- 1Jilin Ginseng Academy, Key Laboratory of Active Substances and Biological Mechanisms of Ginseng Efficacy, Ministry of Education, Jilin Provincial Key Laboratory of Bio-Macromolecules of Chinese Medicine, Changchun University of Chinese Medicine, Changchun, China
- 2College of Laboratory Medicine, Jilin Medical University, Jilin City, China
Diabetic kidney disease (DKD) is a severe microvascular complication in patients with diabetes and is one of the main causes of renal failure. The current clinical treatment methods for DKD are not completely effective, and further exploration of the molecular mechanisms underlying the pathology of DKD is necessary to improve and promote the treatment strategy. Sirtuins are class III histone deacetylases, which play an important role in many biological functions, including DNA repair, apoptosis, cell cycle, oxidative stress, mitochondrial function, energy metabolism, lifespan, and aging. In the last decade, research on sirtuins and DKD has gained increasing attention, and it is important to summarize the relationship between DKD and sirtuins to increase the awareness of DKD and improve the cure rates. We have found that miRNAs, lncRNAs, compounds, or drugs that up-regulate the activity and expression of sirtuins play protective roles in renal function. Therefore, in this review, we summarize the biological functions, molecular targets, mechanisms, and signaling pathways of SIRT1–SIRT7 in DKD models. Existing research has shown that sirtuins have the potential as effective targets for the clinical treatment of DKD. This review aims to lay a solid foundation for clinical research and provide a theoretical basis to slow the development of DKD in patients.
1 Introduction
Diabetes mellitus (DM) is a metabolic disorder with chronic microvascular and macrovascular complications. DM is one of the most problematic health issues of the 21st century due to its severe complications. DM affects approximately 451 million people worldwide and is projected to reach 693 million by 2045 (1). NAD+ plays a key role in redox and energy metabolism. NAD+ acts as a co-substrate in the deacetylation reactions of sirtuins, and the regulation of the NAD+-sirtuins axis is a pivotal pathway for the new therapies of metabolic diseases (2). Moreover, in different renal disease models, such as diabetic kidney disease (DKD), sirtuins have been proven to regulate anti-fibrosis and anti-oxidative stress functions, and maintain the glomerular barrier integrity (3). DKD, diabetic retinopathy, and diabetic peripheral neuropathy are the main complications of DM, among which DKD has attracted worldwide attention due to its high incidence (20%–40% in diabetic patients) and poor prognosis (4, 5). DKD is a chronic disease that leads to renal failure; the treatments for rena0l failure are dialysis and kidney transplantation (6). However, once the disease progresses to end-stage renal disease, the course of this disease is both uncontrollable and irreversible ( (7). Although many researchers have studied the molecular mechanism of DKD and attempted to improve treatment strategies, DKD remains a clinically intractable complication of DM.
Histone deacetylases (HDACs) in eukaryotes are divided into IV classes, among which the I, II, and IV groups depend on Zn2+, whereas class III sirtuins depend on NAD+ to exert catalytic activity (8). The sirtuin family is classified into SIRT1–SIRT7 based on differences in the core structural domain, all of which catalyze the deacetylation of Nℇ-acyl-lysine on histone and non-histone substrates ( (9, 10). SIRT1, SIRT6, and SIRT7 are mainly found in the nucleus, SIRT2 is localized in the cytoplasm, and SIRT3, SIRT4, and SIRT5 are found in mitochondria, and their positions are not fixed (11). Sirtuins are involved in the regulation of various biological activities, including DNA repair, apoptosis, cell cycle, oxidative stress, metabolism, lifespan, and aging (12, 13). Based on biological regulatory functions, many studies have shown that the sirtuin family has therapeutic effects in many diseases. Sirtuins are pharmacological targets in neurodegenerative diseases, including Alzheimer’s disease, Parkinson’s disease, and Huntington’s disease (14). Moreover, the regulation of sirtuins reveals a complex network of cellular metabolism and will provide clues for the diagnosis, treatment, and prevention of cancer (15). Additionally, as mitochondrial sirtuins affect many aspects of mitochondrial metabolism and signal transduction, targeting sirtuins may represent a potential therapeutic target to combat age-related mitochondrial recession (16). Through reviewing the literature, we found many studies on sirtuins and DKD, but a lack of systematic and detailed summaries. Therefore, in this review, we have first introduced the biological regulatory functions of SIRT1–SIRT7 in DKD animal and cell models. Subsequently, we have summarized the signaling pathways for treating DKD with various treatments, and finally, examined the differences and clinical implications of sirtuins in DKD studies.
2 Search Strategy
Data for this review were identified by searching PubMed and Web of Science using the search terms “histone deacetylase”, “sirtuins”, “SIRT”, “diabetic nephropathy”, “diabetic kidney disease”, and “diabetic complication” for collecting articles from 2004 to 2021, with the language limited to English.
3 Pathological Process of DKD
The pathogenesis of DKD is multifactorial, involving structural, physiological, hemodynamic, and inflammatory processes, which ultimately lead to a decreased glomerular filtration rate (17). Hyperglycemia and hypertension are critical factors in the development of DKD (17). Proteinuria is an important factor in the development of DKD, which is directly and predictably associated with kidney damage (18). Proteinuria results from an abnormal permeability function of the glomerular filtration barrier, which consists of three layers of glomerular endothelial cells, the glomerular basement membrane (GBM), and podocytes (19). DKD is a microvascular complication of DM that develops from micro-proteinuria to massive proteinuria, ultimately leading to end-stage renal disease (18). Importantly, metabolic and hemodynamic changes in DM cause ultrastructural changes in the glomerular filtration barrier, including podocyte foot process fusion and separation, GBM thickening, reduction of endothelial cell glycocalyx, accumulation of mesangial extracellular matrix, and glomerular sclerosis, all of which are directly related to the increase in proteinuria (20).
3.1 Relationship Between the Expression of SIRT1–SIRT7 and DKD
The important role of SIRT1 has been demonstrated by the enhanced mitochondrial damage in SIRT1 knockdown mice with DM, and its role in maintaining kidney cell homeostasis under mitochondrial stress or damage (21). Moreover, in advanced glycation end products (AGE)-treated rat primary glomerular mesangial cells (GMCs), investigators found that the overexpression of SIRT1 protected against reactive oxygen species (ROS) production and fibrosis by enhancing the Keap1/Nrf2/ARE pathway (22). Additionally, under the condition of HG-induced HK-2 cells, the deacetylase activity of SIRT1 decreased and resulted in renal tubular injury induced by the SIRT1/NF-κB/microR-29/Keap1 signaling pathway (23).
Furthermore, a reduction in the NAD+/NADH ratio has been shown to induce a decrease in SIRT3 activity and enhance mitochondrial oxidative stress in a DKD rat model (24). Another investigator found that the overexpression of SIRT3 antagonizes apoptosis in HG-induced HK-2 cells via the AKT/FOXO1 and AKT/FOXO3a signaling pathways (25). Similarly, in a streptozotocin (STZ)-induced mouse model, high expression of SIRT3 inhibited aberrant glycolysis and prevented fibrosis via the activation of PKM2 dimer formation and HIF-1α accumulation (26). Moreover, in HG-induced endothelial cells, the overexpression of SIRT3 activated the AMPK/SIRT3 pathway to sustain redox balance and alleviate vascular inflammation (27).
A previous report indicated that the overexpression of SIRT4 reduced the inflammatory effect and restrained apoptosis and the production of ROS in HG-induced mouse podocytes via the mitochondrial pathway (28).
In HG-induced podocytes, the overexpression of SIRT6 reduced mitochondrial dysfunction and apoptosis by activating the AMPK pathway (29). Another report illustrated that overexpression of SIRT6 promoted M2 macrophage transformation and alleviated kidney injury in in vivo and in vitro DKD models by upregulating the expression of Bcl−2 and CD206, and reducing the expression of Bax and CD86 (30). Additionally, another study demonstrated that in db/db mice and AGE/HG-induced human podocytes, overexpression of SIRT6 showed anti-apoptosis and anti-inflammatory effects by inhibiting the Notch pathway (31).
Taken together, these findings indicate that the overexpression of SIRT1, SIRT3, SIRT4, and SIRT6 reduces the biological impairment of kidney function in DKD models.
3.2 Gene Polymorphism and Clinical Research of Sirtuins in DKD
Human gene polymorphism plays an important role in elucidating the susceptibility and tolerance of the human body to diseases and poisons, the diversity of clinical manifestations of diseases, and the response to drug therapy (32–34). Studies have shown that SIRT1 and FOXO1 play important roles in the pathogenesis of DKD. Single nucleotide polymorphisms were analyzed by including 1066 patients with type 2 diabetes (T2DM) (413 without DKD and 653 with DKD), and the results indicated that the SIRT1 gene variant rs10823108 and the FoxO1 gene variant rs17446614 may be associated with DKD in patients with T2DM (35). Another study of gene polymorphisms suggested that, among 1016 patients with T2DM (388 without DKD and 628 with DKD), the transcriptional coactivator p300 rs20551 polymorphism is associated with the development of DKD, and the SIRT1 polymorphism is related to albumin-creatinine ratio progression (36). The researchers analyzed changes in serum vash-1 and other biomarkers in 692 patients with T2DM, and found that the UACR, VASH-1, HbA1c, ESR, CRP, VEGF, HIF-1α, TNF-α, and TGF-β1 levels in all patient groups were significantly higher, and the SIRT1 levels were lower compared to healthy controls. These findings indicated that serum VASH-1 may be associated with the expression of renal inflammation and fibrosis-related factors and have a potential connection with DKD (37). Another two-center, randomized study evaluated 117 patients with stage 2–4 DKD who were treated with sevelamer carbonate. The results showed that sevelamer carbonate increased anti-inflammatory defenses, including nuclear factor like-2, AGE receptor 1, and SIRT1, and decreased pro-inflammatory cytokines, such as TNF receptor 1 (38). In the latest clinical study, 313 patients with T2DM, 102 pre-diabetic patients, and 100 healthy volunteers were selected to study the relationship between SIRT6 and glucolipid metabolism and urinary protein. The clinical study results showed that SIRT6 increased with glucolipid metabolism and urinary protein markers, and is therefore expected to be a potential biomarker for the early prediction and diagnosis of glucolipid metabolism disorders and related nephropathy (39). The results of the above gene polymorphism and clinical studies indicate that sirtuins may represent a molecular target to explore new therapeutic approaches for DKD in the clinic.
