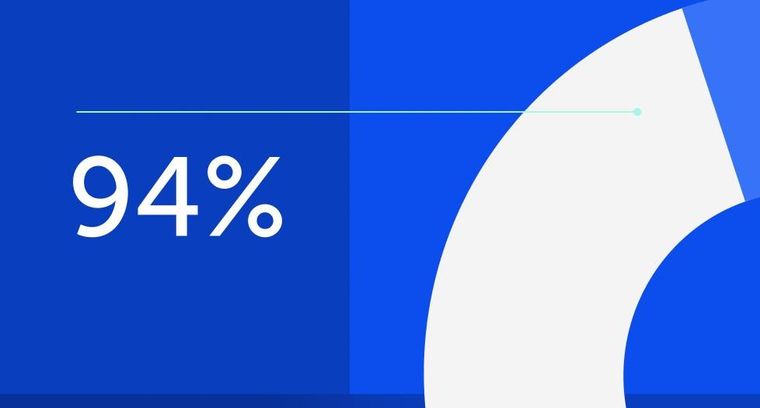
94% of researchers rate our articles as excellent or good
Learn more about the work of our research integrity team to safeguard the quality of each article we publish.
Find out more
REVIEW article
Front. Endocrinol., 28 March 2022
Sec. Bone Research
Volume 13 - 2022 | https://doi.org/10.3389/fendo.2022.861878
Diabetes mellitus (DM) can affect bone metabolism and the bone microenvironment, resulting in impaired bone healing. The mechanisms include oxidative stress, inflammation, the production of advanced glycation end products (AGEs), etc. Improving bone healing in diabetic patients has important clinical significance in promoting fracture healing and improving bone integration. In this paper, we reviewed the methods of improving bone healing under diabetic conditions, including drug therapy, biochemical cues, hyperbaric oxygen, ultrasound, laser and pulsed electromagnetic fields, although most studies are in preclinical stages. Meanwhile, we also pointed out some shortcomings and challenges, hoping to provide a potential therapeutic strategy for accelerating bone healing in patients with diabetes.
Diabetes is a metabolic disorder caused by insufficient insulin secretion and/or insulin resistance in target tissue (1). In 2019, approximately 463 million people worldwide had diabetes, with an adult prevalence rate of 9.3%. The adult prevalence of diabetes is expected to reach 10.2% by 2030 (2). Numerous clinical and in vivo or in vitro studies have shown that type 1 diabetes mellitus (T1DM) and type 2 diabetes mellitus (T2DM) both increase the risk of fractures and have a negative impact on bone healing (3). Compromised bone healing in patients with diabetes is associated with changes in the interaction between osteoblasts, adipocytes, bone marrow stem cells (BMSCs), and the marrow environment (4). Generally, chronic hyperglycemia can lead to oxidative stress, inflammatory reactions, adipogenesis/osteogenesis transformation imbalance, signaling pathway activation/inhibition, and bone microvascular changes (5). The premature accumulation of senescent cells may also lead to accelerated bone aging (6), which results in reduced bone strength and impaired bone formation. And it is worth mentioning that hyperglycemia has been linked to the formation of AGEs, which play a primordial role in damaged bone healing in DM (7), and may increase the risk of fracture in T1DM (8) and T2DM (9). As an important part of inflammatory events in diabetes and its complications, AGEs, interacting with the receptor for AGEs (RAGE), participates in the development of diabetic osteopathy (10). Mechanisms of chronic hyperglycemia on bone healing are summarized in Figure 1. However, the mechanisms leading to compromised bone healing in T1DM and T2DM are different. In T1DM, reduced insulin synthesis and amylin deficiency may initiate cascading reactions (11). In T2DM, compromised bone healing may be attributed to insulin resistance and impaired incretin effects (12). At present, bone healing in diabetic states is mainly improved by hypoglycemic therapy (13). Nevertheless, many other treatments have been shown in laboratory studies to promote bone healing in diabetic states, which may become potential strategies in further clinical studies. Therefore, this paper reviews methods for improving bone healing under diabetic conditions, only mechanisms of drugs or therapies possibly associated with benefits will be reviewed, hoping to provide potential strategies for accelerating bone healing in diabetic states.
Figure 1 Mechanisms of chronic hyperglycemia on bone healing. Chronic hyperglycemia can lead to oxidative stress, inflammatory reactions, adipogenesis/osteogenesis transformation imbalance, the production of AGEs, signaling pathway activation/inhibition, and bone microvascular changes, which can result in impaired bone healing.
Patients with diabetes need to take oral or injectable anti-diabetes drugs to control blood glucose. Studies have suggested that longer disease duration and worse glycemic control is associated with higher fracture risks in patients with diabetes (14). At the same time, anti-diabetes drugs may directly influence bone cells or indirectly affect bone metabolism and bone healing. Metformin is the first-line drug for the treatment of diabetes recognized worldwide and is widely used in all stages of diabetes treatment. Metformin can increase the intracellular AMP/ATP ratio, thereby activating the AMP-activated protein kinase (AMPK) signaling pathway, triggering the rapid activation of insulin and lysosomes, reducing intracellular glucose levels, and ultimately promoting the proliferation and differentiation of osteoblasts (15). The direct effect of metformin on osteogenesis functions by activating the BMP-4/Smad/Runx2 signaling pathway, upregulating the expression of Runt-related transcription factor 2 (Runx2) (16), activating extracellular signal-regulated kinases (ERK) 1/2 and p42/p44 mitogen-activated protein kinase (MAPK) (17), inhibiting the GSK-3β/Wnt/β-catenin pathway to promote the osteogenic differentiation of human BMSCs (18), and significantly increasing the formation of MSC mineralized nodules through the LKB1/AMPK pathway (19). A large number of experiments have confirmed that metformin can directly affect bone formation through the joint regulation of the multiple signaling pathways mentioned above. On the other hand, metformin can reduce intracellular reactive oxygen species (ROS) accumulation and inhibit AGEs-induced cellular inflammation through AMPK activation and RAGE/NF-κB pathway, thereby reducing oxidative stress and indirectly affecting bone healing (20–22). In terms of implants, experiments have proven that metformin can significantly promote the formation of new bone, improve the microstructure of bone, and promote the osseointegration of implants in both direct and indirect ways, such as increasing the autophagy of osteoporotic BMSCs under hypoxic and serum deprivation culture conditions, reducing ROS production and increasing the expression of osteogenic markers (23). This shows that metformin also has potential application prospects in the application of oral implants.
Intestinal hormones can accelerate glucose absorption by inducing the pancreas to secrete insulin, including glucose-dependent insulinotropic peptide (GIP) and glucagon-like peptide-1 (GLP-1). On the one hand, GIP can inhibit the apoptosis of osteoblasts and BMSCs (24, 25). On the other hand, GIP reduces the activity of osteoclasts in a dose-dependent manner and inhibits bone resorption induced by parathyroid hormone (PTH) and receptor activator of nuclear factor-κB ligand (RANKL) (26).
The level of GLP-1 rises immediately after a meal, while the circulating level of intact GLP-1 decreases rapidly under dipeptidyl peptidase-4 (DPP-4)-mediated inactivation. Therefore, GLP-1 receptor agonists and DPP-4 inhibitors are effective drugs for treating T2DM (27). GLP-1 receptor agonists can increase the serum level of the bone formation marker osteocalcin, thereby increasing bone mass and strength (28). Both exendin-4 and liraglutide are GLP-1 receptor agonists that can not only promote bone formation directly but also inhibit osteoclast differentiation and bone resorption. Exendin-4 has been shown to promote the differentiation of BMSCs into osteoblasts through the Wnt/β-catenin signaling pathway (29). At the same time, experiments have demonstrated that liraglutide can activate the PI3K/AKT, ERK1/2, and cAMP/PKA/β-cat-Ser675 signaling pathways through GLP-1 receptors and directly promote osteogenic proliferation and differentiation in the osteoblast cell line MC3T3-E1 (30). On the other hand, studies have found that Exendin-4 can inhibit RANKL-induced osteoclast differentiation and bone resorption by activating GLP-1 receptors, thereby preventing bone trabecular microstructure deterioration and enhancing bone strength (31). It can also inhibit macrophages to produce tumor necrosis factor-α (TNF-α) in lipopolysaccharide (LPS) environments, thereby reducing LPS-induced osteoclast differentiation and bone resorption (32).
DPP-4 inhibitors, including sitagliptin, saxagliptin and linagliptin, achieve blood glucose control in patients with diabetes by extending the circulating half-life period of endogenous insulin. Animal experiments have shown that the DPP-4 inhibitor linagliptin can inhibit the increase in TNF-α and RANKL mRNA expression levels in mice treated with LPS, prevent LPS-induced osteoclast differentiation and bone resorption, induce macrophages to differentiate to the M2 phenotype, and inhibit TNF-α-induced osteoclast differentiation and dental root resorption, thereby improving inflammation-induced bone resorption (33). There are relatively few human studies on the effects of DPP-4 inhibitors on bones. However, meta-analysis and cohort studies have shown that DPP-4 inhibitors can reduce the risk of fractures compared with placebo and other anti-diabetic drugs (34–36).
Studies on the effects of sulfonylureas on bone metabolism are still lacking, and there are conflicting results in existing data. Some studies have shown that it has a potential stimulating impact on the proliferation and differentiation of osteoblasts and has a protective effect on osteoblasts in hyperglycemic environments (5, 37), but some studies have found that it increases the risk of falls and fractures (38, 39). Therefore, further research is needed on the effect of sulfonylureas on bone metabolism.
The effect of anti-diabetes drugs on bone healing under diabetic conditions should be fully considered in clinical application. In the absence of other contraindications or adverse reactions, doctors should minimize the use of drugs such as thiazolidinediones that may lead to diabetes bone-related complications (40) and try to use metformin, DPP-4I, GLP-1 receptor agonists and other drugs that can effectively promote bone healing to improve the overall bone condition of patients with diabetes.
