- 1Department of Orthopedics, Xiangya Hospital, Central South University, Changsha, China
- 2Hunan Engineering Research Center of Biomedical Metal and Ceramic Implants, Xiangya Hospital, Central South University, Changsha, China
- 3XiangYa School of Medicine, Central South University, Changsha, China
Osteoporosis is a bone metabolic disorder characterized by decreased bone density and deteriorated microstructure, which increases the risk of fractures. The imbalance between bone formation and bone resorption results in the occurrence and progression of osteoporosis. Osteoblast-mediated bone formation, osteoclast-mediated bone resorption and macrophage-regulated inflammatory response play a central role in the process of bone remodeling, which together maintain the balance of the osteoblast-osteoclast-macrophage (OB-OC-MΦ) axis under physiological conditions. Bone formation and bone resorption disorders caused by the imbalance of OB-OC-MΦ axis contribute to osteoporosis. Many microRNAs are involved in the regulation of OB-OC-MΦ axis homeostasis, with microRNA-23a (miR-23a) being particularly crucial. MiR-23a is highly expressed in the pathological process of osteoporosis, which eventually leads to the occurrence and further progression of osteoporosis by inhibiting osteogenesis, promoting bone resorption and inflammatory polarization of macrophages. This review focuses on the role and mechanism of miR-23a in regulating the OB-OC-MΦ axis to provide new clinical strategies for the prevention and treatment of osteoporosis.
1 Introduction
Osteoporosis is becoming increasingly prevalent with the aging of the world’s population (1). Osteoporosis is a metabolic disease characterized by bone loss, bone density reduction, bone fragility, and increased risk of fractures, and the risk increases with age (2). The dynamic balance between bone formation and resorption underlies bone remodeling, and Osteoporosis tends to occur if an imbalance occurs. At present, osteoporosis has a high disability rate, long treatment cycle, and high cost. A large study in Manitoba, Canada suggested that the cost of treatment of osteoporosis-related fractures or incidental fractures worldwide far exceeds that of many other serious chronic diseases (3, 4). Rehabilitation therapy, including pharmacological and physical therapy, is mainly used, but the clinical outcome is poor (5). Studies have indicated that dysregulation of the OB-OC-MΦ axis is involved in the development of osteoporosis. For example, loss of WNT16 function can lead to increased osteoprotegerin (OPG) expression in osteoblasts, thereby inhibiting osteoclastogenesis, leading to reduced cortical bone mass (6). In addition, chronic inflammation occurring in osteoporosis can derive a range of pro-inflammatory factors, which in turn directly or indirectly promote osteoclast activation (7, 8). Activated macrophages can further secrete pro-inflammatory factors, which induce bone loss by activating osteoclastogenesis (9).
MicroRNAs (miRNAs) are non-coding RNA molecules of about 19 - 22 nucleotides in length that play a vital role in life activities such as cell differentiation, proliferation, and apoptosis. In 1993, the first miRNA was identified as an RNA transcribed from Caenorhabditis elegans. In 2000, the first mammalian miRNA let-7 was discovered, and since then, a series of studies on the genome have revealed many miRNAs and other non-coding RNAs (10). MiR-23a, located on chromosome 19 of the human genome, is transcribed as part of the miR-23a ~ 27a ~ 24-2 cluster. According to the 3’ and 5’ counting rules, the Dicer enzyme cleaves pre-miRNAs from the 3 ‘end and 5’ end positions, respectively, to form mature miRNA-3p and mature miRNA-5p and perform the corresponding functions (11). MiR-23a plays a critical role in inhibiting osteogenesis and promoting osteoclast- and macrophage-mediated inflammation, thereby promoting osteoporosis development. Studies have demonstrated that miR-23a-3p increased in SM-EVs and neoplastic mast cell-derived EVs inhibit osteoblast maturation by suppressing the expression of runt-related transcription factor 2 (Runx2), small mothers against decapentaplegic 1 (SMAD1) and SMAD5 (12). In addition, exosomes containing miR-23a-5p derived from osteoclasts can effectively inhibit ostegenic differentiation and promote the development of osteoporosis by inhibiting Runx2 (13). MiR-23a, which is highly expressed in macrophages, can promote M1 polarization and the generation of pro-inflammatory factors. It accelerates bone resorption by directly or indirectly stimulating osteoclast activation, which encourages the development of osteoporosis (9, 14, 15). Therefore, targeted inhibition of miR-23a to regulate bone metabolic homeostasis is a promising way of treating osteoporosis. At present, however, there are few clinical trials for miR-23a, and whether targeting miR-23a can be used as a bone disease treatment has not been determined. Elucidating the relationship between miR-23a and osteoporosis can not only provide additional tools for clinical identification of patients at risk of osteoporosis, but also provide more information for the development of targeted miRNA therapies as potential interventions to prevent bone loss. Therefore, it is vital for further studies of miR-23a in osteoporosis.
2 OB-OC-MΦ Axis in Osteoporosis
Bone remodeling is a highly coordinated and dynamic process of replacing old or damaged bone, which is necessary to guarantee bone health (16, 17). Under physiological conditions, bone remodeling is regulated by hormones, cytokines and other factors to ensure bone strength and mineral homeostasis (16, 18). OB-OC-MΦ axis is the core of bone remodeling regulation, and its imbalance will usually lead to a variety of bone metabolism diseases, including osteoporosis (Figure 1).
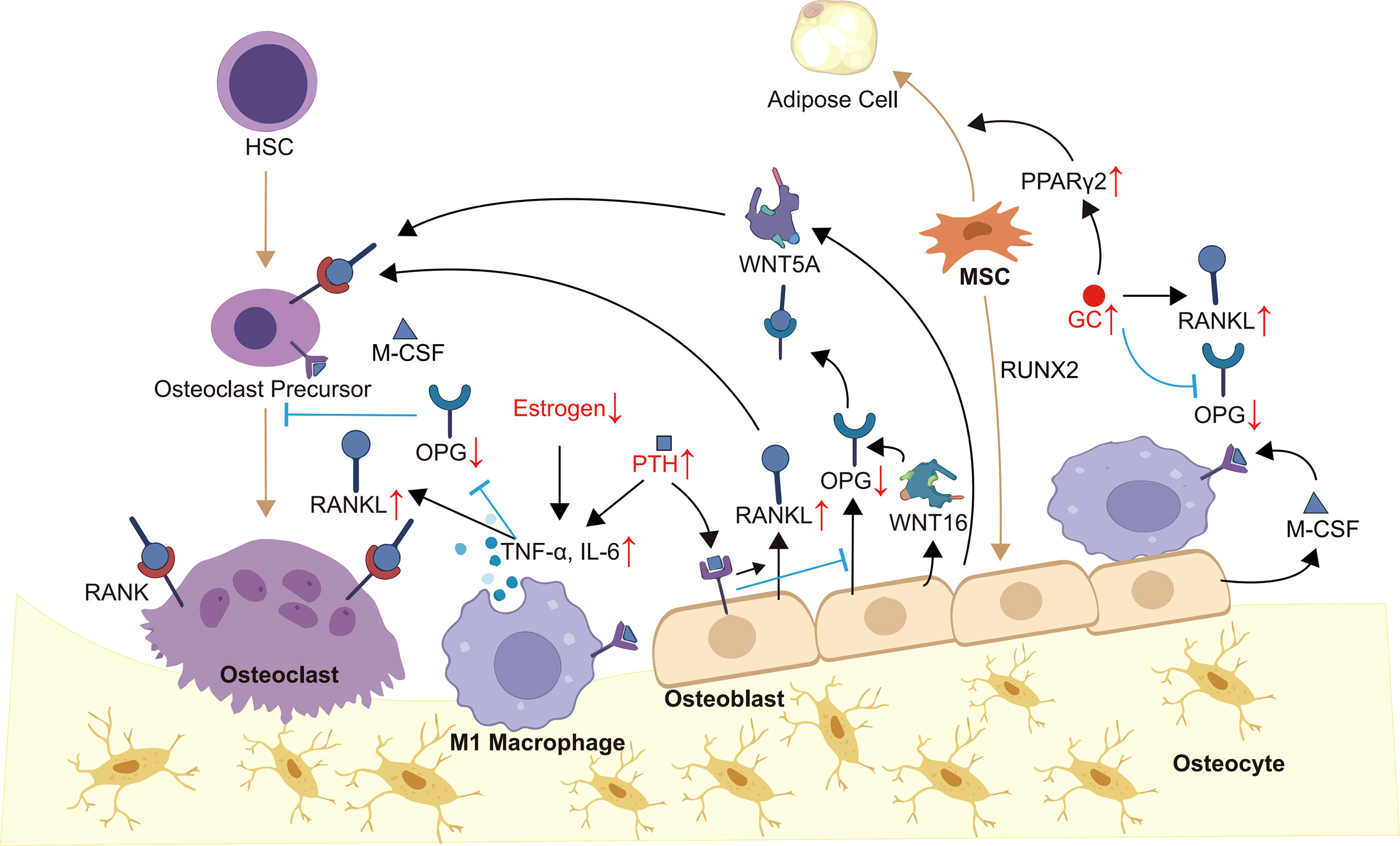
Figure 1 OB-OC-MΦ axis in osteoporosis. Members of the OB-OC-MΦ axis maintain the axis’s equilibrium through intricate communication, which is mostly shown in appropriate levels of cytokines such as RANK, RNAKL, OPG, M-CSF, WNT5A, WNT16, and TNF-α. Endogenous or exogenous factors, such as evident inflammatory responses and aberrant hormone levels, might alter the levels of various cytokines, causing an imbalance of the OB-OC-MΦ axis and, ultimately, the occurrence and progression of osteoporosis. Brown arrows indicate the direction of cell differentiation, and small red arrows indicate abnormal changes in hormone or cytokine levels.