4 Biological Effects of SIRT1–SIRT7 in DKD Models
In the cell models of DKD, injury models are mostly induced by HG or AGE, while most kidney fibrosis models are induced by TGF-β1 or HG in podocytes, mesangial cells, renal tubular cells, and some endothelial cells (Table 1). In podocyte, proximal tubular cell, and mesangial cell models, SIRT1 and SIRT3 are involved in the mechanism by which therapeutic drugs restore mitochondrial biosynthesis. In podocyte and mesangial cell models, SIRT1 and SIRT6 play significant roles in reducing abnormal mitochondrial function. Moreover, SIRT1, SIRT3, and SIRT4 are involved in the anti-oxidative stress effect in podocytes, mesangial cells, and renal tubular cells. SIRT1, SIRT3, SIRT4, SIRT6, and SIRT7 all participate in reducing the apoptosis of podocytes, mesangial cells, and renal tubular cells in DKD models. In most DKD cell models, therapies targeting SIRT1, SIRT3, SIRT4, and SIRT6 have shown anti-inflammatory effects. In DKD tubular cell models, both SIRT1- and SIRT3-targeted therapies displayed anti-fibrosis effects and suppressed epithelial-mesenchymal transition (EMT). Targeting SIRT1 also enhanced autophagy in various DKD models. By summarizing the results of previous research, we found that SIRT1, SIRT3, SIRT4, SIRT6, and SIRT7 play different biological functions in DKD cell models. Notably, SIRT1 is the most widely investigated HDAC with the most diverse biological functions (Figure 1).
Animal models are valuable for studying the pathological origins of human diseases because they allow in-depth investigation of mechanisms, which cannot be explored in clinical studies. As DKD animal models, db/db mice or rats, STZ and/or HFD-induced mice or SD/Wistar rats, and some unique transgenic mouse models are often used as research objects. We summarized the research methods of SIRT1, SIRT3, SIRT4, SIRT6, and SIRT7 in different DKD animal models to understand the methods of animal models more intuitively (Tables 2–9).
Generally, these abnormal manifestations, such as inflammation, oxidative stress, abnormal mitochondrial function, renal fibrosis, podocyte loss and apoptosis, and impaired autophagy, are all likely to occur during the development of DKD. Meanwhile, SIRT1, SIRT3, SIRT4, SIRT6, and SIRT7 play diverse regulatory roles in these physiological processes.
5 The Role of SIRT1–SIRT7 in Signaling Pathways in DKD Models
5.1 AMPK/Sirtuins/PGC-1α Pathway
AMPK and SIRT1 are the two main energy sensors, which directly affect the activity of PGC-1α through phosphorylation and deacetylation, respectively (40). Studies have shown that impaired renal function under HG is directly related to the inactivation of the AMPK/SIRT1/PGC-1α signaling pathway (41). The study results showed that CL316, 243, glycyrrhizic acid, and a polysaccharide from okra (OP) all played antioxidant roles, reduced inflammation, and improved fibrosis through activation of the AMPK/SIRT1/PGC-1α pathway in STZ and/or HFD-induced db/db DKD mouse models (42–44). Resveratrol, pro-renin receptor shRNA, and grape seed procyanidin B2 (GSPB2) regained SIRT1 expression via the AMPK/SIRT1/PGC-1α signaling axis in DKD models, thus restoring mitochondrial biosynthesis and function, reducing oxidative stress, and inhibiting apoptosis (40, 41, 45–47). In DKD animal or cell models, FGF21, metformin, salidroside, and roflumilast increased or restored the expression level of SIRT1 and played anti-apoptotic and anti-oxidative roles by activating the AMPK/SIRT1 pathway (48–51). Moreover, catalpol and geniposide (GE) up-regulated the expression of SIRT1 in DKD models and inhibited oxidative stress and inflammation by activating the AMPK/SIRT1/NF-κB pathway (52, 53). Additionally, in HG-induced renal tubule cells, restoration of SIRT3 expression through stanniocalcin-1 activated the AMPK/SIRT3 pathway to produce antioxidant and anti-apoptotic activities (54). Furthermore, cocoa, metformin, glycyrrhizic acid, and probucol restored SIRT1 expression by activation of the AMPK/SIRT1 pathway, ultimately reducing oxidative stress, apoptosis, and enhancing autophagy in DKD models (26, 55–58). However, one particular study reported that resveratrol improved oxidative stress and enhanced mitochondrial biogenesis without altering SIRT1 expression, and is independent of the AMPK/SIRT1 pathway. The distinction is that they used H2O2-exposed proximal tubular cells as a DKD model, as opposed to HG or AGE, which are more commonly used (59). In HG-induced immortalized human mesangial cells (iHMCs), theobromine could activate SIRT1 and decrease kidney extracellular matrix (ECM) accumulation by activating the AMPK pathway (60). In BTBR ob/ob mice, honokiol protected mitochondrial health by activating mitochondrial SIRT3, which first revealed the renal protective effect of SIRT3 on diabetic glomerular disease (61). Moreover, in STZ-induced mouse models, salidroside and resveratrol restored SIRT1 expression via the SIRT1/PGC‐1α pathway, thus inhibiting fibrosis and reducing mitochondrial oxidative stress, respectively (61–63). BF175, as an activator of SIRT1, increased SIRT1 activity to acetylate PGC‐1α and activate PPARγ to reduce podocyte loss and oxidative stress (64). Furthermore, glucagon−like peptide−1, formononetin, and resveratrol enhanced SIRT1 expression in DKD models to attenuate apoptosis and oxidative stress by activating SIRT1 (65–67). Beyond this, in HG-induced podocytes or mesangial cells, overexpression of lncRNA SOX2OT, overexpression of lncRNA GAS5, or downregulation of miR-138 increased SIRT1 expression or activity to induce autophagy, inhibit fibrosis, and decrease inflammation, respectively, by regulating the miR-9/SIRT1, miR-221/SIRT1, and miR-138/SIRT1 axes (68–70). Through the studies reported above, we conclude that AMPK/Sirtuins/PGC-1α is a crucial pathway in regulating the pathological process of DKD (Table 2).
5.2 SIRT1/p53 Pathway
SIRT1 specifically associates with and acetylates the tumor suppressor protein p53, thereby negatively regulating p53-mediated transcriptional activation. More importantly, p53 deacetylation by SIRT1 prevents DNA damage and stress-induced cell senescence and apoptosis (71, 72). A previous study has shown that in HG-induced podocytes or HK-2 cells, inhibition of miR-150-5p or miR-155-5p, which could bind to the 3’-UTR of SIRT1, promoted autophagy by targeting the SIRT1/p53 pathway (73, 74). Moreover, in DKD animal and cell models, H2S, resveratrol, and calcium dobesilate restored or enhanced SIRT1 expression to prevent apoptosis by activating the SIRT1/p53 pathway (75–77). These reports suggest that the SIRT1/p53 pathway reduces cellular stress in HG-induced cells or STZ-induced animals’ models (Table 3).
5.3 SIRT1/NF-κB-Related Pathway
Previous studies have demonstrated that the ability of SIRT1 deacetylation is critical to control the function of the transcription factor NF-κB, as SIRT1 modulates various biological responses by deacetylating NF-κB, including inflammation and autophagy (78, 79). In DKD models, isoliquiritigenin (ISLQ), baicalin, astragaloside IV, ligustilide, nicotinamide mononucleotide (NMN), and Tangshen formula have been shown to increase or activate SIRT1 through the SIRT1/NF-κB signaling pathway to improve inflammation, decrease apoptosis, and enhance autophagy (80–86). Moreover, in STZ-induced mouse models, BF175 decreased albuminuria and glomerular disease via the transcription factor NF-κB and p53 pathways (87). Additionally, panax notoginseng saponins (PNS) and baicalin have been found to up-regulate SIRT1 to inhibit inflammation, reactivate autophagy, and alleviate fibrosis via the NF-κB and TGF-β pathways in DKD models (88, 89). Furthermore, in HG-induced HK-2 cells, Na2S4 has been shown to directly sulfhydrate two conserved domains of SIRT1, leading to dephosphorylation and deacetylation of NF-κB and STAT3, which improves oxidative stress, apoptosis, and the inflammatory response (90). Thus, SIRT1 also has a protective effect on renal function by regulating downstream of NF-κB in DKD (Table 4).
5.4 Sirtuins and the TGF-β1/Smad3 Pathway
TGF-β superfamily members are critical in regulating fibrosis in most chronic kidney diseases, and the inhibition of TGF-β1 or its downstream signaling (e.g. Smad) has been shown to decrease renal fibrosis (91–94). It has also been reported that the reduction of miR-34a-5p targets the 3’UTR of SIRT1, which inhibits fibrosis by regulating TGF-β1 signaling in HG-induced HK-2 cells (95). Moreover, in AGE stimulated NRK-52E cells, oligo-fucoidan has been shown to improve renal fibrosis via restraint of the pro-fibrosis process caused by TGF-β1 activation (96). Additionally, tetrahydroxystilbene glucoside (TSG) restored SIRT1 expression to alleviate oxidative stress by targeting SIRT1 and TGF-β1 signaling both in vivo and in vitro (97). Moreover, the inhibition of miRNA−135a−5p increased SIRT1 expression and inhibited fibrosis by targeting the TGF-β1/Smad3 pathway in TGF-β1-induced HK-2 and HMC cells (98). As a unique example, FOXO3a binds to the SIRT6 promoter and promoted SIRT6 expression to reduce EMT and fibrosis through FOXO3a-mediated SIRT6/Smad3 pathway in DKD models (99). The above summary highlights the vital function of the TGF-β1/Smad3 pathway in the regulation of renal fibrosis by sirtuins in DKD (Table 5).
5.5 PI3K/AKT/FOXO Pathway
The PI3K/AKT pathway plays a crucial role in cell physiology, which participates in glucose homeostasis, lipid metabolism, protein synthesis, and cell proliferation and survival (100, 101). FOXO1 and FOXO3a, as important substrates of AKT, are regulated by the PI3K/AKT pathway (102). Researchers have found that resveratrol restored SIRT1 expression to attenuate oxidative stress damage in STZ-induced rat models through the SIRT1/FOXO3a or SIRT1/FOXO1 pathway (103–105). Furthermore, fucoxanthin and angiotensin 1–7 restored SIRT1 expression in response to antioxidative stress via the AKT/SIRT1/FOXO3α and SIRT1/FOXO1/ATGL signaling pathways in DKD models, separately (106, 107). Moreover, in STZ- and HFD-induced mouse models, purinergic receptor (P2Y2R) deficiency enhanced autophagy and the expression of SIRT1 by AKT/FOXO3a and SIRT1 signaling pathways (108). Additionally, pyrroloquinoline quinine increased the expression of SIRT3 to antagonize oxidative stress and apoptosis in HG-induced HK-2 cells via the PI3K/AKT/FOXO3a signaling pathway (109). Moreover, it has been reported that progranulin (PGRN) restored both SIRT1 and SIRT3 to maintain mitochondrial biogenesis and mitophagy via SIRT1/PGC-1α/FOXO1 signaling in HG-treated podocytes (110). These findings suggest that the PI3K/AKT/FOXO pathway performs important biological functions in improving DKD by targeting sirtuins (Table 6).
