- 1Department of Zoology, School of Biological Science, Central University of Kerala, Periye, Kerala, India
- 2Manipal Centre for Biotherapeutics Research, Manipal Academy of Higher Education, Manipal, Karnataka, India
Leydig cells (Lc) reside in the interstitial compartment of the testis and are the target of Luteinising hormone (LH) for Testosterone (T) production, thus critically regulates male fertility. Classical histological studies have identified two morphologically different populations of Lc during testicular development [fetal (FLc) and adult (ALc)]. Recent progress in ex vivo cell/organ culture, genome-wide analysis, genetically manipulated mouse models, lineage tracing, and single-cell RNA-seq experiments have revealed the diverse cellular origins with differential transcriptomic and distinct steroidogenic outputs of these populations. FLc originates from both coelomic epithelium and notch-active Nestin-positive perivascular cells located at the gonad–mesonephros borders, and get specified as Nr5a1 (previously known as Ad4BP/SF-1) expressing cells by embryonic age (E) 12.5 days in fetal mouse testes. These cells produce androstenedione (precursor of T, due to lack of HSD17β3 enzyme) and play critical a role in initial virilization and patterning of the male external genitalia. However, in neonatal testis, FLc undergoes massive regression/dedifferentiation and gradually gets replaced by T-producing ALc. Very recent studies suggest a small fraction (5-20%) of FLc still persists in adult testis. Both Nestin-positive perivascular cells and FLc are considered to be the progenitor populations for ALc. This minireview article summarizes the current understanding of Lc development in fetal and adult testes highlighting their common or diverse cellular (progenitor/stem) origins with respective functional significance in both rodents and primates. (227 words)
1 Introduction
Testosterone (T), which is produced by testicular Leydig cells (Lc), is essential for the fetal differentiation of male reproductive track, virilization of male external genitalia, pubertal maturation of testicular Sertoli cells (Sc) followed by meiotic progression of male germ cells (Gc) and spermiation, and controls sex drive/libido, making it an absolutely indispensable factor for male fertility (1). Historically, in 1850, German anatomist Franz von Leydig identified these cells in the interstitial compartment of seminiferous tubules (2). Bouin and Ancel suggested that androgens are produced by the interstitial Lc in 1903 (3). During the 1960s, Hall & Eik-Nes (4) and Ewing & Eik-Nes (5) independently proposed the involvement of pituitary gonadotropins in synthesis of testicular androgens. Finally, in 1969, Hall et al., demonstrated the bioconversion of T from cholesterol by these cells (6).
Classical histological studies have identified two morphologically distinct sub-populations of Lc during testicular development (7–10). In fetal mouse testis, Lc gets specified soon after sex determination (8–10). The expansion of fetal Leydig cells (FLc) occurs throughout the in utero life, peaking during birth, gradually declines and subsequently disappears during neonatal/pre-pubertal life (8–10). During pubertal testicular maturation, a second population known as Adult Leydig cells (ALc) get differentiated and colonize the testicular interstitium to support masculinity and male fertility throughout adulthood (8–10). The ALc are stimulated by luteinizing hormone (LH); LH binds its cognate receptor (LH-R, a typical G protein coupled receptor) on the ALc and initiate cAMP signaling, which in turn activates the protein kinase A (PKA) to induce expression of steroidogenic acute regulatory protein (STAR). STAR operates on mitochondria to stimulate cholesterol transport from outer to inner membranes and thereby initiates the bioconversion of pregnenolone by CYP11A and subsequently leading to synthesis of cholesterol. However, both of the Lc populations are significantly different in terms of origin, morphology, histology, and physiology (8–10). This article critically discusses the fundamental concepts of Lc differentiation during fetal and post-natal testicular development.
2 FLc
2.1 Origin
In mice, the nascent bipotential gonads arise from the coelomic epithelial layers surrounding the mesonephros, at embryonic age (E) E 9.5-10.5 days (10, 11). The transcriptional cascade of sex determination gets triggered with activation of Sry gene by Sc in XY embryos at E 11.5 days (11). FLc get specified in XY gonads by E 12.5 days (11). Multiple cellular origins have been demonstrated in contributing to the FLc population e.g.- coelomic epithelium, neural crest, notch active, Nestin-positive perivascular cells located at the gonad-mesonephros border region (12, 13) or interstitial nuclear receptor subfamily 5, group A, member 1 (Nr5a1or Ad4BP/SF-1)-positive (14) and WT1 (Wilms’ Tumor Gene 1) - positive stem/progenitor cells (15) etc.
2.2 Differentiation
The FLc expands in number throughout the fetal life, peaking around birth (10). Despite being mitotically quiescent (16, 17), the dramatic rise in FLc numbers is considered to be contributed by the constant differentiation of multiple stem/progenitor cells (18). Several factors like NR5A1 or SF1, dosage-sensitive sex reversal, adrenal hypoplasia critical region, on chromosome X, gene 1 (DAX1), desert hedgehog (DHH), platelet-derived growth factor- A (PDGF-A), orphan nuclear receptor chicken ovalbumin upstream promoter transcription factor II (COUP-TFII), insulin-like growth factor 1 (IGF-1), hepatocyte growth factor (HGF), homeobox gene Aristaless (ARX), etc. regulate this differentiation process (19). Double mutants of Sf-1 and Daz1 show a complete loss of FLc suggesting a synergistic effect of these two factors on FLc differentiation (20). Both DHH and PDGF-A are derived from fetal Sc and act via respective receptors e.g.- Patched (Ptch) 1 and PDGF-α expressed by interstitial stem/progenitor cells (18, 19). Furthermore, data from either Dhh-null mouse (showing reduced numbers of FLc) (21) or over-expressing/constitutively activated DHH-induced downstream signaling molecule Gli 1 (Gli- Kru¨ppel family of transcription factor1) (22) and Smo (23) (showing hyperplasia of FLc) confirmed the crucial inductive role of DHH signaling in FLc differentiation. COUP-TFII plays a critical role in maintaining the FLc pool either by promoting the differentiation of FLc-progenitor cells (24) or by repressing the functional maturation of FLc via antagonizing SF1 (25). However, constitutive activation of Notch signaling in fetal testes leads to a dramatic decrease in FLc number, suggesting Notch signaling (downstream target Hes1) being a negative regulator of FLc differentiation (26). Notably, vasculature-dependent Notch signaling has been shown to regulate the critical balance between self-renewal and differentiation of FLc stem/progenitor cells (13). Firstly, FLc-progenitors that are derived from coelomic epithelium progressively lose Notch activation as they ingress into fetal testes, thereby directly get differentiated to FLc (13). Secondly, FLc-progenitors that originate from mesenchymal-perivascular progenitors get exposed to high Notch signal [since they co-migrate with JAG-1-positive (Notch-ligand) endothelial cells from the mesonephros into nascent testis] thereby serving as a potential pool of Lc-stem cells via self-renewal (13).
