare that the research was conducted in the absence of any commercial or financial relationships that could be construed as a potential conflict of interest.
Publisher’s Note
All claims expressed in this article are solely those of the authors and do not necessarily represent those of their affiliated organizations, or those of the publisher, the editors and the reviewers. Any product that may be evaluated in this article, or claim that may be made by its manufacturer, is not guaranteed or endorsed by the publisher.
Acknowledgments
The authors want to acknowledge Dr. Sheyla Cisneros-Montalvo (University of Turku) for drawing the cell types to Figure 5. Sigrid Juselius Foundation, Kirsten and Freddy Johansen Fund, and Turku University Hospital Special Governmental Funding are acknowledged for financial support.
References
1. Burkhart DL, Sage J. Cellular Mechanisms of Tumour Suppression by the Retinoblastoma Gene. Nat Rev Cancer (2008) 8:671–82. doi: 10.1038/nrc2399
2. Dick FA, Rubin SM. Molecular Mechanisms Underlying RB Protein Function. Nat Rev Mol Cell Biol (2013) 14:297–306. doi: 10.1038/nrm3567
3. Munro S, Carr SM, La Thangue NB. Diversity Within the pRb Pathway: Is There a Code of Conduct? Oncogene (2012) 31:4343–52. doi: 10.1038/onc.2011.603
4. Rubin SM, Sage J, Skotheim JM. Integrating Old and New Paradigms of G1/S Control. Mol Cell (2020) 80:183–92. doi: 10.1016/j.molcel.2020.08.020
5. DeGregori J, Kowalik T, Nevins JR. Cellular Targets for Activation by the E2F1 Transcription Factor Include DNA Synthesis- and G1/S-Regulatory Genes. Mol Cell Biol (1995) 15:4215–24. doi: 10.1128/MCB.15.8.4215
7. Stevens C, La Thangue NB. E2F and Cell Cycle Control: A Double-Edged Sword. Arch Biochem Biophys (2003) 412:157–69. doi: 10.1016/S0003-9861(03)00054-7
8. Weijts BGMW, Bakker WJ, Cornelissen PWA, Liang K-H, Schaftenaar FH, Westendorp B. E2F7 and E2F8 Promote Angiogenesis Through Transcriptional Activation of VEGFA in Cooperation With HIF1. EMBO J (2012) 31:3871–84. doi: 10.1038/emboj.2012.231
9. Chong J-L, Wenzel PL, Sáenz-Robles MT, Nair V, Ferrey A, Hagan JP, et al. E2f1-3 Switch From Activators in Progenitor Cells to Repressors in Differentiating Cells. Nature (2009) 462:930–4. doi: 10.1038/nature08677
10. Trikha P, Sharma N, Opavsky R, Reyes A, Pena C, Ostrowski MC, et al. E2f1-3 are Critical for Myeloid Development. J Biol Chem (2011) 286:4783–95. doi: 10.1074/jbc.M110.182733
11. Wenzel PL, Chong J-L, Sáenz-Robles MT, Ferrey A, Hagan JP, Gomez YM, et al. Cell Proliferation in the Absence of E2F1-3. Dev Biol (2011) 351:35–45. doi: 10.1016/j.ydbio.2010.12.025
12. Lee B-K, Bhinge AA, Iyer VR. Wide-Ranging Functions of E2F4 in Transcriptional Activation and Repression Revealed by Genome-Wide Analysis. Nucleic Acids Res (2011) 39:3558–73. doi: 10.1093/nar/gkq1313
13. Polager S, Ginsberg D. P53 and E2f: Partners in Life and Death. Nat Rev Cancer (2009) 9:738–48. doi: 10.1038/nrc2718
14. DeGregori J, Johnson DG. Distinct and Overlapping Roles for E2F Family Members in Transcription, Proliferation and Apoptosis. Curr Mol Med (2006) 6:739–48. doi: 10.2174/1566524010606070739