5.6 Keap1/Nrf2/ARE Pathway
Dysregulation of Nrf2 transcriptional activity has been described in the pathogenesis of various diseases, and the Nrf2/Keap1 axis is a key regulator of cell homeostasis (111). It has been reported that formononetin, resveratrol, and polydatin up-regulate the expression of SIRT1 to anti-oxidative stress and fibrosis by activating the Nrf2/ARE pathway in HG/AGE-induced GMCs (112–114). Investigators have also found that SRT2104 (SIRT1 activators) protect against oxidative stress, inflammation, and fibrosis via the SIRT1/p53/Nrf2 pathway in DKD models (115). Moreover, in HG-induced NRK-52E cells, ISLQ treatment reduced inflammation and oxidative stress by inhibiting MAPK activation and the induction of Nrf2 signaling (116). These findings demonstrate that SIRT1 regulates the transcription factor Nrf2 in DKD models (Table 7).
5.7 STAT and HIF-1α-Related Pathway
It has been reported that connexin 43, LincRNA 1700020I14Rik, and silencing of miR-217 restrain inflammation and fibrosis in both in vivo and in vitro DKD models through SIRT1/HIF-1α signaling (117–119). Additionally, in AGE-induced human podocytes, PYR as an AGE inhibitor, restored SIRT1 expression to reduce kidney injury by decreasing p65 and STAT3 acetylation (120). In one study in HFD-diet DM rats, EX-527, as a SIRT1 inhibitor, reduced SIRT1 expression and increased SIRT3 expression to lessen fibrosis and inflammation by blocking the phosphorylation of EGFR and PDGFR, blocking STAT3 signaling (121). In another study, glucagon-like peptide-1 decreased SIRT1 expression to improve the inflammatory changes in db/db mice by inhibiting JAK/STAT signaling (122). Thus, STAT and HIF-1α-related pathways reduce negative effects in DKD models by targeting sirtuins (Table 8).
5.8 Other Pathways Involved in the Regulation of Sirtuins in DKD
5.8.1 Pathways Associated With SIRT1 in DKD
Researchers have shown that both 1α, 25-Dihydroxyvitamin D3 and puerarin activate and increase SIRT1 expression to achieve anti-oxidative effects by suppressing NOX4 expression in DKD models (123, 124). Carnosine upregulated SIRT1 expression to decrease glycative and lipoperoxidative stress in HG-induced podocytes via the Hsp70/HO-1 pathway. Another report showed that anserine revealed anti-oxidant and glycative stress in HG-induced HK-2 cells via the Hsp70/HO-1 defense system, but did not affect SIRT1 expression (125, 126). Several other studies have shown that aerobic exercise training, inhibition of HIC1, INT-767 (FXR/TGR5 dual agonist), and SGLT2 restored SIRT1 expression under DKD animal and cell models, which improve mitochondrial function, reduce ROS, anti-inflammation, and prevent glucose entry (127–130). These results suggest that SIRT1 largely exhibits anti-inflammatory and anti-oxidant effects through different signaling pathways in DKD models.
5.8.2 Pathways Associated With SIRT3 in DKD
Apigenin (CD38 inhibitor) and empagliflozin (SGLT2 inhibitor) have been shown to increase SIRT3 levels in HG-induced HK-2 cells to relieve mitochondrial oxidative stress and restore aberrant functions; this is mediated by restoring the NAD+/NADH ratio and inhibiting glucose uptake into the proximal tubules, respectively (131, 132). Liraglutide (glucagon−like peptide−1 agonist) has also been shown to increase SIRT3 expression to prevent the activation of mitochondrial apoptosis by activating the ERK−Yap signaling pathway in HG-induced HRMCs (133). It has been reported that INT-777 (TGR5-agonist) increased the activity of both SIRT1 and SIRT3 to improve mitochondrial biogenesis, and reduce oxidative stress and fibrosis via the TGR5 pathway in db/db diabetic mice (134). Moreover, in the C57BL/KsJ db/db mouse model, the overexpression of SIRT3 reduced apoptosis and fibrosis through modulation of mitophagy (135). It can be seen from the above results that high expression of SIRT3 reduced mitochondrial stress response, including oxidative stress and apoptosis.
5.8.3 Pathways Associated With SIRT6 in DKD
SIRT6-knockout male mice have been shown to exhibit an enhanced fibrotic phenotype, which was controlled by the Nampt-SIRT6 axis to regulate extracellular matrix remodeling, and the authors found that SIRT1 is not the controller of SIRT6 expression (136). The results of this article show that SIRT6 plays an important regulatory role in ECM remodeling.
5.8.4 Pathways Associated With SIRT7 in DKD
In HG-treated podocytes, the increase in SIRT7 has been shown to inhibit podocyte apoptosis, while the suppression of microRNA-20b promotes SIRT7 expression to decrease apoptosis (137) (Table 9). This research demonstrated that increasing the expression of SIRT7 reduced the occurrence of apoptosis in podocytes.
5.9 Summary of SIRT1–SIRT7
SIRT1 was the first sirtuin discovered in mammals, and remains the most extensively and deeply studied so far (138). Resveratrol is the most recognized and studied activator of SIRT1 (139). SIRT1 has been extensively studied in DKD models, including podocytes, mesangial cells, and tubular cells. SIRT2 is the only cytoplasmic sirtuin, but its role in treating DKD has not been reported yet so far, nor has that of SIRT5. SIRT3 is normally located in the mitochondria, but under cellular stress, it can translocate into the nucleus (140). Some studies have reported that increased expression of SIRT3 is beneficial to DKD, mainly through AMPK or PI3K pathways (25, 27, 54, 109, 110). However, we found one article that reported that the overexpression of SIRT4 reduced inflammatory effects, and inhibited ROS production and apoptosis in HG-induced podocytes (28). SIRT6 is a nuclear HDAC that plays an important role in the pathological processes of inflammation, aging, cancer, and neurodegenerative diseases (141). However, only a few studies on SIRT6 have been reported, mainly in podocyte and tubular cell models of DKD. Additionally, the catalytic activity of SIRT7 is weak, and a previous report indicated that the suppression of microRNA-20b increased SIRT7 expression and reduced HG-induced podocyte apoptosis (137) (Figure 2).
6 Conclusions and Perspectives
Many researchers are working to investigate the etiology of DKD and explore new treatment methods. In our conventional view, sirtuins are a class of HDACs involved in the regulation of longevity and maintaining the stability of nucleosomes by balancing with histone acetylases (13). However, in addition to deacetylate histones, we discovered that sirtuins also regulate many transcription factors, including FOXO1, FOXO3a, STAT3, Smad2/3, NF-κB, p53, and Nrf-2. These transcription factors are involved in regulating many biological processes, including autophagy, oxidative stress, apoptosis, inflammation, EMT, and fibrosis (Figure 3). We found that in DKD studies, the high expression of SIRT1–SIRT7 alleviated or reduced kidney injury through different mechanisms or molecular pathways, of which SIRT1 is the most widely explored. However, an exception was found in db/db mice, which showed that treatment with glucagon-like peptide-1 reduced SIRT1 expression, while in HUVEC cells, glucagon-like peptide-1 had no significant effect on the SIRT1 expression level. The authors explained that the in vivo results were due to a reduced inflammatory environment that did not stimulate SIRT1, while the in vitro results were due to SIRT1 only participating in transcriptional responses (122). Resveratrol is a recognized activator of SIRT1, but in db/db mice, treatment with resveratrol failed to cause changes in SIRT1 expression, and it still improved oxidative stress and enhanced mitochondrial biogenesis in the AMPK/SIRT1-independent pathway (59). Furthermore, the expression of SIRT1, SIRT2, SIRT3, and SIRT6 was higher than SIRT4, SIRT5, and SIRT7 in the kidney; therefore, the study of SIRT1, SIRT2, SIRT3, and SIRT6 in DKD models is both reasonable and credible (136).
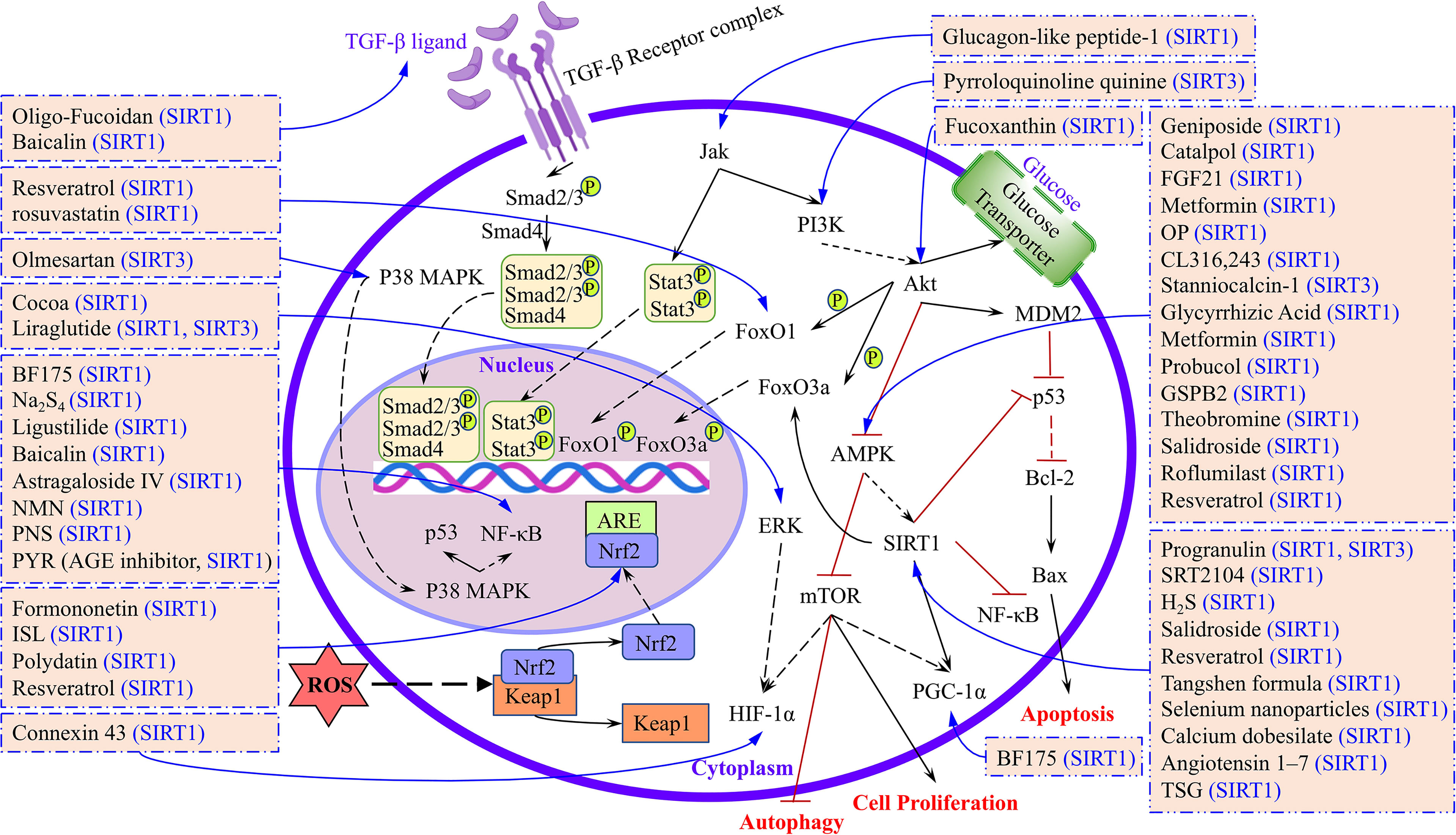
Figure 3 The targets and signaling pathways of different drugs or compounds regulated by sirtuins were summarized.
