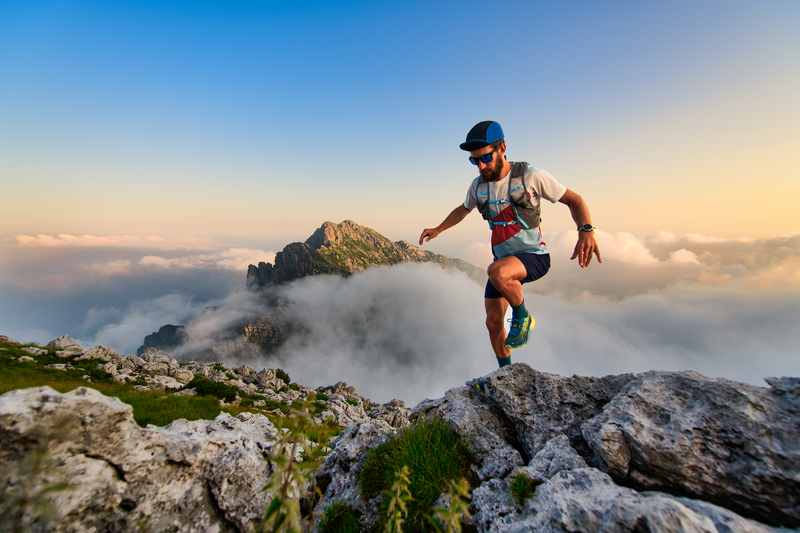
95% of researchers rate our articles as excellent or good
Learn more about the work of our research integrity team to safeguard the quality of each article we publish.
Find out more
REVIEW article
Front. Endocrinol. , 03 May 2022
Sec. Neuroendocrine Science
Volume 13 - 2022 | https://doi.org/10.3389/fendo.2022.799521
This article is part of the Research Topic Neuroendocrine Research in Health and Disease Volume II View all 7 articles
Coronavirus disease 2019 or COVID-19 caused by severe acute respiratory syndrome coronavirus 2 (SARS-CoV-2) has become a significant threat to the health of human beings. While wearing mask, maintaining social distance and performing self-quarantine can reduce virus spreading passively, vaccination actively enhances immune defense against COVID-19. However, mutations of SARS-CoV-2 and presence of asymptomatic carriers frustrate the effort of completely conquering COVID-19. A strategy that can reduce the susceptibility and thus prevent COVID-19 while blocking viral invasion and pathogenesis independent of viral antigen stability is highly desirable. In the pathogenesis of COVID-19, endocrine disorders have been implicated. Correspondingly, many hormones have been identified to possess therapeutic potential of treating COVID-19, such as estrogen, melatonin, corticosteroids, thyroid hormone and oxytocin. Among them, oxytocin has the potential of both treatment and prevention of COVID-19. This is based on oxytocin promotion of immune-metabolic homeostasis, suppression of inflammation and pre-existing comorbidities, acceleration of damage repair, and reduction of individuals’ susceptibility to pathogen infection. Oxytocin may specifically inactivate SARS-COV-2 spike protein and block viral entry into cells via angiotensin-converting enzyme 2 by suppressing serine protease and increasing interferon levels and number of T-lymphocytes. In addition, oxytocin can promote parasympathetic outflow and the secretion of body fluids that could dilute and even inactivate SARS-CoV-2 on the surface of cornea, oral cavity and gastrointestinal tract. What we need to do now is clinical trials. Such trials should fully balance the advantages and disadvantages of oxytocin application, consider the time- and dose-dependency of oxytocin effects, optimize the dosage form and administration approach, combine oxytocin with inhibitors of SARS-CoV-2 replication, apply specific passive immunization, and timely utilize efficient vaccines. Meanwhile, blocking COVID-19 transmission chain and developing other efficient anti-SARS-CoV-2 drugs are also important. In addition, relative to the complex issues with drug applications over a long term, oxytocin can be mobilized through many physiological stimuli, and thus used as a general prevention measure. In this review, we explore the potential of oxytocin for treatment and prevention of COVID-19 and perhaps other similar pathogens.
Coronavirus disease 2019 or COVID-19 is caused by severe acute respiratory syndrome (SARS) coronavirus 2 (CoV) or SARS-CoV-2. It has high morbidity and mortality and constitutes severe threats to human healthcare system. According to the report of Worldometers (https://www.worldometers.info/coronavirus/) on March 7, 2022, the confirmed cases of COVID-19 reached ~447.4 million including ~60.5 million active cases and ~6.0 million deaths. SARS-CoV-2 appears highly contagious; severe COVID-19 has poor prognosis; its treatments largely resolve symptoms only. While vaccination is available, there has been a substantial anti-vaccination pushback. Thus, exploration of novel prevention and treatment strategies that are independent of vaccination becomes essential for conquering COVID-19. In this review, based on knowledge about the correlation between COVID-19 pathogenesis and endocrine disorders, we explore the therapeutic potential of oxytocin against COVID-19 and the prevention value of mobilizing endogenous oxytocin secretion.
SARS-CoV-2 virus belongs to the family of coronaviruses that can cause diseases like severe acute respiratory syndrome or SARS, and Middle East respiratory syndrome or MERS. It has many special features in the epidemiology and pathogenesis.
Human-to-human transmission of SARS-CoV-2 has been confirmed. Small droplets and aerosols containing the virus can spread from an infected person’s nose and mouth as they breathe, cough, sneeze, sing, or speak. Recently, while bats are considered as the reservoir host, potential intermediate hosts are also identified such as pangolins (1) and tree shrews (2). In addition to the wild animals, angiotensin-converting enzyme 2 (ACE2), viral receptor proteins from dog, cat, and cattle are found to be the most permissive route to SARS-CoV-2 entry (3). Since these domestic animals have intimate contact with humans, the possibility for animal-to-human transmission cannot be excluded. Correspondingly, they should be monitored as potential intermediate hosts.
Compared to the youngsters, more severe diseases of COVID-19 are associated with elderly, immune-suppressed, and those who have existing co-morbidities such as coronary artery diseases, hypertension, and diabetes. However, young people are also a target of SARS-CoV-2, particularly among those who are unvaccinated. According to an investigation during May 1-July 25, 2021, among 43,127 reported SARS-CoV-2 infections in Los Angeles County residents aged ≥16 years, 30,801 (71.4%) were in unvaccinated persons, and 10,895 (25.3%) were in fully vaccinated persons. Much lower percentages of fully vaccinated persons infected with SARS-CoV-2 were hospitalized (3.2%) and were admitted to an intensive care unit (0.5%) compared with unvaccinated persons (7.6%, and 1.5%, respectively). However, the percentages of Delta variant infections estimated from 6,752 samples with lineage data increased among fully vaccinated persons (from 8.6% to 91.2%), and unvaccinated persons (from 8.2% to 87.1%) (4). Amidst circulation of the Delta variant, individuals aged 12 years and older in schools also become high-risk population (5, 6). Thus, COVID-19 targets all age groups, particularly the unvaccinated population with neonates as an exception (7).
Most people infected with the SARS-CoV-2 experience mild to moderate respiratory illness and recover spontaneously; however, elderly, and those who had underlying medical problems are more likely to develop serious conditions (8, 9). Thus, direct exposure to the virus, lack of vaccination and poor health conditions are the key factors determining individuals’ susceptibility to COVID-19.
In the efforts of controlling COVID-19, while vaccination has been applied extensively, many drugs have also been explored (10, 11). Recently, molnupiravir has been considered as a breakthrough of COVID-19 treatment since it can reduce the risk of severe cases and mortality to 50% (12). Moreover, Pfizer reported that a 5-day paxlovid pill regimen to treat early infections with SARS-CoV-2 is 89% effective in patients at risk of serious illness (13, 14). Both drugs have gained emergency use authorization by the U.S. Food and Drug Administration [Coronavirus (COVID-19) Update: FDA Authorizes Additional Oral Antiviral for Treatment of COVID-19 in Certain Adults | FDA] (https://www.fda.gov/news-events/press-announcements/coronavirus-covid-19-update-fda-authorizes-first-oral-antiviral-treatment-covid-19). However, the effectiveness of molnupiravir and paxlovid are limited within a narrow window at the early stage of COVID-19. In addition, incomplete inhibition of viral replication and subsequent drug-resistance remain to be monitored. Importantly, some potentially effective drugs act as a double-edged sword, such as ACE inhibitor captopril that decreases tissue anti-inflammatory response while reducing viral entry (15). In addition, after controlling symptoms of severe COVID-19 patients during acute phase, long term sequelae remain a challenge for rehabilitation. Therefore, further clarification of the underlying mechanism and exploration of novel treatment and prevention strategies of COVID-19 are highly demanded.
Among current measures of prevention, passive prevention measures like wearing mask, social distancing and self-isolation could not provide sufficient protection (16) even for those who have received two doses of vaccination (4). It is true that vaccination can substantially reduce the morbidity and mortality at early stage of COVID-19 pandemic; however, when the dominant variants changed from Alpha to Delta and Omicron variants, the initial protective effect of vaccination droped dramatically (17–19). This is because Delta S protein can fuse membranes more efficiently at low levels of cellular receptor ACE2 and its pseudotyped viruses infect target cells substantially faster than all previous variants tested, thereby heightening its transmissibility (20). Similarly, passive immunization by giving antibody isolated from the plasma of recovered patients to another individual who is at risk of infection can provide instant, short-term fortification against infectious agents and slow down the spread of COVID-19 (21, 22). Recent construction of neutralizing nanobodies that target the receptor binding domain of the SARS-CoV-2 spike (S) protein seems more effective that natural antibodies against COVID-19 (23), albeit this remains to be tested in clinical setting.
The latest SARS-CoV-2 variant Omicron (B.1.1.529) has posed further challenges for the anti-COVID-19 efforts due to its contagiousness and vaccine-escape mutations, the latter involving not only nucleotide substitutions and short deletion but also insertions in the S protein that are predicted to lead to escape from neutralizing antibodies and from T-cell immunity. Omicron variant seems to be ten times more contagious than the original virus, about twice as infectious as the Delta variant, twice more likely to escape current vaccines than the Delta variant, and previous effective antibodies may be seriously compromised (24, 25). Table 1 shows SARS-CoV-2 variants classification and virulence.
Although new vaccine and treatment efficiency remains to be tested, the continuous passive defense against COVID-19 reaches a turning point for seeking more stable protective measures. Correspondingly, prevention of COVID-19 should depend not only on current passive measures of prevention and vaccination, but also on boosting individuals’ immunity against COVID-19 while developing more effective treatment strategies.
Understanding of COVID-19 pathogenesis is the prerequisite to treat and prevent COVID-19. Viral entry into host cells is mainly mediated by the binding of its S protein to ACE2 receptor (33). The activation of S protein requires the cleavage of a transmembrane serine protease 2 (TMPRSS2) (34) and furin (35) on ACE2-positive cells. ACE2-positive cells include lung alveolar epithelial cells, enterocytes of the small intestine, renal tubules, heart, arterial and venous endothelial cells, arterial smooth muscle cells, beta cells, cerebral neurons (36–38), as well as monocytes, macrophages and lymphocytes (39). In this process, binding of viral S protein to heparan sulfate proteoglycans (HSPGs) leads to the enrichment of local tissue concentration of SARS-CoV-2 for the subsequent specific binding with ACE2. HSPGs assist ACE2 in endocytosis-mediated SARS-CoV-2 cellular internalization by transiting the virus from the extracellular environment to the inside of cells (40, 41). The endocytosis is clathrin-dependent with virus sequestered into an endosome (42). In the endosome, the lysosomal endopeptidase cathepsin B/L can facilitate the fusion of virus with endosomal membranes. SARS-CoV-2 then exploits the endogenous transcriptional machinery of infected cells to replicate and spread through the entire organ (43). Many cellular host factors and the innate immune cells act to inhibit virus entry and intracellular replication through pattern-recognition receptors and intracellular RNA sensor molecules (44, 45). However, the non-structural proteins of the virus can hijack them by recruiting host partners to form hetero-oligomeric complexes and then suppress interferon synthesis and its antiviral effects (46). Thus, multiple cellular components are involved in the invasion of SARS-CoV-2 and they can be the targets for blocking SARS-CoV-2 infection.
