- 1Department of Endocrinology, Third Xiangya Hospital, Central South University, Changsha, China
- 2Clinical Medicine Eight-Year Program, Xiangya School of Medicine, Central South University, Changsha, China
- 3The Research Centre for Preclinical Medicine, Southwest Medical University, Luzhou, China
- 4Department of Anatomy and Neurobiology, School of Basic Medical Science, Central South University, Changsha, China
- 5Department of Burn and Plastic Surgery, Guangzhou First People’s Hospital, School of Medicine, South China University of Technology, Guangzhou, China
- 6Hunan Key Laboratory of Ophthalmology, Changsha, China
- 7Key Laboratory of Emergency and Trauma, Ministry of Education, College of Emergency and Trauma, Hainan Medical University, Haikou, China
Type 1 diabetes mellitus (T1DM) is an autoimmune disease that attacks pancreatic β-cells, leading to the destruction of insulitis-related islet β-cells. Islet β-cell transplantation has been proven as a curative measure in T1DM. However, a logarithmic increase in the global population with diabetes, limited donor supply, and the need for lifelong immunosuppression restrict the widespread use of β-cell transplantation. Numerous therapeutic approaches have been taken to search for substitutes of β-cells, among which stem cell transplantation is one of the most promising alternatives. Stem cells have demonstrated the potential efficacy to treat T1DM by reconstitution of immunotolerance and preservation of islet β-cell function in recent research. cGMP-grade stem cell products have been used in human clinical trials, showing that stem cell transplantation has beneficial effects on T1DM, with no obvious adverse reactions. To better achieve remission of T1DM by stem cell transplantation, in this work, we explain the progression of stem cell transplantation such as mesenchymal stem cells (MSCs), human embryonic stem cells (hESCs), and bone marrow hematopoietic stem cells (BM-HSCs) to restore the immunotolerance and preserve the islet β-cell function of T1DM in recent years. This review article provides evidence of the clinical applications of stem cell therapy in the treatment of T1DM.
1 Introduction
Diabetes mellitus (DM) characterized by hyperglycemia, caused by insufficient insulin secretion or insulin resistance, is a group of chronic metabolic diseases. According to the International Diabetes Federation (IDF), the global adult diabetes population will exceed 537 million by 2021, and more than three-fourths of people with diabetes live in low- and middle-income countries, indicating that diabetes disproportionately affects the poor (http://www.diabetesatlas.org/). Diabetes is classified into four types: type 1 diabetes mellitus (T1DM), type 2 diabetes mellitus (T2DM), gestational diabetes mellitus (GDM), and monogenic diabetes mellitus (1–5). T1DM is an autoimmune disease, where autoreactive T cells attack pancreatic β-cells, leading to insulitis-related islet β-cell destruction, which results in an absolute lack of insulin secretion causing hyperglycemia, abnormal glucose metabolism, and lifelong dependence on exogenous insulin. The majority of T1DM patients have poor blood glucose control and large blood sugar fluctuation. Chronic hyperglycemia results in the development of serious complications associated with diabetes, such as microvascular and macrovascular complications, reducing the quality of life and causing a considerable economic burden on T1DM patients and the society (6). The incidence rate of T1DM is increasing every year around the world (7, 8). Although there is evidence that a combination of genetic susceptibility and environmental factors can increase the risk of immune disorder in T1DM patients, the exact etiology of the impaired immune system in T1DM is still unclear. More scientific efforts are needed to prevent β-cell loss and improve the quality of life in T1DM.
2 The Difficulties of Insulin Replacement and β-Cell Replacement in T1DM
At present, the treatment and preventive options for T1DM are limited, mainly through insulin replacement therapy. T1DM cannot be cured; patients must rely on exogenous insulin injections for the rest of their lives to maintain glycemic control. Lente and NPH insulin were the only effective methods for the treatment of T1DM in the past (9, 10). In recent years, novel approaches to insulin treatment, such as the introduction of glycosylated hemoglobin assays (HbA1c) and continuous glucose monitoring (CGM), have been used, and the effectiveness of basal/bolus therapy using portable continuous subcutaneous insulin infusion (CSII) pumps and closed-loop artificial pancreas system has been demonstrated. Artificial pancreas combining CGM with CSII pumps could automatically administer an appropriate insulin dose via a dosing algorithm. Some randomized controlled trials proved that the artificial pancreas system could efficiently adjust the glycemic index by automatically delivering exogenous insulin with dosing algorithms based on sensor glucose levels (11). However, the lag time of glycemia detected by CGM and the risk of hypoglycemia and infections limit the application of artificial pancreas, and some of the patients with unawareness of hypoglycemic events such as brittle type T1DM are not qualified to use the artificial pancreas (12, 13). Also, insulin replacement therapy can only supplement the missing insulin and cannot fundamentally restore the function of the pancreas. Although these achievements can better manage blood glucose and large blood sugar fluctuation in T1DM, they can hardly prevent the occurrence of a series of complications, including microvascular, macrovascular, and neuropathy complications (14, 15). As a result, many adjunctive therapies, such as dietary and weight management, nutrition therapy, physical activity and exercise, and some drugs used to treat T2DM, have been proposed to treat T1DM, which alleviate blood glucose fluctuation and reduce the lifetime risk of complications to some extent, but their effectiveness is limited. Therefore, it is very important to develop better technology and equipment for diagnosis and treatment options to prevent T1DM (16–18).
β-Cell replacement has also been proven as a curative measure in T1DM, which may be achieved through pancreas or islet transplantation in selective candidates (19). Pancreatic transplantation has the potential of re-establishing physiologic-regulated insulin production, obviously decreasing the risk of hypoglycemic unawareness and finally decreasing the longtime risk of mortality from severe hypoglycemic complications (20). Since 2000, β-cell replacement through intrahepatic isolated islet transplantation has proven efficacious, indicating that islet transplantation is also an important option in the treatment of T1DM (21). Compared with the artificial pancreas system, islet transplantation and pancreatic transplantation were the better options to relieve the symptom of T1DM patients with unawareness of hypoglycemic events such as brittle type T1DM for a long time (22). T1DM patients can be clinically alleviated through improved control of the levels of blood glucose and restored awareness of hypoglycemia, resulting in the prevention of several life-threatening complications associated with diabetes, such as diabetic foot, microvascular and macrovascular diseases, kidney failure, nerve damage, and blindness (23). During the process of pancreatic or islet transplantation, both the autoimmune and alloimmune systems are still major threats to increase the transplantation risk. Patients treated with cell replacement therapies require immunosuppressive drugs as life-long treatment, and in many cases, these drugs lead to toxicities and side effects that made the adoption of this treatment strategy limited to only the most severe disease cases, inhibiting the widespread adoption of pancreatic or islet transplantation therapies in T1DM (24).
Besides the immune problem, the logarithmic increase in the global population of people with diabetes, the limited donor supply, and the need for lifelong immunosuppression restrict its widespread use (25). Numerous therapeutic approaches have been reported to solve this problem, including the search for β-cell substitutes, porcine islet xenotransplantation, and stem cell transplantations, which present solutions to the donor shortage and may be the most likely alternatives (26, 27).
Although the artificial pancreas system and pancreatic transplantation in T1DM can normalize and improve glycemic control in T1DM, the application of artificial pancreas systems and pancreatic transplantation is still limited due to their shortage. To solve the problem, stem cell transplant is a promising new strategy for patients with T1DM. There are many advantages of stem cells in the treatment of T1DM: first of all, stem cells such as bone marrow-derived stem cells (MSCs) can easily be obtained from bone marrow, umbilical cord blood, adipose tissue, etc. compared with islet and pancreas; secondly, the pluripotent stem cells could differentiate into β-cells and increase the secretion of insulin; thirdly, stem cells can moderate the immunome effect by inhibiting T-cell proliferation and reduce the inflammatory response, which can protect β-cells from autoimmune attack; and finally, stem cells can secrete cytokines by paracrine effects to enhance the antioxidant and proliferation ability of cells, which can help improve the survival of β-cells. To better understand the constitution of immunotolerance and preservation of islet β-cell function, we reviewed the progression of stem cells in recent years and tried to provide support for the clinical applications of stem cell therapy in the treatment of T1DM, especially in the brittle type T1DM.
3 Stem Cell Transplantation Therapy for T1DM
Stem cells are undifferentiated cells capable of self-renewal, giving rise to virtually any tissue or organ (28–33). Stem cells can be grouped into four broad categories based on their origin: adult stem cells (ASCs), fetal stem cells (FSCs), embryonic stem cells (ESCs), and induced pluripotent stem cells (iPSCs). iPSCs and ESCs are pluripotent stem cells (PSCs), whereas ASCs are unipotent or oligopotent (34–36). PSCs, such as human-induced PSCs (iPSCs) and human embryonic stem cells (ESCs), offer a reproducible source of human cells at a very early developmental stage with the potential to form any cell type in the adult body (37–39). iPSCs, human cord blood-derived multipotent stem cells (CB-SCs), hematopoietic stem cells (HSCs), and MSCs were used for the preservation of β-cells by islet protection and regeneration, and another potent function of stem cells is the ability to re-establish peripheral tolerance toward β-cells through remodeling of the immune response as well as through inhibition of autoreactive T-cell function (40, 41). In general, stem cells can increase the mass of islets by the ability of differentiation to β-cells-like organoids, and reconstitute immunotolerance by inhibiting the immune response of T cell and Th1 cells through TGF-β and inflammatory pathways (Figure 1). As T1DM is featured as an autoimmune disease by activating immune cells to attack and destroy pancreatic β-cells, the immunomodulatory properties of stems cells and its potential ability of differentiation into insulin-producing cells should be considered when using stem cell therapy for T1DM treatment.
