- 1The Orthopaedic Center, Wenling First People’s Hospital (The Affiliated Wenling Hospital of Wenzhou Medical University), Wenling, China
- 2Department of Cardiology, Wenling First People’s Hospital (The Affiliated Wenling Hospital of Wenzhou Medical University), Wenling, China
SIRT3 is an NAD+-dependent deacetylase in the mitochondria with an extensive ability to regulate mitochondrial morphology and function. It has been reported that SIRT3 participates in the occurrence and development of many aging-related diseases. Osteoporosis is a common aging-related disease characterized by decreased bone mass and fragility fractures, which has caused a huge burden on society. Current research shows that SIRT3 is involved in the physiological processes of senescence of bone marrow mesenchymal stem cells (BMSCs), differentiation of BMSCs and osteoclasts. However, the specific effects and mechanisms of SIRT3 in osteoporosis are not clear. In the current review, we elaborated on the physiological functions of SIRT3, the cell types involved in bone remodeling, and the role of SIRT3 in osteoporosis. Furthermore, it also provided a theoretical basis for SIRT3 as a therapeutic target for osteoporosis.
Introduction
Osteoporosis is a systemic bone disease characterized by bone loss and bone tissue microstructure destruction (1). After the bones mature, bone formation and bone resorption are under an equilibrium, thereby maintaining normal bone mass (2). Several factors might cause the destruction of this balance and the occurrence of osteoporosis, such as aging, hormone deficiency, genetic factors, etc. (3). As the aging population grows, osteoporosis and the related complication fragility fractures result in a high disability rate. In the United States, approximately 54 million adults over the age of 50 suffer from osteoporosis or are at risk of insufficient bone mass (4). Notably, the incidence of osteoporotic fractures is about 3 to 4 times higher than that of cardiovascular disease or cancer (5). Therefore, it is of great significance to explore its molecular mechanism and develop high-efficiency treatments for osteoporosis.
Mitochondria are the unique organelles of eukaryotes, which play an important role in maintaining homeostasis, such as metabolism, energy production, oxidative stress, and apoptosis (6, 7). Osteoporosis is a prevalent aging-related disease, which is usually accompanied by changes in metabolic processes and mitochondrial dysfunction (8, 9). And mitochondrial dysfunction will lead to the accumulation of reactive oxygen species (ROS) and induce the damage of various macromolecules such as proteins, nucleic acids, and lipids in cells (10). Besides, oxidative stress could increase the activity of osteoclasts and decrease the osteogenic potential of osteoblasts, thereby destroying bone homeostasis (11–13). All these suggest that mitochondrial dysfunction might contribute to the occurrence and development of osteoporosis.
Sirtuins (SIRTs) are a family of highly conserved NAD+-dependent deacetylases in mammals, which influence several metabolism processes (14–16). As a member of mitochondrial sirtuins, SIRT3 is located on chromosome 11 (Chr11p15.5) and serves a critical role in mitochondrial homeostasis, metabolic regulation, gene transcription, and genome stability (17–19). SIRT3 has been reported to be involved in a variety of aging-related diseases, such as Alzheimer’s disease, Parkinson’s disease, cardiovascular disease, and bone diseases (20, 21). A previous study showed that the senescence of bone mesenchymal stem cells (BMSCs) could lead to osteogenic damage and osteoporosis (22). And the maintenance of mitochondrial NAD+ levels and the expression of SIRT3 could delay the senescence of MSCs (23). Though recent evidence indicates that SIRT3 is linked to osteoporosis, the exact mechanism is still unclear. In this review, we are devoted to explaining the role and molecular mechanism of SIRT3 in osteoporosis.
The Physiological Function of SIRT3
In the mitochondrial sirtuins, SIRT3 exhibits strong deacetylase activity, which contains a large Rossman fold domain that binds to NAD+ and a small domain with a zinc finger structure (24). A large amount of evidence proves that SIRT3 could regulate mitochondrial functions from many aspects, such as energy metabolism, oxidative stress, mitophagy, etc. (25). For example, SIRT3 could deacetylate FOXO3 under the induction of hydrogen peroxide, thereby regulating mitochondrial quality, ATP production, and clearance of defective mitochondria (26).
As one of the most basic characteristics of life, energy metabolism mainly includes the release, transfer, storage, and utilization of energy. SIRT3 could promote energy production by deacetylating the subunit proteins of the mitochondrial respiratory chain complex (27). For instance, SIRT3 could maintain intracellular metabolic balance by deacetylating ATP synthase beta (28, 29). In addition, SIRT3 could deacetylate Acetyl-CoA Synthase 2, succinate dehydrogenase and 3-hydroxy-3-methylglutaryl CoA synthase 2, thereby indirectly regulating energy production (30–32). The in-vivo study also confirmed that ATP production in SIRT3-/- mice was approximately reduced by 50% (33). In short, SIRT3 is an important regulator of energy homeostasis.
Mitochondria are not only involved in energy metabolism, but also crucial for the production and scavenging of ROS (34). A previous study showed that SIRT3 reduced the production of ROS by deacetylating FOXO3a (35). Besides, SIRT3 could increase the activity of superoxide dismutase 2 (SOD2), and result in the reduced ROS and prevention of cell senescence (36, 37). All above evidence shows that SIRT3 serves as a key mitochondrial protein to protect cells from ROS via enhancing the activity of the antioxidant defense system.
Mitophagy is a kind of mitochondrial selective autophagy, which degrades damaged mitochondria in cells (38, 39). In the myocardium of SIRT3-/- mice, Li et al. found that SIRT3 deficiency could significantly inhibit p53/Parkin-mediated mitophagy and promote mitochondrial dysfunction (40). The deficiency in SIRT3 could also damage the mitochondrial fission and mitophagy through FOXO3a/Parkin signaling (41). Additionally, SIRT3 acts as a key activator of mitophagy, which may be mediated by the VDAC1/Parkin pathway (42). Therefore, SIRT3 is central to the maintenance of proper mitochondrial function by regulating mitophagy through multiple pathways (43).
The Cell Types Involved in Bone Remodeling
Bone remodeling is a dynamic process including several stages: initiation/activation stage; bone resorption stage; reversal stage; osteogenesis stage; and mineralization stage (44). This process is mainly carried out in an anatomical and functional structure called basic multicellular units, involving multiple types of cells: bone stem cells, osteocytes, osteoclasts, and osteoblasts, etc. (45, 46). MSCs are a group of pluripotent stem cells developed from the mesoderm and mainly exist in the bone marrow and adipose tissue (47, 48). As the common progenitor cells of osteoblasts, adipocytes, and chondrocytes, BMSCs play an important role in bone homeostasis (49). The differentiation of BMSCs is affected by many factors, such as hormones, cytokines, and mechanical factors (49). Previous studies have shown that estrogen and bone morphogenetic protein 2 (BMP2) were the signals of the osteogenic differentiation of BMSCs, and peroxisome proliferator-activated receptor γ (PPARγ) could promote the differentiation of BMSCs into adipocytes (50, 51).
Osteoblasts are vital for bone formation, which can not only differentiate into the most abundant osteocytes but also promote the mineralization of osteoid and regulate the function of osteoclasts (52–54). Osteoclasts are multinucleated cells derived from hematopoietic stem cells and are mainly responsible for bone resorption (55). It is currently clear that nuclear factor receptor activator-B (RANK)/RANK ligand (RANKL) is the main signal pathway for osteoclast differentiation and bone resorption (56, 57). In the process of osteoclast formation, bone marrow-derived macrophages differentiate into tartrate-resistant acid phosphatase+ (TRAP+) preosteoclasts under the action of the RANKL receptor activator (58). Mononuclear preosteoclast cells fuse with each other to form multinucleated mature osteoclasts. Osteoprotegerin (OPG) is secreted by a variety of cells including osteoblasts and mainly reduces bone loss by blocking the combination of RANKL and RANK (59). The OPG levels were reduced due to the aging-related decrease of osteoblasts, thereby activating osteoclast resorption and causing osteoporosis (60). Interestingly, osteocytes, as the protagonist of bone formation, are also the main source of RANKL, thereby promoting the occurrence of osteoclasts (61). In the dynamic process of bone remodeling, all participating cells might interact or restrict each other to achieve bone homeostasis.