After infecting target cells, SARS-CoV-2 completes its own replication inside of the cell and then releases itself outside of the cell, thereby destroying the old cells and infecting new ones. In this process, the amount of cell partitioned ACE2 is also reduced, which results in an elevation of angiotensin II concentration in the blood and inflammation (47). In response to viral attacks, the body first initiates an innate immune antiviral response by recruiting neutrophils and macrophages while releasing cytokines. Then, by activating and recruiting lymphocytes, the body initiates an adaptive immune response that plays a specific antiviral role. In severe cases, particularly in the elderly, the virus can interact with the immune system to induce an excessive innate immune response, or even a cytokine storm, which can disrupt the epithelial cells in the lung and the gas-blood barrier, leading to the acute respiratory distress syndrome (ARDS). At the same time, the virus also attacks lymph tissue and then causes lymphopenia that prevents the virus from clearance (48–50). Correspondingly, relative lymphopenia, cytokine storm, and multiple organ failure become significant indicators of COVID-19 severity and therapeutic targets.
In the pathogenesis of COVID-19, endocrine disorders are a significant feature (51). Studies have revealed that hypothalamus, pituitary, pancreas, thyroid, adrenal glands, testes, and ovaries all express ACE2 (52). Thus, hormones produced in these endocrine organs could all be influenced. In COVID-19 patients, hypothalamic damage with SARS-CoV-2 has been identified, which suppresses hypothalamic neuroendocrine activity (53), similar to some other viral diseases (Table 2). The neural injury caused by SARS-CoV-2 is associated with increased activity of the TMPRSS2 and cathepsin L and secondary increase of angiotensin II following downregulation of ACE2. Resultantly, upregulation of proinflammatory mediators and reactive oxygen species occurs, which causes neuroinflammatory response and blood brain barrier disruption. Furthermore, dysregulation of hormone and neurotransmitter signaling may constitute a fundamental mechanism involved in the neuropathogenic sequelae of SARS-CoV-2 infection (71). Thus, endocrine disorders are present in COVID-19 patients and can play important roles in the development of COVID-19 pathogenesis and prognosis.
Table 2 Effects of viral infection on the oxytocin system, and general antiviral properties and experimental evidence supporting oxytocin anti-COVID-19 functions.
Hypofunctions of the HPA axis have been identified in COVID-19 patients and corticosteroids can effectively alleviate severe ARDS (72). When COVID-19 is more severe, the patients have lower cortisol and adrenocorticotropic hormone levels (73), suggesting a direct link between the COVID-19 infection and impaired glucocorticoid response. However, there is no evidence showing reduced corticotropin-releasing hormone secretion, the top regulator of the HPA axis, and the causal association between the reduced HPA axis activity and COVID-19 severity. In alleviating syndrome of COVID-19, the usage of glucocorticoid hormones can inhibit inflammation and cytokine storm and thus, improve the condition of severe COVID-19 patients by (re)activating ACE2 and reducing interleukin (IL)-6 levels (74). Notably, although the HPA axis activity is deviated during COVID-19, dexamethasone treatment is not applied to simply compensate the insufficiency of corticosteroid production. Dexamethasone is administered to patients under oxygen treatment and having inflammatory symptoms to modulate immune response rather than supplementing basal corticosteroid demand. Glucocorticoid also reduces body’s resistance to viral infection and increases stress reaction (75). Thus, despite that the application of corticosteroids promotes patient’s short-term physical recovery, such as improving hypotension and significant hyponatremia (76), survivors of critical illness have significantly elevated risk of developing lasting cognitive impairment and psychiatric disorders (77).
Similar to the HPA axis, there is also evidence that suggests reduced activity of the hypothalamic pituitary thyroid axis. The levels of total triiodothyronine (T3) and thyroid stimulating hormone (TSH) are lower in COVID-19 patients (78, 79). It was also reported that within the group of COVID-19 cases, 61.9% (52/84) patients presented with thyroid function abnormalities and the proportion of thyroid dysfunction was higher in severe cases than mild/moderate cases. Patients with thyroid dysfunction tended to have longer viral nucleic acid cleaning time in association with decreased number of lymphocytes and increased level of C-reactive protein (80). A positive correlation between low mean T3 level and clinical severity of COVID-19 was also reported (81). Consistently, in six articles qualified for the final analysis which included 1160 patients, most of the patients had lower mean T3 level and normal or low TSH level. Clinically, thyroid hormones may relieve hypothalamic hypometabolism in COVID-19 patients (53). However, the complex interplay between thyroid hormone action and the immune system is still not completely understood (82).
Relative to other hormones, the involvement of sex hormones in COVID-19 is more complex. Men have a higher risk of death from COVID-19 than women and androgens may facilitate entrance of the SARS-CoV-2 virus into respiratory epithelial cells (83, 84). Consistently, that androgens promote transcription of TMPRSS2 (85, 86) seems to suggest that higher testosterone levels promote SARS-CoV-2 entry into the cells. Correspondingly, androgen deprivation therapy reduces infection rates and improves outcomes for COVID-19 (87).
However, there is an increased COVID-19 case fatality in the hypogonadotropic hypogonadism cohort, particularly in aged men; this perhaps reflects an underlying pro-inflammatory state (88). Namely, testosterone can suppress both the cellular and humoral immune systems such as lowering IL-6 and tumor necrosis factor-α levels via inhibition of the NF-κB proinflammatory pathway. Low levels of testosterone in aging men are linked with high inflammatory markers such as IL-6 and increased risk of lung damage after pneumonia (89). In addition, androgen deprivation therapy has been associated with adverse cardiovascular events (90), endothelial dysfunction, thrombosis and defective immune response (91). Consistently, the testicle expresses high levels of ACE2, making it a target of SARS-CoV-2, which could account for the low testosterone and high luteinizing hormone levels in COVID-19 males (86). Thus, application of testosterone to COVID-19 patients should be performed with caution.
In contrast to androgens, estrogens produce pro-inflammatory effects. Estrogens increase the immunological defense against pathogens while estradiol can down-regulate ACE2 in the airway epithelium (92), which may reduce viral invasion and make estrogens a good candidate for increasing the immunity against COVID-19 (93). However, estrogens may promote neutrophilic inflammation in subjects with asthma and chronic obstructive pulmonary disease. Age-associated decreases in estrogen may also mediate proinflammatory reactions that could increase patient's risk of COVID-19 adverse outcomes (89). Thus, estrogen may be effective for postmenopausal women but not suitable for women with menstruation who have periodic fluctuations in estrogen versus progesterone levels (94). In addition, hormone replacement therapy in aged women has been found to possess cancer-evoking effect (95). Thus, estrogen cannot be used as a regular agent against COVID-19.
The neurohypophysial hormones include oxytocin and vasopressin. Oxytocin, a classical hypothalamic neuropeptide mainly produced in the supraoptic nucleus and paraventricular nucleus, carries functions in immunologic defense, homeostasis and surveillance, has the properties of anti-virus, anti-inflammation, antibiotics, and cell protection (96, 97) and thus, has the potential to antagonize COVID-19 (Table 2). This view is supported by the following studies. The transcriptomic signature of carbetocin, an oxytocin agonist, has a pattern of concordance with inflammation (i.e. IL-1β and IL-6) and immune marker knockdown signatures that are consistent with reduction of inflammation and promotion of immune response (i.e. T cell and macrophage cell markers like CD40 and ARG1). Importantly, carbetocin is more effective at inducing immune cell responses than either lopinavir or hydroxychloroquine, both of which have been explored for the treatment of COVID-19 (67). Consistently, in screening 2,701 compounds from a commercial library of drugs that potentially block the interaction between the S protein and ACE2, oxytocin is among four best candidates along with thiostrepton, nilotinib, and hydroxycamptothecin (68). This proposal is also in agreement with the view that oxytocin may be repurposed as an agent for treatment of the COVID-19 patients (98–100). Overall, these studies highlight beneficial potential of oxytocin for treating COVID-19 patients. Figure 1 illustrates a variety of oxytocin anti-COVID-19 functions.
Figure 1 Potential preventive functions of oxytocin against coronavirus disease (COVID-19). The red arrows indicate direct activation; the black arrows indicate direct inhibition. ACE2, angiotensin-converting enzyme 2; ARDS, acute respiratory distress syndrome; ATII, angiotensin II; CTL, cytotoxic T lymphocyte; HS, heparan sulphate; HSPG, heparan sulphate proteoglycan; IgA, immunoglobulin A; NK, nature killer cell; PSNA, parasympathetic nerve activation; SARS-CoV-2, severe acute respiratory syndrome coronavirus 2; SNA, sympathetic nerve activation; TH1, T helper cells 1; TMPRSS2, Transmembrane protease serine 2. [Figure is original drawing; also refer to references (96, 100–102)].
Along with oxytocin, vasopressin is also implicated in the treatment of COVID-19. Vasopressin plays a key role for the maintenance of osmotic, cardiovascular, and stress hormone homeostasis during many diseases. Activation of the vasopressin system is a common finding of respiratory infectious diseases; a pronounced activation of the vasopressin system in COVID-19 patients is associated with an adverse clinical course in COVID-19 patients (103). By contrast, vasopressin is commonly used in COVID-19 respiratory failure. This is because vasopressin infusion reduces total norepinephrine requirements for maintaining blood pressure while sparing the pulmonary vasculature (104). COVID-19 patients with respiratory failure experience hypoxic pulmonary vasoconstriction while vasopressin produces less right heart strain than catecholamine. Interestingly, some of these vasopressin functions could be mediated by oxytocin receptor (OTR). For example, in septic shock, vasopressin is commonly used as a vasopressor to restore blood pressure while having anti-inflammatory effects as well. Vasopressin decreases the responsiveness of human aortic endothelial cells to tumor necrosis factor-α by inducing a disintegrin and metalloprotease 10-dependent ectodomain shedding of tumor necrosis factor receptor 1. These effects of vasopressin are blocked by blocking OTR activation (105). This finding again highlights the importance of oxytocin in controlling COVID-19.
The SARS-CoV-2 S protein, soluble ACE2, and vasopressin can form molecular complexes that facilitate cellular infection through endocytosis mediated by vasopressin receptor-1b, and thus vasopressin treatment could promote cellular infection and systemic viral dissemination (106). Therefore, while vasopressin infusion in COVID-19 is beneficial for critical illness, its potential for increasing viral infection limits its regular clinical application.
In addition to the hypothalamic-pituitary hormones, many other hormones are also implicated in COVID-19 pathogenesis. It is well established that the pathology of COVID-19 is strongly related to the renin-angiotensin system, particularly angiotensin II. Cell surface ACE2 is largely lost following SARS-CoV-2 invasion, which destroys infected cells while reducing ACE2 expression and activity. As a result, angiotensin II could not be fully converted to inactive heptapeptide of the angiotensin 1-7 by the remaining ACE2, which increases blood angiotensin II levels (47). Increased levels of angiotensin II can cause neutrophil accumulation, vascular hyper-permeability, and pulmonary edema (107). By contrast, ARDS patients receiving infusion of ACE2 manifest decreased angiotensin II and IL-6 levels, and increased surfactant protein (108). Thus, reducing ACE2 loss and suppression of angiotensin II activity are considered in controlling COVID-19 development (109). However, this measure potentially increases individuals’ susceptibility to re-infection of SARS-CoV-2.
Another important hormone is melatonin. Melatonin has anti-inflammatory and anti-oxidative effects and is protective against ARDS caused by viral and other pathogens. Thus, melatonin may be effective in critical care patients by reducing vessel permeability, anxiety, sedation use, and improving sleep quality (110). Notably, endogenous melatonin is produced in dark but, as social creatures, people cannot stay in dark while maintaining normal social activity. Thus, mobilization of melatonin is not suitable as a prevention measures against COVID-19.
As stated above, increasing density of virions in the body and their entry into the cells are the initial step of COVID-19 pathogenesis, while poor body resistance to viral invasion is the key mechanism. Thus, in addition to the measures of avoiding SARS-CoV-2 contamination, improving immunological condition, reducing the number of virions in the body and blocking viral entry into the cells should be considered. Oxytocin is a strong candidate to carry out these missions through multiple approaches (Figure 1). Table 2 summarizes the effects of viral infection on the oxytocin system, its general antiviral properties and experimental evidence supporting oxytocin anti-COVID-19 functions.