2.3 Key features & functions
Differentiated FLc are found to be transcriptionally robust as compared to that of the non-steroidogenic, interstitial, SF1-positive stem/progenitor cells. To be specific, the transcription of essential enzymes/cofactors involved in energy-generating critical metabolic pathways e.g. – glycolysis, TCA cycle, oxidative phosphorylation etc. are found to be augmented in FLc (27). Despite being responsive towards embryonic pituitary LH, functions of FLc are completely independent of gonadotropins (10). Notably, unlike ALc, mouse FLc express 11β-hydroxylase (Cyp11β1) & 21-hydroxylase (Cyp21) however lack 17β-hydroxysteroid dehydrogenase type 3 (Hsd17β3). Therefore, although they can respond to ACTH signals but fail to convert androstenedione to T (10). In fetal mouse testes, androstenedione gets converted totestosterone by Sc (10). In mice, intra-testicular T is first detectable at E 13.0 days then peaks at E 17-19 days prior or during the time of birth (1). In utero life,testosterone induces the initial virilization of the male external genitalia (1). The other critical hormone produced by FLc is INSL3, a member of the insulin-relaxin family of peptides which operates via G-protein coupled receptor relaxin/insulin-like family peptide receptor 2 (RXFP2). Null mutations in either Insl3 or Rxfp2 result in cryptorchidism (28, 29). On the other hand, transabdominally descended ovaries are found in female mice over-expressing Insl3 (30, 31).
2.4 Fate in post-natal testes
The differentiation of FLc occurs throughout the fetal life, peaking during birth, gradually declining by the first two weeks of postnatal life, and subsequently disappears completely during pre-pubertal age (1). The concentration of intra-testicular (and in systemic circulation)T also drops along with the progressive regression of FLc in neonatal mouse (32). However, external supplementations of T from day 7 to 11 of postnatal age substantially augments Notch signalling in Androgen Receptor (AR) expressing perivascular cells indicating that T may provide a feedback response to maintain the FLc stem/progenitor pool (32). Classical histological studies (33–35) and recent lineage tracing experiments (36) have demonstrated that a sub-fraction of FLc are retained in adult testes, contributing around 5–20% of the total Lc population. This unique population of FLc persistent in adult testes remains functionally debatable as they remain unresponsive towards T, despite sharing comparable transcriptomic profiles with that of ALc (37, 38).
3 ALc
3.1 Origin, differentiation and capacity of regeneration
Since it has been claimed that FLc are replaced by ALc in pre-pubertal mouse testes, researchers continue to argue on the presence of a common stem/progenitor pool for both FLc and ALc (18, 19) or alternatively having a unique stem/progenitor system specific to ALc (39). Although FLc number remains unaffected in AR knockout (AR-KO) mice, no ALc is detectable in adult testes indicating unlike, FLc, T plays a crucial rolein ALc differentiation (10). Furthermore, fetal androgen deficiency leads to compromised ALc function during adulthood indicating a critical role of T in programming these interstitial stem/progenitor cells during fetal life (40). As discussed in the earlier section, these stem/progenitor cells are non-steroidogenic, interstitial cells of multiple lineages e.g.- SF1-positive cells (18, 19) or Nestin-positive perivascular cells (13) having mesenchymal-like morphological appearances. Like FLc, DHH, PDGF-A, and COUP-TFII are considered to be the critical regulators of ALc differentiation (10, 18, 19). Dhh ablation with Sf1 haploinsufficiency leads to the complete loss of both FLc and ALc (41). Furthermore, insulin-like growth factor I (IGF1), Leukemia inhibiting factor (LIF), and c-kit-ligand/Stem cell Factor (SCF) are also reported to be essential for the functional maturation of ALc (10). The presence of a potent stem/progenitor pool for ALc has been confirmed by the regeneration of ALc within 3 months of administration of alkylating agent ethylene sulfonate (EDS) in adult rat testes (42–45). It is evident that EDS can selectively ablate ALc only and the Lc-stem/progenitor cells remain insensitive towards it (44, 45). However, external administration of T inhibits/delays such regenerative process confirming T acts on perivascular cells and suppresses the differentiation of Lc-stem/progenitor cells (13, 32). Some studies also claim that the peritubular-myoid cells may serve as the precursor of ALc, too (18). Sc-derived Anti Mullerian Hormone (AMH) has been shown as a negative regulator of Lc-stem/progenitor cells (10). AMH over-expressing (46) or AMH-KO (47) adult mice have been shown to have poor ALc number and Lc hyperplasia respectively. Characterization of these stem/progenitor cells of ALc has also been achievable by isolation and culture from 7-days-old neonatal rat testes. These cells continue to show indefinite proliferative capacity (via self-renewal) in long-term culture, thyroid hormone, IGF1, and LH, stimulated in vitro maturation with elevated expression of 3β-HSD & production of T and post-transplantation colonizing ability to the host-testicular interstitium and subsequent differentiation to ALc in vivo (48).
3.2 Uniqueness
As compared to FLc-morphology, ALc have relatively lower lipid droplets with a robust network of smooth endoplasmic reticulum (sER) and tubule-vesicular mitochondria, etc. (10). Moreover, unlike FLc, ALc are ACTH insensitive and the development and function of ALc are completely dependent on LH and T (49). Recent studies have demonstrated the requirement of AR in LH-induced differentiation of ALc by inhibiting the adrenal characteristics in the testicular interstitium (50). The expressions of StAR, Cyp11a1, Cyp17a1, 3β-HSD type 6, and 17β-HSD type 3 are substantially higher in ALc as compared to FLc (1). Finally, unlike FLc, the maturing/differentiating ALc are mitotically active and TGFβ, IGF1, NGF, etc signaling pathways promote such proliferation (10).