The Role of SIRT3 in Bone Remodeling and Osteoporosis
Osteoporosis is a chronic disease caused by the imbalance of bone formation and bone resorption (62). SIRT3 has been demonstrated to play an important role in bone remodeling. Next, we will explain how SIRT3 participates in bone remodeling and osteoporosis from different aspects, including the senescence and differentiation of BMSCs, differentiation of osteoblasts, osteoclastogenesis, and changes in bone mass (Figure 1).
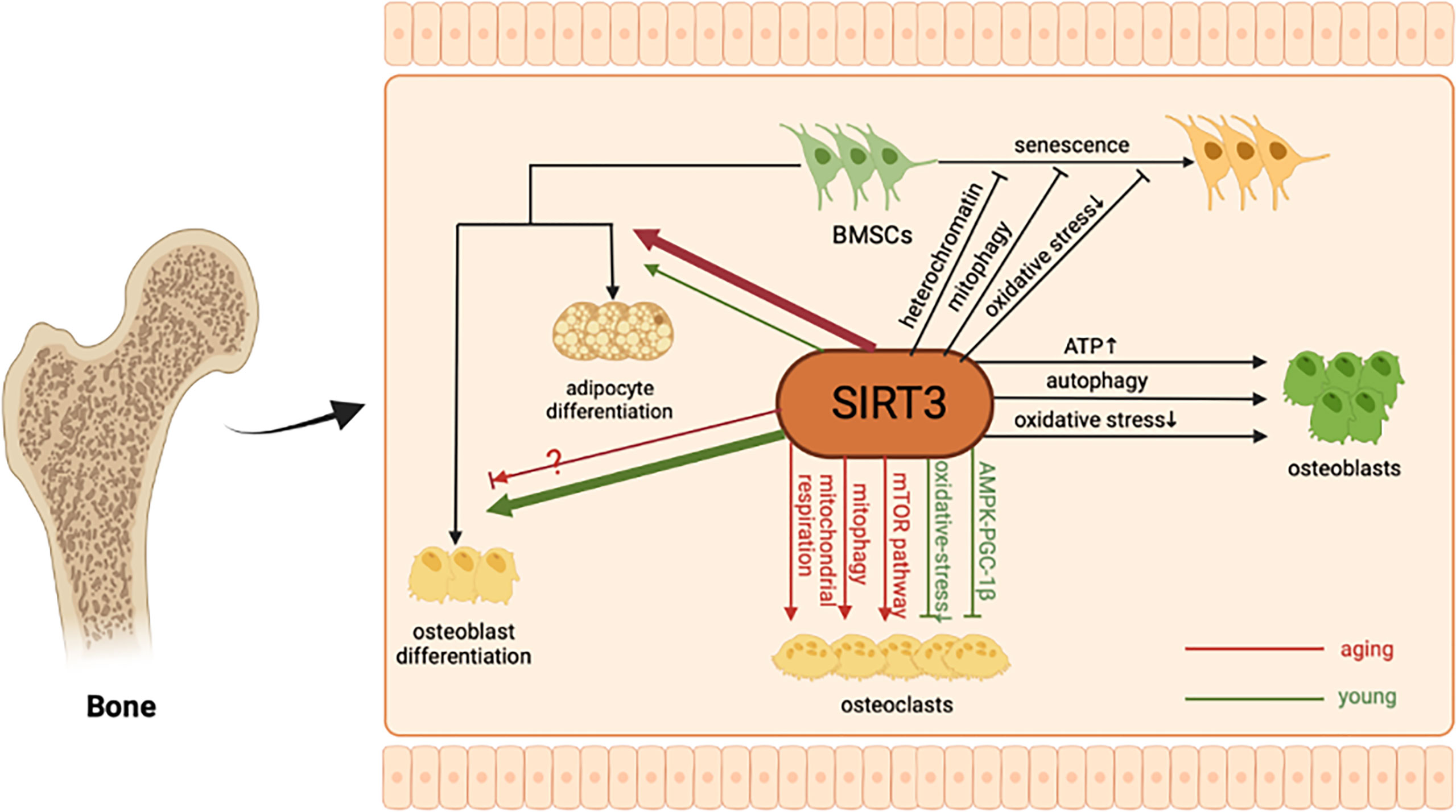
Figure 1 The molecular mechanism of SIRT3 in bone remodeling. As a major mitochondrial protein deacetylase, SIRT3 can alleviate BMSCs senescence by regulating oxidative stress, mitophagy, and stabilizing heterochromatin. In addition, SIRT3 could protect osteoblasts and promote bone formation through multiple pathways. Regarding BMSCs differentiation and osteoclastogenesis, the regulatory role of SIRT3 may be age-related.
The Role of SIRT3 in the Senescence of BMSCs
BMSCs are characterized by self-renewal and multi-differentiation potential (63). And the senescence and aberrant differentiation of BMSCs are related to a variety of pathophysiological processes, including osteoporosis (64, 65). The increased oxidative stress is a major feature of senescent BMSCs (66, 67). SIRT3 could reduce oxidative stress-caused BMSCs apoptosis via activating manganese superoxide dismutase (MnSOD, an alias for SOD2) and catalase (68). In the BMSCs models of natural senescence and H2O2-induced premature senescence, the expression of SIRT3 was significantly reduced, which was related to the decrease of antioxidant capacity and the aggravation of DNA damage (69). SIRT3 supplementation could alleviate BMSCs senescence by reducing ROS-induced damage, and enhancing the expression and activity of SOD2. Besides, SIRT3 positively regulates catalase and SOD2 by translocating FOXO3a into the nucleus, thereby protecting aged donor BMSCs from oxidative damage (70, 71). All the above evidence indicates that SIRT3 could regulate the senescence of BMSCs through oxidative stress-related pathways.
Abnormal mitophagy could damage mitochondrial quality and function, and play a key role in stem cell maintenance and differentiation (39, 72–74). Guo et al. found that advanced glycation end products (AGEs) could destroy mitochondria function and mitophagy, eventually leading to cell senescence (75). Interestingly, the silencing of SIRT3 can further strengthen the effect of AGEs, and the overexpression of SIRT3 can significantly reduce the occurrence of BMSCs aging and osteoporosis. Regulation of mitophagy was another mechanism for SIRT3 to reduce the senescence of BMSCs and senile osteoporosis.
In addition to mitochondrial homeostasis, stem cell aging is also accompanied by various epigenetic changes, including abnormal DNA methylation, histone modification, and disorganized heterochromatin (76, 77). Previous studies have shown that SIRT3 could take a deacetylation effect in mitochondria, thereby regulating the formation of epigenetic regulators acetyl-Coenzyme a and β-hydroxybutyric acid (78, 79). Additionally, heterochromatin is an important epigenetic driving factor in the regulation of aging (80, 81). Diao et al. found that SIRT3 interacted with nuclear lamina proteins and heterochromatin-related proteins to consolidate heterochromatin, which partly explained the mechanism of SIRT3 in preventing the senescence of BMSCs (82).
In summary, SIRT3, as a longevity gene, could not only maintain the mitochondrial function in the mitochondria but also stabilize the heterochromatin in the nucleus, so as to delay the senescence of BMSCs.
The Role of SIRT3 in the Differentiation of BMSCs
BMSCs have great potential for bone remodeling due to their osteogenic differentiation ability. As aging progresses, adipogenic differentiation of BMSCs increases and osteogenic differentiation weakens, causing abnormal bone metabolism (83, 84). The SIRT3 deletion reduces the differentiation of BMSCs into adipocytes and osteoblasts, while overexpression of SIRT3 enhances the differentiation ability of young BMSCs (passage 3) and aging BMSCs (passage 7) (85). Ho et al. found that SIRT3 overexpression resulted in more adipocytes in the bone marrow niche and the decreased bone mass in aging male mice (86). However, the regulation role of SIRT3 in bone was not observed in young (6-month-old) and female mice. Furthermore, BMSCs derived from aging mice overexpressing SIRT3 showed a stronger ability to differentiate into adipocytes compared with the control group, and osteoblastogenesis was suppressed (86). Interestingly, such results are inconsistent with previous research findings. Instead of protecting bone loss, SIRT3 has deleterious effects on osteogenic differentiation of BMSCs. It suggests that SIRT3 plays a complex regulatory role in the differentiation of BMSCs and the maintenance of bone homeostasis, which may be related to age and gender, but its specific mechanism need to be further explored.