In the pathogenesis of COVID-19, oxytocin neuronal activity is inhibited. In those who are susceptible to COVID-19, such as menopausal women (111, 112), aged man (113) and patients with chronic diseases such as diabetes (114), oxytocin secretion is reduced. In COVID-19 patients, hypothalamic neural activity is low (53, 115), likely because of olfactory bulb-mediated infection of the hypothalamus (116, 117). Consistently, COVID-19 patients have lower circulating levels of natriuretic peptides (118) that are under facilitatory regulation of oxytocin (119). The increased angiotensin II due to reduction of ACE2 (120) can also reduce circulating oxytocin levels (121). Consequently, the insufficiency of oxytocin in the brain and blood can make individuals fall in panic, fear and immune-metabolic disorders (122, 123), thereby reducing resistance to COVID-19. Thus, enhancing basal oxytocin neuronal activity and restoring its activity in COVID-19 patients appear critical for controlling the development of COVID-19 pathogenesis as well as prevention of COVID-19.
Oxytocin, based on its extensive immune-regulating properties (97), has recently been recommended as a candidate to treat pathogenesis of COVID-19 (98, 99, 124). This is because oxytocin can suppress cytokine storm, improve lymphocytopenia, prevent thrombosis, and avoid the occurrence of ARDS and multiple organ failures. Moreover, oxytocin has the potential to reduce COVID-19 susceptibility.
COVID-19 has high morbidity and mortality among elderly and individuals having immune-suppressed, and having existing co-morbidities such as coronary artery diseases, hypertension, diabetes and respiratory system disease (113, 125–127). These basal pathological conditions not only reduce individual’s general resistance to COVID-19 but also increase expressions of ACE2 and serine protease (33, 128), and thus increase body’s susceptibility to COVID-19. Correspondingly, improving immunological conditions and reducing these co-morbidities become necessary to reduce the morbidity and mortality of COVID-19. Oxytocin can suppress hyperlipidemia and hyperglycemia (123) that underlie obesity, atherosclerosis, diabetes and their associated diseases, such as hypertension, coronary artery disease and ischemic stroke (129). Oxytocin has immune-regulating functions (96, 102) and thus makes the body ready to fight against viral infection. Oxytocin has anxiolytic and anti-stress functions (130, 131), which, together with the anti-atherosclerosis effect, exerts anti-hypertension effect. In addition, oxytocin has also the potential to reduce cardiovascular complications and promote tissue regeneration (132). By prevention of these co-morbidities, oxytocin may reduce individuals’ susceptibility to COVID-19.
The onset of COVID-19 depends on the number of virions and their opportunity to bind with ACE2 while the presence of mucus and secretory fluids forms a natural barrier to separate virus from ACE2. The secretion of tears, salivary fluid, and gastric acid is mainly regulated by parasympathetic nerve activity. Increased parasympathetic nerve outflow promotes the secretion of these fluids and thus, can dilute the density of these virions. In addition, immunoglobulin and enzymes in some of these fluids also exert innate anti-viral functions. For example, in seventeen COVID-19 patients, all tear samples showed negative results of SARS-CoV-2 while nasopharyngeal swab samples continued to show positive result (133). It is likely that the lysozyme and immunoglobulin in tears have virus-killing effects (134). In addition, patients who use proton pump inhibitors have significantly higher morbidity of COVID-19 (135), indicating that gastric acid has sterile effect on SARS-CoV-2. Importantly, oxytocin can promote the activity of parasympathetic nerves, particularly the vagus (136), and thus indirectly facilitate the secretion of these body fluids and help remove and weaken the infection by SARS-CoV-2.
As stated above, the entry of SARS-CoV-2 into the cells relies on activation of the S protein by serine proteases, binding of the virus to the cell plasma membrane and endocytosis of viral particles. Oxytocin could interrupt these processes through several approaches, such as blocking the binding of SARS-CoV-2 to HSPG by stabilizing heparan sulphate and inhibiting the activity of serine protease like dipeptidyl peptidase-4 (65). Another potential approach of oxytocin against COVID-19 through HSPGs is its influencing the expression of lactoferrin that can bind to HSPGs and block viral attachment to the host cell (137). In addition to immunoglobulin A, lactoferrin is a natural anti-viral protein in milk (138, 139). The promotion of milk ejection by oxytocin is a natural venue to increase delivery of lactoferrin and immunoglobulin A to babies, and thus increases the defense of babies against COVID-19. This may account for why COVID-19 in neonates is uncommon and the majority of them have either asymptomatic infections or mild disease if infected (7), in which the action of oxytocin is at least a contributing factor.
Moreover, oxytocin has the potential to block the viral entry directly or through regulating the secretion of other hormones. As stated above, oxytocin is one of the four compounds that strongly inhibit binding of SARS-CoV-2 to ACE2 (68). Oxytocin may reduce viral entry through influencing estrogen secretion (94). There is a high rate of asymptomatic infection in pregnant women (140) because of a marked down-regulation of the expression of ACE2 in the airway epithelium (92) and TMPRSS2 (141) and activation of other protective functions (93). By increasing estradiol levels (142), oxytocin can decrease SARS-CoV-2 tropism following reduction in the expression of viral cell entry factors.
Oxytocin can also reduce viral entry through other approaches. Cigarette smoke causes a dose-dependent upregulation of ACE2 in a subset of secretory cells in the respiratory tract in rodent and human lungs (143). Intranasal administration of oxytocin reduces smoking craving (144) and thus may reduce the susceptibility to COVID-19. In addition, oxytocin can accelerate wound healing such as the lesions of skin (145), stomach (146), and intestine (147), and thus can strengthen the barrier against viral invasion. As a whole, oxytocin potentially suppresses the activation of S protein and viral entry into ACE2-positive cells.
During COVID-19 pandemic, mental health is a significant concern. Lockdown, quarantine measures, and social distancing are associated with increased depression, anxiety and distress during the pandemic (148). These psychological problems adversely affect immune functions and increase the severity of COVID-19-associated disorders and death rates (149). The perception of stress, isolation, and guilt among younger people was associated with poor mental health outcomes during COVID-19 (150, 151) and greater COVID-related social risk-taking behaviors, such as making and visiting new friends in person (152). Thus, social stress can worsen the pathogenesis in COVID-19 patients while increasing individuals’ susceptibility to SARS-CoV-2 infection.
In relieving COVID-19-related social stress, oxytocin is clearly a strong candidate. Stress-related to social isolation leads to downregulation of OTR in the ventral striatum in association with subjective distress and touch starvation (153). Oxytocin has anxiolytic and anti-stress functions, and thus can reduce the adverse neuroendocrine, autonomic, and behavioral responses (111). For instance, sexual intimacy involves increased oxytocin-associated activity in the limbic structures, nucleus accumbens, anterior cingulate, and prefrontal cortex, which help reduce stress and anxiety as well as their associated psychoneuroimmunity (154). Together with views of other researchers (155, 156), we believe that during the COVID-19 pandemic, activation of the endogenous oxytocin system or intranasal application of oxytocin should help resolve social isolation-induced inactivation of the oxytocin system and psychophysical disorders.
Currently, there is one clinical trial that has been listed on https://clinicaltrials.gov first on May 13, 2020. It is a “Phase II RCT to Assess Efficacy of Intravenous Administration of Oxytocin in Patients Affected by COVID-19” (NCT04386447), carried out by CNRS, France, Azienda Ospedaliero Universitaria di Parma and Ospedale San Francesco Nuoro, Italy. Aiming to assess the effects of oxytocin in addition to standard therapy in reducing the number of patients who enter a critical stage, it proposed that “Intervention in addition to standard treatment, patients in the experimental arm will receive intravenous oxytocin with dilution of 25 IU or 40 IU oxytocin in 500cc physiological solution NaCl 9%. Oxytocin will be administered with continuous pump infusion with 62.5 ml/h. The clinician will have the option to decrease infusion speed based on significant variations in arterial pressure, otherwise, the total 25 IU or 40 IU amount will be infused in 8 hours. Treatment duration will be 10 days.” Currently, it is in withdrawn status since this study was not approved by the Italian Medicines Agency. Thus, presently there is no ongoing registered clinical trial of using oxytocin to treat SARS-CoV2 infections independently or along with other therapies.
However, clinical trials of oxytocin for COVID-19 treatment remain needed. Different approaches of oxytocin administrations and their advantage versus limitations have been considered (98, 100, 157, 158). These approaches mainly include intravenous and intranasal application of oxytocin, and optimal therapeutic usages have been tested experimentally. In an established murine model of chronic psychosocial stress, exposure to chronic subordinate colony housing results in long-lasting increase in anxiety, adrenal hypertrophy and thymus atrophy. Acute oxytocin administration in rodents can dose-dependently improve the behavior and physiology of male mice. However, chronic intracerebroventricular oxytocin infusion at high dose (10 ng/h) induces an anxiogenic phenotype with a concomitant reduction of OTR binding within the septum, the basolateral and medial amygdala, as well as the median raphe nucleus (159). Thus, chronically continuous application of oxytocin is not a preferred approach for treating COVID-19 patients. Alternatively, a single intranasal application of 26 IU of oxytocin causes a substantial rise of oxytocin plasma levels 30 min after intranasal administration in human participants; however, group mean oxytocin plasma level returns to baseline at 90 min post administration (160). Thus, it seems a preferred approach of intranasal oxytocin administration, particularly using dry inhaler but not in liquid spray (158), in an interval of 90 min for COVID-19 patients. This can be used in combination of molnupiravir and paxlovid programs. What we need urgently now is to initiate clinical trials and fully investigate the optimal doses and durations of treatment measures as Carter proposed (157).
Clinical drug use involves a large number of clinical trials, time, dose and dosage form, and users also need a large number of clinical observations. Certainly, new trials can be performed after carefully designing the research protocols; however, the development of COVID-19 cannot wait for a trial result. Thus, seeking the approaches of utilizing oxytocin to prevent individuals from COVID-19 and its serious pathological sequelae is necessary and urgent. Oxytocin can be mobilized endogenously through simple physical stimuli via the following approaches.
Oxytocin secretion can be evoked not only by the classic neuroendocrine reflex including the milk letdown reflex (161) and Ferguson reflex (162), but also their associated physical stimulation, particularly vagal stimulation.
Vagal activation is associated with distension of the gastrointestinal tracts, reduction of heart rate and blood pressure. Physiologically, distension of the gastrointestinal muscle layers by food sends afferent signals to the brainstem and reflex relaxation of the gastrointestinal tracts (163). Meanwhile, the afferents of vagal signals also reach the hypothalamus to increase oxytocin release into the blood (164) by the mediation of the vagus (165). Alternatively, probiotics in the gastrointestinal tracts can significantly increase blood oxytocin levels (166) by the mediation of the vagus (167). Thus, activation of vagal inputs can promote oxytocin secretion through feeding appropriately.
Oxytocin-mediated milk-letdown reflex can be conditioned around breastfeeding (168). In non-lactating females and men, activation of milk-letdown reflex-associated neural signals can elicit oxytocin release (169). The letdown reflex is critically dependent on the hypothalamic oxytocin neurons (161) that have extensive neural connection with specific sensory organs in the head. Thus, hypothalamic oxytocin neurons can receive signals from the olfactory bulbs, auditory cortex, retina, the medial frontal cortex and the mammillary body (170). For instance, aromatherapy with Rosa damascena can reduce the severity of pain and anxiety in the first stage of labor by increasing oxytocin release (171, 172). It has also been reported that baby’ cry can trigger the letdown reflex (173). In addition, listening to music (174), and shining light (175, 176), and even brief meditation (177) can increase oxytocin release independent of breastfeeding. Thus, activation of specific sensory organs and even mediation can increase oxytocin release.