In light of the above, to better illuminate the roles of SIRT1–SIRT7 in DKD and the research progress, we have summarized the therapeutics, targets, and signaling pathways involved in in vitro and in vivo models of DKD (Figure 3). Our aim is that this review will serve as a valuable reference for future studies of sirtuins and DKD, and provide a theoretical foundation for delaying the pathological process of DKD in the clinic.
Author Contributions
WQ and CH contributed to designing and writing the manuscript. DZ and XL approved the submitted version. All authors contributed to the article and approved the submitted version.
Funding
This work was supported by the National Natural Science Foundation of China (U19A2013), the National Key Research and Development Program of China (2017YFC1702103), and the Science and Technology Development Plan Project of Jilin Province (202002053JC).
Conflict of Interest
The authors declare that the research was conducted in the absence of any commercial or financial relationships that could be construed as a potential conflict of interest.
Publisher’s Note
All claims expressed in this article are solely those of the authors and do not necessarily represent those of their affiliated organizations, or those of the publisher, the editors and the reviewers. Any product that may be evaluated in this article, or claim that may be made by its manufacturer, is not guaranteed or endorsed by the publisher.
Acknowledgments
We thank LetPub (www.letpub.com) for its linguistic assistance during the preparation of this manuscript.
Abbreviations
AFSCs, Amniotic fluid stem cells; AGEs, Advanced glycation end products; BAT, Brown adipose tissue; EMT, Epithelial-mesenchymal transition; GBM, Glomerular basement membrane; GMCs, Glomerular mesangial cells; GSPB2, Grape seed procyanidin B2; HFD, High-fat diet; HG, High glucose; HGECs, Human glomerular endothelial cells; HIC1, Hypermethylated in cancer 1; hnRNP F, Heterogeneous Nuclear Ribonucleoprotein F; IRPTCs, ROS generation mediates HG stimulation of angiotensinogen expression in immortalized rat RPT cells; ISLQ, Isoliquiritigenin; KD, Knockdown; Nampt, NMN-producing enzyme nicotinamide phosphoribosyltransferase; NMN, Nicotinamide mononucleotide; OE, Overexpression; OP, A polysaccharide purified from okra; OLETF, Otsuka-Long-Evans-Tokushima-Fatty; PNS, Panax notoginseng saponins; RMCs, Renal mesangial cells; ROS, Reactive oxygen species; STZ, Streptozotocin; T1DM, Type 1 diabetes mellitus; T2DM, Type 2 diabetes mellitus; TSG, Tetrahydroxystilbene glucoside.
References
1. Wang AJ, Wang S, Wang BJ, Xiao M, Guo Y, Tang Y, et al. Epigenetic Regulation Associated With Sirtuin 1 in Complications of Diabetes Mellitus. Front Endocrinol (Lausanne) (2020) 11:598012. doi: 10.3389/fendo.2020.598012
2. Hershberger KA, Martin AS, Hirschey MD. Role of NAD(+) and Mitochondrial Sirtuins in Cardiac and Renal Diseases. Nat Rev Nephrol (2017) 13(4):213–25. doi: 10.1038/nrneph.2017.5
3. Wakino S, Hasegawa K, Itoh H. Sirtuin and Metabolic Kidney Disease. Kidney Int (2015) 88(4):691–8. doi: 10.1038/ki.2015.157
4. Navarro-Gonzalez JF, Mora-Fernandez C, Muros de Fuentes M, Garcia-Perez J. Inflammatory Molecules and Pathways in the Pathogenesis of Diabetic Nephropathy. Nat Rev Nephrol (2011) 7(6):327–40. doi: 10.1038/nrneph.2011.51
5. Gheith O, Farouk N, Nampoory N, Halim MA, Al-Otaibi T. Diabetic Kidney Disease: World Wide Difference of Prevalence and Risk Factors. J Nephropharmacol (2016) 5(1):49–56. doi: 10.4103/1110-9165.197379
6. Koye DN, Magliano DJ, Nelson RG, Pavkov ME. The Global Epidemiology of Diabetes and Kidney Disease. Adv Chronic Kidney Dis (2018) 25(2):121–32. doi: 10.1053/j.ackd.2017.10.011
7. Wang W, Sun W, Cheng Y, Xu Z, Cai L. Role of Sirtuin-1 in Diabetic Nephropathy. J Mol Med (Berl) (2019) 97(3):291–309. doi: 10.1007/s00109-019-01743-7
8. Li X, Zhang J, Xie Y, Jiang Y, Yingjie Z, Xu W. Progress of HDAC Inhibitor Panobinostat in the Treatment of Cancer. Curr Drug Targets (2014) 15(6):622–34. doi: 10.2174/1389450115666140306152642
9. Chen B, Zang W, Wang J, Huang Y, He Y, Yan L, et al. The Chemical Biology of Sirtuins. Chem Soc Rev (2015) 44(15):5246–64. doi: 10.1039/c4cs00373j
10. Morigi M, Perico L, Benigni A. Sirtuins in Renal Health and Disease. J Am Soc Nephrol (2018) 29(7):1799–809. doi: 10.1681/ASN.2017111218
11. Wang Y, He J, Liao M, Hu M, Li W, Ouyang H, et al. An Overview of Sirtuins as Potential Therapeutic Target: Structure, Function and Modulators. Eur J Med Chem (2019) 161:48–77. doi: 10.1016/j.ejmech.2018.10.028
12. Yoon YK, Oon CE. Sirtuin Inhibitors: An Overview From Medicinal Chemistry Perspective. Anticancer Agents Med Chem (2016) 16(8):1003–16. doi: 10.2174/1871520616666160310141622
13. Watroba M, Dudek I, Skoda M, Stangret A, Rzodkiewicz P, Szukiewicz D. Sirtuins, Epigenetics and Longevity. Ageing Res Rev (2017) 40:11–9. doi: 10.1016/j.arr.2017.08.001
14. Leite JA, Ghirotto B, Targhetta VP, de Lima J, Camara NOS. Sirtuins as Pharmacological Targets in Neurodegenerative and Neuropsychiatric Disorders. Br J Pharmacol (2021) 179(8):1496–511. doi: 10.1111/bph.15570
15. Zhu S, Dong Z, Ke X, Hou J, Zhao E, Zhang K, et al. The Roles of Sirtuins Family in Cell Metabolism During Tumor Development. Semin Cancer Biol (2019) 57:59–71. doi: 10.1016/j.semcancer.2018.11.003
16. van de Ven RAH, Santos D, Haigis MC. Mitochondrial Sirtuins and Molecular Mechanisms of Aging. Trends Mol Med (2017) 23(4):320–31. doi: 10.1016/j.molmed.2017.02.005
17. DeFronzo RA, Reeves WB, Awad AS. Pathophysiology of Diabetic Kidney Disease: Impact of SGLT2 Inhibitors. Nat Rev Nephrol (2021) 17(5):319–34. doi: 10.1038/s41581-021-00393-8
18. Zoja C, Xinaris C, Macconi D. Diabetic Nephropathy: Novel Molecular Mechanisms and Therapeutic Targets. Front Pharmacol (2020) 11:586892. doi: 10.3389/fphar.2020.586892
19. Gnudi L, Coward RJM, Long DA. Diabetic Nephropathy: Perspective on Novel Molecular Mechanisms. Trends Endocrinol Metab (2016) 27(11):820–30. doi: 10.1016/j.tem.2016.07.002
20. Liu S, Yuan Y, Xue Y, Xing C, Zhang B. Podocyte Injury in Diabetic Kidney Disease: A Focus on Mitochondrial Dysfunction. Front Cell Dev Biol (2022) 10:832887. doi: 10.3389/fcell.2022.832887
21. Chuang PY, Xu J, Dai Y, Jia F, Mallipattu SK, Yacoub R, et al. In Vivo RNA Interference Models of Inducible and Reversible Sirt1 Knockdown in Kidney Cells. Am J Pathol (2014) 184(7):1940–56. doi: 10.1016/j.ajpath.2014.03.016
22. Huang K, Gao X, Wei W. The Crosstalk Between Sirt1 and Keap1/Nrf2/ARE Anti-Oxidative Pathway Forms a Positive Feedback Loop to Inhibit FN and TGF-Beta1 Expressions in Rat Glomerular Mesangial Cells. Exp Cell Res (2017) 361(1):63–72. doi: 10.1016/j.yexcr.2017.09.042
23. Zhou L, Xu DY, Sha WG, Shen L, Lu GY, Yin X, et al. High Glucose Induces Renal Tubular Epithelial Injury via Sirt1/NF-Kappab/microR-29/Keap1 Signal Pathway. J Transl Med (2015) 13:352. doi: 10.1186/s12967-015-0710-y
24. Ogura Y, Kitada M, Monno I, Kanasaki K, Watanabe A, Koya D. Renal Mitochondrial Oxidative Stress is Enhanced by the Reduction of Sirt3 Activity, in Zucker Diabetic Fatty Rats. Redox Rep (2018) 23(1):153–9. doi: 10.1080/13510002.2018.1487174
25. Jiao X, Li Y, Zhang T, Liu M, Chi Y. Role of Sirtuin3 in High Glucose-Induced Apoptosis in Renal Tubular Epithelial Cells. Biochem Biophys Res Commun (2016) 480(3):387–93. doi: 10.1016/j.bbrc.2016.10.060
26. Srivastava SP, Li J, Kitada M, Fujita H, Yamada Y, Goodwin JE, et al. SIRT3 Deficiency Leads to Induction of Abnormal Glycolysis in Diabetic Kidney With Fibrosis. Cell Death Dis (2018) 9(10):997. doi: 10.1038/s41419-018-1057-0