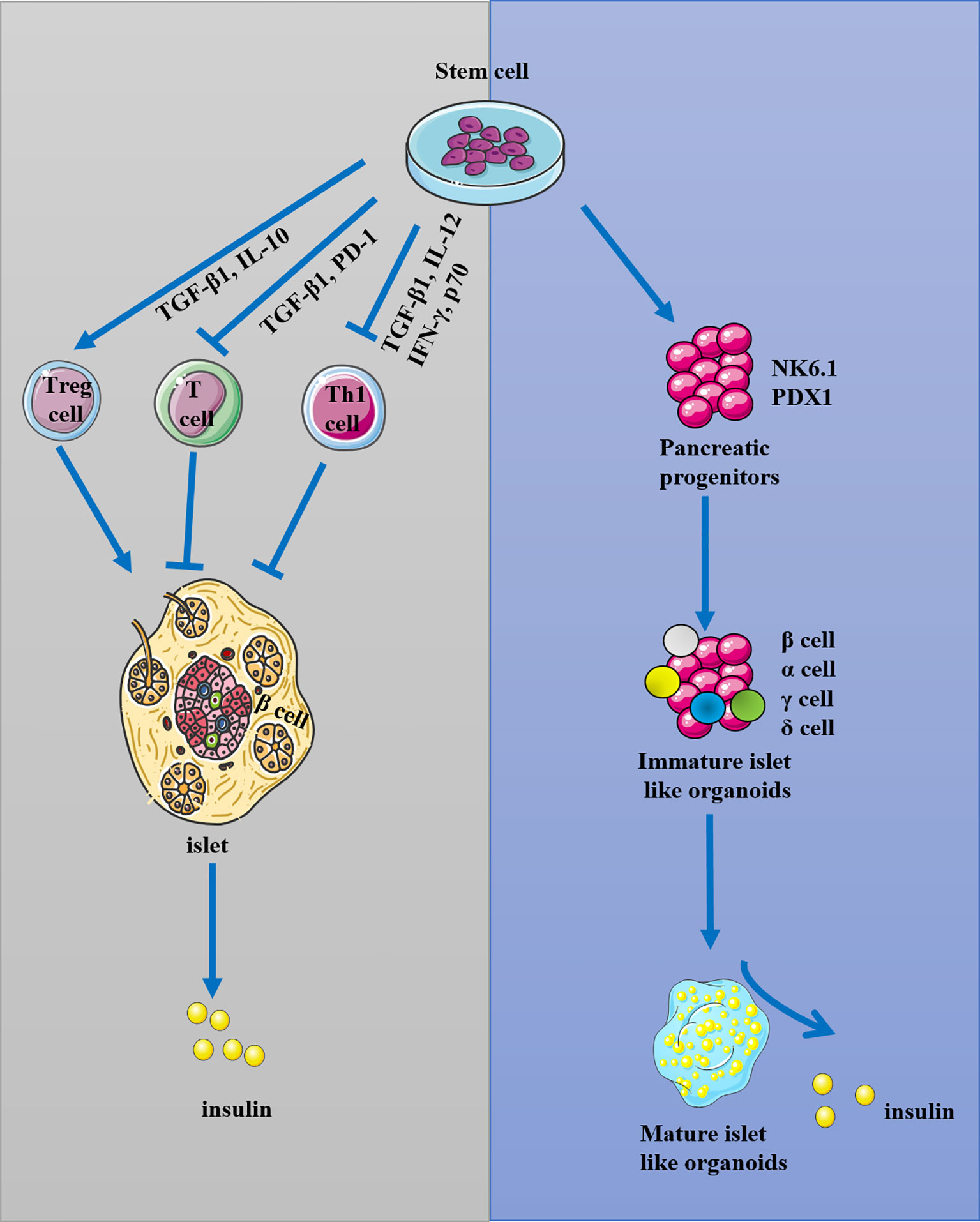
Figure 1 The possible mechanism of stem cells in the treatment of T1DM. Stem cells were used for the reconstitution of immunotolerance through activating T regulatory cells (Treg) and inhibiting T and Th1 cells, and they could also be used for the preservation and regeneration of β-cells.
3.1 MSC Transplantation in T1DM
MSCs are one of the best candidate cells used as cell therapy for T1DM. MSCs are fibroblast-like, multipotent stromal, non-hematopoietic cells that could easily be sourced from various tissues, including adipose tissue, bone marrow, and umbilical cord blood (42). MSCs rapidly undergo mesodermal lineage differentiation, such as adipocytes, myoblasts, cardiomyocytes, chondrocytes, and β-cell-like cells (43–45). The bone marrow and umbilical cord blood could be separated over a gradient of Percoll by density gradient centrifugation to collect the MNCs, and the MNCs were washed with PBS and transferred to a 100-mm culture dish to induce MSCs. The redundant tissues such as arteries and veins were removed from the adipose tissue, human umbilical cord, etc. The region of the remaining adipose or human umbilical cord tissues was diced into small fragments and seeded into a 100-mm culture dish to collect the MSCs. The induced MSCs were stored in liquid nitrogen and cultured for up to five passages for transplantation via intravenous injection. The characteristics of MSCs were defined by the International Society for Cell Therapy (ISCT) as follows: adherence to plastic; expression of the surface molecules CD73, CD90, and CD105 in the absence of CD34, CD45, HLA-DR, and CD14 or CD11b and CD79a or CD19; and the capacity for differentiation to adipocytes, osteoblasts, and chondroblasts in vitro (46). The potential of MSCs as a cell-based therapy in the treatment of immunologic disorders has been well established (47). MSCs can alter the microenvironment in tissues and promote existing β-cell survival and regeneration, resulting in increased β-cell mass and normal blood glucose recovery (48–51). Injection of bone marrow MSCs into diabetic mice can increase insulin levels and downregulate hyperglycemia; the exosomes derived from human umbilical cord stem cells (hUCMSCs) can enhance insulin sensitivity (52). Similarly, monotherapy with human umbilical cord MSCs reverses autoimmunity, promotes islet cell regeneration, and improves blood glucose control (53–55).
The allogeneic MSCs have been attempted in clinical trials, which can improve the level of insulin and C-peptide and reduce blood glucose. Although MSC xenotransplantation was not used in the clinics, several pieces of evidence showed that human-derived MSCs could alleviate the diabetic symptom through β-cell-like organoid differentiation and immunomodulation in NOD mice, rats, and monkeys, while far-red light, gene editing, and other modifications could enhance the function of MSCs in a T1DM animal model, indicating that the intervening and xenograft MSCs are the potential option for T1DM treatment.
3.1.1 Immunomodulatory Ability of MSCs
MSCs can protect β-cell, increase the secretion of insulin, and reduce glycemia in patients with T1DM by regulating the immune system. The application of MSCs in eliminating autoimmune diseases has been fully proven in an animal model, and MSCs have a wide range of regulatory effects on immune cells. Domouky et al. showed that MSCs could reduce hyperglycemia in diabetic rats on day 15 (56). The inhibition of T-cell proliferation in islets and the presence of increased Treg in T1DM were features of MSCs’ autoimmune properties (57). Shigemoto-Kuroda et al. developed T1DM mouse models for autoimmune diseases and discovered that MSCs could suppress type 1 helper T cell (Th1) development and delay the onset of T1DM in mice. CD4+ cells were found in significant numbers in the islets of mice treated with PBS, while fewer CD4+ cells were found in the islets of MSC-treated mice. The level of insulin in plasma was increased by MSC treatment, and there was a significant reduction in the production of IL-12, IFN-γ, p70, and tumor necrosis factor (TNF) (58). Bassi et al. isolated MSCs from epididymal fat tissue from 8-week-old male Balb/c mice and characterized through immunophenotyping its capacity to prevent the proliferation of CD4+ T cells (59, 60). Treatment of NOD mice with MSCs attenuated hyperglycemia of early-onset autoimmune diabetes and increased amylin levels, reflective of autoimmune diabetes improvement; reduced the amount of inflammatory cell infiltration, maintaining insulin expression in pancreatic islets by suppressing the Th1 immune response in the pancreas; and promoted the high expression of active TGF-β1 (60). Meanwhile, syngeneic MSCs were detected for a significantly longer period, albeit with diminishing persistence in immune-deficient mice model (61). In another study, van Megen et al. found that activation of MSCs can take up and process antigen and increase HLA-DR expression and immune inhibitory markers, while their metabolic profile was maintained without enhancing T-cell proliferation. MSCs can also enhance immunosuppressive capacity without stimulating alloreactive T cells (62). In an in-vitro study, Montanucci et al. provided preliminary evidence that immunoisolatory microcapsule-hHUCMS (CpS-hUCMS) may represent a functional biohybrid artificial system, where molecular products can induce effective immunomodulatory effects in vitro and in T1DM patients, making it possible to further clarify their therapeutic potential in humans (63). Montanucci et al. isolated and microencapsulated human umbilical cord Wharton jelly-derived mesenchymal stem cells (hUCMS) for xenograft (TX) in a spontaneous T1DM mouse model (NOD mice). At 10 days of TX, Treg cells did not increase, while at 216 days of TX, CD4+ CD25 high cells increased in terms of both percentage and number. Further research found that at 216 days of TX, only the mild T1DM NOD mice presented sustained and full alleviation of hyperglycemia, while no alleviation of hyperglycemia was observed in severe T1DM NODs. These findings suggested that the successful hUCMS therapy approach for the treatment of T1DM in NOD mice depended on the stage of the T1DM disease process, with severe T1DM NODs exhibiting a continuous decrease in residual β-cell mass (64). All these results provide encouraging first steps in the clinical translation of the use of preactivated MSCs as a cellular immune intervention therapy, which helps to treat inflammatory and autoimmune disorders, including T1DM.
