- 1Guangzhou Institute of Traumatic Surgery, Guangzhou Red Cross Hospital, Jinan University, Guangzhou, China
- 2Department of Neurology, Guangzhou Red Cross Hospital, Jinan University, Guangzhou, China
- 3Department of Otorhinolaryngology, Guangzhou Red Cross Medicine, Jinan University, Guangzhou, China
This study aimed to address the dilemma of low peripheral blood-derived mesenchymal stromal cell (PBMSC) activity and reduced phenotype in bone or cartilage tissue engineering. Rat PBMSCs (rPBMSCs) were obtained by density gradient centrifugation, and stromal cell characteristics were confirmed by flow cytometry (FCM) and multi-differentiation potential induction experiments. Cell growth curve, viability experiments, and clone formation experiments were performed by [3-(4,5-dimethylthiazol-2-yl)-5-(3-carboxymethoxyphenyl)-2-(4-sulfophenyl)-2H-tetrazolium] (MTS) and cell counting, and the cell cycle was confirmed by cell FCM. The proliferation signal pathway and stemness-related proteins were detected by molecular methods including Western blot and real-time polymerase chain reaction. CD73, CD90, and CD105 were highly expressed, and CD14, CD19, CD34, CD45, and HLA-DR were barely expressed in rPBMSCs. rPBMSCs possessed the potential to differentiate into chondrocytes, adipocytes, and osteoblasts under their respective induction conditions. Cell growth curve and viability experiments were performed under hypoxic conditions: 19% O2, 5% O2, and 1% O2. Specifically, 5% O2 accelerated the proliferation and expression of the stemness of PBMSCs. Cycle experiments proved that hypoxia promoted the cell transition from the G1 phase to the S phase. Molecular experiments confirmed that 5% O2 hypoxia significantly elevated the expressions of hypoxia-inducible factor 1α and β-catenin and simultaneously the expressions of cycle-related genes including CyclinE/CDK2 and stemness-related genes including Nanog and SOX2. The appropriate concentration of hypoxia (i.e., 5% O2) enhanced the proliferation and stemness of rPBMSCs and increased the multidirectional differentiation potential of stromal cells. The proposed culture method could improve the viability and maintain the phenotype of rPBMSCs in cartilage or bone tissue engineering.
Introduction
In recent years, mesenchymal stromal cells (MSCs) derived from adults have been widely used not only for bioregenerative tissue engineering but also for pathophysiological research and cell and gene therapy of bone diseases (1–4). Many studies have confirmed that bone marrow-derived MSCs are a relatively stable source, but the low yield and traumatic source of stromal cells had limited preclinical and clinical applications (5, 6). In recent literature, the applications of peripheral blood-derived MSCs (PBMSCs) in tissue engineering have attracted increasing attention because of their relatively easy collection, abundant sources, and multilineage differentiation potential (7, 8).
The committee of the International Society for Cellular Therapy standardized the criteria for defining human MSCs for basic and preclinical research. That is, cells can adhere and the MSC population must positively express CD105, CD73, and CD90 and negatively express CD45, CD34, CD14 or CD11b, CD79a or CD19, and HLA-DR. MSCs also have the potential to differentiate into chondrocytes, osteoblasts, and adipocytes (9). When many cells are needed for research or clinical applications, PBMSCs can take on this important task (10, 11). However, the reduced activity of PBMSCs cultured in vitro and phenotype loss easily limit this demand (11). Therefore, the key to cartilage tissue engineering is to provide phenotype-maintaining MSCs expanded in vitro. Some growth factors or physical factors, such as basic fibroblast growth factor (bFGF) (12), transforming growth factor-β (TGF-β) (13), and oxygen level (14), play a decisive role in stromal cell survival or proliferation. Thus, TGF-β, bFGF, and oxygen levels have affected stromal cell survival or proliferation (12–14). Hypoxia is a simple and easy-to-operate strategy with few side effects. The exposure of MSCs to a hypoxic environment for a moderate time could enhance cell survival characteristics and tissue repair capabilities, and this conclusion was confirmed by recent studies (15). To enhance the therapeutic effect, several studies have conducted hypoxic pretreatments in many disease-related organs and tissues, such as cardiomyocytes (16). In the literature, compared with normoxic conditions, a hypoxic condition significantly promotes MSCs to further express Oct4, cMyc, Nanog, and SOX2. Simultaneously, hypoxia-cultured MSCs exhibited a better growth trend and a higher proportion of S phase cells than normoxia-cultured MSCs (14–16).
Oxygen gradients derived from the bone marrow niche create hypoxic conditions for stromal and stem cells (17). Hypoxia strongly affects several aspects of cell biology, such as angiogenesis, innate immunity, cell proliferation, and stemness (18). The effects of hypoxia on stem cells are usually mediated by HIF-1α and HIF2α (19). The literature reported that incubation of umbilical cord derived mesenchymal stem cells (UC-derived MSCs) with different concentrations of oxygen resulted in increased cell proliferation under hypoxia. In this case, significant levels of HIF-1α could be observed in hypoxic MSCs cultured in 2.5% or 5% O2 (20). Hypoxia-inducible factor 1α (HIF-1α), as a pivotal transcription factor regulating stress and adaptive responses to oxygen concentration (21), usually interacts directly with numerous proteins to regulate its function (22–24). Most classically, differentiation, proliferation, angiogenesis, and migration are directly correlated with HIF-1α and β-catenin (25–27). However, how HIF-1α is expressed in PBMSCs and how it regulates the maintenance of stemness and cell proliferation remain unclear.
This study hypothesized that hypoxia could promote the proliferation and differentiation of rPBMSCs by activating the expressions of HIF-1α, β-catenin, proliferative-related genes, and stemness-related genes. Thus, this study examined the ability of rPBMSCs to proliferate and maintain MSC phenotypes under different concentrations of oxygen in vitro culture to explore the effects and mechanisms of hypoxia on the maintenance of rPBMSC proliferation and stemness.
Materials and Methods
Isolation and Culture of rPBMSCs
The animal ethics committee of Guangzhou Red Cross Hospital approved the research. Following previously published methods (28), a 3 cm × 3 cm wound was made on the back skin of the rats. The wound was disinfected every day, and the rats were provided with enough food and water to ensure their normal activities and survival. After 1 week, 0.8% pentobarbital was injected into the abdominal cavity of these animals for anesthesia. After anesthetization, approximately 5 mL of abdominal aortic blood was collected using a fine-needle approach, and the blood sample was diluted to 1:1 by PBS. Mononuclear cells (MNCs) were separated and collected with Ficoll separation solution (GBCBIO Technologies, Guangzhou, China) and centrifuged at 2000 rpm for 35 min. The middle layer was pipetted with a thin tube and washed twice with phosphate-buffered saline (PBS). MNCs (2×106/mL) were seeded onto the T-25 flask with 10 mL of complete Dulbecco’s Modified Eagle Medium (Gibco, MA). The complete medium contained 1% penicillin/streptomycin (Gibco), 20 ng/mL bFGF (R&D Systems, MN), and 20% fetal bovine serum (Gibco). With 21 days of culture, the cell convergence was 80%, and the third-generation cells digested by 0.25% trypsin were used for subsequent experiments. Representative bright-field images were captured by an inverted phase-contrast microscope (Nikon ECLIPSE Ts2, Nikon).
Immunophenotype Analysis of rPBMSCs
The cell immune phenotypes of third-generation PBMSCs (P3 PBMSCs) were identified by flow cytometry (FCM). CD73, CD105, and CD90 (R&D Systems, US) were selected as positive markers of rPBMSCs, whereas CD14, CD19, CD34, CD45, and HLA-DR (BD biosciences, US) were chosen as negative markers of rPBMSCs. rPBMSCs (2×105 cells/mL) were resuspended in PBS and mixed in CD14, CD19, CD34, CD45, CD73, CD90, CD105, and HLA-DR antibody solutions for 30 min, and the cell samples were then loaded on the machine for analysis.