It is well established that oxytocin release is increased significantly during sexual arousal, intercourse and orgasms for both men and women (178, 179). Thus, in addition to the Ferguson reflex that occurs when cervix is expanded during labor, reflex-release of oxytocin can also be evoked by genital organ stimulation (180, 181). The mechanical stimulation can activate oxytocin neurons in the hypothalamus, and thus increase oxytocin release from the posterior pituitary (182). Interestingly, vaginocervical stimulation can activate oxytocin neurons and their secretory activity by activating the vagus nerves (183, 184). Thus, sexual stimulation in adults is a way to increase endogenous oxytocin secretion.
In addition to vagal stimulation, modulating activity of special sensory organ, conditional reflex and sexual stimulation, oxytocin secretion can be facilitated by physical stimuli featuring low force, low frequency and slow conduction. In the assistant techniques for parturition, the combination of breathing exercises, foot reflexology and back massage can facilitate labor of women by reducing stress, pain, anxiety and promoting cervix ripening via increasing oxytocin release (185, 186). Stimulation with acupuncture at SanyinJiao can increase the release of oxytocin and thus accelerate cervix ripening (187). Similarly, a combination of acupressure, reflexology and aromatherapy can significantly shortened labor duration in nulliparous women (188). Beyond the special situation during parturition, frequent physical touch is associated with higher oxytocin levels for general population (189). In addition, massage (190) and running can also increase the secretion of oxytocin.
The pathogenesis of COVID-19 involves disorders in both innate immunity and adaptive immunity while SARS-CoV-2 entry is a prerequisite for disease onset. Thus, in the prevention of COVID-19, we should not only shield ourselves from the virus, cut potential transmission chains and receive timely vaccination, but also actively mobilize our own immunity to reduce the entry of SARS-CoV-2 into our body and its dissemination. Since oxytocin release can be evoked through numerous neurohumoral reflexes, mobilization of endogenous oxytocin secretion through physiological stimuli (Figure 2) is nevertheless an optimal approach to prevent COVID-19, independent of vaccination. More importantly, we need to set hands on clinical trials of oxytocin for controlling COVID-19. Such trials should fully balance the advantages and potential side effects of oxytocin application (123), consider the time- and dose-dependency of oxytocin/OTR signaling processes (157, 191), optimize the dosage form and administration approach, and combine oxytocin with application of inhibitors of SARS-CoV-2 replication and specific passive immunization (23). Thus, further exploring the preventive potential of mobilizing endogenous oxytocin secretion, executing intense clinical trials and applying exogenous oxytocin or its agonists are warranted in controlling COVID-19 and other viral diseases.
Figure 2 Approach of mobilizing endogenous oxytocin. Oxytocin secretion from the posterior pituitary gland (P.Pit) can be evoked by a variety of stimuli that activate oxytocin neurons in the hypothalamus and involve several pathways such as the sex-/labor-associated Ferguson reflex, the breastfeeding-associated let-down reflex (also called the milk-ejection reflex), the vago-pituitary reflex, as well as a conditioned reflex (acquired as the result of experience) and many other stimuli. The mechanisms underlying pathways from the stimulus to oxytocin secretion largely remain to be identified (the figure is an original drawing).
SW and FZ wrote the first draft and contributed to the key concepts; HZ, HY, YL and PW discussed the contents and drew the figures, VP and Y-FW conceived the study and edited the text. All authors contributed to the article and approved the submitted version.
This work was supported by the National Key Research & Development Program of China (2017YFB0403805, FZ), the National Natural Science Foundation of China (grant No. 31471113, Y-FW), and Fund of the Ministry of Science and Technology of China (grant No. G2021011014L). VP is supported by a grant from the National Institute of General Medical Sciences of the National Institutes of Health (R01GM123971 to VP).
The authors declare that the research was conducted in the absence of any commercial or financial relationships that could be construed as a potential conflict of interest.
All claims expressed in this article are solely those of the authors and do not necessarily represent those of their affiliated organizations, or those of the publisher, the editors and the reviewers. Any product that may be evaluated in this article, or claim that may be made by its manufacturer, is not guaranteed or endorsed by the publisher.
1. Konda M, Dodda B, Konala VM, Naramala S, Adapa S. Potential Zoonotic Origins of SARS-CoV-2 and Insights for Preventing Future Pandemics Through One Health Approach. Cureus (2020) 12:e8932. doi: 10.7759/cureus.8932
2. Zhao Y, Wang J, Kuang D, Xu J, Yang M, Ma C, et al. Susceptibility of Tree Shrew to SARS-CoV-2 Infection. Sci Rep (2020) 10:16007. doi: 10.1038/s41598-020-72563-w
3. Conceicao C, Thakur N, Human S, Kelly JT, Logan L, Bialy D, et al. The SARS-CoV-2 Spike Protein has a Broad Tropism for Mammalian ACE2 Proteins. PloS Biol (2020) 18:e3001016. doi: 10.1371/journal.pbio.3001016
4. Griffin JB, Haddix M, Danza P, Fisher R, Koo TH, Traub E, et al. SARS-CoV-2 Infections and Hospitalizations Among Persons Aged >/=16 Years, by Vaccination Status - Los Angeles County, California, May 1-July 25, 2021. MMWR Morb Mortal Wkly Rep (2021) 70:1170–6. doi: 10.15585/mmwr.mm7034e5
5. Head JR, Andrejko KL, Remais JV. Model-Based Assessment of SARS-CoV-2 Delta Variant Transmission Dynamics Within Partially Vaccinated K-12 School Populations. Lancet Regional Health (2022) 100133. doi: 10.1016/j.lana.2021.100133
6. Lam-Hine T, Mccurdy SA, Santora L, Duncan L, Corbett-Detig R, Kapusinszky B, et al. Outbreak Associated With SARS-CoV-2 B.1.617.2 (Delta) Variant in an Elementary School - Marin County, California, May-June 2021. MMWR Morb Mortal Wkly Rep (2021) 70:1214–9. doi: 10.15585/mmwr.mm7035e2
7. Barrero-Castillero A, Beam KS, Bernardini LB, Ramos EGC, Davenport PE, Duncan AR, et al. COVID-19: Neonatal-Perinatal Perspectives. J Perinatol (2021) 41:940–51. doi: 10.1038/s41372-020-00874-x
8. Libby P, Luscher T. COVID-19 Is, in the End, an Endothelial Disease. Eur Heart J (2020) 41:3038–44. doi: 10.1093/eurheartj/ehaa623
9. Alharthy A, Faqihi F, Memish ZA, Karakitsos D. Fragile Endothelium and Brain Dysregulated Neurochemical Activity in COVID-19. ACS Chem Neurosci (2020) 11:2159–62. doi: 10.1021/acschemneuro.0c00437
10. Wirnsberger G, Monteil V, Eaton B, Postnikova E, Murphy M, Braunsfeld B, et al. Clinical Grade ACE2 as a Universal Agent to Block SARS-CoV-2 Variants. bioRxiv (2021) 10:2021.09.10.459744. doi: 10.1101/2021.09.10.459744
11. Rashid HU, Ahmad N, Abdalla M, Khan K, Martines M, Shabana S. Molecular Docking and Dynamic Simulations of Cefixime, Etoposide and Nebrodenside A Against the Pathogenic Proteins of SARS-CoV-2. J Mol Struct (2022) 1247:131296. doi: 10.1016/j.molstruc.2021.131296
12. Imran M, Kumar Arora M, Asdaq SMB, Khan SA, Alaqel SI, Alshammari MK, et al. Discovery, Development, and Patent Trends on Molnupiravir: A Prospective Oral Treatment for COVID-19. Molecules (2021) 26:5795. doi: 10.3390/molecules26195795
13. Mahase E. Covid-19: Pfizer’s Paxlovid is 89% Effective in Patients at Risk of Serious Illness, Company Reports. BMJ (2021) 375:n2713. doi: 10.1136/bmj.n2713
14. Couzin-Frankel J. Antiviral Pills Could Change Pandemic’s Course. Science (2021) 374:799–800. doi: 10.1126/science.acx9605
15. Pedrosa MA, Valenzuela R, Garrido-Gil P, Labandeira CM, Navarro G, Franco R, et al. Experimental Data Using Candesartan and Captopril Indicate No Double-Edged Sword Effect in COVID-19. Clin Sci (Lond) (2021) 135:465–81. doi: 10.1042/CS20201511
16. Rebmann T, Loux TM, Arnold LD, Charney R, Horton D, Gomel A. SARS-CoV-2 Transmission to Masked and Unmasked Close Contacts of University Students With COVID-19 - St. Louis, Missouri, January-May 2021. MMWR Morb Mortal Wkly Rep (2021) 70:1245–8. doi: 10.15585/mmwr.mm7036a3
17. Scobie HM, Johnson AG, Suthar AB, Severson R, Alden NB, Balter S, et al. Monitoring Incidence of COVID-19 Cases, Hospitalizations, and Deaths, by Vaccination Status - 13 U.S. Jurisdictions, April 4-July 17, 2021. MMWR Morb Mortal Wkly Rep (2021) 70:1284–90. doi: 10.15585/mmwr.mm7037e1
18. Rosenberg ES, Holtgrave DR, Dorabawila V, Conroy M, Greene D, Lutterloh E, et al. New COVID-19 Cases and Hospitalizations Among Adults, by Vaccination Status - New York, May 3-July 25, 2021. MMWR Morb Mortal Wkly Rep (2021) 70:1150–5. doi: 10.15585/mmwr.mm7034e1
19. Brown CM, Vostok J, Johnson H, Burns M, Gharpure R, Sami S, et al. Outbreak of SARS-CoV-2 Infections, Including COVID-19 Vaccine Breakthrough Infections, Associated With Large Public Gatherings - Barnstable County, Massachusetts, July 2021. MMWR Morb Mortal Wkly Rep (2021) 70:1059–62. doi: 10.15585/mmwr.mm7031e2
20. Zhang J, Xiao T, Cai Y, Lavine CL, Peng H, Zhu H, et al. Membrane Fusion and Immune Evasion by the Spike Protein of SARS-CoV-2 Delta Variant. bioRxiv (2021) 374:1353–60. doi: 10.1101/2021.08.17.456689
21. Lindholm PF, Ramsey G, Kwaan HC. Passive Immunity for Coronavirus Disease 2019: A Commentary on Therapeutic Aspects Including Convalescent Plasma. Semin Thromb Hemost (2020) 46:796–803. doi: 10.1055/s-0040-1712157
22. Liu C, Ginn HM, Dejnirattisai W, Supasa P, Wang B, Tuekprakhon A, et al. Reduced Neutralization of SARS-CoV-2 B.1.617 by Vaccine and Convalescent Serum. Cell (2021) 184:4220–36.e4213. doi: 10.1016/j.cell.2021.06.020
