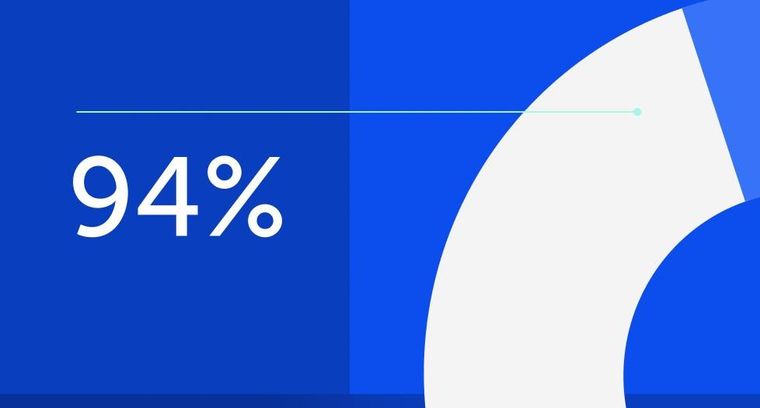
94% of researchers rate our articles as excellent or good
Learn more about the work of our research integrity team to safeguard the quality of each article we publish.
Find out more
REVIEW article
Front. Endocrinol., 09 December 2022
Sec. Molecular and Structural Endocrinology
Volume 13 - 2022 | https://doi.org/10.3389/fendo.2022.1074638
This article is part of the Research TopicThe Role of Gaseous Signaling Molecules in the Endocrine SystemView all 4 articles
Gases such as hydrogen sulfide, nitric oxide and sulfur dioxide have important regulatory effects on the endocrine and physiological processes of the body and are collectively referred to as “gas signaling molecules”. These gas signaling molecules are also closely related to Alzheimer’s disease, the inflammatory response and depression. In this paper, we introduce the production and metabolic pathways of NO, H2S and SO2 in living organisms and review the regulatory functions of gas signaling molecules in the endocrine system and their mechanisms in relation to their clinical applications. This work will provide a basis for finding targets for intervention and establishing novel prevention and treatment strategies for related diseases.
Until the mid-1980s, nitric oxide, hydrogen sulfide and sulfur dioxide were considered to be atmospheric pollutants. The toxicological effects of these gas molecules have been widely demonstrated, and their pharmacological effects and endocrine effects are gradually being revealed. These gas signaling molecules are involved in a wide range of physiological and pathological processes in the neurological, cardiovascular, digestive and metabolic systems of the body. In the organism, L-arginine is metabolized by nitric oxide synthase to produce NO, while sulfur-containing amino acids are metabolized to produce H2S and sulfur dioxide. The endogenous production of SO2, NO and H2S is involved in a wide range of physiological and pathophysiological processes in the body. In mammals, the gas-containing molecules NO, SO2 and HS share similar metabolic pathways and have a translational relationship, suggesting that there may be a close link between their regulatory roles in physiological and pathophysiological processes in vivo. These connections could provide assistance in the discovery of new signaling pathways or the development of new drugs using these gas signaling molecules. In this paper, we present the production and metabolic pathways of NO, H2S and SO2 in organisms, with a focus on the regulatory functions of gas signaling molecules in the central nervous system and endocrine system and their mechanisms in relation to their clinical applications, in the context of previous studies and a large data analysis with references.
In living organisms, endogenous H2S and SO2 can be produced by the metabolism of sulfur-containing amino acids in response to the action of relevant enzymes. Vascular endothelial cells are able to synthesize NO from L-arginine as an extracellular signal. Endogenous SO2 production in vivo is mainly based on L-cysteine as a substrate, which is spontaneously broken down into SO2 and pyruvate by the action of cysteine dioxygenase and aspartate aminotransferase to produce β-sulfinyl pyruvate. In addition, endogenous SO2 can be produced directly from endogenous H2S by the action of related enzymes. SO2 is readily converted to derivatives (HSO3-/SO32-) by water binding in the body and then to sulfate by the action of sulfite oxidase, which is ultimately metabolized by the kidneys and excreted (1).