3.1.2 Islet Protection and Regeneration Ability of MSCs
MSCs can increase the mass of islets and the survival of β-cells by differentiation to β-cell-like cells. MSCs rapidly undergo mesodermal lineage differentiation to β-cell-like cells, and the transdifferentiation of MSCs into insulin-producing cells was successfully attempted in vitro, with the pancreatic and duodenal homeobox 1 (PDX-1), the key marker that was present in the transdifferentiation of MSCs to insulin-producing cells (65). Chen et al. successfully differentiated MSCs into pancreatic islet β-cell-like cells by pre-inducing in L-DMEM with 10 mmol/L nicotinamide and 1 mmol/L β-mercaptoethanol for 24 h and re-inducing in serum-free H-DMEM with 10 mmol/L nicotinamide and 1 mmol/L mercaptoethanol for another 10 h. These induced islet β-cell-like cells with similar morphological characters to pancreatic islet cells and promoted the transcription, translation, and excretion of insulin, which could effectively control the level of blood glucose in diabetic rats (66). Similar results were reported by several other groups that islet-like clusters can be formatted in vitro by cultured MSCs given the appropriate procedure (67, 68). Human umbilical cord Wharton jelly cells (hUCWJCs) are a subtype of MSCs, which were transplanted into a T1DM mouse model with renal damage, and the therapeutic effect of transplantation was evaluated. It was found that hUCWJCs can promote the level of C-peptide and insulin in mice, which certified the potential of intraperitoneal injection of hUCWJCs and the ability of hUCWJCs to migrate to damaged tissues to enhance the secretion of insulin from non-pancreatic local cells (69).
3.1.3 MSC Transplantation in Clinical Trials
MSCs have been used in human clinical trials, showing that stem cell transplantation has beneficial effects on T1DM. In an open-label, non-randomized, parallel-armed prospective study, Lu et al. enrolled 53 participants including 33 adult-onset (≥18 years) and 20 juvenile-onset T1DM (ChiCTR2100045434). The results revealed that an intravenous dose of allogeneic UC-MSCs was safe in people with newly diagnosed T1DM at 12 months of follow-up, which probably led to better islet β-cell protection compared with standard treatment alone during the first year after diagnosis (70). Cai et al. proved that transplantation of UC-MSCs was safe and associated with moderate improvement of metabolic measures in patients with established T1DM too (NCT01374854) (50). Another clinical trial revealed that MSC injection through liver puncture could successfully decrease the levels of insulin, islet cells, and glutamic acid decarboxylase (GAD) antibody in two patients within 1 year, with a decreased concentration of blood glucose and HbA1c and increased concentration of C-peptide, indicating immune regulatory cell tolerance (71).
3.2 HSC Transplantation in T1DM
The conception of HSCs was generated in the 1950s with the discovery that intravenously injected bone marrow cells could rescue irradiated mice from lethality through re-establishing blood cell production (72). The ability to manage expansion and the characteristics of self-renewal of the hematopoietic compartment while maintaining the capacity for differentiation into HSCs were demonstrated (72, 73). Peripheral hematopoietic stem cells are mobilized with cyclophosphamide and granulocyte colony-stimulating factors. Leukapheresis using a continuous-flow blood cell separator was initiated when the rebounding CD34+ cells reached 10 cells/μl. Apheresis was continued daily until the number of harvested progenitor cells reached a minimum of 3.0 × 106 CD34+ cells/kg body weight. Unmanipulated peripheral blood stem cells were frozen in 10% dimethyl sulfoxide in a rate-controlled freezer and stored in the vapor phase of liquid nitrogen (74). Then, the collected cells were injected intravenously. HSCs have proven to be safe in human subjects and have been widely utilized as an effective treatment for hematological malignancies (75). Recently, HSCs have been used in T1DM for the suppressed function of the immune system response in both in-vitro and in-vivo studies.
3.2.1 Immunomodulatory Ability of HSCs
Immunomodulatory activity is the most important ability of HSCs in patients with T1DM. HSCs can inhibit the occurrence of T1DM (76–78). Patients with recent-onset T1DM have been triumphantly reverted to euglycemia by autologous hematopoietic stem and progenitor cell transplantation (AHSCT), and modulation of autologous hematopoietic stem and progenitor cells (HSPCs) with prostaglandins (PGs) in vitro enhances their immunoregulatory properties through increasing the expression of the immune checkpoint-signaling molecule PD-L1 (79). Wang et al. demonstrated a lower proportion of proliferating T conventional cells (Tcon) and a higher absolute number and percentage of Treg cells in pancreatic lymph nodes from resistant mice among the younger recipients compared to the rapid progressors among the older recipients, and older NOD mice progressed more rapidly to the end stage of diabetes (80). Although mixed chimerism with MHC-matched non-autoimmune donor bone marrow (BM) transplants did not prevent T1DM in NOD mice models, induction of either mixed or complete chimerism with MHC-mismatched BM transplants inhibited T1DM in the same mice (81). This limited the translational applications of HSCs to reshape the autoimmune response by myeloablative agents/approaches. The genetically modified HSCs were used to overcome the disadvantage. Ex-vivo genetic manipulation of NOD HSCs to encode proinsulin and transgenically target MHC class II could successfully prevent T1DM onset (78, 82). The increased CXCL12 (SDF-1) level in bone marrow-derived HSCs of NOD mice is considered to change the transport of HSCs and peripheral dendritic cells, which is conducive to the occurrence of T1DM (78, 83).
3.2.2 HSC Transplantation in Clinical Trials
D’Addio et al. enrolled 65 individuals with newly diagnosed T1DM in three independent clinical trials, where the patients transplanted with HSCs showed enhanced C-peptide levels at 6 months after treatment compared with baseline, and the immune system showed an overall stabilization in the remaining follow-up period (84). Gu et al. performed a parallel-assignment, phase-II prospective, non-randomized trial, in which 20 patients were treated only with insulin injections and 20 received autologous hematopoietic stem cell therapy (AHSCT). The results demonstrated the beneficial effects of AHSCT in patients with recent-onset T1DM by increasing the concentration of C-peptide and inducing insulin independence, and the safety and good tolerability of AHSCT compared with conventional intensive insulin therapy was also certified (77). Another clinical trial in Ning’s research also proved that AHSCT was safe without a reduction in the diversity of T-cell receptor (TCR) repertoires, and TCR repertoires tended to be more stable after AHSCT (85). The clinical trial data also showed significant direct correlations between HSPC levels and the coefficient of variation of glucose levels or time in hypoglycemia, which were weaker in patients with long-standing diabetes than in those with short-term diabetes (86). HSC transplantation improves glycated hemoglobin levels in a time-dependent manner (87).
3.3 ESC and iPSC Transplantation in T1DM
ESCs and iPSCs are PSCs that can regenerate the islet β-cells and immune cells through differentiating, which helps to increase the mass of β-cells. D’Amour et al. firstly proved that ESC-derived β-cells could be successfully generated through the in-vitro recapitulation of pancreatic islets and β-cell physiological development by stepwise application of specific factors (88, 89). iPSCs, reprogrammed from somatic cells, have a similar ability to differentiate and proliferate like ESCs. iPSCs collected from the umbilical cord at birth have the potential for self-renewable multipotency and can differentiate into various lineages such as islets (31, 59, 90). Hence, iPSCs provide a promising platform to produce insulin-secreting cells in vitro. However, the utilization of ESCs and iPSCs is less due to law restrictions in many countries, so there is little clinical research on ESCs and iPSCs.