15. Cobrinik D. Pocket Proteins and Cell Cycle Control. Oncogene (2005) 24:2796–809. doi: 10.1038/sj.onc.1208619
16. Kitajima S, Takahashi C. Intersection of Retinoblastoma Tumor Suppressor Function, Stem Cells, Metabolism, and Inflammation. Cancer Sci (2017) 108:1726–31. doi: 10.1111/cas.13312
17. Attwooll C, Oddi S, Cartwright P, Prosperini E, Agger K, Steensgaard P, et al. A Novel Repressive E2F6 Complex Containing the Polycomb Group Protein, EPC1, That Interacts With EZH2 in a Proliferation-Specific Manner. J Biol Chem (2005) 280:1199–208. doi: 10.1074/jbc.M412509200
18. Ouseph MM, Li J, Chen H-Z, Pécot T, Wenzel P, Thompson JC, et al. Atypical E2F Repressors and Activators Coordinate Placental Development. Dev Cell (2012) 22:849–62. doi: 10.1016/j.devcel.2012.01.013
19. Dick FA, Dyson N. pRB Contains an E2F1-Specific Binding Domain That Allows E2F1-Induced Apoptosis to be Regulated Separately From Other E2F Activities. Mol Cell (2003) 12:639–49. doi: 10.1016/S1097-2765(03)00344-7
20. Cecchini MJ, Dick FA. The Biochemical Basis of CDK Phosphorylation-Independent Regulation of E2F1 by the Retinoblastoma Protein. Biochem J (2011) 434:297–308. doi: 10.1042/BJ20101210
21. van Harn T, Foijer F, van Vugt M, Banerjee R, Yang F, Oostra A, et al. Loss of Rb Proteins Causes Genomic Instability in the Absence of Mitogenic Signaling. Genes Dev (2010) 24:1377–88. doi: 10.1101/gad.580710
22. Yadav RP, Mäkelä J-A, Hyssälä H, Cisneros-Montalvo S, Kotaja N. DICER Regulates the Expression of Major Satellite Repeat Transcripts and Meiotic Chromosome Segregation During Spermatogenesis. Nucleic Acids Res (2020) 48:7135–53. doi: 10.1093/nar/gkaa460
23. Gonzalo S, García-Cao M, Fraga MF, Schotta G, Peters AHFM, Cotter SE, et al. Role of the RB1 Family in Stabilizing Histone Methylation at Constitutive Heterochromatin. Nat Cell Biol (2005) 7:420–8. doi: 10.1038/ncb1235
24. Guo J, Grow EJ, Mlcochova H, Maher GJ, Lindskog C, Nie X, et al. The Adult Human Testis Transcriptional Cell Atlas. Cell Res (2018) 28:1141–57. doi: 10.1038/s41422-018-0099-2
25. Green CD, Ma Q, Manske GL, Shami AN, Zheng X, Marini S, et al. A Comprehensive Roadmap of Murine Spermatogenesis Defined by Single-Cell RNA-Seq. Dev Cell (2018) 46:651–67.e10. doi: 10.1016/j.devcel.2018.07.025
26. Hermann BP, Cheng K, Singh A, Roa-De La Cruz L, Mutoji KN, Chen IC, et al. The Mammalian Spermatogenesis Single-Cell Transcriptome, From Spermatogonial Stem Cells to Spermatids. Cell Rep (2018) 25:1650–67.e8. doi: 10.1016/j.celrep.2018.10.026
27. Tan K, Song H-W, Thompson M, Munyoki S, Sukhwani M, Hsieh T-C, et al. Staged Developmental Mapping and X Chromosome Transcriptional Dynamics During Mouse Spermatogenesis. Nat Commun (2019) 10:1251. doi: 10.1038/s41467-019-09182-1
28. Shami AN, Zheng X, Munyoki SK, Ma Q, Manske GL, Green CD, et al. Single-Cell RNA Sequencing of Human, Macaque, and Mouse Testes Uncovers Conserved and Divergent Features of Mammalian Spermatogenesis. Dev Cell (2020) 54:529–47.e12. doi: 10.1016/j.devcel.2020.05.010