The Role of SIRT3 in Osteoblasts
Osteoporosis is associated with increased levels of oxidative stress in osteoblasts, which may be a key component of the pathophysiology of bone loss (87). Li et al. found that nicotine could induce mitochondrial oxidative stress and mitochondrial DNA (mtDNA) damage in osteoblasts, ultimately leading to osteoporosis. Mechanically, nicotine can reduce the SIRT3 level, thereby significantly reducing the deacetylation level and activity of SOD2 in osteoblasts (88). In mouse pre-osteoblastic MC3T3-E1 cells, SIRT3 deletion could downregulate mitochondrial function and biogenesis through the PGC-1α/SOD2 signaling pathway, leading to impairment of osteogenesis (89). More importantly, SIRT3 deficiency could contribute to the impaired osteoblast function, bone loss and osteoporosis in SIRT3−/− mice. Both in-vivo and in-vitro experiments have proved that the SIRT3 could support increased ATP production, robust mitochondrial biogenesis, and osteoblast differentiation via deacetylating SOD2 (90).
Titanium is an important material for prostheses and stents for bone reconstruction, which could increase the durability of mechanical damage (91). With the development of nano-toxicology, nano-materials have been confirmed to be cytotoxic (92). For instance, titanium can cause osteoblast damage through autophagy and excessive mitochondrion-derived ROS (mROS). SIRT3 could reduce the acetylation of SOD2, the production of titanium-induced mROS and the expression level of LC3, thereby improving the viability of osteoblasts (93). Therefore, the SIRT3 and SOD2 may form an important regulatory network to protect osteoblasts against the cytotoxic of TiO2NPs (94). Besides, upregulation of SIRT3 significantly attenuates the titanium particle-induced inhibition of osteogenesis by inhibiting the NLRP3 inflammasome (95). To sum up, SIRT3 plays a protective role in bone formation and maintaining bone homeostasis.
The Role of SIRT3 in Osteoclasts
Osteoclasts, the protagonist of bone resorption, mainly resorb the mineralized bone matrix to maintain bone and mineral homeostasis (96, 97). As an indispensable element in various cell signal transduction, ROS acts as the second messenger in the process of osteoclast differentiation and activation induced by RANKL (98). Haemin et al. found that SIRT3 downregulation enhanced osteoclasts formation and RANKL-induced bone loss in five-week-old female ICR mice. Mechanically, SIRT3 could enhance SOD2 activity through deacetylation of lysine 68 and reduce intracellular ROS level, thereby inhibiting osteoclast differentiation (98). What’s more, SIRT3 could inhibit the osteoclast differentiation by regulating AMPK-PGC-1β pathway (99). And the decreased bone mass was observed in the young SIRT3−/− mice (eight-week-old), which was mainly caused by increased osteoclastogenesis (99). Therefore, SIRT3 might serve as a negative regulator in osteoclast differentiation and bone mass at a young age.
On the contrary, SIRT3 could promote osteoclastogenesis and bone loss by activating the mechanistic target of rapamycin (mTOR) pathway in aging transgenic overexpressing SIRT3 mice (13-month-old) (86). Additional researchers found that deletion of SIRT3 had no effect on bone in young mice, but attenuated age-related bone loss in 16-month-old mice. Loss of SIRT3 impaired bone resorption by reducing the mitochondrial respiration and mitophagy of osteoclasts, but had no effect on osteoclast number (100). It seems that elevated SIRT3 may promote bone loss in old age, partly due to an imbalance in bone resorption.
Aging and sex steroid deficiency are two common causes of osteoporosis (101). Therefore, in addition to aging models, the researchers also constructed estrogen deficiency-related animal models to study the role of SIRT3 in osteoporosis (100). In 5-month-old ovariectomized SIRT3-/- mice, remission of ovariectomy-induced cortical bone loss can be observed, accompanied by a decreased bone resorption instead of an increase in bone formation (100). How estrogen directly or indirectly affects the role of SIRT3 in mitochondria as well as bone homeostasis needs to be dissected.
In addition to aging and sex steroid-related bone loss, SIRT3 also takes part in the pathogenesis of ionizing radiation exposure-induced osteoporosis. Ionizing radiation could increase the expression and enzymatic activity of SIRT3 in osteoclasts and cause osteoclast differentiation and bone loss in young adult male mice (102). Nevertheless, the study did not explore the role of SIRT3 in the response of female and elderly mice to ionizing radiation.
Moreover, titanium has a side effect on the formation and function of osteoclasts. Surprisingly, in the murine model of osteolysis induced by titanium particles, inhibition of SIRT3 could prevent titanium particle-induced bone resorption and osteoclast formation by inhibiting ERK and JNK signals (103). Therefore, SIRT3 could regulate bone resorption to alleviate the cytotoxicity of titanium. It’s still required to elucidate how SIRT3 maintains the balance between bone resorption and bone formation.
Taken together, SIRT3 might play an important regulatory role in bone formation and bone resorption through multiple pathways. It seems that SIRT3 acts to promote BMSCs osteogenic differentiation and inhibit osteoclast differentiation to stabilize bone mass in young age, while in aging stage, SIRT3 may promote adipogenic differentiation of BMSCs, osteoclast differentiation and bone resorption, eventually leading to bone loss. However, it is still unknown when and how this regulatory effect is switched. Second, how SIRT3 stabilizes in bone formation and resorption remains unexplained. Indeed, downregulation of SIRT3 inhibited osteoclast differentiation in aging, accompanied by a small decrease in osteoblast production (100). Therefore, various pathological or health conditions and osteoporosis caused by different reasons should be considered to explain these inconsistent results and the role of SIRT3 in osteoporosis.
Sirt3 as a Potential Target for the Treatment of Osteoporosis
Accumulating evidence has indicated that intervention for SIRT3 could improve osteoporosis, showing its potential as a treatment strategy. Exogenous supplementation or overexpression of SIRT3 could alleviate bone loss and osteoporosis (75, 104). For example, the overexpression of SIRT3 by intravenous injection of recombinant adeno-associated virus 9 carrying SIRT3 plasmid (AAV9-SIRT3) could significantly reduce the occurrence of senile osteoporosis in the mouse model (75). Mild hypoxia pretreatment combined with curcumin could improve the mitochondrial function of BMSCs through PGC-1α/SIRT3/HIF-1α signal, and significantly increase cell survival (105).
Zoledronic acid is currently a common drug for the treatment of osteoporosis (106). It could enhance osteogenic differentiation of BMSCs, inhibit osteoclast activity and induce osteoclast apoptosis, thereby alleviating osteoporosis (107, 108). Recent research showed that zoledronic acid might inhibit oxidative stress through the SIRT3/SOD2 pathway to accelerate BMSCs osteogenesis and alleviate the progression of osteoporosis (109).
As an indolamine hormone and a potent free radical scavenger, melatonin could improve osteoporosis through promoting osteoblast differentiation and bone formation (110–112). Mechanism study showed that melatonin could improve mitochondrial oxidative stress through SIRT3/SOD2 signaling pathway, thereby promoting bone formation and improving bone mass loss (113, 114). Also, melatonin could inhibit oxidative damage in preosteoblasts and promote osteogenesis by activating SIRT1, which then regulates the expression of p66Shc and SIRT3 (115).
Resveratrol is a natural polyphenol found in red wine, which can inhibit osteoclast differentiation (116). Matsuda et al. found that resveratrol could alleviate dexamethasone-induced inhibition of BMP2 and OPG expression and mitochondrial dysfunction (117). Moreover, resveratrol might stimulate the SIRT3/PGC-1α/SOD2 axis by activating AMPK, thereby improving mitochondrial dysfunction and protecting osteoblasts against dexamethasone-induced cytotoxicity.