3.3.1 Islet Protection and Regeneration Ability of ESCs and iPSCs
Transplantation of ESCs or iPSCs in T1DM can regenerate the islet β-cells and increase β-cell mass through differentiating to insulin-producing cells (IPCs), pancreatic progenitors, islet organoids, and interspecific pancreatic chimeras, which benefited the treatment of T1DM. Rezania et al. cultured iPSCs expressing key markers of mature β-cells such as insulin in vitro and obtained cells which have functional similarities to human islets; the iPSCs rapidly reversed hyperglycemia in streptozotocin (STZ)-induced diabetic mice through increasing the level of insulin and C-peptide when transplanted in vivo (91). iPSCs can be generated from the skin fibroblasts of T1DM patients. These iPSCs can differentiate into pancreatic cell lineages and generate T1DM SC β-cells, making autologous stem cell-derived pancreatic progeny transplantation for T1DM possible (92). Korytnikov and Nostro isolated hPSCs successfully in a lab and then transplanted them to mice models to monitor their developmental potential in vivo, and they found that mice transplanted with multipotent pancreatic progenitor cells can form all pancreatic lineages in vivo (93). Nadav et al. used single-cell RNA sequencing of differentiating β-cells and revealed that ESC differentiation toward the mature β-cell phenotype can be tracked at each stage through monitoring the expression of markers identifying each intermediate progenitor, such as the β-cell marker insulin, endocrine precursor marker neurogenin 3, NK6 homeobox (NKX6.1), and PDX-1 (89, 94). They also proved that WNT inhibition and bone morphogenetic protein (BMP) activation could modulate the ratio of progenitors and endocrine cells, which shed light on a possible gene editing target for ESC differentiation toward the mature β-cells (89).
3.3.2 Immunomodulatory Ability of ESCs and iPSCs
Haque et al. characterized autoantigen-specific naturally occurring Treg-like iPSC-Tregs and proved that adoptive transfer of ovalbumin (OVA)-specific iPSC-Tregs greatly suppressed autoimmunity in the mouse model preventing the β-cells from destruction. These tissue-associated Tregs can effectively inhibit the migration and activity of the pathogenic immune cells and accumulate in the diabetic pancreas causing T1DM by downregulating the production of proinflammatory cytokine IFN-γ and suppressing the expression of ICAM-1 (95). Another report declared that pancreatic endoderm derived from hESCs can generate functional insulin-producing cells in vivo regardless of the presence of innate lymphoid cell elements, and the combination of CTLA4Ig and anti-CD40L mAbs can block hESC-PE graft rejection in immunocompetent mice, while regulatory T cells were not needed for the tolerance during hESC-PE transplantation (96).
To increase the function of stem cells, it is very important to sustain the regeneration and differentiation ability of stem cells and prevent the programmed death of stem cells in vivo (30, 97). Several approaches and conditions, including far-red light, genetic engineering, biological material scaffolds, nanofiber tubular, combination treatment with insulin or other drugs, microcapsules, and co-transplantation with more than one type of stem cells, were utilized to promote the survival, differentiation, and immunomodulatory ability of stem cells in vivo and in vitro. These preclinical attempts tried to derive pancreas islet cells, increase the number and function of Tregs, ameliorate the function of islets, and prevent β-cells more effectively (Table 1). Also, stem cells have been used in human clinical trials, which showed that stem cell transplantation had beneficial effects on T1DM, with no obvious adverse reactions (Table 2). Recently, an allogeneic, gene-edited, immune-evasive, stem cell-derived therapy for the treatment of T1DM was approved in Canada for clinical trial application (CTA) (CRISPR Therapeutics and ViaCyte, Inc. to start clinical trial of the first gene-edited cell replacement therapy for the treatment of T1DM, retrieved on November 16, 2021). This CRISPR therapeutics offered novel β-cell replacement therapies to address unmet T1DM needs. All these efforts are aimed at better promoting the effectiveness of stem cells, which proved to be a more viable option for the treatment of T1DM, lessening the suffering of the patients.
4 Concluding Remarks
In recent research, stem cell therapy has demonstrated itself as a rapidly expanding and potentially limitless source of β-cells to arrive at a cure for T1DM by reconstitution of immunotolerance and differentiation into islet β-cell clusters. As the immunosuppression affected the effect of transplantation of stem cells, stem cell intervention before transplantation could help preserve β-cells and remodel the immune response. However, several challenges, such as the ethical problem of autologous and allogeneic stem cells used to preserve the function of β-cells, still need resolution. Although research into β-cell replacement derived from stem cells is increasing every year, we must make more efforts in the future on the intervention with stem cell transplantation, which can help achieve remission of T1DM by β-cell replacement.
Author Contributions
X-XW reviewed the literature, wrote the manuscript, and created the descriptive figures. X-MH and QZ edited the tables and figures. D-YZ and S-YZ assisted in the literature review. MK edited the manuscript. KX and R-HY revised the manuscript. All authors read and approved the final manuscript.
Funding
This work was supported by the National Natural Science Foundation of China (81971891, 82172196, and 81772134), Key Laboratory of Emergency and Trauma (Hainan Medical University) of the Ministry of Education (KLET-202108), Hunan Province Natural Science Foundation of China (2018JJ3804), and the College Students’ Innovation and Entrepreneurship Project (S20210026020013).
Conflict of Interest
The authors declare that the research was conducted in the absence of any commercial or financial relationships that could be construed as a potential conflict of interest.
Publisher’s Note
All claims expressed in this article are solely those of the authors and do not necessarily represent those of their affiliated organizations, or those of the publisher, the editors and the reviewers. Any product that may be evaluated in this article, or claim that may be made by its manufacturer, is not guaranteed or endorsed by the publisher.
References
1. Campbell MR. Review of Current Status of Molecular Diagnosis and Characterization of Monogenic Diabetes Mellitus: A Focus on Next-Generation Sequencing. Expert Rev Mol Diagn (2020) 20(4):413–20. doi: 10.1080/14737159.2020.1730179
2. Miller KM, Hermann J, Foster N, Hofer SE, Rickels MR, Danne T, et al. Longitudinal Changes in Continuous Glucose Monitoring Use Among Individuals With Type 1 Diabetes: International Comparison in the German and Austrian DPV and U.S. T1D Exchange Registries. Diabetes Care (2020) 43(1):e1–2. doi: 10.2337/dc19-1214
3. Quansah DY, Gross J, Gilbert L, Pauchet A, Horsch A, Benhalima K, et al. Cardiometabolic and Mental Health in Women With Early Gestational Diabetes Mellitus: A Prospective Cohort Study. J Clin Endocrinol Metab (2022) 107(3):e996–e1008. doi: 10.1210/clinem/dgab791
4. Vinuela A, Varshney A, van de Bunt M, Prasad RB, Asplund O, Bennett A, et al. Genetic Variant Effects on Gene Expression in Human Pancreatic Islets and Their Implications for T2D. Nat Commun (2020) 11(1):4912.