29. Yu X-W, Li T-T, Du X-M, Shen Q-Y, Zhang M-F, Wei Y-D, et al. Single-Cell RNA Sequencing Reveals Atlas of Dairy Goat Testis Cells. Zoological Res (2021) 42:401–5. doi: 10.24272/j.issn.2095-8137.2020.373
30. Qian P, Kang J, Liu D, Xie G. Single Cell Transcriptome Sequencing of Zebrafish Testis Revealed Novel Spermatogenesis Marker Genes and Stronger Leydig-Germ Cell Paracrine Interactions. Front Genet (2022) 13:851719. doi: 10.3389/fgene.2022.851719
31. Guo J, Nie X, Giebler M, Mlcochova H, Wang Y, Grow EJ, et al. The Dynamic Transcriptional Cell Atlas of Testis Development During Human Puberty. Cell Stem Cell (2020) 26:262–76. doi: 10.1016/j.stem.2019.12.005
32. Guo J, Sosa E, Chitiashvili T, Nie X, Rojas EJ, Oliver E, et al. Single-Cell Analysis of the Developing Human Testis Reveals Somatic Niche Cell Specification and Fetal Germline Stem Cell Establishment. Cell Stem Cell (2021) 28:764–78.e4. doi: 10.1016/j.stem.2020.12.004
33. Hara K, Nakagawa T, Enomoto H, Suzuki M, Yamamoto M, Simons BD, et al. Mouse Spermatogenic Stem Cells Continually Interconvert Between Equipotent Singly Isolated and Syncytial States. Cell Stem Cell (2014) 14:658–72. doi: 10.1016/j.stem.2014.01.019
34. Nakagawa T, Sharma M, Nabeshima Y, Braun RE, Yoshida S. Functional Hierarchy and Reversibility Within the Murine Spermatogenic Stem Cell Compartment. Science (2010) 328:62–7. doi: 10.1126/science.1182868
35. Sharma M, Srivastava A, Fairfield HE, Bergstrom D, Flynn WF, Braun RE. Identification of EOMES-Expressing Spermatogonial Stem Cells and Their Regulation by PLZF. Elife (2019) 8:e43352. doi: 10.7554/eLife.43352
36. La HM, Mäkelä J-A, Chan A-L, Rossello FJ, Nefzger CM, Legrand JMD, et al. Identification of Dynamic Undifferentiated Cell States Within the Male Germline. Nat Commun (2018) 9:2819-018-04827-z. doi: 10.1038/s41467-018-04827-z
37. Schrans-Stassen BH, van de Kant HJ, de Rooij DG, van Pelt AM. Differential Expression of C-Kit in Mouse Undifferentiated and Differentiating Type A Spermatogonia. Endocrinology (1999) 140:5894–900. doi: 10.1210/endo.140.12.7172
38. Pellegrini M, Filipponi D, Gori M, Barrios F, Lolicato F, Grimaldi P, et al. ATRA and KL Promote Differentiation Toward the Meiotic Program of Male Germ Cells. Cell Cycle (2008) 7:3878–88. doi: 10.4161/cc.7.24.7262
39. Zhou Q, Li Y, Nie R, Friel P, Mitchell D, Evanoff RM, et al. Expression of Stimulated by Retinoic Acid Gene 8 (Stra8) and Maturation of Murine Gonocytes and Spermatogonia Induced by Retinoic Acid. Vitro Biol Reprod (2008) 78:537–45. doi: 10.1095/biolreprod.107.064337
40. Ikami K, Tokue M, Sugimoto R, Noda C, Kobayashi S, Hara K, et al. Hierarchical Differentiation Competence in Response to Retinoic Acid Ensures Stem Cell Maintenance During Mouse Spermatogenesis. Development (2015) 142:1582–92. doi: 10.1242/dev.118695
41. Yuan L, JG L, Zhao J, Brundell E, Daneholt B, Höög C. The Murine SCP3 Gene is Required for Synaptonemal Complex Assembly, Chromosome Synapsis, and Male Fertility. Mol Cell (2000) 5:73–83. doi: 10.1016/S1097-2765(00)80404-9
42. Park M, Lee Y, Jang H, Lee O-H, Park S-W, Kim J-H, et al. SOHLH2 is Essential for Synaptonemal Complex Formation During Spermatogenesis in Early Postnatal Mouse Testes. Sci Rep (2016) 6:20980. doi: 10.1038/srep20980