2.1 Composition
Bone tissue cells include osteoprogenitors, osteoblasts, osteocytes, bone lining cells, and osteoclasts, in which osteoblasts, osteoclasts, macrophages and their precursors constitute the OB-OC-MΦ axis. Osteoblasts located on the surface of bone are developed from mesenchymal stem cells (MSCs) and are characterized by the abundant rough endoplasmic reticulum and Golgi complex (19). Osteoblasts play an essential role in bone formation via secreting osteoid (16). Osteoblasts become osteocytes when they are embedded in osteoid, which act as sensors and coordinators of the bone remodeling process (16, 19). Most investigators concur that osteoblasts can recruit osteoclasts and affect their formation and function (15, 20). Mature osteoclasts are multinucleated giant cells derived from hematopoietic stem cells (HSCs) (21, 22), and they can dissolve bone matrix by secreting organic acids and proteolytic enzymes (23). The excessive generation and activation of osteoclasts will lead to pathologic bone loss, which underlies osteoporosis (24). Facts have proved that inflammation is closely related to bone loss (25). Macrophages, known for their phagocytosis, can polarize into two types, M1 and M2, which have pro-inflammatory and anti-inflammatory effects respectively (26, 27). It’s worth mentioning that macrophage polarization can shift between M1 and M2 depending on the local microenvironment (28). Normally, macrophages maintain bone homeostasis in coordination with osteoblasts and osteoclasts by secreting a large number of cytokines (29). For example, M1 macrophages-derived M1 cytokines, including tumor necrosis factor α (TNF-α), IL-1β, IL-6, IL-12 and other pro-inflammatory cytokines, while M2 cytokine library includes IL-4, IL-13, bone morphogenetic protein 2 (BMP-2), transforming growth factor beta (TGF-β), and so on (30). Furthermore, bone resident macrophages such as bone marrow macrophages, osteal macrophages also contribute to bone homeostasis and remodeling.
2.2 Crosstalk Molecular Regulatory Mechanisms
Under physiological conditions, there is a complex and perfect communication system between members of the OB-OC-MΦ axis, which together maintain bone homeostasis through crosstalk in various ways. Table 1 shows the effects of different cell-derived cytokines in the OB-OC-MΦ axis.
2.2.1 Osteoblasts and Osteoclasts
In the process of bone remodeling, osteoblasts and osteoclasts communicate with each other mainly through cell-cell contact and diffusible paracrine factors (56). When osteoblasts and osteoclasts come into contact, gap junctions can be formed, which are beneficial to material and information exchange. Cell-cell contact, in particular, allows bidirectional activation signal transduction via membrane-bound mediators, regulating each other’s differentiation and survival (36). In addition, osteoblasts can release factors to regulate osteoclasts survival and differentiation, such as macrophage colony-stimulating factor (M-CSF, also called CSF1), receptor activator of nuclear factor κ-B (RANK) ligand (RANKL), OPG, WNT5A and WNT16 (36). In terms of mechanism, M-CSF can bind to receptors on osteoclasts and macrophages thereby affecting their survival, differentiation, cell migration and activity (31, 32). RANKL and OPG are secreted by osteoblasts, while RANK presents on the surfaces of osteoclast precursors and mature osteoclasts. The combination of RANKL and RANK will promote the differentiation of osteoclast precursors into osteoclasts and inhibit the apoptosis of osteoclasts (33). OPG secreted by osteoblasts is the decoy receptor of RANK, which inhibits the recruitment and activation of osteoclasts by binding to RANKL (34, 35). Osteoblasts also regulate osteoclastogenesis through WNT signaling pathways. For example, WNT5A expressed by osteoblasts enhances RANK expression through receptor tyrosine kinase-like orphan receptor 2, while WNT16 upregulates OPG (36, 37). In turn, WNT10B and BMPs such as BMP2 and BMP6 secreted by osteoclasts stimulate the recruitment, proliferation and differentiation of osteoblasts (38, 39). Besides, osteoclast-derived semaphorin 4D inhibits insulin-like growth factor-1 (IGF-1) signaling and regulates the motility of osteoblasts by binding to receptors on osteoblasts to inhibit bone formation (40).
2.2.2 Macrophages and Osteoclasts
Macrophages and osteoclasts are different products of myeloid progenitors that compete with each other (57). HSCs differentiate into monocytes/macrophages, followed by the proliferation of pre-osteoclasts and maturation (48). At the moment, it is widely assumed that the monocyte/macrophages are not only the precursors of osteoclasts, but also essential regulators of bone homeostasis. As previously stated, macrophages exhibit distinct polarization in different microenvironments, hence their impacts on osteoclasts differ. Cytokines released by M1 and M2 macrophages can both affect the differentiation and activity of osteoclasts (48). For instance, TNF-α can directly increase the number and/or differentiation of osteoclast progenitor cells, or indirectly promote osteoclast formation by enhancing the secretion of RANKL by osteoblasts and other cells (41–43). IL-1 can stimulate osteoclastogenesis by decreasing OPG levels and increasing RANKL levels (44). IL-6 not only has the ability to stimulate RANKL secretion, but also can mediate the action of TNF-α, and IL-1, and activate JAK/STAT3 (Janus kinase/Signal transducer and activator of transcription 3) pathway to promote osteoclast formation and development (45–47). It cannot be ignored that cytokines produced by M1 macrophages also have negative effects on osteoclasts, for example, IL-12 and IL-18 can inhibit the formation of osteoclasts (48, 49). M2 macrophages can secrete anti-inflammatory factors and bone growth factors, among which IL-10, BMP-2 and osteopontin (OPN) are typical. Studies have proved that IL-10 can inhibit the formation of osteoclasts (58). In mechanism, IL-10 can interfere with NFATc1 expression and nuclear translocation (50), upregulate OPG synthesis, down-regulate RANKL and M-CSF expression (51), and block the expression of pro-osteoclast factors such as IL-1, IL-6 and TNF-α (52) to inhibit osteoclast formation and activity. While BMP-2 promotes the formation, differentiation and activity of osteoclasts by enhancing the synthesis and secretion of RANKL and M-CSF (48, 59). OPN can also improve the activity of osteoclasts and help them adhere to the bone surface (48, 53).
2.2.3 Macrophages, MSCs and Osteoblasts
Resident macrophages and inflammatory macrophages can communicate with MSCs and osteoblasts, which is essential for bone formation. Osteal macrophages, for example, have direct contact with osteoblasts, which can promote bone matrix secretion and mineralization (60). The communication between macrophages and osteoblasts via cytokines was discussed in the preceding section and the relationship between macrophages and MSCs will be emphasized here. It has been proved that early inflammation and the recruitment of monocyte macrophages are required for effective repair (61). The direct contact between M1 macrophages and MSCs can produce oncostatin M (OSM), which is dependent on prostaglandin E2 and cyclooxygenase 2 (54, 62). OSM activates STAT3 phosphorylation, which can regulate osteoblast differentiation via activating Runx2 and inhibiting the expression of sclerostin, an osteocyte-derived mineralization inhibitor (55). What’s more, OSM signaling through STAT3 can directly target WNT5A to promote the differentiation of MSCs into osteoblast (54). More interestingly, STAT3 signaling can lead to the upregulation of OSM receptors to amplify its effects. In turn, macrophages cocultured with MSCs have higher CD206 expression, higher IL-10 and IL-12p40 production, and lower TNF-α, IL-6 and IL-12p70 production (63, 64). It can be guessed that MSCs induce M1 macrophage phenotype to transform into M2 phenotype to coordinate osteogenesis. In summary, the activation of MSCs by inflammatory mediators leads to the transformation of M1 macrophages into M2 phenotype, while OSM derived from M1 macrophages promotes the differentiation of MSCs into osteoblasts.
2.3 Manifestation and Mechanism of OB-OC-MΦ Axis Imbalance in Osteoporosis
The OB-OC-MΦ axis imbalance will result in the onset and progression of osteoporosis, the primary mechanism of which is reduced bone production and increased bone resorption. Osteoporosis pathology is typically accompanied by prominent inflammation and aberrant hormone signaling.
The pathogenesis of osteoporosis is multifactorial, but it is usually accompanied by a local or systemic significant increase of pro-inflammatory cytokines, which further mediates the imbalance of the OB-OC-MΦ axis. For example, the level of TNF-α in patients with osteoporosis is increased. On the one hand, TNF-α induces osteoclastogenesis through the RANK-RANKL-OPG pathway (65). On the other hand, it inhibits osteoblasts by promoting the production of Dickkopf-1 (66). During the development of osteoporosis, the inflammatory cytokine IL-6 is secreted by various cells, such as lymphocytes, macrophages, and osteoblasts. IL-6 initiates signal transduction by forming a complex with the IL-6R and glycoprotein 130 (gp130) (67). In bone metabolism, IL-6 induces the formation and activation of osteoclasts and inhibits their apoptosis, which depends on IL-6R/gp130/RANKL pathway (68–70). Furthermore, other IL-6 cytokine family members, such as IL-11 and cardiotrophin 1, also support osteoclastogenesis (71, 72). To summarize, the inflammatory response in the process of osteoporosis stimulates the osteoclast offset of the OB-OC-MΦ axis, which promotes the disease’s progression.
Aberrant hormone signaling is a major cause of osteoporosis, which can lead to the imbalance of the OB-OC-MΦ axis. It is found that the acceleration of bone loss caused by estrogen deficiency is closely related to the overactivation of the RANK-RANKL pathway, and the incidence rate is higher in menopausal women and people with breast cancer (73, 74). In addition, the occurrence of osteoporosis induced by abnormal estrogen signaling is also related to the amplification of inflammation. Estrogen withdrawal will promote the production of a series of pro-inflammatory cytokines, such as TNF-α, leading to bone loss (75, 76). Parathyroid hormone (PTH) is a crucial regulator of bone development, and patients with hyperparathyroidism are more likely to develop osteoporosis (77, 78). There are PTH receptors on the surface of osteoblasts and osteocytes. PTH can increase the mRNA encoding for RANKL while inhibiting the mRNA encoding for OPG (79), thereby increasing the RANKL/OPG ratio and promoting osteoclast production (45, 80, 81). PTH can also stimulate the production of pro-inflammatory cytokines, such as TNF-α by CD4+ T cells (82). In addition, PTH fosters the production of various acids and enzymes related to bone resorption, such as lactic acid, matrix metalloproteinase 9 (MMP9) and MMP13 (83–85). Glucocorticoid (GC) is commonly used to alleviate inflammation (86), however, it also cause osteoporosis (87). GC directly or indirectly inhibits the generation and function of osteoblasts and induces their apoptosis (88, 89), such as upregulating peroxisome proliferator-activated receptor γ2 (PPARγ2) to differentiate MSCs into adipocytes (87). GC can also increase the RANKL/OPG ratio to promote osteoclastogenesis (88, 90). To summarize, the occurrence of osteoporosis is commonly accompanied by aberrant levels of a range of hormones, which facilitates the progression of osteoporosis by mediating the OB-OC-MΦ axis imbalance.
In summary, the members of the OB-OC-MΦ axis interact and restrict each other in various ways to maintain its balance. The primary mechanism of osteoporosis is the OB-OC-MΦ axis imbalance, which is manifested by the impeded bone formation and enhanced bone resorption. The pathological process of osteoporosis patients is usually accompanied by inflammation or abnormal hormone signaling, which could trigger the imbalance of OB-OC-MΦ axis.