5. Wang J, Lv B, Chen X, Pan Y, Chen K, Zhang Y, et al. An Early Model to Predict the Risk of Gestational Diabetes Mellitus in the Absence of Blood Examination Indexes: Application in Primary Health Care Centres. BMC Pregnancy Childbirth (2021) 21(1):814. doi: 10.1186/s12884-021-04295-2
6. Pang H, Luo S, Xiao Y, Xia Y, Li X, Huang G, et al. Emerging Roles of Exosomes in T1DM. Front Immunol (2020) 11:593348. doi: 10.3389/fimmu.2020.593348
7. Rosell Rask S, Krarup Hansen T, Bjerre M. FGF21 and Glycemic Control in Patients With T1D. Endocrine (2019) 65(3):550–7. doi: 10.1007/s12020-019-02027-3
8. Terrazzano G, Bruzzaniti S, Rubino V, Santopaolo M, Palatucci AT, Giovazzino A, et al. T1D Progression Is Associated With Loss of CD3(+)CD56(+) Regulatory T Cells That Control CD8(+) T Cell Effector Functions. Nat Metab (2020) 2(2):142–52. doi: 10.1038/s42255-020-0173-1
9. Katsarou A, Gudbjornsdottir S, Rawshani A, Dabelea D, Bonifacio E, Anderson BJ, et al. Type 1 Diabetes Mellitus. Nat Rev Dis Primers (2017) 3:17016. doi: 10.1038/nrdp.2017.16
10. Owens DR. Insulin Preparations With Prolonged Effect. Diabetes Technol Ther (2011) 13 Suppl 1:S5–14. doi: 10.1089/dia.2011.0068
11. Haidar A, Legault L, Matteau-Pelletier L, Messier V, Dallaire M, Ladouceur M, et al. Outpatient Overnight Glucose Control With Dual-Hormone Artificial Pancreas, Single-Hormone Artificial Pancreas, or Conventional Insulin Pump Therapy in Children and Adolescents With Type 1 Diabetes: An Open-Label, Randomised Controlled Trial. Lancet Diabetes Endocrinol (2015) 3(8):595–604. doi: 10.1016/S2213-8587(15)00141-2
12. Garg SK, Weinzimer SA, Tamborlane WV, Buckingham BA, Bode BW, Bailey TS, et al. Glucose Outcomes With the In-Home Use of a Hybrid Closed-Loop Insulin Delivery System in Adolescents and Adults With Type 1 Diabetes. Diabetes Technol Ther (2017) 19(3):155–63. doi: 10.1089/dia.2016.0421
13. Nijhoff MF, de Koning EJP. Artificial Pancreas or Novel Beta-Cell Replacement Therapies: A Race for Optimal Glycemic Control? Curr Diabetes Rep (2018) 18(11):110. doi: 10.1007/s11892-018-1073-6
14. Nally LM, Sherr JL, Van Name MA, Patel AD, Tamborlane WV. Pharmacologic Treatment Options for Type 1 Diabetes: What's New? Expert Rev Clin Pharmacol (2019) 12(5):471–9. doi: 10.1080/17512433.2019.1597705
15. Yan WT YY, Hu XM, Ning WY, Liao LS, Lu S, Zhao W, et al. Do Pyroptosis, Apoptosis, and Necroptosis (PANoptosis) Exist in Cerebral Ischemia? Evidence From Cell and Rodent Studies. Neural Regener Res (2022) 17(8):1761–8. doi: 10.4103/1673-5374.331539
16. Atkinson MA, Eisenbarth GS, Michels AW. Type 1 Diabetes. Lancet (2014) 383(9911):69–82. doi: 10.1016/S0140-6736(13)60591-7
17. Gregg EW, Cheng YJ, Srinivasan M, Lin J, Geiss LS, Albright AL, et al. Trends in Cause-Specific Mortality Among Adults With and Without Diagnosed Diabetes in the USA: An Epidemiological Analysis of Linked National Survey and Vital Statistics Data. Lancet (2018) 391(10138):2430–40. doi: 10.1016/S0140-6736(18)30314-3
18. Rawshani A, Sattar N, Franzen S, Rawshani A, Hattersley AT, Svensson AM, et al. Excess Mortality and Cardiovascular Disease in Young Adults With Type 1 Diabetes in Relation to Age at Onset: A Nationwide, Register-Based Cohort Study. Lancet (2018) 392(10146):477–86. doi: 10.1016/S0140-6736(18)31506-X
19. Chiang JL, Kirkman MS, Laffel LM, Peters AL. Type 1 Diabetes Sourcebook A: Type 1 Diabetes Through the Life Span: A Position Statement of the American Diabetes Association. Diabetes Care (2014) 37(7):2034–54. doi: 10.2337/dc14-1140
20. Ryan AJ, O'Neill HS, Duffy GP, O'Brien FJ. Advances in Polymeric Islet Cell Encapsulation Technologies to Limit the Foreign Body Response and Provide Immunoisolation. Curr Opin Pharmacol (2017) 36:66–71. doi: 10.1016/j.coph.2017.07.013
21. Vantyghem MC, de Koning EJP, Pattou F, Rickels MR. Advances in Beta-Cell Replacement Therapy for the Treatment of Type 1 Diabetes. Lancet (2019) 394(10205):1274–85. doi: 10.1016/S0140-6736(19)31334-0
22. Gruessner RW, Sutherland DE, Kandaswamy R, Gruessner AC. Over 500 Solitary Pancreas Transplants in Nonuremic Patients With Brittle Diabetes Mellitus. Transplantation (2008) 85(1):42–7. doi: 10.1097/01.tp.0000296820.46978.3f
23. Abdulreda MH, Rodriguez-Diaz R, Cabrera O, Caicedo A, Berggren PO. The Different Faces of the Pancreatic Islet. Adv Exp Med Biol (2016) 938:11–24. doi: 10.1007/978-3-319-39824-2_2
24. Sneddon JB, Tang Q, Stock P, Bluestone JA, Roy S, Desai T, et al. Stem Cell Therapies for Treating Diabetes: Progress and Remaining Challenges. Cell Stem Cell (2018) 22(6):810–23. doi: 10.1016/j.stem.2018.05.016
25. Coe TM, Markmann JF, Rickert CG. Current Status of Porcine Islet Xenotransplantation. Curr Opin Organ Transplant (2020) 25(5):449–56. doi: 10.1097/MOT.0000000000000794
26. Liang CC, Shaw SW, Huang YH, Lee TH. Human Amniotic Fluid Stem Cells can Improve Cerebral Vascular Remodelling and Neurological Function After Focal Cerebral Ischaemia in Diabetic Rats. J Cell Mol Med (2021) 25(21):10185–96. doi: 10.1111/jcmm.16956
27. Zhang Q WX, Hu XM, Zhao WJ, Ban XX, Huang YX, Yan WT, et al. Targeting Programmed Cell Death to Improve Stem Cell Therapy: Implications for Treating Diabetes and Diabetes-Related Diseases. Front Cell Dev Biol (2021) 9:809656. doi: 10.3389/fcell.2021.809656
28. Wen SJ, Zheng XM, Liu LF, Li NN, Mao HA, Huang L, et al. Effects of Primary Microglia and Astrocytes on Neural Stem Cells in In Vitro and In Vivo models of ischemic stroke. Neural Regener Res (2021) 16(9):1677–85.
29. Sultan N, Amin LE, Zaher AR, Grawish ME, Scheven BA. Dental Pulp Stem Cells Stimulate Neuronal Differentiation of PC12 Cells. Neural Regener Res (2021) 16(9):1821–8. doi: 10.4103/1673-5374.306089
30. Hu XM, Li ZX, Zhang DY, Yang YC, Fu SA, Zhang ZQ, et al. A Systematic Summary of Survival and Death Signalling During the Life of Hair Follicle Stem Cells. Stem Cell Res Ther (2021) 12(1):453. doi: 10.1186/s13287-021-02527-y
31. Francese R, Fiorina P. Immunological and Regenerative Properties of Cord Blood Stem Cells. Clin Immunol (2010) 136(3):309–22. doi: 10.1016/j.clim.2010.04.010
32. Hu XM, Zhang Q, Zhou RX, Wu YL, Li ZX, Zhang DY, et al. Programmed Cell Death in Stem Cell-Based Therapy: Mechanisms and Clinical Applications. World J Stem Cells (2021) 13(5):386–415. doi: 10.4252/wjsc.v13.i5.386
33. Yang R, Liu F, Wang J, Chen X, Xie J, Xiong K. Epidermal Stem Cells in Wound Healing and Their Clinical Applications. Stem Cell Res Ther (2019) 10(1):229. doi: 10.1186/s13287-019-1312-z
34. Ilic D, Polak JM. Stem Cells in Regenerative Medicine: Introduction. Br Med Bull (2011) 98:117–26. doi: 10.1093/bmb/ldr012
35. Bongso A, Richards M. History and Perspective of Stem Cell Research. Best Pract Res Clin Obstet Gynaecol (2004) 18(6):827–42. doi: 10.1016/j.bpobgyn.2004.09.002
36. Yang R, Yang S, Zhao J, Hu X, Chen X, Wang J, et al. Progress in Studies of Epidermal Stem Cells and Their Application in Skin Tissue Engineering. Stem Cell Res Ther (2020) 11(1):303. doi: 10.1186/s13287-020-01796-3
37. Parmar M, Grealish S, Henchcliffe C. The Future of Stem Cell Therapies for Parkinson Disease. Nat Rev Neurosci (2020) 21(2):103–15. doi: 10.1038/s41583-019-0257-7
38. Thomson JA, Itskovitz-Eldor J, Shapiro SS, Waknitz MA, Swiergiel JJ, Marshall VS, et al. Embryonic Stem Cell Lines Derived From Human Blastocysts. Science (1998) 282(5391):1145–7. doi: 10.1126/science.282.5391.1145
39. Takahashi K, Tanabe K, Ohnuki M, Narita M, Ichisaka T, Tomoda K, et al. Induction of Pluripotent Stem Cells From Adult Human Fibroblasts by Defined Factors. Cell (2007) 131(5):861–72. doi: 10.1016/j.cell.2007.11.019
40. Tahbaz M, Yoshihara E. Immune Protection of Stem Cell-Derived Islet Cell Therapy for Treating Diabetes. Front Endocrinol (Lausanne) (2021) 12:716625. doi: 10.3389/fendo.2021.716625
41. Buron F, Reffet S, Badet L, Morelon E, Thaunat O. Immunological Monitoring in Beta Cell Replacement: Towards a Pathophysiology-Guided Implementation of Biomarkers. Curr Diabetes Rep (2021) 21(6):19. doi: 10.1007/s11892-021-01386-4
42. Han C, Wang YJ, Wang YC, Guan X, Wang L, Shen LM, et al. Caveolin-1 Downregulation Promotes the Dopaminergic Neuron-Like Differentiation of Human Adipose-Derived Mesenchymal Stem Cells. Neural Regener Res (2021) 16(4):714–20.