27. Wang Y, Zhang X, Wang P, Shen Y, Yuan K, Li M, et al. Sirt3 Overexpression Alleviates Hyperglycemia-Induced Vascular Inflammation Through Regulating Redox Balance, Cell Survival, and AMPK-Mediated Mitochondrial Homeostasis. J Recept Signal Transduct Res (2019) 39(4):341–9. doi: 10.1080/10799893.2019.1684521
28. Shi JX, Wang QJ, Li H, Huang Q. SIRT4 Overexpression Protects Against Diabetic Nephropathy by Inhibiting Podocyte Apoptosis. Exp Ther Med (2017) 13(1):342–8. doi: 10.3892/etm.2016.3938
29. Fan Y, Yang Q, Yang Y, Gao Z, Ma Y, Zhang L, et al. Sirt6 Suppresses High Glucose-Induced Mitochondrial Dysfunction and Apoptosis in Podocytes Through AMPK Activation. Int J Biol Sci (2019) 15(3):701–13. doi: 10.7150/ijbs.29323
30. Ji L, Chen Y, Wang H, Zhang W, He L, Wu J, et al. Overexpression of Sirt6 Promotes M2 Macrophage Transformation, Alleviating Renal Injury in Diabetic Nephropathy. Int J Oncol (2019) 55(1):103–15. doi: 10.3892/ijo.2019.4800
31. Liu M, Liang K, Zhen J, Zhou M, Wang X, Wang Z, et al. Sirt6 Deficiency Exacerbates Podocyte Injury and Proteinuria Through Targeting Notch Signaling. Nat Commun (2017) 8(1):413. doi: 10.1038/s41467-017-00498-4
32. Abbas S, Raza ST, Ahmed F, Ahmad A, Rizvi S, Mahdi F. Association of Genetic Polymorphism of PPARgamma-2, ACE, MTHFR, FABP-2 and FTO Genes in Risk Prediction of Type 2 Diabetes Mellitus. J BioMed Sci (2013) 20:80. doi: 10.1186/1423-0127-20-80
33. Li YY. ENPP1 K121Q Polymorphism and Type 2 Diabetes Mellitus in the Chinese Population: A Meta-Analysis Including 11,855 Subjects. Metabolism (2012) 61(5):625–33. doi: 10.1016/j.metabol.2011.10.002
34. Dobigny G, Britton-Davidian J, Robinson TJ. Chromosomal Polymorphism in Mammals: An Evolutionary Perspective. Biol Rev Camb Philos Soc (2017) 92(1):1–21. doi: 10.1111/brv.12213
35. Zhao Y, Wei J, Hou X, Liu H, Guo F, Zhou Y, et al. SIRT1 Rs10823108 and FOXO1 Rs17446614 Responsible for Genetic Susceptibility to Diabetic Nephropathy. Sci Rep (2017) 7(1):10285. doi: 10.1038/s41598-017-10612-7
36. Tang K, Sun M, Shen J, Zhou B. Transcriptional Coactivator P300 and Silent Information Regulator 1 (SIRT1) Gene Polymorphism Associated With Diabetic Kidney Disease in a Chinese Cohort. Exp Clin Endocrinol Diabetes (2017) 125(8):530–7. doi: 10.1055/s-0043-103966
37. Ren H, Shao Y, Ma X, Yang M, Liu Y, Wang Q. Expression Levels of Serum Vasohibin-1 and Other Biomarkers in Type 2 Diabetes Mellitus Patients With Different Urinary Albumin to Creatinine Ratios. J Diabetes Complications (2019) 33(7):477–84. doi: 10.1016/j.jdiacomp.2019.04.008
38. Yubero-Serrano EM, Woodward M, Poretsky L, Vlassara H, Striker GE, Group AG-lS. Effects of Sevelamer Carbonate on Advanced Glycation End Products and Antioxidant/Pro-Oxidant Status in Patients With Diabetic Kidney Disease. Clin J Am Soc Nephrol (2015) 10(5):759–66. doi: 10.2215/CJN.07750814
39. Bian C, Gao J, Wang Y, Li J, Luan Z, Lu H, et al. Association of SIRT6 Circulating Levels With Urinary and Glycometabolic Markers in Pre-Diabetes and Diabetes. Acta Diabetol (2021) 58(11):1551–62. doi: 10.1007/s00592-021-01759-x
40. Akhtar S, Siragy HM. Pro-Renin Receptor Suppresses Mitochondrial Biogenesis and Function via AMPK/SIRT-1/ PGC-1alpha Pathway in Diabetic Kidney. PloS One (2019) 14(12):e0225728. doi: 10.1371/journal.pone.0225728
41. Kim MY, Lim JH, Youn HH, Hong YA, Yang KS, Park HS, et al. Resveratrol Prevents Renal Lipotoxicity and Inhibits Mesangial Cell Glucotoxicity in a Manner Dependent on the AMPK-SIRT1-PGC1alpha Axis in Db/Db Mice. Diabetologia (2013) 56(1):204–17. doi: 10.1007/s00125-012-2747-2
42. Liao Z, Zhang J, Wang J, Yan T, Xu F, Wu B, et al. The Anti-Nephritic Activity of a Polysaccharide From Okra (Abelmoschus Esculentus (L.) Moench) via Modulation of AMPK-Sirt1-PGC-1alpha Signaling Axis Mediated Anti-Oxidative in Type 2 Diabetes Model Mice. Int J Biol Macromol (2019) 140:568–76. doi: 10.1016/j.ijbiomac.2019.08.149
43. Cai YY, Zhang HB, Fan CX, Zeng YM, Zou SZ, Wu CY, et al. Renoprotective Effects of Brown Adipose Tissue Activation in Diabetic Mice. J Diabetes (2019) 11(12):958–70. doi: 10.1111/1753-0407.12938
44. Hou S, Zhang T, Li Y, Guo F, Jin X. Glycyrrhizic Acid Prevents Diabetic Nephropathy by Activating AMPK/SIRT1/PGC-1alpha Signaling in Db/Db Mice. J Diabetes Res (2017) 2017:2865912. doi: 10.1155/2017/2865912
45. Park HS, Lim JH, Kim MY, Kim Y, Hong YA, Choi SR, et al. Resveratrol Increases AdipoR1 and AdipoR2 Expression in Type 2 Diabetic Nephropathy. J Transl Med (2016) 14(1):176. doi: 10.1186/s12967-016-0922-9
46. Cai X, Bao L, Ren J, Li Y, Zhang Z. Grape Seed Procyanidin B2 Protects Podocytes From High Glucose-Induced Mitochondrial Dysfunction and Apoptosis via the AMPK-SIRT1-PGC-1alpha Axis In Vitro. Food Funct (2016) 7(2):805–15. doi: 10.1039/c5fo01062d
47. Bao L, Cai X, Zhang Z, Li Y. Grape Seed Procyanidin B2 Ameliorates Mitochondrial Dysfunction and Inhibits Apoptosis via the AMP-Activated Protein Kinase-Silent Mating Type Information Regulation 2 Homologue 1-PPARgamma Co-Activator-1alpha Axis in Rat Mesangial Cells Under High-Dose Glucosamine. Br J Nutr (2015) 113(1):35–44. doi: 10.1017/S000711451400347X
48. Weng W, Ge T, Wang Y, He L, Liu T, Wang W, et al. Therapeutic Effects of Fibroblast Growth Factor-21 on Diabetic Nephropathy and the Possible Mechanism in Type 1 Diabetes Mellitus Mice. Diabetes Metab J (2020) 44(4):566–80. doi: 10.4093/dmj.2019.0089
49. Rogacka D, Audzeyenka I, Rychlowski M, Rachubik P, Szrejder M, Angielski S, et al. Metformin Overcomes High Glucose-Induced Insulin Resistance of Podocytes by Pleiotropic Effects on SIRT1 and AMPK. Biochim Biophys Acta Mol Basis Dis (2018) 1864(1):115–25. doi: 10.1016/j.bbadis.2017.10.014
50. Shati AA. Salidroside Ameliorates Diabetic Nephropathy in Rats by Activating Renal AMPK/SIRT1 Signaling Pathway. J Food Biochem (2020) 44(4):e13158. doi: 10.1111/jfbc.13158
51. Tikoo K, Lodea S, Karpe PA, Kumar S. Calorie Restriction Mimicking Effects of Roflumilast Prevents Diabetic Nephropathy. Biochem Biophys Res Commun (2014) 450(4):1581–6. doi: 10.1016/j.bbrc.2014.07.039
52. Chen J, Yang Y, Lv Z, Shu A, Du Q, Wang W, et al. Study on the Inhibitive Effect of Catalpol on Diabetic Nephropathy. Life Sci (2020) 257:118120. doi: 10.1016/j.lfs.2020.118120
53. Li F, Chen Y, Li Y, Huang M, Zhao W. Geniposide Alleviates Diabetic Nephropathy of Mice Through AMPK/SIRT1/NF-kappaB Pathway. Eur J Pharmacol (2020) 886:173449. doi: 10.1016/j.ejphar.2020.173449
54. Liu Z, Liu H, Xiao L, Liu G, Sun L, He L. STC-1 Ameliorates Renal Injury in Diabetic Nephropathy by Inhibiting the Expression of BNIP3 Through the AMPK/SIRT3 Pathway. Lab Invest (2019) 99(5):684–97. doi: 10.1038/s41374-018-0176-7
55. Ren H, Shao Y, Wu C, Ma X, Lv C, Wang Q. Metformin Alleviates Oxidative Stress and Enhances Autophagy in Diabetic Kidney Disease via AMPK/SIRT1-FoxO1 Pathway. Mol Cell Endocrinol (2020) 500:110628. doi: 10.1016/j.mce.2019.110628
56. Alvarez-Cilleros D, Lopez-Oliva ME, Martin MA, Ramos S. Cocoa Ameliorates Renal Injury in Zucker Diabetic Fatty Rats by Preventing Oxidative Stress, Apoptosis and Inactivation of Autophagy. Food Funct (2019) 10(12):7926–39. doi: 10.1039/c9fo01806a
57. Yang S, Zhao L, Han Y, Liu Y, Chen C, Zhan M, et al. Probucol Ameliorates Renal Injury in Diabetic Nephropathy by Inhibiting the Expression of the Redox Enzyme p66Shc. Redox Biol (2017) 13:482–97. doi: 10.1016/j.redox.2017.07.002