Metformin, a traditional antidiabetic drug, has been shown to have multiple efficacies in the treatment of tumors and aging (118, 119). Metformin may attenuate diabetes-related osteoporosis by improving the hyperglycemic microenvironment (120). And metformin could upregulate SIRT3 expression via PI3K/AKT pathway and reverse H2O2-induced osteoblast apoptosis (121).
Nevertheless, in addition to alleviating the aging of BMSCs and promoting osteogenic differentiation, it was confirmed that SIRT3 could promote osteoclast differentiation and bone resorption in aging male mice. The SIRT3 inhibitor LC-0296 was shown to increase bone mass in aging mice (100). Besides, intervention of osteoclast progenitors in 16-month-old female C57BL/6 mice with LC-0296 reduced osteoclast formation, which was consistent with the results in aged SIRT3-/- mice. From the above-mentioned mechanism of SIRT3 in bone remodeling, the positive or negative effects of SIRT3 may be related to age and gender. In the same way, the use of upregulation and downregulation of SIRT3 to treat osteoporosis needs to be evaluated according to different situations. In the young period, SIRT3 should be upregulated to promote osteogenesis, while in the aging period, SIRT3 should be downregulated to inhibit bone resorption and alleviate osteoporosis. Of course, more animal experiments and clinical studies need to be carried out to confirm this speculation.
Conclusion
Osteoporosis is a common senile disease caused by a variety of factors (112). Since SIRT3 contains an N-terminal mitochondrial signal sequence, it is mainly located in the mitochondria. And SIRT3 has important implications for aging and diseases by regulating mitochondrial biology (122). In this review, SIRT3 could participate in several physiological processes including the senescence and differentiation of BMSCs, and osteoclastogenesis, thereby modulating osteoporosis. In general, SIRT3 alleviates the senescence of BMSCs by regulating oxidative stress, mitophagy, and stabilizing heterochromatin. As for the differentiation of BMSCs, osteoblastogenesis and osteoclastogenesis, the role and mechanism of SIRT3 vary with different conditions, especially age.
In conclusion, SIRT3 plays a vital role in maintaining the balance of bone formation and bone resorption. However, regarding the role of SIRT3 in bone metabolism under physiological and pathological conditions, there is still much to study.
Author Contributions
SW contributed to the conception, design, and final approval of the submitted version. SH contributed to completing the Figure, writing the paper. The authors have read and approved the final manuscript.
Funding
The research was supported by the grant from: National Natural Science Foundation of China (81900441), Natural Science Foundation of Zhejiang Province (LQ19H020002), Zhejiang Provincial Program for Medicine and Health (2022KY446), Social Development Science and Technology Foundation of Taizhou (21ywb115, 21ywb118, 20ywb143), Social Development Science and Technology Foundation of Wenling (2020S0180083, 2021S00156, 2021S00197, 2020S0180127).
Conflict of Interest
The authors declare that the research was conducted in the absence of any commercial or financial relationships that could be construed as a potential conflict of interest.
Publisher’s Note
All claims expressed in this article are solely those of the authors and do not necessarily represent those of their affiliated organizations, or those of the publisher, the editors and the reviewers. Any product that may be evaluated in this article, or claim that may be made by its manufacturer, is not guaranteed or endorsed by the publisher.
References
1. Qaseem A, Forciea MA, McLean RM, Denberg TD, Clinical Guidelines Committee of the American College of Physicians, Barry MJ, et al. Treatment of Low Bone Density or Osteoporosis to Prevent Fractures in Men and Women: A Clinical Practice Guideline Update From the American College of Physicians. . Ann Intern Med (2017) 166(11):818–39. doi: 10.7326/M15-1361
2. Kalinkovich A, Livshits G. Biased and Allosteric Modulation of Bone Cell-Expressing G Protein-Coupled Receptors as a Novel Approach to Osteoporosis Therapy. Pharmacol Res (2021) 171:105794. doi: 10.1016/j.phrs.2021.105794
3. Feng X, McDonald JM. Disorders of Bone Remodeling. Annu Rev Pathol (2011) 6:121–45. doi: 10.1146/annurev-pathol-011110-130203
4. Noel SE, Santos MP, Wright NC. Racial and Ethnic Disparities in Bone Health and Outcomes in the United States. J Bone Miner Res (2021) 36(10):1881–905. doi: 10.1002/jbmr.4417
5. Sozen T, Ozisik L, Basaran NC. An Overview and Management of Osteoporosis. Eur J Rheumatol (2017) 4(1):46–56. doi: 10.5152/eurjrheum.2016.048
6. van de Ven RAH, Santos D, Haigis MC. Mitochondrial Sirtuins and Molecular Mechanisms of Aging. Trends Mol Med (2017) 23(4):320–31. doi: 10.1016/j.molmed.2017.02.005
7. Hu H, Lin Y, Xu X, Lin S, Chen X, Wang S. The Alterations of Mitochondrial DNA in Coronary Heart Disease. Exp Mol Pathol (2020) 114:104412. doi: 10.1016/j.yexmp.2020.104412
8. Schmitt R, Melk A. Molecular Mechanisms of Renal Aging. Kidney Int (2017) 92(3):569–79. doi: 10.1016/j.kint.2017.02.036
9. Das M, Kale V. Involvement of Extracellular Vesicles in Aging Process and Their Beneficial Effects in Alleviating Aging-Associated Symptoms. Cell Biol Int (2021) 45(12):2403–19. doi: 10.1002/cbin.11691
10. Habiballa L, Salmonowicz H, Passos JF. Mitochondria and Cellular Senescence: Implications for Musculoskeletal Ageing. Free Radic Biol Med (2019) 132:3–10. doi: 10.1016/j.freeradbiomed.2018.10.417
11. Altindag O, Erel O, Soran N, Celik H, Selek S. Total Oxidative/Anti-Oxidative Status and Relation to Bone Mineral Density in Osteoporosis. Rheumatol Int (2008) 28(4):317–21. doi: 10.1007/s00296-007-0452-0
12. Domazetovic V, Marcucci G, Iantomasi T, Brandi ML, Vincenzini MT. Oxidative Stress in Bone Remodeling: Role of Antioxidants. Clin cases Miner Bone Metab (2017) 14(2):209–16. doi: 10.11138/ccmbm/2017.14.1.209
13. Agidigbi TS, Kim C. Reactive Oxygen Species in Osteoclast Differentiation and Possible Pharmaceutical Targets of ROS-Mediated Osteoclast Diseases. Int J Mol Sci (2019) 20(14):3576. doi: 10.3390/ijms20143576
14. Hirschey MD, Shimazu T, Goetzman E, Jing E, Schwer B, Lombard DB, et al. SIRT3 Regulates Mitochondrial Fatty-Acid Oxidation by Reversible Enzyme Deacetylation. Nature (2010) 464(7285):121–5. doi: 10.1038/nature08778
15. Park S, Mori R, Shimokawa I. Do Sirtuins Promote Mammalian Longevity? A Critical Review on Its Relevance to the Longevity Effect Induced by Calorie Restriction. Mol Cells (2013) 35(6):474–80. doi: 10.1007/s10059-013-0130-x
16. Chandramowlishwaran P, Vijay A, Abraham D, Li G, Mwangi SM, Srinivasan S. Role of Sirtuins in Modulating Neurodegeneration of the Enteric Nervous System and Central Nervous System. Front Neurosci (2020) 14:614331. doi: 10.3389/fnins.2020.614331
17. Lombard DB, Alt FW, Cheng HL, Bunkenborg J, Streeper RS, Mostoslavsky R, et al. Mammalian Sir2 Homolog SIRT3 Regulates Global Mitochondrial Lysine Acetylation. Mol Cell Biol (2007) 27(24):8807–14. doi: 10.1128/MCB.01636-07
