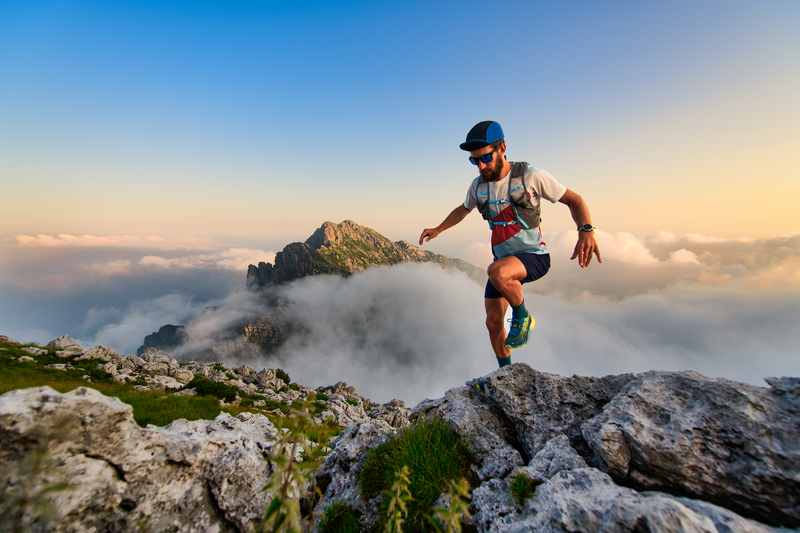
95% of researchers rate our articles as excellent or good
Learn more about the work of our research integrity team to safeguard the quality of each article we publish.
Find out more
REVIEW article
Front. Endocrinol. , 25 May 2022
Sec. Neuroendocrine Science
Volume 13 - 2022 | https://doi.org/10.3389/fendo.2022.882772
This article is part of the Research Topic Reproductive Neuroendocrinology and Social Behavior Vol. II View all 4 articles
Spexin (SPX) and galanin (GAL) are two neuropeptides that are phylogenetically related and have descended from a common ancestral gene. Considerable attention has been given to these two multifunctional neuropeptides because they share GAL receptors 1,2, and 3. Since GAL and SPX-synthesizing neurons have been detected in several brain areas, therefore, it can be speculated that SPX and GAL are involved in various neurophysiological functions. Several studies have shown the functions of these two neuropeptides in energy regulation, reproduction, and response to stress. SPX acts as a satiety factor to suppress food intake, while GAL has the opposite effect as an orexigenic factor. There is evidence that SPX acts as an inhibitor of reproductive functions by suppressing gonadotropin release, while GAL modulates the activity of gonadotropin-releasing hormone (GnRH) neurons in the brain and gonadotropic cells in the pituitary. SPX and GAL are responsive to stress. Furthermore, SPX can act as an anxiolytic factor, while GAL exerts anti-depressant and pro-depressive effects depending on the receptor it binds. This review describes evidence supporting the central roles of SPX and GAL neuropeptides in energy balance, reproduction, stress, and social behaviors, with a particular focus on non-mammalian vertebrate systems.
Neuropeptides are essential signaling molecules that work to integrate information in the nervous systems. In the central nervous system, neuropeptides are especially important in the hypothalamic circuitry, as these molecules underlie both neural and non-neural communications as part of the neuroendocrine system. Two neuropeptides, namely, spexin (SPX) and galanin (GAL), have received considerable attention in recent years. Although GAL has been known for quite a while, SPX is newly discovered, and researchers have uncovered its potential functions. GAL has been implicated in various functions in the neuroendocrine system. Similarly, studies on SPX have identified its functions related to hypothalamic circuitry. It was found that SPX can bind to some GAL receptors, thus, linking these two neuropeptides in addition to their common ancestral origin (1). As such, GAL and SPX are involved in similar processes, namely, in energy regulation, reproduction, stress responses and mood disorders (2, 3).
SPX and GAL are evolutionarily conserved across vertebrate species. As shown in Figure 1, there is a high degree of similarity between the mature sequences of GAL and SPX in different vertebrate species. However, physiological significance of GAL and SPX, in particular, their clinical implications, remain unknown. On the other hand, studies using animal models including non-mammalian vertebrate species provide useful and beneficial information in biomedical research because of the high homology of these peptides in vertebrates. To date, functional characterization has revealed that GAL acts primarily as an orexigenic factor, as it is known to stimulate food intake (3, 4). In contrast, it has been implicated that SPX acts as a satiety factor (3). In addition to appetite regulation, GAL and SPX are involved in reproductive function such as in the regulation of gonadotropin and gonadotropin-releasing hormone (GnRH) release. SPX and GAL are also involved in stress response via the hypothalamic-pituitary-adrenal (HPA) axis (5). For example, GAL is co-localized in CRH neurons in some teleosts (6). Further, SPX and GAL expressions in the brain are sensitive to stress exposure, as seen in rats and Nile tilapia (7, 8). In addition, SPX and GAL have roles in modulating serotonin activity, indicating roles in mood disorders such as anxiety and depression (9, 10). The purpose of this review is to elaborate on the functional roles of SPX, GAL, and their receptors in metabolism and certain social behaviors in non-mammalian vertebrates, with a focus on fish species.
Figure 1 Sequence comparison between the mature peptide sequences of SPX1, SPX2, and GAL of various non-mammalian vertebrate species with some tetrapods. Both SPXs are 14 amino acids long, except for tongue sole SPX2, which is 17 amino acids long. GAL is mostly 29 amino acids long, Amino acids at positions 2, 9, 10, and 12 are conserved across the three neuropeptides.
GAL was first isolated from the porcine intestine (11). Its name was derived from the glycine and alanine at its N- and C-terminals, respectively (11). In most species, including various fish species, GAL is 29 residues long, whereas it has 30 amino acids in humans (6, 12–14) (Figure 1). GAL binds to its receptors, aptly named GAL receptors (GALRs). Three forms of GALRs have been identified, namely GAL receptors 1, 2, and 3 (GALR1, GALR2, and GALR3, respectively) (15). Additionally, there are three other members of the GAL family: galanin message-associated protein (GMAP), galanin-like peptide (GALP), and alarin. GMAP is 59 amino acids long and comes from the preprogalanin precursor (16). GALP is a non-amidated 60 amino acid peptide with sequence similarity with GAL (17). It binds with high affinity with GALRs, with relatively lower affinity with GALR1, and was first isolated from porcine hypothalamus (17). Alarin was discovered recently as a splice variant of GALP. It was observed in the murine brain, thymus, and skin, while exhibiting vasoconstriction and anti-oedema activity. However, alarin’s activity is not mediated by any of the known GALRs. Thus, the cognate receptor for alarin still remains unknown (18). GAL-expressing cells are found in restricted brain regions, including the preoptic area and paraventricular hypothalamus, in various non-mammalian vertebrate species, as shown in Table 1. However, GAL fibers are widespread and extend all over the brain. A phylogenetic tree showcasing comparison between GAL and SPX gene sequences is shown in Figure 2.
Table 1 GAL-expressing cells were found in restricted brain regions of various non-mammalian species. However, GAL fibers were widespread and extended all over the brain.
Figure 2 Phylogenetic tree of SPX and GAL present in fish. Sequences obtained from GenBank. Phylogenetic construction was done in MEGA X, using the Neighbor-Joining method. The percentage of the sequences clustered together is shown on the branches, out of 1000. SPX and GAL form two distinct branches, with SPX1 and SPX2 dividing further.
GALRs are responsible for mediating the functions of both SPX and GAL. GALRs are G-protein coupled receptors (GPCRs) that interact with G-proteins in cells to mediate their functions (Figure 3). In teleosts, there are two types of GALR1 types, namely GALR1a and GALR1b, as shown in Figure 3 (23, 24). Similarly, GALR2 paralogs exist in non-mammalian vertebrates and are known as GALR2a and GALR2b (1, 23, 24). On the other hand, GALR3 is absent in non-mammalian vertebrates. GALR1 and GALR3 are known to interact with the Gi-class of G-proteins and mediate inhibitory effects (25, 26). Interestingly, GALR2 is able to bind to different G-protein classes, which are Gs, Gq/11 and Gi/o, and is able to mediate both stimulatory and inhibitory downstream effects (23). In mammals, GAL binds to all three GALRs, while SPX binds to GALR2 and GALR3. In fish, these receptors are localized in various regions in the brain and periphery (Table 2). Phylogenetic analysis of GALR gene sequence is detailed in Figure 4.
Figure 3 Galanin receptors pair with different G-proteins to execute SPX or GAL functions. In brief, GAL acts on all three of its receptors, while SPX only pairs with GALR2 and GALR3. Although both GALR1 and GALR3 can only pair with Gi/o proteins, GALR2 has been found to pair with several G-proteins, mediating various downstream effects.
Figure 4 Phylogenetic tree of GALRs present in fish. Sequences obtained from GenBank. Phylogenetic construction was done in MEGA X, using the Neighbor-Joining method. The percentage of the sequences clustered together is shown on the branches, out of 1000 boots. GALR1 and GALR2 form distinct branches.