43. Khatri R, Petry SF, Linn T. Intrapancreatic MSC Transplantation Facilitates Pancreatic Islet Regeneration. Stem Cell Res Ther (2021) 12(1):121. doi: 10.1186/s13287-021-02173-4
44. Wan X, Zhu L, Zhao L, Peng L, Xiong J, Yang W, et al. Hper3 Promotes Adipogenesis via Hhsp90aa1-Mediated Inhibition of Notch1 Pathway. Cell Death Dis (2021) 12(4):301. doi: 10.1038/s41419-021-03584-0
45. Wang ZL, He RZ, Tu B, He JS, Cao X, Xia HS, et al. Drilling Combined With Adipose-Derived Stem Cells and Bone Morphogenetic Protein-2 to Treat Femoral Head Epiphyseal Necrosis in Juvenile Rabbits. Curr Med Sci (2018) 38(2):277–88. doi: 10.1007/s11596-018-1876-3
46. Dominici M, Le Blanc K, Mueller I, Slaper-Cortenbach I, Marini F, Krause D, et al. Minimal Criteria for Defining Multipotent Mesenchymal Stromal Cells. The International Society for Cellular Therapy Position Statement. Cytotherapy (2006) 8(4):315–7.
47. Davies LC, Alm JJ, Heldring N, Moll G, Gavin C, Batsis I, et al. Type 1 Diabetes Mellitus Donor Mesenchymal Stromal Cells Exhibit Comparable Potency to Healthy Controls In Vitro. Stem Cells Transl Med (2016) 5(11):1485–95. doi: 10.5966/sctm.2015-0272
48. Gerace D, Martiniello-Wilks R, Nassif NT, Lal S, Steptoe R, Simpson AM. CRISPR-Targeted Genome Editing of Mesenchymal Stem Cell-Derived Therapies for Type 1 Diabetes: A Path to Clinical Success? Stem Cell Res Ther (2017) 8(1):62. doi: 10.1186/s13287-017-0511-8
49. Carlsson PO, Schwarcz E, Korsgren O, Le Blanc K. Preserved Beta-Cell Function in Type 1 Diabetes by Mesenchymal Stromal Cells. Diabetes (2015) 64(2):587–92. doi: 10.2337/db14-0656
50. Cai J, Wu Z, Xu X, Liao L, Chen J, Huang L, et al. Umbilical Cord Mesenchymal Stromal Cell With Autologous Bone Marrow Cell Transplantation in Established Type 1 Diabetes: A Pilot Randomized Controlled Open-Label Clinical Study to Assess Safety and Impact on Insulin Secretion. Diabetes Care (2016) 39(1):149–57. doi: 10.2337/dc15-0171
51. Gimble JM, Katz AJ, Bunnell BA. Adipose-Derived Stem Cells for Regenerative Medicine. Circ Res (2007) 100(9):1249–60. doi: 10.1161/01.RES.0000265074.83288.09
52. Chen MT, Zhao YT, Zhou LY, Li M, Zhang Q, Han Q, et al. Exosomes Derived From Human Umbilical Cord Mesenchymal Stem Cells Enhance Insulin Sensitivity in Insulin Resistant Human Adipocytes. Curr Med Sci (2021) 41(1):87–93. doi: 10.1007/s11596-021-2323-4
53. Zhao Y. Stem Cell Educator Therapy and Induction of Immune Balance. Curr Diabetes Rep (2012) 12(5):517–23. doi: 10.1007/s11892-012-0308-1
54. Nauta AJ, Fibbe WE. Immunomodulatory Properties of Mesenchymal Stromal Cells. Blood (2007) 110(10):3499–506. doi: 10.1182/blood-2007-02-069716
55. Ren G, Zhang L, Zhao X, Xu G, Zhang Y, Roberts AI, et al. Mesenchymal Stem Cell-Mediated Immunosuppression Occurs via Concerted Action of Chemokines and Nitric Oxide. Cell Stem Cell (2008) 2(2):141–50. doi: 10.1016/j.stem.2007.11.014
56. Domouky AM, Hegab AS, Al-Shahat A, Raafat N. Mesenchymal Stem Cells and Differentiated Insulin Producing Cells Are New Horizons for Pancreatic Regeneration in Type I Diabetes Mellitus. Int J Biochem Cell Biol (2017) 87:77–85. doi: 10.1016/j.biocel.2017.03.018
57. Boumaza I, Srinivasan S, Witt WT, Feghali-Bostwick C, Dai Y, Garcia-Ocana A, et al. Autologous Bone Marrow-Derived Rat Mesenchymal Stem Cells Promote PDX-1 and Insulin Expression in the Islets, Alter T Cell Cytokine Pattern and Preserve Regulatory T Cells in the Periphery and Induce Sustained Normoglycemia. J Autoimmun (2009) 32(1):33–42. doi: 10.1016/j.jaut.2008.10.004
58. Shigemoto-Kuroda T, Oh JY, Kim DK, Jeong HJ, Park SY, Lee HJ, et al. MSC-Derived Extracellular Vesicles Attenuate Immune Responses in Two Autoimmune Murine Models: Type 1 Diabetes and Uveoretinitis. Stem Cell Rep (2017) 8(5):1214–25. doi: 10.1016/j.stemcr.2017.04.008
59. Abdi R, Fiorina P, Adra CN, Atkinson M, Sayegh MH. Immunomodulation by Mesenchymal Stem Cells: A Potential Therapeutic Strategy for Type 1 Diabetes. Diabetes (2008) 57(7):1759–67. doi: 10.2337/db08-0180
60. Bassi EJ, Moraes-Vieira PM, Moreira-Sa CS, Almeida DC, Vieira LM, Cunha CS, et al. Immune Regulatory Properties of Allogeneic Adipose-Derived Mesenchymal Stem Cells in the Treatment of Experimental Autoimmune Diabetes. Diabetes (2012) 61(10):2534–45. doi: 10.2337/db11-0844
61. Gerace D, Martiniello-Wilks R, Habib R, Ren B, Nassif NT, O'Brien BA, et al. Ex Vivo Expansion of Murine MSC Impairs Transcription Factor-Induced Differentiation Into Pancreatic Beta-Cells. Stem Cells Int (2019) 2019:1395301. doi: 10.1155/2019/1395301
62. van Megen KM, van 't Wout ET, Lages Motta J, Dekker B, Nikolic T, Roep BO. Activated Mesenchymal Stromal Cells Process and Present Antigens Regulating Adaptive Immunity. Front Immunol (2019) 10:694. doi: 10.3389/fimmu.2019.00694
63. Montanucci P, Alunno A, Basta G, Bistoni O, Pescara T, Caterbi S, et al. Restoration of T Cell Substes of Patients With Type 1 Diabetes Mellitus by Microencapsulated Human Umbilical Cord Wharton Jelly-Derived Mesenchymal Stem Cells: An In Vitro Study. Clin Immunol (2016) 163:34–41. doi: 10.1016/j.clim.2015.12.002
64. Montanucci P, Pescara T, Alunno A, Bistoni O, Basta G, Calafiore R. Remission of Hyperglycemia in Spontaneously Diabetic NOD Mice Upon Transplant of Microencapsulated Human Umbilical Cord Wharton Jelly-Derived Mesenchymal Stem Cells (hUCMS). Xenotransplantation (2019) 26(2):e12476. doi: 10.1111/xen.12476
65. Karnieli O, Izhar-Prato Y, Bulvik S, Efrat S. Generation of Insulin-Producing Cells From Human Bone Marrow Mesenchymal Stem Cells by Genetic Manipulation. Stem Cells (2007) 25(11):2837–44. doi: 10.1634/stemcells.2007-0164
66. Chen LB, Jiang XB, Yang L. Differentiation of Rat Marrow Mesenchymal Stem Cells Into Pancreatic Islet Beta-Cells. World J Gastroenterol (2004) 10(20):3016–20. doi: 10.3748/wjg.v10.i20.3016
67. Gao F, Wu DQ, Hu YH, Jin GX, Li GD, Sun TW, et al. In Vitro Cultivation of Islet-Like Cell Clusters From Human Umbilical Cord Blood-Derived Mesenchymal Stem Cells. Transl Res (2008) 151(6):293–302. doi: 10.1016/j.trsl.2008.03.003
68. Chao KC, Chao KF, Fu YS, Liu SH. Islet-Like Clusters Derived From Mesenchymal Stem Cells in Wharton's Jelly of the Human Umbilical Cord for Transplantation to Control Type 1 Diabetes. PloS One (2008) 3(1):e1451. doi: 10.1371/journal.pone.0001451
69. Maldonado M, Huang T, Yang L, Xu L, Ma L. Human Umbilical Cord Wharton Jelly Cells Promote Extra-Pancreatic Insulin Formation and Repair of Renal Damage in STZ-Induced Diabetic Mice. Cell Commun Signal (2017) 15(1):43. doi: 10.1186/s12964-017-0199-5