3 Regulation of OB-OC-MΦ Axis by miR-23a
3.1 MiR-23a and Osteoblasts and Osteoclasts
A series of miRNA have been identified to be involved in bone formation and bone resorption (91). For instance, osteoclast-derived exosomal miR-214 can inhibit the activity of osteoblasts by targeting EphrinA2/EphA2 and activating transcription factor 4 (92–94), and plays a key role in osteoclast differentiation via autocrine (95). Exosomal miR-503-3p from osteoblasts has been found to decrease RANK production and RANKL-induced osteoclast differentiation (96). The exosomes or microvesicles derived from various cells contain miR-23a, which is closely related to the OB-OC-MΦ axis. Exosomes can be absorbed by cells through autocrine or paracrine pathways, and can also be absorbed by distant target tissues or organs through circulatory system. In addition to exosomes or extracellular vesicles, endogenous or exogenous factors may also cause the expression level of miR-23a in cells to increase. By targeted regulation of related protein-coding genes, miR-23a can disrupt the balance between osteoblast-mediated bone formation and osteoclast-mediated bone erosion and ultimately contributes to osteoporosis.
MiR-23a mainly inhibits osteogenic differentiation by targeting Runx2, WNT signaling pathway and PGC-1α. Runx2, as the most critical downstream molecule of BMP signaling, is the essential transcription factor of osteoblast formation and the critical driving factor of osteogenesis (97, 98). Runx2 and Wnt/β-catenin signaling promote the differentiation of MSCs into immature osteoblasts while suppressing differentiation into chondrocytes or adipocytes (99). It is worth noting that Runx2 inhibits the maturation of osteoblasts and the transformation of osteoblasts into osteoblasts (99). Bioinformatics prediction and double luciferase reporter gene detection demonstrated that miR-23a can directly bind to the 3’UTR of Runx2 (100), thereby inhibiting osteogenesis (101). Studies have found that patients with systemic mastocytosis are often accompanied by pathological features of osteoporosis. The extracellular vesicles released by mast cells contain a large amount of miR-23a-5p, which has an obvious Runx2 inhibitory effect (12). Exosomes generated from osteoclasts contain a high concentration of miR-23a, which inhibits osteogenic differentiation and osteoblast activity (13). In addition, Runx2 and Stabilin 2 (STAB2) synergistically promote osteoblast differentiation (97). Exogenously expressed miR-23a can target and inhibit SATB2 and indirectly inhibit the osteogenic effect of Runx2 (102). MiR-23a can also inhibit WNT/β-catenin signaling pathway (103), because it directly targets low-density lipoprotein-receptor-related protein 5 (LRP5) (104). Activating the WNT/β-catenin signaling pathway can promote the differentiation of bone marrow MSCs into osteoblasts and prevent bone loss (105). As well as inhibiting RUNX2 in immature osteoblasts, miR-23a can inhibit Prdm16 from regulating TGF-β signaling pathway to promote the terminal differentiation of osteoblasts. What’s more, miR-23a in MSCs from irradiation activated gingival fibroblasts can target C-X-C Motif Chemokine Ligand 12 (CXCL12) to inhibit osteogenic differentiation (106, 107). Besides, miR-23a may also inhibit bone formation through the miR-23a-PGC-1α pathway. According to research, miR-23a can bind to the 3’UTR of PGC-1α and limit its expression (108). PGC-1α determines the fate of the stem cell lineage to differentiate into osteoblasts or adipocytes, and the absence of PGC-1α leads to reduced bone formation and indirect promotion of bone resorption (109). In addition, the expression of PGC-1α decreases with aging (109), which may be one of the reasons why osteoporosis is much more common in the elderly. However, there is no direct evidence that miR-23a regulates the differentiation direction of MSCs by inhibiting PGC-1α. MiR-23a from multiple sources can be absorbed by osteoblast precursors and prevent them from differentiating into osteoblasts, or promote the terminal differentiation of osteoblasts, and finally destroying the balance of OB-OC-MΦ axis.
The function of miR-23a on osteoclasts is still being studied, but we assume that it promotes osteoclastogenesis in most cases, and its effect may depend on the bone microenvironment. It was found that upregulation of miR-23a in bone marrow-derived monocytes/macrophages can directly target and inhibit glycogen synthase kinase-3β (GSK3β) (110). As GSK3β inhibits osteoclast formation induced by RANKL (111), we suspect that miR-23a can promote osteoclastogenesis. The activation of JAK1 and STAT3 in osteocytes or T-cell by inflammation can provide RANKL to promote the formation of osteoclasts (112). JAK1 inhibitor has the effect of inhibiting bone loss (113), which inhibits osteoclastogenesis by repressing the expression of RANKL in osteoblasts (114). It is found that miR-23a can inactivate the JAK1/STAT3 signaling pathway (115), therefore, to some extent, miR-23a may prevent the excessive formation of osteoclasts. To determine if miR-23a can regulate osteoclastogenesis via altering the RANKL/OPG ratio or in other ways, more research is needed (Figure 2A).
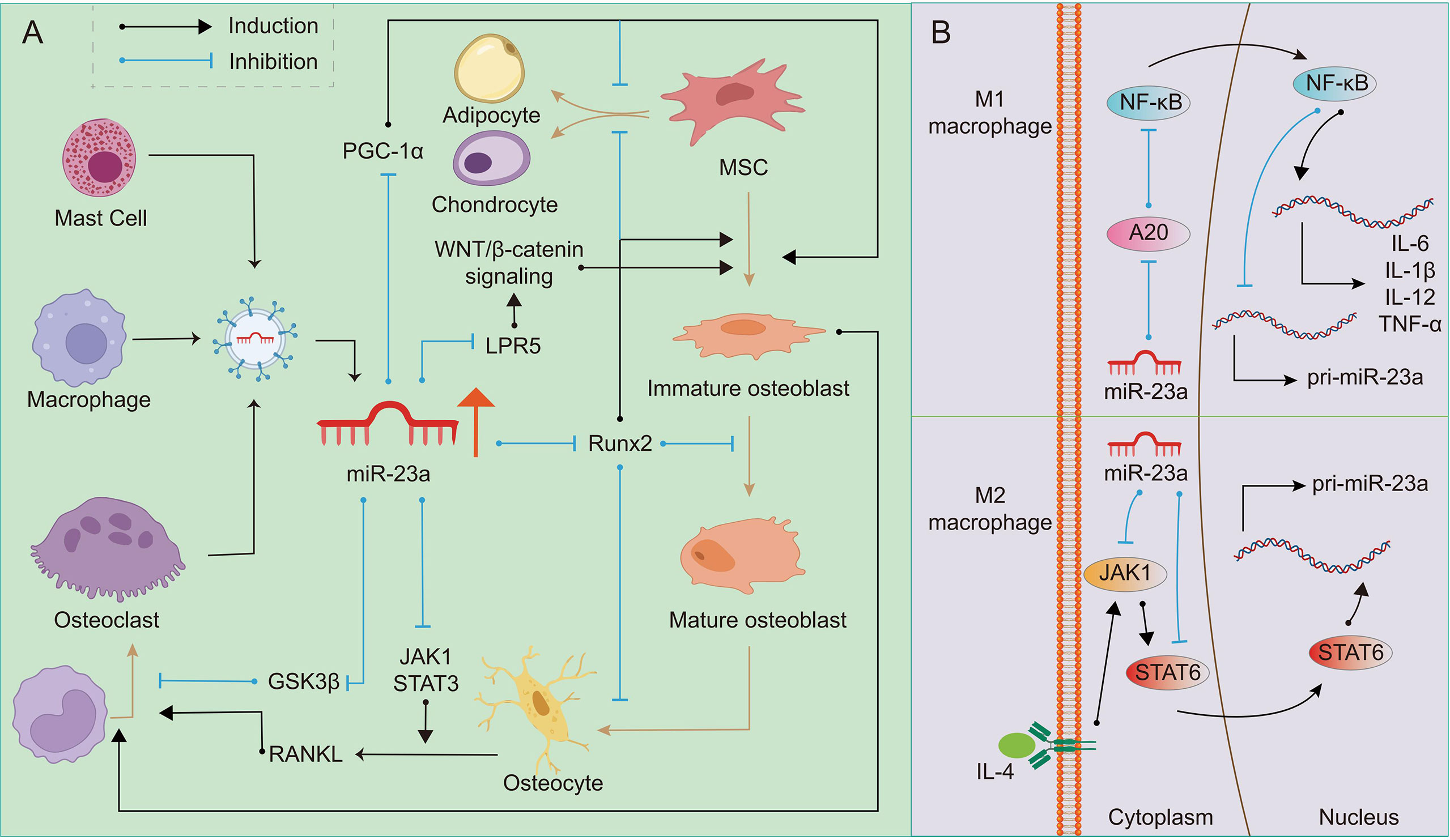
Figure 2 Regulation of miR-23a on OB-OC-MΦ axis. A high level of miR-23a impairs the balance of the OB-OC-MΦ axis, leading to the occurrence of osteoporosis. (A) Many kinds of cells can secrete exosomes or extracellular vesicles containing miR-23a. On the one hand, miR-23a can target Runx2, LPR5 and PGC1 to regulate osteoblast differentiation and maturation; On the other hand, it can target GSK3β and JAK1/STAT3 to regulate osteoclast differentiation. The regulation of miR-23a on osteoblasts can also indirectly affect the formation of osteoclasts. (B) In M1 macrophages, miR-23a specifically inhibits A20, eventually reducing its own expression and promoting the expression of pro-inflammatory factors such as IL-1β, IL-6, IL12 and TNF-α, which promote M1 macrophage polarization and bone resorption. MiR-23a targeted JAK1 and STAT6, and then inhibits the polarization of M2 macrophages.
3.2 MiR-23a and Macrophages
There is a double feedback loop between miR-23a and macrophage polarization (15). It was discovered that stimuli promoting the formation of M1 macrophages might restrict miR-23a expression, but M2 stimulation could boost it. In turn, miR-23a can regulate the polarization of macrophages or the balance between M1 and M2 types (15). The role of the NF-κB signaling in macrophage polarization has been explored, and it has been reported that the activation of this pathway can promote polarization of M1 macrophages while inhibition promotes polarization of M2 macrophages (116). In M1 macrophages, NF-κB binds to miR-23a promoter to inhibit its expression. On the contrary, miR-23a may directly target A20, the upstream inhibitor of NF-κB, to increase M1 macrophage polarization and the expression of M1 cytokines such as IL-1β, IL-6, TNF-α, and IL-12 (15, 117). IL-4/JAK1/STAT6 is an important pathway for the polarization of M2 macrophages. In M2 macrophages, STAT6 can promote the expression of miR-23a by binding to its promoter. However, miR-23a binds to the 3’ UTR of JAK1 or STAT6 to inhibit their expression and thus inhibit the polarization of M2 macrophages (15). In addition, macrophage-derived extracellular vesicles contain miR-23a-3p (118), which may be absorbed by members in the OB-OC-MΦ axis. Although the above research is not based on macrophages in bone microenvironment, based on the important role of macrophages in bone remodeling, it can be speculated that excessive miR-23a may result in the imbalance of the number of M1 and M2 macrophages, and affect other members in OB-OC-MΦ axis. In other words, overexpressed miR-23a may affect the polarization of macrophages, and eventually lead to the destruction of OB-OC-MΦ axis balance and the occurrence and progression of osteoporosis (Figure 2B).