In recent years, natural medicine extracts have received increasing attention. Studies have found that several natural medicine extracts play an active role in bone regeneration. The following is a brief introduction to natural medicine extracts that can improve bone healing. Berberine, curcumin and resveratrol all play a dual role in antidiabetes and promoting bone healing and have broad clinical application prospects. Studies have found that berberine can activate osteoblast and osteoblast biomarker genes and promote bone regeneration. It can also inhibit bone loss caused by the T2DM drug pioglitazone through the AMPK pathway (41). The latest study by Jingjing Shao et al. (42) found that berberine may upregulate the ROS-mediated IRS-1 signaling pathway, thereby reducing the osteogenesis of BMSCs inhibited by high glucose. In addition, in vivo studies have shown that the combined application of berberine and insulin can promote implant osseointegration in diabetic rats (43). Curcumin is a polyphenolic phytochemical that is mainly extracted from turmeric. It has a positive effect on preventing and treating osteoporosis and bone deterioration in rheumatoid arthritis and other inflammatory diseases (44). In improving diabetic bone healing, curcumin has been shown to inhibit bone resorption and reduce the number of osteoclasts stimulated by diabetes (45). Studies in recent years have further confirmed that curcumin can promote bone repair around titanium implants in diabetic rats induced by streptozotocin through the Wnt/β-catenin signaling pathway and upregulation of BMP-2 levels (46). Resveratrol is a polyphenol plant antitoxin that is mainly extracted from plants and fruits (such as grapevines). Research by Yunwei Hua et al. (47) showed that resveratrol, as a Sirtuin1 agonist, can activate Sirtuin1, inhibit sclerostin, and reduce the impact of diabetes on implant osseointegration, resulting in increased bone density, improved trabecular bone structure, and biomechanical fixation enhancement. Moreover, in a double-blind randomized controlled trial in 2018, 192 T2DM patients were randomized to resveratrol 500 mg/day (Resv500), Resv40 mg/day (Resv40), or placebo. The results showed that resveratrol is effective in preventing bone density loss in patients with T2DM (48).
In addition, there are natural medicine extracts such as baicalein, ophiopogonin D (OP-D), and tubeimoside I (TBMS1). Although they do not have anti-diabetic effects, they have also been proven in preclinical studies to improve bone healing in diabetic animal models. These drugs still lack more experiments to verify their effectiveness in clinical applications. In vivo experiments of baicalein have been proven to have no effect on the insulin sensitivity index in chronic periodontitis with DM animal models, but baicalein can reduce alveolar bone loss in diabetic rats by increasing the expression of phospho-nuclear factor erythroid 2-related factor 2 (pNrf2) (49). Increased expression of pNrf2 can mediate the Nrf2/Keap1 signaling pathway to maintain the proliferation, migration, pluripotency and regeneration ability of MSCs in diabetic rats and ultimately promote bone healing in diabetic models (50). OP-D can act as an antioxidant to protect cells from oxidative stress-induced damage (51). Recent studies have found that OP-D inhibits oxidative stress through a Wnt/β-catenin-dependent mechanism, thereby reducing the damaged osseointegration of titanium implants under diabetic conditions (52). TBMS1 is a pentacyclic triterpene saponin compound isolated from the plant Fritillaria Vulgaris. In vivo and in vitro experiments targeting osteoclasts and RANKL-induced signaling pathways have found that TBMS1 has a protective effect on bone loss in T2DM rats by inhibiting osteoclast formation, bone resorption, and the RANKL-induced NF-κB signaling pathway (53).
Morroniside is mainly extracted from Cornus officinalis. A study by Yi Sun et al. (54) found that morroniside can inhibit the AGE-RAGE signaling system by activating Glo1, thereby restoring the osteogenic differentiation of rat BMSCs exposed to hyperglycemic conditions. In vivo experiments in a T1DM rat model also showed that morroniside can reduce bone loss and improve bone microstructure. Glycyrrhizin is a high mobility group box-1 (HMGB1) inhibitor. Experiments have shown that glycyrrhizin can significantly attenuate the upregulation and interaction of HG-induced ligand receptors for RAGE by inhibiting HMGB1, reducing oxidative stress, reversing the downregulation of osteogenic markers, and promoting osteogenic differentiation (55).
Traditional Chinese natural medicine and extracts of natural medicine are often used under diabetic conditions as a supplement rather than professional medical advice. While natural medicine extracts are popular, there are still regulation, safety and efficacy concerns. Except for resveratrol, none of the other natural medicinal extracts listed above has been tested in clinical trials for bone healing in diabetic patients. The preclinical trials of natural medicinal extracts in diabetic bone healing have not been examined thoroughly, and the mechanism of natural medicinal extracts in improving diabetic bone healing is not completely clear. At the same time, there is still a lack of clinical trials. Although we believe that natural medicine extracts have great application prospects and are highly likely to become an important complementary therapy for diabetic bone healing, a large number of reproducible experiments are still needed to confirm the efficacy and safety of natural medicinal extracts in promoting diabetic bone healing.
Effective anti-osteoporosis drugs, such as vitamin D, bisphosphonates (e.g., risedronate, zoledronate and alendronate), sclerotin antibody, selective estrogen receptor modulators (e.g., raloxifene and bazedoxifene) and monoclonal RANKL antibodies, have also been shown to play a positive role in promoting bone healing under diabetic conditions. Vitamin D is an essential steroid hormone for the human body, and 1α,25-dihydroxy vitamin D3 (1,25VD3), as the main active form of vitamin D3, binds to the vitamin D binding protein in the plasma to reach target tissues and exert endocrine effects, regulating the metabolism of calcium and phosphorus. Experiments have shown that 1,25VD3 can reverse the reduction in osseointegration and mechanical strength, reduce the damage caused by AGEs-induced osteogenic differentiation, and downregulate the expression of RAGE, thereby promoting the osseointegration of T2DM titanium implants (56). A recent longitudinal study in 35 postmenopausal women found that bone fragility due to T2DM and aging was significantly improved after 1000 IU daily vitamin D supplementation for 12 months (57). In an in vivo study, risedronate reduced the number and function of osteoclasts in diabetic mice, and increased bone mineral density and vertebral mechanical strength in the femoral shaft and vertebral body (58). In another experiment, both alendronate and raloxifene were found to have an anti-bone resorption effect in diabetic animal models, reducing bone turnover rate and increasing bone mechanical strength (59). Zoledronate and sclerotin antibodies also prevented bone defects by reversing the adverse effects of diabetes on bone mass and strength in rats (60, 61). At the same time, there are clinical trials demonstrating the effectiveness of these drugs in the treatment of diabetic osteoporosis patients. A retrospective analysis of the 3-year placebo-controlled freedom study and the 7-year extension study found that denosumab significantly increased bone mineral density and reduced the risk of vertebral fracture in patients with osteoporosis and diabetes (62). Another retrospective national cohort study in Denmark found that patients treated with alendronate and denosumab had a similar risk of osteoporotic fractures in patients with diabetes, suggesting that the two agents have similar efficacy in preventing osteoporotic fractures in patients with diabetes (63). In exploratory trials of postmenopausal women with T2DM, treatment with either bazedoxifene or raloxifene improved bone resorption markers without affecting glucose metabolism (64, 65). Therefore, we believe that these anti-osteoporosis drugs have a potential role in promoting bone healing in diabetic patients, which may provide new ideas for the treatment of diabetic osteoporosis or poor bone healing. Finding drugs that can effectively control blood glucose while maintaining bone health may become a direction for future research in the treatment of diabetic osteoporosis.
In addition to the abovementioned anti-diabetes drugs, natural medicine extracts and osteoporosis drugs, some drugs also have a significant effect on promoting bone healing in patients with diabetes. Doxycycline is a tetracyclic antibiotic that is often used clinically to treat various infectious diseases caused by sensitive gram-positive bacteria and gram-negative bacilli. In vitro experiments have found that doxycycline may restore the vitality and proliferation of osteoblasts and the osteogenesis process of BMSCs under diabetic conditions through the Wnt/β-catenin signaling pathway (66). In addition, zinc is considered to be a potential drug to prevent bone loss caused by diabetes. Studies have found that both zinc carbonate and zinc sulfate have a positive effect on maintaining bone structure and biomechanical parameters (67). Among them, zinc sulfate can prevent diabetic osteoporosis, and its protective mechanism is mainly related to its hypoglycemic effect, inhibiting bone marrow adipogenesis and upregulating the OPG/RANKL ratio and RUNX2 (68). At present, relevant clinical studies have reported that zinc supplements have achieved obvious efficacy in the treatment of postmenopausal women with osteoporosis, but unfortunately, there is currently a lack of clinical trials and reports on the application of doxycycline and zinc supplements in the treatment of compromised bone healing under diabetic conditions. We believe that both doxycycline and zinc supplements can be used as complementary treatments for compromised diabetic bone healing. Doxycycline is especially suitable for the nonantibacterial treatment of patients with systemic inflammation, while zinc supplements are suitable for promoting diabetic bone healing by reducing the RANK/OPG ratio.
Moreover, previous studies have shown that diabetic complications also play an important role in causing bone fragility and damaging bone health in patients with diabetes. A study concerning aging individuals with T1DM for more than 50 years indicated that lipid profile is associated with bone mineral density (BMD) values, suggesting that drugs controlling cardiovascular disease may be useful in promoting bone health in T1DM (69). Additionally, diabetic microvascular complications including neuropathy, retinopathy, and nephropathy are relevant to increased fracture risks (70, 71). Therefore, drugs that can prevent or improve diabetic microvascular complications such as aspirin, angiotensin-converting enzyme inhibitors, statins may be of potential benefits to bone health under diabetic conditions (72).
Previous studies have revealed that various hormones can promote bone healing under diabetic conditions. Uncarboxylated osteocalcin is a multifunctional hormone secreted by mature osteoblasts (73) that plays an essential role in promoting the osteogenic differentiation of BMSCs (74). In hyperglycemic environments, uncarboxylated osteocalcin can inhibit adipogenic differentiation and promote osteogenic differentiation of BMSCs, the mechanism of which is to reduce the expression of TP63 and then affect the PTEN/Akt/GSK3β signaling pathway (75). Adrenomedullin 2 (ADM2) is an endogenous peptide belonging to the calcitonin family (76) that decreases significantly under diabetic conditions, and the reduction in ADM2 levels is associated with DM-related metabolic disorders (77). Studies have shown that ADM2 therapy can promote M1 macrophage polarization toward the M2 phenotype by activating the PPARγ-mediated NF-κB signaling pathway and improve bone regeneration in diabetic rats (78). Norepinephrine (NE) is the primary medium of the sympathetic nervous system, and its ability to affect MSC migration has been shown in previous experiments both in vitro and in vivo (79). A study comparing BMSCs in diabetic mice undergoing sympathectomy with those that did not experience sympathectomy found that NE may have a protective effect on hyperglycemia-induced MSC apoptosis through the AKT/BCL-2 pathway (80). PTH enhances the MSC survival rate by inhibiting their aging and apoptosis (81) and promotes MSC differentiation toward osteogenesis rather than adipogenesis (82). In the fight against glucolipotoxicity, PTH can promote osteogenic differentiation of BMSCs by activating the p38 MAPK signaling pathway (83). Moreover, in vivo studies have shown that human parathyroid hormone (1–34) has a positive effect on bone fracture healing in T2DM (84). Recombinant human parathyroid hormone has been used as a drug to cure osteoporosis clinically (85). However, clinical trials are needed to further assess the effectiveness of recombinant hPTH in bone healing under diabetic conditions. Adiponectin (APN) plays a vital role in regulating energy metabolism at the cellular and systemic levels in diabetic environments (86). In patients with T2DM, APN concentration in plasma was significantly reduced, which is associated with damage in bone healing in diabetic states (87). In addition, APN activates the AMPK signaling pathway, plays an antioxidant role and protects mitochondria, reverses osteoblast damage, and improves bone integration in titanium implants (88). Therefore, integrating APN in scaffold-based systems may serve as a potential strategy to promote bone healing, but it still needs further investigation.