43. Rotgers E, Nurmio M, Pietila E, Cisneros-Montalvo S, Toppari J. E2F1 Controls Germ Cell Apoptosis During the First Wave of Spermatogenesis. Andrology (2015) 3:1000–14. doi: 10.1111/andr.12090
44. Nalam RL, Andreu-Vieyra C, Braun RE, Akiyama H, Matzuk MM. Retinoblastoma Protein Plays Multiple Essential Roles in the Terminal Differentiation of Sertoli Cells. Mol Endocrinol (2009) 23:1900–13. doi: 10.1210/me.2009-0184
45. Rotgers E, Rivero-Muller A, Nurmio M, Parvinen M, Guillou F, Huhtaniemi I, et al. Retinoblastoma Protein (RB) Interacts With E2F3 to Control Terminal Differentiation of Sertoli Cells. Cell Death Dis (2014) 5:e1274. doi: 10.1038/cddis.2014.232
46. Yan W, Kero J, Suominen J, Toppari J. Differential Expression and Regulation of the Retinoblastoma Family of Proteins During Testicular Development and Spermatogenesis: Roles in the Control of Germ Cell Proliferation, Differentiation and Apoptosis. Oncogene (2001) 20:1343–56. doi: 10.1038/sj.onc.1204254
47. Rotgers E, Cisneros-Montalvo S, Nurmio M, Toppari J. Retinoblastoma Protein Represses E2F3 to Maintain Sertoli Cell Quiescence in Mouse Testis. J Cell Sci (2019) 132:jcs229849. doi: 10.1242/jcs.229849
48. El-Darwish KS, Parvinen M, Toppari J. Differential Expression of Members of the E2F Family of Transcription Factors in Rodent Testes. Reprod Biol Endocrinol (2006) 4:63. doi: 10.1186/1477-7827-4-63
49. Lee EY, Chang CY, Hu N, Wang YC, CC L, Herrup K, et al. Mice Deficient for Rb are Nonviable and Show Defects in Neurogenesis and Haematopoiesis. Nature (1992) 359:288–94. doi: 10.1038/359288a0
50. Clarke AR, Maandag ER, van Roon M, van der Lugt NM, van der Valk M, Hooper ML, et al. Requirement for a Functional Rb-1 Gene in Murine Development. Nature (1992) 359:328–30. doi: 10.1038/359328a0
51. Jacks T, Fazeli A, Schmitt EM, Bronson RT, Goodell MA, Weinberg RA. Effects of an Rb Mutation in the Mouse. Nature (1992) 359:295–300. doi: 10.1038/359295a0
52. Hu YC, de Rooij DG, Page DC. Tumor Suppressor Gene Rb is Required for Self-Renewal of Spermatogonial Stem Cells in Mice. Proc Natl Acad Sci U. S. A. (2013) 110:12685–90. doi: 10.1073/pnas.1311548110
53. Du G, Oatley MJ, Law NC, Robbins C, Wu X, Oatley JM. Proper Timing of a Quiescence Period in Precursor Prospermatogonia is Required for Stem Cell Pool Establishment in the Male Germline. Development (2021) 148:dev194571. doi: 10.1242/dev.194571
54. Yang Q-E, Gwost I, Oatley MJ, Oatley JM. Retinoblastoma Protein (RB1) Controls Fate Determination in Stem Cells and Progenitors of the Mouse Male Germline. Biol Reprod (2013) 89:113. doi: 10.1095/biolreprod.113.113159
55. Spiller CM, Wilhelm D, Koopman P. Retinoblastoma 1 Protein Modulates XY Germ Cell Entry Into G1/G0 Arrest During Fetal Development in Mice. Biol Reprod (2010) 82:433–43. doi: 10.1095/biolreprod.109.078691
56. Cobrinik D, MH L, Hannon G, Mulligan G, Bronson RT, Dyson N, et al. Shared Role of the pRB-Related P130 and P107 Proteins in Limb Development. Genes Dev (1996) 10:1633–44. doi: 10.1101/gad.10.13.1633
