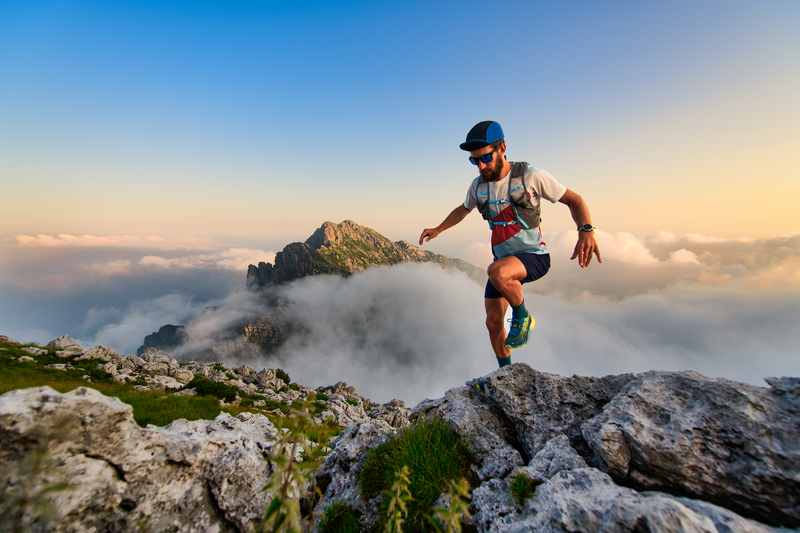
94% of researchers rate our articles as excellent or good
Learn more about the work of our research integrity team to safeguard the quality of each article we publish.
Find out more
REVIEW article
Front. Endocrinol. , 04 April 2022
Sec. Reproduction
Volume 13 - 2022 | https://doi.org/10.3389/fendo.2022.850219
This article is part of the Research Topic Research Advances in Male Fertility: New Horizons for Investigating Human Testicular Function and Development of Clinical Fertility Preservation Approaches View all 11 articles
Fertility preservation via biobanking of testicular tissue retrieved from testicular biopsies is now generally recommended for boys who need to undergo gonadotoxic treatment prior to the onset of puberty, as a source of spermatogonial stem cells (SSCs). SSCs have the potential of forming spermatids and may be used for therapeutic fertility approaches later in life. Although in the past 30 years many milestones have been reached to work towards SSC-based fertility restoration therapies, including transplantation of SSCs, grafting of testicular tissue and various in vitro and ex vivo spermatogenesis approaches, unfortunately, all these fertility therapies are still in a preclinical phase and not yet available for patients who have become infertile because of their treatment during childhood. Therefore, it is now time to take the preclinical research towards SSC-based therapy to the next level to resolve major issues that impede clinical implementation. This review gives an outline of the state of the art of the effectiveness and safety of fertility preservation and SSC-based therapies and addresses the hurdles that need to be taken for optimal progression towards actual clinical implementation of safe and effective SSC-based fertility treatments in the near future.
In recent decades, spermatogonial stem cell (SSC)-based therapeutic fertility approaches have become an important topic of investigation to overcome gonadotoxic treatment-induced infertility. The increasing survival rates over time of young cancer patients have highlighted the impact of gonadotoxic cancer treatments and the consequences for their fertility later in life. Currently, there is a survival rate of 80% among pediatric cancer patients in Europe (1), leading to a large population of childhood cancer survivors who are at risk of cancer treatment related infertility, for whom these SSC-based therapeutic approaches may be an opportunity to father genetically related children.
In the male, functional spermatogenesis is crucial for fertility. Spermatogenesis is initiated at puberty and relies on functional SSCs, which are located at the basement membrane of the seminiferous tubules within the testes. The SSCs will either self-renew to maintain the number of stem cells or become differentiating spermatogonia that will develop into spermatocytes which, after two subsequent meiotic divisions, form haploid spermatids that will ultimately give rise to spermatozoa. Spermatogenesis is tightly regulated by the surrounding testicular somatic cells including Sertoli cells, peritubular cells and Leydig cells (2). Irradiation and the majority of chemotherapeutic agents use mechanisms that target proliferating cells which, unfortunately, include the proliferative SSCs, thereby impairing spermatogenesis (3). Prior to such a gonadotoxic treatment, adult men are given the option to cryopreserve sperm to enable them to sire a child via current fertility treatments. For young boys who have to undergo a gonadotoxic treatment, but do not produce sperm yet, there is currently no option to preserve and later on restore their fertility. However, the immature pre-pubertal testis does contain SSCs and cryopreservation of a testicular biopsy prior to the gonadotoxic treatment is offered in multiple medical centers around the world. Although still under development, SSC-based treatments thus provide an opportunity to preserve and restore fertility for pre-pubertal boys (4, 5). The development of methods for preservation and restoration of fertility has become an important aspect of research to further enhance the quality of life for male patients requiring gonadotoxic treatment during childhood. In trying to offer fertility preservation and restoration options, many avenues have been pursued through use of the SSCs residing within testicular tissues after birth (Figure 1). With the in vivo capacity of developing into sperm, SSCs are prime candidates for possible interventions to restore fertility, which include spermatogonial stem cell transplantation (SSCT), testicular tissue grafting and in vitro or ex vivo spermatogenesis.
Figure 1 Pathways in fertility restoration. After cryopreservation of a testicular biopsy, clinical application of SSC-based techniques can be sought via SSCT, testicular tissue grafting or in vitro spermatogenesis, either through cell-based or tissue-based culture. For SSCT, natural conception could be achieved whereas for therapies based on grafting or in vitro spermatogenesis, an ICSI procedure would be performed using elongated spermatids derived from the technique.
In this review we will pinpoint which hurdles still need to be overcome in (preclinical) research to fulfill the promise of SSC-based approaches, in anticipation of providing these fertility treatments for patients.
The use of testicular tissue is a prerequisite for preclinical research on SSC-based techniques. In designing research and moving towards clinical applications, the target patient group should always be taken into consideration and the tissues used should represent this population as closely as possible.
In addition to a scarcity of available human testicular tissue for research in general, not all testicular tissues are equally suited for all lines of research in SSC-based approaches, as inherent differences can affect the presence and functionality of specific cell populations present within the testicular tissue that might influence the success rate of SSC-based research (Table 1). This is illustrated by the fact that prenatal, neonatal and adult tissues show distinct germ cell populations (6) and even within prenatal tissues differences in spermatogonial populations are evident between the sequential trimesters (7, 8). In addition, testicular somatic cells gain maturity over time, thereby altering the testicular micro-environment in the tissues used for research.
Table 1 Suitability of various testicular tissues as sources for preclinical research on fertility restoration techniques.
For preclinical research on SSC-based therapies there is a clear preference for the use of pre-pubertal tissue, both because of good representation of the intended patient population as well as the technical suitability of this tissue for these studies.
When pre-pubertal material is not available, research on SSCT can use adult testicular tissues, preferably of those patients in which the functionality of the SSCs has been established through histological detection of complete spermatogenesis. Tissues bearing pathologies that affect the spermatogonial population, such as congenital abnormalities or acquired Sertoli cell only (SCO) syndrome, are unsuitable for in vitro studies on SSC proliferation because of the absence of (functional) SSCs. Pathologies which only affect germ cells during their post-spermatogonial differentiating stages, as is the case in non-obstructive azoospermia patients with maturation arrest at the level of meiosis or spermiogenesis, may leave spermatogonial function unaffected. However, the level of maturation arrest may influence culture outcomes when propagating SSCs, as in vitro culture of testicular cells with early (pre-meiotic) maturation arrest may lead to more colonies, with larger diameters, than cultures using testicular cells of tissues with late (post-meiotic) maturation arrest (9).
For research on testicular tissue grafting and in vitro spermatogenesis, adult testicular material is unsuitable as study results would be influenced by either the presence of already ongoing spermatogenesis or, in its absence, by factors such as maturation arrest hampering any possibility of differentiation. The use of mouse neonatal or pre-pubertal testicular tissue for grafting in mice has been successful in the derivation of fertilization-capable sperm from the graft (10). However, caution is warranted with optimization of grafting protocols using human neonatal or fetal tissues, as the immature population of germ and somatic cells may respond differently than the germ cells present within the tissue of the pre-pubertal patient population.
Generally, testicular tissues derived from patients with underlying conditions, or undergoing treatments that affect meiosis and/or spermiogenesis, such as hormonal treatments for gender dysphoria (11, 12) or infectious diseases (13), could be used under the assumption that spermatogonial function is not affected. It is known that the vast majority of testicular tissue obtained during orchiectomy in transwomen contains spermatogonia (14). However, to our knowledge, the effects of these conditions on SSCs have not been systematically studied.
Likewise, when a patient has already started chemotherapy or radiation regiments, the SSCs within their testicular tissue may have deteriorated or undergone changes that will cause impairment or apoptosis of SSCs in vitro (15–17).
Finally, the use of tissues containing testicular cancer is not recommended for any preclinical SSC-based research as cellular function may be influenced by the cancerous cells. In the case of germ cell neoplasia in situ (GCNIS) these cancerous cells, which originated from precursors of SSCs, will have many molecular characteristics similar to SSCs, which may influence study outcomes (18).
To interpret results in preclinical research on SSC-based approaches, thorough documentation of patient characteristics, demography and (testicular) pathology of tissues used in these studies is essential.
For the future clinical application of SSC-based therapies, the testicular tissue biopsies are cryopreserved until the patient has a wish to have children, years after the pre-pubertal tissue biopsies were taken. During the freezing process of this testicular tissue, testicular cells might suffer from cryogenic damage caused by the formation of ice crystals. In an isotonic solution, water will crystallize spontaneously at ‐10°C (19). As a result, the osmolarity of the solution will increase, which will cause the cells in solution to lose intracellular water. When the cooling rate is too high, the cells are not able to lose all the intracellular water in time and intracellular ice crystals will be formed. When the cooling rate is too low, the cells will be exposed to a hyper‐osmotic environment for too long, which will cause cell shrinking and apoptosis. To prevent these forms of cryodamage, different freezing methods, devices and cryoprotectants can be used for correct storage of biopsies, to allow future use of the tissue in SSC-based therapies (19).
The freezing of either a testicular cell suspension or testicular tissue have been proposed as options to cryopreserve SSCs. The freezing of testicular tissue has preference due to greater viability of germ cells and in particular SSCs, compared to the freezing of a testicular cell suspension (20). Furthermore, the cryopreservation of intact testicular tissue fragments permits the future opportunity to pursue tissue-based therapies as well as retaining the option to generate a cell suspension from the testicular fragments for stem cell-based therapies. For cryopreservation, the testicular tissue is divided into small fragments (approx. 3 mm3), which are deposited in cryotubes or cryostraws containing a cryoprotective medium.
Currently, no standardized cryopreservation method has been established that is optimal for male fertility preservation. However, the most commonly used freezing protocol is controlled slow freezing (CSF) with seeding. In this method, testicular fragments in 5% dimethylsulfoxide (DMSO) are cooled with a rate of 1°C/min, maintained at 0°C for 5 min, followed by further cooling at 0.5°C/min until −8°C for hand seeding and continued freezing until −40°C and subsequently, after a short stop, to −70°C with a cooling rate of 7°C/min before transfer to liquid or vaporized nitrogen (21).
An alternative to slow freezing is the use of solid-surface vitrification (SSV). Using this method, a droplet of DMSO-based cryoprotectant containing tissue fragments is exposed to liquid nitrogen and subsequently stored (22). However, slow freezing seems to provide greater protection to testicular tissue than SSV. This is in line with other studies reporting the ability of a slow freezing protocol to maintain tubular architecture and integrity of pre-pubertal human testicular tissue fragments (21, 23–31).
Besides the different freezing regimes, various cryoprotectant freezing media can be used. To examine the effect of cryopreservation on testicular tissue fragments, Keros and colleagues analyzed morphology and structure of the tissue as well as the presence of MAGE-A4+ spermatogonia using light- and electron microscopy (21). In these experiments, the fragments were thawed and subsequently cultured for one day at 33°C in 5% CO2 prior to fixation. The results indicate that a freezing medium containing 5% DMSO (0.7 mol/l) during cryopreservation causes a reduced amount of cryodamage in comparison to other cryoprotectants such as 1.5 mol/l 1,2-propanediol (PrOH). Over 90% of MAGE-A4+ cells were undamaged (i.e. not detached from the basement membrane or neighboring Sertoli cells) when using DMSO, compared to 60% using PrOH.