Many studies have demonstrated that activating or inhibiting signaling pathways associated with BMSC osteogenesis by designing specific signaling pathway regulators can promote bone healing in diabetic environments. GSK-3β, a kinase involved in blood glucose regulation (89), is a negative regulator of the Wnt signaling pathway and plays an important role in the regulation of bone metabolism (90). In hyperglycemic environments, GSK-3β is activated, and the Wnt signaling pathway is inhibited, damaging the proliferation of BMSCs (91). CHIR99021 is a GSK-3β inhibitor that inhibits the expression of β-catenin and CyclinD1 in hyperglycemic environments, promoting osteogenesis of BMSCs (92). In addition, previous studies have found that LiCl at 15 mM, as an inhibitor of GSK-3β, can also effectively reverse the inhibitory effect of hyperglycemia on BMSC osteogenesis (93). Brain and muscle ARNT-like protein 1 (BMAL1) is a core biological clock protein secreted by the suprachiasmatic nucleus, peripheral tissue, and stem cells (94). The study found that the overexpression of BMAL1 restores the bone-forming ability of BMSCs from diabetic rats by inhibiting the expression of the NF-κB signaling pathway (95). Therefore, bone metabolic balance in T2DM can be reconstructed by overexpression of BMAL1. As a glycoprotein secreted on the surface of cells, Semaphorin3B is closely related to the bone metabolism process, and it can improve defects in BMSC proliferation and osteogenesis in hyperglycemic environments by activating the Akt signaling pathway (96). Growth differentiation factor 11 (GDF11) is a bone morphological protein (BMP) whose expression is positively correlated with the incidence of osteoporosis in diabetic patients (97). A study was conducted to regularly inject GDF11 inhibitors into the tooth extraction socket in T2DM pigs, and it found that GDF11 can improve bone healing in the tooth extraction socket and promote osteogenesis of MSCs under T2DM conditions (98). Although these signaling pathway regulators mentioned above are only currently used in laboratories, they help us understand the underlying mechanisms in compromised bone healing under diabetic conditions, and they can be used as potential therapeutic targets to recover abnormal bone homeostasis caused by DM.
BMP is one of the most potent inducers of bone differentiation in MSCs (99). In the BMP family, BMP2, BMP4, BMP6, BMP7 and BMP9 all have bone-forming properties (100). Studies in streptozocin-induced diabetic mice have shown that BMP6 treatment can reduce bone loss in diabetic mice and that BMP6 plays an important role in T1DM-related bone loss (101). Vascular endothelial growth factors (VEGF) induce bone formation through direct and indirect pathways. VEGF can directly attract MSCs and promote osteogenesis while also promoting local angiogenesis, enhancing vascular permeability, accumulating MSCs, and indirectly promoting bone regeneration (102). Basic fibroblast growth factor (bFGF) is a mitogen that regulates bone cell proliferation, differentiation and mineralization (103). Several studies have shown a synergistic effect on promoting bone differentiation of MSCs between BMP and VEGF (100) and between BMP, VEGF and bFGF (104). This joint application is a new and promising improvement strategy in bone tissue engineering. In future studies, researchers can further explore the factors affecting its synergy and effectively promote bone regeneration in diabetic environments. Insulin-like growth factors (IGF1), when combined with IGF1 receptors, play an essential role in bone development, growth and physiological strength maintenance but require daily injections or surgical implants. A current study has shown that expressing codon-optimized Pro-IGF-1 with e-peptide in the chloroplast can be administered orally, significantly promoting bone regeneration in diabetic mice (105). This new type of administration can not only facilitate affordability but also enhance patient compliance; therefore, it may be a potential treatment for bone healing in diabetic patients. Progranulin (PGRN) is a multifunctional cytokine that has been shown to promote cartilage formation and participate in physiological fracture healing mainly through TNF receptor 2 (TNFR2) signaling pathways (106). The latest experiments have found that local application of recombinant PGRN in diabetic rats can effectively promote the healing of diabetic fractures. The mechanism of promoting cartilage formation may be related to the TNFR2-Akt and ERK1/2 pathways, as well as its role in inhibiting inflammation in the process of diabetic bone regeneration (107, 108).
The combination of bone-induced growth factors with bone-conductive biomaterials is also an important and promising way to promote bone regeneration (109). Studies have shown that implanting 3D bioprint scaffolds containing MSNs/BMP-4, BMSCs and RAW264.7 in bone defects can significantly promote bone healing in diabetic rats, and the mechanism is that BMP-4 can directly promote BMSC bone formation. BMP-4 can regulate macrophage cell differentiation to M2 macrophage polarization to improve the inflammatory microenvironment (110). Jian Li et al. (111) developed a BMP-2 and VEGF-derived peptide-decorated n-HA/PA66 (BVHP66) scaffold that significantly enhances the proliferation of BMSCs and human umbilical vein endothelial cells (HUVECs), promotes bone differentiation of BMSCs and blood vessel formation in hyperglycemic environments, and therefore improves bone healing in diabetic environments. In addition, some studies have achieved the delivery of growth factors through nonviral gene therapy, which can avoid the side effects of overdosing on recombinant human proteins (112). Behnoush Khorsand et al. (113) prepared a composite scaffold by building plasmid DNA encoding BMP-2 and FGF-2 combined with polyethylenimine through electrostatic action, which has been shown to improve bone regeneration in diabetic rabbits. In complicated diabetic environments, an autonomous tissue engineering system that can release growth factors accurately according to the dynamic environment is required in future research. Moreover, clinical experiments are also expected to be evaluated.
Exosomes, with a diameter of 50-100 nm, are extracellular organelles secreted by cells and are capable of carrying bioactive substances such as noncoding RNA, mRNA, DNA, proteins, and other molecules (114). Exosomes can transport specific miRNAs and mediate cell and tissue-to-tissue communication (115). Studies have shown that both exosomes secreted by bone marrow stem cells in rats with T1DM (dBMSC-exos) and exosomes secreted by normal rat bone marrow stem cells (nBMSC-exos) can promote bone regeneration and angiogenesis, but the effect of dBMSC-exos is weaker than that of nBMSC-exos (116). Studies have also shown that exosomes derived from adipose-derived mesenchymal stem cells (AMSCs) inhibit the secretion of IL-1β and IL-18 by osteoclasts in hyperglycemic environments, reducing bone absorption and restoring bone loss (117). However, at present, MSC exosome-related research is still in the preclinical stage, and the traditional methods of exosome separation and characterization identification are not effective for clinical application. In the future, we need to further explore the exact mechanism of MSC exosomes in bone formation, bone cell differentiation, angiogenesis, inflammatory response, etc. Developing large-scale methods for the production, separation and purification of exosomes is also of vital importance.
Several miRNAs regulate BMSC proliferation, migration, differentiation and apoptosis and are important regulatory factors in bone healing (118). The miRNA from the exosome source can be steadily transferred from the bone microenvironment to BMSCs, regulating bone differentiation and bone healing, but the hyperglycemic environment can affect its expression. The study found that in hyperglycemic environments, the expression of miR-124-3p in osteocyte-derived exosomes may inhibit the expression of Gal-3 in osteoblasts, which in turn can lead to a decrease in bone capacity (119). MiR-144-5p levels also rise in exosomes secreted by diabetic bone marrow-derived macrophages, which can be transferred to BMSCs to inhibit bone regeneration by targeting Smad1 (120). Designing specific inhibitors of the miRNA as mentioned above may be a potential strategy to reverse the adverse effects of hyperglycemia on bone healing in diabetic patients.
Hyperbaric oxygen (HBO) treatment refers to intermittent inhalation of 100% oxygen at pressures above 1.5 absolute atmospheric pressures (121). Hyperbaric oxygen therapy can improve the biometric properties of the femur in diabetic animal models and increase the content of collagen and crystalline hydroxyapatite (122). In addition, some studies have evaluated the effectiveness of hyperbaric oxygen therapy for implant bone integration in diabetic states through diabetic animal models, and the results showed that HBO therapy can enhance implant bone integration by calculating BIC through tissue morphology (123). However, some studies suggest that HBO only improves early bone integration in diabetic rabbits, which is not enough to improve the mechanical stability of implants (124). Therefore, it can be determined that HBO has positive effects on bone integration in diabetic environments at the histological and biomechanical levels, but there is a lack of studies of its specific mechanisms, and further clinical research is needed to evaluate the effectiveness of HBO as an auxiliary treatment for diabetic patients.
Ultrasound is an oscillating longitudinal pressure wave with a frequency over 20 kHz that cannot be detected by the human auditory system. Ultrasound has applications in many medical fields, including low-intensity pulsed ultrasound (LIPUS) for diagnostic imaging, medium-intensity ultrasound for physical therapy, and high-intensity focused ultrasound for surgical resection. LIPUS has been used clinically for more than 20 years. A large number of in vivo and in vitro experiments and clinical trials have verified the safety and effectiveness of LIPUS in promoting bone healing (125, 126). However, there are relatively few studies on LIPUS in bone healing in patients with diabetes. Past experiments have shown that in diabetic rat fracture models, LIPUS can increase the expression of growth factors in the diabetic group and promote cartilage formation and angiogenesis (127). An experiment aimed at the effect of LIPUS on the alveolar structure in the process of orthodontic force in diabetic patients found that the application of LIPUS treatment, 10 minutes a day for one week, can promote normal and diabetic mandibular slice organ culture (MSOCs) bone remodeling and restoration of cementum and dentin (128). Although we believe that ultrasound has clinical potential in promoting bone healing in patients with diabetes and can even be used in the dental field, more experiments are still needed to prove and explore the underlying mechanism.
Photobiomodulation (PBM) is a nonthermal light treatment that involves endogenous chromophores. Previous experiments have shown that PBM can promote bone healing in diabetic rat models by improving the viability of osteoblasts and mesenchymal cells (129, 130). Experiments on diabetic rats proved that PBM treatment can improve the viability of osteoblasts, significantly increase the mRNA expression of RUNX2 and osteocalcin, and increase the activity of alkaline phosphatase and the production of the mineralized matrix, thereby regulating the bone healing process (131, 132). In addition, PBM has also been shown to improve the survival, proliferation and apoptosis of BMSCs in diabetic rats (133).