57. Lee MH, Williams BO, Mulligan G, Mukai S, Bronson RT, Dyson N, et al. Targeted Disruption of P107: Functional Overlap Between P107 and Rb. Genes Dev (1996) 10:1621–32. doi: 10.1101/gad.10.13.1621
58. de Rooij DG. The Nature and Dynamics of Spermatogonial Stem Cells. Development (2017) 144:3022–30. doi: 10.1242/dev.146571
59. Makela J-A, Hobbs RM. Molecular Regulation of Spermatogonial Stem Cell Renewal and Differentiation. Reproduction (2019) 158:R169–87. doi: 10.1530/REP-18-0476
60. Sage J. The Retinoblastoma Tumor Suppressor and Stem Cell Biology. Genes Dev (2012) 26:1409–20. doi: 10.1101/gad.193730.112
61. Mäkelä JA, Koskenniemi JJ, Virtanen HE, Toppari J. Testis Development. Endocr Rev (2019) 40:857–905. doi: 10.1210/er.2018-00140
62. Coucouvanis EC, Sherwood SW, Carswell-Crumpton C, Spack EG, Jones PP. Evidence That the Mechanism of Prenatal Germ Cell Death in the Mouse is Apoptosis. Exp Cell Res (1993) 209:238–47. doi: 10.1006/excr.1993.1307
63. Wang RA, Nakane PK, Koji T. Autonomous Cell Death of Mouse Male Germ Cells During Fetal and Postnatal Period. Biol Reprod (1998) 58:1250–6. doi: 10.1095/biolreprod58.5.1250
64. Nguyen DH, Soygur B, Peng S-P, Malki S, Hu G, Laird DJ. Apoptosis in the Fetal Testis Eliminates Developmentally Defective Germ Cell Clones. Nat Cell Biol (2020) 22:1423–35. doi: 10.1038/s41556-020-00603-8
65. Meng XJ, Lindahl M, Hyvonen ME, Parvinen M, de Rooij DG, MW H, et al. Regulation of Cell Fate Decision of Undifferentiated Spermatogonia by GDNF. Sci (80-. ). (2000) 287:1489–93. doi: 10.1126/science.287.5457.1489
66. La HM, Makela JA, Chan AL, Rossello FJ, Nefzger CM, Legrand JMD, et al. Germline Stem Cell Activity Is Sustained by SALL4-Dependent Silencing of Distinct Tumor Suppressor Genes. Stem Cell Rep (2017) 9:956–71. doi: 10.1016/j.stemcr.2017.08.001
67. Chan AL, La HM, Legrand JMD, Makela JA, Eichenlaub M, De Seram M, et al. Distinct Germline Progenitor Subsets Defined Through Tsc2-Mtorc1 Signaling. EMBO Rep (2015) 16:467–80. doi: 10.15252/embr.201439379
68. Mäkelä JA, Toppari J. Endocrinology of the Testis and Male Reproduction. In: Simoni M, Huhtaniemi I, editors. Endocrinology of the Testis and Male Reproduction. Cham:Springer International Publishing (2017). p. 1–39. doi: 10.1007/978-3-319-29456-8_13-1
69. Rebourcet D, Darbey A, Monteiro A, Soffientini U, Tsai YT, Handel I, et al. Sertoli Cell Number Defines and Predicts Germ and Leydig Cell Population Sizes in The Adult Mouse Testis. Endocrinology (2017) 158:2955–69. doi: 10.1210/en.2017-00196
70. Ahmed EA, Barten-van Rijbroek AD, Kal HB, Sadri-Ardekani H, Mizrak SC, van Pelt AM, et al. Proliferative Activity In Vitro and DNA Repair Indicate That Adult Mouse and Human Sertoli Cells are Not Terminally Differentiated, Quiescent Cells. Biol Reprod (2009) 80:1084–91. doi: 10.1095/biolreprod.108.071662
71. Mäkelä JA, Toppari J, Rivero-Muller A, Ventela S. Reconstruction of Mouse Testicular Cellular Microenvironments in Long-Term Seminiferous Tubule Culture. PloS One (2014) 9:e90088. doi: 10.1371/journal.pone.0090088
72. Greenspan LJ, Matunis EL. Retinoblastoma Intrinsically Regulates Niche Cell Quiescence, Identity, and Niche Number in the Adult Drosophila Testis. Cell Rep (2018) 24:3466–3476.e8. doi: 10.1016/j.celrep.2018.08.083