3.3 MiR-23a and OB-OC-MΦ Axis
Members of the OB-OC-MΦ axis or other cells can secrete miR-23a through exosomes or extracellular vesicles, and miR-23a can be absorbed by osteoblasts, osteoclasts, macrophages and their precursors. Some factors can also directly cause miR-23a overexpression in these cells, resulting in OB-OC-MΦ axis imbalance. Table 2 shows the sources, secretion modes, possible target genes and biological effects of miR-23a in bone microenvironment. What’s more, miR-23a may be a possible medium for the communication of members in the OB-OC-MΦ axis, which further enhances the destruction effect of miR-23a on this axis, leading to the occurrence and progression of osteoporosis. Overall, miR-23a plays a major role in regulating the OB-OC-MΦ axis, and targeted control of miR-23a is considered to be a potential strategy for therapeutic therapy of osteoporosis caused by OB-OC- MΦ axis imbalance.
4 MicroRNAs and Bone Diseases
4.1 Other microRNAs and OB-OC-MΦ Axis
MicroRNAs have developed into an important regulatory factor necessary to maintain bone homeostasis, which is of great significance to bone health and diseases (119). In addition to miR-23a, there are many microRNAs involved in the regulation of the OB-OC-MΦ axis, and there are synergistic or antagonistic effects among them.
Besides miR-23a, the extracellular vesicles released by neoplastic mast cells contain miR-30a, which can also inhibit Runx2 and SMAD1/5, and inhibit osteoblast differentiation and bone formation (12). MiR-17 and miR-31 are expressed in the process of osteogenic differentiation of adipose-derived stem cells (ASCs), and have inhibitory effects on osteogenesis mediated by BMP2 (101). This effect similar to miR-23a is related to miR-17 inhibiting BMP-2 expression and miR-31 inhibiting Osterix expression. What’s more, miR-103-3p directly targets methyltransferase-like 14 to inhibit osteoblast activity and bone formation (120).
In the process of OB-OC-MΦ axis regulation, there are also many microRNAs that have antagonistic effects with miR-23a. For instance, during the osteogenic differentiation of ASCs, the expression of endogenous miR-26a increases, which promotes osteoblast formation by inhibiting GSK3β (121). MiR-375 targets YAP1 (Yes-associated protein 1) to promote the osteogenic differentiation of ASCs (122); In MSCs, the overexpression of miR-130a can promote the osteogenic differentiation of MSCs and inhibit the formation of adipocytes, which is related to the fact that miR-130a directly binds the 3’UTR of Smad regulatory factors 2 and PPARγ (123). MiR-1246 activates the STAT3 signaling pathway and inhibits the NF-κB signaling pathway by targeting TERF2IP (telomeric repeat-binding factor 2-interacting protein) to induce M2 macrophage polarization (124). The inhibition of NF-κB signaling will reduce the production of pro-inflammatory cytokines, which further affects the OB-OC-MΦ axis.
It should not be ignored that there are complex positive and negative feedback loops between these miRNA and their targets (125). For example, miR-23a and A20/NF-κB, miR-23a and JAK1/STAT6, and miR-375 and YAP1 (15, 122). Many factors work together to maintain the balance of microRNAs and consequently the OB-OC-MΦ axis. When the expression leve of microRNAs is aberrant, the OB-OC-MΦ axis is destroyed inevitably, resulting in an imbalance between bone formation and bone absorption and, ultimately, increasing the incidence and progression of bone diseases such as osteoporosis.
4.2 MiR-23a and Other Bone Diseases
MiR-23a is implicated in the incidence and progression of various bone diseases, in addition to impacting osteoporosis by disrupting the balance of the OB-OC-MΦ axis. In rheumatoid arthritis, miR-23a can reduce bone loss and increase calcium retention by inhibiting the expression of LRP5, thus inhibiting the canonical WNT signaling pathway in cells (126). In osteosarcoma tissues and cells, miR-23a expression is decreased due to hypermethylation of promoter (127). The restoration of miR-23a expression can delay osteosarcoma cell proliferation, migration, and invasion. Connexin-43 is continually up-regulated during osteoblast differentiation, and its expression is also enhanced during human osteosarcoma cell differentiation. It has been revealed that miR-23a can target connexin-43 to prevent bone differentiation in osteosarcoma (128). Certainly, miR-23a can also inhibit the expression of other genes, such as Runx2 and CXCL12, to inhibit the progression of osteosarcoma (127).
MiR-23a may also affect bone diseases through synergistic or antagonistic effects of other microRNAs. For example, in osteonecrosis, miR-122-5p inhibits Sprouty2 and enhances the activity of receptor tyrosine kinase, thus promoting the proliferation and differentiation of osteoblasts (129). Similar to miR-23a, miR-137-3p targets Runx2 and CXCL12, and its silencing can promote bone formation and angiogenesis, thus preventing osteonecrosis. MiR-23a is an important member of the bone-related microRNA network. Targeted regulation of its expression has potential value for restoring the balance of OB-OC-MΦ axis and preventing and treating various bone diseases including osteoporosis.
5 Summary and Prospect
Osteoporosis is a disease that increases fracture risk due to decreased bone density. Osteoporosis will not only cost substantial medical care costs but also seriously reduce the life quality of patients and increase the mortality rate (130). Therefore, getting timely prevention and treatment is of great clinical and practical significance. In terms of mechanism, osteoporosis is mainly caused by bone resorption that is stronger than bone formation during bone remodeling. We first proposed the concept of the OB-OC-MΦ axis, pointed out the close connection between it and osteoporosis, and expounded the crucial role of miR-23a in osteoporosis induced by the imbalance of the OB-OC-MΦ axis.
Osteoblasts, osteoclasts, macrophages, and other members in the OB-OC-MΦ axis interact through direct contact, autocrine or paracrine to regulate bone homeostasis. Pathologically, the abnormal levels of inflammatory cytokines and hormones lead to the imbalance of the OB-OC-MΦ axis, enhanced bone resorption and inhibited bone formation, which promotes the occurrence and progression of osteoporosis. Similarly, the role of miR-23a in the occurrence and progression of osteoporosis is also realized through the OB-OC-MΦ axis. MiR-23a can inhibit bone formation by targeting the expression of genes encoding essential proteins in the process of osteoblast formation (101), among which miR-23a-Runx2, miR-23a-PGC-1α and miR23a-Wnt/β-catenin are the key ways. The high expression of miR-23a in macrophages promotes the polarization of M1 macrophages, stimulates the secretion of a variety of pro-inflammatory cytokines, and inhibits the polarization of M2 macrophages (14, 15). A variety of pro-inflammatory cytokines secreted by M1 macrophages, not only can inhibit the formation of osteoblasts, but also promote osteoclastogenesis (131). Simply put, the multiple effects of miR-23a on the OB-OC-MΦ axis make it easier for osteoporosis to occur when the level of miR-23a is high. Based on the multi-target and multi-pathway regulation effect of miR-23a on the OB-OC-MΦ axis, targeting miR-23a is expected to become an emerging potential and highly effective prevention and treatment strategy for osteoporosis.
Clinical trials targeting miRNAs are currently in full swing. As of 2018, more than 100 clinical trials have been initiated worldwide focusing on miRNAs for the treatment of psoriasis, cardiovascular disease, and cancer. In addition, miRNAs have been shown to be involved in various osteoclast-related diseases (132). Studies have shown that anti-miR-21 oligonucleotide inhibits the activity of miR-21 in aseptic loosening after arthroplasty, and the use of antagomir-21 in mouse tissues with particle-induced osteolysis improves osteolytic symptoms (133). In rheumatoid arthritis, regulating the expression of miRNAs such as miR-146a, miR-223, and miR-155 in peripheral blood mononuclear cells is a potential target for the treatment of rheumatoid arthritis (134–136).
Some clinical studies have been conducted to target miRNAs for the treatment of osteoporosis. Zoledronic acid (ZOL) inhibits the expression of RANKL by regulating miR-101-3p/RANKL, miR-302/PRKACB/RANKL and miR-145/SMAD3/RANKL signaling pathways. In HIV-positive subjects treated with tenofovir, down-regulation of RANKL expression by ZOL treatment alleviates osteoporosis (137). In patients with degenerative lumbar disease treated with posterior lumbar intervertebral fusion with cages, treatment with pluronic nanoparticles and oligosaccharide nanomedicine of alginate sodium reduces the occurrence of osteoporosis by regulating miR-155 (138). There are few clinical trials targeting miR-23a in bone disease, and only some studies have shown that icariin promotes osteogenic differentiation of bone marrow stem cells and improves osteonecrosis of the femoral head by reducing miR-23a-3p levels and regulating BMP-2/Smad5/Runx2 and WNT/β-catenin pathways (139). At present, there is no clinical trial targeting miR-23a for the treatment of osteoporosis. However, in view of the effect of miR-23a on inhibiting osteogenesis and promoting osteoclast- and macrophage-mediated inflammation, inhibiting the expression of miR-23a may become a crucial method for the treatment of osteoporosis. Further study of miR-23a may bring a new clinical horizon for the treatment of osteoporosis.
Detection of the expression of miR-23a in cells or tissues and targeted regulation of miR-23a provide potential strategies for the prevention and treatment of osteoporosis. However, the outcome of tailored modulation of miR-23a expression or cutting off relevant signal pathways in the prevention and treatment of osteoporosis, as well as the potential negative effects on the body, remains to be investigated. In general, the critical regulatory role of miR-23a in the OB-OC-MΦ axis provides a solid and sufficient theoretical basis for the multidisciplinary and multi-modal prevention and treatment of osteoporosis centered on miR-23a. With the in-depth study of miR-23a in osteoporosis, its clinical potential will be explored and applied.
Author Contributions
T-LM and PZ wrote the original draft. T-LM, PZ, Z-RK and J-XC participated in writing and editing the review. PZ prepared the figures. Y-HH and JX edited the manuscript. All authors read and approved the final manuscript.