This is in agreement with results showing that cryopreservation of testicular tissue by slow-freezing in 5% DMSO does not seem to affect the long-term culture of human testicular cells with regard to the presence of germ cell and somatic cell populations (32). Further optimization and possible addition of novel cryoprotectants, such as the antioxidant pentoxifylline (33) to the freezing medium may further improve the preservation of human SSC viability. Our research group cryopreserved testicular tissues obtained from orchiectomies of two adult patients with prostate cancer using various protocols in a pilot study. We compared cryoprotectant TEST-yolk buffer (TYB) with 7.5% human serum albumin (HSA, Albuman), 5% DMSO with 5% HSA and 8% DMSO with 20% HSA using a controlled slow freezing method described for tissue CSF as described above (21). Furthermore, we tested other freezing methods by bringing the straws with tissue fragments and cryoprotectant directly in the nitrogen vapor or using a shorter method of controlled slow freezing originally designed for cryopreserving sperm (CSFS), during which cryostraws were cooled with 0.5°C/min to 5°C followed by a cooling rate of 2°C/min until the samples reached 2°C. Finally, the samples were cooled until ‐80°C with a cooling rate of 10°C/min. No seeding was performed in this method. Morphology of the tissue was assessed after a 24-hour culture compared to fresh tissue by MAGE-A4 staining. Long-term cultures of isolated testicular cells from these tissues were assessed as well, including by SSC colony counts. Morphological damage was most pronounced in all CSFS frozen tissue and was also found to be increased in all those testis fragments cryopreserved in TYB-containing medium compared to align with DMSO-containing medium. The immunostaining of MAGE-A4+ cells in these tissues, 24 hours after culture, reflected these results. When testicular cells were isolated and cultured, cells frozen by CSFS and in nitrogen vapor of one patient did not survive long term culture. However, the cultures of isolated cells from testicular fragment frozen tissue in all other conditions were all capable of SSC colony formation, indicating presence and proliferation of SSCs. Collectively, the slow freezing method CSF (21) with 5% or 8% DMSO as a cryoprotective agent appears to be favorable for cryopreserving testicular tissue for future in vitro spermatogonial proliferation prior to transplantation as a fertility restoration treatment.
The fertility restoring potential of cryopreserved tissue has often been investigated with regards to testicular grafting, with the use of both fresh (10, 34, 35) and cryopreserved tissues (36–40) which have been shown to be successful in pig, mice and primate models. Direct comparisons of the use of fresh versus frozen-thawed tissues intended for tissue grafting have been performed in various species, but show variable results. In mice, spermatogenic progress within the testicular fragments after grafting was similar between grafted fresh tissue fragments and fragments that were frozen-thawed prior to grafting (41). In a later study, a lower number of intact tubuli at one day after grafting within grafted frozen-thawed tissues was found, but at later timepoints, up until two months, no differences were observed between grafted fresh and frozen-thawed tissues (42). Fayomi et al. did not show any differences between fresh and frozen-thawed conditions regarding graft weight, stage of spermatogenesis or percentage of tubuli with complete spermatogenesis, whether the grafts were ectopically or orthotopically placed (43). The use of human frozen-thawed testicular tissues xenografted in mice resulted in similar levels of structural integrity and germ cell survival as the use of fresh tissues, although the number of Sertoli cell-only (SCO) tubules was higher in the frozen-thawed tissue group (44).
For in vitro spermatogenesis with testicular tissue, cryopreserved testicular tissues have also been tested compared to fresh testicular organ culture. The organ culture used was first described for mouse testis fragments (45). This group compared fresh with cryopreserved mouse testis tissue with regard to their ability to develop spermatogonia to germ cells of late meiosis onwards under the reporter of Gsg-GFP (46). They applied uncontrolled slow freezing, using a Bicell biofreezing vessel, with a cooling rate of 1°C/min until -80°C before plunging in liquid nitrogen, using either 1.5 mol/l (11%) DMSO, 1.5 mol/l PrOH or Cell Banker 1 as cryoprotectant, or vitrification with Stem Cell Keep as cryoprotectant. They found that slow freezing with DMSO and vitrification with Stem Cell Keep showed similar progression of spermatogenesis as fresh cultured tissue, based on GFP expression levels. Also, for human testicular organ culture the use of fresh and cryopreserved tissues using CSF with 5% DMSO were compared (47). Reassuringly, no significant difference in culture outcome on spermatogonial maintenance and proliferation and hormone production between fresh and cryopreserved testicular fragments of human pre-pubertal boys could be found (47).
Auto-transplantation of SSCs harvested from the patient prior to treatment could provide recolonization of the seminiferous tubules and subsequent in vivo spermatogenesis, in theory restoring fertility and the ability of the patient to achieve progeny through natural conception (Figure 1). To reintroduce the SSCs into the human testis, the preferential method seems the ultrasonically-guided injection of cells in the rete testis, a comprehensive review of which is written by Gul et al. (48).
Brinster et al. were the first to demonstrate the process of SSCT to be successful in establishing colonization and spermatogenesis in recipient testes in mice (49, 50), as well as in resulting in offspring with the donor haplotype (50). Since then, auto- or allo-transplantation of SSCs has been achieved in various mammals, including rodent and non-rodent species, as reviewed by Takashima & Shinohara (51), and non-human primates (52, 53), resulting in functional spermatogenesis within the recipient animals.
In humans, clinical trials with SSCT have not yet been established; a single report on SSCT in seven men receiving injections of cryopreserved testicular cells has not been followed up by a report on the outcome of these procedures (54). However, from studies in mice it has become clear that transplanted numbers of SSC colonies gradually decrease during the homing process after transplantation (55) and that the concentration of transplanted SSCs is highly linked to the success of colonization of SSCs (56) and donor-derived spermatogenesis within the recipient testis (57).
Because only biopsies of limited size can be collected from patients, propagation of SSCs retrieved from these biopsies will be necessary to achieve sufficient numbers for successful transplantation and recolonization of the seminiferous tubules within the recipient testis. Kanatsu-Shinohara et al. first demonstrated effective long-term propagation of murine SSCs, which were able to restore fertility in sterile recipients (58). In humans, long-term propagation of testicular cells was demonstrated to be possible as well, with cultured testicular cells from both adult (59) and pre-pubertal tissues (60). Identity and propagation between two time points in culture of these human SSCs could be demonstrated through xenotransplantation into sterile mice, by counting donor SSCs that had migrated to the spermatogonial stem cell niche within the seminiferous tubules.
Still, the identification of SSCs within a testicular tissue or cell culture in vitro is a major challenge. Testicular cell cultures include both somatic cells and germ cells, the latter of which is a heterogeneous group of spermatogonia of varying states of differentiation. At this moment there is no agreement on a specific, unambiguous marker for human and/or non-human primate SSCs in vivo and in vitro. For many currently used markers, including ITGA6, KIT, GPR125 and DAZL, expression is not strictly limited to spermatogonia and can also be found in testicular somatic cells (61, 62). For other markers, spermatogonial expression of the marker is controversial, as not all study results are in agreement. This is the case for GFRA1, THY1 and UCHL1 (22, 61, 63–79). Expression of certain markers can also rely on the developmental stage of the testicular tissue; for instance, expression of POU5F1 is limited to subpopulations of fetal and neonatal germ cells (gonocytes) in humans and expression is downregulated along with progressing differentiation (7, 8, 18, 80).
Single-cell sequencing studies have identified various ‘states’ of germ cells, with progression of development of differentiation from State 0 to State 4, where each state is characterized by a unique set of markers, although at times partially overlapping (6, 81). UTF1 and PIWIL4 are examples of markers expressed in the earliest state, with PIWIL4 being the more specific marker (6, 82, 83). Another newly identified candidate marker for undifferentiated spermatogonia, carrying SSC characteristics, involves the LPPR3 protein (6).
Furthermore, it is uncertain whether various testicular cell types retain their transcriptomic and metabolic signatures when isolated from their natural niche (62). Due to these dynamic processes, the heterogeneity of the testicular cell populations and the many potential markers to choose from, it is difficult to establish inter-study comparisons of culture outcomes and efficiency with regard to SSC propagation. To extend studies into the field of SSCs and their potential use, the scientific community would benefit from more insight into specific markers for clearly-identified spermatogonial populations, combined with functional studies as to their SSC potential with regard to in vitro use. In identifying markers, a focus on surface markers as opposed to nuclear markers may be more beneficial for isolation and enrichment of SSCs from cryopreserved biopsies or after culture.
Currently, the gold standard to demonstrate SSC functionality is still the (xeno)transplantation assay, in which donor-derived SSCs are shown to be capable to migrate to the stem cell niche within the seminiferous tubules of the recipient testis and, in a compatible species, initiate and maintain donor-derived spermatogenesis, although this latter part cannot be demonstrated in human to mice xenotransplantation assays.
In working towards clinical application of SSCT, study protocols have to be adapted to clinical requirements and regulations. Therefore, it will be essential to work towards xenofree, clinical grade media and methods in compliance with good manufacturing practice (GMP) and good clinical practice (GCP) systems, while adhering to the regulations regarding the production of advanced therapy medicinal products (ATMP, regulation no. 1394/2007 [European Medicines Agency (EMA)] (84).
The culture methods and media should support SSC proliferation while preventing their differentiation. Besides basic nutrients, additional components such as specific cytokines, metabolites, hormones and other signaling molecules, that are known to stimulate spermatogonial proliferation, may be included in the culture medium. In 2003, Kanatsu-Shinohara et al. developed a successful culture protocol, using medium based on StemPro-34 Serum Free medium, for long-term in vitro propagation of murine SSCs (58). Although a comparable medium can also support long-term propagation of human SSCs (59, 60), overgrowth of testicular somatic cells within the culture system remains problematic, as the SSC signature is diluted over time (62). The presence of feeder cells is beneficial to the survival of SSCs as they provide mechanical and metabolic support and paracrine signals (85, 86). The use of exogenous feeder layers however is unsuitable for clinical implementation of the technique. Therefore, somatic cells which support SSC proliferation and which are already present within the testicular suspension, isolated from a testicular biopsy, are used for SSC culture. To prevent overgrowth of these somatic cells, the culture method and medium used should steer towards an optimal balance between the growth of somatic cells and SSCs. Alternatively, the testicular somatic cells may be replaced by a (synthetic) matrix for cell support, combined with supplementation of the medium with nutrients and growth factors to ensure the metabolic support for SSCs otherwise provided by the feeder cells. This process however heavily relies on the ability to isolate and remove the somatic cell population from the germ cell population prior to culture, which is currently limited by the lack of a specific SSC marker (87, 88).
Optimization of the method for in vitro culture of human SSCs is thus a necessary step prior to clinical implementation. Investigation of the SSC niche in humans might be a pivotal step in identifying the factors necessary for effective maintenance and propagation of SSCs (89).
In vivo spermatogenesis can also be achieved through autologous grafting of immature testicular tissue fragments underneath the skin of the patient (Figure 1). Through angiogenesis, the tissue receives endocrine signals from the body circulation which enable spermatogenesis within the tissue (90, 91). After full progression of spermatogenesis, spermatids can then be harvested from the retrieved graft and used in current assisted reproductive techniques like intracytoplasmic sperm injection (ICSI).
Testicular (xeno)grafting has been shown to lead to successful spermatogenesis in testicular tissues of many mammalian species, including mice, pigs and monkeys (34, 35, 37), subsequently leading in some cases to live offspring through application of ICSI (10, 35, 40, 43, 92). Host conditions may impede full spermatogenesis within xenotransplants with cryopreserved pre-pubertal tissues (39), although in non-human primates complete spermatogenesis has been achieved with autologous transplantations of fresh testicular pre-pubertal tissue as well as with xenografts of similar origin (34, 93).