Low-level laser therapy (LLLT) refers to radiation with a wavelength range of 500-1100 nm and a power of 1 mW-500 mW. It has the characteristics of relatively low energy density and has been used clinically to treat various diseases (134). The effect of LLLT in promoting bone healing has been verified by a large number of experiments, mainly by promoting the proliferation of osteoblasts, increasing the growth factor secreted by osteoblasts (135), the transportation of calcium (136) and increasing angiogenesis to promote bone healing (137). However, there are relatively few studies on diabetic bone healing. A previous study on the effect of LLLT and dual-type allograft materials on the healing of diabetic bone found that LLLT can effectively stimulate osteoblast production but cannot promote bone formation (138). However, other studies have shown that LLLT can stimulate bone metabolism, reduce bone resorption area, increase RUNX-2 expression, increase serum alkaline phosphatase levels, increase cortical area, fracture strength, BMD and bone mineral content (BMC), and promote bone healing (139, 140). Different experiments have shown almost the opposite results, which suggests that whether LLLT can promote the healing of diabetic bone, as well as the specific mechanism of promoting diabetic bone healing, still need many experiments to be studied.
In the past few decades, low-intensity pulsed electromagnetic fields (LIPEMFs) have played a positive role in the skeletal system. The application of PEMF treatment for patients with osteoporosis can significantly increase bone density and prevent bone loss (141, 142). Studies on animal models of diabetes found that LIPEMF can restore the expression of Runx2 through the Wnt/β-catenin signaling pathway and reverse the deterioration of bone microstructure and strength, thereby preventing bone loss caused by diabetes, but has no effect on osteoclasts (143–145). The positive effect of LIPEMF in promoting bone healing has been confirmed in a large number of experiments. Many preclinical experiments have proven that LIPEMF can effectively promote bone healing in diabetic animal models. However, there is still a lack of clinical trials for bone healing in diabetic patients. Although we believe that LIPEMF has clinical potential in promoting diabetic bone healing and may become a potential method to inhibit diabetic osteoporosis, its effectiveness lacks clinical experimental support.
To date, with the continuous progress of preclinical studies, the mechanism of HBO therapy, LIPUS, PBM, LLLT and LIPEMF in promoting diabetic bone healing has been gradually clarified. We believe that the physical therapies mentioned above have clinical potential in promoting diabetic bone healing. However, there have been no clinical studies to confirm these findings. With the gradual increase in the number of patients with diabetes and the occurrence of a large number of related bone healing complications, we believe that it is necessary to further study the potential role and safety of physical replacement therapy in bone healing in patients with diabetes, and these therapies may become an important part of the treatment of bone healing complications of diabetes.
With the improvement of living standards and the rising prevalence of diabetes, it is urgent to explore how to improve bone healing repair in diabetes. This paper reviews the effects and possible mechanisms of diabetes on bone healing and summarizes several current methods to improve bone healing under diabetic conditions, although some studies are still in their early stages, including the use of drugs, hormones, signaling pathway regulators, growth factors, exosomes, etc. In addition, some physical therapies, such as hyperbaric oxygen, ultrasound, laser, and pulsed electromagnetic fields, also have a certain clinical potential in promoting bone healing under diabetic conditions (Figure 2).
Some clinical trials have proven that drugs related to glycemic control are beneficial to bone health in patients with diabetes. For example, metformin can increase the level of bone formation marker in T2DM patients (146), liraglutide has an anti-resorptive effect on bone turnover in patients with T2DM (147), GLP-1 receptor agonists can increase BMD at multiple sites of the body in T2DM patients (148), denosumab can significantly increase BMD and decrease vertebral fracture risk in postmenopausal women with osteoporosis and diabetes (62), etc. LLLT can improve implant stability in patients with diabetes in 6 months trial (149). However, there is no clinical research evidence specifically designed to improve fracture healing in patients with diabetes (72). And at present, there is no direct proof in existing clinical trials that the abovementioned methods have beneficial effects in improving bone healing under diabetic conditions. Therefore, clinical research on the methods mentioned above still lacks research, and their effectiveness and potential for clinical application still need to be further explored. Whether they can be adopted by clinical applications also needs further discussion.
YC and YZ conceived and wrote the manuscript. JL and SZ reviewed and edited the manuscript. All authors contributed to the article and approved the submitted version.
This work was supported by grants from the Crosswise Tasks of Sichuan University (Document No. 21H0441, Funding No. 00403055A1042).
The authors declare that the research was conducted in the absence of any commercial or financial relationships that could be construed as a potential conflict of interest.
All claims expressed in this article are solely those of the authors and do not necessarily represent those of their affiliated organizations, or those of the publisher, the editors and the reviewers. Any product that may be evaluated in this article, or claim that may be made by its manufacturer, is not guaranteed or endorsed by the publisher.
1. American Diabetes Association. Diagnosis and Classification of Diabetes Mellitus. Diabetes Care (2014) 37(Suppl 1):S81–90. doi: 10.2337/dc14-S081
2. Saeedi P, Petersohn I, Salpea P, Malanda B, Karuranga S, Unwin N, et al. Global and Regional Diabetes Prevalence Estimates for 2019 and Projections for 2030 and 2045: Results From the International Diabetes Federation Diabetes Atlas, 9(Th) Edition. Diabetes Res Clin Pract (2019) 157:107843. doi: 10.1016/j.diabres.2019.107843
3. Sellmeyer DE, Civitelli R, Hofbauer LC, Khosla S, Lecka-Czernik B, Schwartz AV. Skeletal Metabolism, Fracture Risk, and Fracture Outcomes in Type 1 and Type 2 Diabetes. Diabetes (2016) 65(7):1757–66. doi: 10.2337/db16-0063
4. Murray CE, Coleman CM. Impact of Diabetes Mellitus on Bone Health. Int J Mol Sci (2019) 20(19):4873. doi: 10.3390/ijms20194873
5. Napoli N, Chandran M, Pierroz DD, Abrahamsen B, Schwartz AV, Ferrari SL. Mechanisms of Diabetes Mellitus-Induced Bone Fragility. Nat Rev Endocrinol (2017) 13(4):208–19. doi: 10.1038/nrendo.2016.153
6. Khosla S, Samakkarnthai P, Monroe DG, Farr JN. Update on the Pathogenesis and Treatment of Skeletal Fragility in Type 2 Diabetes Mellitus. Nat Rev Endocrinol (2021) 17(11):685–97. doi: 10.1038/s41574-021-00555-5
7. Santana RB, Xu L, Chase HB, Amar S, Graves DT. Trackman PC. A Role for Advanced Glycation End Products in Diminished Bone Healing in Type 1 Diabetes. Diabetes (2003) 52(6):1502–10. doi: 10.2337/diabetes.52.6.1502
8. Rubin MR, Paschalis EP, Poundarik A, Sroga GE, McMahon DJ, Gamsjaeger S, et al. Advanced Glycation Endproducts and Bone Material Properties in Type 1 Diabetic Mice. PloS One (2016) 11(5):e0154700. doi: 10.1371/journal.pone.0154700
9. Furst JR, Bandeira LC, Fan WW, Agarwal S, Nishiyama KK, McMahon DJ, et al. Advanced Glycation Endproducts and Bone Material Strength in Type 2 Diabetes. J Clin Endocrinol Metab (2016) 101(6):2502–10. doi: 10.1210/jc.2016-1437
10. Asadipooya K, Uy EM. Advanced Glycation End Products (Ages), Receptor for Ages, Diabetes, and Bone: Review of the Literature. J Endocr Soc (2019) 3(10):1799–818. doi: 10.1210/js.2019-00160
11. Romero-Díaz C, Duarte-Montero D, Gutiérrez-Romero SA, Mendivil CO. Diabetes and Bone Fragility. Diabetes Ther (2021) 12(1):71–86. doi: 10.1007/s13300-020-00964-1
12. Napoli N, Strollo R, Paladini A, Briganti SI, Pozzilli P, Epstein S. The Alliance of Mesenchymal Stem Cells, Bone, and Diabetes. Int J Endocrinol (2014) 2014:690783. doi: 10.1155/2014/690783
13. Lecka-Czernik B. Diabetes, Bone and Glucose-Lowering Agents: Basic Biology. Diabetologia (2017) 60(7):1163–9. doi: 10.1007/s00125-017-4269-4
14. Leanza G, Maddaloni E, Pitocco D, Conte C, Palermo A, Maurizi AR, et al. Risk Factors for Fragility Fractures in Type 1 Diabetes. Bone (2019) 125:194–9. doi: 10.1016/j.bone.2019.04.017