3.3 Function
In the adult male, androgens critically maintain masculinity (sex drive/erectile function) and fertility (1). ALc expresses both LH-receptor (LH-R) and AR (10). LH critically regulates Lc steroidogenesis (biosynthesis of testicular androgen, T) and T acts on ALc via an autocrine fashion (1). Although Lc-specific AR knockout (KO) mice are found to be sub-fertile (51, 52), both LH-β (53) and LH-R (54) null mice are sterile, indicating critical endocrine dependence of ALc.T is absolutely required for pubertal maturation of Sc (55), the establishment of blood-testis barrier (BTB) (56), progression and completion Gc meiosis and spermiation (57).
4 Difference between rodent and primate Lc
Rodent and primate Lc differ in structure, development and function (10). There are three fundamental differences observed in human FLc as compared to that of the rodents.
Firstly, FLc of both the species are independent of fetal LH action, despite being responsive towards LH signal (58). Mouse FLc express LH-R by E 16 days (10), whereas in humans LHCG-R (both for LH and hCG) is detectable in testes by the 11th week of gestation (10). FLc number or external genitalia are unaffected in hpg (hypogonadal lacking GnRH) (59), LH-R (54), LH-β (53) and ARKO (60, 61) adult male mice suggesting murine FLc are functionally independent of LH or T. In humans, T concentration peaks during 12-14 weeks of gestation fetal circulation coinciding with placental hCG which is around 10-fold higher than pituitary LH (10). The decline in fetal T is also well-correlated with the drop in circulatory hCG during the second trimester (10). Furthermore, although patients having LH-β mutations show normal masculinized development (62, 63), LHCG-R mutations lead to pseudo-hermaphroditism (64) indicating a definite role of hCG on FLc functioning in men.
Secondly,T is the major androgen in fetal murine testes (FLc produces androstenedione via the canonical pathway which gets converted toT in Sc).However, dihydrotestosterone (5α-DHT) is a more potent/bioactive androgen recently reported to be critical for the virilization of human male external genitalia (1). In the reproductive tract of adult men,T gets bio-converted to 5α-DHT by 5α-reductase type 2 (coded by the Srd5a2 gene) and men with inactive Srd5a2 mutations have ambiguous genitalia (10). However, recent studies have indicated that an alternative backdoor pathway is operational in human male fetal testes where 5α-DHT is biosynthesized [without getting converted to dehydroepiandrosterone (DHEA), using 5α-dihydro-progesterone, allopregnanolone,17-hydroxyallopregnanolone, androsterone and androstanediol as intermediates] from androstanediol by alfa-keto-reductases (coded by Akrc2 and Akrc4) (65). The critical role of this DHEA-independent backdoor pathway has been established from comparable birth defects found in men with Srd5a2 or Akrc2/4 inactivating mutations (65, 66). Intriguingly, male fetal genital development and fertility remain unaffected in Hsd17β3null mice having complete ablation of canonical production ofT (via intermediates like DHEA and androstenedione) (67).
Finally, unlike mice and rats, primate Lc development is triphasic (68, 69). In humans, FLc gets dedifferentiated by the end of the second trimester with a decline of hCG, very few FLc successfully escape this event and remain active during the time of birth (69). A unique population of neonatal-Lc (NLc) is reported in neonatal/infant boys for the first 4-6 months of age when the hypothalamic-hypophyseal testicular (HHT) axis remains active (70). These NLc are morphologically comparable to FLc with anastomosing sER, pleomorphic mitochondria, extensive trans-Golgi network, etc. (69). However, like ALc these NLc lack cytoplasmic reticulum Reinke crystals (69).Multiple claims have been postulated on the fate of NLc, e.g. during the onset of the juvenile period (inactive HHT axis) massive involution occurs in NLC population, NLc undergoes a partial regression at the end of in the infantile period and a limited fraction of NLc survive synthesizing a low basal T throughput the juvenile age (69). The ALc population originates from the dedifferentiating NLc population or directly differentiates from the stem/progenitor cells (69).
5 Lc aging
Progressive decline in T production is manifested with testicular aging (71–74). Studies in both humans and rats have demonstrated that the efficacy of LH-induced T production gets diminished with testicular age, despite no significant change in the number of ALc (75, 76). Features of Lc aging include morphological alteration of sER and mitochondrial structure, compromised LH-R signaling/responsiveness, impaired cholesterol trafficking, poor expression and activity of steroidogenic enzymes, accumulation of reactive oxygen species (ROS) due to metabolic impairment/disruption of the pro/antioxidant balance and deficiency in autophagy leading to poor cellular homeostasis (75). Furthermore, the EDS–induced regenerative capacity of the Lc-stem/progenitor population is also not maintained for the long term indicating a probable influence of the aged microenvironment on Lc function (75). A most recent model of premature aging having constitutive CISD2 (CDGSH iron sulfur domain 2, a redox-active protein of ER) deficiency confirmed that the age-related dysfunction of Lc is not completely intrinsic but largely depends on the associated supportive microenvironment of the testes (77).
6 Conclusion and future directions
In summary, both traditional histological studies and modern high-throughput multi-omics approaches using genetically manipulated mice models have revealed the diverse origin of Lc during testicular development (8–10, 12, 13). Both FLc and ALc may be lineage independent of each other or share multiple common cellular precursor cells (18, 19). However, both of them significantly differ in terms of structure and function (1). The migration of mesonephric endothelial cells to the nascent testis establishes a testis-specific vasculature pattern which is considered to be the prerequisite of testicular cord formation- precursor structure of seminiferous tubules (11, 78). This testicular vascular niche recently has been shown to direct the Notch signaling to maintain the critical balance between perivascular- stem/progenitor cells and differentiating Lc (12, 13). The existence of persistently present androgen-insensitive FLc in adult testes also has been established (36). Although FLc from rodents or primates is independent of fetal LH signaling, human FLc is fully dependent on placental hCG (10). However, both LH andT signaling are critical regulators of ALc function in both species (10). Figure 1 schematically represents the developmental schedule of Lc in both mouse (A-I, A-II) and human (B-I, B-II) discussed here, whereas Table 1 summarizes the critical difference between FLc and ALc in both mouse (A) and human (B).