73. Dominado N, La Marca JE, Siddall NA, Heaney J, Tran M, Cai Y, et al. Rbf Regulates Drosophila Spermatogenesis via Control of Somatic Stem and Progenitor Cell Fate in the Larval Testis. Stem Cell Rep (2016) 7:1152–63. doi: 10.1016/j.stemcr.2016.11.007
74. Bremner WJ, Millar MR, Sharpe RM, Saunders PT. Immunohistochemical Localization of Androgen Receptors in the Rat Testis: Evidence for Stage-Dependent Expression and Regulation by Androgens. Endocrinology (1994) 135:1227–34. doi: 10.1210/endo.135.3.8070367
75. Faisal I, Cisneros-Montalvo S, Hamer G, Tuominen MM, Laurila P-P, Tumiati M, et al. Transcription Factor USF1 Is Required for Maintenance of Germline Stem Cells in Male Mice. Endocrinology (2019) 160:1119–36. doi: 10.1210/en.2018-01088
76. Chen H-Z, Tsai S-Y, Leone G. Emerging Roles of E2Fs in Cancer: An Exit From Cell Cycle Control. Nat Rev Cancer (2009) 9:785–97. doi: 10.1038/nrc2696
77. Dick FA, Goodrich DW, Sage J, Dyson NJ. Non-Canonical Functions of the RB Protein in Cancer. Nat Rev Cancer (2018) 18:442–51. doi: 10.1038/s41568-018-0008-5
78. Krishnan B, Yasuhara T, Rumde P, Stanzione M, Lu C, Lee H, et al. Active RB Causes Visible Changes in Nuclear Organization. J Cell Biol (2022) 221:e202102144. doi: 10.1083/jcb.202102144
79. Tarulli GA, Stanton PG, Meachem SJ. Is the Adult Sertoli Cell Terminally Differentiated? Biol Reprod (2012) 87:1–11,13. doi: 10.1095/biolreprod.111.095091
80. Nalam RL, Andreu-Vieyra C, Matzuk MM. Absence of Inhibin Alpha and Retinoblastoma Protein Leads to Early Sertoli Cell Dysfunction. PloS One (2010) 5:e11797. doi: 10.1371/journal.pone.0011797
81. Matzuk MM, Finegold MJ, Su JG, Hsueh AJ, Bradley A. Alpha-Inhibin is a Tumour-Suppressor Gene With Gonadal Specificity in Mice. Nature (1992) 360:313–9. doi: 10.1038/360313a0
82. Yamasaki L, Jacks T, Bronson R, Goillot E, Harlow E, Dyson NJ. Tumor Induction and Tissue Atrophy in Mice Lacking E2F-1. Cell (1996) 85:537–48. doi: 10.1016/S0092-8674(00)81254-4
83. Hoja M-R, Liu J-G, Mohammadieh M, Kvist U, Yuan L. E2F1 Deficiency Impairs Murine Spermatogenesis and Augments Testicular Degeneration In SCP3-Nullizygous Mice. Cell Death Differentiation (2004) 11:354–6. doi: 10.1038/sj.cdd.4401362
84. Jorgez CJ, Seth A, Wilken N, Bournat JC, Chen CH, Lamb DJ. E2F1 Regulates Testicular Descent and Controls Spermatogenesis by Influencing WNT4 Signaling. Development (2021) 148:dev191189. doi: 10.1242/dev.191189
85. Agger K, Santoni-Rugiu E, Holmberg C, Karlström O, Helin K. Conditional E2F1 Activation in Transgenic Mice Causes Testicular Atrophy and Dysplasia Mimicking Human CIS. Oncogene (2005) 24:780–9. doi: 10.1038/sj.onc.1208248
86. Novotny GW, Sonne SB, Nielsen JE, Jonstrup SP, Hansen MA, Skakkebaek NE, et al. Translational Repression of E2F1 mRNA in Carcinoma in Situ and Normal Testis Correlates With Expression of the miR-17-92 Cluster. Cell Death Differentiation (2007) 14:879–82. doi: 10.1038/sj.cdd.4402090