15. Zhang CS, Li M, Ma T, Zong Y, Cui J, Feng JW, et al. Metformin Activates Ampk Through the Lysosomal Pathway. Cell Metab (2016) 24(4):521–2. doi: 10.1016/j.cmet.2016.09.003
16. Liang C, Sun R, Xu Y, Geng W, Li J. Effect of the Abnormal Expression of Bmp-4 in the Blood of Diabetic Patients on the Osteogenic Differentiation Potential of Alveolar Bmscs and the Rescue Effect of Metformin: A Bioinformatics-Based Study. BioMed Res Int (2020) 2020:7626215. doi: 10.1155/2020/7626215
17. Cortizo AM, Sedlinsky C, McCarthy AD, Blanco A, Schurman L. Osteogenic Actions of the Anti-Diabetic Drug Metformin on Osteoblasts in Culture. Eur J Pharmacol (2006) 536(1-2):38–46. doi: 10.1016/j.ejphar.2006.02.030
18. Ma J, Zhang ZL, Hu XT, Wang XT, Chen AM. Metformin Promotes Differentiation of Human Bone Marrow Derived Mesenchymal Stem Cells Into Osteoblast Via Gsk3β Inhibition. Eur Rev Med Pharmacol Sci (2018) 22(22):7962–8. doi: 10.26355/eurrev_201811_16424
19. Wang P, Ma T, Guo D, Hu K, Shu Y, Xu HHK, et al. Metformin Induces Osteoblastic Differentiation of Human Induced Pluripotent Stem Cell-Derived Mesenchymal Stem Cells. J Tissue Eng Regener Med (2018) 12(2):437–46. doi: 10.1002/term.2470
20. Jiating L, Buyun J, Yinchang Z. Role of Metformin on Osteoblast Differentiation in Type 2 Diabetes. BioMed Res Int (2019) 2019:9203934. doi: 10.1155/2019/9203934
21. Lin J, Xu R, Shen X, Jiang H, Du S. Metformin Promotes the Osseointegration of Titanium Implants Under Osteoporotic Conditions by Regulating Bmscs Autophagy, and Osteogenic Differentiation. Biochem Biophys Res Commun (2020) 531(2):228–35. doi: 10.1016/j.bbrc.2020.06.146
22. Zhou Z, Tang Y, Jin X, Chen C, Lu Y, Liu L, et al. Metformin Inhibits Advanced Glycation End Products-Induced Inflammatory Response in Murine Macrophages Partly Through Ampk Activation and Rage/Nfκb Pathway Suppression. J Diabetes Res (2016) 2016:4847812. doi: 10.1155/2016/4847812
23. Sun R, Liang C, Sun Y, Xu Y, Geng W, Li J. Effects of Metformin on the Osteogenesis of Alveolar Bmscs From Diabetic Patients and Implant Osseointegration in Rats. Oral Dis (2021). doi: 10.1111/odi.13808
24. Tsukiyama K, Yamada Y, Yamada C, Harada N, Kawasaki Y, Ogura M, et al. Gastric Inhibitory Polypeptide as an Endogenous Factor Promoting New Bone Formation After Food Ingestion. Mol Endocrinol (2006) 20(7):1644–51. doi: 10.1210/me.2005-0187
25. Berlier JL, Kharroubi I, Zhang J, Dalla Valle A, Rigutto S, Mathieu M, et al. Glucose-Dependent Insulinotropic Peptide Prevents Serum Deprivation-Induced Apoptosis in Human Bone Marrow-Derived Mesenchymal Stem Cells and Osteoblastic Cells. Stem Cell Rev Rep (2015) 11(6):841–51. doi: 10.1007/s12015-015-9616-6
26. Zhong Q, Itokawa T, Sridhar S, Ding KH, Xie D, Kang B, et al. Effects of Glucose-Dependent Insulinotropic Peptide on Osteoclast Function. Am J Physiol Endocrinol Metab (2007) 292(2):E543–8. doi: 10.1152/ajpendo.00364.2006
27. Kitaura H, Ogawa S, Ohori F, Noguchi T, Marahleh A, Nara Y, et al. Effects of Incretin-Related Diabetes Drugs on Bone Formation and Bone Resorption. Int J Mol Sci (2021) 22(12):6578. doi: 10.3390/ijms22126578
28. Eller-Vainicher C, Cairoli E, Grassi G, Grassi F, Catalano A, Merlotti D, et al. Pathophysiology and Management of Type 2 Diabetes Mellitus Bone Fragility. J Diabetes Res (2020) 2020:7608964. doi: 10.1155/2020/7608964
29. Meng J, Ma X, Wang N, Jia M, Bi L, Wang Y, et al. Activation of Glp-1 Receptor Promotes Bone Marrow Stromal Cell Osteogenic Differentiation Through B-Catenin. Stem Cell Rep (2016) 6(4):579–91. doi: 10.1016/j.stemcr.2016.02.002
30. Wu X, Li S, Xue P, Li Y. Liraglutide, a Glucagon-Like Peptide-1 Receptor Agonist, Facilitates Osteogenic Proliferation and Differentiation in Mc3t3-E1 Cells Through Phosphoinositide 3-Kinase (Pi3k)/Protein Kinase B (Akt), Extracellular Signal-Related Kinase (Erk)1/2, and Camp/Protein Kinase a (Pka) Signaling Pathways Involving B-Catenin. Exp Cell Res (2017) 360(2):281–91. doi: 10.1016/j.yexcr.2017.09.018
31. Ma X, Meng J, Jia M, Bi L, Zhou Y, Wang Y, et al. Exendin-4, a Glucagon-Like Peptide-1 Receptor Agonist, Prevents Osteopenia by Promoting Bone Formation and Suppressing Bone Resorption in Aged Ovariectomized Rats. J Bone Miner Res (2013) 28(7):1641–52. doi: 10.1002/jbmr.1898
32. Shen WR, Kimura K, Ishida M, Sugisawa H, Kishikawa A, Shima K, et al. The Glucagon-Like Peptide-1 Receptor Agonist Exendin-4 Inhibits Lipopolysaccharide-Induced Osteoclast Formation and Bone Resorption Via Inhibition of Tnf-A Expression in Macrophages. J Immunol Res (2018) 2018:5783639. doi: 10.1155/2018/5783639
33. Ishida M, Shen WR, Kimura K, Kishikawa A, Shima K, Ogawa S, et al. Dpp-4 Inhibitor Impedes Lipopolysaccharide-Induced Osteoclast Formation and Bone Resorption in Vivo. BioMed Pharmacother (2019) 109:242–53. doi: 10.1016/j.biopha.2018.10.052
34. Hou WH, Chang KC, Li CY, Ou HT. Dipeptidyl Peptidase-4 Inhibitor Use Is Associated With Decreased Risk of Fracture in Patients With Type 2 Diabetes: A Population-Based Cohort Study. Br J Clin Pharmacol (2018) 84(9):2029–39. doi: 10.1111/bcp.13636
35. Dombrowski S, Kostev K, Jacob L. Use of Dipeptidyl Peptidase-4 Inhibitors and Risk of Bone Fracture in Patients With Type 2 Diabetes in Germany-A Retrospective Analysis of Real-World Data. Osteoporos Int (2017) 28(8):2421–8. doi: 10.1007/s00198-017-4051-y
36. Choi HJ, Park C, Lee YK, Ha YC, Jang S, Shin CS. Risk of Fractures and Diabetes Medications: A Nationwide Cohort Study. Osteoporos Int (2016) 27(9):2709–15. doi: 10.1007/s00198-016-3595-6
37. Palermo A, D'Onofrio L, Eastell R, Schwartz AV, Pozzilli P, Napoli N. Oral Anti-Diabetic Drugs and Fracture Risk, Cut to the Bone: Safe or Dangerous? A Narrative Review. Osteoporos Int (2015) 26(8):2073–89. doi: 10.1007/s00198-015-3123-0
38. Napoli N, Strotmeyer ES, Ensrud KE, Sellmeyer DE, Bauer DC, Hoffman AR, et al. Fracture Risk in Diabetic Elderly Men: The Mros Study. Diabetologia (2014) 57(10):2057–65. doi: 10.1007/s00125-014-3289-6
39. Johnston SS, Conner C, Aagren M, Ruiz K, Bouchard J. Association Between Hypoglycaemic Events and Fall-Related Fractures in Medicare-Covered Patients With Type 2 Diabetes. Diabetes Obes Metab (2012) 14(7):634–43. doi: 10.1111/j.1463-1326.2012.01583.x
40. Wei W, Wan Y. Thiazolidinediones on Pparγ: The Roles in Bone Remodeling. PPAR Res (2011) 2011:867180. doi: 10.1155/2011/867180
41. Yuhan Z, Jinlong M, Weifen Z. Berberine for Bone Regeneration: Therapeutic Potential and Molecular Mechanisms. J Ethnopharmacol (2021) 277:114249. doi: 10.1016/j.jep.2021.114249
42. Shao J, Liu S, Zheng X, Chen J, Li L, Zhu Z. Berberine Promotes Peri-Implant Osteogenesis in Diabetic Rats by Ros-Mediated Irs-1 Pathway. Biofactors (2021) 47(1):80–92. doi: 10.1002/biof.1692
43. Lu L, Zhijian H, Lei L, Wenchuan C, Zhimin Z, Patwardhan B. Berberine in Combination With Insulin Has Additive Effects on Titanium Implants Osseointegration in Diabetes Mellitus Rats. Evidence-Based Complement Altern Med (2015) 2015:824259. doi: 10.1155/2015/824259
44. Shang W, Zhao LJ, Dong XL, Zhao ZM, Li J, Zhang BB, et al. Curcumin Inhibits Osteoclastogenic Potential in Pbmcs From Rheumatoidarthritis Patients Via the Suppression of Mapk/Rank/C-Fos/Nfatc1 Signaling Pathways. Mol Med Rep (2016) 14(4):3620–6. doi: 10.3892/mmr.2016.5674
45. Hie M, Yamazaki M, Tsukamoto I. Curcumin Suppresses Increased Bone Resorption by Inhibiting Osteoclastogenesis in Rats With Streptozotocin-Induced Diabetes. Eur J Pharmacol (2009) 621(1):1–9. doi: 10.1016/j.ejphar.2009.08.025
46. Cirano FR, Pimentel SP, Casati MZ, Corrêa MG, Pino DS, Messora MR, et al. Effect of Curcumin on Bone Tissue in the Diabetic Rat: Repair of Peri-Implant and Critical-Sized Defects. Int J Oral Maxillofac Surg (2018) 47(11):1495–503. doi: 10.1016/j.ijom.2018.04.018
47. Hua Y, Bi R, Li Z, Li Y. Resveratrol Treatment Promotes Titanium Implant Osseointegration in Diabetes Mellitus Rats. J Orthop Res (2020) 38(10):2113–9. doi: 10.1002/jor.24651
48. Bo S, Gambino R, Ponzo V, Cioffi I, Goitre I, Evangelista A, et al. Effects of Resveratrol on Bone Health in Type 2 Diabetic Patients. A Double-Blind Randomized-Controlled Trial. Nutr Diabetes (2018) 8(1):51. doi: 10.1038/s41387-018-0059-4
49. Zhu C, Zhao Y, Wu X, Qiang C, Liu J, Shi J, et al. The Therapeutic Role of Baicalein in Combating Experimental Periodontitis With Diabetes Via Nrf2 Antioxidant Signaling Pathway. J Periodontal Res (2020) 55(3):381–91. doi: 10.1111/jre.12722
50. Rabbani PS, Soares MA, Hameedi SG, Kadle RL, Mubasher A, Kowzun M, et al. Dysregulation of Nrf2/Keap1 Redox Pathway in Diabetes Affects Multipotency of Stromal Cells. Diabetes (2019) 68(1):141–55. doi: 10.2337/db18-0232