58. Hou S, Zheng F, Li Y, Gao L, Zhang J. The Protective Effect of Glycyrrhizic Acid on Renal Tubular Epithelial Cell Injury Induced by High Glucose. Int J Mol Sci (2014) 15(9):15026–43. doi: 10.3390/ijms150915026
59. Kitada M, Kume S, Imaizumi N, Koya D. Resveratrol Improves Oxidative Stress and Protects Against Diabetic Nephropathy Through Normalization of Mn-SOD Dysfunction in AMPK/SIRT1-Independent Pathway. Diabetes (2011) 60(2):634–43. doi: 10.2337/db10-0386
60. Papadimitriou A, Silva KC, Peixoto EB, Borges CM, Lopes de Faria JM, Lopes de Faria JB. Theobromine Increases NAD(+)/Sirt-1 Activity and Protects the Kidney Under Diabetic Conditions. Am J Physiol Renal Physiol (2015) 308(3):F209–25. doi: 10.1152/ajprenal.00252.2014
61. Locatelli M, Zoja C, Zanchi C, Corna D, Villa S, Bolognini S, et al. Manipulating Sirtuin 3 Pathway Ameliorates Renal Damage in Experimental Diabetes. Sci Rep (2020) 10(1):8418. doi: 10.1038/s41598-020-65423-0
62. Zhang T, Chi Y, Kang Y, Lu H, Niu H, Liu W, et al. Resveratrol Ameliorates Podocyte Damage in Diabetic Mice via SIRT1/PGC-1alpha Mediated Attenuation of Mitochondrial Oxidative Stress. J Cell Physiol (2019) 234(4):5033–43. doi: 10.1002/jcp.27306
63. Xue H, Li P, Luo Y, Wu C, Liu Y, Qin X, et al. Salidroside Stimulates the Sirt1/PGC-1alpha Axis and Ameliorates Diabetic Nephropathy in Mice. Phytomedicine (2019) 54:240–7. doi: 10.1016/j.phymed.2018.10.031
64. Hong Q, Zhang L, Das B, Li Z, Liu B, Cai G, et al. Increased Podocyte Sirtuin-1 Function Attenuates Diabetic Kidney Injury. Kidney Int (2018) 93(6):1330–43. doi: 10.1016/j.kint.2017.12.008
65. Oza MJ, Kulkarni YA. Formononetin Attenuates Kidney Damage in Type 2 Diabetic Rats. Life Sci (2019) 219:109–21. doi: 10.1016/j.lfs.2019.01.013
66. Shi JX, Huang Q. Glucagonlike Peptide1 Protects Mouse Podocytes Against High Glucoseinduced Apoptosis, and Suppresses Reactive Oxygen Species Production and Proinflammatory Cytokine Secretion, Through Sirtuin 1 Activation In Vitro. Mol Med Rep (2018) 18(2):1789–97. doi: 10.3892/mmr.2018.9085
67. Ma L, Fu R, Duan Z, Lu J, Gao J, Tian L, et al. Sirt1 is Essential for Resveratrol Enhancement of Hypoxia-Induced Autophagy in the Type 2 Diabetic Nephropathy Rat. Pathol Res Pract (2016) 212(4):310–8. doi: 10.1016/j.prp.2016.02.001
68. Ge X, Xu B, Xu W, Xia L, Xu Z, Shen L, et al. Long Noncoding RNA GAS5 Inhibits Cell Proliferation and Fibrosis in Diabetic Nephropathy by Sponging miR-221 and Modulating SIRT1 Expression. Aging (Albany NY) (2019) 11(20):8745–59. doi: 10.18632/aging.102249
69. Zhang Y, Chang B, Zhang J, Wu X. LncRNA SOX2OT Alleviates the High Glucose-Induced Podocytes Injury Through Autophagy Induction by the miR-9/SIRT1 Axis. Exp Mol Pathol (2019) 110:104283. doi: 10.1016/j.yexmp.2019.104283
70. Liu F, Guo J, Qiao Y, Pan S, Duan J, Liu D, et al. MiR-138 Plays an Important Role in Diabetic Nephropathy Through SIRT1-P38-TTP Regulatory Axis. J Cell Physiol (2021) 236(9):6607–18. doi: 10.1002/jcp.30238
71. Smith J. Human Sir2 and the 'Silencing' of P53 Activity. Trends Cell Biol (2002) 12(9):404–6. doi: 10.1016/s0962-8924(02)02342-5
72. Cohen HY, Miller C, Bitterman KJ, Wall NR, Hekking B, Kessler B, et al. Calorie Restriction Promotes Mammalian Cell Survival by Inducing the SIRT1 Deacetylase. Science (2004) 305(5682):390–2. doi: 10.1126/science.1099196
73. Wang Y, Zheng ZJ, Jia YJ, Yang YL, Xue YM. Role of P53/miR-155-5p/Sirt1 Loop in Renal Tubular Injury of Diabetic Kidney Disease. J Transl Med (2018) 16(1):146. doi: 10.1186/s12967-018-1486-7
74. Dong W, Zhang H, Zhao C, Luo Y, Chen Y. Silencing of miR-150-5p Ameliorates Diabetic Nephropathy by Targeting SIRT1/p53/AMPK Pathway. Front Physiol (2021) 12:624989. doi: 10.3389/fphys.2021.624989
75. Ahmed HH, Taha FM, Omar HS, Elwi HM, Abdelnasser M. Hydrogen Sulfide Modulates SIRT1 and Suppresses Oxidative Stress in Diabetic Nephropathy. Mol Cell Biochem (2019) 457(1-2):1–9. doi: 10.1007/s11010-019-03506-x
76. Wang XL, Wu LY, Zhao L, Sun LN, Liu HY, Liu G, et al. SIRT1 Activator Ameliorates the Renal Tubular Injury Induced by Hyperglycemia In Vivo and In Vitro via Inhibiting Apoptosis. BioMed Pharmacother (2016) 83:41–50. doi: 10.1016/j.biopha.2016.06.009
77. Wang Y, Zuo B, Wang N, Li S, Liu C, Sun D. Calcium Dobesilate Mediates Renal Interstitial Fibrosis and Delay Renal Peritubular Capillary Loss Through Sirt1/p53 Signaling Pathway. BioMed Pharmacother (2020) 132:110798. doi: 10.1016/j.biopha.2020.110798
78. Deng Z, Jin J, Wang Z, Wang Y, Gao Q, Zhao J. The Metal Nanoparticle-Induced Inflammatory Response is Regulated by SIRT1 Through NF-kappaB Deacetylation in Aseptic Loosening. Int J Nanomed (2017) 12:3617–36. doi: 10.2147/IJN.S124661
79. Nopparat C, Sinjanakhom P, Govitrapong P. Melatonin Reverses H2 O2 -Induced Senescence in SH-SY5Y Cells by Enhancing Autophagy via Sirtuin 1 Deacetylation of the RelA/p65 Subunit of NF-Kappab. J Pineal Res (2017) 63(1):1–13. doi: 10.1111/jpi.12407
80. Li J, Ling Y, Yin S, Yang S, Kong M, Li Z. Baicalin Serves a Protective Role in Diabetic Nephropathy Through Preventing High Glucose-Induced Podocyte Apoptosis. Exp Ther Med (2020) 20(1):367–74. doi: 10.3892/etm.2020.8701
81. Alzahrani S, Zaitone SA, Said E, El-Sherbiny M, Ajwah S, Alsharif SY, et al. Protective Effect of Isoliquiritigenin on Experimental Diabetic Nephropathy in Rats: Impact on Sirt-1/NFkappaB Balance and NLRP3 Expression. Int Immunopharmacol (2020) 87:106813. doi: 10.1016/j.intimp.2020.106813
82. Wang X, Gao Y, Tian N, Wang T, Shi Y, Xu J, et al. Astragaloside IV Inhibits Glucose-Induced Epithelial-Mesenchymal Transition of Podocytes Through Autophagy Enhancement via the SIRT-NF-kappaB P65 Axis. Sci Rep (2019) 9(1):323. doi: 10.1038/s41598-018-36911-1
83. Du YG, Zhang KN, Gao ZL, Dai F, Wu XX, Chai KF. Tangshen Formula Improves Inflammation in Renal Tissue of Diabetic Nephropathy Through SIRT1/NF-kappaB Pathway. Exp Ther Med (2018) 15(2):2156–64. doi: 10.3892/etm.2017.5621
84. Chen Y, Liang Y, Hu T, Wei R, Cai C, Wang P, et al. Endogenous Nampt Upregulation is Associated With Diabetic Nephropathy Inflammatory-Fibrosis Through the NF-kappaB P65 and Sirt1 Pathway; NMN Alleviates Diabetic Nephropathy Inflammatory-Fibrosis by Inhibiting Endogenous Nampt. Exp Ther Med (2017) 14(5):4181–93. doi: 10.3892/etm.2017.5098
85. Wang X, Gao Y, Tian N, Zhu Z, Wang T, Xu J, et al. Astragaloside IV Represses High Glucose-Induced Mesangial Cells Activation by Enhancing Autophagy via SIRT1 Deacetylation of NF-kappaB P65 Subunit. Drug Des Devel Ther (2018) 12:2971–80. doi: 10.2147/DDDT.S174058
86. Xu F, Ye Z, Tao S, Liu W, Su J, Fang X, et al. Ligustilide Alleviates Podocyte Injury via Suppressing the SIRT1/NF-kappaB Signaling Pathways in Rats With Diabetic Nephropathy. Ann Transl Med (2020) 8(18):1154. doi: 10.21037/atm-20-5811
87. Feng J, Bao L, Wang X, Li H, Chen Y, Xiao W, et al. Low Expression of HIV Genes in Podocytes Accelerates the Progression of Diabetic Kidney Disease in Mice. Kidney Int (2021) 99(4):914–25. doi: 10.1016/j.kint.2020.12.012
88. Zheng XP, Nie Q, Feng J, Fan XY, Jin YL, Chen G, et al. Kidney-Targeted Baicalin-Lysozyme Conjugate Ameliorates Renal Fibrosis in Rats With Diabetic Nephropathy Induced by Streptozotocin. BMC Nephrol (2020) 21(1):174. doi: 10.1186/s12882-020-01833-6
89. Du YG, Wang LP, Qian JW, Zhang KN, Chai KF. Panax Notoginseng Saponins Protect Kidney From Diabetes by Up-Regulating Silent Information Regulator 1 and Activating Antioxidant Proteins in Rats. Chin J Integr Med (2016) 22(12):910–7. doi: 10.1007/s11655-015-2446-1