Cell Cycle Distribution Assay
rPBMSCs treated under normoxic and hypoxic (5% O2) conditions for 24 h were collected, and rPBMSCs were then fixed with 70% (V/V) ethanol overnight. Moreover, 50 μg/mL propidium iodide (PI) (Beyotime Biotechnology, Shanghai, China) was diluted by PBS solution containing 1% Triton X-100. Cells were fully infiltrated in the freshly prepared PI solution for 30 min and were analyzed by a BD FACScan flow cytometer (BD Company, CA).
Multilineage Differentiation Potential Assay
P3 rPBMSCs were seeded into a 24-well plate at a density of 2 × 104/well and cultured at 37°C in an incubator with 5% CO2. When the cells grow to 70% confluence, chondrogenesis induction, osteoinduction, and adipogenesis tests were performed. For chondrogenesis, cells were induced for 21 days in a chondrogenesis induction medium kit (RAXMX-90041, Cyagen Biosciences, US). The differentiation was evaluated by alcian blue staining. For osteogenesis, the cultures were induced with an osteogenesis induction medium kit (RAXMX-90021, Cyagen Biosciences, CA). After culture for 21 days, alizarin red staining was performed to evaluate the osteogenic products. For adipogenesis, cells were induced for 21 days in an adipogenesis induction medium kit (RAXMX-90031, Cyagen Biosciences). The formation of lipid vacuoles was assessed by Oil Red O staining. All images were captured under an inverted phase-contrast microscope (Nikon ECLIPSE Ts2, Nikon).
Multilineage Differentiation Potential of rPBMSCs Cultured Under Normoxic (21% O2 and 5% CO2) or Hypoxic (5% O2 and 5% CO2) Conditions
P3 rPBMSCs were seeded into a 24-well plate at a density of 2 × 104/well and cultured at 37°C in a 21% O2 and 5% CO2 incubator or a 5% O2 and 5% CO2 incubator. The induction medium and experimental procedures performed in the subsequent experiments were the same as the methods described in “Multilineage Differentiation Potential Assay.”
Determination of the Growth Curve of rPBMSCs and the MTS Assay
P3, P5, and P6 rPBMSCs (2× 103/well) were inoculated in microplates (24-well) in 5% CO2 incubators with a gradient concentration of oxygen at 37°C. The experiments were set up as the control group (21% O2 and 5% CO2), 19% O2 and 5% CO2 hypoxia group, 5% O2 and 5% CO2 hypoxia group, and 1% O2 and 5% CO2 hypoxia group, with three replicate wells in each group. Starting from the next day, each group of cells was digested and counted accurately with a cell counter at each time point (Days 1–8). The growth curves of each cell group were made according to the number of cells. For the MTS assay, the above-mentioned groups of cells were planted on the well plate after Day 8, and the absorbance was measured at 450 nm by a multifunctional microplate reader (BioTek, US).
Assessment of Population Doubling Levels
After the cells reached 80-90% confluency, cells were passaged and counted. Calculate the cumulative population doubling (CPD) value using the following formula (29):
CPD was plotted against time in culture and performed in triplicate for each counting procedure.
Assay for Colony Formation
Moreover, 500 rPBMSCs were cultured in 6-well plates in an incubator capable of adjusting oxygen concentration for 14 days. After fixation with paraformaldehyde for 15 min, 1 mL of crystal violet staining solution was added to the culture plate for staining clones for 30 min. Under an inverted phase-contrast microscope (Nikon ECLIPSE Ts2, Nikon), the number of clones containing more than 50 cells was counted.
Western Blot
rPBMSCs were collected after normoxic and hypoxic (5% O2) treatments for 5 days, and whole-cell lysates were prepared for Western blotting in radioimmunoprecipitation assay buffer. Then, 30 μg of protein was loaded into the sample well, dispersed in the gel according to the molecular weight, and directly transferred to the poly(vinylidene fluoride) membrane (Bio-Rad, CA) in a band-to-band manner through the semi-dry transfer method. The membranes were immersed in a square dish filled with primary antibody diluent. These antibodies (HIF-1α, 36169; β-catenin, 8400; SOX2, 3579; CyclinE, 4132; Nanog, 8822; GAPDH [glyceraldehyde 3-phosphate dehydrogenase], 5174) were purchased from Cell Signaling Technology (MA), and when used, the dilution ratio was 1:1000. On the next day, membranes were incubated with secondary anti-rabbit/mouse IgG, HRP-linked antibody (#7074/7076, 1:3000, Cell Signaling Technology). The electrochemiluminescence detection mixture was used to detect the protein on the membranes. ChemiDoc XRS imaging system with Image Lab software (Bio-Rad) was used to analyze the graphs.
Immunofluorescence Microscopy
Furthermore, 104/well rPBMSCs were seeded in glass slides placed in plates treated under normoxia and hypoxia (5% O2) for 5 days. After sequential fixation, blocking, incubation of primary (β-catenin,1:200, 8242, Cell Signaling Technology; HIF-1α, 1:200, #36169, Cell Signaling Technology) and secondary (1:200, ZF0311, OriGene Technologies, MD) antibodies, a fluorescence microscope (Ti2-U, Nikon) was used to observe and capture pictures of interest.
Real-Time Polymerase Chain Reaction (PCR)
The culture method of rPBMSCs was the same as with Western blot. Total RNA obtained by the TRIzol method was reversed into cDNA in the PrimeScript RT Master mix reaction system (Takara Bio, Japan). With reference to the instructions, SYBR-Green reagent (Takara Bio) was used to perform real-time PCR in triplicate in a fluorescence quantitative PCR instrument (Jena, Germany). GAPDH was used as a control to analyze relative gene expression in the 2-ΔΔCt formula (30). Primer sequences are presented in Table 1.
Statistical Analysis
Data in three replicates are presented as mean ± standard deviation. Student’s t-test or one-way analysis of variance was used to analyze differences between the two groups and among multiple groups; P < 0.05 was used to mark significant differences.
Results
In this study, rPBMSCs were successfully isolated and cultured. Stromal cell characteristics were proved by FCM and multi-differentiation potential induction experiments. The cell growth curves of P3, P5, and P6 rPBMSCs cultured under different oxygen concentrations were drawn based on the number counted at each time point. Then, the 5% hypoxia condition that significantly promoted cell growth was used for subsequent experiments. Hypoxia (5%) significantly increased the number of stromal cell clones and the proportion of S phase cells. Real-time RCR and Western blot results revealed that hypoxia (5%) significantly promoted the expressions of HIF-1α, β-catenin, and proliferation-related and stemness-related genes.
Characterization and Identification of rPBMSCs
On the day after inoculation, round or polygonal adherent cells were observed in the primary culture. After 7 days, colonies gradually formed. After approximately 16 days, the cell coverage area was 70%–80% of the bottom of the culture flask. At approximately 21 days later, the cell growth reached 100% (Figure 1A). Flow cytometry experiments revealed that rPBMSCs had high expression of CD73, CD90, and CD105, extremely low expressions of CD14, CD19, CD34, CD45, and HLA-DR (Figure 1B). Oil Red O staining indicated that rPBMSCs can differentiate into adipocytes embellished by red-stained lipid droplets. Alician blue staining demonstrated that after 21 days of induction, rPBMSCs could differentiate into chondrocytes embellished by blue-stained proteoglycans. Alizarin red staining presented that rPBMSCs could differentiate into osteoblasts embellished by red-stained bone nodules under osteogenic conditions (Figure 1C).