In the xenografting of non-human primate testicular tissue to mice, the spermatogenic maturation depended on the location of the graft, with orthotopic (intra-testicular) grafts leading to further spermatogenic progression in a higher number of grafts than ectopic grafts under the skin of the back (38, 94). The ectopic location of the grafts in these experiments may have caused a higher tissue temperature and different hormone concentrations than those physiologically present in the scrotal area, potentially contributing to spermatogenic arrest. Recently, Fayomi et al. succeeded in fertilization of primate oocytes using autologous graft-derived primate spermatozoa, resulting in the birth of a healthy rhesus macaque (43). As of yet, no autologous grafting has been described with human tissue; only xenografts to mice have been studied. The use of human tissue in xenografts to mice has not yet resulted in complete spermatogenesis (95, 96), although meiotic activity could be observed (44).
For clinical use, the quantity of collected material is likely small, which could represent an obstacle for the success of this technique. Low survival rates of grafted tissues have been reported, potentially necessitating unilateral orchiectomy to retrieve sufficient amounts of material (39).
Success of this technique also relies on adequate vascularization of the grafted tissue, delivering both the necessary oxygen and hormones for SSC proliferation and differentiation. Hypoxia in the graft center may lead to loss of testicular tissue and all germ cells within (42). Vascularization of human grafts has been shown to benefit from a short-term culture supplemented with vascular endothelial growth factor (VEGF) prior to placement of the graft (93), but additional growth factors other than VEGF may be necessary for successful graft survival.
Spermatogenesis is one of the most complicated developmental processes in the human body, and thus remains very challenging to re-create by in vitro differentiation of stem cells or ex vivo cultures of testicular fragments (Figure 1). Nevertheless, application of in vitro or ex vivo spermatogenesis holds great potential for future fertility restoration or preservation, as haploid spermatids derived from these methods could be used for assisted reproductive technologies such as ICSI.
The use of SSCs as basis for in vitro spermatogenesis (97–101), isolated from a testicular biopsy taken prior to gonadotoxic treatment, would have high clinical relevance. Although many efforts have been made to re-create spermatogenesis in vitro using pluripotent stem cells (PSCs) (102, 103), blastocyst embryos to isolate the required embryonic stem cells (ESCs) will likely not be available for clinical use. Moreover, even though induced pluripotent stem cells (iPSCs), generated by genetic reprogramming of cells from the patient’s own somatic tissues could be a possible alternative source, the safety of the use of iPSCs for reproductive purposes has still not been sufficiently investigated. However, regardless of PSCs or SSCs as the cell type of origin, the most challenging spermatogenic process to mimic in vitro is the process of meiosis, the process by which genetically different haploid spermatids are produced via two successive meiotic cell divisions. Although several studies reported generation of round spermatid-like cells in vitro using SSCs (97, 98, 100, 101), only a few studies (98, 101) investigated the key meiotic events that are required for successful meiosis and true in vitro-derived gametes (104). When mouse SSCs were induced to complete meiosis, using immortalized Sertoli cells as feeder layer in a three-step induced culture system, despite the in vitro formation of pachytene-like spermatocytes, the homologous chromosomes did not display full synapsis and no meiotic crossovers were formed. Despite these meiotic problems, many cells still proceeded to the first meiotic division (MI), occasionally even forming round spermatid-like cells (98). Apparently, these aberrant spermatocytes were not timely eliminated by the meiotic checkpoints that normally (in vivo) induce apoptosis in order to prevent possible generation of aneuploid sperm (105). Because of a lack of meiotic crossovers in the in vitro-generated pachytene spermatocytes, the MI-spermatocytes did not form chiasmata (physical connection between the homologous chromosomes) and thus displayed univalent (pairs of sister chromatids) instead of bivalent (pairs of homologous chromosomes) chromosome pairs, which will almost certainly cause subsequent formation of aneuploid spermatids. Meanwhile, Sun et al. reported complete chromosome synapsis and meiotic crossover formation in vitro using human male germline stem cells (101). However, the detection of chiasmata in MI-spermatocytes was not described.
The difficulty in mimicking spermatogenesis in vitro could be due to the lack of a suitable testicular micro‐environment needed to successfully support the spermatogenic process. Spermatogenesis in vivo requires spatio-temporal interactions between germ cells and testicular somatic cells. Therefore, including an in vitro somatic niche in culture could be instrumental in supporting the germ cells. In mice and humans several essential regulators, such as retinoic acid and gonadotropins, have been identified and are appropriately discussed in reviews such as that of Rombaut and colleagues (106).
Testicular somatic cells, including peritubular myoid cells, Leydig cells, Sertoli cells and endothelial cells, as well as immune cells, play important roles in supporting spermatogenesis. Unlike in vitro spermatogenesis, in ex vivo spermatogenesis methods where testicular fragments are cultured instead of isolated cells, testicular organization is still in place during culture, thereby circumventing the need to structurally re-create the right microenvironment.
The first successful ex vivo spermatogenesis was demonstrated in 2011 (45, 107). In this study, murine testicular fragments were cultured at a gas-liquid interphase using an agarose stand and ex vivo elongated spermatids were identified after five weeks of tissue culture and could be used for the generation of live offspring. Repeating this method for rat immature testicular tissue resulted in progression of spermatogenesis up to round spermatids (108). Studies using human testicular tissue for ex vivo spermatogenesis showed no initiation of meiosis (109, 110). Using histological analysis, one study reported that spermatids could be observed morphologically in ex vivo cultures with human testis tissue (111). No studies using human or non-human primate tissues describe the production of spermatids that were shown to be capable of fertilization.
Despite the achievements that have been reached with ex vivo spermatogenesis using tissue culture, the efficiency to perform ex vivo spermatogenesis is very low. More research is required to improve the efficiency and further translation of the method for primate testicular tissue.
More fundamental knowledge on non-human primate and human spermatogenesis and the differences with that of rodents will be helpful to successfully mimic this process through either in vitro or ex vivo spermatogenesis.
As any medical procedure may involve short- or long-term health risks, it is important to consider the impact that taking testicular biopsies may have on the patient. In many cases, the patient’s parent(s) or caretaker(s) will have to make the decision for the child to undergo this procedure and they will have to be duly informed of its potential risks, including those of the general anesthesia under which the procedure is performed. These risks however may be limited by concomitant execution of other medical procedures that are inherent to the initial (cancer) treatment, such as central venous line placement or bone marrow aspiration. Worries about the risks of the biopsy itself comprise the chance of acute post-operative complications and chronic changes within the testicular tissue. Post-operative complications include post-operative bleeding and wound infection. Rates of occurrence of these complications are low and vary between 0 – 3.8% (112–115). Transiently, extra- and intra-testicular hematomas may occur (112). Additionally, when the testicular biopsy is performed, to reduce the burden of repeated anesthesia, as an additional procedure during general anesthesia for a concomitant procedure, such as central venous line placement or bone marrow aspiration, this may lead to increased post-operative pain levels compared to the main procedure only, potentially necessitating additional analgesics in these patients (116).
Unilateral testicular biopsies of a maximum of 1 ml or 50% of total testicular volume of pre-pubertal patients did not lead to decreased growth, compared to the contralateral non-biopsied testis, as measured by ultrasound during one year after surgery (112). In 6.3% of the patients fibrotic testicular lesions were found using ultrasound as part of a long-term follow up (112). Although gonadotrophin levels have been observed to be abnormal in the follow-up of pre- and peri-pubertal boys from whom a testicular biopsy was taken, the incidence of these abnormal levels matches those of childhood cancer survivors who have not undergone testicular biopsies (113). Similarly, results of semen analyses of patients who had undergone testicular biopsies prior to treatment (88% of which with alkylating agents) showed comparable rates of normo-, oligo- and azoospermia to large-scale cohort studies of childhood cancer survivors (113).
In conclusion, the reproductive health of patients undergoing testicular biopsies does not seem to decline as a result of the testis biopsy procedure.
For fertility preservation, the biopsies are subsequently stored in nitrogen. Although cryopreserved tissue function has been shown to be similar to that of fresh tissue, as described above, the effect of cryopreservation on the genetic and epigenetic status of cells within cryopreserved tissues is largely unknown. Despite the availability of studies on molecular changes on cryopreserved sperm, oocytes and embryos (117), studies researching the effect of cryopreservation on SSCs are limited. In mice, SSCT was as successful with freshly isolated germ cells as with germ cells isolated from long-term cryopreserved tissues, in establishing spermatogenesis in recipient animals and subsequent fertilization of murine oocytes through ICSI (118). The resulting offspring did not show a significant amount of DNA copy number changes (chromosomal deletions, duplications) nor changes in DNA methylation patterns in whole-genome DNA analysis of liver tissue, compared to the offspring of wild-type mice, obtained through natural conception, suggesting that potential DNA methylation changes of SSCs in cryopreserved tissues are reversed upon transplantation, or in the offspring.
A major concern with regard to SSCT in cancer patients is the possible reintroduction of malignant cells from the original biopsy during the autotransplantation of (cultured) testicular cells to the patient who recovered from cancer. Research data indicate that acute lymphoblastic leukemia (ALL) cells do not survive for longer than 16 days in a human testicular cell culture system (119). In vitro propagation to increase SSC numbers prior to autotransplantation therefore seems to have the additional benefit of eliminating ALL-cells, although this may not be the case for other cancer types. Further purification methods, such as immunomagnetic bead-based sorting (MACS) using a combination of spermatogonial markers showed promising results in a mouse model (120). In contrast, the use of Percoll density gradients appeared insufficient to prevent transmission of leukemic cells to the recipient mice (120). Fluorescence-activated cell sorting (FACS) also showed promising results in experiments with human cells. The combined use of the markers EPCAM, HLA-ABC and CD49e allowed the separation of putative spermatogonia and leukemic cells prior to xenotransplantation. Tumor formation was only observed in the testis of transplanted animals having received the leukemic fraction, and tumors were absent in animals receiving the spermatogonia-enriched fraction, whilst the latter also showed the highest number of spermatogonial colonies (121). However, such separations are highly dependent on knowledge of specific antigens that are different between spermatogonial and cancerous cells. However, cancer cells are known to express many genes that are normally only present within germ cells (122, 123), including antigens that may be used for sorting (124). In addition, purification strategies need to be highly reliable, as only 20 leukemic cells are needed to cause a terminal relapse, as was shown in a rat model (125). Any patient-specific aberrations in tumor antigen expression could therefore be disastrous, when relying on the selection of cells by specific antigens. Prior to cell enrichment, a thorough characterization of the cancer cells from every individual patient would thus be pertinent.
Although a valid concern for cell culture and manipulation in general, the culture of human SSCs does not seem to induce chromosomal abnormalities (126, 127). This is similar to the genetic stability of long-term (over two years, with an approximate 1085-fold expansion) cultured murine SSCs, which have been shown to maintain their androgenic imprint based on five imprinted regions and their capacity for spermatogenesis after transplantation, leading to fertile progeny (128). DNA methylation patterns of cultured human testicular cells, sorted for ITGA6, do not overlap with those found in seminomas (127), indicating cultured cells do not acquire a seminoma signature. However, specific DNA demethylation patterns of paternally imprinted genes and concurrent DNA hypermethylation of maternally imprinted genes were observed in cultured and enriched human spermatogonia (126). It is currently unknown whether these changes are correlated with the fertilization potential of spermatozoa that would be derived from these spermatogonia, or whether they pose a health risk towards the patient or their offspring, although studies in mice do not seem to indicate this (129, 130).
As there has not yet been a clinical trial investigating the application of SSCT in human patients, no information regarding the long-term health in such patients is available. However, research in mice shows no difference in life-span up to the age of 18 months between transplanted and non-transplanted groups of busulfan-treated animals, with no increase in the occurrence of malignancies (129). Regarding the offspring, in non-human primates the sperm derived from the donor-SSCs after non-cultured SSC allogenic transplantation has been shown to possess competence for fertilization via ICSI and subsequent embryonic development up to the morula stage (52, 53). However, (epi)genetic stability of these embryos was not assessed and no progeny was derived in this study, limiting assessment of safety in respect to health of the procedure in primates. Nevertheless, in mice the long-term follow up of two generations of progeny of transplant-recipient mice showed no significant differences compared to the control group regarding congenital abnormalities, childhood development, lifespan, reproductive health and adult general health (130). These findings are supported by studies in multiple mouse strains reporting no chromosomal abnormalities or genetic deviations (131). To add, no changes were seen in DNA methylation patterns in spermatozoa derived from animals receiving SSCT of non-cryopreserved and uncultured spermatogonia, nor in spermatozoa and somatic cells of multiple organs of their first and second generation offspring (132). However, a later study on transplantation of freshly isolated spermatogonia did find that the expression pattern of histone lysines H4K5ac and H4K8ac in murine germ cells in the various stages of the seminiferous cycle in testicular tissue of animals having received SSCT differed from that in controls, as analyzed by immunohistochemistry. However, H3K4me3, H3K9ac, H4K12ac and H4K16ac of germ cells after SSCT were comparable to controls, as was their DNA methylation status (133).