Funding
This work was supported by the National Natural Science Foundation of China (Grant No. 81974339), the Science and Technology Plan Project of Hunan Province (Grant Nos. 2019JJ40499), and the National University Student Innovation Program (S2021105330596).
Conflict of Interest
The authors declare that the research was conducted in the absence of any commercial or financial relationships that could be construed as a potential conflict of interest.
Publisher’s Note
All claims expressed in this article are solely those of the authors and do not necessarily represent those of their affiliated organizations, or those of the publisher, the editors and the reviewers. Any product that may be evaluated in this article, or claim that may be made by its manufacturer, is not guaranteed or endorsed by the publisher.
Acknowledgments
We thank Y-HH and JX for the critical reading of the manuscript. We apologize to those authors whose primary work we did not directly reference due to space restrictions.
Abbreviations
ASC, adipose-derived stem cell; BMP, bone morphogenetic protein; CXCL12, C-X-C motif chemokine ligand 12; GC, glucocorticoid; gp130, glycoprotein 130; GSK3β, glycogen synthase kinase-3β; HSC, hematopoietic stem cell; IGF-1, insulin-like growth factor-1; JAK, Janus kinase; LRP5, low-density lipoprotein-receptor-related protein 5; M-CSF, macrophage colony-stimulating factor; miR-23a, microRNA-23a; miRNA, microRNA; MSC, mesenchymal stem cell; OB-OC-MΦ axis, osteoblast-osteoclast-macrophage axis; OPG, osteoprotegerin; OPN, osteopontin; OSM, oncostatin M; PPARγ2, peroxisome proliferator-activated receptor γ2; PTH, parathyroid hormone; RANK, receptor activator of nuclear factor κ-B; RANKL, receptor activator of nuclear factor κ-B ligand; Runx2, runt-related transcription factor 2; SMAD1, small mothers against decapentaplegic 1; STAB2, Stabilin 2; STAT3, signal transducer and activator of transcription 3; TGF-β, transforming growth factor β; TNF-α, tumor necrosis factor α; YAP1, Yes-associated protein 1.
References
1. Lane NE. Epidemiology, Etiology, and Diagnosis of Osteoporosis. Am J Obstet Gynecol (2006) 194(2 Suppl):S3–11. doi: 10.1016/j.ajog.2005.08.047
2. Chen S, Liu D, Zhou Z, Qin S. Role of Long Non-Coding RNA H19 in the Development of Osteoporosis. Mol Med (2021) 27(1):122. doi: 10.1186/s10020-021-00386-0
3. Singer A, Exuzides A, Spangler L, O'Malley C, Colby C, Johnston K, et al. Burden of Illness for Osteoporotic Fractures Compared With Other Serious Diseases Among Postmenopausal Women in the United States. Mayo Clin Proc (2015) 90(1):53–62. doi: 10.1016/j.mayocp.2014.09.011
4. Watts NB. Insights From the Global Longitudinal Study of Osteoporosis in Women (GLOW). Nat Rev Endocrinol (2014) 10(7):412–22. doi: 10.1038/nrendo.2014.55
5. Tella SH, Gallagher JC. Prevention and Treatment of Postmenopausal Osteoporosis. J Steroid Biochem Mol Biol (2014) 142:155–70. doi: 10.1016/j.jsbmb.2013.09.008
6. Trajanoska K, Rivadeneira F. The Genetic Architecture of Osteoporosis and Fracture Risk. Bone (2019) 126:2–10. doi: 10.1016/j.bone.2019.04.005
7. Souza PPC, Lerner UH. The Role of Cytokines in Inflammatory Bone Loss. Immunol Invest (2013) 42(7):555–622. doi: 10.3109/08820139.2013.822766
8. Kim JH, Jin HM, Kim K, Song I, Youn BU, Matsuo K, et al. The Mechanism of Osteoclast Differentiation Induced by IL-1. J Immunol (2009) 183(3):1862–70. doi: 10.4049/jimmunol.0803007
9. Yang D-H, Yang M-Y. The Role of Macrophage in the Pathogenesis of Osteoporosis. Int J Mol Sci (2019) 20(9):2093. doi: 10.3390/ijms20092093
10. Rupaimoole R, Slack FJ. MicroRNA Therapeutics: Towards a New Era for the Management of Cancer and Other Diseases. Nat Rev Drug Discov (2017) 16(3):203–22. doi: 10.1038/nrd.2016.246
11. Ha M, Kim VN. Regulation of microRNA Biogenesis. Nat Rev Mol Cell Biol (2014) 15(8):509–24. doi: 10.1038/nrm3838
12. Kim DK, Bandara G, Cho YE, Komarow HD, Donahue DR, Karim B, et al. Mastocytosis-Derived Extracellular Vesicles Deliver miR-23a and miR-30a Into Pre-Osteoblasts and Prevent Osteoblastogenesis and Bone Formation. Nat Commun (2021) 12(1):2527. doi: 10.1038/s41467-021-22754-4
13. Yang J-X, Xie P, Li Y-S, Wen T, Yang X-C. Osteoclast-Derived miR-23a-5p-Containing Exosomes Inhibit Osteogenic Differentiation by Regulating Runx2. Cell Signal (2020) 70:109504. doi: 10.1016/j.cellsig.2019.109504
14. Luers AJ, Loudig OD, Berman JW. MicroRNAs Are Expressed and Processed by Human Primary Macrophages. Cell Immunol (2010) 263(1):1–8. doi: 10.1016/j.cellimm.2010.03.011
15. Ma S, Liu M, Xu Z, Li Y, Guo H, Ge Y, et al. A Double Feedback Loop Mediated by microRNA-23a/27a/24-2 Regulates M1 Versus M2 Macrophage Polarization and Thus Regulates Cancer Progression. Oncotarget (2016) 7(12):13502–19. doi: 10.18632/oncotarget.6284
16. Florencio-Silva R, Sasso GR, Sasso-Cerri E, Simões MJ, Cerri PS. Biology of Bone Tissue: Structure, Function, and Factors That Influence Bone Cells. BioMed Res Int (2015) 2015:421746. doi: 10.1155/2015/421746
17. Sims NA, Gooi JH. Bone Remodeling: Multiple Cellular Interactions Required for Coupling of Bone Formation and Resorption. Semin Cell Dev Biol (2008) 19(5):444–51. doi: 10.1016/j.semcdb.2008.07.016
18. Katsimbri P. The Biology of Normal Bone Remodelling. Eur J Cancer Care (Engl) (2017) 26(6):10.1111/ecc.12740. doi: 10.1111/ecc.12740
19. Raggatt LJ, Partridge NC. Cellular and Molecular Mechanisms of Bone Remodeling. J Biol Chem (2010) 285(33):25103–8. doi: 10.1074/jbc.R109.041087
20. Capulli M, Paone R, Rucci N. Osteoblast and Osteocyte: Games Without Frontiers. Arch Biochem Biophys (2014) 561:3–12. doi: 10.1016/j.abb.2014.05.003
21. Boyle WJ, Simonet WS, Lacey DL. Osteoclast Differentiation and Activation. Nature (2003) 423(6937):337–42. doi: 10.1038/nature01658
22. Matsuura T, Ichinose S, Akiyama M, Kasahara Y, Tachikawa N, Nakahama KI. Involvement of CX3CL1 in the Migration of Osteoclast Precursors Across Osteoblast Layer Stimulated by Interleukin-1ß. J Cell Physiol (2017) 232(7):1739–45. doi: 10.1002/jcp.25577
23. Faulkner B, Astleford K, Mansky KC. Regulation of Osteoclast Differentiation and Skeletal Maintenance by Histone Deacetylases. Molecules (2019) 24(7):1355. doi: 10.3390/molecules24071355
24. Fujii T, Murata K, Mun SH, Bae S, Lee YJ, Pannellini T, et al. MEF2C Regulates Osteoclastogenesis and Pathologic Bone Resorption via C-FOS. Bone Res (2021) 9(1):4. doi: 10.1038/s41413-020-00120-2
25. Muthukuru M, Darveau RP. TLR Signaling That Induces Weak Inflammatory Response and SHIP1 Enhances Osteogenic Functions. Bone Res (2014) 2:14031. doi: 10.1038/boneres.2014.31
26. Michalski MN, McCauley LK. Macrophages and Skeletal Health. Pharmacol Ther (2017) 174:43–54. doi: 10.1016/j.pharmthera.2017.02.017
27. Shapouri-Moghaddam A, Mohammadian S, Vazini H, Taghadosi M, Esmaeili SA, Mardani F, et al. Macrophage Plasticity, Polarization, and Function in Health and Disease. J Cell Physiol (2018) 233(9):6425–40. doi: 10.1002/jcp.26429
28. Liu H, Wu X, Gang N, Wang S, Deng W, Zan L, et al. Macrophage Functional Phenotype Can Be Consecutively and Reversibly Shifted to Adapt to Microenvironmental Changes. Int J Clin Exp Med (2015) 8(2):3044–53.