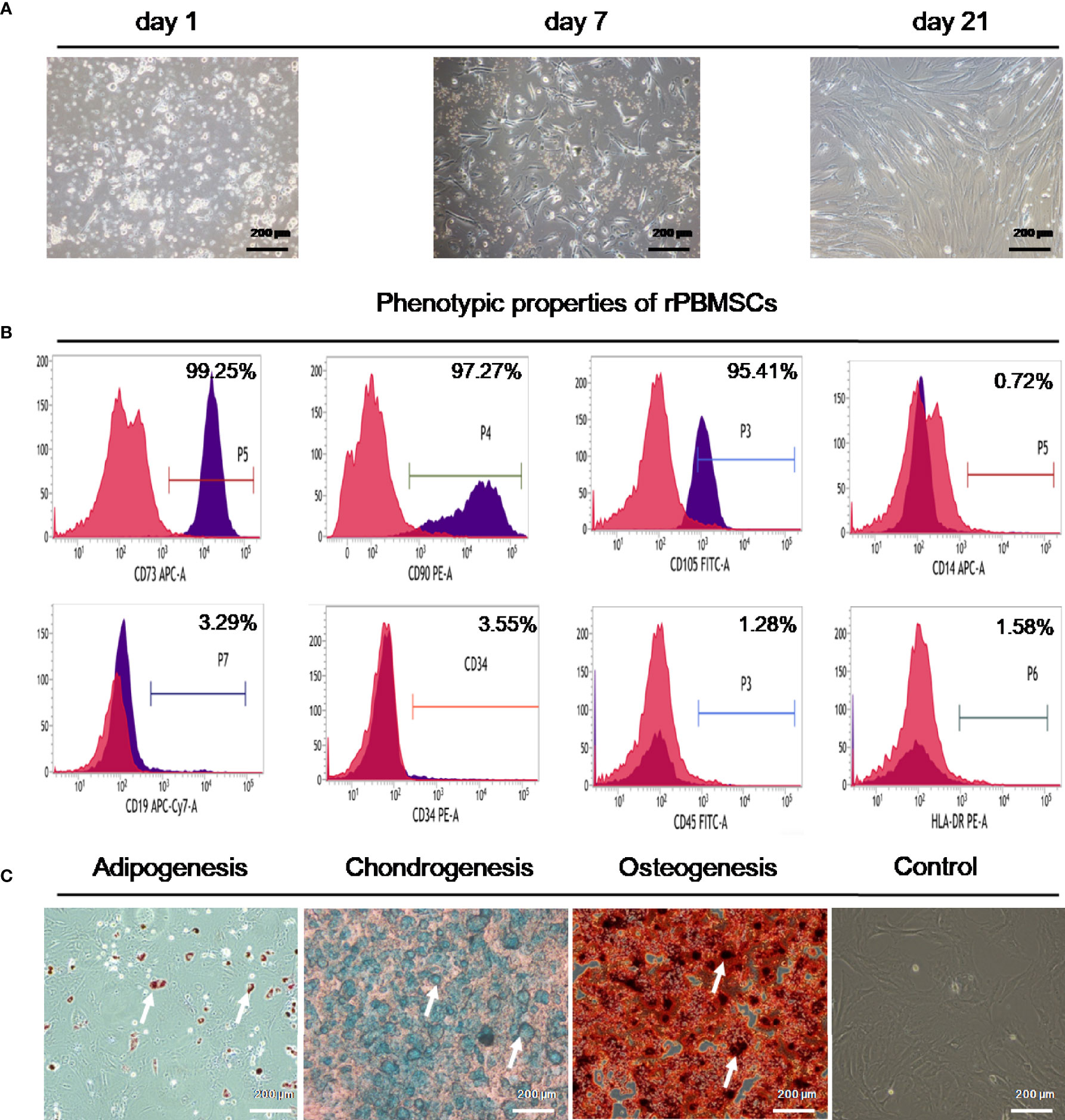
Figure 1 Characteristics of rPBMSCs. (A) Morphology of rPBMSCs cultured for 1 day, 7 days, and 21 days. (B) Immunocytochemical staining demonstrated a positive expression of CD73, CD90, and CD105 and negative expressions of CD14, CD19, CD34, CD45 CD45, and HLA-DR. (C) Multilineage differentiation capacities of rPBMSCs. Magnification, 200×. rPBMSCs, rat peripheral blood-derived mesenchymal stromal cells.
Hypoxia Promoted rPBMSC Growth and Proliferation
The results of the experiments are displayed in Figures 2A, B. In the first 2 days of culture, different concentrations of O2 had no noticeable effects on the proliferation of third-generation PBMSCs (P3 rPBMSCs), fifth-generation PBMSCs (P5 rPBMSCs), and sixth-generation PBMSCs (P6 rPBMSCs). After 3 days, hypoxia (5% O2) significantly increased the number of cells and proliferation rate of P3, P5, and P6 rPBMSCs. After 8 days, the cells approached the plateau stage. At this time, the number of P3 rPBMSCs in the control group, 19% O2 hypoxia, 5% O2 hypoxia, and 1% O2 hypoxia groups were 55 × 103, 70 × 103, 96 × 103, and 71 × 103, respectively. Statistical analysis showed that compared with the number of P3 rPBMSCs in the control group, those in the 19% O2 hypoxia, 5% O2 hypoxia, and 1% O2 hypoxia groups were increased significantly (P < 0.05). Compared with 19% O2 hypoxia and 5% O2 hypoxia, 1% O2 hypoxia further increased the number of P3 rPBMSCs (P < 0.05). Similar to the growth curve, 5% O2 hypoxia significantly promoted the absorbance of P3 rPBMSCs seeded at Day 8. The CPD curve of P6 PBMSCs proved that the CPD value of PBMSCs in the 5% hypoxia group was significantly higher than that in the normoxia group. Compared with the normoxia group, the CPD values for P6 PBMSCs in the 19% O2 hypoxia and 1% O2 hypoxia groups did not change significantly on Day 7. The shape of the growth curve and viability of P5 and P6 rPBMSCs were similar with those of P3 rPBMSCs, but the amounts of rPBMSCs and optical density values of P5 and P6 on Day 8 were lower than those of P3 rPBMSCs. Based on the cell growth curve and MTT assay results, 5% O2 hypoxia was selected for subsequent experiments. As presented in Figures 2C, D, 5% O2 hypoxia significantly promoted the formation of rPBMSC colonies. The number of rPBMSC colonies in the 5% O2 hypoxia group was increased by 53% compared with that in the control group (P < 0.05).