As clinical trials have not commenced, no safety studies concerning human testicular grafts have been performed. While the main focus of studies has been to achieve spermatogenesis within the graft, little has been published regarding the (epi)genetic stability of the procedure and the cells within the graft, for both the recipient and his progeny. In mice, graft-derived spermatids showed normal fertilization capacity and progeny derived from graft-derived sperm showed good reproductive health, as demonstrated through mating experiments (10). However, general health of this offspring was not assessed.
Studies in testicular grafts of rhesus macaques showed good fertilization potential of graft-derived spermatids through testicular sperm extraction and intracytoplasmic sperm injection (TESE-ICSI), with good embryonic development up to blastocyst stage (34) and even a healthy live birth of one female (43). Behavior assessments of this graft-derived infant were age-appropriate and no health defects were reported by the authors (43).
Although these results are promising, the basis of the presumed safety of this procedure is still very small. Using immunohistochemistry markers on mouse tissue, it was shown that, compared to wildtype animals, testicular grafting does not alter the spermatogenic methylation patterns of germ cells in various stages of the seminiferous cycle as analyzed by immunohistochemistry analysis of expression of DNA methyltransferases -1 and -3A and 5-methylcytosine (DNMT1, DNMT3A and 5-MC) (133). Concerning histone modifications on H3K4me3, H3K9ac, H4K5ac, H4K8ac, H4K12ac and H4K16ac, only H4K5ac showed a significant difference in expression pattern between grafted and control groups (133).
To our knowledge, there are currently no other studies on the genetic integrity of spermatids derived from (xeno)grafts. Similarly, reports on assessment of the health of the recipient and their progeny are limited. Furthermore, in anticipation of the clinical use of this technique, it will be essential to ascertain whether the placement of an autologous graft from a cancer patient with potential infiltration of systemic cancer in the testis will pose a risk of recurrence of the cancer after grafting. Further studies are needed to establish reliable methods to secure transplantations of cancer-free materials only (5).
For in vitro or ex vivo spermatogenesis, one of the foremost risks is the derivation of sperm with an incorrect number of chromosomes, referred to as aneuploidy. An estimated 20–40% of all human conceptions contains aneuploid cells, of which most are due to errors in meiosis (134). In all males, a proportion of sperm can be observed to be aneuploid (135, 136). Approximately 3–5% of spermatozoa of males with proven fertility are aneuploid, and these levels are significantly higher in infertile men (136–139). Aneuploidy in gametes may cause early pregnancy loss or severe developmental defects (140, 141). Meiotic problems, like incomplete chromosome synapsis and impaired recombination, are considered to be main factors in the emergence of chromosome nondisjunction and subsequent aneuploidy (138, 142, 143). In order to prevent generation of aneuploid sperm, key meiotic events such as chromosome synapsis, recombination and subsequent meiotic crossover formation, are strictly monitored by checkpoint mechanisms to timely arrest meiotic progression (105, 144). However, when spermatogenesis was re-created in vitro using mouse SSCs, these checkpoint mechanisms did not appear to be functional (98). For ex vivo cultures of testicular fragments, most meiotic events or meiotic checkpoint function have not yet been systemically investigated. Since meiotic DNA damage repair, recombination and checkpoints appear not to be fully functional in current in vitro spermatogenesis protocols, these processes should be thoroughly investigated and monitored before considering clinical application of in vitro or ex vivo spermatogenesis.
Epigenetic modifications, being DNA methylation, histone modification and the production of small non-coding RNAs, regulate many processes of spermatogenesis. Besides spermiogenesis, also spermatogonial differentiation steps and early meiosis are subject to epigenetic regulation. For example, some changes in DNA methylation may occur in spermatogonia and early spermatocytes (145). These processes include meiotic silencing of unsynapsed chromosomes (MSUC), XY-body formation and the histone-to-protamine transition during spermiogenesis (146). These are all crucial for germ cell function and post-fertilization embryonic development (147). The establishment of epigenetic patterns during human germ cell development is dynamic and age and phase-dependent (148, 149). Aberrant DNA methylation during spermatogenesis may impair spermatogenesis, causing infertility (150). Despite post-fertilization (embryonic) epigenetic reprogramming as a mean to prevent aberrant progeny, successful fertilization of oocytes with epigenetically abnormal sperm may still pose a risk, because some abnormal epigenetic marks may persist (151) and potentially influence embryonic development and health of the offspring. In couples experiencing recurrent pregnancy loss, sperm showed more DNA methylation abnormalities of multiple imprinted genes, compared to couples without history of miscarriage or infertility (152), illustrating the potential detrimental effect of epigenetic aberrations in germ cell function.
Moreover, long-term culture of primary cells in general may induce changes in DNA methylation (153). Therefore, when spermatogenesis is re-created in vitro, the epigenetic status has often been investigated to make sure that no aberrant epigenetic patterns are present in the in vitro-derived germ cells (100, 101).
When murine SSCs were used as a starting point to generate a multipotent adult GSC line (maGSCs) that could subsequently be induced to differentiate into haploid cells, these haploid cells showed incomplete epigenetic imprinting of the H19 gene (100). Nevertheless, a study using human male germline stem cells reported normal epigenetic status in round spermatids-like cells (101).
The research on epigenetic status of cells derived from testicular tissue cultures is limited. The short-term culture of rat fetal testicular tissue shows a similar chronology in epigenetic remodeling of DNA methylation patterns in gonocytes, compared to gonocytes in vivo (154). Similarly, Yokonishi et al. described a normal state of methylation in the offspring derived from the culture of cryopreserved mouse testicular tissue fragments (46). However, DNA methylation is not the only type of epigenetic regulation and more studies are needed to ascertain the epigenetic properties of cultured SSCs, spermatocytes and spermatids.
Unlike ex vivo spermatogenesis, in which formation of elongated spermatids could be achieved that could be used for ICSI (45, 46, 155), differentiation of human SSCs by cell culture has so far, to our knowledge, only produced round spermatid-like cells (97, 98, 100, 101). Round spermatid injection (ROSI) technique would enable the use of these immature haploid precursors of spermatozoa to fertilize oocytes and give rise to the offspring. However, some concerns regarding ROSI have arisen as round spermatids and elongated spermatids have significant differences in chromatin structure (156). The use of round spermatids may not establish proper imprints or global methylation, thereby affecting embryonic development (157). Although in two human studies the children born after ROSI appeared healthy without any epigenetic problems (158, 159), data from a mouse study described that, in contrast of the normal paternal genome derived from mature spermatozoa, embryos derived from round spermatids appeared to have genome-wide aberrant DNA methylation of their paternal genome (160). This likely explains the observed poor development of these embryos. In addition, the overall low success rates of ROSI (161) compared with TESE-ICSI with testicular elongated spermatids may further limit the application of ROSI for human fertility restoration. Therefore, further development of these round spermatids or spermatid-like cells into more mature elongated spermatids is crucial for future clinical application.
As the patient population of pre-pubertal boys who have been offered fertility preservation slowly reaches their reproductive age, the issue of offering fertility restoration becomes more urgent. The research on SSCT, tissue grafting and in vitro spermatogenesis has concomitantly matured over the years and currently applications are on the horizon. For SSCT, the proof of principle has been delivered through allotransplantations in monkeys and the safety of the procedure has been extensively studied in mice. Potential for further optimization lays in in vitro human SSC propagation to increase their numbers and to purge cell suspensions of cancerous cells prior to transplantation. The use of testicular tissue grafting is also within reach of the clinic, with the existence of an autologous non-human primate model having resulted in healthy offspring. Challenges for grafting still exist with regard to the harvest of sufficient amounts of testicular tissue for grafting, the prevention of apoptosis within these grafts and assessment of the genomic status of the graft derived spermatids, as well as finding ways of preventing reintroduction of cancerous cells to the patient from within the grafted tissues.
Although spermatogenesis in vitro or ex vivo, either through cell or tissue culture, shows great promise with regard to the controlled production of spermatids, complete spermatogenesis with human tissue or isolated SSCs has not yet been indisputably achieved. Along with major concerns surrounding the genomic stability of haploid spermatid (like) cells generated by in vitro spermatogenesis, this technique does not yet seem ready for clinical application.
Despite these challenges, as continuous achievements are made within this field of research, all three avenues towards fertility restoration remain worth pursuing. To ensure progress, it is necessary to explore all options for tissue resources in preclinical research, although we should remain vigilant of the effect the tissue of choice has on study outcomes. In addition, more fundamental research towards identification of reliable in vivo and in vitro markers for identification and confirmation of testicular cell populations is vital for progress in this field.
For current and future patients, we should seek to offer comprehensible up-to-date and honest information on the progress of potential fertility preservation and restoration strategies and to inquire as to their wishes regarding these procedures.
IS and JM took the lead in drafting this manuscript, all other co-authors (JE, QL, AM, AAM, GH, AP, and CM) wrote sections of this manuscript. AAM, AM, AP, and JE contributed substantially to the cryopreservation section, while QL and GH contributed substantially in the (safety of) in vitro or ex vivo spermatogenesis. JM designed the figure and table. All authors took part in critical review and revising of the manuscript and approved the final version.
This research was funded by ZonMW TAS, grant number 116003002. QL is the recipient of a China Scholarship Council grant (201706300107).
The authors declare that the research was conducted in the absence of any commercial or financial relationships that could be construed as a potential conflict of interest.
All claims expressed in this article are solely those of the authors and do not necessarily represent those of their affiliated organizations, or those of the publisher, the editors and the reviewers. Any product that may be evaluated in this article, or claim that may be made by its manufacturer, is not guaranteed or endorsed by the publisher.