70. Lu J, Shen SM, Ling Q, Wang B, Li LR, Zhang W, et al. One Repeated Transplantation of Allogeneic Umbilical Cord Mesenchymal Stromal Cells in Type 1 Diabetes: An Open Parallel Controlled Clinical Study. Stem Cell Res Ther (2021) 12(1):340. doi: 10.1186/s13287-021-02417-3
71. Mesples A, Majeed N, Zhang Y, Hu X. Early Immunotherapy Using Autologous Adult Stem Cells Reversed the Effect of Anti-Pancreatic Islets in Recently Diagnosed Type 1 Diabetes Mellitus: Preliminary Results. Med Sci Monit (2013) 19:852–7. doi: 10.12659/MSM.889525
72. Eaves CJ. Hematopoietic Stem Cells: Concepts, Definitions, and the New Reality. Blood (2015) 125(17):2605–13. doi: 10.1182/blood-2014-12-570200
73. Carroll D, St Clair DK. Hematopoietic Stem Cells: Normal Versus Malignant. Antioxid Redox Signal (2018) 29(16):1612–32. doi: 10.1089/ars.2017.7326
74. Voltarelli JC, Couri CE, Stracieri AB, Oliveira MC, Moraes DA, Pieroni F, et al. Autologous Nonmyeloablative Hematopoietic Stem Cell Transplantation in Newly Diagnosed Type 1 Diabetes Mellitus. JAMA (2007) 297(14):1568–76. doi: 10.1001/jama.297.14.1568
75. Copelan EA. Hematopoietic Stem-Cell Transplantation. N Engl J Med (2006) 354(17):1813–26. doi: 10.1056/NEJMra052638
76. Tian C, Ansari MJ, Paez-Cortez J, Bagley J, Godwin J, Donnarumma M, et al. Induction of Robust Diabetes Resistance and Prevention of Recurrent Type 1 Diabetes Following Islet Transplantation by Gene Therapy. J Immunol (2007) 179(10):6762–9. doi: 10.4049/jimmunol.179.10.6762
77. Gu B, Miao H, Zhang J, Hu J, Zhou W, Gu W, et al. Clinical Benefits of Autologous Haematopoietic Stem Cell Transplantation in Type 1 Diabetes Patients. Diabetes Metab (2018) 44(4):341–5. doi: 10.1016/j.diabet.2017.12.006
78. Pastore I, Assi E, Ben Nasr M, Bolla AM, Maestroni A, Usuelli V, et al. Hematopoietic Stem Cells in Type 1 Diabetes. Front Immunol (2021) 12:694118. doi: 10.3389/fimmu.2021.694118
79. Ben Nasr M, D'Addio F, Malvandi AM, Faravelli S, Castillo-Leon E, Usuelli V, et al. Prostaglandin E2 Stimulates the Expansion of Regulatory Hematopoietic Stem and Progenitor Cells in Type 1 Diabetes. Front Immunol (2018) 9:1387. doi: 10.3389/fimmu.2018.01387
80. Wang N, Rajasekaran N, Hou T, Macaubas C, Mellins ED. Immunological Basis for Rapid Progression of Diabetes in Older NOD Mouse Recipients Post BM-HSC Transplantation. PloS One (2015) 10(5):e0128494. doi: 10.1371/journal.pone.0128494
81. Racine J, Wang M, Zhang C, Lin CL, Liu H, Todorov I, et al. Induction of Mixed Chimerism With MHC-Mismatched But Not Matched Bone Marrow Transplants Results in Thymic Deletion of Host-Type Autoreactive T-Cells in NOD Mice. Diabetes (2011) 60(2):555–64. doi: 10.2337/db10-0827
82. Chan J, Clements W, Field J, Nasa Z, Lock P, Yap F, et al. Transplantation of Bone Marrow Genetically Engineered to Express Proinsulin II Protects Against Autoimmune Insulitis in NOD Mice. J Gene Med (2006) 8(11):1281–90. doi: 10.1002/jgm.968
83. Leng Q, Nie Y, Zou Y, Chen J. Elevated CXCL12 Expression in the Bone Marrow of NOD Mice Is Associated With Altered T Cell and Stem Cell Trafficking and Diabetes Development. BMC Immunol (2008) 9:51. doi: 10.1186/1471-2172-9-51
84. D'Addio F, Valderrama Vasquez A, Ben Nasr M, Franek E, Zhu D, Li L, et al. Autologous Nonmyeloablative Hematopoietic Stem Cell Transplantation in New-Onset Type 1 Diabetes: A Multicenter Analysis. Diabetes (2014) 63(9):3041–6. doi: 10.2337/db14-0295
85. Zhang J, Hu M, Wang B, Gao J, Wang L, Li L, et al. Comprehensive Assessment of T-Cell Repertoire Following Autologous Hematopoietic Stem Cell Transplantation for Treatment of Type 1 Diabetes Using High-Throughput Sequencing. Pediatr Diabetes (2018) 19(7):1229–37. doi: 10.1111/pedi.12728
86. Boscari F, D'Anna M, Bonora BM, Tresso S, Cappellari R, Avogaro A, et al. Effects of Glucose Variability on Hematopoietic Stem/Progenitor Cells in Patients With Type 1 Diabetes. J Endocrinol Invest (2021) 44(1):119–26. doi: 10.1007/s40618-020-01278-6
87. Snarski E, Szmurlo D, Halaburda K, Krol M, Urbanowska E, Milczarczyk A, et al. An Economic Analysis of Autologous Hematopoietic Stem Cell Transplantation (AHSCT) in the Treatment of New Onset Type 1 Diabetes. Acta Diabetol (2015) 52(5):881–8. doi: 10.1007/s00592-015-0724-1
88. D'Amour KA, Agulnick AD, Eliazer S, Kelly OG, Kroon E, Baetge EE. Efficient Differentiation of Human Embryonic Stem Cells to Definitive Endoderm. Nat Biotechnol (2005) 23(12):1534–41. doi: 10.1038/nbt1163
89. Loretelli C, Assi E, Seelam AJ, Ben Nasr M, Fiorina P. Cell Therapy for Type 1 Diabetes. Expert Opin Biol Ther (2020) 20(8):887–97. doi: 10.1080/14712598.2020.1748596
90. Tyndall A, Walker UA, Cope A, Dazzi F, De Bari C, Fibbe W, et al. Immunomodulatory Properties of Mesenchymal Stem Cells: A Review Based on an Interdisciplinary Meeting Held at the Kennedy Institute of Rheumatology Division, London, UK, 31 October 2005. Arthritis Res Ther (2007) 9(1):301. doi: 10.4103/1673-5374.331539
91. Rezania A, Bruin JE, Arora P, Rubin A, Batushansky I, Asadi A, et al. Reversal of Diabetes With Insulin-Producing Cells Derived In Vitro From Human Pluripotent Stem Cells. Nat Biotechnol (2014) 32(11):1121–33. doi: 10.1038/nbt.3033
92. Maehr R, Chen S, Snitow M, Ludwig T, Yagasaki L, Goland R, et al. Generation of Pluripotent Stem Cells From Patients With Type 1 Diabetes. Proc Natl Acad Sci USA (2009) 106(37):15768–73. doi: 10.1073/pnas.0906894106
93. Korytnikov R, Nostro MC. Generation of Polyhormonal and Multipotent Pancreatic Progenitor Lineages From Human Pluripotent Stem Cells. Methods (2016) 101:56–64. doi: 10.1016/j.ymeth.2015.10.017
94. Zhu C, Ishikami S, Wang P, Zhao H, Li H. Optimal Design and Fabrication of Multichannel Helical Long-Period Fiber Gratings Based on Phase-Only Sampling Method. Opt Express (2019) 27(3):2281–91. doi: 10.1364/OE.27.002281
95. Haque M, Lei F, Xiong X, Das JK, Ren X, Fang D, et al. Stem Cell-Derived Tissue-Associated Regulatory T Cells Suppress the Activity of Pathogenic Cells in Autoimmune Diabetes. JCI Insight (2019) 4(7):e126471. doi: 10.1172/jci.insight.126471
96. Szot GL, Yadav M, Lang J, Kroon E, Kerr J, Kadoya K, et al. Tolerance Induction and Reversal of Diabetes in Mice Transplanted With Human Embryonic Stem Cell-Derived Pancreatic Endoderm. Cell Stem Cell (2015) 16(2):148–57. doi: 10.1016/j.stem.2014.12.001
97. Yan WT, Lu S, Yang YD, Ning WY, Cai Y, Hu XM, et al. Research Trends, Hot Spots and Prospects for Necroptosis in the Field of Neuroscience. Neural Regener Res (2021) 16(8):1628–37.