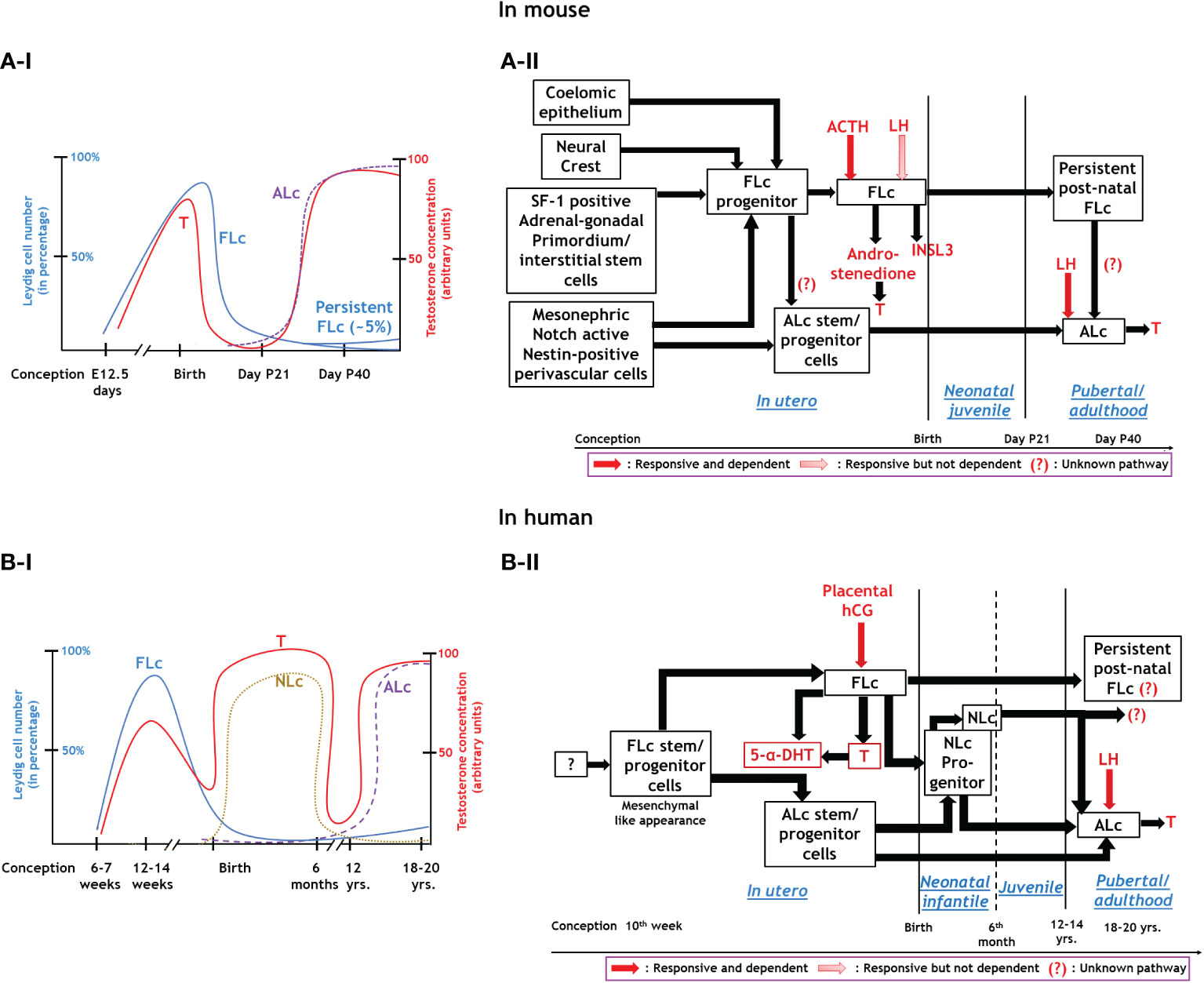
Figure 1 Endocrinal regulation and functions of three major types of Leydig cells. Panel A: In mouse; A-I: Relationship between the development of FLc and ALc with blood testosterone level during various phases of murine life. A-II: Synthesis, hormonal regulation, and function of Leydig cells in mice. Panel B: Human; B-I: Association between FLc, NLc, and ALc development and blood testosterone levels throughout human life. B-II: Synthesis, hormonal regulation, and function of different lineages of Leydig cells in humans.
Recent advancements in targeted reprogramming [like selective ablation of Wt1 in Ctnnb1 (cadherin-associated protein β1) over-expressing Sc results into Lc cell-like tumor development (79), manipulation of SF1, GATA4,etc. in either fibroblast cells (80) or in induced human pluripotent stem cells (81) leads to T producing Lc- like cells,etc.] has revolutionized the field with a potential clinical promise for cell-based therapy for hypogonadism (82, 83). However, more in-depth studies are still required in different stages of testicular maturation (fetal, neonatal, pre-pubertal, and adult) using lineage tracing and single-cell RNA-seq approaches to reveal unique molecular markers for each different stage of Lc differentiation of each lineage including Lc-stem/progenitor cells. Testicular macrophages [critical for fetal testicular morphogenesis (84) and spermatogonial differentiation (85)] are developmentally coupled with interstitial Lc (86, 87). Sincere efforts are also to be made in the future to investigate the molecular crosstalk between these two cells with respect to testicular development and aging.
Author contributions
IB conceived the idea and designed the initial draft. IB prepared the text and figure and generated the final form with active support from SD. SD revised the manuscript. All authors contributed to the article and approved the submitted version.
Funding
IB acknowledges the financial support from the University Grants Commission (F.30104/2015BSR) and Department of Science and Technology (ECR/2018/000868) New Delhi and Core fund to Dept. of Zoology, Central University of Kerala, Kasaragod, Kerala, India. SD appreciates the support obtained from Prof. Raviraja NS, Co-ordinator, Manipal Centre for Biotherapeutics Research, MAHE, Manipal. SD thanks DBT (BT/RLF/Re-entry/08/2019), New Delhi, India, for financial assistance. However, the funder was not involved in the study design, collection, analysis, interpretation of data, writing of this review.