In a study looking at SPX binding to GALRs, SPX is found to exhibit high affinities to human, zebrafish, and Xenopus GALR2 and GALR3 (1), showcasing that SPX can activate GALR2 and GALR3 in mammalian and non-mammalian systems. Both SPXs, SPX1 and SPX2, can bind strongly to human GALR2/3. Although GALR2 has a higher binding affinity towards GAL as compared to SPX, GALR3 has a stronger preference for SPX binding than for GAL (1). Interestingly, SPXs have higher potency for zebrafish, GALR2b compared to GAL, while GALR2a interacts with both SPXs and GAL with similar affinities (1).
SPX (also known as C12ORF29, according to its locus on human chromosome 12) was first discovered in the human proteome by bioinformatics (31–33). Since then, this peptide has been found in many other vertebrate species, including in teleost fish. A few paralogous forms of SPX have been identified. Spx1a, spx1b, and spx2 are found in non-mammalian vertebrate genomes, whereas only spx1 has been identified in mammals (1). It is likely that spx paralogues arose after two rounds of whole genome duplication in non-mammalian vertebrates (1). Additionally, cichlids express a paralogous spx1b gene, but not spx2 (23). Both SPX genes encode for preprohormones that include a signalling peptide and the mature peptide flanked by dibasic cleavage sites. The mature SPX1 peptide sequence is 14 residues long and is conserved across species (34–39). Similarly, most known SPX2 sequences are also 14 amino acids long, except for the half-smooth tongue sole (Cynoglossus semilaevis) SPX2, which has 17 residues instead (1, 9, 37, 40). The mature peptide sequences of SPX1 and SPX2 are highly conserved across the species. Mature peptide and gene sequence comparison of GAL and SPX are depicted in Figures 1, 2.
In teleosts, SPX neurons have been detected in various brain regions, as depicted in Table 3. In goldfish (Cassasius auratus), SPX-immunoreactive cells are located at the anterior hypothalamus, ventromedial thalamic nucleus, and medial longitudinal fasciculus (35). In the Nile tilapia (Oreochromis niloticus), SPX1a-expressing cells are found in the torus semicircularis (TS), while SPX1b-expressing cells are observed in the telencephalon (8). Comparatively, SPX expression in rats is particularly restricted to the mesopontine tegmentum (32). In a study focusing on the human magnocellular hypothalamus, SPX-expressing neurons were observed in the supraoptic and paraventricular nuclei (44).
In addition to SPX and GAL, kisspeptin (KISS) also descended from the same ancestral gene (1). Kim et al. found that the ancestral forms of these three genes are closely located on the same ancient chromosome (1). Kisspeptin is a neuropeptide encoded by the gene KISS1 in humans and mammals, while orthologs exist in non-mammals, namely kiss2 and kiss3 (45). It can be speculated that SPX, GAL, and KISS genes arose from local duplications on the ancient chromosome before splitting into the many paralogous forms in modern vertebrate chromosomes after two rounds of whole-genome duplication. For example, spx1 and kiss2 are present on the same chromosome in zebrafish, anole lizard, and coelacanth, although in humans, KISS2 is absent in the same corresponding chromosome. In the same species, spx2 and gal are also localized in near vicinity but SPX2 is absent in humans. Additionally, galp and kiss3 are present on the same chromosome in the coelacanth and Xenopus, while KISS3 is absent from the human genome (1).
The close relationship of SPX, GAL, and KISS means that they share similar functions. On that note, KISS has been implicated in metabolic functions, reproduction, mood, and behavior, similar to SPX and GAL. As SPX and GAL share receptors, this review will focus on these two neuropeptides and GALRs.
SPX has been highly implicated in appetite regulation and food consumption in both mammals and non-mammalian vertebrates. In fish brains, different nutritional states affect spx gene expression. In the goldfish, spx1 mRNA expression increases in the telencephalon, optic tectum, and the hypothalamus, as well as in the periphery after feeding (39, 46). The same effect is seen in the hypothalamus of Siberian sturgeon (Acipenser baerii), whereas in Ya-fish (Schizothorax prenanti), the increase is observed only in the forebrain (41, 43). On the other hand, spx expression is responsive to food deprivation, however, effects vary according to species, experimental design, and probably metabolic conditions. In the orange-spotted grouper (Epinephelus coioides), seven days of fasting caused an increase in hypothalamic spx1 expression, with the levels recovering after refeeding (34). Similarly, spx1 upregulation after food deprivation has also been observed in the spotted scat (Scatophagus argus) after two and seven days of fasting (42) and in the half-smooth tongue sole, although effects were only seen after 35 days of starvation and not after two or seven days (38). Additionally, in the tongue sole, spx2 is upregulated after four weeks but not after two weeks of starvation (37). In contrast, other fish species such as goldfish, sturgeon, and Ya-fish display downregulation of spx gene expression after food restriction (39, 41, 43). In the brain of Nile tilapia, fasted adults have low expression levels of spx1a, spx1b, and galr2a but galr2b receptors remain unaffected (23). Thus, although feeding seems to clearly increase SPX expression, food deprivation instead has various outcomes in different fish species. This may be attributed to the different fasting duration between studies or to species-specific effects. The effects of different metabolic statuses on spx gene expression are detailed in Table 4.
Peripheral and central treatment of SPX in fish either reduces food consumption or causes change to appetite-regulating genes, or both, suggesting SPX as a satiety factor. Intraperitoneal (IP) or intracerebroventricular (ICV) injections of SPX suppresses food consumption in goldfish (39). SPX co-treatment with either neuropeptide Y (NPY) or orexin successfully block the appetite-stimulating effects of these two neuropeptides (39). ICV injections of SPX in the goldfish downregulates appetite-stimulating genes such as npy, apelin and agouti-related protein (agrp) in the brain (39). On the other hand, expressions of appetite-suppressing genes such as cholecystokinin (cck), proopiomelanocortin (pomc), cocaine and amphetamine-regulated transcript (cart), and melanin-concentrating hormone (mch) are upregulated following SPX treatment (39), suggesting SPX as an indirect appetite suppressant by acting on the aforementioned genes. Similarly, intracranial administration of SPX downregulates agrp1 and galr2a expression, while pomc1 and galr2b are significantly upregulated in zebrafish (47). Besides, IP treatment of SPX upregulates pomc mRNA expression in the hypothalamus, while at high doses, SPX downregulates hcrt expression in the orange-spotted grouper (34). IP treatment of SPX to the Siberian sturgeon results in an increase in anorexigenic factors nucleobindin 2 (nucb2), cart, urocortin 3 (ucn3), and peptide YY (pyy), while reducing npy expression in the hypothalamus (43). Effects of SPX treatment are summarized in Table 5.
Table 5 Details of SPX treatment in various animal models and mediums, with the effects in metabolism and reproduction.
Furthermore, spx1 gene knockout zebrafish exhibits an increase in agrp1 expression in the brain and an increase in food intake, which is reversed by SPX1 intracranial treatment (47). It is clear from these studies in fish that SPX acts to increase satiety by increasing known satiety factors via downregulating appetite-stimulating genes. Interestingly, an IP injection of insulin into the goldfish resulted in an increase in spx expression in the liver and the brain (46) suggesting a possible link between food intake, insulin and SPX. It is possible that feeding-induced insulin surge triggers central spx gene expression to inhibit food intake via controlling both orexigenic and anorexigenic factors in the hypothalamus. Currently, there is limited information on the interaction between SPX and orexigenic and anorexigenic neurons in feeding behavior non-mammalian species.
In chicken, fasting results in rising blood SPX levels (52). The authors went on to investigate the expression of spx in tissues related to carbohydrate and lipid metabolism and discovered changes to spx and galr expressions. Thus, it seems that in chickens, both SPX and its receptors are affected by nutritional status, at least in the periphery (52). Treating rodents with SPX has an anorexigenic and weight loss effect (48–50, 53). IP treatment of SPX into mice inhibits food intake, even in mice fasted prior to treatment (48). ICV treatment into the third ventricle also inhibits food intake and lower body weight, without any changes to energy expenditure in mice (50). As SPX binds to GALR2 and GALR3, the former study identified hypothalamic GALR3 as the important receptor, resulting in suppression of NPY expression, whereas the latter study implicated GALR2 (48, 50). As further confirmation, wild type mice treated with SPX-based GALR2 agonist, known as SG2A, displayed a reduction in body weight and food consumption (54). A possible explanation to SPX’s body weight regulation is that SPX may prevent fatty acid uptake by adipocytes, as found in a study using mice adipocyte cells in vitro (49) or fatty acids could induce SPX, GALR2, and GALR3 expression as seen in mice hypothalamic cell lines (55). An increase in circulating fatty acids after a meal may act as a trigger for SPX-GALR2 and GALR3 signalling in the hypothalamus to reduce body weight and food consumption.
In diet-induced obese rats, subcutaneous SPX treatment is able to alter the food intake behavior, resulting in smaller portions and shorter mealtimes, possibly leading to weight loss (49). This suggests that SPX has potential as a therapy for weight loss. Interestingly, SPX is the most downregulated gene in fat from obese patients (49). In fact, circulating SPX levels are lower in obese patients compared to their normal-weight counterparts (49, 56, 57). This is also observed in type 2 diabetes patients (58). Further, in vitro SPX treatment stimulates lipolysis but inhibits lipogenesis and glucose uptake in isolated human adipocytes (51). Thus, it can be presumed that peripheral SPX is dysregulated in metabolic disorders, allowing for an increase in food consumption and body weight.