In mammals, endogenous H2S is produced by the enzymatic reactions of cystathione-β-synthase (CBS), cystathione-γ-lyase (CSE) and 3-mercaptopyruvate transferase (3-MST) using L-cysteine and homocysteine as substrates. Among them, the key enzymes CBS and CSE are 5 ‘phosphopyridoxal-dependent enzymes with a distinct tissue-specific distribution (2–4). CBS is mainly distributed in the central nervous system, while CSE is mainly highly expressed in cardiovascular and other peripheral organs. In addition, 3-MST activity was higher in the brain and erythrocytes. The different tissue distributions suggest that H2S may have different physiological roles (5, 6). Endogenous H2S exists in the body in two main forms: sodium hydrosulfide (NaHS) and hydrogen sulfide gas (H2S). NaHS is ionized and combined with hydrogen ions to convert to H2S, a dynamic balance that maintains the pH of the internal environment. Eventually, most of the H2S in the body is converted to thiosulfate or sulfate and eliminated from the body (7–9).
In recent years, researchers have found that nitric oxide and NO donor molecules have a wide range of applications in the regulation of immunosuppression and anti-inflammation (10–14). Nitric oxide is involved in regulating mitogen-activated protein kinase (MAPK)-related signaling pathways and c-Jun amino-terminal kinase (JNK) signaling pathways, inhibiting the release of inflammatory cytokines such as tumor necrosis factor-α and macrophage inflammatory protein factor-1β (15–18). In addition, in Jurkat T cells, NO produced by NO-1 has been shown to inhibit T-cell proliferation by downregulating IL-2 secretion (19–21). Several in vitro experiments have demonstrated that NO donor molecules, by enhancing endogenous NO activity and NO-1 expression, can inhibit LPS-mediated inflammatory responses in RAW264.7 mouse macrophages (22–25). Nitric oxide releasing molecule III(NORM-3) inhibited the inflammatory response of BV-2 microglia induced by thrombin, cytokines and hypoxia. In addition, it has been shown that the anti-inflammatory effect of NORM-3 is significantly enhanced during MAPK pathway inhibition, suggesting that the anti-inflammatory mechanism of NORM-3 is not merely inhibiting enzyme activity but is closely related to deeper cellular signaling pathways. Yu et al. (26) synthesized polymeric nanomicelles formed by the self-assembly of an amphiphilic block copolymer containing an NO donor. The rate and concentration of NO release were dependent on the type of NO donor and the content of sulfhydryl material. He demonstrated the anti-inflammatory effect of NO as a signaling molecule. Incubation of the cells with the NO-releasing micelle significantly reduced the release of SEAP, suggesting that the NO containing nanomicelles could effectively inhibit the LPS-induced inflammatory response.
However, NO donors alone did not exhibit an inhibitory effect but rather exacerbated the inflammatory response (27–30). They speculated that this might be related to the byproducts. Cytotoxicity assays showed that the NO donor was cytotoxic at higher concentrations (IC50 = 650 μmol/L), while the NO-releasing micelle showed no significant cytotoxicity at 0.5 mmol/L. In addition, the NO donor was loaded with phenylboronic acid nanoparticles. In addition, a polymeric nanoparticle containing phenylboronic acid loaded with an NO donor could be prepared as an NO-releasing gas for anti-inflammatory therapy. In a model of LPS-induced inflammation, the NO donor significantly inhibited the secretion of interleukin IL-6 by macrophages. It was also less cytotoxic. NO has a good inhibitory effect on inflammation and has a promising future in the field of inflammation therapy.
NO is an important intracellular and intercellular transmitter and, as an informative substance, is associated with excitatory amino acid-mediated neurotoxicity, consciousness, learning and memory (31, 32). cGMP is then involved in the regulation of various physiological effects. Many studies now suggest that the NO/cGMP signaling system may play an important role in the molecular mechanisms underlying the anesthetic effects of general anesthetics (33).