Conflict of interest
The authors declare that the research was conducted in the absence of any commercial or financial relationships that could be construed as a potential conflict of interest.
Publisher’s note
All claims expressed in this article are solely those of the authors and do not necessarily represent those of their affiliated organizations, or those of the publisher, the editors and the reviewers. Any product that may be evaluated in this article, or claim that may be made by its manufacturer, is not guaranteed or endorsed by the publisher.
References
1. R Zirkin B, Papadopoulos V. Leydig cells: formation, function, and regulation. Biol Reprod (2018) 99(1):101–11. doi: 10.1093/biolre/ioy059
2. Leydig F. Zur anatomie der m¨annlichen geschlechtsorgane und analdru¨ sen der sa¨ugethiere. Z f Wiss Zool (1850) 2:1–57.
3. Bouin P, Ancel P. Recherches sur les cellules interstitielles du testicle des mammif`eres. Arch Zool Exp Gen (1903) 1:437–523.
4. Hall PF, Eik-Nes KB. The action of gonadotropic hormones upon rabbit testis in vitro. Biochim Biophys Acta (1962) 63(3):411–22. doi: 10.1016/0006-3002(62)90105-1
5. Ewing LL, Eik-Nes KB. On the formation of testosterone by the perfused rabbit testis. Can J Biochem (1966) 44(10):1327–44. doi: 10.1139/o66-152
6. Hall PF, Irby DC, De Kretser DM. Conversion of cholesterol to androgens by rat testes: comparison of interstitial cells and seminiferous tubules. Endocrinology (1969) 84(3):488–96. doi: 10.1210/endo-84-3-488
7. Roosen-Runge EC, Anderson D. The development of the interstitial cells in the testis of the albino rat. Acta Anat. (Basel). (1959) 37:125–37.
8. Habert R, Lejeune H, Saez JM. Origin, differentiation and regulation of fetal and adult leydig cells. Mol Cell Endocrinol (2001) 179:47–74. doi: 10.1016/S0303-7207(01)00461-0
9. Haider SG. Cell biology of leydig cells in the testis. Int Rev Cytol. (2004) 233:181–241. doi: 10.1016/S0074-7696(04)33005-6
10. Teerds KJ, Huhtaniem IT. Morphological and functional maturation of Leydig cells: from rodent models to primates. Human reproduction update (2015) 21(3):310–28. doi: 10.1093/humupd/dmv008
11. Brennan J, Capel B. One tissue, two fates: molecular genetic events that underlie testis versus ovary development. Nat Rev Genet (2004) 5:509–21. doi: 10.1038/nrg1381
12. DeFalco T, Takahashi S, Capel B. Two distinct origins for leydig cell progenitors in the fetal testis. Dev Biol (2011) 352:14–26. doi: 10.1016/j.ydbio.2011.01.011
13. L Kumar D, DeFalco T. A perivascular niche for multipotent progenitors in the fetal testis. Nat Commun (2018) 9(1):4519. doi: 10.1038/s41467-018-06996-3
14. Stevant I, Neirijnck Y, Borel C, Escoffier J, Smith LB, Antonarakis SE, et al. Deciphering cell lineage specification during male sex determination with single-cell RNA sequencing. Cell Rep (2018) 22:1589–99. doi: 10.1016/j.celrep.2018.01.043
15. Liu C, Rodriguez K, Yao HH. Mapping lineage progression of somatic progenitor cells in the mouse fetal testis. Development (2016) 143:3700–10. doi: 10.1242/dev.135756
16. Orth JM. Proliferation of sertoli cells in fetal and postnatal rats: a quantitative autoradiographic study. Anat Rec (1982) 203:485–92. doi: 10.1002/ar.1092030408
17. Byskov AG. Differentiation of mammalian embryonic gonad. Physiol Rev (1986) 66:71–117. doi: 10.1152/physrev.1986.66.1.71
18. Shima Y, Morohashi K-I. Leydig progenitor cells in fetal testis. Mol Cell Endocrinol (2017) 15:445:55–64. doi: 10.1016/j.mce.2016.12.006
19. Inoue M, Baba T, Morohashi K-I. Recent progress in understanding the mechanisms of leydig cell differentiation. Mol Cell Endocrinol (2018) 15(468):39–46. doi: 10.1016/j.mce.2017.12.013
20. Susan Y, Park JJ, Meeks G, Raverot LE, Pfaff J, Weiss GD, et al. Nuclear receptors Sf1 and Dax1 function cooperatively to mediate somatic cell differentiation during testis development. Development (2005) 132:2415–23. doi: 10.1242/dev.01826
21. Yao HH, Whoriskey W, Capel B. Desert Hedgehog/Patched 1 signaling specifies fetal leydig cell fate in testis organogenesis. Genes Dev (2002) 16:1433–40. doi: 10.1101/gad.981202
22. Kroft TL, Patterson J, Won Yoon J, Doglio L, Walterhouse DO, Iannaccone PM, et al. GLI1 localization in the germinal epithelial cells alternates between cytoplasm and nucleus: upregulation in transgenic mice blocks spermatogenesis in pachytene. Biol Reprod (2001) 65:1663–71. doi: 10.1095/biolreprod65.6.1663
23. Barsoum IB, Kaur J, Ge RS, Cooke PS, Yao HH. Dynamic changes in fetal leydig cell populations influence adult leydig cell populations in mice. FASEB J (2013) 27:2657–66. doi: 10.1096/fj.12-225060
24. Qin J, Tsai MJ, Tsai SY. Essential roles of COUP-TFII in leydig cell differentiation and male fertility. PloS One (2008). doi: 10.1371/journal.pone.0003285
25. Van den Driesche S, Walker M, McKinnell C, Scott HM, Eddie SL, Mitchell RT, et al. Proposed role for COUP-TFII in regulating fetal leydig cell steroidogenesis, perturbation of which leads to masculinization disorders in rodents. PloS One (2012) 7(5):e37064. doi: 10.1371/journal.pone.0037064
26. Tang H, Brennan J, Karl J, Hamada Y, Raetzman L, Capel B. Notch signaling maintains leydig progenitor cells in the mouse testis. Development (2008) 135:3745–53. doi: 10.1242/dev.024786
27. Inoue M, Shima Y, Miyabayashi K, Tokunaga K, Sato T, Baba T, et al. Isolation and characterization of fetal leydig progenitor cells of Male mice. Endocrinology (2016) 157:1222–33. doi: 10.1210/en.2015-1773
28. Nef S, Parada LF. Cryptorchidism in mice mutant for Insl3. Nat Genet (1999) 22:295–9. doi: 10.1038/10364
29. Ivell R, Bathgate RAD. Insulin-like peptide 3 in leydig cells. In: Payne AH, Hardy MP, editors. The leydig cell in health and disease. Totowa, New Jersey: Humana Press, Inc. (2007). p. 279–89.