SPX is likely a satiety factor in vertebrates that acts centrally to mediate appetite-regulating genes and reduce food intake. Peripherally, SPX may act to regulate lipid metabolism. A dysregulation in SPX signaling can potentially affect food consumption behavior and adipose tissue regulation, leading to metabolic disorders. Using SPX as a treatment can likely counter these problems, although more studies are warranted to observe its benefits.
Contrary to SPX, GAL has been shown to stimulate feeding behavior. In the goldfish, preprogalanin (gal) mRNA are localized in the brain regions which are related to the regulation of food intake, namely in the area ventralis telencephali pars ventralis, nucleus preopticus periventricularis, nucleus lateralis tuberis, and the nucleus recessus lateralis, while GAL immunoreactivity has been detected in the gustatory nuclei of the vagal lobe (19, 59). Unfed goldfish have a significantly higher expression of gal mRNA in the telencephalon and hypothalamus (19). In young Japanese quails, 6 hours of fasting lowered gal expression in the hypothalamus compared to fed birds (60). In goldfish, ICV injections of GAL stimulate feeding behavior, which is blocked by a GAL receptor antagonist, galantide, and yohimbine, an α-adrenergic receptor antagonist (61). Similarly, in chicks, ICV GAL injections stimulate food intake, which is attenuated by co-treatment with yohimbine (62). Further, several studies have shown a GAL-induced increase in food consumption with GAL treatment into the PVN of rats (53, 63–66). This suggests that the α-adrenergic system may mediate orexigenic action of GAL via hypothalamic GAL neurons.
PVN GAL treatment also stimulates a preference for a high-fat diet while increasing carbohydrate metabolism and glucose uptake in muscles in rats (53, 66). In reverse, mice that were given a high-fat diet, plasma GAL as well as hypothalamic GAL and GALR1 expression increased (67). Rats treated with GALR1 selective agonist M617 show an increase in food intake (68). This suggests that GALR1 mediates GAL-induced feeding behavior in mammals. Table 6 details the effects of GAL treatment.
Studies in humans have shown the role of GAL in metabolic disorders. Obese patients and patients experiencing gestational diabetes mellitus have significantly higher plasma levels of circulating GAL (75–77). As orexigenic peptide NPY immunoreactive fibers innervate GAL immunoreactive cells in the hypothalamus of the human brain (4), it is possible that NPY can affect plasma GAL levels in metabolic conditions, as the NPY system is dysregulated in obese conditions (78).
SPX neurons and SPX receptors, GALR2a and GALR2b have been observed in the preoptic area of several fish species, indicating a possible role in the reproductive axis (8, 23, 35). SPX was first implicated in reproductive function because it suppressed LH release from cultured goldfish pituitary cells in an in vitro study, as well as after IP injections in an in vivo study performed on goldfish (35). This was also observed in the Nile tilapia, where IP injections of SPX inhibited LH and FSH release (23). It is likely that SPX exerts inhibitory effect on gonadotropin release. At the transcriptional level, there are species-specific effects of peripheral SPX treatment on central gene expression. IP injections of SPX does not affect fshb and lhb mRNA expression in the pituitary of the orange-spotted grouper (34). In the half-smooth tongue sole, high dose of SPX treatment suppressed fshß expression but not lhß, gnih, gnrh2/3 and orexin in the hypothalamus (38). Thus, peripheral SPX might have a role in affecting LH or FSH release in some teleost species. It will be beneficial to uncover the role of central SPX in reproduction. Additionally, as SPX receptors are localized to the preoptic area, there is a possibility that central SPX may act on reproductive-related neurons in this region. Furthermore, brain spx expression is low during the breeding season but is high during development and puberty in the grouper and goldfish (34, 35). This suggest SPX is suppressed during the breeding season to facilitate reproductive function mediated by gonadal hormones, as estradiol treatment downregulates spx expression (35, 42). On the other hand, high expression of spx during development and puberty is likely to prevent premature LH/FSH release. Despite the evidence, spx1 knockout transgenic zebrafish did not show changes to reproductive capability, puberty onset, or gamete maturation (79). It is likely that the loss of SPX signalling in knockout zebrafish is compensated by other neuropeptides involved in the control of reproduction in fish (79). More evidence from GALR2 or GALR3 knockout studies may lead to clearer understanding of the role of SPX in the reproductive system. Importantly, fatty acids are able to induce SPX, GALR2, and GALR3 expression in a mice hypothalamic-GnRH cell line (80), which could be similar to an obese condition. If SPX does negatively regulate the reproductive axis in mammals, then the increase in SPX expression could partially explain the underlying obesity-induced reproductive dysfunction (81). In women with the reproductive disorder, polycystic ovarian syndrome (PCOS), circulating SPX levels are low compared to women with normal menstrual cycles (82). It is, therefore, possible that at least peripheral SPX is involved in the pathophysiology of PCOS, which often have high levels of LH secretion. Although some studies have suggested the effect of SPX on LH release in the pituitary, the function of SPX neurons, SPX-GAL2/3 signaling on reproduction-related neurons in the brain remains unclear.
Stress-activated HPA axis exerts inhibitory action on the reproductive system. SPX has been implicated in stress response. Chronic stress exposure in the Nile tilapia show high cortisol levels, low reproductive activities, and upregulation of spx1a/1b expression in several parts of the brain, namely, in the midbrain, hypothalamus, and optic tectum (8). In mice, chronic unpredictable stress exposure and CRH treatment reduces SPX gene expression in the hippocampus, hypothalamus, and pituitary (83). Therefore, it is likely that different types of stress might have varying effects on site-specific SPX1 expression in the brain.
Stress-activated HPA axis also exerts emotional control in the brain. SPX1 neurons in the dorsal habenula project to GALR2a/2b expressing cells in the interpeduncular nucleus (IPN) of the zebrafish (27, 40). Transgenic zebrafish that overexpress spx1 in the dorsal habenula show reduced anxiety but increased serotonergic-related genes in the raphe compared to wild-type (9). The IPN project fibers to the raphe. Therefore, it is likely that SPX1 mediates anxiety and induces anxiolytic effects by modulating the serotonergic system via GALR2a/2b in the IPN of zebrafish (9).
SPX-based GALR2 agonist relieves anxiety-like and depressive-like symptoms in mice (54, 84) as in zebrafish (9). Chronic stress in mice is associated with an increase in CRH, a decrease in spx expression in the hippocampus and a display of anxiety-like behavior (83). These studies suggest that SPX is involved in mood and negative emotions.
GAL neurons have been identified in the preoptic area of several fish species, including Xiphophorus, rainbow trout, brown ghost fish, green molly, sailfin molly, European sea bass, flounder, killifish, and tilapia (6, 85–87), with fiber projections to the entire brain and gonadotrophs in the pituitary (6, 88).
GAL gene expressions in the brain and gonads are differently regulated by sex steroids production in male and females in sea bass (89). The sailfin molly displays sexual dimorphic localization of GAL fibers on the brain. Males have a higher number of GAL immunoreactive fibers in the optic tectum, torus semicircularis, brainstem tegmentum, spinal cord, while in females fibers are limited to the ventromedial tegmentum and trigeminal area (87). Similarly, sexual dimorphism in GAL expression is observed in the goldfish, red salmon, and brown trout (90–92). Specifically, gender-specific differences in GAL immunoreactivity at the preoptic area has been observed in the molly, goldfish, and red salmon (87, 90, 91). GAL neurons in the preoptic area are stimulated during mating behavior in teleost, indicating the involvement of the GAL circuitry in reproductive-related social behavior (93).
Sexual dimorphism of GAL expression is also observed in the rat but not in the ovine brain (94, 95). In sheep, all GnRH cells co-express GAL, suggesting GAL’s significant role in the reproductive axis (95). Additionally, GnRH neurons express all three GALRs with evidence of GAL synapsing directly onto GnRH cell bodies in rodents (73, 96, 97). ICV administration of GAL into rats primed with estradiol benzoate and progesterone results in an increase in plasma LH levels, while pre-treatment with galantide, a non-specific GALR antagonist, blocked the increase (69). GAL treatment directly on pituitary cultures from rats upregulates basal LH secretion in a dose-dependent manner, while co-treatment with GnRH potentiates GnRH-induced LH secretion in vitro (72). Thus, it seems that GAL has a stimulatory effect on LH release, likely by directly influencing the pituitary. In addition, GAL may be able to modulate GnRH activity. A study in mice identified GAL as a regulator of GnRH release, by modulating kisspeptin-induced GnRH stimulation. GAL acts to reduce kisspeptin-induced GnRH neuronal activation in vitro (73). Therefore, GAL has a possible inhibitory effect on GnRH release when directly acting on GnRH neurons via GALR1, probably acting as a balancing factor for GnRH neuronal activation. A cluster of GAL-expressing cells in the medial preoptic area (mPOA) is activated during parental behavior, while a separate cell population in the same area is active during mating. Ablation of mPOA GAL cells results in loss of parental behavior in both male and female rats. These results show the importance of GAL of mPOA in social behavior related to reproduction and offspring care (98).
Intravenous infusion of GAL to women does not change LH and FSH levels but enhance growth hormone release (99). In women with PCOS, there is an increase in GAL immunoreactive fibers that innervate polycystic ovaries; however, there is no significant difference in plasma GAL levels (100, 101). Thus, in humans, the role of GAL in reproductive disorders remains largely unknown.