18. Giralt A, Villarroya F. SIRT3, a Pivotal Actor in Mitochondrial Functions: Metabolism, Cell Death and Aging. Biochem J (2012) 444(1):1–10. doi: 10.1042/BJ20120030
19. Lombard DB, Zwaans BM. SIRT3: As Simple as it Seems? Gerontology (2014) 60(1):56–64. doi: 10.1159/000354382
20. Ansari A, Rahman MS, Saha SK, Saikot FK, Deep A, Kim KH. Function of the SIRT3 Mitochondrial Deacetylase in Cellular Physiology, Cancer, and Neurodegenerative Disease. Aging Cell (2017) 16(1):4–16. doi: 10.1111/acel.12538
21. Liu Y, Shen X, Pang M, Sun Z, Qian Y, Xue W, et al. Role of Histone Deacetylase Sirt3 in the Development and Regression of Atherosclerosis. Life Sci (2021) 272:119178. doi: 10.1016/j.lfs.2021.119178
22. Shao J, Liu S, Zhang M, Chen S, Gan S, Chen C, et al. A Dual Role of HIF1alpha in Regulating Osteogenesis-Angiogenesis Coupling. Stem Cell Res Ther (2022) 13(1):59. doi: 10.1186/s13287-022-02742-1
23. Son MJ, Kwon Y, Son T, Cho YS. Restoration of Mitochondrial NAD(+) Levels Delays Stem Cell Senescence and Facilitates Reprogramming of Aged Somatic Cells. Stem Cells (2016) 34(12):2840–51. doi: 10.1002/stem.2460
24. Nguyen GT, Schaefer S, Gertz M, Weyand M, Steegborn C. Structures of Human Sirtuin 3 Complexes With ADP-Ribose and With Carba-NAD+ and SRT1720: Binding Details and Inhibition Mechanism. Acta Crystallogr D Biol Crystallogr (2013) 69(Pt 8):1423–32. doi: 10.1107/S0907444913015448
25. Chen Y, Fu LL, Wen X, Wang XY, Liu J, Cheng Y, et al. Sirtuin-3 (SIRT3), a Therapeutic Target With Oncogenic and Tumor-Suppressive Function in Cancer. Cell Death Dis (2014) 5:e1047. doi: 10.1038/cddis.2014.14
26. Tseng AH, Shieh SS, Wang DL. SIRT3 Deacetylates FOXO3 to Protect Mitochondria Against Oxidative Damage. Free Radic Biol Med (2013) 63:222–34. doi: 10.1016/j.freeradbiomed.2013.05.002
27. Wang S, Zhang J, Deng X, Zhao Y, Xu K. Advances in Characterization of SIRT3 Deacetylation Targets in Mitochondrial Function. Biochimie (2020) 179:1–13. doi: 10.1016/j.biochi.2020.08.021
28. Rahman M, Nirala NK, Singh A, Zhu LJ, Taguchi K, Bamba T, et al. Drosophila Sirt2/mammalian SIRT3 Deacetylates ATP Synthase Beta and Regulates Complex V Activity. J Cell Biol (2014) 206(2):289–305. doi: 10.1083/jcb.201404118
29. Vassilopoulos A, Pennington JD, Andresson T, Rees DM, Bosley AD, Fearnley IM, et al. SIRT3 Deacetylates ATP Synthase F1 Complex Proteins in Response to Nutrient- and Exercise-Induced Stress. Antioxid Redox Signal (2014) 21(4):551–64. doi: 10.1089/ars.2013.5420
30. Hirschey MD, Shimazu T, Capra JA, Pollard KS, Verdin E. SIRT1 and SIRT3 Deacetylate Homologous Substrates: AceCS1,2 and HMGCS1,2. Aging (Albany NY) (2011) 3(6):635–42. doi: 10.18632/aging.100339
31. Finley LW, Haas W, Desquiret-Dumas V, Wallace DC, Procaccio V, Gygi SP, et al. Succinate Dehydrogenase is a Direct Target of Sirtuin 3 Deacetylase Activity. PloS One (2011) 6(8):e23295. doi: 10.1371/journal.pone.0023295
32. Nogueiras R, Habegger KM, Chaudhary N, Finan B, Banks AS, Dietrich MO, et al. Sirtuin 1 and Sirtuin 3: Physiological Modulators of Metabolism. Physiol Rev (2012) 92(3):1479–514. doi: 10.1152/physrev.00022.2011
33. Ahn BH, Kim HS, Song S, Lee IH, Liu J, Vassilopoulos A, et al. A Role for the Mitochondrial Deacetylase Sirt3 in Regulating Energy Homeostasis. Proc Natl Acad Sci USA (2008) 105(38):14447–52. doi: 10.1073/pnas.0803790105
34. Shi T, Wang F, Stieren E, Tong Q. SIRT3, A Mitochondrial Sirtuin Deacetylase, Regulates Mitochondrial Function and Thermogenesis in Brown Adipocytes. J Biol Chem (2005) 280(14):13560–7. doi: 10.1074/jbc.M414670200
35. Sundaresan NR, Gupta M, Kim G, Rajamohan SB, Isbatan A, Gupta MP. Sirt3 Blocks the Cardiac Hypertrophic Response by Augmenting Foxo3a-Dependent Antioxidant Defense Mechanisms in Mice. J Clin Invest (2009) 119(9):2758–71. doi: 10.1172/JCI39162
36. Xie X, Wang L, Zhao B, Chen Y, Li J. SIRT3 Mediates Decrease of Oxidative Damage and Prevention of Ageing in Porcine Fetal Fibroblasts. Life Sci (2017) 177:41–8. doi: 10.1016/j.lfs.2017.01.010
37. He J, Liu X, Su C, Wu F, Sun J, Zhang J, et al. Inhibition of Mitochondrial Oxidative Damage Improves Reendothelialization Capacity of Endothelial Progenitor Cells via SIRT3 (Sirtuin 3)-Enhanced SOD2 (Superoxide Dismutase 2) Deacetylation in Hypertension. Arterioscler Thromb Vasc Biol (2019) 39(8):1682–98. doi: 10.1161/ATVBAHA.119.312613
38. Ploumi C, Daskalaki I, Tavernarakis N. Mitochondrial Biogenesis and Clearance: A Balancing Act. FEBS J (2017) 284(2):183–95. doi: 10.1111/febs.13820
39. Wang S, Deng Z, Ma Y, Jin J, Qi F, Li S, et al. The Role of Autophagy and Mitophagy in Bone Metabolic Disorders. Int J Biol Sci (2020) 16(14):2675–91. doi: 10.7150/ijbs.46627
40. Li Y, Ma Y, Song L, Yu L, Zhang L, Zhang Y, et al. SIRT3 Deficiency Exacerbates P53/Parkinmediated Mitophagy Inhibition and Promotes Mitochondrial Dysfunction: Implication for Aged Hearts. Int J Mol Med (2018) 41(6):3517–26. doi: 10.3892/ijmm.2018.3555
41. Yu W, Gao B, Li N, Wang J, Qiu C, Zhang G, et al. Sirt3 Deficiency Exacerbates Diabetic Cardiac Dysfunction: Role of Foxo3A-Parkin-Mediated Mitophagy. Biochim Biophys Acta Mol Basis Dis (2017) 1863(8):1973–83. doi: 10.1016/j.bbadis.2016.10.021
42. Qiao A, Wang K, Yuan Y, Guan Y, Ren X, Li L, et al. Sirt3-Mediated Mitophagy Protects Tumor Cells Against Apoptosis Under Hypoxia. Oncotarget (2016) 7(28):43390–400. doi: 10.18632/oncotarget.9717
43. He Y, Wu Z, Xu L, Xu K, Chen Z, Ran J, et al. The Role of SIRT3-Mediated Mitochondrial Homeostasis in Osteoarthritis. Cell Mol Life Sci (2020) 77(19):3729–43. doi: 10.1007/s00018-020-03497-9
44. Hadjidakis DJ. And Androulakis, II: Bone Remodeling. Ann NY Acad Sci (2006) 1092:385–96. doi: 10.1196/annals.1365.035
45. Chan WCW, Tan Z, To MKT, Chan D. Regulation and Role of Transcription Factors in Osteogenesis. Int J Mol Sci (2021) 22(11):5445. doi: 10.3390/ijms22115445
46. Sims NA, Martin TJ. Coupling the Activities of Bone Formation and Resorption: A Multitude of Signals Within the Basic Multicellular Unit. Bonekey Rep (2014) 3:481. doi: 10.1038/bonekey.2013.215
47. Wang S, Hu S, Wang J, Liu Y, Zhao R, Tong M, et al. Conditioned Medium From Bone Marrow-Derived Mesenchymal Stem Cells Inhibits Vascular Calcification Through Blockade of the BMP2-Smad1/5/8 Signaling Pathway. Stem Cell Res Ther (2018) 9(1):160. doi: 10.1186/s13287-018-0894-1
48. Wang LT, Liu KJ, Sytwu HK, Yen ML, Yen BL. Advances in Mesenchymal Stem Cell Therapy for Immune and Inflammatory Diseases: Use of Cell-Free Products and Human Pluripotent Stem Cell-Derived Mesenchymal Stem Cells. Stem Cells Transl Med (2021) 10(9):1288–303. doi: 10.1002/sctm.21-0021
49. Fu X, Liu G, Halim A, Ju Y, Luo Q, Song AG. Mesenchymal Stem Cell Migration and Tissue Repair. Cells (2019) 8(8):784. doi: 10.3390/cells8080784
50. Wang Z, Bao HW. Cnidium Lactone Stimulates Osteogenic Differentiation of Bone Marrow Mesenchymal Stem Cells via BMP-2/Smad-Signaling Cascades Mediated by Estrogen Receptor. Am J Transl Res (2019) 11(8):4984–91.