29. Pieters BCH, Cappariello A, van den Bosch MHJ, van Lent P, Teti A, van de Loo FAJ. Macrophage-Derived Extracellular Vesicles as Carriers of Alarmins and Their Potential Involvement in Bone Homeostasis. Front Immunol (2019) 10:1901. doi: 10.3389/fimmu.2019.01901
30. Muñoz J, Akhavan NS, Mullins AP, Arjmandi BH. Macrophage Polarization and Osteoporosis: A Review. Nutrients (2020) 12(10):2999. doi: 10.3390/nu12102999
31. Kim JH, Kim N. Signaling Pathways in Osteoclast Differentiation. Chonnam Med J (2016) 52(1):12–7. doi: 10.4068/cmj.2016.52.1.12
32. Wiktor-Jedrzejczak W, Bartocci A, Ferrante AW Jr., Ahmed-Ansari A, Sell KW, Pollard JW, et al. Total Absence of Colony-Stimulating Factor 1 in the Macrophage-Deficient Osteopetrotic (Op/Op) Mouse. Proc Natl Acad Sci U S A (1990) 87(12):4828–32. doi: 10.1073/pnas.87.12.4828
33. Ono T, Hayashi M, Sasaki F, Nakashima T. RANKL Biology: Bone Metabolism, the Immune System, and Beyond. Inflammation Regen (2020) 40:2. doi: 10.1186/s41232-019-0111-3
34. Udagawa N, Koide M, Nakamura M, Nakamichi Y, Yamashita T, Uehara S, et al. Osteoclast Differentiation by RANKL and OPG Signaling Pathways. J Bone Miner Metab (2021) 39(1):19–26. doi: 10.1007/s00774-020-01162-6
35. Theoleyre S, Wittrant Y, Tat SK, Fortun Y, Redini F, Heymann D. The Molecular Triad OPG/RANK/RANKL: Involvement in the Orchestration of Pathophysiological Bone Remodeling. Cytokine Growth Factor Rev (2004) 15(6):457–75. doi: 10.1016/j.cytogfr.2004.06.004
36. Kim JM, Lin C, Stavre Z, Greenblatt MB, Shim JH. Osteoblast-Osteoclast Communication and Bone Homeostasis. Cells (2020) 9(9):2073. doi: 10.3390/cells9092073
37. Maeda K, Kobayashi Y, Udagawa N, Uehara S, Ishihara A, Mizoguchi T, et al. Wnt5a-Ror2 Signaling Between Osteoblast-Lineage Cells and Osteoclast Precursors Enhances Osteoclastogenesis. Nat Med (2012) 18(3):405–12. doi: 10.1038/nm.2653
38. Han Y, You X, Xing W, Zhang Z, Zou W. Paracrine and Endocrine Actions of Bone-the Functions of Secretory Proteins From Osteoblasts, Osteocytes, and Osteoclasts. Bone Res (2018) 6:16. doi: 10.1038/s41413-018-0019-6
39. Kreja L, Brenner RE, Tautzenberger A, Liedert A, Friemert B, Ehrnthaller C, et al. Non-Resorbing Osteoclasts Induce Migration and Osteogenic Differentiation of Mesenchymal Stem Cells. J Cell Biochem (2010) 109(2):347–55. doi: 10.1002/jcb.22406
40. Negishi-Koga T, Shinohara M, Komatsu N, Bito H, Kodama T, Friedel RH, et al. Suppression of Bone Formation by Osteoclastic Expression of Semaphorin 4D. Nat Med (2011) 17(11):1473–80. doi: 10.1038/nm.2489
41. Kobayashi K, Takahashi N, Jimi E, Udagawa N, Takami M, Kotake S, et al. Tumor Necrosis Factor Alpha Stimulates Osteoclast Differentiation by a Mechanism Independent of the ODF/RANKL-RANK Interaction. J Exp Med (2000) 191(2):275–86. doi: 10.1084/jem.191.2.275
42. Zhao Z, Hou X, Yin X, Li Y, Duan R, Boyce BF, et al. TNF Induction of NF-κb RelB Enhances RANKL-Induced Osteoclastogenesis by Promoting Inflammatory Macrophage Differentiation But Also Limits It Through Suppression of NFATc1 Expression. PloS One (2015) 10(8):e0135728. doi: 10.1371/journal.pone.0135728
43. Horwood NJ, Elliott J, Martin TJ, Gillespie MT. Osteotropic Agents Regulate the Expression of Osteoclast Differentiation Factor and Osteoprotegerin in Osteoblastic Stromal Cells. Endocrinology (1998) 139(11):4743–6. doi: 10.1210/endo.139.11.6433
44. Hofbauer LC, Lacey DL, Dunstan CR, Spelsberg TC, Riggs BL, Khosla S. Interleukin-1beta and Tumor Necrosis Factor-Alpha, But Not Interleukin-6, Stimulate Osteoprotegerin Ligand Gene Expression in Human Osteoblastic Cells. Bone (1999) 25(3):255–9. doi: 10.1016/S8756-3282(99)00162-3
45. O'Brien CA, Nakashima T, Takayanagi H. Osteocyte Control of Osteoclastogenesis. Bone (2013) 54(2):258–63. doi: 10.1016/j.bone.2012.08.121
46. Wu Q, Zhou X, Huang D, Ji Y, Kang F. IL-6 Enhances Osteocyte-Mediated Osteoclastogenesis by Promoting JAK2 and RANKL Activity In Vitro. Cell Physiol Biochem (2017) 41(4):1360–9. doi: 10.1159/000465455
47. Devlin RD, Reddy SV, Savino R, Ciliberto G, Roodman GD. IL-6 Mediates the Effects of IL-1 or TNF, But Not PTHrP or 1,25(OH)2D3, on Osteoclast-Like Cell Formation in Normal Human Bone Marrow Cultures. J Bone Miner Res (1998) 13(3):393–9. doi: 10.1359/jbmr.1998.13.3.393
48. Sun Y, Li J, Xie X, Gu F, Sui Z, Zhang K, et al. Macrophage-Osteoclast Associations: Origin, Polarization, and Subgroups. Front Immunol (2021) 12:778078. doi: 10.3389/fimmu.2021.778078
49. Horwood NJ, Elliott J, Martin TJ, Gillespie MT. IL-12 Alone and in Synergy With IL-18 Inhibits Osteoclast Formation In Vitro. J Immunol (2001) 166(8):4915–21. doi: 10.4049/jimmunol.166.8.4915
50. Evans KE, Fox SW. Interleukin-10 Inhibits Osteoclastogenesis by Reducing NFATc1 Expression and Preventing Its Translocation to the Nucleus. BMC Cell Biol (2007) 8:4. doi: 10.1186/1471-2121-8-4
51. Liu D, Yao S, Wise GE. Effect of Interleukin-10 on Gene Expression of Osteoclastogenic Regulatory Molecules in the Rat Dental Follicle. Eur J Oral Sci (2006) 114(1):42–9. doi: 10.1111/j.1600-0722.2006.00283.x
52. Houri-Haddad Y, Soskolne WA, Halabi A, Shapira L. IL-10 Gene Transfer Attenuates P. Gingivalis-Induced Inflammation. J Dent Res (2007) 86(6):560–4. doi: 10.1177/154405910708600614
53. Icer MA, Gezmen-Karadag M. The Multiple Functions and Mechanisms of Osteopontin. Clin Biochem (2018) 59:17–24. doi: 10.1016/j.clinbiochem.2018.07.003
54. Horwood NJ. Macrophage Polarization and Bone Formation: A Review. Clin Rev Allergy Immunol (2016) 51(1):79–86. doi: 10.1007/s12016-015-8519-2
55. Walker EC, McGregor NE, Poulton IJ, Solano M, Pompolo S, Fernandes TJ, et al. Oncostatin M Promotes Bone Formation Independently of Resorption When Signaling Through Leukemia Inhibitory Factor Receptor in Mice. J Clin Invest (2010) 120(2):582–92. doi: 10.1172/JCI40568
56. Matsuo K, Irie N. Osteoclast-Osteoblast Communication. Arch Biochem Biophys (2008) 473(2):201–9. doi: 10.1016/j.abb.2008.03.027
57. Yang D, Wan Y. Molecular Determinants for the Polarization of Macrophage and Osteoclast. Semin Immunopathol (2019) 41(5):551–63. doi: 10.1007/s00281-019-00754-3
58. Tanaka K, Yamagata K, Kubo S, Nakayamada S, Sakata K, Matsui T, et al. Glycolaldehyde-Modified Advanced Glycation End-Products Inhibit Differentiation of Human Monocytes Into Osteoclasts via Upregulation of IL-10. Bone (2019) 128:115034. doi: 10.1016/j.bone.2019.115034
59. Mandal CC, Ghosh Choudhury G, Ghosh-Choudhury N. Phosphatidylinositol 3 Kinase/Akt Signal Relay Cooperates With Smad in Bone Morphogenetic Protein-2-Induced Colony Stimulating Factor-1 (CSF-1) Expression and Osteoclast Differentiation. Endocrinology (2009) 150(11):4989–98. doi: 10.1210/en.2009-0026
60. Alexander KA, Chang MK, Maylin ER, Kohler T, Müller R, Wu AC, et al. Osteal Macrophages Promote In Vivo Intramembranous Bone Healing in a Mouse Tibial Injury Model. J Bone Miner Res (2011) 26(7):1517–32. doi: 10.1002/jbmr.354
61. Chan JK, Glass GE, Ersek A, Freidin A, Williams GA, Gowers K, et al. Low-Dose TNF Augments Fracture Healing in Normal and Osteoporotic Bone by Up-Regulating the Innate Immune Response. EMBO Mol Med (2015) 7(5):547–61. doi: 10.15252/emmm.201404487
62. Nicolaidou V, Wong MM, Redpath AN, Ersek A, Baban DF, Williams LM, et al. Monocytes Induce STAT3 Activation in Human Mesenchymal Stem Cells to Promote Osteoblast Formation. PloS One (2012) 7(7):e39871. doi: 10.1371/journal.pone.0039871
63. Kim J, Hematti P. Mesenchymal Stem Cell-Educated Macrophages: A Novel Type of Alternatively Activated Macrophages. Exp Hematol (2009) 37(12):1445–53. doi: 10.1016/j.exphem.2009.09.004
64. Maggini J, Mirkin G, Bognanni I, Holmberg J, Piazzón IM, Nepomnaschy I, et al. Mouse Bone Marrow-Derived Mesenchymal Stromal Cells Turn Activated Macrophages Into a Regulatory-Like Profile. PloS One (2010) 5(2):e9252. doi: 10.1371/journal.pone.0009252
65. Zha L, He L, Liang Y, Qin H, Yu B, Chang L, et al. TNF-α Contributes to Postmenopausal Osteoporosis by Synergistically Promoting RANKL-Induced Osteoclast Formation. BioMed Pharmacother (2018) 102:369–74. doi: 10.1016/j.biopha.2018.03.080
66. Llorente I, García-Castañeda N, Valero C, González-Álvaro I, Castañeda S. Osteoporosis in Rheumatoid Arthritis: Dangerous Liaisons. Front Med (Lausanne) (2020) 7:601618. doi: 10.3389/fmed.2020.601618