In some teleost fish, namely sailfin molly and killifish, GAL immunoreactivity is co-localized in CRH-like-immunoreactive cell bodies, implicating the involvement of GAL with the hypothalamic-pituitary-adrenal axis (6). However, social stress does not affect the expression of GAL mRNA in the brains of zebrafish (102).
Rats exposed to immobilization stress show upregulated GAL protein levels and intense GAL immunoreactivity in the hypothalamus and increased GAL mRNA in the adrenal gland (7). Stress because of exposure to predator scent reduces GAL mRNA levels in the frontal cortex and hippocampus and induces anxiety-like behavior. Further, the anxiety-like behavioral response is reduced when galnon, a GAL receptor agonist is given immediately after exposure to predator scent (103). In rats, immobilization stress and formalin injections increase GAL mRNA levels in the hypothalamus (104), and chronic social stress upregulates the expression of pGal mRNA in the locus coeruleus (105). On the other hand, subcutaneous GAL injections increase adrenocorticotropic hormone (ACTH), aldosterone, and corticosterone levels (74). Central injections of GAL stimulate CRH release from the rat hypothalamus in a dose-dependent manner (106). Allowing mice to freely exercise results in an elevation of locus coeruleus-derived GAL and resistance to anxiogenic conditions, suggesting that GAL could be mediating stress resilience (107). Overall, it seems that in stressful conditions, GAL upregulation can stimulate the HPA axis to release stress hormones. This suggests that GAL can trigger the body’s stress response.
In rodents, there is a clear involvement of GAL in anxiety and depression. Firstly, GAL is co-expressed in serotonergic neurons in the dorsal raphe of rats (108, 109). GAL exerts an inhibitory influence on the noradrenergic and serotonergic systems (10, 70, 110). GAL treatment reduces serotonin release into the ventral hippocampus, and receptor antagonist treatment blocks this effect (10). Similarly, pre-treatment with a GALR2 antagonist blocked the anxiogenic effects of GAL administration into the dorsal hippocampal area (111). It is likely that GAL affects serotonin synthesis, as GAL significantly reduces the mRNA expression of tryptophan hydroxylase in the dorsal raphe nucleus (10). GALR1 mediates pro-depressive effects of GAL after stress exposure by affecting the expression of the serotonin receptor, 5HT1AR (112). ICV GAL treatment mediates pro-depressive effects, which are overcome by its antagonist treatment (71, 112). ICV treatment of GAL, GALR1 agonist or GALR2 antagonist increases the immobility time, and GALR2 agonist decreases the immobility time of rats in the forced swim test. This indicates differences in the activity of GALR1 and GALR2, in which GALR1 may be pro-depressive while GALR2 mediates GAL’s anti-depressant activity (112). In addition to that, a GALR2 agonist mediated the anti-depressive effects of GAL when treated into the dorsal raphe nucleus, which was blocked by its antagonist (113). However, as GALR2 can induce different cellular signalling pathways, therefore, it is not impossible for GALR2 to mediate pro-depressive effects. It is also likely that GALR3 has pro-depressive effects, since an antagonist treatment results in anxiolytic and anti-depressive effects, by reversing GAL’s inhibition of serotonin release and transmission in the hippocampus (114). These findings in rodents indicate GAL’s possible role in developing and mediating depression and anxiety.
Interestingly, in humans, certain gene variants of GAL and their receptors confer vulnerability towards psychosocial stress and increase the risk of developing anxiety and depression (115). The activity of GAL is more prominent in a highly stressed population; GALR1 SNPs are linked to negative childhood events while GALR2 and GALR3 mediate effects of recent negative experiences. Thus, it is likely that GAL has a crucial role in developing depression, particularly if a person experiences childhood trauma or recently experienced negative events (115).
As discussed above, SPX and GAL show opposing actions and functions in the metabolic, reproductive system and emotional control. The likely reason to this is the existence of different receptors. It is interesting that GAL and SPX share receptors. However, it is difficult to distinguish if GALR2 or GALR3-linked effects are due to GAL or SPX binding. Different ligand-receptor interactions may lead to different downstream signaling. For example, GALR1 activation results in pro-depressive effects while GALR2 stimulation is linked with anti-depressant activity (71). GAL is known to be pro-depressive; thus, it must have acted on GALR1 receptors. In contrast, GALR2 anti-depressant effects can possibly be due to SPX activation instead of GAL. GALRs affinities towards the two ligands may dictate which neuropeptide ends up binding and inducing its action. For example, GALR3 has a higher affinity for SPX than GAL (1), thus, GALR3-related actions are likely the result of SPX binding. SPX and GAL interaction with other circulating factors, such as neurotransmitters or neuropeptides, may also influence downstream effects. On top of that, upstream regulation or external factors of SPX and GAL, such as fatty acids or steroid, certain physiological conditions and states, or other neuropeptides, can likely determine which neuropeptide will exert its action on which receptor. Accordingly, estradiol, as an upstream regulator, can suppress SPX expression in the spotted scat hypothalamus (42) while GAL expression is induced in GnRH neurons of rats (116). The complexity increases with GALR2’s ability to bind to different G proteins. A possible speculation is that GALR2-G-protein interaction is dependent on cell type although this warrants further investigation.
In relation to appetite regulation, GALR1 activation lead to increased food intake as per GAL’s role (68). Restricted feeding increased hypothalamic GALR2 in mice while GALR2 activation reduced food intake (54, 67). In addition, there is the likelihood that SPX may act on hypothalamic GALR3 to suppress appetite-stimulating gene expression, meaning that SPX can act via both GALR2 and GALR3 as a satiety factor (48). In zebrafish, GALR2b upregulation with consequent GALR2a reduction in the brain is linked with satiety (47). This suggests GALR2b as SPX’s receptor in appetite regulation in non-mammalian vertebrates.
Single cell analysis of GnRH neurons identified GALR1 expression, but not GALR2 or GALR3 (73). In another study, GALR1 and GALR2 were detected in mice GnRH cells (117). However, it was found that GALR2 and GALR3 had low basal levels and were stimulated by fatty acids in vitro (80). It is possible that physiological levels of GALR2 is low in GnRH neurons and can be stimulated during abnormal conditions, such as high circulating fatty acid levels. At this point, it is unknown if GAL or SPX can act on GALR2 to mediate dysfunction on GnRH cells. GALRs are also expressed in the pituitary, and both SPX and GAL act to suppress or stimulate LH/FSH release in vitro, respectively (35, 69).
GALR2 is the likely receptor for SPX-induced anxiolytic functions. This seems to be the case in zebrafish and mice (9, 54, 83). In fact, a SPX-based GALR2 agonist was developed and can induce anxiolytic effects as well as regulate appetite, even when given intranasally (54, 118). Most studies implicate GALR2 as an anti-depressive, while in contrast, GAL likely acts on GALR1 and GALR3 to induce changes to the serotonergic system, resulting in pro-depressive effects (70, 71).
In summary, SPX and GAL are two neuropeptides that have been receiving considerable interest in metabolic and reproductive medical research. GALRs mediate the actions of both SPX and GAL. While GAL can bind to all three GALRs, SPX can only act on GALR2 and GALR3. A significant amount of evidence describes the involvement of SPX and GAL in metabolic functions and social behaviours, including reproduction, stress responses and emotional control in non-mammalian and mammalian vertebrates. SPX acts as a satiety factor and is affected by nutritional conditions, while GAL is an orexigenic factor. SPX inhibits gonadotropin release, while GAL modulates gonadotropin release by acting on the pituitary or on GnRH neurons. SPX and GAL are involved in the body’s stress response. Further, SPX and GAL are also involved in depression and anxiety, where studies in fish have shown a possible anxiolytic function of SPX. Although lacking in fish studies, GAL’s pro-depressive and anxiety functions in rodents is clear; however, its effects depend on the receptor it interacts with. GAL and SPX are important neuropeptides for feeding, energy metabolism, reproduction, and emotional control via the correlation with other neurons and the three GALRs in the brain. GAL and SPX likely contribute to balancing the functions of the complex neuroendocrine system. In the future, more understanding of the physiological effects of GAL and SPX with three GALRs could lead to the development of new therapeutic strategies such as neuroendocrine-based obesity elimination and reproductive abnormalities using GAL and SPX.
IMZ wrote the initial draft of the manuscript and drew the figures. TS, SO, and NAD edited the manuscript. ISP conceptualized and edited the manuscript. All authors contributed to the article and approved the submitted version.
This work was supported by the JCSMHS SEED GRANT SED-000082 (2020) (TS*, SO, NAD, IMZ, ISP) from School of Medicine and Health Sciences, Monash University Malaysia. IMZ is the recipient of Monash University Malaysia Graduate Research Merit Scholarship.
The authors declare that the research was conducted in the absence of any commercial or financial relationships that could be construed as a potential conflict of interest.
All claims expressed in this article are solely those of the authors and do not necessarily represent those of their affiliated organizations, or those of the publisher, the editors and the reviewers. Any product that may be evaluated in this article, or claim that may be made by its manufacturer, is not guaranteed or endorsed by the publisher.