90. Sun HJ, Xiong SP, Cao X, Cao L, Zhu MY, Wu ZY, et al. Polysulfide-Mediated Sulfhydration of SIRT1 Prevents Diabetic Nephropathy by Suppressing Phosphorylation and Acetylation of P65 NF-kappaB and STAT3. Redox Biol (2021) 38:101813. doi: 10.1016/j.redox.2020.101813
91. Munoz-Felix JM, Gonzalez-Nunez M, Martinez-Salgado C, Lopez-Novoa JM. TGF-Beta/BMP Proteins as Therapeutic Targets in Renal Fibrosis. Where Have We Arrived After 25 Years of Trials and Tribulations? Pharmacol Ther (2015) 156:44–58. doi: 10.1016/j.pharmthera.2015.10.003
92. Meng XM, Nikolic-Paterson DJ, Lan HY. TGF-Beta: The Master Regulator of Fibrosis. Nat Rev Nephrol (2016) 12(6):325–38. doi: 10.1038/nrneph.2016.48
93. Sutariya B, Jhonsa D, Saraf MN. TGF-Beta: The Connecting Link Between Nephropathy and Fibrosis. Immunopharmacol Immunotoxicol (2016) 38(1):39–49. doi: 10.3109/08923973.2015.1127382
94. Frangogiannis N. Transforming Growth Factor-Beta in Tissue Fibrosis. J Exp Med (2020) 217(3):e20190103. doi: 10.1084/jem.20190103
95. Xue M, Li Y, Hu F, Jia YJ, Zheng ZJ, Wang L, et al. High Glucose Up-Regulates microRNA-34a-5p to Aggravate Fibrosis by Targeting SIRT1 in HK-2cells. Biochem Biophys Res Commun (2018) 498(1):38–44. doi: 10.1016/j.bbrc.2017.12.048
96. Yu WC, Huang RY, Chou TC. Oligo-Fucoidan Improves Diabetes-Induced Renal Fibrosis via Activation of Sirt-1, GLP-1R, and Nrf2/HO-1: An In Vitro and In Vivo Study. Nutrients (2020) 12(10):1–15. doi: 10.3390/nu12103068
97. Li C, Cai F, Yang Y, Zhao X, Wang C, Li J, et al. Tetrahydroxystilbene Glucoside Ameliorates Diabetic Nephropathy in Rats: Involvement of SIRT1 and TGF-Beta1 Pathway. Eur J Pharmacol (2010) 649(1-3):382–9. doi: 10.1016/j.ejphar.2010.09.004
98. Zhang J, Zhang L, Zha D, Wu X. Inhibition of Mirna135a5p Ameliorates TGFbeta1induced Human Renal Fibrosis by Targeting SIRT1 in Diabetic Nephropathy. Int J Mol Med (2020) 46(3):1063–73. doi: 10.3892/ijmm.2020.4647
99. Wang X, Ji T, Li X, Qu X, Bai S. FOXO3a Protects Against Kidney Injury in Type II Diabetic Nephropathy by Promoting Sirt6 Expression and Inhibiting Smad3 Acetylation. Oxid Med Cell Longev (2021) 2021:5565761. doi: 10.1155/2021/5565761
100. Huang XF, Chen JZ. Obesity, the PI3K/Akt Signal Pathway and Colon Cancer. Obes Rev (2009) 10(6):610–6. doi: 10.1111/j.1467-789X.2009.00607.x
101. du Rusquec P, Blonz C, Frenel JS, Campone M. Targeting the PI3K/Akt/mTOR Pathway in Estrogen-Receptor Positive HER2 Negative Advanced Breast Cancer. Ther Adv Med Oncol (2020) 12:1758835920940939. doi: 10.1177/1758835920940939
102. Burgering BM, Kops GJ. Cell Cycle and Death Control: Long Live Forkheads. Trends Biochem Sci (2002) 27(7):352–60. doi: 10.1016/s0968-0004(02)02113-8
103. Hussein MM, Mahfouz MK. Effect of Resveratrol and Rosuvastatin on Experimental Diabetic Nephropathy in Rats. BioMed Pharmacother (2016) 82:685–92. doi: 10.1016/j.biopha.2016.06.004
104. Wang X, Meng L, Zhao L, Wang Z, Liu H, Liu G, et al. Resveratrol Ameliorates Hyperglycemia-Induced Renal Tubular Oxidative Stress Damage via Modulating the SIRT1/FOXO3a Pathway. Diabetes Res Clin Pract (2017) 126:172–81. doi: 10.1016/j.diabres.2016.12.005
105. Wu L, Zhang Y, Ma X, Zhang N, Qin G. The Effect of Resveratrol on FoxO1 Expression in Kidneys of Diabetic Nephropathy Rats. Mol Biol Rep (2012) 39(9):9085–93. doi: 10.1007/s11033-012-1780-z
106. Yang G, Jin L, Zheng D, Tang X, Yang J, Fan L, et al. Fucoxanthin Alleviates Oxidative Stress Through Akt/Sirt1/FoxO3alpha Signaling to Inhibit HG-Induced Renal Fibrosis in GMCs. Mar Drugs (2019) 17(12):1–16. doi: 10.3390/md17120702
107. Mori J, Patel VB, Ramprasath T, Alrob OA, DesAulniers J, Scholey JW, et al. Angiotensin 1-7 Mediates Renoprotection Against Diabetic Nephropathy by Reducing Oxidative Stress, Inflammation, and Lipotoxicity. Am J Physiol Renal Physiol (2014) 306(8):F812–21. doi: 10.1152/ajprenal.00655.2013
108. Dusabimana T, Kim SR, Park EJ, Je J, Jeong K, Yun SP, et al. P2Y2R Contributes to the Development of Diabetic Nephropathy by Inhibiting Autophagy Response. Mol Metab (2020) 42:101089. doi: 10.1016/j.molmet.2020.101089
109. Wang Z, Li Y, Wang Y, Zhao K, Chi Y, Wang B. Pyrroloquinoline Quinine Protects HK-2cells Against High Glucose-Induced Oxidative Stress and Apoptosis Through Sirt3 and PI3K/Akt/FoxO3a Signaling Pathway. Biochem Biophys Res Commun (2019) 508(2):398–404. doi: 10.1016/j.bbrc.2018.11.140
110. Zhou D, Zhou M, Wang Z, Fu Y, Jia M, Wang X, et al. PGRN Acts as a Novel Regulator of Mitochondrial Homeostasis by Facilitating Mitophagy and Mitochondrial Biogenesis to Prevent Podocyte Injury in Diabetic Nephropathy. Cell Death Dis (2019) 10(7):524. doi: 10.1038/s41419-019-1754-3
111. Kopacz A, Kloska D, Forman HJ, Jozkowicz A, Grochot-Przeczek A. Beyond Repression of Nrf2: An Update on Keap1. Free Radic Biol Med (2020) 157:63–74. doi: 10.1016/j.freeradbiomed.2020.03.023
112. Huang K, Chen C, Hao J, Huang J, Wang S, Liu P, et al. Polydatin Promotes Nrf2-ARE Anti-Oxidative Pathway Through Activating Sirt1 to Resist AGEs-Induced Upregulation of Fibronetin and Transforming Growth Factor-Beta1 in Rat Glomerular Messangial Cells. Mol Cell Endocrinol (2015) 399:178–89. doi: 10.1016/j.mce.2014.08.014
113. Zhuang K, Jiang X, Liu R, Ye C, Wang Y, Wang Y, et al. Formononetin Activates the Nrf2/ARE Signaling Pathway Via Sirt1 to Improve Diabetic Renal Fibrosis. Front Pharmacol (2020) 11:616378. doi: 10.3389/fphar.2020.616378
114. Huang K, Huang J, Xie X, Wang S, Chen C, Shen X, et al. Sirt1 Resists Advanced Glycation End Products-Induced Expressions of Fibronectin and TGF-Beta1 by Activating the Nrf2/ARE Pathway in Glomerular Mesangial Cells. Free Radic Biol Med (2013) 65:528–40. doi: 10.1016/j.freeradbiomed.2013.07.029
115. Ma F, Wu J, Jiang Z, Huang W, Jia Y, Sun W, et al. P53/NRF2 Mediates SIRT1's Protective Effect on Diabetic Nephropathy. Biochim Biophys Acta Mol Cell Res (2019) 1866(8):1272–81. doi: 10.1016/j.bbamcr.2019.04.006
116. Huang X, Shi Y, Chen H, Le R, Gong X, Xu K, et al. Isoliquiritigenin Prevents Hyperglycemia-Induced Renal Injuries by Inhibiting Inflammation and Oxidative Stress via SIRT1-Dependent Mechanism. Cell Death Dis (2020) 11(12):1040. doi: 10.1038/s41419-020-03260-9
117. Shao Y, Lv C, Wu C, Zhou Y, Wang Q. Mir-217 Promotes Inflammation and Fibrosis in High Glucose Cultured Rat Glomerular Mesangial Cells via Sirt1/HIF-1alpha Signaling Pathway. Diabetes Metab Res Rev (2016) 32(6):534–43. doi: 10.1002/dmrr.2788
118. Li A, Peng R, Sun Y, Liu H, Peng H, Zhang Z. LincRNA 1700020I14Rik Alleviates Cell Proliferation and Fibrosis in Diabetic Nephropathy via miR-34a-5p/Sirt1/HIF-1alpha Signaling. Cell Death Dis (2018) 9(5):461. doi: 10.1038/s41419-018-0527-8
119. Sun X, Huang K, Haiming X, Lin Z, Yang Y, Zhang M, et al. Connexin 43 Prevents the Progression of Diabetic Renal Tubulointerstitial Fibrosis by Regulating the SIRT1-HIF-1alpha Signaling Pathway. Clin Sci (Lond) (2020) 134(13):1573–92. doi: 10.1042/CS20200171
120. Liu R, Zhong Y, Li X, Chen H, Jim B, Zhou MM, et al. Role of Transcription Factor Acetylation in Diabetic Kidney Disease. Diabetes (2014) 63(7):2440–53. doi: 10.2337/db13-1810
121. Kundu A, Richa S, Dey P, Kim KS, Son JY, Kim HR, et al. Protective Effect of EX-527 Against High-Fat Diet-Induced Diabetic Nephropathy in Zucker Rats. Toxicol Appl Pharmacol (2020) 390:114899. doi: 10.1016/j.taap.2020.114899
122. Zitman-Gal T, Einbinder Y, Ohana M, Katzav A, Kartawy A, Benchetrit S. Effect of Liraglutide on the Janus Kinase/Signal Transducer and Transcription Activator (JAK/STAT) Pathway in Diabetic Kidney Disease in Db/Db Mice and in Cultured Endothelial Cells. J Diabetes (2019) 11(8):656–64. doi: 10.1111/1753-0407.12891