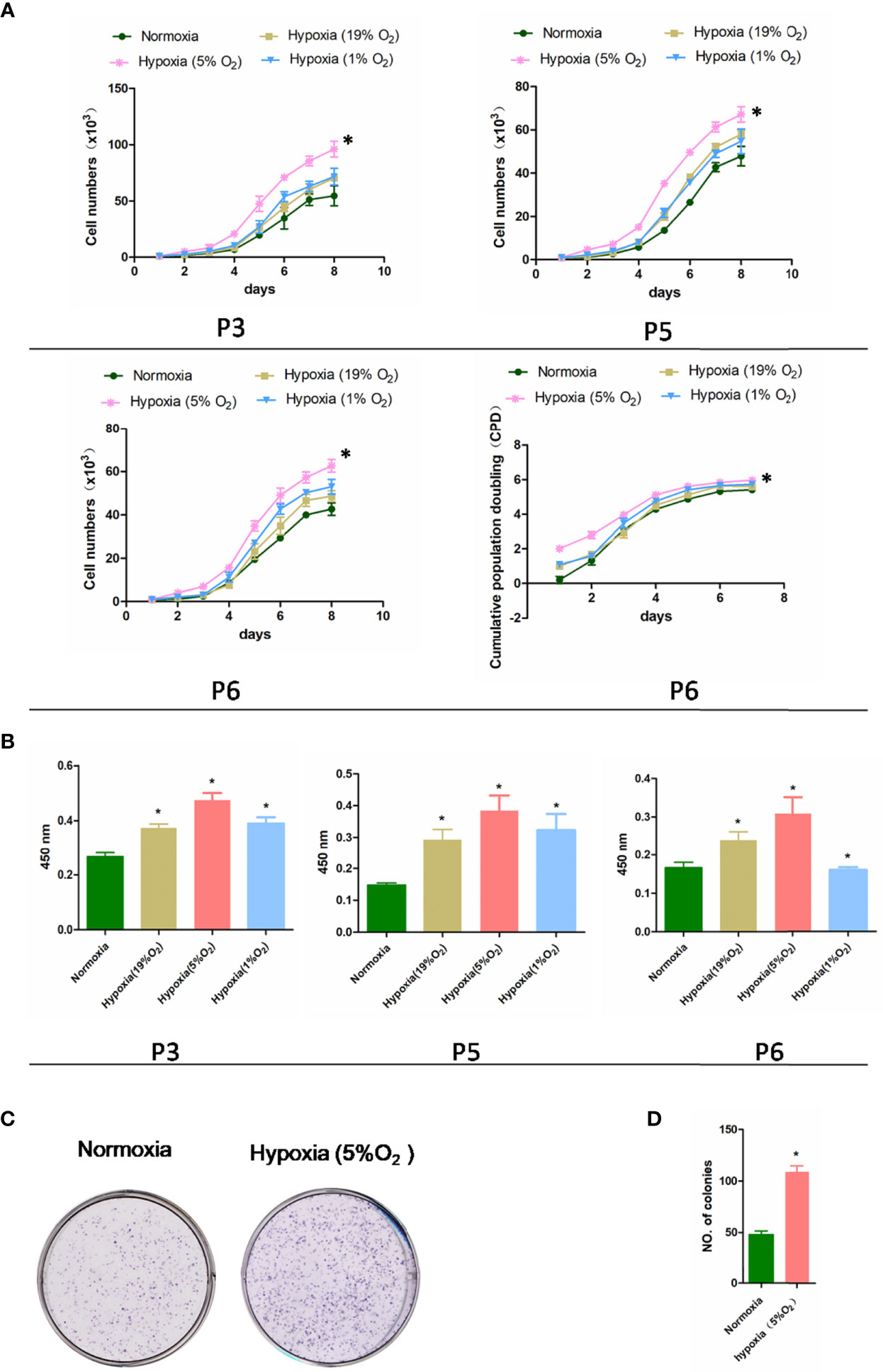
Figure 2 Effect of hypoxia on rPBMSC proliferation. (A) The cell growth curves of third-generation PBMSCs (P3 PBMSCs), fifth-generation PBMSCs (P5 PBMSCs), and sixth-generation PBMSCs (P6 PBMSCs) were drawn based on the number of cells counted at each time point, the cumulative population doubling curve of sixth-generation PBMSCs (P6 PBMSCs) was determined based on cell culture time. (B) Absorbance of P3, P5, and P6 rPBMSCs treated under normoxia and hypoxia (5% O2) at Day 8. (C) Hypoxia increased the number of rPBMSC colonies. (D) Measurement of the number of colonies in each group. All data are presented as means ± SEM. P < 0. 05; ∗ vs control group. rPBMSCs, rat peripheral blood-derived mesenchymal stromal cells.
Hypoxia Promoted Cell Cycle Transition and Maintained the Trilineage Differentiation Capacity of rPBMSCs
FCM was used to investigate the cell cycle transition of rPBMSCs treated under normoxia and hypoxia (5% O2). Hypoxia exerted a significant increase and decrease in the number of S phase and G1 phase cells, respectively (Figures 3A, B). Moreover, 5% O2 hypoxia increased the percentage of rPBMSCs in the S phase from 27.26% to 46.32% (P < 0.05) and reduced the percentage of rPBMSCs in the G1 phase from 58.24% to 47.19% (P < 0.05). These data indicated that 5% O2 hypoxia increased the DNA synthesis and cell cycle of rPBMSC progression at the S phase. After 21 days of culture with a differentiation agent under hypoxia or normoxia, the effect of hypoxia on the pluripotency of rPBMSCs was investigated. Figure 3C illustrates that hypoxia increased the ability of induced cells to differentiate into three lines, including osteoblasts, chondrocytes, and adipocytes.
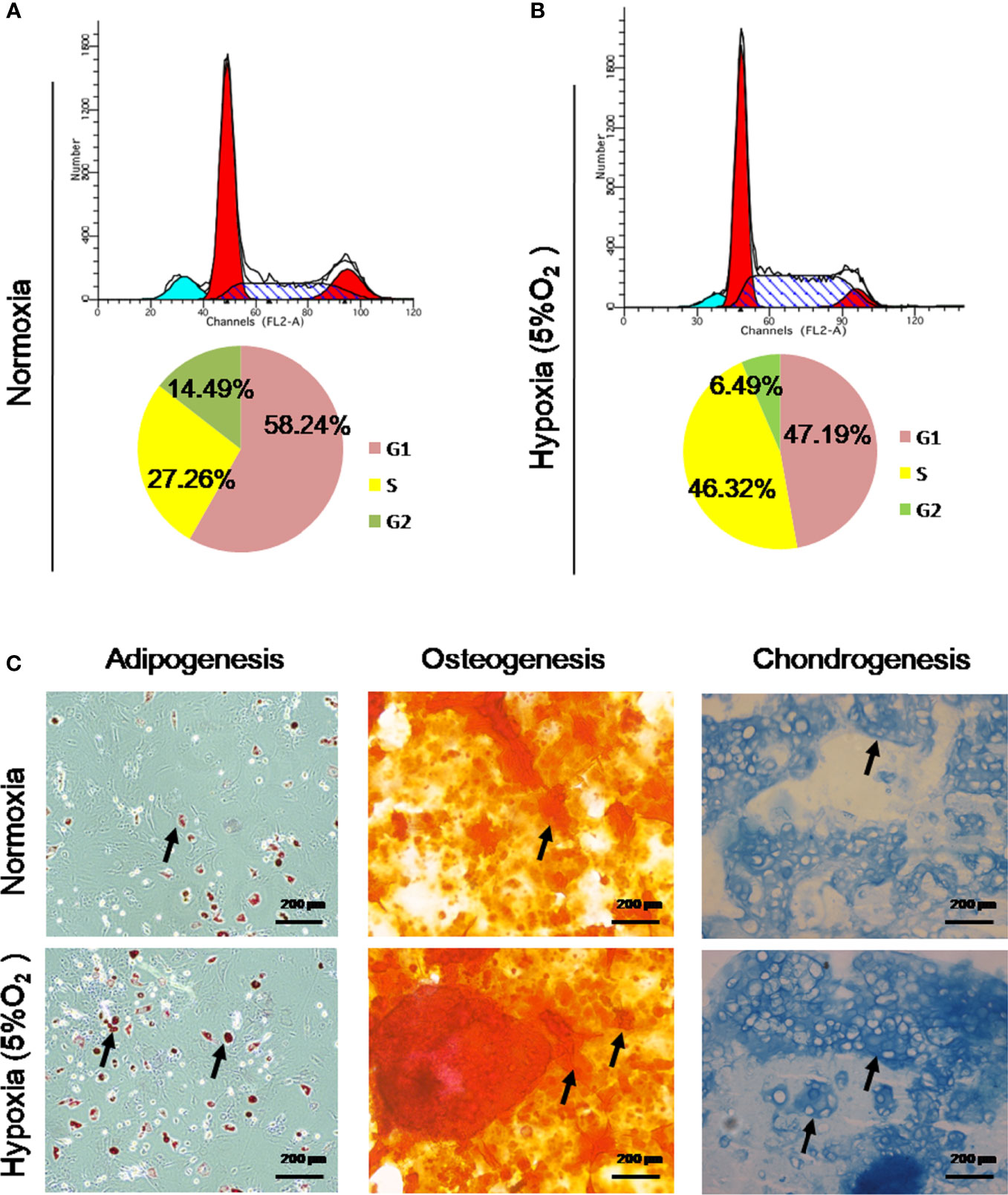
Figure 3 Hypoxia promoted cell cycle transition and maintained the trilineage differentiation capacity of rPBMSCs. (A, B) Hypoxia promoted cell cycle transition, as determined by flow cytometry. (C) rPBMSCs were cultured under normoxia and hypoxia (5% O2) for chondrogenic differentiation, osteogenic differentiation, and adipogenic differentiation for 21 days. Magnification, 200×. Arrows indicate lipid droplets, proteoglycans, and calcium nodules. rPBMSCs, rat peripheral blood-derived mesenchymal stromal cells.
Hypoxia Activated the Expression of β-Catenin and HIF-1α in rPBMSCs
As displayed in Figure 4A, 5% O2 hypoxia significantly increased the HIF-1α (red) nuclei expression in rPBMSCs, compared with the control rPBMSCs. Simultaneously, the nuclei expression for β-catenin (green) in rPBMSCs was also upregulated significantly with 5% O2 hypoxia. Immunohistochemistry results (Figure 4B) that 5% O2 hypoxia stimulated the upregulation of HIF-1α and β-catenin expressions are consistent with the promotion of HIF-1α and β-catenin stabilization and nuclear translocation in immunofluorescence experiments (Figures 4C, D).