1. Kim D-K, Yun S, Son GH, Hwang J-I, Park CR, Kim JI, et al. Coevolution of the Spexin/Galanin/Kisspeptin Family: Spexin Activates Galanin Receptor Type II and III. Endocrinology (2014) 155(5):1864–73. doi: 10.1210/en.2013-2106
2. Lim CH, Lee MYM, Soga T, Parhar I. Evolution of Structural and Functional Diversity of Spexin in Mammalian and Non-Mammalian Vertebrate Species. Front Endocrinol (2019) 10:379. doi: 10.3389/fendo.2019.00379
3. Mills EG, Izzi-Engbeaya C, Abbara A, Comninos AN, Dhillo WS. Functions of Galanin, Spexin and Kisspeptin in Metabolism, Mood and Behaviour. Nat Rev Endocrinol (2021) 17(2):97–113. doi: 10.1038/s41574-020-00438-1
4. Merchenthaler I, Rotoli G, Grignol G, Dudas B. Intimate Associations Between the Neuropeptide Y System and the Galanin-Immunoreactive Neurons in the Human Diencephalon. Neuroscience (2010) 170(3):839–45. doi: 10.1016/j.neuroscience.2010.07.003
5. Godoy LD, Rossignoli MT, Delfino-Pereira P, Garcia-Cairasco N, de Lima Umeoka EH. A Comprehensive Overview on Stress Neurobiology: Basic Concepts and Clinical Implications. Front Behav Neurosci (2018) 12:127. doi: 10.3389/fnbeh.2018.00127
6. Batten TFC, Moons L, Cambre M, Vandesande F. Anatomical Distribution of Galanin-Like Immunoreactivity in the Brain and Pituitary of Teleost Fishes. Neurosci Lett (1990) 111(1):12–7. doi: 10.1016/0304-3940(90)90336-8
7. Lakić I, Jevdović T, Jasnić N, Dakić T, Vujović P, Dordević J. Stress-Specific Changes of Galanin and Pacap Expression in the Rat Hypothalamus and Adrenal Gland. Arch Biol Sci (2018) 70(3):481–8. doi: 10.2298/ABS180228008L
8. Lim CH, Soga T, Levavi-Sivan B, Parhar IS. Chronic Social Defeat Stress Up-Regulates Spexin in the Brain of Nile Tilapia (Oreochromis Niloticus). Sci Rep (2020) 10(1):7666. doi: 10.1038/s41598-020-64639-4
9. Jeong I, Kim E, Seong JY, Park H-C. Overexpression of Spexin 1 in the Dorsal Habenula Reduces Anxiety in Zebrafish. Front Neural Circuits (2019) 13:53. doi: 10.3389/fncir.2019.00053
10. Kehr J, Yoshitake T, Wang FH, Razani H, Gimenez-Llort L, Jansson A, et al. Galanin Is a Potent in Vivo Modulator of Mesencephalic Serotonergic Neurotransmission. Neuropsychopharmacology (2002) 27(3):341–56. doi: 10.1016/s0893-133x(02)00309-3
11. Tatemoto K, Rökaeus Å, Jörnvall H, McDonald TJ, Mutt V. Galanin — A Novel Biologically Active Peptide From Porcine Intestine. FEBS Lett (1983) 164(1):124–8. doi: 10.1016/0014-5793(83)80033-7
12. Anglade I, Wang Y, Jensen J, Tramu G, Kah O, Conlon JM. Characterization of Trout Galanin and Its Distribution in Trout Brain and Pituitary. J Comp Neurol (1994) 350(1):63–74. doi: 10.1002/cne.903500105
13. Bersani M, Johnsen AH, Højrup P, Dunning BE, Andreasen JJ, Holst JJ. Human Galanin: Primary Structure and Identification of Two Molecular Forms. FEBS Lett (1991) 283(2):189–94. doi: 10.1016/0014-5793(91)80585-q
14. Wang Y, Barton BA, Thim L, Nielsen PF, Conlon JM. Purification and Characterization of Galanin and Scyliorhinin I From the Hybrid Sturgeon,Scaphirhynchus Platorynchus×Scaphirhynchus Albus(Acipenseriformes). Gen Comp Endocrinol (1999) 113(1):38–45. doi: 10.1006/gcen.1998.7174
15. Webling KEB, Runesson J, Bartfai T, Langel U. Galanin Receptors and Ligands. Front Endocrinol (2012) 3:146. doi: 10.3389/fendo.2012.00146
16. Rökaeus A, Brownstein MJ. Construction of a Porcine Adrenal Medullary Cdna Library and Nucleotide Sequence Analysis of Two Clones Encoding a Galanin Precursor. Proc Natl Acad Sci USA (1986) 83(17):6287–91. doi: 10.1073/pnas.83.17.6287
17. Ohtaki T, Kumano S, Ishibashi Y, Ogi K, Matsui H, Harada M, et al. Isolation and Cdna Cloning of a Novel Galanin-Like Peptide (Galp) From Porcine Hypothalamus. J Biol Chem (1999) 274(52):37041–5. doi: 10.1074/jbc.274.52.37041
18. Santic R, Schmidhuber SM, Lang R, Rauch I, Voglas E, Eberhard N, et al. Alarin Is a Vasoactive Peptide. Proc Natl Acad Sci USA (2007) 104(24):10217–22. doi: 10.1073/pnas.0608585104
19. Unniappan S, Cerdá-Reverter JM, Peter RE. In Situ Localization of Preprogalanin Mrna in the Goldfish Brain and Changes in Its Expression During Feeding and Starvation. Gen Comp Endocrinol (2004) 136(2):200–7. doi: 10.1016/j.ygcen.2003.12.010
20. Józsa R, Mess B. Galanin-Like Immunoreactivity in the Chicken Brain. Cell Tissue Res (1993) 273(2):391–9. doi: 10.1007/bf00312842
21. Jiménez AJ, Mancera JM, Pérez-Fígares JM, Fernández-Llebrez P. Distribution of Galanin-Like Immunoreactivity in the Brain of the Turtle Mauremys Caspica. J Comp Neurol (1994) 349(1):73–84. doi: 10.1002/cne.903490106
22. Azumaya Y, Tsutsui K. Localization of Galanin and Its Binding Sites in the Quail Brain. Brain Res (1996) 727(1-2):187–95. doi: 10.1016/0006-8993(96)00379-4
23. Cohen Y, Hausken K, Bonfil Y, Gutnick M, Levavi-Sivan B. Spexin and a Novel Cichlid-Specific Spexin Paralog Both Inhibit Fsh and Lh Through a Specific Galanin Receptor (Galr2b) in Tilapia. Front Endocrinol (2020) 11:71. doi: 10.3389/fendo.2020.00071
24. Martins RST, Pinto PIS, Guerreiro PM, Zanuy S, Carrillo M, Canário AVM. Novel Galanin Receptors in Teleost Fish: Identification, Expression and Regulation by Sex Steroids. Gen Comp Endocrinol (2014) 205:109–20. doi: 10.1016/j.ygcen.2014.06.030
25. Bai YF, Ma HT, Liu LN, Li H, Li XX, Yang YT, et al. Activation of Galanin Receptor 1 Inhibits Locus Coeruleus Neurons Via Girk Channels. Biochem Biophys Res Commun (2018) 503(1):79–85. doi: 10.1016/j.bbrc.2018.05.181
26. Kolakowski LF Jr., O'Neill GP, Howard AD, Broussard SR, Sullivan KA, Feighner SD, et al. Molecular Characterization and Expression of Cloned Human Galanin Receptors Galr2 and Galr3. J Neurochem (1998) 71(6):2239–51. doi: 10.1046/j.1471-4159.1998.71062239.x
27. Kim E, Jeong I, Kim S, Kim H-K, Lee D-W, Kim B, et al. Distribution of Galanin Receptor 2b Neurons and Interaction With Galanin in the Zebrafish Central Nervous System. Neurosci Lett (2016) 628:153–60. doi: 10.1016/j.neulet.2016.06.025
28. Li L, Wei S, Huang Q, Feng D, Zhang S, Liu Z. A Novel Galanin Receptor 1a Gene in Zebrafish: Tissue Distribution, Developmental Expression Roles in Nutrition Regulation. Comp Biochem Physiol - B Biochem Mol Biol (2013) 164(3):159–67. doi: 10.1016/j.cbpb.2012.12.004
29. Ho JCW, Jacobs T, Wang Y, Leung FC. Identification and Characterization of the Chicken Galanin Receptor Galr2 and a Novel Galr2-Like Receptor (Galr2-L). Gen Comp Endocrinol (2012) 179(2):305–12. doi: 10.1016/j.ygcen.2012.09.005
30. Ho JCW, Kwok AHY, Zhao D, Wang Y, Leung FC. Characterization of the Chicken Galanin Type I Receptor (Galr1) and a Novel Galr1-Like Receptor (Galr1-L). Gen Comp Endocrinol (2011) 170(2):391–400. doi: 10.1016/j.ygcen.2010.10.011
31. Mirabeau O, Perlas E, Severini C, Audero E, Gascuel O, Possenti R, et al. Identification of Novel Peptide Hormones in the Human Proteome by Hidden Markov Model Screening. Genome Res (2007) 17(3):320–7. doi: 10.1101/gr.5755407
32. Sonmez K, Zaveri NT, Kerman IA, Burke S, Neal CR, Xie X, et al. Evolutionary Sequence Modeling for Discovery of Peptide Hormones. PloS Comput Biol (2009) 5(1):e1000258–e. doi: 10.1371/journal.pcbi.1000258