51. Zhang J, Li Q, Yan Y, Sun B, Wang Y, Tang L, et al. Effect of Ciglitazone on Adipogenic Transdifferentiation of Bovine Skeletal Muscle Satellite Cells. J Anim Sci Technol (2021) 63(4):934–53. doi: 10.5187/jast.2021.e87
52. Crockett JC, Rogers MJ, Coxon FP, Hocking LJ, Helfrich MH. Bone Remodelling at a Glance. J Cell Sci (2011) 124(Pt 7):991–8. doi: 10.1242/jcs.063032
53. Han Y, You X, Xing W, Zhang Z, Zou W. Paracrine and Endocrine Actions of Bone-The Functions of Secretory Proteins From Osteoblasts, Osteocytes, and Osteoclasts. Bone Res (2018) 6:16. doi: 10.1038/s41413-018-0019-6
54. Al Rifai O, Chow J, Lacombe J, Julien C, Faubert D, Susan-Resiga D, et al. Proprotein Convertase Furin Regulates Osteocalcin and Bone Endocrine Function. J Clin Invest (2017) 127(11):4104–17. doi: 10.1172/JCI93437
55. Yi SJ, Lee H, Lee J, Lee K, Kim J, Kim Y, et al. Bone Remodeling: Histone Modifications as Fate Determinants of Bone Cell Differentiation. Int J Mol Sci (2019) 20(13):3147. doi: 10.3390/ijms20133147
56. Boyle WJ, Simonet WS, Lacey DL. Osteoclast Differentiation and Activation. Nature (2003) 423(6937):337–42. doi: 10.1038/nature01658
57. Li J, Sarosi I, Yan XQ, Morony S, Capparelli C, Tan HL, et al. RANK is the Intrinsic Hematopoietic Cell Surface Receptor That Controls Osteoclastogenesis and Regulation of Bone Mass and Calcium Metabolism. Proc Natl Acad Sci USA (2000) 97(4):1566–71. doi: 10.1073/pnas.97.4.1566
58. Charles JF, Aliprantis AO. Osteoclasts: More Than 'Bone Eaters'. Trends Mol Med (2014) 20(8):449–59. doi: 10.1016/j.molmed.2014.06.001
59. Dutka M, Bobinski R, Wojakowski W, Francuz T, Pajak C, Zimmer K. Osteoprotegerin and RANKL-RANK-OPG-TRAIL Signalling Axis in Heart Failure and Other Cardiovascular Diseases. Heart Fail Rev (2021). doi: 10.1007/s10741-021-10153-2
60. Chandra A, Rajawat J. Skeletal Aging and Osteoporosis: Mechanisms and Therapeutics. Int J Mol Sci (2021) 22(7):3553. doi: 10.3390/ijms22073553
61. Nakashima T, Hayashi M, Fukunaga T, Kurata K, Oh-Hora M, Feng JQ, et al. Evidence for Osteocyte Regulation of Bone Homeostasis Through RANKL Expression. Nat Med (2011) 17(10):1231–4. doi: 10.1038/nm.2452
62. Sirikul W, Siri-Angkul N, Chattipakorn N, Chattipakorn SC. Fibroblast Growth Factor 23 and Osteoporosis: Evidence From Bench to Bedside. Int J Mol Sci (2022) 23(5):2500. doi: 10.3390/ijms23052500
63. Post S, Abdallah BM, Bentzon JF, Kassem M. Demonstration of the Presence of Independent Pre-Osteoblastic and Pre-Adipocytic Cell Populations in Bone Marrow-Derived Mesenchymal Stem Cells. Bone (2008) 43(1):32–9. doi: 10.1016/j.bone.2008.03.011
64. Boyette LB, Tuan RS. Adult Stem Cells and Diseases of Aging. J Clin Med (2014) 3(1):88–134. doi: 10.3390/jcm3010088
65. Xu Y, An JJ, Tabys D, Xie YD, Zhao TY, Ren HW, et al. Effect of Lactoferrin on the Expression Profiles of Long Non-Coding RNA During Osteogenic Differentiation of Bone Marrow Mesenchymal Stem Cells. Int J Mol Sci (2019) 20(19):4834. doi: 10.3390/ijms20194834
66. Brandl A, Meyer M, Bechmann V, Nerlich M, Angele P. Oxidative Stress Induces Senescence in Human Mesenchymal Stem Cells. Exp Cell Res (2011) 317(11):1541–7. doi: 10.1016/j.yexcr.2011.02.015
67. Ma C, Pi C, Yang Y, Lin L, Shi Y, Li Y, et al. Nampt Expression Decreases Age-Related Senescence in Rat Bone Marrow Mesenchymal Stem Cells by Targeting Sirt1. PloS One (2017) 12(1):e0170930. doi: 10.1371/journal.pone.0170930
68. Wang XQ, Shao Y, Ma CY, Chen W, Sun L, Liu W, et al. Decreased SIRT3 in Aged Human Mesenchymal Stromal/Stem Cells Increases Cellular Susceptibility to Oxidative Stress. J Cell Mol Med (2014) 18(11):2298–310. doi: 10.1111/jcmm.12395
69. Ma C, Sun Y, Pi C, Wang H, Sun H, Yu X, et al. Sirt3 Attenuates Oxidative Stress Damage and Rescues Cellular Senescence in Rat Bone Marrow Mesenchymal Stem Cells by Targeting Superoxide Dismutase 2. Front Cell Dev Biol (2020) 8:599376. doi: 10.3389/fcell.2020.599376
70. Zhang DY, Zhang CF, Fu BC, Sun L, Wang XQ, Chen W, et al. Sirtuin3 Protects Aged Human Mesenchymal Stem Cells Against Oxidative Stress and Enhances Efficacy of Cell Therapy for Ischaemic Heart Diseases. J Cell Mol Med (2018) 22(11):5504–17. doi: 10.1111/jcmm.13821
71. Zhang DY, Gao T, Xu RJ, Sun L, Zhang CF, Bai L, et al. SIRT3 Transfection of Aged Human Bone Marrow-Derived Mesenchymal Stem Cells Improves Cell Therapy-Mediated Myocardial Repair. Rejuvenation Res (2020) 23(6):453–64. doi: 10.1089/rej.2019.2260
72. Naik PP, Birbrair A, Bhutia SK. Mitophagy-Driven Metabolic Switch Reprograms Stem Cell Fate. Cell Mol Life Sci (2019) 76(1):27–43. doi: 10.1007/s00018-018-2922-9
73. Qadir A, Liang S, Wu Z, Chen Z, Hu L, Qian A. Senile Osteoporosis: The Involvement of Differentiation and Senescence of Bone Marrow Stromal Cells. Int J Mol Sci (2020) 21(1):349. doi: 10.3390/ijms21010349
74. Hou Y, Dan X, Babbar M, Wei Y, Hasselbalch SG, Croteau DL, et al. Ageing as a Risk Factor for Neurodegenerative Disease. Nat Rev Neurol (2019) 15(10):565–81. doi: 10.1038/s41582-019-0244-7
75. Guo Y, Jia X, Cui Y, Song Y, Wang S, Geng Y, et al. Sirt3-Mediated Mitophagy Regulates AGEs-Induced BMSCs Senescence and Senile Osteoporosis. Redox Biol (2021) 41:101915. doi: 10.1016/j.redox.2021.101915
76. Ren R, Ocampo A, Liu GH, Izpisua Belmonte JC. Regulation of Stem Cell Aging by Metabolism and Epigenetics. Cell Metab (2017) 26(3):460–74. doi: 10.1016/j.cmet.2017.07.019
77. Zhang W, Qu J, Liu GH, Belmonte JCI. The Ageing Epigenome and Its Rejuvenation. Nat Rev Mol Cell Biol (2020) 21(3):137–50. doi: 10.1038/s41580-019-0204-5