87. Jorgez CJ, Wilken N, Addai JB, Newberg J, Vangapandu HV, Pastuszak AW, et al. Genomic and Genetic Variation in E2F Transcription Factor-1 in Men With Nonobstructive Azoospermia. Fertil Steril (2015) 103:44–52.e1. doi: 10.1016/j.fertnstert.2014.09.021
88. Rocca MS, Di Nisio A, Sabovic I, Ghezzi M, Foresta C, Ferlin A, et al. E2F1 Copy Number Variations Contribute to Spermatogenic Impairment and Cryptorchidism by Increasing Susceptibility to Heat Stress. Andrology (2019) 7:251–6. doi: 10.1111/andr.12583
89. Field SJ, FY T, Kuo F, Zubiaga AM, Kaelin WGJ, Livingston DM, et al. E2F-1 Functions in Mice to Promote Apoptosis and Suppress Proliferation. Cell (1996) 85:549–61. doi: 10.1016/S0092-8674(00)81255-6
90. Sada A, Suzuki A, Suzuki H, Saga Y. The RNA-Binding Protein NANOS2 is Required to Maintain Murine Spermatogonial Stem Cells. Science (2009) 325:1394–8. doi: 10.1126/science.1172645
91. Suzuki A, Saba R, Miyoshi K, Morita Y, Saga Y. Interaction Between NANOS2 and the CCR4-NOT Deadenylation Complex is Essential for Male Germ Cell Development in Mouse. PloS One (2012) 7:e33558. doi: 10.1371/journal.pone.0033558
92. Zhou Z, Shirakawa T, Ohbo K, Sada A, Wu Q, Hasegawa K, et al. RNA Binding Protein Nanos2 Organizes Post-Transcriptional Buffering System to Retain Primitive State of Mouse Spermatogonial Stem Cells. Dev Cell (2015) 34:96–107. doi: 10.1016/j.devcel.2015.05.014
93. Codino A, Turowski T, van de Lagemaat LN, Ivanova I, Tavosanis A, Much C, et al. NANOS2 is a Sequence-Specific mRNA-Binding Protein That Promotes Transcript Degradation in Spermatogonial Stem Cells. iScience (2021) 24:102762. doi: 10.1016/j.isci.2021.102762
94. Cafe SL, Skerrett-Byrne DA, De Oliveira CS, Nixon B, Oatley MJ, Oatley JM, et al. A Regulatory Role for CHD4 in Maintenance of the Spermatogonial Stem Cell Pool. Stem Cell Rep (2021) 16:1555–67. doi: 10.1016/j.stemcr.2021.04.003
95. Zhuang T, Hess RA, Kolla V, Higashi M, Raabe TD, Brodeur GM, et al. CHD5 is Required for Spermiogenesis and Chromatin Condensation. Mech Dev (2014) 131:35–46. doi: 10.1016/j.mod.2013.10.005
96. Li W, Wu J, Kim S-Y, Zhao M, Hearn SA, Zhang MQ, et al. Chd5 Orchestrates Chromatin Remodelling During Sperm Development. Nat Commun (2014) 5:3812. doi: 10.1038/ncomms4812
97. Yeh JR, Zhang X, Nagano MC. Indirect Effects of Wnt3a/beta-Catenin Signalling Support Mouse Spermatogonial Stem Cells In Vitro. PloS One (2012) 7:e40002. doi: 10.1371/journal.pone.0040002
98. Takase HM, Nusse R. Paracrine Wnt/beta-Catenin Signaling Mediates Proliferation of Undifferentiated Spermatogonia in the Adult Mouse Testis. Proc Natl Acad Sci USA (2016) 113:E1489–97. doi: 10.1073/pnas.1601461113
99. Tokue M, Ikami K, Mizuno S, Takagi C, Miyagi A, Takada R, et al. SHISA6 Confers Resistance to Differentiation-Promoting Wnt/beta-Catenin Signaling in Mouse Spermatogenic Stem Cells. Stem Cell Rep (2017) 8:561–75 doi: 10.1016/j.stemcr.2017.01.006
100. Boyer A, Yeh JR, Zhang X, Paquet M, Gaudin A, Nagano MC, et al. CTNNB1 Signaling in Sertoli Cells Downregulates Spermatogonial Stem Cell Activity via WNT4. PloS One (2012) 7:e29764. doi: 10.1371/journal.pone.0029764