33. Wan B, Wang X-R, Zhou Y-B, Zhang X, Huo K, Han Z-G. C12orf39, a Novel Secreted Protein With a Typical Amidation Processing Signal. Biosci Rep (2009) 30(1):1–10. doi: 10.1042/bsr20080156
34. Li S, Liu Q, Xiao L, Chen H, Li G, Zhang Y, et al. Molecular Cloning and Functional Characterization of Spexin in Orange-Spotted Grouper (Epinephelus Coioides). Comp Biochem Physiol Part - B: Biochem Mol Biol (2016) 196-197:85–91. doi: 10.1016/j.cbpb.2016.02.009
35. Liu Y, Li S, Qi X, Zhou W, Liu X, Lin H, et al. A Novel Neuropeptide in Suppressing Luteinizing Hormone Release in Goldfish, Carassius Auratus. Mol Cell Endocrinol (2013) 374(1):65–72. doi: 10.1016/j.mce.2013.04.008
36. Lomet D, Robert V, Poissenot K, Beltramo M, Dardente H. No Evidence That Spexin Impacts Lh Release and Seasonal Breeding in the Ewe. Theriogenology (2020) 158:1–7. doi: 10.1016/j.theriogenology.2020.08.031
37. Wang B, Cui A, Tian J, Zhang Y, Jiang Y, Xu Y, et al. Characterization of a Novel Spexin Gene (Spx2) in the Half-Smooth Tongue Sole and Regulation of Its Expression by Nutritional Status. Aquacult Rep (2020) 18:100544. doi: 10.1016/j.aqrep.2020.100544
38. Wang S, Wang B, Chen S. Spexin in the Half-Smooth Tongue Sole (Cynoglossus Semilaevis): Molecular Cloning, Expression Profiles, and Physiological Effects. Fish Physiol Biochem (2018) 44(3):829–39. doi: 10.1007/s10695-018-0472-6
39. Wong MKH, Sze KH, Chen T, Cho CK, Law HCH, Chu IK, et al. Goldfish Spexin: Solution Structure and Novel Function as a Satiety Factor in Feeding Control. Am J Physiol - Endocrinol Metab (2013) 305(3):E348–E66. doi: 10.1152/ajpendo.00141.2013
40. Kim E, Jeong I, Chung A-Y, Kim S, Kwon S-H, Seong JY, et al. Distribution and Neuronal Circuit of Spexin 1/2 Neurons in the Zebrafish Cns. Sci Rep (2019) 9(1):5025. doi: 10.1038/s41598-019-41431-7
41. Wu H, Lin F, Chen H, Liu J, Gao Y, Zhang X, et al. Ya-Fish (Schizothorax Prenanti) Spexin: Identification, Tissue Distribution and Mrna Expression Responses to Periprandial and Fasting. Fish Physiol Biochem (2016) 42(1):39–49. doi: 10.1007/s10695-015-0115-0
42. Deng S-P, Chen H-P, Zhai Y, Jia L-Y, Liu J-Y, Wang M, et al. Molecular Cloning, Characterization and Expression Analysis of Spexin in Spotted Scat (Scatophagus Argus). Gen Comp Endocrinol (2018) 266:60–6. doi: 10.1016/j.ygcen.2018.04.018
43. Tian Z, Xu S, Wang M, Li Y, Chen H, Tang N, et al. Identification, Tissue Distribution, Periprandial Expression, and Anorexigenic Effect of Spexin in Siberian Sturgeon. Acipenser Baeri Fish Physiol Biochem (2020) 46(6):2073–84. doi: 10.1007/s10695-020-00856-y
44. Pałasz A, Suszka-Świtek A, Kaśkosz A, Plewka D, Bogus K, Filipczyk Ł, et al. Spexin-Expressing Neurons in the Magnocellular Nuclei of the Human Hypothalamus. J Chem Neuroanat (2021) 111:1–6. doi: 10.1016/j.jchemneu.2020.101883
45. Sivalingam M, Parhar IS. Hypothalamic Kisspeptin and Kisspeptin Receptors: Species Variation in Reproduction and Reproductive Behaviours. Front Neuroendocrinol (2022) 64:100951. doi: 10.1016/j.yfrne.2021.100951
46. Ma A, He M, Bai J, Wong MK, Ko WK, Wong AO. Dual Role of Insulin in Spexin Regulation: Functional Link Between Food Intake and Spexin Expression in a Fish Model. Endocrinology (2017) 158(3):560–77. doi: 10.1210/en.2016-1534
47. Zheng B, Li S, Liu Y, Li Y, Chen H, Tang H, et al. Spexin Suppress Food Intake in Zebrafish: Evidence From Gene Knockout Study. Sci Rep (2017) 7(1):1–9. doi: 10.1038/s41598-017-15138-6
48. Lv S, Zhou Y, Feng Y, Zhang X, Wang X, Yang Y, et al. Peripheral Spexin Inhibited Food Intake in Mice. Int J Endocrinol (2020) 2020:1–9. doi: 10.1155/2020/4913785
49. Walewski JL, Ge F, Lobdell Iv H, Levin N, Schwartz GJ, Vasselli JR, et al. Spexin Is a Novel Human Peptide That Reduces Adipocyte Uptake of Long Chain Fatty Acids and Causes Weight Loss in Rodents With Diet-Induced Obesity. Obesity (2014) 22(7):1643–52. doi: 10.1002/oby.20725
50. Ha N-H, Kim NH, Kim C-H, Song M-G, Lee H-J, Jin B-Y, et al. Hypothalamic Spexin and Galanin Receptor 2 Are Novel Regulators of Energy Balance in Mice. Diabetes (2018) 67(Supplement 1):1800–P. doi: 10.2337/db18-1800-P
51. Kolodziejski PA, Pruszynska-Oszmalek E, Micker M, Skrzypski M, Wojciechowicz T, Szwarckopf P, et al. Spexin: A Novel Regulator of Adipogenesis and Fat Tissue Metabolism. Biochim Biophys Acta - Mol Cell Biol Lipids (2018) 1863(10):1228–36. doi: 10.1016/j.bbalip.2018.08.001
52. Kołodziejski PA, Pruszyńska-Oszmałek E, Hejdysz M, Sassek M, Leciejewska N, Ziarniak K, et al. Effect of Fasting on the Spexin System in Broiler Chickens. Anim (Basel) (2021) 11(2):1–11. doi: 10.3390/ani11020518
53. Yun R, Dourmashkin JT, Hill J, Gayles EC, Fried SK, Leibowitz SF. Pvn Galanin Increases Fat Storage and Promotes Obesity by Causing Muscle to Utilize Carbohydrate More Than Fat. Peptides (2005) 26(11):2265–73. doi: 10.1016/j.peptides.2005.04.005
54. Yun S, Reyes-Alcaraz A, Lee YN, Yong HJ, Choi J, Ham BJ, et al. Spexin-Based Galanin Receptor Type 2 Agonist for Comorbid Mood Disorders and Abnormal Body Weight. Front Neurosci (2019) 13:391. doi: 10.3389/fnins.2019.00391
55. Tran A, Loganathan N, McIlwraith EK, Belsham DD. Palmitate and Nitric Oxide Regulate the Expression of Spexin and Galanin Receptors 2 and 3 in Hypothalamic Neurons: Nitric Oxide and Palmitate Regulate Spx, Galr2 and Galr3 Mrna. Neuroscience (2020) 447:41–52. doi: 10.1016/j.neuroscience.2019.10.028
56. Behrooz M, Vaghef-Mehrabany E, Ostadrahimi A. Different Spexin Level in Obese Vs Normal Weight Children and Its Relationship With Obesity Related Risk Factors. Nutrition Metab Cardiovasc Dis (2020) 30(4):674–82. doi: 10.1016/j.numecd.2019.11.008
57. Khadir A, Kavalakatt S, Madhu D, Devarajan S, Abubaker J, Al-Mulla F, et al. Spexin as an Indicator of Beneficial Effects of Exercise in Human Obesity and Diabetes. Sci Rep (2020) 10(1):10635. doi: 10.1038/s41598-020-67624-z
58. Gu L, Ma Y, Gu M, Zhang Y, Yan S, Li N, et al. Spexin Peptide Is Expressed in Human Endocrine and Epithelial Tissues and Reduced After Glucose Load in Type 2 Diabetes. Peptides (2015) 71:232–9. doi: 10.1016/j.peptides.2015.07.018
59. Farrell WJ, Böttger B, Ahmadi F, Finger TE. Distribution of Cholecystokinin, Calcitonin Gene-Related Peptideand Galanin in the Primary Gustatory Nuclei of the Goldfish. J Comp Neurol (2002) 450(2):103–14. doi: 10.1002/cne.10317
60. McConn BR, Gilbert ER, Cline MA. Fasting and Refeeding Induce Differential Changes in Hypothalamic Mrna Abundance of Appetite-Associated Factors in 7 Day-Old Japanese Quail, Coturnix Japonica. Comp Biochem Physiol A Mol Integr Physiol (2019) 227:60–7. doi: 10.1016/j.cbpa.2018.08.015
61. de Pedro N, Céspedes MV, Delgado MJ, Alonso-Bedate M. The Galanin-Induced Feeding Stimulation Is Mediated Via Alpha 2-Adrenergic Receptors in Goldfish. Regul Pept (1995) 57(1):77–84. doi: 10.1016/0167-0115(95)91255-4
62. Tachibana T, Mori M, Khan MS, Ueda H, Sugahara K, Hiramatsu K. Central Administration of Galanin Stimulates Feeding Behavior in Chicks. Comp Biochem Physiol A Mol Integr Physiol (2008) 151(4):637–40. doi: 10.1016/j.cbpa.2008.08.001
63. Kyrkouli SE, Stanley BG, Leibowitz SF. Galanin: Stimulation of Feeding Induced by Medial Hypothalamic Injection of This Novel Peptide. Eur J Pharmacol (1986) 122(1):159–60. doi: 10.1016/0014-2999(86)90175-5