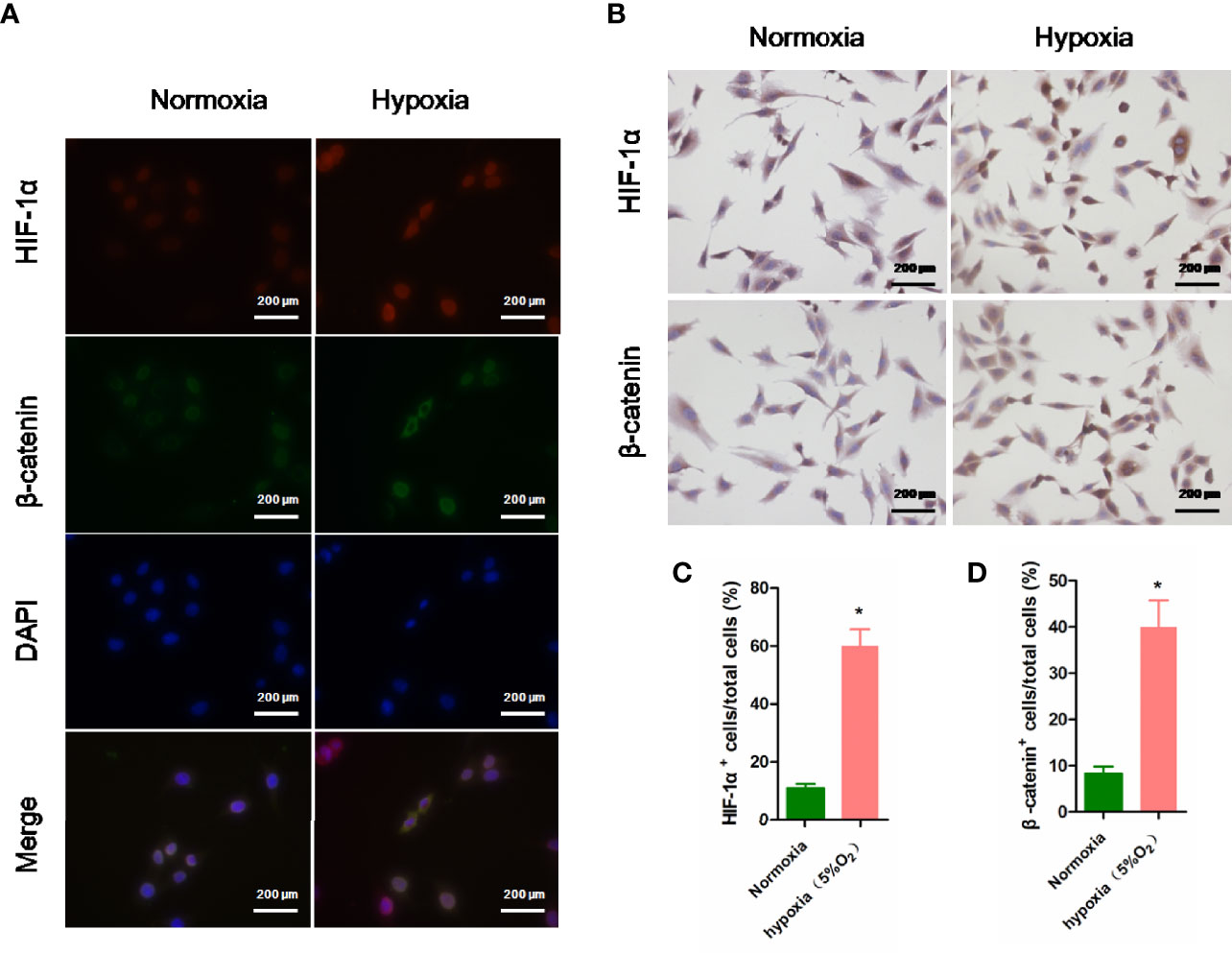
Figure 4 Immunoassay of HIF-1α and β-catenin in rPBMSCs under normoxia and hypoxia (5% O2). (A) Nuclear expression of HIF-1α (red) and β-catenin (green) in rPBMSCs treated under normoxia and hypoxia (5% O2) for 5 days. (B) Nuclear expression of HIF-1α (brown) and β-catenin (brown) in rPBMSCs treated under normoxia and hypoxia (5% O2) for 5 days. (C, D) Quantitative analysis of HIF-1α (brown) and β-catenin (brown) in rPBMSCs in panel (B). Magnification, 200×. All data are presented as means ± SEM. P < 0. 05; ∗ vs control group. rPBMSCs, rat peripheral blood-derived mesenchymal stromal cells; HIF-1α, hypoxia-inducible factor 1α.
Hypoxia Intensified the Expression of Cycle-Associated Genes and Stemness Genes in rPBMSCs
For the objective investigation that hypoxia regulated the self-renewal and stemness of rPBMSCs, pluripotency factors and Cyclin E/CDK2 were primarily selected as indicators. Compared with normoxia, hypoxia significantly triggered upregulation of mRNA and protein expression for β-catenin, CDK2, and Cyclin E in rPBMSCs (Figures 5A–C). Moreover, the mRNA and protein expressions of HIF-1α, Nanog, and SOX2 were significantly increased by hypoxia (Figures 5D–F).
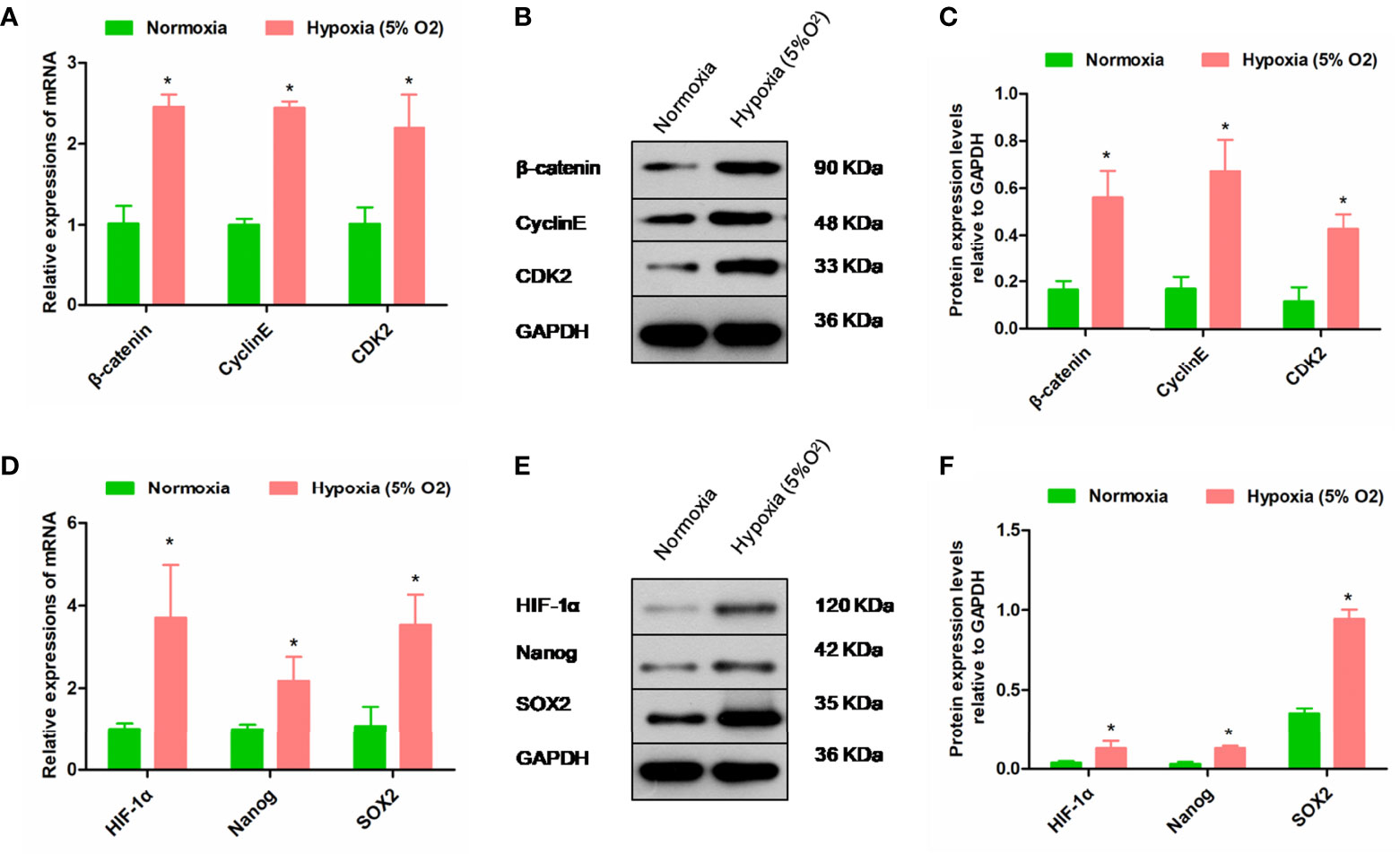
Figure 5 Hypoxia regulates the expressions of cycle-related and self-renewal-related molecules. (A–C) mRNA and protein expression levels of β-catenin, Cyclin E, and CDK2 in rPBMSCs treated under normoxic and hypoxic (5% O2) conditions for 5 days. (D–F) mRNA and protein expression levels of HIF-1α, Nanog, and SOX2 in rPBMSCs treated under normoxic and hypoxic (5% O2) conditions for 24 h. All data are presented as means ± SEM. P < 0. 05; ∗ vs control group. rPBMSCs, rat peripheral blood-derived mesenchymal stromal cells; HIF-1α, hypoxia-inducible factor 1α.