67. Mihara M, Hashizume M, Yoshida H, Suzuki M, Shiina M. IL-6/IL-6 Receptor System and Its Role in Physiological and Pathological Conditions. Clin Sci (Lond) (2012) 122(4):143–59. doi: 10.1042/CS20110340
68. Leng SX, Elias JA. Interleukin-11. Int J Biochem Cell Biol (1997) 29(8-9):1059–62. doi: 10.1016/S1357-2725(97)00017-4
69. Udagawa N, Takahashi N, Katagiri T, Tamura T, Wada S, Findlay DM, et al. Interleukin (IL)-6 Induction of Osteoclast Differentiation Depends on IL-6 Receptors Expressed on Osteoblastic Cells But Not on Osteoclast Progenitors. J Exp Med (1995) 182(5):1461–8. doi: 10.1084/jem.182.5.1461
70. Gao Y, Morita I, Maruo N, Kubota T, Murota S, Aso T. Expression of IL-6 Receptor and GP130 in Mouse Bone Marrow Cells During Osteoclast Differentiation. Bone (1998) 22(5):487–93. doi: 10.1016/S8756-3282(98)00040-4
71. Schmidt-Arras D, Rose-John S. Endosomes as Signaling Platforms for IL-6 Family Cytokine Receptors. Front Cell Dev Biol (2021) 9:688314. doi: 10.3389/fcell.2021.688314
72. Sims NA. Cell-Specific Paracrine Actions of IL-6 Family Cytokines From Bone, Marrow and Muscle That Control Bone Formation and Resorption. Int J Biochem Cell Biol (2016) 79:14–23. doi: 10.1016/j.biocel.2016.08.003
73. Eghbali-Fatourechi G, Khosla S, Sanyal A, Boyle WJ, Lacey DL, Riggs BL. Role of RANK Ligand in Mediating Increased Bone Resorption in Early Postmenopausal Women. J Clin Invest (2003) 111(8):1221–30. doi: 10.1172/JCI200317215
74. Rachner TD, Khosla S, Hofbauer LC. Osteoporosis: Now and the Future. Lancet (2011) 377(9773):1276–87. doi: 10.1016/S0140-6736(10)62349-5
75. Mundy GR. Osteoporosis and Inflammation. Nutr Rev (2007) 65(12 Pt 2):S147–51. doi: 10.1111/j.1753-4887.2007.tb00353.x
76. Raehtz S, Bierhalter H, Schoenherr D, Parameswaran N, McCabe LR. Estrogen Deficiency Exacerbates Type 1 Diabetes-Induced Bone TNF-α Expression and Osteoporosis in Female Mice. Endocrinology (2017) 158(7):2086–101. doi: 10.1210/en.2016-1821
77. Yin Y, Chen G, Gong L, Ge K, Pan W, Li N, et al. DNAzyme-Powered Three-Dimensional DNA Walker Nanoprobe for Detection Amyloid Beta-Peptide Oligomer in Living Cells and In Vivo. Anal Chem (2020) 92(13):9247–56. doi: 10.1021/acs.analchem.0c01592
78. Qu Z, Yang F, Hong J, Wang W, Yan S. Parathyroid Hormone and Bone Mineral Density: A Mendelian Randomization Study. J Clin Endocrinol Metab (2020) 105(11):dgaa579. doi: 10.1210/clinem/dgaa579
79. Huang JC, Sakata T, Pfleger LL, Bencsik M, Halloran BP, Bikle DD, et al. PTH Differentially Regulates Expression of RANKL and OPG. J Bone Miner Res (2004) 19(2):235–44. doi: 10.1359/JBMR.0301226
80. Silva BC, Bilezikian JP. Parathyroid Hormone: Anabolic and Catabolic Actions on the Skeleton. Curr Opin Pharmacol (2015) 22:41–50. doi: 10.1016/j.coph.2015.03.005
81. O'Brien CA, Jilka RL, Fu Q, Stewart S, Weinstein RS, Manolagas SC. IL-6 is Not Required for Parathyroid Hormone Stimulation of RANKL Expression, Osteoclast Formation, and Bone Loss in Mice. Am J Physiol Endocrinol Metab (2005) 289(5):E784–93. doi: 10.1152/ajpendo.00029.2005
82. Neale Weitzmann M, Pacifici R. Parathyroid Diseases and T Cells. Curr Osteoporos Rep (2017) 15(3):135–41. doi: 10.1007/s11914-017-0359-y
83. Parfitt AM. The Actions of Parathyroid Hormone on Bone: Relation to Bone Remodeling and Turnover, Calcium Homeostasis, and Metabolic Bone Diseases. II. PTH and Bone Cells: Bone Turnover and Plasma Calcium Regulation. Metabolism (1976) 25(8):909–55. doi: 10.1016/0026-0495(76)90124-4
84. Strege DW, Kahn AJ, Jeffrey JJ, Partridge NC. Stimulation of Collagenase Production by Rat Osteosarcoma Cells Can Occur in a Subpopulation of Cells. J Bone Miner Res (1990) 5(9):963–71. doi: 10.1002/jbmr.5650050910
85. Witty JP, Foster SA, Stricklin GP, Matrisian LM, Stern PH. Parathyroid Hormone-Induced Resorption in Fetal Rat Limb Bones Is Associated With Production of the Metalloproteinases Collagenase and Gelatinase B. J Bone Miner Res (1996) 11(1):72–8. doi: 10.1002/jbmr.5650110111
86. Vandewalle J, Luypaert A, De Bosscher K, Libert C. Therapeutic Mechanisms of Glucocorticoids. Trends Endocrinol Metab (2018) 29(1):42–54. doi: 10.1016/j.tem.2017.10.010
87. Chotiyarnwong P, McCloskey EV. Pathogenesis of Glucocorticoid-Induced Osteoporosis and Options for Treatment. Nat Rev Endocrinol (2020) 16(8):437–47. doi: 10.1038/s41574-020-0341-0
88. Canalis E. Mechanisms of Glucocorticoid Action in Bone. Curr Osteoporos Rep (2005) 3(3):98–102. doi: 10.1007/s11914-005-0017-7
89. Canalis E, Mazziotti G, Giustina A, Bilezikian JP. Glucocorticoid-Induced Osteoporosis: Pathophysiology and Therapy. Osteoporos Int (2007) 18(10):1319–28. doi: 10.1007/s00198-007-0394-0
90. Weinstein RS, Chen JR, Powers CC, Stewart SA, Landes RD, Bellido T, et al. Promotion of Osteoclast Survival and Antagonism of Bisphosphonate-Induced Osteoclast Apoptosis by Glucocorticoids. J Clin Invest (2002) 109(8):1041–8. doi: 10.1172/JCI0214538
91. Zhu S, Yao F, Qiu H, Zhang G, Xu H, Xu J. Coupling Factors and Exosomal Packaging microRNAs Involved in the Regulation of Bone Remodelling. Biol Rev Camb Philos Soc (2018) 93(1):469–80. doi: 10.1111/brv.12353
92. Sun W, Zhao C, Li Y, Wang L, Nie G, Peng J, et al. Osteoclast-Derived microRNA-Containing Exosomes Selectively Inhibit Osteoblast Activity. Cell Discov (2016) 2:16015. doi: 10.1038/celldisc.2016.15
93. Wang X, Guo B, Li Q, Peng J, Yang Z, Wang A, et al. miR-214 Targets ATF4 to Inhibit Bone Formation. Nat Med (2013) 19(1):93–100. doi: 10.1038/nm.3026
94. Li D, Liu J, Guo B, Liang C, Dang L, Lu C, et al. Osteoclast-Derived Exosomal miR-214-3p Inhibits Osteoblastic Bone Formation. Nat Commun (2016) 7:10872. doi: 10.1038/ncomms10872
95. Zhao C, Sun W, Zhang P, Ling S, Li Y, Zhao D, et al. miR-214 Promotes Osteoclastogenesis by Targeting Pten/PI3k/Akt Pathway. RNA Biol (2015) 12(3):343–53. doi: 10.1080/15476286.2015.1017205
96. Chen C, Cheng P, Xie H, Zhou HD, Wu XP, Liao EY, et al. MiR-503 Regulates Osteoclastogenesis via Targeting RANK. J Bone Miner Res (2014) 29(2):338–47. doi: 10.1002/jbmr.2032
97. Hassan MQ, Gordon JA, Beloti MM, Croce CM, van Wijnen AJ, Stein JL, et al. A Network Connecting Runx2, SATB2, and the miR-23a~27a~24-2 Cluster Regulates the Osteoblast Differentiation Program. Proc Natl Acad Sci USA (2010) 107(46):19879–84. doi: 10.1073/pnas.1007698107
98. Tian F, Ji XL, Xiao WA, Wang B, Wang F. CXCL13 Promotes Osteogenic Differentiation of Mesenchymal Stem Cells by Inhibiting miR-23a Expression. Stem Cells Int (2015) 2015:632305. doi: 10.1155/2015/632305
99. Komori T. Regulation of Bone Development and Maintenance by Runx2. Front Biosci (2008) 13:898–903. doi: 10.2741/2730
100. Ma Y, Gao J, Guo H. miR-23a-3p Regulates Runx2 to Inhibit the Proliferation and Metastasis of Oral Squamous Cell Carcinoma. J Oncol (2022) 2022:8719542. doi: 10.1155/2022/8719542
101. Hodges WM, O'Brien F, Fulzele S, Hamrick MW. Function of microRNAs in the Osteogenic Differentiation and Therapeutic Application of Adipose-Derived Stem Cells (ASCs). Int J Mol Sci (2017) 18(12):2597. doi: 10.3390/ijms18122597
102. Park J, Wada S, Ushida T, Akimoto T. The microRNA-23a has Limited Roles in Bone Formation and Homeostasis In Vivo. Physiol Res (2015) 64(5):711–9. doi: 10.33549/physiolres.932901
103. Xu Y, Jiang Y, Jia B, Wang Y, Li T. Icariin Stimulates Osteogenesis and Suppresses Adipogenesis of Human Bone Mesenchymal Stem Cells via miR-23a-Mediated Activation of the Wnt/β-Catenin Signaling Pathway. Phytomedicine (2021) 85:153485. doi: 10.1016/j.phymed.2021.153485
104. Li T, Li H, Wang Y, Li T, Fan J, Xiao K, et al. microRNA-23a Inhibits Osteogenic Differentiation of Human Bone Marrow-Derived Mesenchymal Stem Cells by Targeting LRP5. Int J Biochem Cell Biol (2016) 72:55–62. doi: 10.1016/j.biocel.2016.01.004
105. Shen G, Ren H, Shang Q, Zhao W, Zhang Z, Yu X, et al. Foxf1 Knockdown Promotes BMSC Osteogenesis in Part by Activating the Wnt/β-Catenin Signalling Pathway and Prevents Ovariectomy-Induced Bone Loss. EBioMedicine (2020) 52:102626. doi: 10.1016/j.ebiom.2020.102626
106. Zhuang XM, Zhou B. Exosome Secreted by Human Gingival Fibroblasts in Radiation Therapy Inhibits Osteogenic Differentiation of Bone Mesenchymal Stem Cells by Transferring miR-23a. BioMed Pharmacother (2020) 131:110672. doi: 10.1016/j.biopha.2020.110672