78. Shimazu T, Hirschey MD, Hua L, Dittenhafer-Reed KE, Schwer B, Lombard DB, et al. SIRT3 Deacetylates Mitochondrial 3-Hydroxy-3-Methylglutaryl CoA Synthase 2 and Regulates Ketone Body Production. Cell Metab (2010) 12(6):654–61. doi: 10.1016/j.cmet.2010.11.003
79. Hallows WC, Lee S, Denu JM. Sirtuins Deacetylate and Activate Mammalian Acetyl-CoA Synthetases. Proc Natl Acad Sci USA (2006) 103(27):10230–5. doi: 10.1073/pnas.0604392103
80. Zhang W, Li J, Suzuki K, Qu J, Wang P, Zhou J, et al. Aging Stem Cells. A Werner Syndrome Stem Cell Model Unveils Heterochromatin Alterations as a Driver of Human Aging. Science (2015) 348(6239):1160–3. doi: 10.1126/science.aaa1356
81. Liu J, Ali M, Zhou Q. Establishment and Evolution of Heterochromatin. Ann NY Acad Sci (2020) 1476(1):59–77. doi: 10.1111/nyas.14303
82. Diao Z, Ji Q, Wu Z, Zhang W, Cai Y, Wang Z, et al. SIRT3 Consolidates Heterochromatin and Counteracts Senescence. Nucleic Acids Res (2021) 49(8):4203–19. doi: 10.1093/nar/gkab161
83. Moerman EJ, Teng K, Lipschitz DA, Lecka-Czernik B. Aging Activates Adipogenic and Suppresses Osteogenic Programs in Mesenchymal Marrow Stroma/Stem Cells: The Role of PPAR-Gamma2 Transcription Factor and TGF-Beta/BMP Signaling Pathways. Aging Cell (2004) 3(6):379–89. doi: 10.1111/j.1474-9728.2004.00127.x
84. Jiang Y, Mishima H, Sakai S, Liu YK, Ohyabu Y, Uemura T. Gene Expression Analysis of Major Lineage-Defining Factors in Human Bone Marrow Cells: Effect of Aging, Gender, and Age-Related Disorders. J Orthop Res (2008) 26(7):910–7. doi: 10.1002/jor.20623
85. Denu RA. SIRT3 Enhances Mesenchymal Stem Cell Longevity and Differentiation. Oxid Med Cell Longev (2017) 2017:5841716. doi: 10.1155/2017/5841716
86. Ho L, Wang L, Roth TM, Pan Y, Verdin EM, Hsiao EC, et al. Sirtuin-3 Promotes Adipogenesis, Osteoclastogenesis, and Bone Loss in Aging Male Mice. Endocrinology (2017) 158(9):2741–53. doi: 10.1210/en.2016-1739
87. Rached MT, Kode A, Xu L, Yoshikawa Y, Paik JH, Depinho RA, et al. FoxO1 is a Positive Regulator of Bone Formation by Favoring Protein Synthesis and Resistance to Oxidative Stress in Osteoblasts. Cell Metab (2010) 11(2):147–60. doi: 10.1016/j.cmet.2010.01.001
88. Li Y, Yu C, Shen G, Li G, Shen J, Xu Y, et al. Sirt3-MnSOD Axis Represses Nicotine-Induced Mitochondrial Oxidative Stress and mtDNA Damage in Osteoblasts. Acta Biochim Biophys Sin (Shanghai) (2015) 47(4):306–12. doi: 10.1093/abbs/gmv013
89. Ding Y, Yang H, Wang Y, Chen J, Ji Z, Sun H. Sirtuin 3 is Required for Osteogenic Differentiation Through Maintenance of PGC-1a-SOD2-Mediated Regulation of Mitochondrial Function. Int J Biol Sci (2017) 13(2):254–64. doi: 10.7150/ijbs.17053
90. Gao J, Feng Z, Wang X, Zeng M, Liu J, Han S, et al. SIRT3/SOD2 Maintains Osteoblast Differentiation and Bone Formation by Regulating Mitochondrial Stress. Cell Death Differ (2018) 25(2):229–40. doi: 10.1038/cdd.2017.144
91. Tautzenberger A, Kovtun A, Ignatius A. Nanoparticles and Their Potential for Application in Bone. Int J Nanomed (2012) 7:4545–57. doi: 10.2147/IJN.S34127
92. Chen Z, Wang Y, Ba T, Li Y, Pu J, Chen T, et al. Genotoxic Evaluation of Titanium Dioxide Nanoparticles In Vivo and In Vitro. Toxicol Lett (2014) 226(3):314–9. doi: 10.1016/j.toxlet.2014.02.020
93. Wang S, Yang J, Lin T, Huang S, Ma J, Xu X. Excessive Production of Mitochondrionderived Reactive Oxygen Species Induced by Titanium Ions Leads to Autophagic Cell Death of Osteoblasts via the SIRT3/SOD2 Pathway. Mol Med Rep (2020) 22(1):257–64. doi: 10.3892/mmr.2020.11094
94. Niska K, Pyszka K, Tukaj C, Wozniak M, Radomski MW, Inkielewicz-Stepniak I. Titanium Dioxide Nanoparticles Enhance Production of Superoxide Anion and Alter the Antioxidant System in Human Osteoblast Cells. Int J Nanomed (2015) 10:1095–107. doi: 10.2147/IJN.S73557
95. Zheng K, Bai J, Li N, Li M, Sun H, Zhang W, et al. Protective Effects of Sirtuin 3 on Titanium Particle-Induced Osteogenic Inhibition by Regulating the NLRP3 Inflammasome via the GSK-3beta/Beta-Catenin Signalling Pathway. Bioact Mater (2021) 6(10):3343–57. doi: 10.1016/j.bioactmat.2021.02.039
96. Manolagas SC. Birth and Death of Bone Cells: Basic Regulatory Mechanisms and Implications for the Pathogenesis and Treatment of Osteoporosis. Endocr Rev (2000) 21(2):115–37. doi: 10.1210/edrv.21.2.0395
97. Farr JN, Almeida M. The Spectrum of Fundamental Basic Science Discoveries Contributing to Organismal Aging. J Bone Miner Res (2018) 33(9):1568–84. doi: 10.1002/jbmr.3564
98. Kim H, Lee YD, Kim HJ, Lee ZH, Kim HH. SOD2 and Sirt3 Control Osteoclastogenesis by Regulating Mitochondrial ROS. J Bone Miner Res (2017) 32(2):397–406. doi: 10.1002/jbmr.2974
99. Huh JE, Shin JH, Jang ES, Park SJ, Park DR, Ko R, et al. Sirtuin 3 (SIRT3) Maintains Bone Homeostasis by Regulating AMPK-PGC-1beta Axis in Mice. Sci Rep (2016) 6:22511. doi: 10.1038/srep22511
100. Ling W, Krager K, Richardson KK, Warren AD, Ponte F, Aykin-Burns N, et al. Mitochondrial Sirt3 Contributes to the Bone Loss Caused by Aging or Estrogen Deficiency. JCI Insight (2021) 6(10):e146728. doi: 10.1172/jci.insight.146728
101. Almeida M, Laurent MR, Dubois V, Claessens F, O'Brien CA, Bouillon R, et al. Estrogens and Androgens in Skeletal Physiology and Pathophysiology. Physiol Rev (2017) 97(1):135–87. doi: 10.1152/physrev.00033.2015
102. Richardson KK, Ling W, Krager K, Fu Q, Byrum SD, Pathak R, et al. Ionizing Radiation Activates Mitochondrial Function in Osteoclasts and Causes Bone Loss in Young Adult Male Mice. Int J Mol Sci (2022) 23(2):675. doi: 10.3390/ijms23020675