Discussion
As a typical representative of adult pluripotent stromal cells, PBMSCs provide various possibilities for clinical application and transformation in the field of regenerative medicine (31). PBMSCs are abundant in the body and have strong proliferation and self-renewal ability, as well as the potential to differentiate into multiple cell types (32). However, some problems and obstacles are still encountered in the application and transformation of MSCs in cartilage tissue engineering, including the reduced activity and phenotype of seed cells in vitro (33). Thus, this study aimed to determine a hypoxic culture method that allows PBMSCs to maintain their ability to proliferate and self-renew during expansion in vitro. For this reason, this study mainly focused on three aspects, namely, (1) successful isolation, culture, and identification of rPBMSCs; (2) proliferation, phenotype maintenance, and differentiation potential of hypoxia-treated rPBMSCs; and (3) proliferation- and phenotype-related gene expressions of hypoxia-treated PBMSCs. This study proposes a culture method that is conducive to maintaining self-renewal and proliferation capabilities to ensure cell yield and long-term expansion.
In this study, rPBMSCs were successfully isolated and cultured, and third-generation rPBMSCs were selected for FCM for phenotypic identification. rPBMSCs did not express CD45, CD34, CD14, CD19, and HLA II, but highly expressed CD73, CD90, and CD105, indicating that the rPBMSCs had stromal cell performance, without other surface antigen markers (34, 35). Successfully isolated rPBMSCs positively expressed CD90, CD73, and CD105. Cell growth curve determination and cell cloning experiments revealed that 5% O2 hypoxia can significantly promote the formation of clones and the rapid proliferation of PBMSCs. Many recent studies have reported that an appropriate hypoxic condition can significantly stimulate the proliferation of MSCs and PBMSCs, which is consistent with the results of the present study. The recent studies confirmed hypoxia accelerated proliferation of PBMSCs, increased migration of PBMSCs, and reduced PBMSC differentiation into osteoblasts by increasing Notch1 expression (36). In our study, four oxygen concentration gradients were set up to more rigorously explore the effects of various oxygen concentrations on the proliferation and stemness of PBMSCs, as well as the role of HIF-1α pathway in the proliferation and stemness maintenance of PBMSCs.
Other studies have also reported that hypoxia promotes the proliferation of cord blood derived MSCs without changing the cellular immune phenotype (37). In this study, hypoxia significantly promoted the transition of PBMSCs from the G1 phase to the S phase. Since the S phase is an important stage of cell DNA synthesis (38, 39), under hypoxic conditions, PBMSCs pass the G1/S phase checkpoint and enter the DNA synthesis phase. Moreover, a study revealed that hypoxia can drive cells into the cell cycle and promote the expression of cyclins and related kinases to drive umbilical cord derived MSCs through cell cycle checkpoints, thereby promoting DNA synthesis (40).
HIF-1α is an extremely critical transcription factor, which is strongly induced during hypoxia and adapts to hypoxic tension (41). Studies have reported that HIF-1α causes cell cycle arrest in the G0/G1 phase through p27 expression (42); however, under different cell environments, HIF-1α activation can demonstrate varying results by affecting various aspects of cell biology (39). The present study presents that upregulating the expression of HIF-1α under 5% hypoxia can increase the proportion of rPBMSCs in the S phase. β-catenin is a typical cytoplasmic protein, as part of the classic Wnt signaling, which plays a role in cell adhesion (43). β-catenin coactivated LRH-1 on the cyclin E1 promoter and induced G1 cyclin-mediated cell proliferation, Cyclin E interacts with CDK2 to control the G1/S phase transition (44). In this experiment, after hypoxic treatment of rPBMSCs, the transition of cells from the G1 phase to the S phase and the activation of Cyclin E/CDK2 indicated that hypoxia may regulate the cell cycle position to control the self-renewal of rPBMSCs. Similar reports have suggested that HIF-1α promotes cell vitality and proliferation of MSCs (45, 46).
The expression of stemness markers was observed under hypoxic conditions. The significant role of low oxygen in altering the characteristics of various types of stromal cells was previously investigated (47). A study suggested the upregulation of stemness genes such as OCT4 and Nanog of BMSCs cultured in 1% oxygen (48). Similar results were observed in the present study, where the expressions of Nanog and SOX2 of rPBMSCs under 5% oxygen conditions were promoted, indicating that the stemness of rPBMSCs was enhanced by a hypoxic environment. The inhibition of senescence of MSCs suggests the increased expression of pluripotency markers (49). In this study, hypoxia-treated rPBMSCs demonstrated a higher differentiation potential, including cartilage, osteogenic, and adipogenic potentials compared with normoxia-treated cells. However, the control of hypoxia on stromal cells involves transcription factors such as HIF-1α and β-catenin. However, further research is needed to understand how HIF-1α and β-catenin regulate and interact with each other.
Conclusion
In summary, the stemness, proliferation, and self-renewal potential of hypoxia-treated rPBMSCs were enhanced. Therefore, conditional hypoxia (5%) culture can be used as a convenient strategy to maintain the function of rPBMSCs. The general process and conclusions of this study are clearly illustrated in Figure 6.
Data Availability Statement
The original contributions presented in the study are included in the article/supplementary material. Further inquiries can be directed to the corresponding author.
Ethics Statement
This animal study was reviewed and approved by animal ethics committee of Guangzhou Red Cross Hospital.
Author Contributions
Study design: PW; Data collection: PZ; Data analysis: CY and JW; Interpretation of data: PW; Draft manuscript: PW; Review manuscript: PW. All authors contributed to the article and approved the submitted version.
Funding
This work was supported by the Medical Science and Technology Research Foundation of Guangdong (A2021335, PW), Traditional Chinese Medicine Bureau of Guangdong Province (20222166, PW), and Guangdong Provincial Basic and Applied Basic Regional Joint Fund (2020A1515110009, PZ).
Conflict of Interest
The authors declare that the research was conducted in the absence of any commercial or financial relationships that could be construed as a potential conflict of interest.
Publisher’s Note
All claims expressed in this article are solely those of the authors and do not necessarily represent those of their affiliated organizations, or those of the publisher, the editors and the reviewers. Any product that may be evaluated in this article, or claim that may be made by its manufacturer, is not guaranteed or endorsed by the publisher.