The majority of intravenous anaesthetics are known to have a close relationship with the NO/cGMP signaling system, and this signaling system plays an important role in the study of the mechanism of general anaesthetic action of these drugs (34, 35). The effects of ketamine, tetracycline and isoproterenol on the NO/cGMP signaling system have been studied more systematically, and it is thought that inhibition of the NO/cGMP signaling system may be an aspect of the mechanism of action of these drugs in general anaesthesia. It has been shown that inhalation anesthetics inhibit the NO/cGMP signaling pathway, reducing the level of consciousness and enhancing anesthesia, analgesia and sedation.
The physiological role of endogenous H2S in the cardiovascular system has been extensively studied, and its physiological effects on the endocrine system have also received considerable attention due to the tissue-specific nature of its production-dependent enzyme distribution.
Endogenous H2S is thought to be a neuromodulatory substance whose production depends on the wide distribution of CBS and 3-MST in the brain. It was found that physiological concentrations of H2S dose-dependently predispose the hippocampal CA1 region to hippocampal long-range memory enhancement (LTP) production. A weak electrical stimulus applied to hippocampal CA1 did not induce LTP, but in the presence of H2S, the stimulus facilitated hippocampal LTP production, an effect equivalent to that induced by a tonic electrical stimulus alone. It is worth noting that neither a weak electrical stimulus alone nor a physiological amount of H2S alone triggers LTP and that the application of NaSH (the donor of H2S) 10 min before and after stimulation also does not lead to LTP production in the hippocampus, so the simultaneous application of H2S and stimulation is also an important factor (36).
In addition, the application of the NMDA receptor blocker AP-5 blocked endogenous H2S-induced LTP, thus suggesting that the occurrence of endogenous H2S-induced LTP is NMDA receptor dependent. Found that the administration of a certain concentration of NaHS (a donor of H2S) increased the amount of cyclic adenosine monophosphate (cAMP) in neuronal cells and glial cells in vitro, and thus, endogenous H2S-induced LTP was dependent on cAMP acting as a second messenger in the relevant cells (37, 38).
The hypothalamic−pituitary−adrenal axis (HPA axis) is an important part of the neuroendocrine system that is involved in the control of stress responses and is closely related to mood disorders and functional disorders (39, 40). In 2000, ex vivo experiments revealed that H2S specifically and dose-dependently inhibited the release of adrenocorticotropin-releasing hormone (CRH) in the hypothalamus and that H2S significantly inhibited KCl-induced CRH release, which may be related to the regulation of K+ channels (activation) and Ca2+ channels (inhibition) by H2S. High concentrations of H2S exhibited its toxic gas effect, a process that was associated with the inactivation of cytochrome oxidase and cytochrome activity by H2S. The hypothalamic paraventricular nucleus (PVN) is known for its activation of the HPA axis through CRH secretion, and CBS enzymes are expressed in the PVN. Zhang et al. (41) identified a new brain fat feedback axis, the leptin-FOXO3a-hypothalamic CBS/H2S system, which regulates energy homeostasis by modulating neuroendocrine hormone (TRH, ACTH) and leptin levels. The proposal of this axis complements the important role of H2S in the neuroendocrine system.