1. Mulder RL, Font-Gonzalez A, Green DM, Loeffen EAH, Hudson MM, Loonen J, et al. Fertility Preservation for Male Patients With Childhood, Adolescent, and Young Adult Cancer: Recommendations From the PanCareLIFE Consortium and the International Late Effects of Childhood Cancer Guideline Harmonization Group. Lancet Oncol (2021) 22(2):e57–67. doi: 10.1016/S1470-2045(20)30582-9
2. Hess R, de Franca L. Spermatogenesis and Cycle of the Seminiferous Epithelium. In: Adv Exp Med Biol (2008) p:1–15. doi: 10.1007/978-0-387-09597-4_1
3. Allen CM, Lopes F, Mitchell RT, Spears N. How Does Chemotherapy Treatment Damage the Prepubertal Testis? Reproduction (2018) 156(6): R209–33. doi: 10.1530/REP-18-0221
4. Anderson RA, Mitchell RT, Kelsey TW, Spears N, Telfer EE, Wallace WHB. Cancer Treatment and Gonadal Function: Experimental and Established Strategies for Fertility Preservation in Children and Young Adults. Lancet Diabetes Endocrinol (2015) 3(7):556–67. doi: 10.1016/S2213-8587(15)00039-X
5. Goossens E, Jahnukainen K, Mitchell R, van Pelt A, Pennings G, Rives N, et al. Fertility Preservation in Boys: Recent Developments and New Insights †. Hum Reprod Open (2020) 2020(3):1–18. doi: 10.1093/hropen/hoaa016
6. Sohni A, Tan K, Song HW, Burow D, de Rooij DG, Laurent L, et al. The Neonatal and Adult Human Testis Defined at the Single-Cell Level. Cell Rep (2019) 26(6):1501–1517.e4. doi: 10.1016/j.celrep.2019.01.045
7. Gaskell TL, Esnal A, Robinson LLL, Anderson RA, Saunders PTK. Immunohistochemical Profiling of Germ Cells Within the Human Fetal Testis: Identification of Three Subpopulations. Biol Reprod (2004) 71(6):2012–21. doi: 10.1095/biolreprod.104.028381
8. Anderson RA, Fulton N, Cowan G, Coutts S, Saunders PTK. Conserved and Divergent Patterns of Expression of DAZL, VASA and OCT4 in the Germ Cells of the Human Fetal Ovary and Testis. BMC Dev Biol (2007) 7:1–10. doi: 10.1186/1471-213X-7-136
9. Nowroozi MR, Ahmadi H, Rafiian S, Mirzapour T, Movahedin M. In Vitro Colonization of Human Spermatogonia Stem Cells: Effect of Patient’s Clinical Characteristics and Testicular Histologic Findings. Urology (2011) 78(5):1075–81. doi: 10.1016/j.urology.2011.06.035
10. Schlatt S, Honaramooz A, Boiani M, Schöler HR, Dobrinski I. Progeny From Sperm Obtained After Ectopic Grafting of Neonatal Mouse Testes. Biol Reprod (2003) 68(6):2331–5. doi: 10.1095/biolreprod.102.014894
11. Schneider F, Neuhaus N, Wistuba J, Zitzmann M, Heß J, Mahler D, et al. Testicular Functions and Clinical Characterization of Patients With Gender Dysphoria (GD) Undergoing Sex Reassignment Surgery (SRS). J Sex Med (2015) 12(11):2190–200. doi: 10.1111/jsm.13022
12. Vereecke G, Defreyne J, Van Saen D, Collet S, Van Dorpe J, T’Sjoen G, et al. Characterisation of Testicular Function and Spermatogenesis in Transgender Women. Hum Reprod (2021) 36(1):5–15. doi: 10.1093/humrep/deaa254
13. He Y, Wang J, Ren J, Zhao Y, Chen J, Chen X. Effect of COVID-19 on Male Reproductive System – A Systematic Review. Front Endocrinol (Lausanne) (2021) 12:677701. doi: 10.3389/fendo.2021.677701
14. de Nie I, Mulder CL, Meißner A, Schut Y, Holleman EM, van der Sluis WB, et al. Histological Study on the Influence of Puberty Suppression and Hormonal Treatment on Developing Germ Cells in Transgender Women. Hum Reprod (2021) 37(2):deab240. doi: 10.1093/humrep/deab240
15. Lopes F, Tholeti P, Adiga SK, Anderson RA, Mitchell RT, Spears N. Chemotherapy Induced Damage to Spermatogonial Stem Cells in Prepubertal Mouse In Vitro Impairs Long-Term Spermatogenesis. Toxicol Rep (2021) 8:114–23. doi: 10.1016/j.toxrep.2020.12.023
16. Grewenig A, Schuler N, Rübe CE. Persistent DNA Damage in Spermatogonial Stem Cells After Fractionated Low-Dose Irradiation of Testicular Tissue. Int J Radiat Oncol Biol Phys (2015) 92(5):1123–31. doi: 10.1016/j.ijrobp.2015.04.033
17. Tharmalingam MD, Matilionyte G, Wallace WHB, Stukenborg JB, Jahnukainen K, Oliver E, et al. Cisplatin and Carboplatin Result in Similar Gonadotoxicity in Immature Human Testis With Implications for Fertility Preservation in Childhood Cancer. BMC Med (2020) 18(1):1–16. doi: 10.1186/s12916-020-01844-y
18. Rajpert-De Meyts E, Hanstein R, Jørgensen N, Græm N, Vogt PH, Skakkebæk NE. Developmental Expression of POU5F1 (OCT-3/4) in Normal and Dysgenetic Human Gonads. Hum Reprod (2004) 19(6):1338–44. doi: 10.1093/humrep/deh265
19. Onofre J, Baert Y, Faes K, Goossens E. Cryopreservation of Testicular Tissue or Testicular Cell Suspensions: A Pivotal Step in Fertility Preservation. Hum Reprod Update (2016) 22(6):744–61. doi: 10.1093/humupd/dmw029
20. Onofre J, Kadam P, Baert Y, Goossens E. Testicular Tissue Cryopreservation Is the Preferred Method to Preserve Spermatogonial Stem Cells Prior to Transplantation. Reprod BioMed Online (2020) 40(2):261–9. doi: 10.1016/j.rbmo.2019.10.016
21. Keros V, Rosenlund B, Hultenby K, Aghajanova L, Levkov L, Hovatta O. Optimizing Cryopreservation of Human Testicular Tissue: Comparison of Protocols With Glycerol, Propanediol and Dimethylsulphoxide as Cryoprotectants. Hum Reprod (2005) 20(6):1676–87. doi: 10.1093/humrep/deh797
22. Baert Y, Goossens E, Van Saen D, Ning L, In’T Veld P, Tournaye H. Orthotopic Grafting of Cryopreserved Prepubertal Testicular Tissue: In Search of a Simple Yet Effective Cryopreservation Protocol. Fertil Steril (2012) 97(5):1152–7.e2. doi: 10.1016/j.fertnstert.2012.02.010
23. Rives-Feraille A, Liard A, Bubenheim M, Barbotin AL, Giscard d’Estaing S, Mirallié S, et al. Assessment of the Architecture and Integrity of Frozen-Thawed Testicular Tissue From (Pre)Pubertal Boys With Cancer. Andrology (2021) 1–12. doi: 10.1111/andr.13116
24. Keros V, Hultenby K, Borgström B, Fridström M, Jahnukainen K, Hovatta O. Methods of Cryopreservation of Testicular Tissue With Viable Spermatogonia in Pre-Pubertal Boys Undergoing Gonadotoxic Cancer Treatment. Hum Reprod (2007) 22(5):1384–95. doi: 10.1093/humrep/del508
25. Wyns C, Curaba M, Martinez-Madrid B, Van Langendonckt A, François-Xavier W, Donnez J. Spermatogonial Survival After Cryopreservation and Short-Term Orthotopic Immature Human Cryptorchid Testicular Tissue Grafting to Immunodeficient Mice. Hum Reprod (2007) 22(6):1603–11. doi: 10.1093/humrep/dem062
26. Curaba M, Verleysen M, Amorim CA, Dolmans M-M, Van Langendonckt A, Hovatta O, et al. Cryopreservation of Prepubertal Mouse Testicular Tissue by Vitrification. Fertil Steril (2011) 95(4):1229–34.e1. doi: 10.1016/j.fertnstert.2010.04.062
27. Babayev SN, Arslan E, Kogan S, Moy F, Oktay K. Evaluation of Ovarian and Testicular Tissue Cryopreservation in Children Undergoing Gonadotoxic Therapies. J Assist Reprod Genet (2013) 30(1):3–9. doi: 10.1007/s10815-012-9909-5
28. Poels J, Van Langendonckt A, Many M-C, Wese F-X, Wyns C. Vitrification Preserves Proliferation Capacity in Human Spermatogonia. Hum Reprod (2013) 28(3):578–89. doi: 10.1093/humrep/des455
29. Ginsberg JP, Li Y, Carlson CA, Gracia CR, Hobbie WL, Miller VA, et al. Testicular Tissue Cryopreservation in Prepubertal Male Children: An Analysis of Parental Decision-Making. Pediatr Blood Cancer (2014) 61(9):1673–8. doi: 10.1002/pbc.25078
30. Ho WLC, Bourne H, Gook D, Clarke G, Kemertzis M, Stern K, et al. A Short Report on Current Fertility Preservation Strategies for Boys. Clin Endocrinol (Oxf) (2017) 87(3):279–85. doi: 10.1111/cen.13377
31. Valli-Pulaski H, Peters KA, Gassei K, Steimer SR, Sukhwani M, Hermann BP, et al. Testicular Tissue Cryopreservation: 8 Years of Experience From a Coordinated Network of Academic Centers. Hum Reprod (2019) 34(6):966–77. doi: 10.1093/humrep/dez043
32. Baert Y, Braye A, Struijk RB, Van Pelt AMM, Goossens E. Cryopreservation of Testicular Tissue Before Long-Term Testicular Cell Culture Does Not Alter In Vitro Cell Dynamics. Fertil Steril (2015) 104(5):1244–52.e4. doi: 10.1016/j.fertnstert.2015.07.1134
33. Malekzadeh M, Takzaree N, Toolee H, Kazemzadeh S, Khanmohammadi N, Solhjoo S, et al. Cryoprotective Effect of Pentoxifylline on Spermatogonial Stem Cell During Transplantation Into Azoospermic Torsion Mouse Model. Reprod Sci (2022) 29(2):526–39. doi: 10.1007/s43032-021-00729-6
34. Honaramooz A, Li MW, Penedo MCT, Meyers S, Dobrinski I. Accelerated Maturation of Primate Testis by Xenografting Into Mice. Biol Reprod (2004) 70(5):1500–3. doi: 10.1095/biolreprod.103.025536
35. Kaneko H, Kikuchi K, Nakai M, Somfai T, Noguchi J, Tanihara F, et al. Generation of Live Piglets for the First Time Using Sperm Retrieved From Immature Testicular Tissue Cryopreserved and Grafted Into Nude Mice. PloS One (2013) 8(7):1–8. doi: 10.1371/journal.pone.0070989
36. Kaneko H, Kikuchi K, Men NT, Nakai M, Noguchi J, Kashiwazaki N, et al. Production of Sperm From Porcine Fetal Testicular Tissue After Cryopreservation and Grafting Into Nude Mice. Theriogenology (2017) 91:154–62. doi: 10.1016/j.theriogenology.2016.12.036
37. Honaramooz A, Snedaker A, Boiani M, Schöler H, Dobrinski I, Schlatt S. Sperm From Neonatal Mammalia Testes Grafted in Mice. Nature (2002) 418(6899):778–81. doi: 10.1038/nature00918
38. Ntemou E, Kadam P, Van Saen D, Wistuba J, Mitchell RT, Schlatt S, et al. Complete Spermatogenesis in Intratesticular Testis Tissue Xenotransplants From Immature Non-Human Primate. Hum Reprod (2019) 34(3):403–13. doi: 10.1093/humrep/dey373
39. Jahnukainen K, Ehmcke J, Nurmio M, Schlatt S. Autologous Ectopic Grafting of Cryopreserved Testicular Tissue Preserves the Fertility of Prepubescent Monkeys That Receive Sterilizing Cytotoxic Therapy. Cancer Res (2012) 72(20):5174–8. doi: 10.1158/0008-5472.CAN-12-1317
40. Shinohara T, Inoue K, Ogonuki N, Kanatsu-Shinohara M, Miki H, Nakata K, et al. Birth of Offspring Following Transplantation of Cyropreserved Immature Testicular Pieces and in-Vitro Microinsemination. Hum Reprod (2002) 17(12):3039–45. doi: 10.1093/humrep/17.12.3039
41. Van Saen D, Goossens E, De Block G, Tournaye H. Regeneration of Spermatogenesis by Grafting Testicular Tissue or Injecting Testicular Cells Into the Testes of Sterile Mice: A Comparative Study. Fertil Steril (2009) 91(5 SUPPL.):2264–72. doi: 10.1016/j.fertnstert.2008.02.100