123. Wang D, Li Y, Wang N, Luo G, Wang J, Luo C, et al. 1alpha,25-Dihydroxyvitamin D3 Prevents Renal Oxidative Damage via the PARP1/SIRT1/NOX4 Pathway in Zucker Diabetic Fatty Rats. Am J Physiol Endocrinol Metab (2020) 318(3):E343–E56. doi: 10.1152/ajpendo.00270.2019
124. Li X, Cai W, Lee K, Liu B, Deng Y, Chen Y, et al. Puerarin Attenuates Diabetic Kidney Injury Through the Suppression of NOX4 Expression in Podocytes. Sci Rep (2017) 7(1):14603. doi: 10.1038/s41598-017-14906-8
125. Scuto M, Trovato Salinaro A, Modafferi S, Polimeni A, Pfeffer T, Weigand T, et al. Carnosine Activates Cellular Stress Response in Podocytes and Reduces Glycative and Lipoperoxidative Stress. Biomedicines (2020) 8(6):1–14. doi: 10.3390/biomedicines8060177
126. Peters V, Calabrese V, Forsberg E, Volk N, Fleming T, Baelde H, et al. Protective Actions of Anserine Under Diabetic Conditions. Int J Mol Sci (2018) 19(9):1–12. doi: 10.3390/ijms19092751
127. Zeng S, Wu X, Chen X, Xu H, Zhang T, Xu Y. Hypermethylated in Cancer 1 (HIC1) Mediates High Glucose Induced ROS Accumulation in Renal Tubular Epithelial Cells by Epigenetically Repressing SIRT1 Transcription. Biochim Biophys Acta Gene Regul Mech (2018) 1861(10):917–27. doi: 10.1016/j.bbagrm.2018.08.002
128. Wang XX, Wang D, Luo Y, Myakala K, Dobrinskikh E, Rosenberg AZ, et al. FXR/TGR5 Dual Agonist Prevents Progression of Nephropathy in Diabetes and Obesity. J Am Soc Nephrol (2018) 29(1):118–37. doi: 10.1681/ASN.2017020222
129. Umino H, Hasegawa K, Minakuchi H, Muraoka H, Kawaguchi T, Kanda T, et al. High Basolateral Glucose Increases Sodium-Glucose Cotransporter 2 and Reduces Sirtuin-1 in Renal Tubules Through Glucose Transporter-2 Detection. Sci Rep (2018) 8(1):6791. doi: 10.1038/s41598-018-25054-y
130. Tang LX, Wang B, Wu ZK. Aerobic Exercise Training Alleviates Renal Injury by Interfering With Mitochondrial Function in Type-1 Diabetic Mice. Med Sci Monit (2018) 24:9081–9. doi: 10.12659/MSM.912877
131. Ogura Y, Kitada M, Xu J, Monno I, Koya D. CD38 Inhibition by Apigenin Ameliorates Mitochondrial Oxidative Stress Through Restoration of the Intracellular NAD(+)/NADH Ratio and Sirt3 Activity in Renal Tubular Cells in Diabetic Rats. Aging (Albany NY) (2020) 12(12):11325–36. doi: 10.18632/aging.103410
132. Li J, Liu H, Takagi S, Nitta K, Kitada M, Srivastava SP, et al. Renal Protective Effects of Empagliflozin via Inhibition of EMT and Aberrant Glycolysis in Proximal Tubules. JCI Insight (2020) 5(6):1–17. doi: 10.1172/jci.insight.129034
133. Li J, Li N, Yan S, Lu Y, Miao X, Gu Z, et al. Liraglutide Protects Renal Mesangial Cells Against Hyperglycemiamediated Mitochondrial Apoptosis by Activating the ERKYap Signaling Pathway and Upregulating Sirt3 Expression. Mol Med Rep (2019) 19(4):2849–60. doi: 10.3892/mmr.2019.9946
134. Wang XX, Edelstein MH, Gafter U, Qiu L, Luo Y, Dobrinskikh E, et al. G Protein-Coupled Bile Acid Receptor TGR5 Activation Inhibits Kidney Disease in Obesity and Diabetes. J Am Soc Nephrol (2016) 27(5):1362–78. doi: 10.1681/ASN.2014121271
135. Feng J, Lu C, Dai Q, Sheng J, Xu M. SIRT3 Facilitates Amniotic Fluid Stem Cells to Repair Diabetic Nephropathy Through Protecting Mitochondrial Homeostasis by Modulation of Mitophagy. Cell Physiol Biochem (2018) 46(4):1508–24. doi: 10.1159/000489194
136. Muraoka H, Hasegawa K, Sakamaki Y, Minakuchi H, Kawaguchi T, Yasuda I, et al. Role of Nampt-Sirt6 Axis in Renal Proximal Tubules in Extracellular Matrix Deposition in Diabetic Nephropathy. Cell Rep (2019) 27(1):199–212.e5. doi: 10.1016/j.celrep.2019.03.024
137. Wang X, Lin B, Nie L, Li P. microRNA-20b Contributes to High Glucose-Induced Podocyte Apoptosis by Targeting SIRT7. Mol Med Rep (2017) 16(4):5667–74. doi: 10.3892/mmr.2017.7224
138. Howitz KT, Bitterman KJ, Cohen HY, Lamming DW, Lavu S, Wood JG, et al. Small Molecule Activators of Sirtuins Extend Saccharomyces Cerevisiae Lifespan. Nature (2003) 425(6954):191–6. doi: 10.1038/nature01960
139. Domi E, Hoxha M, Prendi E, Zappacosta B. A Systematic Review on the Role of SIRT1 in Duchenne Muscular Dystrophy. Cells (2021) 10(6):1–28. doi: 10.3390/cells10061380
140. Scher MB, Vaquero A, Reinberg D. SirT3 is a Nuclear NAD+-Dependent Histone Deacetylase That Translocates to the Mitochondria Upon Cellular Stress. Genes Dev (2007) 21(8):920–8. doi: 10.1101/gad.1527307
141. Akter R, Afrose A, Rahman MR, Chowdhury R, Nirzhor SSR, Khan RI, et al. A Comprehensive Analysis Into the Therapeutic Application of Natural Products as SIRT6 Modulators in Alzheimer's Disease, Aging, Cancer, Inflammation, and Diabetes. Int J Mol Sci (2021) 22(8):1–23. doi: 10.3390/ijms22084180
142. Gu J, Yang M, Qi N, Mei S, Chen J, Song S, et al. Olmesartan Prevents Microalbuminuria in db/db Diabetic Mice Through Inhibition of Angiotensin II/p38/SIRT1-Induced Podocyte Apoptosis. Kidney Blood Press Res (2016) 41(6):848–64. doi: 10.1159/000452588
143. Kumar GS, Kulkarni A, Khurana A, Kaur J, Tikoo K. Selenium Nanoparticles Involve HSP-70 and SIRT1 in Preventing the Progression of Type 1 Diabetic Nephropathy. Chem Biol Interact (2014) 223:125–33. doi: 10.1016/j.cbi.2014.09.017
144. Lo CS, Shi Y, Chenier I, Ghosh A, Wu CH, Cailhier JF, et al. Heterogeneous Nuclear Ribonucleoprotein F Stimulates Sirtuin-1 Gene Expression and Attenuates Nephropathy Progression in Diabetic Mice. Diabetes (2017) 66(7):1964–78. doi: 10.2337/db16-1588
145. Sun Z, Ma Y, Chen F, Wang S, Chen B, Shi J. miR-133b and miR-199b Knockdown Attenuate TGF-beta1-iIduced Epithelial to Mesenchymal Transition and Renal Fibrosis By Targeting SIRT1 in Diabetic Nephropathy. Eur J Pharmacol (2014) 223:125–33. doi: 10.1016/j.cbi.2014.09.017
146. Zhu H, Fang Z, Chen J, Yang Y, Gan J, Luo L, et al. PARP-1 and SIRT-1 Are Interacted in Diabetic Nephropathy By Activating AMPK/PGC-1alpha Signaling Pathway. Diabetes Metab Syndr Obes (2021) 14:355–66. doi: 10.2147/DMSO.S291314
147. Zhou L, Xu DY, Sha WG, Shen L, Lu GY. Long Non-Coding RNA MALAT1 Interacts With Transcription Factor Foxo1 to Regulate SIRT1 Transcription in High Glucose-Induced HK-2 Cells Injury. Biochem Biophys Res Commun (2018) 503(2):849–55. doi: 10.1016/j.bbrc.2018.06.086
148. Huang KP, Chen C, Hao J, Huang JY, Liu PQ, Huang HQ. AGEs-RAGE System Down-Regulates Sirt1 Through the Ubiquitin-Proteasome Pathway to Promote FN and TGF-Beta1 Expression in Male Rat Glomerular Mesangial Cells. Endocrinology (2015) 156(1):268–79. doi: 10.1210/en.2014-1381
Keywords: biological function, signaling pathway, diabetes kidney disease, pathological process, sirtuins
Citation: Qi W, Hu C, Zhao D and Li X (2022) SIRT1–SIRT7 in Diabetic Kidney Disease: Biological Functions and Molecular Mechanisms. Front. Endocrinol. 13:801303. doi: 10.3389/fendo.2022.801303
Received: 25 October 2021; Accepted: 15 April 2022;
Published: 13 May 2022.
Edited by:
Ronadip R Banerjee, Johns Hopkins Medicine, United StatesReviewed by:
Sungjin Chung, Catholic University of Korea, South KoreaPawan Kumar Kare, Gandhi Medical College Bhopal, India
Copyright © 2022 Qi, Hu, Zhao and Li. This is an open-access article distributed under the terms of the Creative Commons Attribution License (CC BY). The use, distribution or reproduction in other forums is permitted, provided the original author(s) and the copyright owner(s) are credited and that the original publication in this journal is cited, in accordance with accepted academic practice. No use, distribution or reproduction is permitted which does not comply with these terms.
*Correspondence: Wenxiu Qi, cWl3ZW54aXUwNTE3QDE2My5jb20=
†These authors have contributed equally to this work