In addition to this, hydrogen sulphide can also regulate endocrine function by regulating glucose and lipid metabolism in adipocytes Adipocyte uptake of excess energy and its storage as fat is a fundamental function of adipocytes. In human mature adipocytes, hydrogen sulphide donors dose-dependently inhibit adipocyte basal and insulin-stimulated glucose uptake, and conversely, CSE inhibitors promote adipocyte glucose uptake. The application of PI3K inhibitors blocked this effect, suggesting that the function of hydrogen sulfide in regulating adipocyte glucose uptake is partially mediated by post-insulin receptor signaling. TNFα upregulated CSE-hydrogen sulphide upregulation in 3T3L1 adipocytes and induced a downregulation of adipocyte glucose uptake and a decrease in insulin responsiveness, but hydrogen sulphide donors reversed TNFα-induced insulin resistance. Hydrogen sulfide donors or precursors can inhibit homologous phosphatase-tensin (PTEN) phosphorylation of PI3K-Akt-PKCζ/λ signaling and promote glucose transporter 4 expression to increase adipocyte glucose utilization; they can also increase insulin receptor phosphorylation and activate Akt signaling to promote sugar uptake. In addition, vitamin D promoted increased sugar uptake in insulin-resistant adipocytes by inducing the expression of CSE-hydrogen sulfide. Activation of peroxisome proliferator-activated receptorγ(PPARγ) promotes glucose transporter translocation to the cell membrane, facilitating glucose uptake, and also promotes insulin signaling and transcription of multiple genes for fatty acid synthesis, improving insulin resistance. Hydrogen sulfide modifies the sulfhydration of cysteine residues at position 139 of PPARγ, increasing its transcriptional activity and also promoting glucose uptake in adipocytes. Thus, endogenous hydrogen sulfide can stabilize adipocyte glucose uptake, and when CSE-hydrogen sulfide is sufficient under physiological conditions, additional hydrogen sulfide can inhibit glucose uptake and utilization; while when stimuli such as high glucose suppress the endogenous hydrogen sulfide system and cause insulin resistance, hydrogen sulfide supplementation can improve their glucose metabolism and restore their regulation of energy. Adipocytes convert excess glucose into fatty acids which are then esterified into triglycerides and stored in lipid droplets. During starvation or stress, triglycerides are hydrolysed and free fatty acids are released, while free fatty acids are β-oxidised and ATP is released to supply the body’s needs. Inhibitors of CSE can activate PKA, which leads to the activation and translocation of hormone-sensitive lipase to the lipid droplet surface, and phosphorylation of the lipid droplet envelope protein perilipin-1, which exposes the lipid droplet to the hormone-sensitive lipase binding site and accelerates lipolysis. Conversely, hydrogen sulphide donors inhibit lipolysis.
The addition of hydrogen sulphide donors (NaHS and GYY4137) during the differentiation of adipocytes into adipocytes can increase the expression of differentiation markers such as AP2 and CEBP and increase the number of adipocytes; the application of CBS inhibitor AOAA or CSE inhibitor PPG can partially block their differentiation, and the blocking effect of PPG is more significant. Overexpression of CSE increased the differentiation of adipocytes, while knockdown of endogenous CSE significantly inhibited their differentiation. On the one hand, hydrogen sulphide acts as a phosphodiesterase inhibitor, increasing intracellular cAMP levels and activating CEBP; on the other hand, hydrogen sulphide directly thiolates the cysteine residue at 139 of PPARγ, increasing its nuclear translocation and DNA binding activity and promoting the expression of lipogenic genes. Sulfhydrylation of the 139 cysteine residue of PPARγ may regulate its protein stability and increase the expression level of PPARγ protein. It is suggested that endogenous hydrogen sulfide may act as an energy switch to regulate glycolipid conversion in adipocytes. Under physiological conditions, excess hydrogen sulfide inhibits glucose uptake and promotes the conversion of sugar into lipids for storage; when pathological conditions such as hyperglycemia and hyperlipidemia occur, CSE-hydrogen sulfide is reduced, resulting in inactivation of PPARγ, which inhibits insulin signaling and causes a decrease in glucose uptake, while inducing lipolytic reactions, releasing excess free fatty acids, causing ectopic deposition of fat, and promoting oxidative stress and inflammation in tissue cells, It also contributes to oxidative stress and inflammation in tissue cells, exacerbating disease damage.