30. Adham IM, Steding G, Thamm T, Bullesbach EE, Schwabe C, Paprotta I, et al. The overexpression of the insl3 in female mice causes descent of the ovaries. Mol Endocrinol (2002) 16:244–52. doi: 10.1210/mend.16.2.0772
31. Koskimies P, Suvanto M, Nokkala E, Huhtaniemi IT, McLuskey A, Themmen APN, et al. Female mice carrying a ubiquitin promoter-Insl3 transgene have descended ovaries and inguinal hernias but normal fertility. Mol Cell Endocrinol (2003) 206:159–66. doi: 10.1016/S0303-7207(02)00425-2
32. Defalco T, Saraswathula A, Briot A, Iruela-Arispe ML, Capel B. Testosterone levels influence mouse fetal leydig cell progenitors through notch signaling. Biol Reprod (2013) 88(4):91. doi: 10.1095/biolreprod.112.106138
33. Kerr JB, Knell CM. The fate of fetal leydig cells during the development of the fetal and postnatal rat testis. Development (1988) 103:535–44. doi: 10.1242/dev.103.3.535
34. Mendis Handagama M, Risbridger GP, de Kretser DM. Morphometric analysis of the components of the neonatal and the adult rat testis interstitium. Int J Androl (1987) 10:525–34. doi: 10.1111/j.1365-2605.1987.tb00352.x
35. Ariyaratne HB, Chamindrani S, Mendis-Handagama. Changes in the testis interstitium of sprague dawley rats from birth to sexual maturity. Biol Reprod (2000) 62:680–90. doi: 10.1095/biolreprod62.3.680
36. Shima Y, Matsuzaki S, Miyabayashi K, Otake H, Baba T, Kato S, et al. Fetal leydig cells persist as an androgen-independent subpopulation in the postnatal testis. Mol Endocrinol (2015) 29:1581–93. doi: 10.1210/me.2015-1200
37. McClelland S, Bell K, Larney C, Harley VR, Sinclair AH, Oshlack A, et al. Purification and transcriptomic analysis of mouse fetal leydig cells reveals candidate genes for specification of gonadal steroidogenic cells. Biol Reprod (2015) 92(6):145. doi: 10.1095/biolreprod.115.128918
38. Miyabayashi K, Shima Y, Inoue M, Sato T, Baba T, Ohkawa Y, et al. Alterations in fetal leydig cell gene expression during fetal and adult development. Sex Dev (2017) 11:53–63. doi: 10.1159/000453323
39. M. Kaftanovskaya E, Lopez C, Ferguson L, Myhr C, Agoulnik AI. Genetic ablation of androgen receptor signaling in fetal leydig cell lineage affects leydig cell functions in adult testis. FASEB J (2015) 29(6):2327–37. doi: 10.1096/fj.14-263632
40. R Kilcoyne K, B Smith L, Atanassova N, Macpherson S, McKinnell C, van den Driesche S, et al. Fetal programming of adult leydig cell function by androgenic effects on stem/progenitor cells. Proc Natl Acad Sci U.S.A. (2014) 111(18):E1924–32. doi: 10.1073/pnas.1320735111
41. Park SY, Tong M, Jameson JL. Distinct roles for steroidogenic factor 1 and desert hedgehog pathways in fetal and adult leydig cell development. Endocrinology (2007) 148:3704–10. doi: 10.1210/en.2006-1731
42. Kerr JB, Donachie K, Rommerts FF. Selective destruction and regeneration of rat leydig cells in vivo. a new method for the study of seminiferous tubular-interstitial tissue interaction. Cell Tissue Res (1985) 242:145–56. doi: 10.1007/BF00225571
43. Molenaar R, de Rooij DG, Rommerts FF, Reuvers PJ, van der Molen HJ. Specific destruction of leydig cells in mature rats after in vivo administration of ethane dimethyl sulfonate. BiolReprod (1985) 33:1213–22. doi: 10.1095/biolreprod33.5.1213
44. Teerds KJ, De Rooij DG, Rommerts FF, Wensing CJ. The regulation of the proliferation and differentiation of rat leydig cell precursor cells after EDS administration or daily HCG treatment. J Androl (1988) 9:343–51. doi: 10.1002/j.1939-4640.1988.tb01061.x
45. Jackson AE, O'Leary PC, Ayers MM, de Kretser DM. The effects of ethylene dimethane sulphonate (EDS) on rat leydig cells: evidence to support a connective tissue origin of leydig cells. Biol Reprod (1986) 35:425–37. doi: 10.1095/biolreprod35.2.425
46. Racine C, Rey R, Forest MG, Louis F, Ferre´ A, Huhtaniemi I, et al. Receptors for anti-mu¨llerian hormone on leydig cells are responsible for its effects on steroidogenesis and cell differentiation. Proc Natl Acad Sci USA (1998) 95:594–9. doi: 10.1073/pnas.95.2.594
47. Lyet L, Louis F, Forest MG, Josso N, Behringer RR, Vigier B. Ontogeny of reproductive abnormalities induced by deregulation of anti-mu¨llerian hormone expression in transgenic mice. Biol Reprod (1995) 52:444–54. doi: 10.1095/biolreprod52.2.444
48. Ge R-S, Dong Q, M Sottas C, Papadopoulos V, R Zirkin B, P Hardy M. In search of rat stem leydig cells: identification, isolation, and lineage-specific development. Proc Natl Acad Sci U.S.A. (2006) 103(8):2719–24. doi: 10.1073/pnas.0507692103
49. O’Shaughnessy PJ, Flemming LM, Jackson G, Hochgeschwender U, Reed P, Baker PJ. Adrenocorticotropic hormone directly stimulates testosterone production by the fetal and neonatal mouse testis. Endocrinology (2003) 144:3279–84. doi: 10.1210/en.2003-0277