107. Zhuang XM, Zhou B, Yuan KF. Role of P53 Mediated miR-23a/CXCL12 Pathway in Osteogenic Differentiation of Bone Mesenchymal Stem Cells on Nanostructured Titanium Surfaces. BioMed Pharmacother (2019) 112:108649. doi: 10.1016/j.biopha.2019.108649
108. Sun LY, Wang N, Ban T, Sun YH, Han Y, Sun LL, et al. MicroRNA-23a Mediates Mitochondrial Compromise in Estrogen Deficiency-Induced Concentric Remodeling via Targeting PGC-1α. J Mol Cell Cardiol (2014) 75:1–11. doi: 10.1016/j.yjmcc.2014.06.012
109. Yu B, Huo L, Liu Y, Deng P, Szymanski J, Li J, et al. PGC-1α Controls Skeletal Stem Cell Fate and Bone-Fat Balance in Osteoporosis and Skeletal Aging by Inducing TAZ. Cell Stem Cell (2018) 23(2):193–209.e5. doi: 10.1016/j.stem.2018.06.009
110. Sujitha S, Rasool M. Berberine Coated Mannosylated Liposomes Curtail RANKL Stimulated Osteoclastogenesis Through the Modulation of GSK3β Pathway via Upregulating miR-23a. Int Immunopharmacol (2019) 74:105703. doi: 10.1016/j.intimp.2019.105703
111. Jang HD, Shin JH, Park DR, Hong JH, Yoon K, Ko R, et al. Inactivation of Glycogen Synthase Kinase-3β Is Required for Osteoclast Differentiation. J Biol Chem (2011) 286(45):39043–50. doi: 10.1074/jbc.M111.256768
112. Sims NA. The JAK1/STAT3/SOCS3 Axis in Bone Development, Physiology, and Pathology. Exp Mol Med (2020) 52(8):1185–97. doi: 10.1038/s12276-020-0445-6
113. Farr JN, Xu M, Weivoda MM, Monroe DG, Fraser DG, Onken JL, et al. Targeting Cellular Senescence Prevents Age-Related Bone Loss in Mice. Nat Med (2017) 23(9):1072–9. doi: 10.1038/nm.4385
114. Murakami K, Kobayashi Y, Uehara S, Suzuki T, Koide M, Yamashita T, et al. A Jak1/2 Inhibitor, Baricitinib, Inhibits Osteoclastogenesis by Suppressing RANKL Expression in Osteoblasts In Vitro. PloS One (2017) 12(7):e0181126. doi: 10.1371/journal.pone.0181126
115. Su R, Dong L, Zou D, Zhao H, Ren Y, Li F, et al. microRNA-23a, -27a and -24 Synergistically Regulate JAK1/Stat3 Cascade and Serve as Novel Therapeutic Targets in Human Acute Erythroid Leukemia. Oncogene (2016) 35(46):6001–14. doi: 10.1038/onc.2016.127
116. Yunna C, Mengru H, Lei W, Weidong C. Macrophage M1/M2 Polarization. Eur J Pharmacol (2020) 877:173090. doi: 10.1016/j.ejphar.2020.173090
117. Peng P, Li Z, Liu X. Reduced Expression of miR-23a Suppresses A20 in TLR-Stimulated Macrophages. Inflammation (2015) 38(5):1787–93. doi: 10.1007/s10753-015-0156-7
118. Qu Y, Xu Y, Jiang Y, Yu D, Jiang X, Zhao L. Macrophage-Derived Extracellular Vesicles Regulates USP5-Mediated HDAC2/NRF2 Axis to Ameliorate Inflammatory Pain. FASEB J (2021) 35(9):e21332. doi: 10.1096/fj.202001185RR
119. Grillari J, Mäkitie RE, Kocijan R, Haschka J, Vázquez DC, Semmelrock E, et al. Circulating miRNAs in Bone Health and Disease. Bone (2021) 145:115787. doi: 10.1016/j.bone.2020.115787
120. Sun Z, Wang H, Wang Y, Yuan G, Yu X, Jiang H, et al. MiR-103-3p Targets the M(6) A Methyltransferase METTL14 to Inhibit Osteoblastic Bone Formation. Aging Cell (2021) 20(2):e13298. doi: 10.1111/acel.13298
121. Wang Z, Xie Q, Yu Z, Zhou H, Huang Y, Bi X, et al. A Regulatory Loop Containing miR-26a, GSK3β and C/Ebpα Regulates the Osteogenesis of Human Adipose-Derived Mesenchymal Stem Cells. Sci Rep (2015) 5:15280. doi: 10.1038/srep15280
122. Chen S, Zheng Y, Zhang S, Jia L, Zhou Y. Promotion Effects of miR-375 on the Osteogenic Differentiation of Human Adipose-Derived Mesenchymal Stem Cells. Stem Cell Rep (2017) 8(3):773–86. doi: 10.1016/j.stemcr.2017.01.028
123. Lin Z, He H, Wang M, Liang J. MicroRNA-130a Controls Bone Marrow Mesenchymal Stem Cell Differentiation Towards the Osteoblastic and Adipogenic Fate. Cell Prolif (2019) 52(6):e12688. doi: 10.1111/cpr.12688
124. Qian M, Wang S, Guo X, Wang J, Zhang Z, Qiu W, et al. Hypoxic Glioma-Derived Exosomes Deliver microRNA-1246 to Induce M2 Macrophage Polarization by Targeting TERF2IP via the STAT3 and NF-κb Pathways. Oncogene (2020) 39(2):428–42. doi: 10.1038/s41388-019-0996-y
125. Lian JB, Stein GS, van Wijnen AJ, Stein JL, Hassan MQ, Gaur T, et al. MicroRNA Control of Bone Formation and Homeostasis. Nat Rev Endocrinol (2012) 8(4):212–27. doi: 10.1038/nrendo.2011.234
126. Sujitha S, Dinesh P, Rasool M. Berberine Encapsulated PEG-Coated Liposomes Attenuate Wnt1/β-Catenin Signaling in Rheumatoid Arthritis via miR-23a Activation. Eur J Pharm Biopharm (2020) 149:170–91. doi: 10.1016/j.ejpb.2020.02.007
127. He Y, Meng C, Shao Z, Wang H, Yang S. MiR-23a Functions as a Tumor Suppressor in Osteosarcoma. Cell Physiol Biochem (2014) 34(5):1485–96. doi: 10.1159/000366353
128. Gindin Y, Jiang Y, Francis P, Walker RL, Abaan OD, Zhu YJ, et al. miR-23a Impairs Bone Differentiation in Osteosarcoma via Down-Regulation of GJA1. Front Genet (2015) 6:233. doi: 10.3389/fgene.2015.00233
129. Liao W, Ning Y, Xu HJ, Zou WZ, Hu J, Liu XZ, et al. BMSC-Derived Exosomes Carrying microRNA-122-5p Promote Proliferation of Osteoblasts in Osteonecrosis of the Femoral Head. Clin Sci (Lond) (2019) 133(18):1955–75. doi: 10.1042/CS20181064
130. Srivastava M, Deal C. Osteoporosis in Elderly: Prevention and Treatment. Clin Geriatr Med (2002) 18(3):529–55. doi: 10.1016/S0749-0690(02)00022-8
131. Wang T, He C. TNF-α and IL-6: The Link Between Immune and Bone System. Curr Drug Targets (2020) 21(3):213–27. doi: 10.2174/1389450120666190821161259
132. Li H, Zhai Z, Qu X, Xu J, Qin A, Dai K. MicroRNAs as Potential Targets for Treatment of Osteoclast-Related Diseases. Curr Drug Targets (2018) 19(5):422–31. doi: 10.2174/1389450117666160502144329
133. Zhou Y, Liu Y, Cheng L. miR-21 Expression is Related to Particle-Induced Osteolysis Pathogenesis. J Orthop Res (2012) 30(11):1837–42. doi: 10.1002/jor.22128
134. Shibuya H, Nakasa T, Adachi N, Nagata Y, Ishikawa M, Deie M, et al. Overexpression of microRNA-223 in Rheumatoid Arthritis Synovium Controls Osteoclast Differentiation. Mod Rheumatol (2013) 23(4):674–85. doi: 10.3109/s10165-012-0710-1
135. Blüml S, Bonelli M, Niederreiter B, Puchner A, Mayr G, Hayer S, et al. Essential Role of microRNA-155 in the Pathogenesis of Autoimmune Arthritis in Mice. Arthritis Rheumatol (2011) 63(5):1281–8. doi: 10.1002/art.30281
136. Nakasa T, Shibuya H, Nagata Y, Niimoto T, Ochi M. The Inhibitory Effect of microRNA-146a Expression on Bone Destruction in Collagen-Induced Arthritis. Arthritis Rheumatol (2011) 63(6):1582–90. doi: 10.1002/art.30321
137. Lin W, Li X-F, Ren D-C, Song M, Duan L, Liu J-Z, et al. Administration of Zoledronic Acid Alleviates Osteoporosis in HIV Patients by Suppressing Osteoclastogenesis via Regulating RANKL Expression. Mol Med (2021) 27(1):19. doi: 10.1186/s10020-021-00276-5
138. Qu Y, Wang Z, Zhou H, Kang M, Dong R, Zhao J. Oligosaccharide Nanomedicine of Alginate Sodium Improves Therapeutic Results of Posterior Lumbar Interbody Fusion With Cages for Degenerative Lumbar Disease in Osteoporosis Patients by Downregulating Serum miR-155. Int J Nanomed (2017) 12:8459–69. doi: 10.2147/IJN.S143824
Keywords: miR-23a, osteoporosis, osteoblast (OB), osteoclast (OC), macrophage, cytokine
Citation: Ma T-L, Zhu P, Ke Z-R, Chen J-X, Hu Y-H and Xie J (2022) Focusing on OB-OC-MΦ Axis and miR-23a to Explore the Pathogenesis and Treatment Strategy of Osteoporosis. Front. Endocrinol. 13:891313. doi: 10.3389/fendo.2022.891313
Received: 07 March 2022; Accepted: 20 June 2022;
Published: 14 July 2022.
Edited by:
Chandi C. Mandal, Central University of Rajasthan, IndiaReviewed by:
Jiake Xu, University of Western Australia, AustraliaJing Ai, Harbin Medical University, China
Junfen Fan, Capital Medical University, China
Copyright © 2022 Ma, Zhu, Ke, Chen, Hu and Xie. This is an open-access article distributed under the terms of the Creative Commons Attribution License (CC BY). The use, distribution or reproduction in other forums is permitted, provided the original author(s) and the copyright owner(s) are credited and that the original publication in this journal is cited, in accordance with accepted academic practice. No use, distribution or reproduction is permitted which does not comply with these terms.
*Correspondence: Yi-He Hu, Y3N1aHV5aWhlQDE2My5jb20=; Jie Xie, ZHJfeGllamllQDE2My5jb20=
†These authors have contributed equally to this work