64. Kyrkouli SE, Stanley BG, Seirafi RD, Leibowitz SF. Stimulation of Feeding by Galanin: Anatomical Localization and Behavioral Specificity of This Peptide's Effects in the Brain. Peptides (1990) 11(5):995–1001. doi: 10.1016/0196-9781(90)90023-x
65. Corwin RL, Robinson JK, Crawley JN. Galanin Antagonists Block Galanin-Induced Feeding in the Hypothalamus and Amygdala of the Rat. Eur J Neurosci (1993) 5(11):1528–33. doi: 10.1111/j.1460-9568.1993.tb00221.x
66. Tempel DL, Leibowitz KJ, Leibowitz SF. Effects of Pvn Galanin on Macronutrient Selection. Peptides (1988) 9(2):309–14. doi: 10.1016/0196-9781(88)90265-3
67. Sun J, She Y, Fang P, Gu X, Zhang Z. Time-Restricted Feeding Prevents Metabolic Diseases Through the Regulation of Galanin/Galr1 Expression in the Hypothalamus of Mice. Eat Weight Disord (2021) 27:1415–25. doi: 10.1007/s40519-021-01280-8
68. Saar I, Runesson J, McNamara I, Järv J, Robinson JK, Langel Ü. Novel Galanin Receptor Subtype Specific Ligands in Feeding Regulation. Neurochem Int (2011) 58(6):714–20. doi: 10.1016/j.neuint.2011.02.012
69. Sahu A, Xu B, Kalra SP. Role of Galanin in Stimulation of Pituitary Luteinizing Hormone Secretion as Revealed by a Specific Receptor Antagonist, Galantide. Endocrinology (1994) 134(2):529–36. doi: 10.1210/endo.134.2.7507825
70. Razani H, Diaz-Cabiale Z, Fuxe K, Ogren SO. Intraventricular Galanin Produces a Time-Dependent Modulation of 5-Ht1a Receptors in the Dorsal Raphe of the Rat. Neuroreport (2000) 11(18):3943–8. doi: 10.1097/00001756-200012180-00008
71. Kuteeva E, Wardi T, Hökfelt T, Ogren SO. Galanin Enhances and a Galanin Antagonist Attenuates Depression-Like Behaviour in the Rat. Eur Neuropsychopharmacol (2007) 17(1):64–9. doi: 10.1016/j.euroneuro.2006.03.003
72. Leiva LA, Croxatto HB. Comparison of the Effect of Hypothalamic Neuropeptides Upon Luteinizing Hormone Secretion by Cultured Rat Anterior Pituitary Cells. Biol Res (1994) 27(2):113–21.
73. Constantin S, Wray S. Galanin Activates G Protein Gated Inwardly Rectifying Potassium Channels and Suppresses Kisspeptin-10 Activation of Gnrh Neurons. Endocrinology (2016) 157(8):3197–212. doi: 10.1210/en.2016-1064
74. Malendowicz LK, Nussdorfer GG, Nowak KW, Mazzocchi G. The Possible Involvement of Galanin in the Modulation of the Function of Rat Pituitary-Adrenocortical Axis Under Basal and Stressful Conditions. Endocr Res (1994) 20(3):307–17. doi: 10.1080/07435809409035866
75. Baranowska B, Wasilewska-Dziubińska E, Radzikowska M, Płonowski A, Roguski K, Neuropeptide Y. Galanin, and Leptin Release in Obese Women and in Women With Anorexia Nervosa. Metabolism (1997) 46(12):1384–9. doi: 10.1016/s0026-0495(97)90136-0
76. Sandoval-Alzate HF, Agudelo-Zapata Y, González-Clavijo AM, Poveda NE, Espinel-Pachón CF, Escamilla-Castro JA, et al. Serum Galanin Levels in Young Healthy Lean and Obese Non-Diabetic Men During an Oral Glucose Tolerance Test. Sci Rep (2016) 6:31661. doi: 10.1038/srep31661
77. Fang P, Bo P, Shi M, Yu M, Zhang Z. Circulating Galanin Levels Are Increased in Patients With Gestational Diabetes Mellitus. Clin Biochem (2013) 46(9):831–3. doi: 10.1016/j.clinbiochem.2012.12.013
78. Beck B. Neuropeptide Y in Normal Eating and in Genetic and Dietary-Induced Obesity. Philos Trans R Soc Lond B Biol Sci (2006) 361(1471):1159–85. doi: 10.1098/rstb.2006.1855
79. Tang H, Liu Y, Luo D, Ogawa S, Yin Y, Li S, et al. The Kiss/Kissr Systems Are Dispensable for Zebrafish Reproduction: Evidence From Gene Knockout Studies. Endocrinology (2015) 156(2):589–99. doi: 10.1210/en.2014-1204
80. Wang L, Tran A, Lee J, Belsham DD. Palmitate Differentially Regulates Spexin, and Its Receptors Galr2 and Galr3, in Gnrh Neurons Through Mechanisms Involving Pkc, Mapks, and Tlr4. Mol Cell Endocrinol (2020) 518:110991. doi: 10.1016/j.mce.2020.110991
81. Fullston T, Wu L, Teede HJ, Moran LJ. Chapter 11 - Obesity and Reproductive Dysfunction in Men and Women. In: Watson RR, editor. Handbook of Fertility. San Diego: Academic Press (2015). p. 119–32.
82. Guler A, Demir İ. Decreased Levels of Spexin Are Associated With Hormonal and Metabolic Disturbance in Subjects With Polycystic Ovary Syndrome. J Obstet Gynaecol (2021) 41(3):408–13. doi: 10.1080/01443615.2020.1737660
83. Zhuang M, Lai Q, Yang C, Ma Y, Fan B, Bian Z, et al. Spexin as an Anxiety Regulator in Mouse Hippocampus: Mechanisms for Transcriptional Regulation of Spexin Gene Expression by Corticotropin Releasing Factor. Biochem Biophys Res Commun (2020) 525(2):326–33. doi: 10.1016/j.bbrc.2020.02.023
84. Reyes-Alcaraz A, Lee YN, Son GH, Kim NH, Kim DK, Yun S, et al. Development of Spexin-Based Human Galanin Receptor Type Ii-Specific Agonists With Increased Stability in Serum and Anxiolytic Effect in Mice. Sci Rep (2016) 6:1–10. doi: 10.1038/srep21453
85. Magliulo-Cepriano L, Schreibman MP, Blüm V. The Distribution of Immunoreactive Fmrf-Amide, Neurotensin, and Galanin in the Brain and Pituitary Gland of Three Species of Xiphophorus From Birth to Sexual Maturity. Gen Comp Endocrinol (1993) 92(2):269–80. doi: 10.1006/gcen.1993.1163
86. Yamamoto T, Maler L, Nagy JI. Organization of Galanin-Like Immunoreactive Neuronal Systems in Weakly Electric Fish (Apteronotus Leptorhynchus). J Chem Neuroanat (1992) 5(1):19–38. doi: 10.1016/0891-0618(92)90031-K
87. Cornbrooks EB, Parsons RL. Sexually Dimorphic Distribution of a Galanin-Like Peptide in the Central Nervous System of the Teleost Fish Poecilia Latipinna. J Comp Neurol (1991) 304(4):639–57. doi: 10.1002/cne.903040410
88. Olivereau M, Olivereau JM. Immunocytochemical Localization of a Galanin-Like Peptidergic System in the Brain and Pituitary of Some Teleost Fish. Histochemistry (1991) 96(4):343–54. doi: 10.1007/bf00271356
89. Martins RST, Sousa C, Andrade AR, Molés G, Zanuy S, Gómez A, et al. Galanin Isoforms by Alternative Splicing: Structure, Expression, and Immunohistochemical Location in the Gonads of European Sea Bass. Gen Comp Endocrinol (2021) 305:113730. doi: 10.1016/j.ygcen.2021.113730
90. Jadhao AG, Meyer DL. Sexually Dimorphic Distribution of Galanin in the Preoptic Area of Red Salmon, Oncorhynchus Nerka. Cell Tissue Res (2000) 302(2):199–203. doi: 10.1007/s004410000278
91. Rao PD, Murthy CK, Cook H, Peter RE. Sexual Dimorphism of Galanin-Like Immunoreactivity in the Brain and Pituitary of Goldfish, Carassius Auratus. J Chem Neuroanat (1996) 10(2):119–35. doi: 10.1016/0891-0618(96)00110-x
92. Rodríguez MA, Anadón R, Rodríguez-Moldes I. Development of Galanin-Like Immunoreactivity in the Brain of the Brown Trout (Salmo Trutta Fario), With Some Observations on Sexual Dimorphism. J Comp Neurol (2003) 465(2):263–85. doi: 10.1002/cne.10832
93. Tripp JA, Salas-Allende I, Makowski A, Bass AH. Mating Behavioral Function of Preoptic Galanin Neurons Is Shared Between Fish With Alternative Male Reproductive Tactics and Tetrapods. J Neurosci (2020) 40(7):1549–59. doi: 10.1523/jneurosci.1276-19.2019
94. Merchenthaler I, López FJ, Lennard DE, Negro-Vilar A. Sexual Differences in the Distribution of Neurons Coexpressing Galanin and Luteinizing Hormone-Releasing Hormone in the Rat Brain. Endocrinology (1991) 129(4):1977–86. doi: 10.1210/endo-129-4-1977