Hydrogen sulphide can also cause adipocytes to secrete a variety of adipokines and inflammatory factors. In animals in which adipocytes were induced with hydrogen sulphide, plasma leptin levels were increased. In differentiated adipocytes from rats, overexpression of CSE increased leptin expression. Under high glucose induction, overexpression of CSE increased adiponectin expression in 3T3L1 differentiated adipocytes and decreased MCP-1 expression, but had no effect on TNFα. Therefore, hydrogen sulfide, as an autocrine factor, can promote the expression of protective adipokines, inhibit the release of inflammatory factors and regulate endocrine function.
With the discovery of the endogenous SO2 production system, the dual regulatory functions of SO2 in the central nervous system are gradually being revealed.
It was found that SO2 decreased the activity of antioxidant enzymes and increased the content of markers reflecting oxidative damage in multiple organs of the brain, lung and heart of mice. Fu et al. (42) found that in a mouse model of pulmonary hypertension and myocardial ischaemia−reperfusion injury, SO2 increased the levels of antioxidants and decreased oxidants (ROS, H2O2), and this effect was dependent on SO2 upregulating the myocardial H2S/CSE pathway and downregulating the NO/iNOS pathway. In a colitis model in which trinitrobenzenesulfonic acid induced a disturbance in the redox status of colonic tissue, Ran et al. (43) discovered that SO2 (Na2SO3:NaHSO3, 3:1) treatment restored the expression levels of antioxidant molecules while reducing the expression of GST and GSH. SO2 reduced CCH injury in a model of chronic cerebral ischemia (CCH) by increasing the antioxidant capacity of the hippocampus. All of these studies indicate that SO2 may reverse the oxidative damage process in certain diseases due to its antioxidant properties.
In earlier years, the reduction of sulfite from SO2 was widely demonstrated as an inflammatory mediator in models of acute lung injury. Schalley et al. (44) found that SO2 significantly reduced the inflammatory response to lipopolysaccharide-induced ALI by downregulating the expression of Raf-1, MEK-1 and p-ERK in the MAPK signaling pathway and inhibiting IL-1β and IL-6 production during ALI. Schalley found that in colitis, SO2 reduced inflammation by decreasing TNF-α expression and NF-κB activation. Macrophage activation-mediated inflammatory responses are an important component of inflammatory diseases. The discovery that the endogenous SO2/AAT pathway exists in macrophages and that macrophage-derived SO2 inhibits macrophage-mediated inflammatory responses via the NF-κB pathway opens new horizons for the study of anti-inflammatory therapy and mechanisms.
Lin et al. (45) found that downregulation of the endogenous SO2/AAT system is an important initiator of hypoxia-stimulated apoptosis in human pulmonary artery endothelial cells, which is mediated by upregulation of bcl-2. This suggests that the endogenous SO2/AAT system may regulate the apoptosis of neurons in epileptic rats through the activation of the endoplasmic reticulum stress-related signaling pathway by PERK and affect epileptogenesis (46). Recently, the anti-apoptotic effect of SO2 was demonstrated, and SO2 significantly reduced the rate of cell necrosis and apoptosis in the hippocampal CA1 region of transient whole brain ischemia/reperfusion (I/R) rats and improved the learning memory impairment induced by I/R (47, 48). The above studies suggest that the regulatory effect of SO2 on apoptosis may be dual in nature.
The pathogenesis of Parkinson’s disease (PD) involves degeneration of the substantia nigra-striatal dopamine pathway, significantly reduced dopamine levels and dysfunction of the Ach system. Chatterjee (49) found that endogenous H2S levels were significantly reduced in the substantia nigra (SN) in a 6-hydroxydopamine (6-OHDA)- and rotenone-induced PD rat model. NaHS reverses neurodegeneration by inhibiting NaHS and reverses neurotoxin-induced neurodegeneration by inhibiting NADPH oxidase and anti-microglia activation. In addition, H2S reversed 6-OHDA neurological damage through a nigrostriatal leptin-dependent pathway, enhancing the Warburg effect (50).