23. Koenig PA, Das H, Liu H, Kummerer BM, Gohr FN, Jenster LM, et al. Structure-Guided Multivalent Nanobodies Block SARS-CoV-2 Infection and Suppress Mutational Escape. Science (2021) 371:eabe6230. doi: 10.1126/science.abe6230
24. Petersen E, Ntoumi F, Hui DS, Abubakar A, Kramer LD, Obiero C, et al. Emergence of New SARS-CoV-2 Variant of Concern Omicron (B.1.1.529) - Highlights Africa’s Research Capabilities, But Exposes Major Knowledge Gaps, Inequities of Vaccine Distribution, Inadequacies in Global COVID-19 Response and Control Efforts. Int J Infect Dis (2021) 114:268–72. doi: 10.1016/j.ijid.2021.11.040
25. Torjesen I. Covid-19: Omicron may be More Transmissible Than Other Variants and Partly Resistant to Existing Vaccines, Scientists Fear. BMJ (2021) 375:n2943. doi: 10.1136/bmj.n2943
26. Case JB, Chen RE, Cao L, Ying B, Winkler ES, Johnson M, et al. Ultrapotent Miniproteins Targeting the SARS-CoV-2 Receptor-Binding Domain Protect Against Infection and Disease. Cell Host Microbe (2021) 29:1151–61.e5. doi: 10.1016/j.chom.2021.06.008
27. Weisblum Y, Schmidt F, Zhang F, DaSilva J, Poston D, Lorenzi JC, et al. Escape From Neutralizing Antibodies by SARS-CoV-2 Spike Protein Variants. eLife (2020) 9:e61312. doi: 10.7554/eLife.61312
28. Villoutreix BO, Calvez V, Marcelin AG, Khatib AM. In Silico Investigation of the New UK (B.1.1.7) and South African (501y.V2) SARS-CoV-2 Variants With a Focus at the ACE2-Spike RBD Interface. Int J Mol Sci (2021) 22:1695. doi: 10.3390/ijms22041695
29. Penetra SLS, da Silva MFB, Resende P, Pina-Costa A, Santos HFP, Guaraldo L, et al. Post-Acute COVID-19 Syndrome After Reinfection and Vaccine Breakthrough by the SARS-CoV-2 Gamma Variant in Brazil. Intern J Infect Dis (2021) 114:58–61. doi: 10.1016/j.ijid.2021.10.048
30. Moghaddar M, Radman R, Macreadie I. Severity, Pathogenicity and Transmissibility of Delta and Lambda Variants of SARS-CoV-2, Toxicity of Spike Protein and Possibilities for Future Prevention of COVID-19. Microorganisms (2021) 9:2167. doi: 10.3390/microorganisms9102167
31. Kannan SR, Spratt AN, Sharma K, Chand HS, Byrareddy SN, Singh K. Omicron SARS-CoV-2 Variant: Unique Features and Their Impact on Pre-Existing Antibodies. J Autoimmun (2021) 126:102779. doi: 10.1016/j.jaut.2021.102779
32. Metzger C, Lienhard R, Seth-Smith HMB, Roloff T, Wegner F, Sieber J, et al. PCR Performance in the SARS-CoV-2 Omicron Variant of Concern? Swiss Med Weekly (2021) 151:w30120. doi: 10.4414/smw.2021.w30120
33. Breidenbach JD, Dube P, Ghosh S, Abdullah BN, Modyanov NN, Malhotra D, et al. Impact of Comorbidities on SARS-CoV-2 Viral Entry-Related Genes. J Pers Med (2020) 10:146. doi: 10.3390/jpm10040146
34. Bestle D, Heindl MR, Limburg H, Van Lam Van T, Pilgram O, Moulton H, et al. TMPRSS2 and Furin are Both Essential for Proteolytic Activation of SARS-CoV-2 in Human Airway Cells. Life Sci Alliance (2020) 3:e202000786. doi: 10.1101/2020.04.15.042085
35. Johnson BA, Xie X, Bailey AL, Kalveram B, Lokugamage KG, Muruato A, et al. Loss of Furin Cleavage Site Attenuates SARS-CoV-2 Pathogenesis. Nature (2021) 591:293–9. doi: 10.1038/s41586-021-03237-4
36. Couselo-Seijas M, Almenglo C, Agra.-Bermejo R,M, Luis Fernandez A, Alvarez E, González.-Juanatey J,R, et al. Higher ACE2 Expression Levels in Epicardial Cells Than Subcutaneous Stromal Cells From Patients With Cardiovascular Disease: Diabetes and Obesity as Possible Enhancer. Eur J Clin Invest (2021) 51:e13463. doi: 10.1111/eci.13463
37. Yeung ML, Teng JLL, Jia L, Zhang C, Huang C, Cai JP, et al. Soluble ACE2-Mediated Cell Entry of SARS-CoV-2 via Interaction With Proteins Related to the Renin-Angiotensin System. Cell (2021) 184:2212–28.e2212. doi: 10.1016/j.cell.2021.02.053
38. Wu CT, Lidsky PV, Xiao Y, Lee IT, Cheng R, Nakayama T, et al. SARS-CoV-2 infects human pancreatic beta cells and elicits beta cell impairment. Cell Metab (2021) 33:1565–76.e5. doi: 10.1016/j.cmet.2021.05.013
39. Annweiler C, Cao Z, Wu Y, Faucon E, Mouhat S, Kovacic H, et al. Counter-Regulatory ‘Renin-Angiotensin’ System-Based Candidate Drugs to Treat COVID-19 Diseases in SARS-CoV-2-Infected Patients. Infect Disord Drug Targets (2020) 20:407–8. doi: 10.2174/1871526520666200518073329
40. Tavassoly O, Safavi F, Tavassoly I. Heparin-Binding Peptides as Novel Therapies to Stop SARS-CoV-2 Cellular Entry and Infection. Mol Pharmacol (2020) 98:612–9. doi: 10.1124/molpharm.120.000098
41. Zhang Q, Chen CZ, Swaroop M, Xu M, Wang L, Lee J, et al. Targeting Heparan Sulfate Proteoglycan-Assisted Endocytosis as a COVID-19 Therapeutic Option. bioRxiv (2020) 2020.07.14.202549. doi: 10.1101/2020.07.14.202549.
42. Bayati A, Kumar R, Francis V, Mcpherson PS. SARS-CoV-2 Infects Cells Following Viral Entry via Clathrin-Mediated Endocytosis. J Biol Chem (2021) 100306:e202000786. doi: 10.1016/j.jbc.2021.100306.
43. Sureda A, Alizadeh J, Nabavi SF, Berindan-Neagoe I, Cismaru CA, Jeandet P, et al. Endoplasmic Reticulum as a Potential Therapeutic Target for Covid-19 Infection Management? Eur J Pharmacol (2020) 882:173288. doi: 10.1016/j.ejphar.2020.173288
44. Khanmohammadi S, Rezaei N. Role of Toll-Like Receptors in the Pathogenesis of COVID-19. J Med Virol (2021) 93:2735–9. doi: 10.1002/jmv.26826
45. Dyavar SR, Singh R, Emani R, Pawar GP, Chaudhari VD, Podany AT, et al. Role of Toll-Like Receptor 7/8 Pathways in Regulation of Interferon Response and Inflammatory Mediators During SARS-CoV2 Infection and Potential Therapeutic Options. BioMed Pharmacother (2021) 141:111794. doi: 10.1016/j.biopha.2021.111794
46. Belizario JE. Immunity, Virus Evolution, and Effectiveness of SARS-CoV-2 Vaccines. Braz J Med Biol Res (2021) 54:e10725. doi: 10.1590/1414-431x202010725
47. Osman IO, Melenotte C, Brouqui P, Million M, Lagier JC, Parola P, et al. Expression of ACE2, Soluble ACE2, Angiotensin I, Angiotensin II and Angiotensin-(1-7) Is Modulated in COVID-19 Patients. Front Immunol (2021) 12:625732. doi: 10.3389/fimmu.2021.625732
48. Lee KY, Rhim JW, Kang JH. Immunopathogenesis of COVID-19 and Early Immunomodulators. Clin Exp Pediatr (2020) 63:239–50. doi: 10.3345/cep.2020.00759
49. Grivas P, Khaki AR, Wise-Draper TM, French B, Hennessy C, Hsu CY, et al. Association of Clinical Factors and Recent Anticancer Therapy With COVID-19 Severity Among Patients With Cancer: A Report From the COVID-19 and Cancer Consortium. Ann Oncol (2021) 32:787–800. doi: 10.1016/j.annonc.2021.02.024
50. Yoo EH, Chang SH, Song DY, Lee CH, Cheong GY, Park S, et al. Comprehensive Laboratory Data Analysis to Predict the Clinical Severity of Coronavirus Disease 2019 in 1,952 Patients in Daegu, Korea. Ann Lab Med (2022) 42:24–35. doi: 10.3343/alm.2022.42.1.24
51. Paludan SR, Pradeu T, Masters SL, Mogensen TH. Constitutive Immune Mechanisms: Mediators of Host Defence and Immune Regulation. Nat Rev Immunol (2021) 21:137–50. doi: 10.1038/s41577-020-0391-5
52. Kazakou P, Paschou SA, Psaltopoulou T, Gavriatopoulou M, Korompoki E, Stefanaki K, et al. Early and Late Endocrine Complications of COVID-19. Endocr Connect (2021) 10:R229–39. doi: 10.1530/EC-21-0184
53. Guedj E, Million M, Dudouet P, Tissot-Dupont H, Bregeon F, Cammilleri S, et al. (18)F-FDG Brain PET Hypometabolism in Post-SARS-CoV-2 Infection: Substrate for Persistent/Delayed Disorders? Eur J Nucl Med Mol Imaging (2021) 48:592–5. doi: 10.1007/s00259-020-04973-x
54. Langford D, Baron D, Joy J, Del Valle L, Shack J. Contributions of HIV Infection in the Hypothalamus and Substance Abuse/Use to HPT Dysregulation. Psychoneuroendocrinology (2011) 36:710–9. doi: 10.1016/j.psyneuen.2010.10.005
55. Gu J, Gong E, Zhang B, Zheng J, Gao Z, Zhong Y, et al. Multiple Organ Infection and the Pathogenesis of SARS. J Exp Med (2005) 202:415–24. doi: 10.1084/jem.20050828
56. Guedj E, Million M, Dudouet P, Tissot-Dupont H, Bregeon F, Cammilleri S, et al. (18)F-FDG Brain PET Hypometabolism in Post-SARS-CoV-2 Infection: Substrate for Persistent/Delayed Disorders? Eur J Nucl Med Mol Imaging (2021) 48:592–5. doi: 10.1007/s00259-020-04973-x
57. Fekete EM, Antoni MH, Lopez C, Mendez AJ, Szeto A, Fletcher MA, et al. Stress Buffering Effects of Oxytocin on HIV Status in Low-Income Ethnic Minority Women. Psychoneuroendocrinology (2011) 36:881–90. doi: 10.1016/j.psyneuen.2010.12.003
58. Nass SR, Lark ARS, Hahn YK, McLane VD, Ihrig TM, Contois L, et al. HIV-1 Tat and Morphine Decrease Murine Inter-Male Social Interactions and Associated Oxytocin Levels in the Prefrontal Cortex, Amygdala, and Hypothalamic Paraventricular Nucleus. Hormones Behav (2021) 133:105008. doi: 10.1016/j.yhbeh.2021.105008
59. Miller VM, Zhu Y, Bucher C, McGinnis W, Ryan LK, Siegel A, et al. Gestational Flu Exposure Induces Changes in Neurochemicals, Affiliative Hormones and Brainstem Inflammation, in Addition to Autism-Like Behaviors in Mice. Brain Behavior Immun (2013) 33:153–63. doi: 10.1016/j.bbi.2013.07.002
60. Johnson HM, Torres BA. Regulation of Lymphokine Production by Arginine Vasopressin and Oxytocin: Modulation of Lymphocyte Function by Neurohypophyseal Hormones. J Immunol (1985) 135:773s–5s.
61. Chen D, Hou Z, Jiang D, Zheng M, Li G, Zhang Y, et al. GILT Restricts the Cellular Entry Mediated by the Envelope Glycoproteins of SARS-CoV, Ebola Virus and Lassa Fever Virus. Emerging Microbes Infect (2019) 8:1511–23. doi: 10.1080/22221751.2019.1677446
62. Zhu Y, Huang P, Yang N, Liu R, Liu X, Dai H, et al. Establishment and Application of a High Throughput Screening System Targeting the Interaction Between HCV Internal Ribosome Entry Site and Human Eukaryotic Translation Initiation Factor 3. Front Microbiol (2017) 8:977. doi: 10.3389/fmicb.2017.00977
63. Genis S, Aris A, Kaur M, Cerri RLA. Effect of Metritis on Endometrium Tissue Transcriptome During Puerperium in Holstein Lactating Cows. Theriogenology (2018) 122:116–23. doi: 10.1016/j.theriogenology.2018.09.004
64. Markovits A, Premecz G, Bagi G, Foldes I. Oxytocin Stimulates Translocation of Protein Kinase C and Induces Antiviral State in Human Amnion Cells. Acta Microbiol Hungarica (1992) 39:41–5.