References
1. Kanno K, Sakaue T, Hamaguchi M, Namiguchi K, Nanba D, Aono J. Hypoxic Culture Maintains Cell Growth of the Primary Human Valve Interstitial Cells With Stemness. Int J Mol Sci (2021) 29:10534. doi: 10.3390/ijms221910534
2. Abu Awwad HAM, Thiagarajan L, Kanczler JM, Amer MH, Bruce G, Lanham S, et al. Genetically-Programmed, Mesenchymal Stromal Cell-Laden & Mechanically Strong 3D Bioprinted Scaffolds for Bone Repair. J Contr Relea (2020) 325:335–46. doi: 10.1016/j.jconrel.2020.06.035
3. Lecourt S, Vanneaux V, Cras A, Freida D, Heraoui D, Herbi L, et al. Bone Marrow Microenvironment in an In Vitro Model of Gaucher Disease: Consequences of Glucocerebrosidase Deficiency. Stem Cells Dev (2012) 21:239–48. doi: 10.1089/scd.2011.0365
4. Granchi D, Ochoa G, Leonardi E, Devescovi V, Bagl SR, Osaba L, et al. Gene Expression Patterns Related to Osteogenic Differentiation of Bone Marrow-Derived Mesenchymal Stem Cells During Ex Vivo Expansion. Tissue Eng Part C Methods (2010) 16:511–24. doi: 10.1089/ten.tec.2009.0405
5. Takeuchi R, Katagiri W, Endo S, Kobayashi T. Exosomes From Conditioned Media of Bone Marrow-Derived Mesenchymal Stem Cells Promote Bone Regeneration by Enhancing Angiogenesis. PloS One (2019) 21:14:e0225472. doi: 10.1371/journal.pone.0225472
6. Zhang B, Yeo RWY, Lai RC, Sim EWK, Chin KC, Lim SK. Mesenchymal Stromal Cell Exosome-Enhanced Regulatory T-Cell Production Through an Antigen-Presenting Cell-Mediated Pathway. Cytotherapy (2018) 20(5):687–96. doi: 10.1016/j.jcyt.2018.02.372
7. Maria S, Samsonraj RM, Munmun F, Glas J, Silvestros M, Kotlarczyk MP, et al. Biological Effects of Melatonin on Osteoblast/Osteoclast Cocultures, Bone, and Quality of Life: Implications of a Role for MT2 Melatonin Receptors, MEK1/2, and MEK5 in Melatonin-Mediated Osteoblastogenesis. J Pineal Res (2018) 64(3):10.1111/jpi.12465. doi: 10.1111/jpi.12465
8. Fu WL, Xiang Z, Huang FG, Gu ZP, Yu XX, Cen SQ, et al. Coculture of Peripheral Blood-Derived Mesenchymal Stem Cells and Endothelial Progenitor Cells on Strontium-Doped Calcium Polyphosphate Scaffolds to Generate Vascularized Engineered Bone. Tissue Eng Part A (2015) 21:948–59. doi: 10.1089/ten.tea.2014.0267
9. Dominici M, Le Blanc K, Mueller I, Slaper-Cortenbach I, Marini F, Krause D, et al. Minimal Criteria for Defining Multipotent Mesenchymal Stromal Cells. The International Society for Cellular Therapy Position Statement. Cytotherapy (2006) 8(4):315–7. doi: 10.1080/14653240600855905
10. Toh WS, Lai RC, Hui JHP, Lim SK. MSC Exosome as a Cell-Free MSC Therapy for Cartilage Regeneration: Implications for Osteoarthritis Treatment. Semin Cell Dev Biol (2017) 67:56–64. doi: 10.1016/j.semcdb.2016.11.008
11. Cianflone E, Torella M, Biamonte F, De Angelis A, Urbanek K, Costanzo FS. Targeting Cardiac Stem Cell Senescence to Treat Cardiac Aging and Disease. Cells (2020) 9:1558. doi: 10.3390/cells9061558
12. Zhen G, Wen C, Jia X, Li Y, Crane JL, Mears SC, et al. Inhibition of TGF-β Signaling in Mesenchymal Stem Cells of Subchondral Bone Attenuates Osteoarthritis. Nat Med (2013) 19:704–12. doi: 10.1038/nm.3143
13. Ludwig TE, Levenstein ME, Jones JM, Berggren WT, Mitchen ER, Frane JL, et al. Derivation of Human Embryonic Stem Cells in Defined Conditions. Nat Biotechnol (2006) 24:185–7. doi: 10.1038/nbt1177
14. Foyt DA, Taheem DK, Ferreira SA, Norman MDA, Petzold J, Jell G, et al. Hypoxia Impacts Human MSC Response to Substrate Stiffness During Chondrogenic Differentiation. Acta Biomat (2019) 89:73–83. doi: 10.1016/j.actbio.2019.03.002
15. Theus MH, Wei L, Cui L, K Francis XH, Keogh C. In Vitro Hypoxic Preconditioning of Embryonic Stem Cells as a Strategy of Promoting Cell Survival and Functional Benefits After Transplantation Into the Ischemic Rat Brain. Exp Neurol (2008) 210:656–70. doi: 10.1016/j.expneurol.2007.12.020
16. Tsai CC, Yew TL, Yang DC, Huang WH, Hung SC. Benefits of Hypoxic Culture on Bone Marrow Multipotent Stromal Cells. Am J Blood Res (2012) 2:148–59.
17. Parmar K, Mauch P, Vergilio JA, Sackstein R, Down JD. Distribution of Hematopoietic Stem Cells in the Bone Marrow According to Regional Hypoxia. Proc Natl Acad Sci USA (2007) 104(13):5431–6. doi: 10.1073/pnas.0701152104
18. Majmundar AJ, Wong WJ, Simon MC. Hypoxiainducible Factors and the Response to Hypoxic Stress. Mol Cell (2010) 40(2):294–309. doi: 10.1016/j.molcel.2010.09.022
19. Semenza GL. Molecular Mechanisms Mediating Metastasis of Hypoxic Breast Cancer Cells. Trends Mol Med (2012) 18(9):534–43. doi: 10.1016/j.molmed.2012.08.001
20. Lavrentieva A, Majore I, Kasper C, Hass R. Effects of Hypoxic Culture Conditions on Umbilical Cordderived Human Mesenchymal Stem Cells. Cell Commun Signal (2010) 8(1):18. doi: 10.1186/1478-811X-8-18
21. Semenza GL. HIF-1 and Human Disease: One Highly Involved Factor. Genes Dev (2000) 14:1983–91. doi: 10.1101/gad.14.16.1983
22. Li H, Jia Y, Wang Y. Targeting HIF-1α Signaling Pathway for Gastric Cancer Treatment. Pharmazie (2019) 74:3–7. doi: 10.1691/ph.2019.8674
23. Conde E, Giménez-Moyano S, Martín-Gómez L, Rodríguez M, Ramos ME, Aguado-Fraile E, et al. HIF-1α Induction During Reperfusion Avoids Maladaptive Repair After Renal Ischemia/Reperfusion Involving Mir127-3p. Sci Rep (2017) 7:41099. doi: 10.1038/srep41099
24. Warbrick I, Rabkin SW. Hypoxia-Inducible Factor 1-Alpha (HIF-1α) as a Factor Mediating the Relationship Between Obesity and Heart Failure With Preserved Ejection Fraction. Obes Rev (2019) 20:701–12. doi: 10.1111/obr.12828
25. Sui H, Zhao J, Zhou L, Wen H, Deng W, Li C. Tanshinone IIA Inhibits β-Catenin/VEGF-Mediated Angiogenesis by Targeting TGF-β1 in Normoxic and HIF-1α in Hypoxic Microenvironments in Human Colorectal Cancer. Cancer Lett (2017) 403:86–97. doi: 10.1016/j.canlet.2017.05.013
26. Fang Y, Yu S, Ma Y, Sun P, Ma D, Ji C, et al. Association of Dll4/notch and HIF-1a -VEGF Signaling in the Angiogenesis of Missed Abortion. PloS One (2013) 8:e70667. doi: 10.1371/journal.pone.0070667
27. Mohyeldin A, Garzón-Muvdi T, Quiñones-Hinojosa A. Oxygen in Stem Cell Biology: A Critical Component of the Stem Cell Niche. Cell Stem Cell (2010) 6:7:150–61. doi: 10.1016/j.stem.2010.07.007
28. Fu Q, Liu Y, Liu X, Zhang Q, Chen L, Peng J, et al. Engrafted Peripheral Blood-Derived Mesenchymal Stem Cells Promote Locomotive Recovery in Adult Rats After Spinal Cord Injury. Am J Transl Res (2017) 9:3950–66.