103. Li N, Li X, Zheng K, Bai J, Zhang W, Sun H, et al. Inhibition of Sirtuin 3 Prevents Titanium Particle-Induced Bone Resorption and Osteoclastsogenesis via Suppressing ERK and JNK Signaling. Int J Biol Sci (2021) 17(5):1382–94. doi: 10.7150/ijbs.53992
104. Buccoliero C, Dicarlo M, Pignataro P, Gaccione F, Colucci S, Colaianni G, et al. The Novel Role of PGC1alpha in Bone Metabolism. Int J Mol Sci (2021) 22(9):4670. doi: 10.3390/ijms22094670
105. Wang X, Shen K, Wang J, Liu K, Wu G, Li Y, et al. Hypoxic Preconditioning Combined With Curcumin Promotes Cell Survival and Mitochondrial Quality of Bone Marrow Mesenchymal Stem Cells, and Accelerates Cutaneous Wound Healing via PGC-1alpha/SIRT3/HIF-1alpha Signaling. Free Radic Biol Med (2020) 159:164–76. doi: 10.1016/j.freeradbiomed.2020.07.023
106. Mochizuki T, Yano K, Ikari K, Okazaki K. Safety and Efficacy of Zoledronic Acid Treatment With and Without Acetaminophen and Eldecalcitol for Osteoporosis. Intern Med (2021) 60(16):2585–91. doi: 10.2169/internalmedicine.6607-20
107. Reid IR. Zoledronate: Efficacy and Safety. J Bone Miner Res (2006) 21 Suppl 2:P83–7. doi: 10.1359/jbmr.06s215
108. Dalle Carbonare L, Mottes M, Malerba G, Mori A, Zaninotto M, Plebani M, et al. Enhanced Osteogenic Differentiation in Zoledronate-Treated Osteoporotic Patients. Int J Mol Sci (2017) 18(6):1261. doi: 10.3390/ijms18061261
109. Jin ZH, Wang SF, Liao W. Zoledronic Acid Accelerates Osteogenesis of Bone Marrow Mesenchymal Stem Cells by Attenuating Oxidative Stress via the SIRT3/SOD2 Pathway and Thus Alleviates Osteoporosis. Eur Rev Med Pharmacol Sci (2020) 24(4):2095–101. doi: 10.26355/eurrev_202002_20389
110. Amstrup AK, Sikjaer T, Heickendorff L, Mosekilde L, Rejnmark L. Melatonin Improves Bone Mineral Density at the Femoral Neck in Postmenopausal Women With Osteopenia: A Randomized Controlled Trial. J Pineal Res (2015) 59(2):221–9. doi: 10.1111/jpi.12252
111. Zhang J, Jia G, Xue P, Li Z. Melatonin Restores Osteoporosis-Impaired Osteogenic Potential of Bone Marrow Mesenchymal Stem Cells and Alleviates Bone Loss Through the HGF/PTEN/Wnt/beta-Catenin Axis. Ther Adv Chronic Dis (2021) 12:2040622321995685. doi: 10.1177/2040622321995685
112. Muzzammil M, Khan AS, Jahanzeb S, Qadir A, Jabbar S. Osteoporosis: Prevalence, Awareness and Pertinent Risk Factors in a Cohort of Elderly Asian Population Presenting With Low-Energy Distal Radius Fractures. Int J Clin Pract (2021) 75(11):e14828. doi: 10.1111/ijcp.14828
113. Zhou W, Liu Y, Shen J, Yu B, Bai J, Lin J, et al. Melatonin Increases Bone Mass Around the Prostheses of OVX Rats by Ameliorating Mitochondrial Oxidative Stress via the SIRT3/SOD2 Signaling Pathway. Oxid Med Cell Longev (2019) 2019:4019619. doi: 10.1155/2019/4019619
114. Xiao L, Lin J, Chen R, Huang Y, Liu Y, Bai J, et al. Sustained Release of Melatonin From GelMA Liposomes Reduced Osteoblast Apoptosis and Improved Implant Osseointegration in Osteoporosis. Oxid Med Cell Longev (2020) 2020:6797154. doi: 10.1155/2020/6797154
115. Liu HD, Ren MX, Li Y, Zhang RT, Ma NF, Li TL, et al. Melatonin Alleviates Hydrogen Peroxide Induced Oxidative Damage in MC3T3-E1 Cells and Promotes Osteogenesis by Activating Sirt1. Free Radic Res (2022) 56(1):63–76. doi: 10.1080/10715762.2022.2037580
116. Matsuda Y, Minagawa T, Okui T, Yamazaki K. Resveratrol Suppresses the Alveolar Bone Resorption Induced by Artificial Trauma From Occlusion in Mice. Oral Dis (2018) 24(3):412–21. doi: 10.1111/odi.12785
117. Wang L, Li Q, Yan H, Jiao G, Wang H, Chi H, et al. Resveratrol Protects Osteoblasts Against Dexamethasone-Induced Cytotoxicity Through Activation of AMP-Activated Protein Kinase. Drug Des Devel Ther (2020) 14:4451–63. doi: 10.2147/DDDT.S266502
118. Bilusic M, Toney NJ, Donahue RN, Wroblewski S, Zibelman M, Ghatalia P, et al. A Randomized Phase 2 Study of Bicalutamide With or Without Metformin for Biochemical Recurrence in Overweight or Obese Prostate Cancer Patients (BIMET-1). Prostate Cancer Prostat Dis (2022). doi: 10.1038/s41391-022-00492-y
119. Glossmann HH, Lutz OMD. Metformin and Aging: A Review. Gerontology (2019) 65(6):581–90. doi: 10.1159/000502257
120. Shen CL, Kaur G, Wanders D, Sharma S, Tomison MD, Ramalingam L, et al. Annatto-Extracted Tocotrienols Improve Glucose Homeostasis and Bone Properties in High-Fat Diet-Induced Type 2 Diabetic Mice by Decreasing the Inflammatory Response. Sci Rep (2018) 8(1):11377. doi: 10.1038/s41598-018-29063-9
121. Yang K, Pei L, Zhou S, Tao L, Zhu Y. Metformin Attenuates H2O2-Induced Osteoblast Apoptosis by Regulating SIRT3 via the PI3K/AKT Pathway. Exp Ther Med (2021) 22(5):1316. doi: 10.3892/etm.2021.10751
Keywords: SIRT3, osteoporosis, bone formation, bone resorption, mitochondria
Citation: Hu S and Wang S (2022) The Role of SIRT3 in the Osteoporosis. Front. Endocrinol. 13:893678. doi: 10.3389/fendo.2022.893678
Received: 10 March 2022; Accepted: 19 April 2022;
Published: 25 May 2022.
Edited by:
Xingming Shi, Augusta University, United StatesReviewed by:
Daniel Lajeunesse, Université de Montréal, CanadaHa-Neui Kim, University of Arkansas for Medical Sciences, United States
Copyright © 2022 Hu and Wang. This is an open-access article distributed under the terms of the Creative Commons Attribution License (CC BY). The use, distribution or reproduction in other forums is permitted, provided the original author(s) and the copyright owner(s) are credited and that the original publication in this journal is cited, in accordance with accepted academic practice. No use, distribution or reproduction is permitted which does not comply with these terms.
*Correspondence: Shuangshuang Wang, d2FuZ3NzMTAyM0AxMjYuY29t