65. Chittepu V, Kalhotra P, Osorio-Gallardo T, Jimenez-Martinez C, Torre RRR, Gallardo-Velazquez T, et al. New Molecular Insights Into the Inhibition of Dipeptidyl Peptidase-4 by Natural Cyclic Peptide Oxytocin. Molecules (2019) 24:3887. doi: 10.3390/molecules24213887
66. Phyu Khin P, Cha SH, Jun HS, Lee JH. A Potential Therapeutic Combination for Treatment of COVID-19: Synergistic Effect of DPP4 and RAAS Suppression. Med Hypotheses (2020) 144:110186. doi: 10.1016/j.mehy.2020.110186
67. Imami AS, O’donovan SM, Creeden JF, Wu X, Eby H, Mccullumsmith CB, et al. Oxytocin’s Anti-Inflammatory and Proimmune Functions in COVID-19: A Transcriptomic Signature-Based Approach. Physiol Genomics (2020) 52:401–7. doi: 10.1152/physiolgenomics.00095.2020
68. Tsegay KB, Adeyemi CM, Gniffke EP, Sather DN, Walker JK, Smith SEP. A Repurposed Drug Screen Identifies Compounds That Inhibit the Binding of the COVID-19 Spike Protein to ACE2. Front Pharmacol (2021) 12:685308. doi: 10.3389/fphar.2021.685308
69. Long R, Wu D, Lin X, Lv D, Wang R, Jin L, et al. COVID-19 and Pregnancy: A Case Study. Global Challenges (2021):2000074. doi: 10.1002/gch2.202000074
70. Sever IH, Ozkul B, Erisik Tanriover D, Ozkul O, Elgormus CS, Gur SG, et al. Protective Effect of Oxytocin Through its Anti-Inflammatory and Antioxidant Role in a Model of Sepsis-Induced Acute Lung Injury: Demonstrated by CT and Histological Findings. Exp Lung Res (2021) 47:426–35. doi: 10.1080/01902148.2021.1992808
71. Welcome MO, Mastorakis NE. Neuropathophysiology of Coronavirus Disease 2019: Neuroinflammation and Blood Brain Barrier Disruption are Critical Pathophysiological Processes That Contribute to the Clinical Symptoms of SARS-CoV-2 Infection. Inflammopharmacology (2021) 29:939–63. doi: 10.1007/s10787-021-00806-x
72. Pal R. COVID-19, Hypothalamo-Pituitary-Adrenal Axis and Clinical Implications. Endocrine (2020) 68:251–2. doi: 10.1007/s12020-020-02325-1
73. Alzahrani AS, Mukhtar N, Aljomaiah A, Aljamei H, Bakhsh A, Alsudani N, et al. The Impact of COVID-19 Viral Infection on the Hypothalamic-Pituitary-Adrenal Axis. Endocr Pract (2021) 27:83–9. doi: 10.1016/j.eprac.2020.10.014
74. Xiang Z, Liu J, Shi D, Chen W, Li J, Yan R, et al. Glucocorticoids Improve Severe or Critical COVID-19 by Activating ACE2 and Reducing IL-6 Levels. Int J Biol Sci (2020) 16:2382–91. doi: 10.7150/ijbs.47652
75. Li H, Chen C, Hu F, Wang J, Zhao Q, Gale RP, et al. Impact of Corticosteroid Therapy on Outcomes of Persons With SARS-CoV-2, SARS-CoV, or MERS-CoV Infection: A Systematic Review and Meta-Analysis. Leukemia (2020) 34:1503–11. doi: 10.1038/s41375-020-0848-3
76. Hashim M, Athar S, Gaba WH. New Onset Adrenal Insufficiency in a Patient With COVID-19. BMJ Case Rep (2021) 14:e237690. doi: 10.1136/bcr-2020-237690
77. Hill AR, Spencer-Segal JL. Glucocorticoids and the Brain After Critical Illness. Endocrinology (2021) 162:bqaa242. doi: 10.1210/endocr/bqaa242
78. Chen M, Zhou W, Xu W. Thyroid Function Analysis in 50 Patients With COVID-19: A Retrospective Study. Thyroid (2020) 31:8–11. doi: 10.1089/thy.2020.0363
79. Ahn J, Lee MK, Lee JH, Sohn SY. Thyroid Hormone Profile and Its Prognostic Impact on the Coronavirus Disease 2019 in Korean Patients. Endocrinol Metab (Seoul) (2021) 36:769–77. doi: 10.3803/EnM.2021.1109
80. Wang W, Su X, Ding Y, Fan W, Zhou W, Su J, et al. Thyroid Function Abnormalities in COVID-19 Patients. Front Endocrinol (Lausanne) (2020) 11:623792. doi: 10.3389/fendo.2020.623792
81. Malik J, Zaidi SMJ, Waqar AU, Khawaja H, Malik A, Ishaq U, et al. Association of Hypothyroidism With Acute COVID-19: A Systematic Review. Expert Rev Endocrinol Metab (2021) 16:251–7. doi: 10.1080/17446651.2021.1968830
82. Montesinos MDM, Pellizas CG. Thyroid Hormone Action on Innate Immunity. Front Endocrinol (Lausanne) (2019) 10:350. doi: 10.3389/fendo.2019.00350
83. Pivonello R, Auriemma RS, Pivonello C, Isidori AM, Corona G, Colao A, et al. Sex Disparities in COVID-19 Severity and Outcome: Are Men Weaker or Women Stronger? Neuroendocrinology (2021) 111:1066–85. doi: 10.1159/000513346
84. Deng Q, Rasool RU, Russell RM, Natesan R, Asangani IA. Targeting Androgen Regulation of TMPRSS2 and ACE2 as a Therapeutic Strategy to Combat COVID-19. iScience (2021) 24:102254. doi: 10.1016/j.isci.2021.102254
85. Clinckemalie L, Spans L, Dubois V, Laurent M, Helsen C, Joniau S, et al. Androgen Regulation of the TMPRSS2 Gene and the Effect of a SNP in an Androgen Response Element. Mol Endocrinol (2013) 27:2028–40. doi: 10.1210/me.2013-1098
86. Sengupta P, Dutta S. COVID-19 and Hypogonadism: Secondary Immune Responses Rule-Over Endocrine Mechanisms. Hum Fertil (Camb) (2021) 1–6. doi: 10.1080/14647273.2020.1867902
87. Gedeborg R, Styrke J, Loeb S, Garmo H, Stattin P. Androgen Deprivation Therapy and Excess Mortality in Men With Prostate Cancer During the Initial Phase of the COVID-19 Pandemic. PloS One (2021) 16:e0255966. doi: 10.1371/journal.pone.0255966
88. Kalra S, Bhattacharya S, Kalhan A. Testosterone in COVID-19 - Foe, Friend or Fatal Victim? Eur Endocrinol (2020) 16:88–91. doi: 10.17925/EE.2020.16.2.88
89. Al-Lami RA, Urban RJ, Volpi E, Algburi AMA, Baillargeon J. Sex Hormones and Novel Corona Virus Infectious Disease (COVID-19). Mayo Clin Proc (2020) 95:1710–4. doi: 10.1016/j.mayocp.2020.05.013
90. Challa AA, Calaway AC, Cullen J, Garcia J, Desai N, Weintraub NL, et al. Cardiovascular Toxicities of Androgen Deprivation Therapy. Curr Treat Options Oncol (2021) 22:47. doi: 10.1007/s11864-021-00846-z
91. Giagulli VA, Guastamacchia E, Magrone T, Jirillo E, Lisco G, De Pergola G, et al. Worse Progression of COVID-19 in Men: Is Testosterone a Key Factor? Andrology (2021) 9:53–64. doi: 10.1111/andr.12836
92. Stelzig KE, Canepa-Escaro F, Schiliro M, Berdnikovs S, Prakash YS, Chiarella SE. Estrogen Regulates the Expression of SARS-CoV-2 Receptor ACE2 in Differentiated Airway Epithelial Cells. Am J Physiol Lung Cell Mol Physiol (2020) 318:L1280–1. doi: 10.1152/ajplung.00153.2020
93. Breithaupt-Faloppa AC, Correia CJ, Prado CM, Stilhano RS, Ureshino RP, Moreira LFP. 17beta-Estradiol, a Potential Ally to Alleviate SARS-CoV-2 Infection. Clinics (Sao Paulo) (2020) 75:e1980. doi: 10.6061/clinics/2020/e1980
94. Liu N, Yang H, Han L, Ma M. Oxytocin in Women’s Health and Disease. Front Endocrinol (2022) 13:786271. doi: 10.3389/fendo.2022.786271
95. Reyes-Garcia J, Montano LM, Carbajal-Garcia A, Wang YX. Sex Hormones and Lung Inflammation. Adv Exp Med Biol (2021) 1304:259–321. doi: 10.1007/978-3-030-68748-9_15
96. Wang P, Yang HP, Tian S, Wang L, Wang SC, Zhang F, et al. Oxytocin-Secreting System: A Major Part of the Neuroendocrine Center Regulating Immunologic Activity. J Neuroimmunol (2015) 289:152–61. doi: 10.1016/j.jneuroim.2015.11.001
97. Li T, Wang P, Wang SC, Wang YF. Approaches Mediating Oxytocin Regulation of the Immune System. Front Immunol (2016) 7:693. doi: 10.3389/fimmu.2016.00693
98. Buemann B, Marazziti D, Uvnas-Moberg K. Can Intravenous Oxytocin Infusion Counteract Hyperinflammation in COVID-19 Infected Patients? World J Biol Psychiatry (2021) 22:387–98. doi: 10.1080/15622975.2020.1814408
99. Thakur P, Shrivastava R, Shrivastava VK. Oxytocin as a Potential Adjuvant Against COVID-19 Infection. Endocr Metab Immune Disord Drug Targets (2021) 21:1155–62. doi: 10.2174/1871530320666200910114259
100. Wang SC, Wang YF. Cardiovascular Protective Properties of Oxytocin Against COVID-19. Life Sci (2021) 270:119130. doi: 10.1016/j.lfs.2021.119130
101. Yang HP, Wang L, Han L, Wang SC. Nonsocial Functions of Hypothalamic Oxytocin. ISRN Neurosci (2013) 2013:179272. doi: 10.1155/2013/179272
102. Wang Y-F. Center Role of the Oxytocin-Secreting System in Neuroendocrine-Immune Network Revisited. J Clin Exp Neuroimmunol (2016) 1:102. doi: 10.4172/jceni.1000102.