51. Qian J, Jiang F, Wang B, Yu Y, Zhang X, Yin Z, et al. Ophiopogonin D Prevents H2o2 -Induced Injury in Primary Human Umbilical Vein Endothelial Cells. J Ethnopharmacol (2010) 128(2):438–45. doi: 10.1016/j.jep.2010.01.031
52. Ma XY, Wen XX, Yang XJ, Zhou DP, Wu Q, Feng YF, et al. Ophiopogonin D Improves Osteointegration of Titanium Alloy Implants Under Diabetic Conditions by Inhibition of Ros Overproduction Via Wnt/B-Catenin Signaling Pathway. Biochimie (2018) 152:31–42. doi: 10.1016/j.biochi.2018.04.022
53. Yang M, Xie J, Lei X, Song Z, Gong Y, Liu H, et al. Tubeimoside I Suppresses Diabetes-Induced Bone Loss in Rats, Osteoclast Formation, and Rankl-Induced Nuclear Factor-Kb Pathway. Int Immunopharmacol (2020) 80:106202. doi: 10.1016/j.intimp.2020.106202
54. Sun Y, Zhu Y, Liu X, Chai Y, Xu J. Morroniside Attenuates High Glucose-Induced Bmsc Dysfunction by Regulating the Glo1/Age/Rage Axis. Cell Prolif (2020) 53(8):e12866. doi: 10.1111/cpr.12866
55. Liu B, Gan X, Zhao Y, Yu H, Gao J, Yu H. Inhibition of Hmgb1 Promotes Osseointegration Under Hyperglycemic Condition Through Improvement of Bmsc Dysfunction. Oxid Med Cell Longev (2019) 2019:1703709. doi: 10.1155/2019/1703709
56. Jia T, Wang YN, Zhang D, Xu X. 1α,25-Dihydroxyvitamin D3 Promotes Osseointegration of Titanium Implant Via Downregulating Ages/Rage Pathway in T2dm. Endocr Connect (2018) 7(11):1186–95. doi: 10.1530/ec-18-0241
57. Bentes CM, Costa PB, Resende M, Netto C, Dias I, da Silveira ALB, et al. Effects of 12 Months of Vitamin D Supplementation on Physical Fitness Levels in Postmenopausal Women With Type 2 Diabetes. J Funct Morphol Kinesiol (2021) 6(4):87. doi: 10.3390/jfmk6040087
58. Nomura S, Kitami A, Takao-Kawabata R, Takakura A, Nakatsugawa M, Kono R, et al. Teriparatide Improves Bone and Lipid Metabolism in a Male Rat Model of Type 2 Diabetes Mellitus. Endocrinology (2019) 160(10):2339–52. doi: 10.1210/en.2019-00239
59. Lee YS, Gupta R, Kwon JT, Cho DC, Seo YJ, Seu SY, et al. Effect of a Bisphosphonate and Selective Estrogen Receptor Modulator on Bone Remodeling in Streptozotocin-Induced Diabetes and Ovariectomized Rat Model. Spine J Off J North Am Spine Soc (2018) 18(10):1877–87. doi: 10.1016/j.spinee.2018.05.020
60. Cui M, Yu LZ, Zhang N, Wang LJ, Sun J, Cong J. Zoledronic Acid Improves Bone Quality in the Streptozotocin-Induced Diabetes Rat Through Affecting the Expression of the Osteoblast-Regulating Transcription Factors. Exp Clin Endocrinol Diabetes Off journal German Soc Endocrinol German Diabetes Assoc (2019) 127(1):68–75. doi: 10.1055/s-0042-105282
61. Hamann C, Rauner M, Höhna Y, Bernhardt R, Mettelsiefen J, Goettsch C, et al. Sclerostin Antibody Treatment Improves Bone Mass, Bone Strength, and Bone Defect Regeneration in Rats With Type 2 Diabetes Mellitus. J Bone Mineral Res Off J Am Soc Bone Mineral Res (2013) 28(3):627–38. doi: 10.1002/jbmr.1803
62. Ferrari S, Eastell R, Napoli N, Schwartz A, Hofbauer LC, Chines A, et al. Denosumab in Postmenopausal Women With Osteoporosis and Diabetes: Subgroup Analysis of Freedom and Freedom Extension. Bone (2020) 134:115268. doi: 10.1016/j.bone.2020.115268
63. Viggers R, Al-Mashhadi Z, Starup-Linde J, Vestergaard P. The Efficacy of Alendronate Versus Denosumab on Major Osteoporotic Fracture Risk in Elderly Patients With Diabetes Mellitus: A Danish Retrospective Cohort Study. Front Endocrinol (Lausanne) (2021) 12:826997. doi: 10.3389/fendo.2021.826997
64. Yoshii T, Yamada M, Minami T, Tsunoda T, Sasaki M, Kondo Y, et al. The Effects of Bazedoxifene on Bone, Glucose, and Lipid Metabolism in Postmenopausal Women With Type 2 Diabetes: An Exploratory Pilot Study. J Clin Med Res (2015) 7(10):762–9. doi: 10.14740/jocmr2278w
65. Mori H, Okada Y, Kishikawa H, Inokuchi N, Sugimoto H, Tanaka Y. Effects of Raloxifene on Lipid and Bone Metabolism in Postmenopausal Women With Type 2 Diabetes. J Bone Mineral Metab (2013) 31(1):89–95. doi: 10.1007/s00774-012-0379-8
66. Gomes PS, Resende M, Fernandes MH. Doxycycline Restores the Impaired Osteogenic Commitment of Diabetic-Derived Bone Marrow Mesenchymal Stromal Cells by Increasing the Canonical Wnt Signaling. Mol Cell Endocrinol (2020) 518:110975. doi: 10.1016/j.mce.2020.110975
67. Bortolin RH, Abreu B, Ururahy MAG, de Souza KSC, Bezerra JF, Loureiro MB, et al. Protection Against T1dm-Induced Bone Loss by Zinc Supplementation: Biomechanical, Histomorphometric, and Molecular Analyses in Stz-Induced Diabetic Rats. PloS One (2017) 10(5):e0125349. doi: 10.1371/journal.pone.0125349
68. Shanshan Q, Jia H, Hongxing Z, Chen C, Hai J, Shiqiang L. Zinc Supplementation Increased Bone Mineral Density, Improves Bone Histomorphology, and Prevents Bone Loss in Diabetic Rat. Biol Trace Element Res (2020) 194(2):493–501. doi: 10.1007/s12011-019-01810-7
69. Maddaloni E, D'Eon S, Hastings S, Tinsley LJ, Napoli N, Khamaisi M, et al. Bone Health in Subjects With Type 1 Diabetes for More Than 50 Years. Acta Diabetol (2017) 54(5):479–88. doi: 10.1007/s00592-017-0973-2
70. Vilaca T, Paggiosi M, Walsh JS, Selvarajah D, Eastell R. The Effects of Type 1 Diabetes and Diabetic Peripheral Neuropathy on the Musculoskeletal System: A Case-Control Study. J Bone Miner Res (2021) 36(6):1048–59. doi: 10.1002/jbmr.4271
71. Shanbhogue VV, Hansen S, Frost M, Jørgensen NR, Hermann AP, Henriksen JE, et al. Bone Geometry, Volumetric Density, Microarchitecture, and Estimated Bone Strength Assessed by Hr-Pqct in Adult Patients With Type 1 Diabetes Mellitus. J Bone Miner Res (2015) 30(12):2188–99. doi: 10.1002/jbmr.2573
72. Hofbauer LC, Busse B, Eastell R, Ferrari S, Frost M, Müller R, et al. Bone Fragility in Diabetes: Novel Concepts and Clinical Implications. Lancet Diabetes Endocrinol (2022) 10(3):207–20. doi: 10.1016/s2213-8587(21)00347-8
73. Zoch ML, Clemens TL, Riddle RC. New Insights Into the Biology of Osteocalcin. Bone (2016) 82:42–9. doi: 10.1016/j.bone.2015.05.046
74. Liu Z, Yang J. Uncarboxylated Osteocalcin Promotes Osteogenic Differentiation of Mouse Bone Marrow-Derived Mesenchymal Stem Cells by Activating the Erk-Smad/B-Catenin Signalling Pathways. Cell Biochem Funct (2020) 38(1):87–96. doi: 10.1002/cbf.3457
75. Gong F, Gao L, Ma L, Li G, Yang J. Uncarboxylated Osteocalcin Alleviates the Inhibitory Effect of High Glucose on Osteogenic Differentiation of Mouse Bone Marrow-Derived Mesenchymal Stem Cells by Regulating Tp63. BMC Mol Cell Biol (2021) 22(1):24. doi: 10.1186/s12860-021-00365-7
76. Naot D, Musson DS, Cornish J. The Activity of Peptides of the Calcitonin Family in Bone. Physiol Rev (2019) 99(1):781–805. doi: 10.1152/physrev.00066.2017
77. Li H, Bian Y, Zhang N, Guo J, Wang C, Lau WB, et al. Intermedin Protects Against Myocardial Ischemia-Reperfusion Injury in Diabetic Rats. Cardiovasc Diabetol (2013) 12:91. doi: 10.1186/1475-2840-12-91
78. Wang F, Kong L, Wang W, Shi L, Wang M, Chai Y, et al. Adrenomedullin 2 Improves Bone Regeneration in Type 1 Diabetic Rats by Restoring Imbalanced Macrophage Polarization and Impaired Osteogenesis. Stem Cell Res Ther (2021) 12(1):288. doi: 10.1186/s13287-021-02368-9
79. Du Z, Wang L, Zhao Y, Cao J, Wang T, Liu P, et al. Sympathetic Denervation-Induced Msc Mobilization in Distraction Osteogenesis Associates With Inhibition of Msc Migration and Osteogenesis by Norepinephrine/Adrb3. PloS One (2014) 9(8):e105976. doi: 10.1371/journal.pone.0105976
80. Kong Y, Cheng L, Ma L, Li H, Cheng B, Zhao Y. Norepinephrine Protects Against Apoptosis of Mesenchymal Stem Cells Induced by High Glucose. J Cell Physiol (2019) 234(11):20801–15. doi: 10.1002/jcp.28686
81. Di Bernardo G, Galderisi U, Fiorito C, Squillaro T, Cito L, Cipollaro M, et al. Dual Role of Parathyroid Hormone in Endothelial Progenitor Cells and Marrow Stromal Mesenchymal Stem Cells. J Cell Physiol (2010) 222(2):474–80. doi: 10.1002/jcp.21976
82. Fan Y, Hanai JI, Le PT, Bi R, Maridas D, DeMambro V, et al. Parathyroid Hormone Directs Bone Marrow Mesenchymal Cell Fate. Cell Metab (2017) 25(3):661–72. doi: 10.1016/j.cmet.2017.01.001
83. Wang Y, Huang L, Qin Z, Yuan H, Li B, Pan Y, et al. Parathyroid Hormone Ameliorates Osteogenesis of Human Bone Marrow Mesenchymal Stem Cells Against Glucolipotoxicity Through P38 Mapk Signaling. IUBMB Life (2020) 73(1):213–22. doi: 10.1002/iub.2420
84. Alder KD, White AH, Chung YH, Lee I, Back J, Kwon HK, et al. Systemic Parathyroid Hormone Enhances Fracture Healing in Multiple Murine Models of Type 2 Diabetes Mellitus. JBMR Plus (2020) 4(5):e10359. doi: 10.1002/jbm4.10359
85. Greenspan SL, Bone HG, Ettinger MP, Hanley DA, Lindsay R, Zanchetta JR, et al. Effect of Recombinant Human Parathyroid Hormone (1-84) on Vertebral Fracture and Bone Mineral Density in Postmenopausal Women With Osteoporosis: A Randomized Trial. Ann Intern Med (2007) 146(5):326–39. doi: 10.7326/0003-4819-146-5-200703060-00005