42. Van Saen D, Goossens E, Aerts JL, Haentjens P, Tournaye H. Does Early Cell Death Cause Germ Cell Loss After Intratesticular Tissue Grafting? Fertil Steril (2013) 99(5):1264–1272.e1. doi: 10.1016/j.fertnstert.2012.12.019
43. Fayomi AP, Peters K, Sukhwani M, Valli-Pulaski H, Shetty G, Meistrich ML, et al. Autologous Grafting of Cryopreserved Prepubertal Rhesus Testis Produces Sperm and Offspring. Sci (80-) (2019) 363(6433):1314–9. doi: 10.1126/science.aav2914
44. Van Saen D, Goossens E, Haentjens P, Baert Y, Tournaye H. Exogenous Administration of Recombinant Human FSH Does Not Improve Germ Cell Survival in Human Prepubertal Xenografts. Reprod BioMed Online (2013) 26(3):286–98. doi: 10.1016/j.rbmo.2012.11.013
45. Sato T, Katagiri K, Gohbara A, Inoue K, Ogonuki N, Ogura A, et al. In Vitro Production of Functional Sperm in Cultured Neonatal Mouse Testes. Nature (2011) 471(7339):504–8. doi: 10.1038/nature09850
46. Yokonishi T, Sato T, Komeya M, Katagiri K, Kubota Y, Nakabayashi K, et al. Offspring Production With Sperm Grown In Vitro From Cryopreserved Testis Tissues. Nat Commun (2014) 5:1–6. doi: 10.1038/ncomms5320
47. Portela JMD, de Winter-Korver CM, van Daalen SKM, Meißner A, de Melker AA, Repping S, et al. Assessment of Fresh and Cryopreserved Testicular Tissues From (Pre)Pubertal Boys During Organ Culture as a Strategy for In Vitro Spermatogenesis. Hum Reprod (2019) 34(12):2443–55. doi: 10.1093/humrep/dez180
48. Gul M, Hildorf S, Dong L, Thorup J, Hoffmann ER, Jensen CFS, et al. Review of Injection Techniques for Spermatogonial Stem Cell Transplantation. Hum Reprod Update (2020) 26(3):368–91. doi: 10.1093/humupd/dmaa003
49. Brinster RL, Zimmermannt JW. Spermatogenesis Following Male Germ-Cell Transplantation (Spermatogonia/Stem Cd/Testes/Nsc Mice). Proc Nat Acad Sci USA (1994) 91(24):11298–302. doi: 10.1073/pnas.91.24.11298
50. Brinster RL, Avarbock MR. Germline Transmission of Donor Haplotype Following Spermatogonial Transplantation (Testis/Tem Cels/Spermaoneis/Transec Mice/Fertty). Dev Biol (1994) 91(24):11303–7. doi: 10.1073/pnas.91.24.11303
51. Takashima S, Shinohara T. Culture and Transplantation of Spermatogonial Stem Cells. Stem Cell Res (2018) 29:46–55. doi: 10.1016/j.scr.2018.03.006
52. Hermann BP, Sukhwani M, Winkler F, Pascarella JN, Peters KA, Sheng Y, et al. Spermatogonial Stem Cell Transplantation Into Rhesus Testes Regenerates Spermatogenesis Producing Functional Sperm. Cell Stem Cell (2012) 11(5):715–26. doi: 10.1016/j.stem.2012.07.017
53. Shetty G, Mitchell JM, Lam TNA, Phan TT, Zhang J, Tailor RC, et al. Postpubertal Spermatogonial Stem Cell Transplantation Restores Functional Sperm Production in Rhesus Monkeys Irradiated Before and After Puberty. Andrology (2021) :1–14. doi: 10.1111/andr.13033
54. Radford JA. Is Prevention of Sterility Possible in Men? Ann Oncol (2000) 11(SUPPL. 3):173–4. doi: 10.1093/annonc/11.suppl_3.173
55. Nagano MC. Homing Efficiency and Proliferation Kinetics of Male Germ Line Stem Cells Following Transplantation in Mice. Biol Reprod (2003) 69(2):701–7. doi: 10.1095/biolreprod.103.016352
56. Mirzapour T, Movahedin M, Koruji M, Nowroozi MR. Xenotransplantation Assessment: Morphometric Study of Human Spermatogonial Stem Cells in Recipient Mouse Testes. Andrologia (2015) 47(6):626–33. doi: 10.1111/and.12310
57. Dobrinski I, Ogawa T, Avarbock MR, Brinster RL. Computer Assisted Image Analysis to Assess Colonization of Recipient Seminiferous Tubules by Spermatogonial Stem Cells From Transgenic Donor Mice. Mol Reprod Dev (1999) 53(2):142–8. doi: 10.1002/(SICI)1098-2795(199906)53:2<142::AID-MRD3>3.0.CO;2-O
58. Kanatsu-Shinohara M, Ogonuki N, Inoue K, Miki H, Ogura A, Toyokuni S, et al. Long-Term Proliferation in Culture and Germline Transmission of Mouse Male Germline Stem Cells. Biol Reprod (2003) 69(2):612–6. doi: 10.1095/biolreprod.103.017012
59. Sadri-Ardekani H, Mizrak SC, Van Daalen SKM, de Winter-Korver CM, Roepers-gajadien HL, Koruji M, et al. Propagation of Human Spermatogonial. Am Med Assoc (2009) 302(19):2127–34. doi: 10.1001/jama.2009.1689
60. Sadri-Ardekani H, Akhondi MM, van der veen F, Repping S, van Pelt AMM. In Vitro Propagation of Human Prepubertal Spermatogonial Stem Cells. JAMA (2011) 305(23):2416–8. doi: 10.1001/jama.2011.791
61. Kossack N, Terwort N, Wistuba J, Ehmcke J, Schlatt S, Schöler H, et al. A Combined Approach Facilitates the Reliable Detection of Human Spermatogonia In Vitro. Hum Reprod (2013) 28(11):3012–25. doi: 10.1093/humrep/det336
62. Struijk RB, Mulder CL, van Daalen SKM, de Winter-Korver CM, Jongejan A, Repping S, et al. Itga6+ Human Testicular Cell Populations Acquire a Mesenchymal Rather Than Germ Cell Transcriptional Signature During Long-Term Culture. Int J Mol Sci (2020) 21(21):1–20. doi: 10.3390/ijms21218269
63. Bhang DH, Kim BJ, Kim BG, Schadler K, Baek KH, Kim YH, et al. Testicular Endothelial Cells Are a Critical Population in the Germline Stem Cell Niche. Nat Commun (2018) 9(1):1–16. doi: 10.1038/s41467-018-06881-z
64. Chen B, Wang YB, Zhang ZL, Xia WL, Wang HX, Xiang ZQ, et al. Xeno-Free Culture of Human Spermatogonial Stem Cells Supported by Human Embryonic Stem Cell-Derived Fibroblast-Like Cells. Asian J Androl (2009) 11(5):557–65. doi: 10.1038/aja.2009.21
65. Gat I, Maghen L, Filice M, Wyse B, Zohni K, Jarvi K, et al. Optimal Culture Conditions Are Critical for Efficient Expansion of Human Testicular Somatic and Germ Cells In Vitro. Fertil Steril (2017) 107(3):595–605.e7. doi: 10.1016/j.fertnstert.2016.12.028
66. Izadyar F, Wong J, Maki C, Pacchiarotti J, Ramos T, Howerton K, et al. Identification and Characterization of Repopulating Spermatogonial Stem Cells From the Adult Human Testis. Hum Reprod (2011) 26(6):1296–306. doi: 10.1093/humrep/der026
67. Valli H, Sukhwani M, Dovey SL, Peters KA, Donohue J, Castro CA, et al. FACS and MACS Sorting Strategies to Isolate and Enrich Human Spermatogonial Stem Cells. Fertil Steril (2014) 102(2):566–80. doi: 10.1016/j.fertnstert.2014.04.036
68. Medrano JV, Rombaut C, Simon C, Pellicer A, Goossens E. Human Spermatogonial Stem Cells Display Limited Proliferation In Vitro Under Mouse Spermatogonial Stem Cell Culture Conditions. Fertil Steril (2016) 106(6):1539–49.e8. doi: 10.1016/j.fertnstert.2016.07.1065
69. Piravar Z, Jeddi-tehrani M, Sadeghi MR, Mohazzab A, Eidi A, Akhondi MM. Original Article, Vol. 14 Tehran: Avicenna Research Insitute (ARI), part of the Academic Center for Education, Culture and Research (ACECR) (2013) pp. 17–22.
70. Von Kopylow K, Kirchhoff C, Jezek D, Schulze W, Feig C, Primig M, et al. Screening for Biomarkers of Spermatogonia Within the Human Testis: A Whole Genome Approach. Hum Reprod (2010) 25(5):1104–12. doi: 10.1093/humrep/deq053
71. Smith JF, Yango P, Altman E, Choudhry S, Poelzl A, Zamah AM, et al. Testicular Niche Required for Human Spermatogonial Stem Cell Expansion. Stemcells Transl Med Express (2014) 3:1043–54. doi: 10.5966/sctm.2014-0045
72. Mohammadzadeh E, Mirzapour T, Nowroozi MR, Nazarian H, Piryaei A, Alipour F, et al. Differentiation of Spermatogonial Stem Cells by Soft Agar Three-Dimensional Culture System. Artif Cells Nanomed Biotechnol (2019) 47(1):1772–81. doi: 10.1080/21691401.2019.1575230
73. Kim YH, Kang HG, Kim BJ, Jung SE, Karmakar PC, Kim SM, et al. Enrichment and In Vitro Culture of Spermatogonial Stem Cells From Pre-Pubertal Monkey Testes. Tissue Eng Regener Med (2017) 14(5):557–66. doi: 10.1007/s13770-017-0058-x
74. Guo Y, Liu L, Sun M, Hai Y, Li Z, He Z. Expansion and Long-Term Culture of Human Spermatogonial Stem Cells via the Activation of SMAD3 and AKT Pathways. Exp Biol Med (2015) 240(8):1112–22. doi: 10.1177/1535370215590822
75. He Z, Kokkinaki M, Jiang J, Dobrinski I, Dym M. Isolation, Characterization, and Culture of Human Spermatogonia. Biol Reprod (2010) 82(2):363–72. doi: 10.1095/biolreprod.109.078550
76. Lim JJ, Sung SY, Kim HJ, Song SH, Hong JY, Yoon TK, et al. Long-Term Proliferation and Characterization of Human Spermatogonial Stem Cells Obtained From Obstructive and Non-Obstructive Azoospermia Under Exogenous Feeder-Free Culture Conditions. Cell Prolif (2010) 43:405–17. doi: 10.1111/j.1365-2184.2010.00691.x
77. Eildermann K, Gromoll J, Behr R. Misleading and Reliable Markers to Differentiate Between Primate Testis-Derived Multipotent Stromal Cells and Spermatogonia in Culture. Hum Reprod (2012) 27(6):1754–67. doi: 10.1093/humrep/des091
78. Abdul Wahab AY, Muhammad ML, Ramli R. Spermatogonial Stem Cells Protein Identification in In Vitro Culture From Non-Obstructive Azoospermia Patient. Malaysian J Med Sci (2016) 23(3):40–8.
79. Altman E, Yango P, Moustafa R, Smith JF, Klatsky PC, Tran ND. Characterization of Human Spermatogonial Stem Cell Markers in Fetal, Pediatric, and Adult Testicular Tissues. Reproduction (2014) 148(4):417–27. doi: 10.1530/REP-14-0123
80. Guo F, Yan L, Guo H, Li L, Hu B, Zhao Y, et al. The Transcriptome and DNA Methylome Landscapes of Human Primordial Germ Cells. Cell (2015) 161(6):1437–52. doi: 10.1016/j.cell.2015.05.015
81. Guo J, Grow EJ, Mlcochova H, Maher GJ, Lindskog C, Nie X, et al. The Adult Human Testis Transcriptional Cell Atlas. Cell Res (2018) 28(12):1141–57. doi: 10.1038/s41422-018-0099-2
82. Di Persio S, Tekath T, Siebert-Kuss LM, Cremers J, Li X, Meyer G, et al. EGR4-Dependent Decrease of UTF1 Is Associated With Failure to Reserve Spermatogonial Stem Cells in Infertile Men. bioRxiv (2021). doi: 10.1101/2021.02.02.429371
83. Di Persio S, Saracino R, Fera S, Muciaccia B, Esposito V, Boitani C, et al. Spermatogonial Kinetics in Humans. Development (2017) 144(19):3430–9. doi: 10.1242/dev.150284
84. European Medicines Agency (EMA). Legal Framework: Advanced Therapies [Internet]. Available at: https://www.ema.europa.eu/en/human-regulatory/overview/advanced-therapies/legal-framework-advanced-therapies.