101. Chassot AA, Le Rolle M, Jourden M, Taketo MM, Ghyselinck NB, Chaboissier MC. Constitutive WNT/CTNNB1 Activation Triggers Spermatogonial Stem Cell Proliferation and Germ Cell Depletion. Dev Biol (2017) 426:17–27. doi: 10.1016/j.ydbio.2017.04.010
102. Kitadate Y, Jorg DJ, Tokue M, Maruyama A, Ichikawa R, Tsuchiya S, et al. Competition for Mitogens Regulates Spermatogenic Stem Cell Homeostasis in an Open Niche. Cell Stem Cell (2019) 24:79–92.e6. doi: 10.1016/j.stem.2018.11.013
103. Hobbs RM, Seandel M, Falciatori I, Rafii S, Pandolfi PP. Plzf Regulates Germline Progenitor Self-Renewal by Opposing Mtorc1. Cell (2010) 142:468–79. doi: 10.1016/j.cell.2010.06.041
104. Busada JT, Niedenberger BA, Velte EK, Keiper BD, Geyer CB. Mammalian Target of Rapamycin Complex 1 (Mtorc1) Is Required for Mouse Spermatogonial Differentiation. Vivo Dev Biol (2015) 407:90–102. doi: 10.1016/j.ydbio.2015.08.004
105. Lindeman GJ, Dagnino L, Gaubatz S, Xu Y, Bronson RT, Warren HB, et al. A Specific, Nonproliferative Role for E2F-5 in Choroid Plexus Function Revealed by Gene Targeting. Genes Dev (1998) 12:1092–8. doi: 10.1101/gad.12.8.1092
106. Humbert PO, Rogers C, Ganiatsas S, Landsberg RL, Trimarchi JM, Dandapani S, et al. E2F4 is Essential for Normal Erythrocyte Maturation and Neonatal Viability. Mol Cell (2000) 6:281–91. doi: 10.1016/S1097-2765(00)00029-0
107. Rempel RE, Saenz-Robles MT, Storms R, Morham S, Ishida S, Engel A, et al. Loss of E2F4 Activity Leads to Abnormal Development of Multiple Cellular Lineages. Mol Cell (2000) 6:293–306. doi: 10.1016/S1097-2765(00)00030-7
108. Xie H, Kang Y, Wang S, Zheng P, Chen Z, Roy S, et al. E2f5 is a Versatile Transcriptional Activator Required for Spermatogenesis and Multiciliated Cell Differentiation in Zebrafish. PloS Genet (2020) 16:e1008655. doi: 10.1371/journal.pgen.1008655
109. Pohlers M, Truss M, Frede U, Scholz A, Strehle M, Kuban R-J, et al. A Role for E2F6 in the Restriction of Male-Germ-Cell-Specific Gene Expression. Curr Biol (2005) 15:1051–7. doi: 10.1016/j.cub.2005.04.060
Keywords: retinoblastoma protein, spermatogenesis, testis, E2F transcription factor, germ cell, Sertoli cell
Citation: Mäkelä J-A and Toppari J (2022) Retinoblastoma-E2F Transcription Factor Interplay Is Essential for Testicular Development and Male Fertility. Front. Endocrinol. 13:903684. doi: 10.3389/fendo.2022.903684
Received: 24 March 2022; Accepted: 11 April 2022;
Published: 19 May 2022.
Edited by:
Barry Zirkin, Johns Hopkins University, United StatesReviewed by:
Kate Lakoski Loveland, Monash University, AustraliaWei Yan, Lundquist Institute for Biomedical Innovation, United States
Copyright © 2022 Mäkelä and Toppari. This is an open-access article distributed under the terms of the Creative Commons Attribution License (CC BY). The use, distribution or reproduction in other forums is permitted, provided the original author(s) and the copyright owner(s) are credited and that the original publication in this journal is cited, in accordance with accepted academic practice. No use, distribution or reproduction is permitted which does not comply with these terms.
*Correspondence: Jorma Toppari, am9ybWEudG9wcGFyaUB1dHUuZmk=