95. Dufourny L, Schofield N, Skinner DC. Immunoreactive Galanin Expression in Ovine Gonadotropin-Releasing Hormone Neurones: No Effects of Gender or Reproductive Status. J Neuroendocrinol (2003) 15(11):1062–9. doi: 10.1046/j.1365-2826.2003.01098.x
96. Mitchell V, Bouret S, Prévot V, Jennes L, Beauvillain JC. Evidence for Expression of Galanin Receptor Gal-R1 Mrna in Certain Gonadotropin Releasing Hormone Neurones of the Rostral Preoptic Area. J Neuroendocrinol (1999) 11(10):805–12. doi: 10.1046/j.1365-2826.1999.00399.x
97. Rajendren G. Increased Galanin Synapses Onto Activated Gonadotropin-Releasing Hormone Neuronal Cell Bodies in Normal Female Mice and in Functional Preoptic Area Grafts in Hypogonadal Mice. J Neuroendocrinol (2002) 14(6):435–41. doi: 10.1046/j.1365-2826.2002.00796.x
98. Wu Z, Autry AE, Bergan JF, Watabe-Uchida M, Dulac CG. Galanin Neurons in the Medial Preoptic Area Govern Parental Behaviour. Nature (2014) 509(7500):325–30. doi: 10.1038/nature13307
99. Giustina A, Gastaldi C, Bugari G, Chiesa L, Loda G, Tironi C, et al. Role of Galanin in the Regulation of Somatotrope and Gonadotrope Function in Young Ovulatory Women. Metabolism (1995) 44(8):1028–32. doi: 10.1016/0026-0495(95)90100-0
100. Baranowska B, Radzikowska M, Wasilewska-Dziubińska E, Kapliński A, Roguski K, Płonowski A. Neuropeptide Y, Leptin, Galanin and Insulin in Women With Polycystic Ovary Syndrome. Gynecol Endocrinol (1999) 13(5):344–51. doi: 10.3109/09513599909167578
101. Wojtkiewicz J, Jana B, Kozłowska A, Crayton R, Majewski M, Zalecki M, et al. Innervation Pattern of Polycystic Ovaries in the Women. J Chem Neuroanat (2014) 61-62:147–52. doi: 10.1016/j.jchemneu.2014.05.003
102. Pavlidis M, Sundvik M, Chen Y-C, Panula P. Adaptive Changes in Zebrafish Brain in Dominant–Subordinate Behavioral Context. Behav Brain Res (2011) 225(2):529–37. doi: 10.1016/j.bbr.2011.08.022
103. Kozlovsky N, Matar MA, Kaplan Z, Zohar J, Cohen H. The Role of the Galaninergic System in Modulating Stress-Related Responses in an Animal Model of Posttraumatic Stress Disorder. Biol Psychiatry (2009) 65(5):383–91. doi: 10.1016/j.biopsych.2008.10.034
104. Palkovits M. Stress-Induced Expression of Co-Localized Neuropeptides in Hypothalamic and Amygdaloid Neurons. Eur J Pharmacol (2000) 405(1-3):161–6. doi: 10.1016/s0014-2999(00)00549-5
105. Holmes A, Li Q, Koenig EA, Gold E, Stephenson D, Yang RJ, et al. Phenotypic Assessment of Galanin Overexpressing and Galanin Receptor R1 Knockout Mice in the Tail Suspension Test for Depression-Related Behavior. Psychopharmacology (2005) 178(2):276–85. doi: 10.1007/s00213-004-1997-1
106. Bergonzelli GE, Pralong FP, Glauser M, Cavadas C, Grouzmann E, Gaillard RC. Interplay Between Galanin and Leptin in the Hypothalamic Control of Feeding Via Corticotropin-Releasing Hormone and Neuropeptide Y. Diabetes (2001) 50(12):2666–72. doi: 10.2337/diabetes.50.12.2666
107. Tillage RP, Wilson GE, Liles LC, Holmes PV, Weinshenker D. Chronic Environmental or Genetic Elevation of Galanin in Noradrenergic Neurons Confers Stress Resilience in Mice. J Neurosci (2020) 40(39):7464–74. doi: 10.1523/jneurosci.0973-20.2020
108. Melander T, Hökfelt T, Rökaeus A, Cuello AC, Oertel WH, Verhofstad A, et al. Coexistence of Galanin-Like Immunoreactivity With Catecholamines, 5-Hydroxytryptamine, Gaba and Neuropeptides in the Rat Cns. J Neurosci (1986) 6(12):3640–54. doi: 10.1523/jneurosci.06-12-03640.1986
109. Xu ZQ, Hökfelt T. Expression of Galanin and Nitric Oxide Synthase in Subpopulations of Serotonin Neurons of the Rat Dorsal Raphe Nucleus. J Chem Neuroanat (1997) 13(3):169–87. doi: 10.1016/s0891-0618(97)00043-4
110. Seutin V, Verbanck P, Massotte L, Dresse A. Galanin Decreases the Activity of Locus Coeruleus Neurons in Vitro. Eur J Pharmacol (1989) 164(2):373–6. doi: 10.1016/0014-2999(89)90481-0
111. Funck VR, Fracalossi MP, Vidigal APP, Beijamini V. Dorsal Hippocampal Galanin Modulates Anxiety-Like Behaviours in Rats. Brain Res (2018) 1687:74–81. doi: 10.1016/j.brainres.2018.02.036
112. Kuteeva E, Wardi T, Lundström L, Sollenberg U, Langel U, Hökfelt T, et al. Differential Role of Galanin Receptors in the Regulation of Depression-Like Behavior and Monoamine/Stress-Related Genes at the Cell Body Level. Neuropsychopharmacology (2008) 33(11):2573–85. doi: 10.1038/sj.npp.1301660
113. de Souza MM, Silote GP, Herbst LS, Funck VR, Joca SRL, Beijamini V. The Antidepressant-Like Effect of Galanin in the Dorsal Raphe Nucleus of Rats Involves Gal(2) Receptors. Neurosci Lett (2018) 681:26–30. doi: 10.1016/j.neulet.2018.05.029
114. Swanson CJ, Blackburn TP, Zhang X, Zheng K, Xu ZQ, Hökfelt T, et al. Anxiolytic- and Antidepressant-Like Profiles of the Galanin-3 Receptor (Gal3) Antagonists Snap 37889 and Snap 398299. Proc Natl Acad Sci USA (2005) 102(48):17489–94. doi: 10.1073/pnas.0508970102
115. Juhasz G, Hullam G, Eszlari N, Gonda X, Antal P, Anderson IM, et al. Brain Galanin System Genes Interact With Life Stresses in Depression-Related Phenotypes. Proc Natl Acad Sci USA (2014) 111(16):E1666–E73. doi: 10.1073/pnas.1403649111
116. Rossmanith WG, Marks DL, Clifton DK, Steiner RA. Induction of Galanin Mrna in Gnrh Neurons by Estradiol and Its Facilitation by Progesterone. J Neuroendocrinol (1996) 8(3):185–91. doi: 10.1046/j.1365-2826.1996.04473.x
117. Todman MG, Han SK, Herbison AE. Profiling Neurotransmitter Receptor Expression in Mouse Gonadotropin-Releasing Hormone Neurons Using Green Fluorescent Protein-Promoter Transgenics and Microarrays. Neuroscience (2005) 132(3):703–12. doi: 10.1016/j.neuroscience.2005.01.035
Keywords: neuropeptide, energy regulation, reproduction, stress, anxiety, depression, hypothalamus
Citation: Mohd Zahir I, Ogawa S, Dominic NA, Soga T and Parhar IS (2022) Spexin and Galanin in Metabolic Functions and Social Behaviors With a Focus on Non-Mammalian Vertebrates. Front. Endocrinol. 13:882772. doi: 10.3389/fendo.2022.882772
Received: 24 February 2022; Accepted: 19 April 2022;
Published: 25 May 2022.
Edited by:
Honoo Satake, Suntory Foundation for Life Sciences, JapanCopyright © 2022 Mohd Zahir, Ogawa, Dominic, Soga and Parhar. This is an open-access article distributed under the terms of the Creative Commons Attribution License (CC BY). The use, distribution or reproduction in other forums is permitted, provided the original author(s) and the copyright owner(s) are credited and that the original publication in this journal is cited, in accordance with accepted academic practice. No use, distribution or reproduction is permitted which does not comply with these terms.
*Correspondence: Ishwar S. Parhar, aXNod2FyQG1vbmFzaC5lZHU=
Disclaimer: All claims expressed in this article are solely those of the authors and do not necessarily represent those of their affiliated organizations, or those of the publisher, the editors and the reviewers. Any product that may be evaluated in this article or claim that may be made by its manufacturer is not guaranteed or endorsed by the publisher.
Research integrity at Frontiers
Learn more about the work of our research integrity team to safeguard the quality of each article we publish.