103. Gregoriano C, Molitor A, Haag E, Kutz A, Koch D, Haubitz S, et al. Activation of Vasopressin System During COVID-19 is Associated With Adverse Clinical Outcomes: An Observational Study. J Endocr Soc (2021) 5:bvab045. doi: 10.1210/jendso/bvab045
104. Currigan DA, Hughes RJ, Wright CE, Angus JA, Soeding PF. Vasoconstrictor Responses to Vasopressor Agents in Human Pulmonary and Radial Arteries: An In Vitro Study. Anesthesiology (2014) 121:930–6. doi: 10.1097/ALN.0000000000000430
105. Yang WS, Moon SY, Han NJ, Lee MJ, Park SK. Arginine Vasopressin Attenuates the Effects of TNF-Alpha in Aortic Endothelial Cells by Inducing Ectodomain Shedding of TNF Receptor 1. Biochem Biophys Res Commun (2019) 511:780–6. doi: 10.1016/j.bbrc.2019.02.125
106. Leisman DE, Mehta A, Li Y, Kays KR, Li JZ, Filbin MR, et al. Vasopressin Infusion in COVID-19 Critical Illness is Not Associated With Impaired Viral Clearance: A Pilot Study. Br J Anaesth (2021) 127:e146–8. doi: 10.1016/j.bja.2021.07.005
107. Liu Y, Yang Y, Zhang C, Huang F, Wang F, Yuan J, et al. Clinical and Biochemical Indexes From 2019-Ncov Infected Patients Linked to Viral Loads and Lung Injury. Sci China Life Sci (2020) 63:364–74. doi: 10.1007/s11427-020-1643-8
108. Khan A, Benthin C, Zeno B, Albertson TE, Boyd J, Christie JD, et al. A Pilot Clinical Trial of Recombinant Human Angiotensin-Converting Enzyme 2 in Acute Respiratory Distress Syndrome. Crit Care (2017) 21:234. doi: 10.1186/s13054-017-1823-x
109. Khezri MR, Yousefi K, Ghasemnejad-Berenji M. Angiotensin II: A Possible Target for Therapeutic Intervention in COVID-19. BioMed Pharmacother (2021) 139:111564. doi: 10.1016/j.biopha.2021.111564
110. Zhang R, Wang X, Ni L, Di X, Ma B, Niu S, et al. COVID-19: Melatonin as a Potential Adjuvant Treatment. Life Sci (2020) 250:117583. doi: 10.1016/j.lfs.2020.117583
111. De Melo VU, Saldanha RR, Dos Santos CR, De Campos Cruz J, Lira VA, Santana-Filho VJ, et al. Ovarian Hormone Deprivation Reduces Oxytocin Expression in Paraventricular Nucleus Preautonomic Neurons and Correlates With Baroreflex Impairment in Rats. Front Physiol (2016) 7:461. doi: 10.3389/fphys.2016.00461
112. Light KC, Grewen KM, Amico JA, Brownley KA, West SG, Hinderliter AL, et al. Oxytocinergic Activity is Linked to Lower Blood Pressure and Vascular Resistance During Stress in Postmenopausal Women on Estrogen Replacement. Horm Behav (2005) 47:540–8. doi: 10.1016/j.yhbeh.2004.12.010
113. Madjid M, Safavi-Naeini P, Solomon SD, Vardeny O. Potential Effects of Coronaviruses on the Cardiovascular System: A Review. JAMA Cardiol (2020) 5:831–40. doi: 10.1001/jamacardio.2020.1286
114. Eisenberg Y, Dugas LR, Akbar A, Reddivari B, Layden BT, Barengolts E. Oxytocin is Lower in African American Men With Diabetes and Associates With Psycho-Social and Metabolic Health Factors. PloS One (2018) 13:e0190301. doi: 10.1371/journal.pone.0190301
115. Pascual-Goni E, Fortea J, Martinez-Domeno A, Rabella N, Tecame M, Gomez-Oliva C, et al. COVID-19-Associated Ophthalmoparesis and Hypothalamic Involvement. Neurol Neuroimmunol Neuroinflamm (2020) 7:e823. doi: 10.1212/NXI.0000000000000823
116. Serrano GE, Walker JE, Arce R, Glass MJ, Vargas D, Sue LI, et al. Mapping of SARS-CoV-2 Brain Invasion and Histopathology in COVID-19 Disease. medRxiv (2021). doi: 10.1101/2021.02.15.21251511
117. Mussa BM, Srivastava A, Verberne AJM. COVID-19 and Neurological Impairment: Hypothalamic Circuits and Beyond. Viruses (2021) 13:498. doi: 10.3390/v13030498
118. Currie MG, Zimmer DP, Halushka PV. An Impaired Natriuretic Peptide Hormone System May Play a Role in COVID-19 Severity in Vulnerable Populations. FASEB Bioadv (2020) 10.1096/fba.2020-00042. doi: 10.31219/osf.io/g4apd
119. Houshmand F, Faghihi M, Zahediasl S. Role of Atrial Natriuretic Peptide in Oxytocin Induced Cardioprotection. Heart Lung Circ (2015) 24:86–93. doi: 10.1016/j.hlc.2014.05.023
120. Wu Z, Hu R, Zhang C, Ren W, Yu A, Zhou X. Elevation of Plasma Angiotensin II Level is a Potential Pathogenesis for the Critically Ill COVID-19 Patients. Crit Care (2020) 24:290. doi: 10.1186/s13054-020-03015-0
121. Srinivasa S, Aulinas A, O’malley T, Maehler P, Adler GK, Grinspoon SK, et al. Oxytocin Response to Controlled Dietary Sodium and Angiotensin II Among Healthy Individuals. Am J Physiol Endocrinol Metab (2018) 315:E671–5. doi: 10.1152/ajpendo.00190.2018
122. Gottschalk MG, Domschke K. Oxytocin and Anxiety Disorders. Curr Top Behav Neurosci (2018) 35:467–98. doi: 10.1007/7854_2017_25
123. Wang P, Wang SC, Yang H, Lv C, Jia S, Liu X, et al. Therapeutic Potential of Oxytocin in Atherosclerotic Cardiovascular Disease: Mechanisms and Signaling Pathways. Front Neurosci (2019) 13:454. doi: 10.3389/fnins.2019.00454
124. Diep PT, Talash K, Kasabri V. Hypothesis: Oxytocin is a Direct COVID-19 Antiviral. Med Hypotheses (2020) 145:110329. doi: 10.1016/j.mehy.2020.110329
125. Yang J, Zheng Y, Gou X, Pu K, Chen Z, Guo Q, et al. Prevalence of Comorbidities and its Effects in Patients Infected With SARS-CoV-2: A Systematic Review and Meta-Analysis. Int J Infect Dis (2020) 94:91–5. doi: 10.1016/j.ijid.2020.03.017
126. Zhang J, Zhou L, Yang Y, Peng W, Wang W, Chen X. Therapeutic and Triage Strategies for 2019 Novel Coronavirus Disease in Fever Clinics. Lancet Respir Med (2020) 8:e11–2. doi: 10.1016/S2213-2600(20)30071-0
127. Gustafson D, Raju S, Wu R, Ching C, Veitch S, Rathnakumar K, et al. Overcoming Barriers: The Endothelium As a Linchpin of Coronavirus Disease 2019 Pathogenesis? Arterioscler Thromb Vasc Biol (2020) 40:1818–29. doi: 10.1161/ATVBAHA.120.314558
128. Rajpal A, Rahimi L, Ismail-Beigi F. Factors Leading to High Morbidity and Mortality of COVID-19 in Patients With Type 2 Diabetes. J Diabetes (2020) 12:895–908. doi: 10.1111/1753-0407.13085
129. Wang SC, Meng D, Yang H, Wang X, Jia S, Wang P, et al. Pathological Basis of Cardiac Arrhythmias: Vicious Cycle of Immune-Metabolic Dysregulation. Cardiovasc Disord Med (2017) 3:1–7. doi: 10.15761/CDM.1000158
130. Petersson M, Uvnas-Moberg K. Postnatal Oxytocin Treatment of Spontaneously Hypertensive Male Rats Decreases Blood Pressure and Body Weight in Adulthood. Neurosci Lett (2008) 440:166–9. doi: 10.1016/j.neulet.2008.05.091
131. Jameson H, Bateman R, Byrne P, Dyavanapalli J, Wang X, Jain V, et al. Oxytocin Neuron Activation Prevents Hypertension That Occurs With Chronic Intermittent Hypoxia/Hypercapnia in Rats. Am J Physiol Heart Circ Physiol (2016) 310:H1549–57. doi: 10.1152/ajpheart.00808.2015.
132. Wang P, Wang SC, Li D, Li T, Yang HP, Wang L, et al. Role of Connexin 36 in Autoregulation of Oxytocin Neuronal Activity in Rat Supraoptic Nucleus. ASN Neuro (2019) 11:1759091419843762. doi: 10.1177/1759091419843762
133. Seah IYJ, Anderson DE, Kang AEZ, Wang L, Rao P, Young BE, et al. Assessing Viral Shedding and Infectivity of Tears in Coronavirus Disease 2019 (COVID-19) Patients. Ophthalmology (2020) 127:977–9. doi: 10.1016/j.ophtha.2020.03.026
134. Brandtzaeg P. Secretory Immunity With Special Reference to the Oral Cavity. J Oral Microbiol (2013) 5:10.3402/jom.v5i0.20401. doi: 10.3402/jom.v5i0.20401
135. Almario CV, Chey WD, Spiegel BMR. Increased Risk of COVID-19 Among Users of Proton Pump Inhibitors. Am J Gastroenterol (2020) 115:1707–15. doi: 10.14309/ajg.0000000000000798
136. Iwasaki Y, Maejima Y, Suyama S, Yoshida M, Arai T, Katsurada K, et al. Peripheral Oxytocin Activates Vagal Afferent Neurons to Suppress Feeding in Normal and Leptin-Resistant Mice: A Route for Ameliorating Hyperphagia and Obesity. Am J Physiol Regul Integr Comp Physiol (2015) 308:R360–9. doi: 10.1152/ajpregu.00344.2014
137. Sato M, Hayashi Y, Yoshida H, Yanagawa T, Yura Y, Nitta T. Search for Specific Markers of Neoplastic Epithelial Duct and Myoepithelial Cell Lines Established From Human Salivary Gland and Characterization of Their Growth In Vitro. Cancer (1984) 54:2959–67. doi: 10.1002/1097-0142(19841215)54:12<2959::AID-CNCR2820541225>3.0.CO;2-5
138. Hanson LA, Soderstrom T. Human Milk: Defense Against Infection. Prog Clin Biol Res (1981) 61:147–59.
139. Isobe N. Control Mechanisms for Producing Antimicrobial Factors in Ruminant Mammary Gland. Anim Sci J (2017) 88:937–43. doi: 10.1111/asj.12808
140. Sutton D, Fuchs K, D’alton M, Goffman D. Universal Screening for SARS-CoV-2 in Women Admitted for Delivery. N Engl J Med (2020) 382:2163–4. doi: 10.1056/NEJMc2009316
141. Palanisamy A, Giri T. Reduced Severe Acute Respiratory Syndrome Coronavirus 2 Entry Factors and Enhanced Innate Immune Gene Expression in the Nasal Epithelium of Pregnant Rats. Am J Obstet Gynecol (2021) 224:118–20. doi: 10.1016/j.ajog.2020.10.010
142. El-Shalofy AS, Hedia MG. Exogenous Oxytocin Administration Improves the Testicular Blood Flow in Rams. Andrologia (2021) 53:e14193. doi: 10.1111/and.14193
143. Smith JC, Sausville EL, Girish V, Yuan ML, Vasudevan A, John KM, et al. Cigarette Smoke Exposure and Inflammatory Signaling Increase the Expression of the SARS-CoV-2 Receptor ACE2 in the Respiratory Tract. Dev Cell (2020) 53:514–529 e513. doi: 10.1016/j.devcel.2020.05.012
144. Van Hedger K, Kushner MJ, Lee R, De Wit H. Oxytocin Reduces Cigarette Consumption in Daily Smokers. Nicotine Tob Res (2019) 21:799–804. doi: 10.1093/ntr/nty080
145. Sorg H, Grambow E, Eckl E, Vollmar B. Oxytocin Effects on Experimental Skin Wound Healing. Innov Surg Sci (2017) 2:219–32. doi: 10.1515/iss-2017-0033
146. Iseri SO, Gedik IE, Erzik C, Uslu B, Arbak S, Gedik N, et al. Oxytocin Ameliorates Skin Damage and Oxidant Gastric Injury in Rats With Thermal Trauma. Burns (2008) 34:361–9. doi: 10.1016/j.burns.2007.03.022
147. Chen D, Zhao J, Wang H, An N, Zhou Y, Fan J, et al. Oxytocin Evokes a Pulsatile PGE2 Release From Ileum Mucosa and is Required for Repair of Intestinal Epithelium After Injury. Sci Rep (2015) 5:11731. doi: 10.1038/srep11731
148. Benke C, Autenrieth LK, Asselmann E, Pane-Farre CA. Lockdown, Quarantine Measures, and Social Distancing: Associations With Depression, Anxiety and Distress at the Beginning of the COVID-19 Pandemic Among Adults From Germany. Psychiatry Res (2020) 293:113462. doi: 10.1016/j.psychres.2020.113462
149. Kramer P, Bressan P. Infection Threat Shapes Our Social Instincts. Behav Ecol Sociobiol (2021) 75:47. doi: 10.1007/s00265-021-02975-9
150. Yitayih Y, Lemu YK, Mekonen S, Mecha M, Ambelu A. Psychological Impact of COVID-19 Outbreak Among Jimma University Medical Centere Visitors in Southwestern Ethiopia: A Cross-Sectional Study. BMJ Open (2021) 11:e043185. doi: 10.1136/bmjopen-2020-043185
151. Vowels LM, Carnelley KB, Stanton SCE. Attachment Anxiety Predicts Worse Mental Health Outcomes During COVID-19: Evidence From Two Studies. Pers Individ Dif (2022) 185:111256. doi: 10.1016/j.paid.2021.111256
152. Ayers JD, Guevara Beltran D, Van Horn A, Cronk L, Todd PM, Aktipis A. Younger People and People With Higher Subjective SES Experienced More Negative Effects of the Pandemic on Their Friendships. Pers Individ Dif (2022) 185:111246. doi: 10.1016/j.paid.2021.111246
153. Parker KJ, Buckmaster CL, Schatzberg AF, Lyons DM. Intranasal Oxytocin Administration Attenuates the ACTH Stress Response in Monkeys. Psychoneuroendocrinology (2005) 309(9):111246. doi: 10.1016/j.psyneuen.2005.04.002
154. Kim S-W, Su K-P. Using Psychoneuroimmunity Against COVID-19. Brain Behav Immun (2020) 87:4–5. doi: 10.1016/j.bbi.2020.03.025
155. Soumier A, Sirigu A. Oxytocin as a Potential Defence Against Covid-19? Med Hypotheses (2020) 140:109785. doi: 10.1016/j.mehy.2020.109785
156. Grinevich V, Neumann ID. Brain Oxytocin: How Puzzle Stones From Animal Studies Translate Into Psychiatry. Mol Psychiatry (2021) 26:265–79. doi: 10.1038/s41380-020-0802-9
158. Diep PT, De Kok V. Could Oxytocin Reduce Autoimmune Disease in COVID-19? Autoimmun Rev (2021) 21:102994. doi: 10.1016/j.autrev.2021.102994
159. Peters S, Slattery DA, Uschold-Schmidt N, Reber SO, Neumann ID. Dose-Dependent Effects of Chronic Central Infusion of Oxytocin on Anxiety, Oxytocin Receptor Binding and Stress-Related Parameters in Mice. Psychoneuroendocrinology (2014) 42:225–36. doi: 10.1016/j.psyneuen.2014.01.021
160. Gossen A, Hahn A, Westphal L, Prinz S, Schultz RT, Grunder G, et al. Oxytocin Plasma Concentrations After Single Intranasal Oxytocin Administration - a Study in Healthy Men. Neuropeptides (2012) 46:211–5. doi: 10.1016/j.npep.2012.07.001
161. Hatton GI, Wang YF. Neural Mechanisms Underlying the Milk Ejection Burst and Reflex. Prog Brain Res (2008) 170:155–66. doi: 10.1016/S0079-6123(08)00414-7