50. J O'Shaughnessy P, T Mitchell R, Monteiro A, O'Hara L, Cruickshanks L, Claahsen-van der Grinten H, et al. Androgen receptor expression is required to ensure development of adult leydig cells and to prevent development of steroidogenic cells with adrenal characteristics in the mouse testis. BMC Dev Biol (2019) 19(1):8. doi: 10.1186/s12861-019-0189-5
51. Xu Q, Lin HY, Yeh SD, Yu IC, Wang RS, Chen YT, et al. Infertility with defective spermatogenesis and steroidogenesis in male mice lacking androgen receptor in leydig cells. Endocrine (2007) 32:96–106. doi: 10.1007/s12020-007-9015-0
52. O'Hara L, McInnes K, Simitsidellis I, Morgan S, Atanassova N, Slowikowska-Hilczer J, et al. Autocrine androgen action is essential for leydig cell maturation and function, and protects against late-onset leydig cell apoptosis in both mice and men. FASEB J (2015) 29(3):894–910. doi: 10.1096/fj.14-255729
53. Ma X, Dong Y, M Matzuk M, Kumar TR. Targeted disruption of luteinizing hormone beta-subunit leads to hypogonadism, defects in gonadal steroidogenesis, and infertility. Proc Natl Acad Sci U S A. (2004) 101(49):17294–9. doi: 10.1073/pnas.0404743101
54. Zhang F-P, Poutanen M, Wilbertz J, Huhtaniemi I. Normal prenatal but arrested postnatal sexual development of luteinizing hormone receptor knockout (LuRKO) mice. Mol Endocrinol (2001) 15(1):172–83. doi: 10.1210/mend.15.1.0582
55. M Sharpe R, McKinnell C, Kivlin C, S Fisher J. Proliferation and functional maturation of sertoli cells, and their relevance to disorders of testis function in adulthood. Reproduction (2003) 125(6):769–84. doi: 10.1530/rep.0.1250769
56. J. McCabe M, M. Allan C, F.H. Foo C, K. Nicholls P, J. McTavish K, G. Stanton. P. Androgen initiates sertoli cell tight junction formation in the hypogonadal (hpg) mouse. BiolReprod (2012) 87(2):1–8. doi: 10.1095/biolreprod.111.094318
57. B Smith L, H Walker W. The regulation of spermatogenesis by androgens. Semin Cell Dev Biol (2014) 30:2–13. doi: 10.1016/j.semcdb.2014.02.012
58. O’Shaughnessy PJ, Baker P, Sohnius U, Haavisto AM, Charlton HM, Huhtaniemi I. Fetal development of leydig cell activity in the mouse is independent of pituitary gonadotroph function. Endocrinology (1998) 139:1141–6. doi: 10.1210/endo.139.3.5788
59. Cattanach BM, Iddon CA, Charlton HM, Chiappa SA, Fink G. Gonadotrophin-releasing hormone deficiency in a mutant mouse with hypogonadism. Nature (1977) 269:338–40. doi: 10.1038/269338a0
60. Yeh S, Tsai M-Y, Xu Q, Mu X-M, Lardy H, Huang K-E, et al. Generation and characterization of androgen receptor knockout (ARKO) mice: an in vivo model for the study of androgen functions in selective tissues. Proc Natl Acad Sci U S A. (2002) 99(21):13498–503. doi: 10.1073/pnas.212474399
61. J O'Shaughnessy P, Johnston H, Willerton L, J Baker P. Failure of normal adult leydig cell development in androgen-receptor-deficient mice. J Cell Sci (2002) 115(Pt 17):3491–6. doi: 10.1242/jcs.115.17.3491
62. Valdes-Socin 1 Hernán, Salvi R, F Daly A, C Gaillard R, Quatresooz P, Tebeu P-M, et al. Hypogonadism in a patient with a mutation in the luteinizing hormone β-subunit gene. N Engl J Med (2004) 351:2619–25. doi: 10.1056/NEJMoa040326
63. Lofrano-Porto 1 A, Barcelos Barra G, Abdala Giacomini L, Pires Nascimento P, Latronico AC, Casulari LA, et al. Luteinizing hormone beta mutation and hypogonadism in men and women. N Engl J Med (2007) 357:897–904. doi: 10.1056/NEJMoa071999
64. Themmen, Huhtaniemi. Mutations of gonadotropins and gonadotropin receptors: elucidating the physiology and pathophysiology of pituitary-gonadal function. Endocr Rev (2000) 21:551–83. doi: 10.1210/edrv.21.5.0409
65. Fluck CE, Meyer M. Why boys will be boys: two pathways of fetal testicular androgen biosynthesis are needed for male sexual differentiation. Am J Hum Genet (2011) 89:201–18. doi: 10.1016/j.ajhg.2011.06.009
66. O'Shaughnessy PJ, Antignac JP, Bizec BL, Morvan ML, Svechnikov K, Söder O, et al. Alternative (backdoor) androgen production and masculinization in the human fetus. PloS Biol (2019) 17(2):e3000002. doi: 10.1371/journal.pbio.3000002
67. Rebourcet D, Mackay R, Darbey A, K Curley M, Jørgensen A, Frederiksen H, et al. Ablation of the canonical testosterone production pathway via knockout of the steroidogenic enzyme HSD17B3, reveals a novel mechanism of testicular testosterone production. FASEB J (2020) 34(8):10373–86. doi: 10.1096/fj.202000361R
68. Nistal M, Paniagua R, Regadera J, Santamaria L, Amat P. A quantitative morphological study of human leydig cells from birth to adulthood. Cell Tissue Res (1986) 246:229–36. doi: 10.1007/BF00215884
69. Prince FP. The triphasic nature of leydig cell development in humans, and comments on nomenclature. J Endocrinol (2001) 168:213–6. doi: 10.1677/joe.0.1680213