85. Rossi P, Dolci S. Paracrine Mechanisms Involved in the Control of Early Stages of Mammalian Spermatogenesis. Front Endocrinol (Lausanne) (2013) 4:1–8. doi: 10.3389/fendo.2013.00181
86. Voigt AL, Thiageswaran S, de Lima E Martins Lara N, Dobrinski I. Metabolic Requirements for Spermatogonial Stem Cell Establishment and Maintenance In Vivo and In Vitro. Int J Mol Sci (2021) 22(4):1–20. doi: 10.3390/ijms22041998
87. Murdock MH, David S, Swinehart IT, Reing JE, Tran K, Gassei K, et al. Human Testis Extracellular Matrix Enhances Human Spermatogonial Stem Cell Survival In Vitro. Tissue Eng Part A (2019) 25(7–8):663–76. doi: 10.1089/ten.tea.2018.0147
88. Kokkinaki M, Djourabtchi A. Long-Term Culture of Human SSEA-4 Positive Spermatogonial Stem Cells (SSCs). J Stem Cell Res Ther (2011) 2(2):2488. doi: 10.4172/2157-7633.S2-003
89. Kurek M, Åkesson E, Yoshihara M, Oliver E, Cui Y, Becker M, et al. Spermatogonia Loss Correlates With LAMA 1 Expression in Human Prepubertal Testes Stored for Fertility Preservation. Cells (2021) 10(2):241. doi: 10.3390/cells10020241
90. Schlatt S, Westernströer B, Gassei K, Ehmcke J. Donor-Host Involvement in Immature Rat Testis Xenografting Into Nude Mouse Hosts. Biol Reprod (2010) 82(5):888–95. doi: 10.1095/biolreprod.109.082073
91. Eggermont J, Donnez J, Casanas-Roux F, Scholtes H, Van Langendonckt A. Time Course of Pelvic Endometriotic Lesion Revascularization in a Nude Mouse Model. Fertil Steril (2005) 84(2):492–9. doi: 10.1016/j.fertnstert.2005.03.034
92. Liu Z, Nie YH, Zhang CC, Cai YJ, Wang Y, Lu HP, et al. Generation of Macaques With Sperm Derived From Juvenile Monkey Testicular Xenografts. Cell Res (2016) 26(1):139–42. doi: 10.1038/cr.2015.112
93. Ntemou E, Kadam P, Van Laere S, Van Saen D, Vicini E, Goossens E. Effect of Recombinant Human Vascular Endothelial Growth Factor on Testis Tissue Xenotransplants From Prepubertal Boys: A Three-Case Study. Reprod BioMed Online (2019) 39(1):119–33. doi: 10.1016/j.rbmo.2019.02.012
94. Luetjens CM, Stukenborg JB, Nieschlag E, Simoni M, Wistuba J. Complete Spermatogenesis in Orthotopic But Not in Ectopic Transplants of Autologously Grafted Marmoset Testicular Tissue. Endocrinology (2008) 149(4):1736–47. doi: 10.1210/en.2007-1325
95. Hutka M, Kadam P, Van Saen D, Homer NZM, Onofre J, Wallace WHB, et al. Fertility Preservation in Childhood Cancer: Endocrine Activity in Prepubertal Human Testis Xenografts Exposed to a Pubertal Hormone Environment. Cancers (Basel) (2020) 12(10):1–21. doi: 10.3390/cancers12102830
96. Hutka M, Smith LB, Goossens E, Wallace WHB, Stukenborg J-B, Mitchell RT. Exogenous Gonadotrophin Stimulation Induces Partial Maturation of Human Sertoli Cells in a Testicular Xenotransplantation Model for Fertility Preservation. J Clin Med (2020) 9(1):266. doi: 10.3390/jcm9010266
97. Feng LX, Chen Y, Dettin L, Reijo Pera RA, Herr JC, Goldberg E, et al. Generation and In Vitro Differentiation of a Spermatogonial Cell Line. Science (80-) (2002) 297(5580):392–5. doi: 10.1126/science.1073162
98. Lei Q, Lai X, Eliveld J, Chuva de Sousa Lopes SM, van Pelt AMM, Hamer G. In Vitro Meiosis of Male Germline Stem Cells. Stem Cell Rep (2020) 15(5):1140–53. doi: 10.1016/j.stemcr.2020.10.006
99. Lei Q, Zhang E, van Pelt AMM, Hamer G. Meiotic Chromosome Synapsis and XY-Body Formation In Vitro. Front Endocrinol (Lausanne) (2021) 12:1–11. doi: 10.3389/fendo.2021.761249
100. Nolte J, Michelmann HW, Wolf M, Wulf G, Nayernia K, Meinhardt A, et al. PSCDGs of Mouse Multipotent Adult Germline Stem Cells can Enter and Progress Through Meiosis to Form Haploid Male Germ Cells In Vitro. Differentiation (2010) 80(4–5):184–94. doi: 10.1016/j.diff.2010.08.001
101. Sun M, Yuan Q, Niu M, Wang H, Wen L, Yao C, et al. Efficient Generation of Functional Haploid Spermatids From Human Germline Stem Cells by Three-Dimensional-Induced System. Cell Death Differ (2018) 25(4):747–64. doi: 10.1038/s41418-017-0015-1
102. Ishikura Y, Ohta H, Sato T, Murase Y, Yabuta Y, Kojima Y, et al. In Vitro Reconstitution of the Whole Male Germ-Cell Development From Mouse Pluripotent Stem Cells. Cell Stem Cell (2021) 28(12):2167–79.e9. doi: 10.1016/j.stem.2021.08.005
103. Zhou Q, Wang M, Yuan Y, Wang X, Fu R, Wan H, et al. Complete Meiosis From Embryonic Stem Cell-Derived Germ Cells In Vitro. Cell Stem Cell (2016) 18(3):330–40. doi: 10.1016/j.stem.2016.01.017
104. Handel MA, Eppig JJ, Schimenti JC. Applying “Gold Standards” to in-Vitro-Derived Germ Cells. Cell (2014) 157(6):1257–61. doi: 10.1016/j.cell.2014.05.019
105. Subramanian VV, Hochwagen A. The Meiotic Checkpoint Network: Step-By-Step Through Meiotic Prophase. Cold Spring Harb Perspect Biol (2014) 6(10). doi: 10.1101/cshperspect.a016675
106. Rombaut C, Mertes H, Heindryckx B, Goossens E. Human In Vitro Spermatogenesis From Pluripotent Stem Cells: In Need of a Stepwise Differentiation Protocol? Mol Hum Reprod (2018) 24(2):47–54:a016675. doi: 10.1093/molehr/gax065
107. Sato T, Katagiri K, Yokonishi T, Kubota Y, Inoue K, Ogonuki N, et al. In Vitro Production of Fertile Sperm From Murine Spermatogonial Stem Cell Lines. Nat Commun (2011) 2:472. doi: 10.1038/ncomms1478
108. Reda A, Hou M, Winton TR, Chapin RE, Söder O, Stukenborg J-B. In Vitro Differentiation of Rat Spermatogonia Into Round Spermatids in Tissue Culture. Mol Hum Reprod (2016) 22(9):601–12. doi: 10.1093/molehr/gaw047
109. Portela JMD, Mulder CL, van Daalen SKM, de Winter-Korver CM, Stukenborg JB, Repping S, et al. Strains Matter: Success of Murine In Vitro Spermatogenesis Is Dependent on Genetic Background. Dev Biol (2019) 456(1):25–30. doi: 10.1016/j.ydbio.2019.08.007
110. de Michele F, Poels J, Weerens L, Petit C, Evrard Z, Ambroise J, et al. Preserved Seminiferous Tubule Integrity With Spermatogonial Survival and Induction of Sertoli and Leydig Cell Maturation After Long-Term Organotypic Culture of Prepubertal Human Testicular Tissue. Hum Reprod (2017) 32(1):32–45. doi: 10.1093/humrep/dew300
111. De Michele F, Poels J, Vermeulen M, Ambroise J, Gruson D, Guiot Y, et al. Haploid Germ Cells Generated in Organotypic Culture of Testicular Tissue From Prepubertal Boys. Front Physiol (2018) 9:1–18. doi: 10.3389/fphys.2018.01413
112. Uijldert M, Meißner A, De Melker AA, Van Pelt AMM, Van De Wetering MD, Van Rijn RR, et al. Development of the Testis in Pre-Pubertal Boys With Cancer After Biopsy for Fertility Preservation. Hum Reprod (2017) 32(12):2366–72. doi: 10.1093/humrep/dex306
113. Kanbar M, De Michele F, Giudice MG, Desmet L, Poels J, Wyns C. Long-Term Follow-Up of Boys Who Have Undergone a Testicular Biopsy for Fertility Preservation. Hum Reprod (2021) 36(1):26–39. doi: 10.1093/humrep/deaa281
114. Wyns C, Curaba M, Petit S, Vanabelle B, Laurent P, Wese JFX, et al. Management of Fertility Preservation in Prepubertal Patients: 5 Years’ Experience at the Catholic University of Louvain. Hum Reprod (2011) 26(4):737–47. doi: 10.1093/humrep/deq387
115. Brodigan K, Kapadia M, Frazier AL, Laufer MR, Yu R, Weil BR, et al. Safety of Surgical Fertility Preservation Procedures in Children Prior to Hematopoietic Stem Cell Transplant. Transplant Cell Ther (2021) 27(8):696. doi: 10.1016/j.jtct.2021.04.001
116. Borgström B, Fridström M, Gustafsson B, Ljungman P, Rodriguez-Wallberg KA. A Prospective Study on the Long-Term Outcome of Prepubertal and Pubertal Boys Undergoing Testicular Biopsy for Fertility Preservation Prior to Hematologic Stem Cell Transplantation. Pediatr Blood Cancer (2020) 67(9):1–11. doi: 10.1002/pbc.28507
117. Estudillo E, Jiménez A, Bustamante-Nieves PE, Palacios-Reyes C, Velasco I, López-Ornelas A. Cryopreservation of Gametes and Embryos and Their Molecular Changes. Int J Mol Sci (2021) 22(19):10864. doi: 10.3390/ijms221910864
118. Wu X, Goodyear SM, Abramowitz LK, Bartolomei MS, Tobias JW, Avarbock MR, et al. Fertile Offspring Derived From Mouse Spermatogonial Stem Cells Cryopreserved for More Than 14 Years. Hum Reprod (2012) 27(5):1249–59. doi: 10.1093/humrep/des077
119. Sadri-Ardekani H, Homburg CH, Van Capel TMM, Van Den Berg H, van der Veen F, van der Schoot CE, et al. Eliminating Acute Lymphoblastic Leukemia Cells From Human Testicular Cell Cultures: A Pilot Study. Fertil Steril (2014) 101(4):1072–1078.e1. doi: 10.1016/j.fertnstert.2014.01.014
120. Tian J, Ma K, Bin PC, SH Z, Li X, Zhou Y, et al. Relative Safety of Various Spermatogenic Stem Cell Purification Methods for Application in Spermatogenic Stem Cell Transplantation. Stem Cell Res Ther (2019) 10(1):1–12. doi: 10.1186/s13287-019-1481-9
121. Dovey SL, Valli H, Hermann BP, Sukhwani M, Donohue J, Castro CA, et al. Eliminating Malignant Contamination From Therapeutic Human Spermatogonial Stem Cells. J Clin Invest (2013) 123(4):1833–43. doi: 10.1172/JCI65822
122. Bruggeman JW, Koster J, Lodder P, Repping S, Hamer G. Massive Expression of Germ Cell-Specific Genes Is a Hallmark of Cancer and a Potential Target for Novel Treatment Development. Oncogene (2018) 37(42):5694–700. doi: 10.1038/s41388-018-0357-2
123. Bruggeman JW, Irie N, Lodder P, van Pelt AMM, Koster J, Hamer G. Tumors Widely Express Hundreds of Embryonic Germline Genes. Cancers (Basel) (2020) 12(12):1–16. doi: 10.3390/cancers12123812
124. Whitehurst AW. Cause and Consequence of Cancer/Testis Antigen Activation in Cancer. Annu Rev Pharmacol Toxicol (2014) 54:251–72. doi: 10.1146/annurev-pharmtox-011112-140326
125. Jahnukainen K, Hou M, Petersen C, Setchell B, Söder O. Intratesticular Transplantation of Testicular Cells From Leukemic Rats Causes Transmission of Leukemia. Cancer Res (2001) 61(2):706–10.