162. Ferguson JKW. A Study of the Motility of the Intact Uterus at Term. Surg Gynecol Obstet (1941) 73:359–66.
163. Travagli RA, Anselmi L. Vagal Neurocircuitry and its Influence on Gastric Motility. Nat Rev Gastroenterol Hepatol (2016) 13:389–401. doi: 10.1038/nrgastro.2016.76
164. Flanagan LM, Verbalis JG, Stricker EM. Effects of Anorexigenic Treatments on Gastric Motility in Rats. Am J Physiol (1989) 256:R955–61. doi: 10.1152/ajpregu.1989.256.4.R955
165. Verbalis JG, Mccann MJ, Mchale CM, Stricker EM. Oxytocin Secretion in Response to Cholecystokinin and Food: Differentiation of Nausea From Satiety. Science (1986) 232:1417–9. doi: 10.1126/science.3715453
166. Narmaki E, Borazjani M, Ataie-Jafari A, Hariri N, Doost AH, Qorbani M, et al. The Combined Effects of Probiotics and Restricted Calorie Diet on the Anthropometric Indices, Eating Behavior, and Hormone Levels of Obese Women With Food Addiction: A Randomized Clinical Trial. Nutr Neurosci (2020) 1–13. doi: 10.1080/1028415X.2020.1826763
167. Bear T, Dalziel J, Coad J, Roy N, Butts C, Gopal P. The Microbiome-Gut-Brain Axis and Resilience to Developing Anxiety or Depression Under Stress. Microorganisms (2021) 9:723. doi: 10.3390/microorganisms9040723
168. Cowley KC. Psychogenic and Pharmacologic Induction of the Let-Down Reflex can Facilitate Breastfeeding by Tetraplegic Women: A Report of 3 Cases. Arch Phys Med Rehabil (2005) 86:1261–4. doi: 10.1016/j.apmr.2004.10.039
169. Machmudah, Khayati N, Widodo S, Hapsari ED, Haryanti F. Increasing Oxytocin Hormone Levels in Postpartum Mothers Receiving Oketani Massage and Pressure in The GB-21 Acupressure Point. Int J Adv Life Sci Res (2018) 2:22–7. doi: 10.31632/ijalsr.2019v02i01.004
170. Hou D, Jin F, Li J, Lian J, Liu M, Liu X, et al. Model Roles of the Hypothalamo-Neurohypophysial System in Neuroscience Study. Biochem Pharmacol (Los Angel) (2016) 5:211. doi: 10.4172/2167-0501.1000211
171. Burns EE, Blamey C, Ersser SJ, Barnetson L, Lloyd AJ. An Investigation Into the Use of Aromatherapy in Intrapartum Midwifery Practice. J Altern Complement Med (2000) 6:141–7. doi: 10.1089/acm.2000.6.141
172. Hamdamian S, Nazarpour S, Simbar M, Hajian S, Mojab F, Talebi A. Effects of Aromatherapy With Rosa Damascena on Nulliparous Women’s Pain and Anxiety of Labor During First Stage of Labor. J Integr Med (2018) 16:120–5. doi: 10.1016/j.joim.2018.02.005
173. Mcneilly AS, Robinson IC, Houston MJ, Howie PW. Release of Oxytocin and Prolactin in Response to Suckling. Br Med J (Clin Res Ed) (1983) 286:257–9. doi: 10.1136/bmj.286.6361.257
174. Nilsson U. Soothing Music can Increase Oxytocin Levels During Bed Rest After Open-Heart Surgery: A Randomised Control Trial. J Clin Nurs (2009) 18:2153–61. doi: 10.1111/j.1365-2702.2008.02718.x
175. Devarajan K, Marchant EG, Rusak B. Circadian and Light Regulation of Oxytocin and Parvalbumin Protein Levels in the Ciliated Ependymal Layer of the Third Ventricle in the C57 Mouse. Neuroscience (2005) 134:539–47. doi: 10.1016/j.neuroscience.2005.04.034
176. Ciosek J, Drobnik J. Function of the Hypothalamo-Neurohypophysial System in Rats With Myocardial Infarction is Modified by Melatonin. Pharmacol Rep (2012) 64:1442–54. doi: 10.1016/S1734-1140(12)70942-8
177. Bellosta-Batalla M, Del Carmen Blanco-Gandia M, Rodriguez-Arias M, Cebolla A, Perez-Blasco J, Moya-Albiol L. Brief Mindfulness Session Improves Mood and Increases Salivary Oxytocin in Psychology Students. Stress Health (2020) 36:469–77. doi: 10.1002/smi.2942
178. Cera N, Vargas-Caceres S, Oliveira C, Monteiro J, Branco D, Pignatelli D, et al. How Relevant is the Systemic Oxytocin Concentration for Human Sexual Behavior? A Systematic Review. Sex Med (2021) 9:100370. doi: 10.1016/j.esxm.2021.100370
179. Dekker A, Wenzlaff F, Biedermann SV, Briken P, Fuss J. VR Porn as “Empathy Machine”? Perception of Self and Others in Virtual Reality Pornography. J Sex Res (2021) 58:273–8. doi: 10.1080/00224499.2020.1856316
180. Debackere M, Peeters G, Tuyittens N. Reflex Release of an Oxytocic Hormone by Stimulation of Genital Organs in Male and Female Sheep Studied by a Cross-Circulation Technique. J Endocrinol (1961) 22:321–34. doi: 10.1677/joe.0.0220321
181. Jong TR, Menon R, Bludau A, Grund T, Biermeier V, Klampfl SM, et al. Salivary Oxytocin Concentrations in Response to Running, Sexual Self-Stimulation, Breastfeeding and the TSST: The Regensburg Oxytocin Challenge (ROC) Study. Psychoneuroendocrinology (2015) 62:381–8. doi: 10.1016/j.psyneuen.2015.08.027
182. Summerlee AJ. Extracellular Recordings From Oxytocin Neurones During the Expulsive Phase of Birth in Unanaesthetized Rats. J Physiol (1981) 321:1–9. doi: 10.1113/jphysiol.1981.sp013967
183. Komisaruk BR, Sansone G. Neural Pathways Mediating Vaginal Function: The Vagus Nerves and Spinal Cord Oxytocin. Scand J Psychol (2003) 44:241–50. doi: 10.1111/1467-9450.00341
184. Morales R, Criollo MA, Gonzalez M, Medina G, Manriquez OM, Gonzalez VM, et al. Benefit of Oxytocin Released by Cervix Stimulation in Mexican Holstein Cattle. J Adv Vet Anim Res (2020) 7:608–13. doi: 10.5455/javar.2020.g458
185. Baljon KJ, Romli MH, Ismail AH, Khuan L, Chew BH. Effectiveness of Breathing Exercises, Foot Reflexology and Back Massage (BRM) on Labour Pain, Anxiety, Duration, Satisfaction, Stress Hormones and Newborn Outcomes Among Primigravidae During the First Stage of Labour in Saudi Arabia: A Study Protocol for a Randomised Controlled Trial. BMJ Open (2020) 10:e033844. doi: 10.1136/bmjopen-2019-033844
186. Morhenn V, Beavin LE, Zak PJ. Massage Increases Oxytocin and Reduces Adrenocorticotropin Hormone in Humans. Altern Ther Health Med (2012) 18:11–8.
187. Torkzahrani S, Ghobadi K, Heshmat R, Shakeri N, Jalali Aria K. Effect of Acupressure on Cervical Ripening. Iran Red Crescent Med J (2015) 17:e28691. doi: 10.5812/ircmj.28691
188. Koh LM, Percival B, Pauley T, Pathak S. Complementary Therapy and Alternative Medicine: Effects on Induction of Labour and Pregnancy Outcome in Low Risk Post-Dates Women. Heliyon (2019) 5:e02787. doi: 10.1016/j.heliyon.2019.e02787
189. Thomas PA, Kim S. Lost Touch? Implications of Physical Touch for Physical Health. J Gerontol B Psychol Sci Soc Sci (2021) 76:e111–5. doi: 10.1093/geronb/gbaa134
190. Yahya FD, Ahmad M, Usman AN, Sinrang AW, Alasiry E, Bahar B. Potential Combination of Back Massage Therapy and Acupressure as Complementary Therapy in Postpartum Women for the Increase in the Hormone Oxytocin. Enferm Clin (2020) 30 Suppl 2:570–2. doi: 10.1016/j.enfcli.2019.07.163
Keywords: hypothalamus, neuroendocrinology, neurohumoral reflex, physiotherapy, prevention, SARS-CoV-2, treatment, virus
Citation: Wang SC, Zhang F, Zhu H, Yang H, Liu Y, Wang P, Parpura V and Wang Y-F (2022) Potential of Endogenous Oxytocin in Endocrine Treatment and Prevention of COVID-19. Front. Endocrinol. 13:799521. doi: 10.3389/fendo.2022.799521
Received: 21 October 2021; Accepted: 14 March 2022;
Published: 03 May 2022.
Edited by:
Hubert Vaudry, Université de Rouen, FranceReviewed by:
Shetty Ravi Dyavar, Adicet Bio Inc, United StatesCopyright © 2022 Wang, Zhang, Zhu, Yang, Liu, Wang, Parpura and Wang. This is an open-access article distributed under the terms of the Creative Commons Attribution License (CC BY). The use, distribution or reproduction in other forums is permitted, provided the original author(s) and the copyright owner(s) are credited and that the original publication in this journal is cited, in accordance with accepted academic practice. No use, distribution or reproduction is permitted which does not comply with these terms.
*Correspondence: Yu-Feng Wang, eXVmZW5nd2FuZ0BlbXMuaHJibXUuZWR1LmNu; Vladimir Parpura, dmxhZEB1YWIuZWR1; Fengmin Zhang, ZmVuZ21pbnpoYW5nQGVtcy5ocmJtdS5lZHUuY24=; Stephani C. Wang, c3RlcGhjdzJAaHMudWNpLmVkdQ==
†These authors have contributed equally to this work
Disclaimer: All claims expressed in this article are solely those of the authors and do not necessarily represent those of their affiliated organizations, or those of the publisher, the editors and the reviewers. Any product that may be evaluated in this article or claim that may be made by its manufacturer is not guaranteed or endorsed by the publisher.
Research integrity at Frontiers
Learn more about the work of our research integrity team to safeguard the quality of each article we publish.