86. Yamauchi T, Kadowaki T. Adiponectin Receptor as a Key Player in Healthy Longevity and Obesity-Related Diseases. Cell Metab (2013) 17(2):185–96. doi: 10.1016/j.cmet.2013.01.001
87. Khan MP, Singh AK, Joharapurkar AA, Yadav M, Shree S, Kumar H, et al. Pathophysiological Mechanism of Bone Loss in Type 2 Diabetes Involves Inverse Regulation of Osteoblast Function by Pgc-1α and Skeletal Muscle Atrogenes: Adipor1 as a Potential Target for Reversing Diabetes-Induced Osteopenia. Diabetes (2015) 64(7):2609–23. doi: 10.2337/db14-1611
88. Hu XF, Wang L, Lu YZ, Xiang G, Wu ZX, Yan YB, et al. Adiponectin Improves the Osteointegration of Titanium Implant Under Diabetic Conditions by Reversing Mitochondrial Dysfunction Via the Ampk Pathway in Vivo and in Vitro. Acta Biomater (2017) 61:233–48. doi: 10.1016/j.actbio.2017.06.020
89. Zhang Y, Huang NQ, Yan F, Jin H, Zhou SY, Shi JS, et al. Diabetes Mellitus and Alzheimer's Disease: Gsk-3β as a Potential Link. Behav Brain Res (2018) 339:57–65. doi: 10.1016/j.bbr.2017.11.015
90. Forde JE, Dale TC. Glycogen Synthase Kinase 3: A Key Regulator of Cellular Fate. Cell Mol Life Sci (2007) 64(15):1930–44. doi: 10.1007/s00018-007-7045-7
91. Zhang B, Liu N, Shi H, Wu H, Gao Y, He H, et al. High Glucose Microenvironments Inhibit the Proliferation and Migration of Bone Mesenchymal Stem Cells by Activating Gsk3β. J Bone Miner Metab (2016) 34(2):140–50. doi: 10.1007/s00774-015-0662-6
92. Li Z, Zhao H, Chu S, Liu X, Qu X, Li J, et al. Mir-124-3p Promotes Bmsc Osteogenesis Via Suppressing the Gsk-3β/B-Catenin Signaling Pathway in Diabetic Osteoporosis Rats. In Vitro Cell Dev Biol Anim (2020) 56(9):723–34. doi: 10.1007/s11626-020-00502-0
93. Chen Y, Chen L, Huang R, Yang W, Chen S, Lin K, et al. Investigation for Gsk3β Expression in Diabetic Osteoporosis and Negative Osteogenic Effects of Gsk3β on Bone Marrow Mesenchymal Stem Cells Under a High Glucose Microenvironment. Biochem Biophys Res Commun (2021) 534:727–33. doi: 10.1016/j.bbrc.2020.11.010
94. Janich P, Pascual G, Merlos-Suárez A, Batlle E, Ripperger J, Albrecht U, et al. The Circadian Molecular Clock Creates Epidermal Stem Cell Heterogeneity. Nature (2011) 480(7376):209–14. doi: 10.1038/nature10649
95. Li X, Liu N, Gu B, Hu W, Li Y, Guo B, et al. Bmal1 Regulates Balance of Osteogenic-Osteoclastic Function of Bone Marrow Mesenchymal Stem Cells in Type 2 Diabetes Mellitus Through the Nf-Kb Pathway. Mol Biol Rep (2018) 45(6):1691–704. doi: 10.1007/s11033-018-4312-7
96. Xing Q, Feng J, Zhang X. Semaphorin3b Promotes Proliferation and Osteogenic Differentiation of Bone Marrow Mesenchymal Stem Cells in a High-Glucose Microenvironment. Stem Cells Int (2021) 2021:6637176. doi: 10.1155/2021/6637176
97. Jin M, Song S, Guo L, Jiang T, Lin ZY. Increased Serum Gdf11 Concentration Is Associated With a High Prevalence of Osteoporosis in Elderly Native Chinese Women. Clin Exp Pharmacol Physiol (2016) 43(11):1145–7. doi: 10.1111/1440-1681.12651
98. Wang S, Wang L, Shi S, Wang X, He C, Yuan L, et al. Inhibition of Gdf11 Could Promote Bone Healing in the Tooth Extraction Socket and Facilitate Mesenchymal Stem Cell Osteogenic Differentiation in T2dm Pigs. J Periodontol (2020) 91(12):1645–52. doi: 10.1002/jper.20-0011
99. Chen D, Zhao M, Mundy GR. Bone Morphogenetic Proteins. Growth Factors (2004) 22(4):233–41. doi: 10.1080/08977190412331279890
100. Li B, Wang H, Qiu G, Su X, Wu Z. Synergistic Effects of Vascular Endothelial Growth Factor on Bone Morphogenetic Proteins Induced Bone Formation in Vivo: Influencing Factors and Future Research Directions. BioMed Res Int (2016) 2016:2869572. doi: 10.1155/2016/2869572
101. Wang JF, Lee MS, Tsai TL, Leiferman EM, Trask DJ, Squire MW, et al. Bone Morphogenetic Protein-6 Attenuates Type 1 Diabetes Mellitus-Associated Bone Loss. Stem Cells Transl Med (2019) 8(6):522–34. doi: 10.1002/sctm.18-0150
102. Kempen DH, Lu L, Heijink A, Hefferan TE, Creemers LB, Maran A, et al. Effect of Local Sequential Vegf and Bmp-2 Delivery on Ectopic and Orthotopic Bone Regeneration. Biomaterials (2009) 30(14):2816–25. doi: 10.1016/j.biomaterials.2009.01.031
103. Fei Y, Xiao L, Doetschman T, Coffin DJ, Hurley MM. Fibroblast Growth Factor 2 Stimulation of Osteoblast Differentiation and Bone Formation Is Mediated by Modulation of the Wnt Signaling Pathway. J Biol Chem (2011) 286(47):40575–83. doi: 10.1074/jbc.M111.274910
104. Bai Y, Li P, Yin G, Huang Z, Liao X, Chen X, et al. Bmp-2, Vegf and Bfgf Synergistically Promote the Osteogenic Differentiation of Rat Bone Marrow-Derived Mesenchymal Stem Cells. Biotechnol Lett (2013) 35(3):301–8. doi: 10.1007/s10529-012-1084-3
105. Park J, Yan G, Kwon KC, Liu M, Gonnella PA, Yang S, et al. Oral Delivery of Novel Human Igf-1 Bioencapsulated in Lettuce Cells Promotes Musculoskeletal Cell Proliferation, Differentiation and Diabetic Fracture Healing. Biomaterials (2020) 233:119591. doi: 10.1016/j.biomaterials.2019.119591
106. Zhao YP, Tian QY, Frenkel S, Liu CJ. The Promotion of Bone Healing by Progranulin, a Downstream Molecule of Bmp-2, Through Interacting With Tnf/Tnfr Signaling. Biomaterials (2013) 34(27):6412–21. doi: 10.1016/j.biomaterials.2013.05.030
107. Ding Y, Wei J, Hettinghouse A, Li G, Li X, Einhorn TA, et al. Progranulin Promotes Bone Fracture Healing Via Tnfr Pathways in Mice With Type 2 Diabetes Mellitus. Ann N Y Acad Sci (2021) 1490(1):77–89. doi: 10.1111/nyas.14568
108. Wei J, Zhang L, Ding Y, Liu R, Guo Y, Hettinghouse A, et al. Progranulin Promotes Diabetic Fracture Healing in Mice With Type 1 Diabetes. Ann N Y Acad Sci (2020) 1460(1):43–56. doi: 10.1111/nyas.14208
109. Giannoudis PV, Einhorn TA, Marsh D. Fracture Healing: The Diamond Concept. Injury (2007) 38(Suppl 4):S3–6. doi: 10.1016/s0020-1383(08)70003-2
110. Sun X, Ma Z, Zhao X, Jin W, Zhang C, Ma J, et al. Three-Dimensional Bioprinting of Multicell-Laden Scaffolds Containing Bone Morphogenic Protein-4 for Promoting M2 Macrophage Polarization and Accelerating Bone Defect Repair in Diabetes Mellitus. Bioact Mater (2021) 6(3):757–69. doi: 10.1016/j.bioactmat.2020.08.030
111. Li J, Wei J, Li A, Liu H, Sun J. Qiao H. A Dual Peptide Sustained-Release System Based on Nanohydroxyapatite/Polyamide 66 Scaffold for Synergistic-Enhancing Diabetic Rats' Fracture Healing in Osteogenesis and Angiogenesis. Front Bioeng Biotechnol (2021) 9:657699. doi: 10.3389/fbioe.2021.657699
112. Winn SR, Hu Y, Sfeir C, Hollinger JO. Gene Therapy Approaches for Modulating Bone Regeneration. Adv Drug Deliv Rev (2000) 42(1-2):121–38. doi: 10.1016/s0169-409x(00)00057-0
113. Khorsand B, Acri TM, Do AV, Femino JE, Petersen E, Fredericks DC, et al. A Multi-Functional Implant Induces Bone Formation in a Diabetic Model. Adv Healthc Mater (2020) 9(18):e2000770. doi: 10.1002/adhm.202000770
114. Pegtel DM, Gould SJ. Exosomes. Annu Rev Biochem (2019) 88:487–514. doi: 10.1146/annurev-biochem-013118-111902
115. Reithmair M, Buschmann D, Märte M, Kirchner B, Hagl D, Kaufmann I, et al. Cellular and Extracellular Mirnas Are Blood-Compartment-Specific Diagnostic Targets in Sepsis. J Cell Mol Med (2017) 21(10):2403–11. doi: 10.1111/jcmm.13162
116. Zhu Y, Jia Y, Wang Y, Xu J, Chai Y. Impaired Bone Regenerative Effect of Exosomes Derived From Bone Marrow Mesenchymal Stem Cells in Type 1 Diabetes. Stem Cells Transl Med (2019) 8(6):593–605. doi: 10.1002/sctm.18-0199
117. Zhang L, Wang Q, Su H, Cheng J. Exosomes From Adipose Derived Mesenchymal Stem Cells Alleviate Diabetic Osteoporosis in Rats Through Suppressing Nlrp3 Inflammasome Activation in Osteoclasts. J Biosci Bioeng (2021) 131(6):671–8. doi: 10.1016/j.jbiosc.2021.02.007
118. Gennari L, Bianciardi S, Merlotti D. Micrornas in Bone Diseases. Osteoporos Int (2017) 28(4):1191–213. doi: 10.1007/s00198-016-3847-5
119. Li J, Guo Y, Chen YY, Liu Q, Chen Y, Tan L, et al. Mir-124-3p Increases in High Glucose Induced Osteocyte-Derived Exosomes and Regulates Galectin-3 Expression: A Possible Mechanism in Bone Remodeling Alteration in Diabetic Periodontitis. FASEB J (2020) 34(11):14234–49. doi: 10.1096/fj.202000970RR
120. Zhang D, Wu Y, Li Z, Chen H, Huang S, Jian C, et al. Mir-144-5p, an Exosomal Mirna From Bone Marrow-Derived Macrophage in Type 2 Diabetes, Impairs Bone Fracture Healing Via Targeting Smad1. J Nanobiotechnol (2021) 19(1):226. doi: 10.1186/s12951-021-00964-8
121. Sunkari VG, Lind F, Botusan IR, Kashif A, Liu ZJ, Ylä-Herttuala S, et al. Hyperbaric Oxygen Therapy Activates Hypoxia-Inducible Factor 1 (Hif-1), Which Contributes to Improved Wound Healing in Diabetic Mice. Wound Repair Regener (2015) 23(1):98–103. doi: 10.1111/wrr.12253