98. Aghazadeh Y, Poon F, Sarangi F, Wong FTM, Khan ST, Sun X, et al. Microvessels Support Engraftment and Functionality of Human Islets and hESC-Derived Pancreatic Progenitors in Diabetes Models. Cell Stem Cell (2021) 28(11):1936–1949.e1938. doi: 10.1016/j.stem.2021.08.001
99. Yu G, Zhang M, Gao L, Zhou Y, Qiao L, Yin J, et al. Far-Red Light-Activated Human Islet-Like Designer Cells Enable Sustained Fine-Tuned Secretion of Insulin for Glucose Control. Mol Ther J Am Soc Gene Ther (2022) 30(1):341–54. doi: 10.1016/j.ymthe.2021.09.004
100. Castro-Gutierrez R, Alkanani A, Mathews CE, Michels A, Russ HA. Protecting Stem Cell Derived Pancreatic Beta-Like Cells From Diabetogenic T Cell Recognition. Front Endocrinol (2021) 12:707881. doi: 10.3389/fendo.2021.707881
101. Shaheen R, Gurlin RE, Gologorsky R, Blaha C, Munnangi P, Santandreu A, et al. Superporous Agarose Scaffolds for Encapsulation of Adult Human Islets and Human Stem-Cell-Derived β Cells for Intravascular Bioartificial Pancreas Applications. J Biomed materials Res Part A (2021) 109(12):2438–48. doi: 10.1002/jbm.a.37236
102. Wang X, Maxwell KG, Wang K, Bowers DT, Flanders JA, Liu W, et al. A Nanofibrous Encapsulation Device for Safe Delivery of Insulin-Producing Cells to Treat Type 1 Diabetes. Sci Trans Med (2021) 13(596):eabb4601. doi: 10.1126/scitranslmed.abb4601
103. Navabi R, Negahdari B, Hajizadeh-Saffar E, Hajinasrollah M, Jenab Y, Rabbani S, et al. Combined Therapy of Mesenchymal Stem Cells With a GLP-1 Receptor Agonist, Liraglutide, on an Inflammatory-Mediated Diabetic Non-Human Primate Model. Life Sci (2021) 276:119374. doi: 10.1016/j.lfs.2021.119374
104. Song L, Gou W, Wang J, Wei H, Lee J, Strange C, et al. Overexpression of Alpha-1 Antitrypsin in Mesenchymal Stromal Cells Improves Their Intrinsic Biological Properties and Therapeutic Effects in Nonobese Diabetic Mice. Stem Cells Trans Med (2021) 10(2):320–31. doi: 10.1002/sctm.20-0122
105. Montanucci P, Pescara T, Greco A, Leonardi G, Marini L, Basta G, et al. Co-Microencapsulation of Human Umbilical Cord-Derived Mesenchymal Stem and Pancreatic Islet-Derived Insulin Producing Cells in Experimental Type 1 Diabetes. Diabetes-Metabolism Res Rev (2021) 37(2):e3372. doi: 10.1002/dmrr.3372
106. Sarvestani FS, Zare MA, Saki F, Koohpeyma F, Al-Abdullah IH, Azarpira N. The Effect of Human Wharton's Jelly-Derived Mesenchymal Stem Cells on MC4R, NPY, and LEPR Gene Expression Levels in Rats With Streptozotocin-Induced Diabetes. Iranian J Basic Med Sci (2020) 23(2):214–23.
107. Kuppan P, Seeberger K, Kelly S, Rosko M, Adesida A, Pepper AR, et al. Co-Transplantation of Human Adipose-Derived Mesenchymal Stem Cells With Neonatal Porcine Islets Within a Prevascularized Subcutaneous Space Augments the Xenograft Function. Xenotransplantation (2020) 27(4):e12581. doi: 10.1111/xen.12581
108. Hashemi SM, Hassan ZM, Hossein-Khannazer N, Pourfathollah AA, Soudi S. Investigating the Route of Administration and Efficacy of Adipose Tissue-Derived Mesenchymal Stem Cells and Conditioned Medium in Type 1 Diabetic Mice. Inflammopharmacology (2020) 28(2):585–601. doi: 10.1007/s10787-019-00661-x
109. Su M, Lin Y, He Z, Lai L. Transplantation of MHC-Mismatched Mouse Embryonic Stem Cell-Derived Thymic Epithelial Progenitors and MHC-Matched Bone Marrow Prevents Autoimmune Diabetes. Stem Cell Res Ther (2019) 10(1):239. doi: 10.1186/s13287-019-1347-1
110. Ben Nasr M, Tezza S, D'Addio F, Mameli C, Usuelli V, Maestroni A, et al. PD-L1 Genetic Overexpression or Pharmacological Restoration in Hematopoietic Stem and Progenitor Cells Reverses Autoimmune Diabetes. Sci Trans Med (2017) 9(416):eaam7543. doi: 10.1126/scitranslmed.aam7543
111. Alagpulinsa DA, Cao JJL, Driscoll RK, Sîrbulescu RF, Penson MFE, Sremac M, et al. Alginate-Microencapsulation of Human Stem Cell-Derived β Cells With CXCL12 Prolongs Their Survival and Function in Immunocompetent Mice Without Systemic Immunosuppression. Am J Transplant Off J Am Soc Transplant Am Soc Transplant Surgeons (2019) 19(7):1930–40.
112. Long G, Zhang G, Zhang F, Li M, Ye D, Yang D, et al. Cotransplantation of Mesenchymal Stem Cells and Immature Dendritic Cells Potentiates the Blood Glucose Control of Islet Allografts. BioMed Res Int (2017) 2017:4107943. doi: 10.1155/2017/4107943
113. Manzar GS, Kim EM, Zavazava N. Demethylation of Induced Pluripotent Stem Cells From Type 1 Diabetic Patients Enhances Differentiation Into Functional Pancreatic β Cells. J Biol Chem (2017) 292(34):14066–79. doi: 10.1074/jbc.M117.784280
114. Daneshmandi S, Karimi MH, Pourfathollah AA. TGF-β Engineered Mesenchymal Stem Cells (TGF-β/MSCs) for Treatment of Type 1 Diabetes (T1D) Mice Model. Int Immunopharmacol (2017) 44:191–6. doi: 10.1016/j.intimp.2017.01.019
115. Richardson T, Barner S, Candiello J, Kumta PN, Banerjee I. Capsule Stiffness Regulates the Efficiency of Pancreatic Differentiation of Human Embryonic Stem Cells. Acta biomaterialia (2016) 35:153–65. doi: 10.1016/j.actbio.2016.02.025
116. Bal T, Nazli C, Okcu A, Duruksu G, Karaöz E, Kizilel S. Mesenchymal Stem Cells and Ligand Incorporation in Biomimetic Poly(Ethylene Glycol) Hydrogels Significantly Improve Insulin Secretion From Pancreatic Islets. J Tissue Eng Regenerative Med (2017) 11(3):694–703. doi: 10.1002/term.1965
117. Yaochite JN, Caliari-Oliveira C, de Souza LE, Neto LS, Palma PV, Covas DT, et al. Therapeutic Efficacy and Biodistribution of Allogeneic Mesenchymal Stem Cells Delivered by Intrasplenic and Intrapancreatic Routes in Streptozotocin-Induced Diabetic Mice. Stem Cell Res Ther (2015) 6(1):31. doi: 10.1186/s13287-015-0017-1
118. Araujo DB, Dantas JR, Silva KR, Souto DL, Pereira MFC, Moreira JP, et al. Allogenic Adipose Tissue-Derived Stromal/Stem Cells and Vitamin D Supplementation in Patients With Recent-Onset Type 1 Diabetes Mellitus: A 3-Month Follow-Up Pilot Study. Front Immunol (2020) 11:993. doi: 10.3389/fimmu.2020.00993
119. Delgado E, Perez-Basterrechea M, Suarez-Alvarez B, Zhou H, Revuelta EM, Garcia-Gala JM, et al. Modulation of Autoimmune T-Cell Memory by Stem Cell Educator Therapy: Phase 1/2 Clinical Trial. EBioMedicine (2015) 2(12):2024–36. doi: 10.1016/j.ebiom.2015.11.003
120. Zhao Y, Jiang Z, Zhao T, Ye M, Hu C, Yin Z, et al. Reversal of Type 1 Diabetes via Islet Beta Cell Regeneration Following Immune Modulation by Cord Blood-Derived Multipotent Stem Cells. BMC Med (2012) 10:3. doi: 10.1186/1741-7015-10-3
Keywords: type 1 diabetes mellitus, stem cell, β-cell, immunotolerance, transplantation
Citation: Wan X-X, Zhang D-Y, Khan MA, Zheng S-Y, Hu X-M, Zhang Q, Yang R-H and Xiong K (2022) Stem Cell Transplantation in the Treatment of Type 1 Diabetes Mellitus: From Insulin Replacement to Beta-Cell Replacement. Front. Endocrinol. 13:859638. doi: 10.3389/fendo.2022.859638
Received: 21 January 2022; Accepted: 16 February 2022;
Published: 18 March 2022.
Edited by:
Gaetano Santulli, Albert Einstein College of Medicine, United StatesCopyright © 2022 Wan, Zhang, Khan, Zheng, Hu, Zhang, Yang and Xiong. This is an open-access article distributed under the terms of the Creative Commons Attribution License (CC BY). The use, distribution or reproduction in other forums is permitted, provided the original author(s) and the copyright owner(s) are credited and that the original publication in this journal is cited, in accordance with accepted academic practice. No use, distribution or reproduction is permitted which does not comply with these terms.
*Correspondence: Kun Xiong, eGlvbmdrdW4yMDAxQDE2My5jb20=; Rong-Hua Yang, MjE3MjAwOTFAcXEuY29t