126. Nickkholgh B, Mizrak SC, Van Daalen SKM, Korver CM, Sadri-Ardekani H, Repping S, et al. Genetic and Epigenetic Stability of Human Spermatogonial Stem Cells During Long-Term Culture. Fertil Steril (2014) 102(6):1700–7.e1. doi: 10.1016/j.fertnstert.2014.08.022
127. Struijk RB, Dorssers LCJ, Henneman P, Rijlaarsdam MA, Venema A, Jongejan A, et al. Comparing Genome-Scale DNA Methylation and CNV Marks Between Adult Human Cultured ITGA6+ Testicular Cells and Seminomas to Assess In Vitro Genomic Stability. PloS One (2020) 15(3):1–18. doi: 10.1371/journal.pone.0230253
128. Kanatsu-Shinohara M, Ogonuki N, Iwano T, Lee J, Kazuki Y, Inoue K, et al. Genetic and Epigenetic Properties of Mouse Male Germline Stem Cells During Long-Term Culture. Development (2005) 132(18):4155–63. doi: 10.1242/dev.02004
129. Mulder CL, Catsburg LAE, Zheng Y, Winter-Korver CMD, Daalen SKMV, van Wely M, et al. Long-Term Health in Recipients of Transplanted In Vitro Propagated Spermatogonial Stem Cells. Hum Reprod (2018) 33(1):81–90. doi: 10.1093/humrep/dex348
130. Serrano JB, van Eekelen R, de Winter-Korver CM, van Daalen SKM, Tabeling NC, Catsburg LAE, et al. Impact of Restoring Male Fertility With Transplantation of In Vitro Propagated Spermatogonial Stem Cells on the Health of Their Offspring Throughout Life. Clin Transl Med (2021) 11(10):1–6. doi: 10.1002/ctm2.531
131. Goossens E, De Vos P, Tournaye H. Array Comparative Genomic Hybridization Analysis Does Not Show Genetic Alterations in Spermatozoa and Offspring Generated After Spermatogonial Stem Cell Transplantation in the Mouse. Hum Reprod (2010) 25(7):1836–42. doi: 10.1093/humrep/deq108
132. Goossens E, De Rycke M, Haentjens P, Tournaye H. DNA Methylation Patterns of Spermatozoa and Two Generations of Offspring Obtained After Murine Spermatogonial Stem Cell Transplantation. Hum Reprod (2009) 24(9):2255–63. doi: 10.1093/humrep/dep213
133. Goossens E, Bilgec T, Van Saen D, Tournaye H. Mouse Germ Cells Go Through Typical Epigenetic Modifications After Intratesticular Tissue Grafting. Hum Reprod (2011) 26(12):3388–400. doi: 10.1093/humrep/der334
134. Nagaoka SI, Hassold TJ, Hunt PA. Human Aneuploidy: Mechanisms and New Insights Into an Age-Old Problem. Nat Rev Genet (2012) 13(7):493–504. doi: 10.1038/nrg3245
135. Shi Q, Martin RH. Aneuploidy in Human Sperm: A Review of the Frequency and Distribution of Aneuploidy, Effects of Donor Age and Lifestyle Factors. Cytogenet Cell Genet (2000) 90(3–4):219–26. doi: 10.1159/000056773
136. Tempest HG, Homa ST, Dalakiouridou M, Christopikou D, Wright D, Zhai XP, et al. The Association Between Male Infertility and Sperm Disomy: Evidence for Variation in Disomy Levels Among Individuals and a Correlation Between Particular Semen Parameters and Disomy of Specific Chromosome Pairs. Reprod Biol Endocrinol (2004) 2:1–9. doi: 10.1186/1477-7827-2-82
137. Ioannou D, Fortun J, Tempest HG. Meiotic Nondisjunction and Sperm Aneuploidy in Humans. Reprod (2019) 157(1):R15–31. doi: 10.1530/REP-18-0318
138. Lu S, Zong C, Fan W, Yang M, Li J, Chapman AR, et al. Probing Meiotic Recombination and Aneuploidy of Single Sperm Cells by Whole-Genome Sequencing. Sci (80-) (2012) 338(6114):1627–30. doi: 10.1126/science.1229112
139. Pang MG, Hoegerman SF, Cuticchia AJ. Hybridization in Spermatozoa From Nine Patients With Oligoasthenoteratozoospermia Undergoing Intracytoplasmic Sperm Injection. Hum Reprod (1999) 14(5):1266–73. doi: 10.1093/humrep/14.5.1266
140. Hassold T, Hunt P. To Err (Meiotically) Is Human: The Genesis of Human Aneuploidy. Nat Rev Genet (2001) 2(4):280–91. doi: 10.1038/35066065
141. Ramasamy R, Scovell JM, Kovac JR, Cook PJ, Lamb DJ, Lipshultz LI. Fluorescence in Situ Hybridization Detects Increased Sperm Aneuploidy in Men With Recurrent Pregnancy Loss. Fertil Steril (2015) 103(4):906–9. doi: 10.1016/j.fertnstert.2015.01.029
142. Ferguson KA, Wong EC, Chow V, Nigro M, Ma S. Abnormal Meiotic Recombination in Infertile Men and Its Association With Sperm Aneuploidy. Hum Mol Genet (2007) 16(23):2870–9. doi: 10.1093/hmg/ddm246
143. Hassold T, Hall H, Hunt P. The Origin of Human Aneuploidy: Where We Have Been, Where We Are Going. Hum Mol Genet (2007) 16(R2):203–8. doi: 10.1093/hmg/ddm243
144. Jan SZ, Jongejan A, Korver CM, van Daalen SKM, van Pelt AMM, Repping S, et al. Distinct Prophase Arrest Mechanisms in Human Male Meiosis. Dev (2018) 145(16):dev160614. doi: 10.1242/dev.160614
145. Oakes CC, La Salle S, Smiraglia DJ, Robaire B, Trasler JM. Developmental Acquisition of Genome-Wide DNA Methylation Occurs Prior to Meiosis in Male Germ Cells. Dev Biol (2007) 307(2):368–79. doi: 10.1016/j.ydbio.2007.05.002
146. Jan SZ, Hamer G, Repping S, de Rooij DG, Van Pelt AMM, Vormer TL. Molecular Control of Rodent Spermatogenesis. Biochim Biophys Acta Mol Basis Dis (2012) 1822(12):1838–50. doi: 10.1016/j.bbadis.2012.02.008
147. Kota SK, Feil R. Epigenetic Transitions in Germ Cell Development and Meiosis. Dev Cell (2010) 19(5):675–86. doi: 10.1016/j.devcel.2010.10.009
148. Ly L, Chan D, Trasler JM. Developmental Windows of Susceptibility for Epigenetic Inheritance Through the Male Germline. Semin Cell Dev Biol (2015) 43:96–105. doi: 10.1016/j.semcdb.2015.07.006
149. Rotondo JC, Lanzillotti C, Mazziotta C, Tognon M, Martini F. Epigenetics of Male Infertility: The Role of DNA Methylation. Front Cell Dev Biol (2021) 9:689624. doi: 10.3389/fcell.2021.689624
150. Di Persio S, Leitão E, Wöste M, Tekath T, Cremers JF, Dugas M, et al. Whole-Genome Methylation Analysis of Testicular Germ Cells From Cryptozoospermic Men Points to Recurrent and Functionally Relevant DNA Methylation Changes. Clin Epigenetics (2021) 13(1):1–19. doi: 10.1186/s13148-021-01144-z
151. Smith ZD, Chan MM, Humm KC, Karnik R, Mekhoubad S, Regev A, et al. DNA Methylation Dynamics of the Human Preimplantation Embryo. Nature (2014) 511(7511):611–5. doi: 10.1038/nature13581
152. Khambata K, Raut S, Deshpande S, Mohan S, Sonawane S, Gaonkar R, et al. DNA Methylation Defects in Spermatozoa of Male Partners From Couples Experiencing Recurrent Pregnancy Loss. Hum Reprod (2021) 36(1):48–60. doi: 10.1093/humrep/deaa278
153. Franzen J, Georgomanolis T, Selich A, Kuo CC, Stöger R, Brant L, et al. DNA Methylation Changes During Long-Term In Vitro Cell Culture Are Caused by Epigenetic Drift. Commun Biol (2021) 4(1):1–12. doi: 10.1038/s42003-021-02116-y
154. Rwigemera A, Joao F, Delbes G. Fetal Testis Organ Culture Reproduces the Dynamics of Epigenetic Reprogramming in Rat Gonocytes. Epigenet Chromatin (2017) 10(1):1–14. doi: 10.1186/s13072-017-0127-3
155. Perrard MH, Sereni N, Schluth-Bolard C, Blondet A, Giscard d’Estaing S, Plotton I, et al. Complete Human and Rat Ex Vivo Spermatogenesis From Fresh or Frozen Testicular Tissue. Biol Reprod (2016) 95(4):1–10. doi: 10.1095/biolreprod.116.142802
156. Kimmins S, Sassone-Corsi P. Chromatin Remodelling and Epigenetic Features of Germ Cells. Nature (2005) 434(7033):583–9. doi: 10.1038/nature03368
157. Carrell DT. Epigenetics of the Male Gamete. Fertil Steril (2012) 97(2):267–74. doi: 10.1016/j.fertnstert.2011.12.036
158. Tanaka A, Suzuki K, Nagayoshi M, Tanaka A, Takemoto Y, Watanabe S, et al. Ninety Babies Born After Round Spermatid Injection Into Oocytes: Survey of Their Development From Fertilization to 2 Years of Age. Fertil Steril (2018) 110(3):443–51. doi: 10.1016/j.fertnstert.2018.04.033
159. Tanaka A, Nagayoshi M, Takemoto Y, Tanaka I, Kusunoki H, Watanabe S, et al. Erratum: Fourteen Babies Born After Round Spermatid Injection Into Human Oocytes. Proc Natl Acad Sci USA (2015) 112:14629–34. doi: 10.1073/pnas.1517466112
160. Kishigami S, Van Thuan N, Hikichi T, Ohta H, Wakayama S, Mizutani E, et al. Epigenetic Abnormalities of the Mouse Paternal Zygotic Genome Associated With Microinsemination of Round Spermatids. Dev Biol (2006) 289(1):195–205. doi: 10.1016/j.ydbio.2005.10.026
Keywords: spermatogonial stem cells, fertility preservation, fertility restoration, childhood cancer, preclinical research, spermatogonial stem cell transplantation, testicular grafting, in vitro spermatogenesis
Citation: Sanou I, van Maaren J, Eliveld J, Lei Q, Meißner A, de Melker AA, Hamer G, van Pelt AMM and Mulder CL (2022) Spermatogonial Stem Cell-Based Therapies: Taking Preclinical Research to the Next Level. Front. Endocrinol. 13:850219. doi: 10.3389/fendo.2022.850219
Received: 07 January 2022; Accepted: 07 March 2022;
Published: 04 April 2022.
Edited by:
Jan-Bernd Stukenborg, Karolinska Institutet (KI), SwedenReviewed by:
Francesca Mancuso, University of Perugia, ItalyCopyright © 2022 Sanou, van Maaren, Eliveld, Lei, Meißner, de Melker, Hamer, van Pelt and Mulder. This is an open-access article distributed under the terms of the Creative Commons Attribution License (CC BY). The use, distribution or reproduction in other forums is permitted, provided the original author(s) and the copyright owner(s) are credited and that the original publication in this journal is cited, in accordance with accepted academic practice. No use, distribution or reproduction is permitted which does not comply with these terms.
*Correspondence: Callista L. Mulder, Yy5sLm11bGRlckBhbXN0ZXJkYW11bWMubmw=
†These authors have contributed equally to this work and share first authorship
Disclaimer: All claims expressed in this article are solely those of the authors and do not necessarily represent those of their affiliated organizations, or those of the publisher, the editors and the reviewers. Any product that may be evaluated in this article or claim that may be made by its manufacturer is not guaranteed or endorsed by the publisher.
Research integrity at Frontiers
Learn more about the work of our research integrity team to safeguard the quality of each article we publish.