122. Limirio P, da Rocha Junior HA, Morais RB, Hiraki KRN, Balbi APC, Soares PBF, et al. Influence of Hyperbaric Oxygen on Biomechanics and Structural Bone Matrix in Type 1 Diabetes Mellitus Rats. PloS One (2018) 13(2):e0191694. doi: 10.1371/journal.pone.0191694
123. Oliveira PA, Oliveira AM, Pablos AB, Costa FO, Silva GA, Santos JN, et al. Influence of Hyperbaric Oxygen Therapy on Peri-Implant Bone Healing in Rats With Alloxan-Induced Diabetes. J Clin Periodontol (2012) 39(9):879–86. doi: 10.1111/j.1600-051X.2012.01922.x
124. Altug HA, Tatli U, Coskun AT, Erdogan Ö, Özkan A, Sencimen M, et al. Effects of Hyperbaric Oxygen Treatment on Implant Osseointegration in Experimental Diabetes Mellitus. J Appl Oral Sci (2018) 26:e20180083. doi: 10.1590/1678-7757-2018-0083
125. Harrison A, Alt V. Low-Intensity Pulsed Ultrasound (Lipus) for Stimulation of Bone Healing - a Narrative Review. Injury (2021) 52(Suppl 2):S91–s6. doi: 10.1016/j.injury.2021.05.002
126. Kusuyama J, Seong C, Makarewicz NS, Ohnishi T, Shima K, Semba I, et al. Low Intensity Pulsed Ultrasound (Lipus) Maintains Osteogenic Potency by the Increased Expression and Stability of Nanog Through Spleen Tyrosine Kinase (Syk) Activation. Cell Signal (2019) 62:109345. doi: 10.1016/j.cellsig.2019.109345
127. Coords M, Breitbart E, Paglia D, Kappy N, Gandhi A, Cottrell J, et al. The Effects of Low-Intensity Pulsed Ultrasound Upon Diabetic Fracture Healing. J Orthop Res (2011) 29(2):181–8. doi: 10.1002/jor.21223
128. Alshihah N, Alhadlaq A, El-Bialy T, Aldahmash A, Bello IO. The Effect of Low Intensity Pulsed Ultrasound on Dentoalveolar Structures During Orthodontic Force Application in Diabetic Ex-Vivo Model. Arch Oral Biol (2020) 119:104883. doi: 10.1016/j.archoralbio.2020.104883
129. Ataroalsadat M, Sara R, Mohammadamin A, Abdollah A, Kamran GS, Fatemehalsadat R, et al. Evaluation of the Effects of Photobiomodulation on Bone Healing in Healthy and Streptozotocin-Induced Diabetes in Rats. Photomed Laser Surg (2017) 35(10):537–45. doi: 10.1089/pho.2016.4224
130. Ataroalsadat M, Reza A, Mohammadamin A, Kamran GS, Ali J, Mohammad B. Evaluation of the Effects of Photobiomodulation on Biomechanical Properties and Hounsfield Unit of Partial Osteotomy Healing in an Experimental Rat Model of Type I Diabetes and Osteoporosis. Photomed Laser Surg (2017) 35(10):520–9. doi: 10.1089/pho.2016.4191
131. Mostafavinia A, Masteri Farahani R, Abdollahifar MA, Ghatrehsamani M, Ghoreishi SK, Hajihossainlou B, et al. Evaluation of the Effects of Photobiomodulation on Partial Osteotomy in Streptozotocin-Induced Diabetes in Rats. Photomed Laser Surg (2018) 36(8):406–14. doi: 10.1089/pho.2018.4438
132. Bueno NP, Copete IN, Lopes HB, Arany PR, Marques MM, Ferraz EP. Recovering the Osteoblastic Differentiation Potential of Mesenchymal Stem Cells Derived From Diabetic Rats by Photobiomodulation Therapy. J Biophotonics (2021) 14(3):e202000393. doi: 10.1002/jbio.202000393
133. Zare F, Bayat M, Aliaghaei A, Piryaei A. Photobiomodulation Therapy Compensate the Impairments of Diabetic Bone Marrow Mesenchymal Stem Cells. Lasers Med Sci (2020) 35(3):547–56. doi: 10.1007/s10103-019-02844-y
134. AlGhamdi KM, Kumar A, Moussa NA. Low-Level Laser Therapy: A Useful Technique for Enhancing the Proliferation of Various Cultured Cells. Lasers Med Sci (2012) 27(1):237–49. doi: 10.1007/s10103-011-0885-2
135. Saygun I, Nizam N, Ural AU, Serdar MA, Avcu F, Tözüm TF. Low-Level Laser Irradiation Affects the Release of Basic Fibroblast Growth Factor (Bfgf), Insulin-Like Growth Factor-I (Igf-I), and Receptor of Igf-I (Igfbp3) From Osteoblasts. Photomed Laser Surg (2012) 30(3):149–54. doi: 10.1089/pho.2011.3079
136. Nissan J, Assif D, Gross MD, Yaffe A, Binderman I. Effect of Low Intensity Laser Irradiation on Surgically Created Bony Defects in Rats. J Oral Rehabil (2006) 33(8):619–924. doi: 10.1111/j.1365-2842.2006.01601.x
137. Khadra M, Kasem N, Haanaes HR, Ellingsen JE, Lyngstadaas SP. Enhancement of Bone Formation in Rat Calvarial Bone Defects Using Low-Level Laser Therapy. Oral Surg Oral Med Oral Pathol Oral Radiol Endod (2004) 97(6):693–700. doi: 10.1016/j.tripleo.2003.11.008
138. Diker N, Aytac D, Helvacioglu F, Dagdelen C, Oguz Y. Evaluation of the Effects of Low-Level Laser Therapy on Diabetic Bone Healing. J Craniofac Surg (2019) 30(7):1994–8. doi: 10.1097/SCS.0000000000005654
139. Yildirimturk S, Sirin Y, Tekkesin MS, Gurler G, Firat D. The Effects of Low-Level Laser Therapy on the Healing of Bone Defects in Streptozotocin-Induced Diabetic Rats: A Histological and Morphometric Evaluation. J Cosmet Laser Ther (2017) 19(7):397–403. doi: 10.1080/14764172.2017.1341048
140. Diamantino AG, Nicolau RA, Costa DR, Almeida A, Mato D, de Oliveira MA, et al. Effect of Non-Coherent Infrared Light (Led, Λ 945 ± 20 Nm) on Bone Repair in Diabetic Rats—Morphometric and Spectral Analyses. Lasers Med Sci (2017) 32(5):1041–9. doi: 10.1007/s10103-017-2205-y
141. Tabrah F, Hoffmeier M, Gilbert F Jr., Batkin S, Bassett CA. Bone Density Changes in Osteoporosis-Prone Women Exposed to Pulsed Electromagnetic Fields (Pemfs). J Bone Mineral Res Off J Am Soc Bone Mineral Res (1990) 5(5):437–42. doi: 10.1002/jbmr.5650050504
142. Garland DE, Adkins RH, Matsuno NN, Stewart CA. The Effect of Pulsed Electromagnetic Fields on Osteoporosis at the Knee in Individuals With Spinal Cord Injury. J Spinal Cord Med (1999) 22(4):239–45. doi: 10.1080/10790268.1999.11719576
143. Shao X, Yang Y, Tan Z, Ding Y, Luo E, Jing D, et al. Amelioration of Bone Fragility by Pulsed Electromagnetic Fields in Type 2 Diabetic Kk-Ay Mice Involving Wnt/B-Catenin Signaling. Am J Physiol Endocrinol Metab (2021) 320(5):E951–e66. doi: 10.1152/ajpendo.00655.2020
144. Cai J, Li W, Sun T, Li X, Luo E, Jing D. Pulsed Electromagnetic Fields Preserve Bone Architecture and Mechanical Properties and Stimulate Porous Implant Osseointegration by Promoting Bone Anabolism in Type 1 Diabetic Rabbits. Osteoporos Int (2018) 29(5):1177–91. doi: 10.1007/s00198-018-4392-1
145. Zhou J, Li X, Liao Y, Feng W, Fu C, Guo X. Pulsed Electromagnetic Fields Inhibit Bone Loss in Streptozotocin-Induced Diabetic Rats. Endocrine (2015) 49(1):258–66. doi: 10.1007/s12020-014-0439-z
146. Nordklint AK, Almdal TP, Vestergaard P, Lundby-Christensen L, Jørgensen NR, Boesgaard TW, et al. Effect of Metformin Vs. Placebo in Combination With Insulin Analogues on Bone Markers P1np and Ctx in Patients With Type 2 Diabetes Mellitus. Calcif Tissue Int (2020) 107(2):160–9. doi: 10.1007/s00223-020-00711-5
147. Hygum K, Harsløf T, Jørgensen NR, Rungby J, Pedersen SB, Langdahl BL. Bone Resorption Is Unchanged by Liraglutide in Type 2 Diabetes Patients: A Randomised Controlled Trial. Bone (2020) 132:115197. doi: 10.1016/j.bone.2019.115197
148. Cai TT, Li HQ, Jiang LL, Wang HY, Luo MH, Su XF, et al. Effects of Glp-1 Receptor Agonists on Bone Mineral Density in Patients With Type 2 Diabetes Mellitus: A 52-Week Clinical Study. BioMed Res Int (2021) 2021:3361309. doi: 10.1155/2021/3361309
Keywords: diabetic bone disease, bone healing, hyperglycemia, mesenchymal stem cells, biomedical cues, diabetic drug
Citation: Chen Y, Zhou Y, Lin J and Zhang S (2022) Challenges to Improve Bone Healing Under Diabetic Conditions. Front. Endocrinol. 13:861878. doi: 10.3389/fendo.2022.861878
Received: 25 January 2022; Accepted: 02 March 2022;
Published: 28 March 2022.
Edited by:
Melissa Orlandin Premaor, Federal University of Minas Gerais, BrazilReviewed by:
Akalpita Arvindekar, Shivaji University, IndiaCopyright © 2022 Chen, Zhou, Lin and Zhang. This is an open-access article distributed under the terms of the Creative Commons Attribution License (CC BY). The use, distribution or reproduction in other forums is permitted, provided the original author(s) and the copyright owner(s) are credited and that the original publication in this journal is cited, in accordance with accepted academic practice. No use, distribution or reproduction is permitted which does not comply with these terms.
*Correspondence: Jie Lin, ODQyMDQzNjJAcXEuY29t; Shiwen Zhang, c3cuemhhbmcyMDE4QHNjdS5lZHUuY24=
†These authors have contributed equally to this work and share first authorship
Disclaimer: All claims expressed in this article are solely those of the authors and do not necessarily represent those of their affiliated organizations, or those of the publisher, the editors and the reviewers. Any product that may be evaluated in this article or claim that may be made by its manufacturer is not guaranteed or endorsed by the publisher.
Research integrity at Frontiers
Learn more about the work of our research integrity team to safeguard the quality of each article we publish.