70. Bhattacharya I, Sen Sharma S, S Majumdar S. Pubertal orchestration of hormones and testis in primates. Mol Reprod Dev (2019) 86(11):1505–30. doi: 10.1002/mrd.23246
71. Gunes S, Hekim GNT, Arslan MA, Asci R. Effects of aging on the male reproductive system. J Assist Reprod Genet (2016) 33:441–54. doi: 10.1007/s10815-016-0663-y
72. Harman SM, Metter EJ, Tobin JD, Pearson J, Blackman MR. Baltimore Longitudinal study of aging. longitudinal effects of aging on serumtotal and free testosterone levels in healthy men. J Clin Endocrinol Metab (2001) 86:724–31. doi: 10.1210/jcem.86.2.7219
73. Feldman HA, Longcope C, Derby CA, Johannes CB, Araujo AB, Coviello AD, et al. Age trends in the level of serum testosterone and other hormones in middle-agedmen: longitudinal results fromtheMassachusettsmale aging study. J Clin Endocrinol Metab (2002) 87:589–98. doi: 10.1210/jcem.87.2.8201
74. Wu FC, Tajar A, Pye SR, Silman AJ, Finn JD, O’Neill ,TW, et al. European Male Aging study group. hypothalamicpituitary– testicular axis disruptions in older men are differentially linked to age andmodifiable risk factors: the EuropeanMale aging study. J Clin Endocrinol Metab (2008) 93:2737–45. doi: 10.1210/jc.2007-1972
75. Zirkin BR, Tenover LJ. Aging and declining testosterone: past, present and hopes for the future. J Androl (2012) 33:1111–8. doi: 10.2164/jandrol.112.017160
76. Zirkin BR, Santulli R, Strandberg JD, Wright WW, Ewing LL. Testicular steroidogenesis in the aging brown Norway rat. J Androl (1993) 14:118–23.
77. Curley M, Milne L, Smith S, Jørgensen A, Frederiksen H, Hadoke P, et al. A young testicular microenvironment protects leydig cells against age-related dysfunction in a mouse model of premature aging. FASEB J (2019) 33(1):978–95. doi: 10.1096/fj.201800612R
78. Svingen T, Koopman P. Building the mammalian testis: origins, differentiation, and assembly of the component cell populations. Genes Dev (2013) 27(22):2409–26. doi: 10.1101/gad.228080.113
79. Zhang L, Chen M, Wen Q, Li Y, Wang Y, Wang Y, et al. Reprogramming of sertoli cells to fetal-like leydig cells by Wt1 ablation. Proc Natl Acad Sci U.S.A. (2015) 112(13):4003–8. doi: 10.1073/pnas.1422371112
80. Yang Z, Wu X, Chen H, Xu W, Xiang Q, Zhang Q, et al. Direct reprogramming of mouse fibroblasts toward leydig-like cells by defined factors. Stem Cell Rep (2017) 8:39–53. doi: 10.1016/j.stemcr.2016.11.010
81. Ishida T, Koyanagi-Aoi M, Yamamiya D, Onishi A, Sato K, Uehara K, et al. Differentiation of human induced pluripotent stem cells into testosterone-producing leydig-like cells. Endocrinology (2021) 162(12):bqab202. doi: 10.1210/endocr/bqab202
82. Lu Li, Papadopoulos V. Advances in stem cell research for the treatment of primary hypogonadism. Nat Rev Urol (2021) 18(8):487–507. doi: 10.1038/s41585-021-00480-2
83. Gu X, DeFalco T. In vitro differentiation of leydig cells from hiPSCs: A first step towards a cellular therapy for hypogonadism? Endocrinology (2022) 163(1):bqab221. doi: 10.1210/endocr/bqab221
84. DeFalco T, Bhattacharya I, V Williams A, M Sams D, Capel B. Yolk-sac-derived macrophages regulate fetal testis vascularization and morphogenesis. Proc Natl Acad Sci U.S.A. (2014) 111(23):E2384–93. doi: 10.1073/pnas.1400057111
85. DeFalco T, J Potter S, V Williams A, Waller B, J Kan M, Capel B. Macrophages contribute to the spermatogonial niche in the adult testis. Cell Rep (2015) 12(7):1107–19. doi: 10.1016/j.celrep.2015.07.015
86. Gaytan F, Bellido C, Aguilar E, van Rooijen N. Requirement for testicular macrophages in leydig cell proliferation and differentiation during prepubertal development in rats. J Reprod Fertil. (1994) 102:393–9. doi: 10.1530/jrf.0.1020393
Keywords: testosterone, fetal Leydig cell, adult Leydig cell, neonatal testis, progenitor
Citation: Bhattacharya I and Dey S (2023) Emerging concepts on Leydig cell development in fetal and adult testis. Front. Endocrinol. 13:1086276. doi: 10.3389/fendo.2022.1086276
Received: 01 November 2022; Accepted: 12 December 2022;
Published: 04 January 2023.
Edited by:
Lawrence Merle Nelson, Mary Elizabeth Conover Foundation, Inc., United StatesReviewed by:
Giovanni Luca, University of Perugia, ItalyCopyright © 2023 Bhattacharya and Dey. This is an open-access article distributed under the terms of the Creative Commons Attribution License (CC BY). The use, distribution or reproduction in other forums is permitted, provided the original author(s) and the copyright owner(s) are credited and that the original publication in this journal is cited, in accordance with accepted academic practice. No use, distribution or reproduction is permitted which does not comply with these terms.
*Correspondence: Indrashis Bhattacharya, aW5kcmFzaGlzLmJoYXR0YWNoYXJ5YUBjdWtlcmFsYS5hYy5pbg==; aW5kcmFzaGlzLmJoYXR0YWNoYXJ5YUBnbWFpbC5jb20=; Souvik Dey, c291dmlrLmRleUBtYW5pcGFsLmVkdQ==
†These authors have contributed equally to this work