29. Yusop N, Battersby P, Alraies A, Sloan AJ, Moseley R, Waddington RJ. Isolation and Characterisation of Mesenchymal Stem Cells From Rat Bone Marrow and the Endosteal Niche: A Comparative Study. Stem Cells Int (2018) 2018:6869128. doi: 10.1155/2018/6869128
30. Livak KJ, Schmittgen TD. Analysis of Relative Gene Expression Data Using Real-Time Quantitative PCR and the 2(-Delta Delta C(T)) Method. Methods (2001) 25:402–8. doi: 10.1006/meth.2001.1262
31. Calle A, Gutiérrez-Reinoso MÁ, Re M, Blanco J, de la Fuente J, Monguió-Tortajada M, et al. Bovine Peripheral Blood MSCs Chemotax Towards Inflammation and Embryo Implantation Stimuli. J Cell Physiol (2021) 236:1054–67. doi: 10.1002/jcp.29915
32. Yang R, Gao H, Chen L, Fang N, Chen H, Song G, et al. Effect of Peripheral Blood-Derived Mesenchymal Stem Cells on Macrophage Polarization and Th17/Treg Balance In Vitro. Regener Ther (2020) 14:275–83. doi: 10.1016/j.reth.2020.03.008
33. Otte A, Bucan V, Reimers K, Hass R. Mesenchymal Stem Cells Maintain Long-Term In Vitro Stemness During Explants Culture. Tissue Eng Part C Methods (2013) 19:937–48. doi: 10.1089/ten.tec.2013.0007
34. De Ravin SS, Reik A, Liu PQ, Li L, Wu X, Su L, et al. Targeted Gene Addition in Human CD34(+) Hematopoietic Cells for Correction of X-Linked Chronic Granulomatous Disease. Nat Biotechnol (2016) 34:424–9. doi: 10.1038/nbt.3513
35. Fu Q, Zhang Q, Jia LY, Fang N, Chen L, Yu LM, et al. Isolation and Characterization of Rat Mesenchymal Stem Cells Derived From Granulocyte Colony-Stimulating Factor-Mobilized Peripheral Blood. Cells Tissue Organ (2016) 201(6):412–22. doi: 10.1159/000445855
36. Yang M, Liu H, Wang Y, Wu G, Qiu S, Liu C, et al. Hypoxia Reduces the Osteogenic Differentiation of Peripheral Blood Mesenchymal Stem Cells by Upregulating Notch-1 Expression. Conne Tissue Res (2019) 60:583–96. doi: 10.1080/03008207.2019.1611792
37. Zhao D, Liu L, Chen Q, Wang F, Li Q, Zeng Q, et al. Hypoxia With Wharton’s Jelly Mesenchymal Stem Cell Coculture Maintains Stemness of Umbilical Cord Blood-Derived CD34+ Cells. Stem Cell Res Ther (2018) 9:158. doi: 10.1186/s13287-018-0902-5
38. Williams GH, Stoeber K. The Cell Cycle and Cancer. J Pathol (2012) 226:352–64. doi: 10.1002/path.3022
39. Ramasamy R, Tong CK, Yip WK, Vellasamy S, Tan BC, Seow HF. Basic Fibroblast Growthfactor Modulates Cell Cycle of Human Umbilical Cord-Derived Mesenchymal Stem Cells. Cell Prolif (2012) 45:132–9. doi: 10.1111/j.1365-2184.2012.00808.x
40. Carmeliet P, Dor Y, Herbert JM, Fukumura D, Brusselmans K, Dewerchin M, et al. Role of HIF-1alpha in Hypoxia-Mediated Apoptosis, Cell Proliferation and Tumour Angiogenesis. Nature (1998) 394:485–90. doi: 10.1038/28867
41. Kumar S, Vaidya M. Hypoxia Inhibits Mesenchymal Stem Cell Proliferation Through HIF1alpha-Dependent Regulation of P27. Mol Cell Biochem (2016) 415:29–38. doi: 10.1007/s11010-016-2674-5
42. Palomaki S, Pietila M, Laitinen S, Pesala J, Sormunen R, Lehenkari P, et al. HIF-1alpha Is Upregulated in Human Mes-Enchymal Stem Cells. Stem Cells (2013) 31:1902–9. doi: 10.1002/stem.1435
43. Botrugno OA, Fayard E, Annicotte JS, Haby C, Brennan T, Wendling O, et al. Synergy Between LRH-1 and Beta-Catenin Induces G1 Cyclin-Mediated Cell Proliferation. Mol Cell (2004) 15:499–509. doi: 10.1016/j.molcel.2004.07.009
44. Odajima J, Wills ZP, Ndassa YM, Terunuma M, Kretschmannova K, Deeb TZ, et al. Cyclin E Constrains Cdk5 Activity to Regulate Synaptic Plasticity and Memory Formation. Dev Cell (2011) 21:655–68. doi: 10.1016/j.devcel.2011.08.009
45. Yu J, Liu XL, Cheng QG, Lu SS, Xu XQ, Zu QQ, et al. G-CSF and Hypoxic Conditioning Improve the Proliferation, Neural Differentiation and Migration of Canine Bone Marrow Mesenchymal Stem Cells. Exp Ther Med (2016) 12:1822–8. doi: 10.3892/etm.2016.3535
46. Stoeltzing O, McCarty MF, Wey JS, Fan F, Liu W, Belcheva A, et al. Role of Hypoxia-Inducible Factor 1alpha in Gastric Cancer Cell Growth, Angiogenesis, and Vessel Maturation. J Natl Cancer Inst (2004) 96:946–56. doi: 10.1093/jnci/djh168
47. Yamamoto Y, Fujita M, Tanaka Y, Kojima I, Kanatani Y, Ishihara M, et al. Low Oxygen Tension Enhances Proliferation and Maintains Stemness of Adipose Tissue-Derived Stromal Cells. Biores Open Access (2013) 2:199–205. doi: 10.1089/biores.2013.0004
48. Hung SP, Ho JH, Shih YR, Lo T, Lee OK. Hypoxia Promotes Proliferation and Osteogenic Differentiation Potentials of Human Mesenchymal Stem Cells. J Orthop Res (2012) 30:260–6. doi: 10.1002/jor.21517
Keywords: peripheral blood-derived mesenchymal stromal cells (PBMSCs), hypoxia, HIF-1α, proliferation, stemness
Citation: Wang P, Zhu P, Yu C and Wu J (2022) The Proliferation and Stemness of Peripheral Blood-Derived Mesenchymal Stromal Cells Were Enhanced by Hypoxia. Front. Endocrinol. 13:873662. doi: 10.3389/fendo.2022.873662
Received: 11 February 2022; Accepted: 05 April 2022;
Published: 12 May 2022.
Edited by:
Ihtisham Bukhari, Fifth Affiliated Hospital of Zhengzhou University, ChinaReviewed by:
Fu Wang, Xi’an Jiaotong University, ChinaZoey Xia, Second Hospital of Tianjin Medical University, China
Copyright © 2022 Wang, Zhu, Yu and Wu. This is an open-access article distributed under the terms of the Creative Commons Attribution License (CC BY). The use, distribution or reproduction in other forums is permitted, provided the original author(s) and the copyright owner(s) are credited and that the original publication in this journal is cited, in accordance with accepted academic practice. No use, distribution or reproduction is permitted which does not comply with these terms.
*Correspondence: Pengzhen Wang, d2FuZzUyMWpudUAxNjMuY29t