Alzheimer’s disease (AD) is a neurodegenerative disorder characterised by neuronal fibrillary tangles, with the typical clinical manifestation being a reduction in cognitive ability in the brain. The main cause of Alzheimer’s disease is the deterioration of cognitive and memory functions due to central neurodegeneration and characteristic brain degeneration. Homocysteine (Hcy) is a high risk factor for Alzheimer’s disease and high expression of Hcy is associated with characteristic brain degeneration, which in turn causes Alzheimer’s disease in patients. Wu, Y (51) found that serum levels of Hcy were significantly higher in AD patients than in normal subjects. It was found that the level of S-adenosylmethionine (AdoMet) in the brains of patients was 0.18 ± 0.04 nmol/mg, which was much lower than the normal level of 0.52 ± 0.07 nmol/mg. This was due to the low level of AdoMet in the brains of AD patients, which affected CBS activity and thus reduced the synthesis of H2S. In a severe AD model, H2S supply reduced Aβ deposition, hyperphosphorylation of p-APP (the precursor protein of Aβ) and Tau isoforms, and the inflammatory response to improve cognitive activity (52). This provides a new idea for the mechanism of the protective effect of H2S on AD.
Earlier, researchers identified a pro-apoptotic effect of NO gas on tumour cells. p53 inhibits apoptosis by causing mutations in p53. p53 regulates redox stress, induces cell death, inhibits cell migration and angiogenesis, and limits stem cell renewal, thereby inhibiting tumorigenesis (53). p53 is regulated by ubiquitin ligase (Mdm2), which targets Mdm2 targets p53 for degradation and directly inhibits p53 activity by binding to the transcriptional activation domain. The mutation rate of the tumour suppressor gene p53 in human malignant tumours is 30%-50%, and the presence of one mutated p53 protein in different combinations of p53 tetramers effectively interferes with the function of the entire tetramer, rendering its apoptotic function completely inactive (54). Thus, mutated p53 not only eliminates wild-type p53 function but also significantly impairs wild-type p53 function.
NO causes p53 mutation at concentrations below 400 nmol/L, and when NO contributes to p53 mutation, the mutated p53 has no tumour suppressive activity and impairs wild-type p53 function, leading to cell survival (55). Chromogranin C and ultimately activation of caspase-3 can also effectively inhibit apoptosis. The process of this role is shown in Figure 1.
Zhao et al. (56) used a rat model of chronic unpredictable stress (CUMS) depression and found that CUMS induced a downregulation of hippocampal CBS expression and a decrease in H2S production in rats. Exogenous H2S treatment significantly improved CUMS-induced depressive-like behaviour. Regarding the protective mechanism of H2S, Begara et al. (57) showed that the BDNF-TrkB pathway mediated the antidepressant effects of H2S in CUMS-induced rats by inhibiting hippocampal endoplasmic reticulum stress. H2S enhanced antioxidant capacity and exerted antidepressant and anxiolytic effects in streptozotocin-treated diabetic rats.
Fix et al. (58) investigated whether the effect of SO2 on brain injury in rats with recurrent febrile convulsions (FS) was dose dependent. This study found that the SO2/AAT system is involved in the FS process and that in a rat FS model, low concentrations of SO2 (Na2SO3:NaHSO3, 3:1, 1-10 μmol/kg) pretreatment reduced neuronal injury and apoptosis, whereas AAT inhibitors or high concentrations of SO2 (Na2SO3:NaHSO3, 3:1, 100 μmol/kg) produced neuronal damage effects. However, Lv et al. (59) found that in epileptic rats, endogenous SO2 induced apoptosis in hippocampal neurons via the PERK signaling pathway. Inhibition of endogenous SO2 production attenuated neuronal apoptosis at the beginning of seizures, but this attenuating effect only delayed the onset of neuronal apoptosis but did not prevent neuronal apoptosis.
As endogenous gas signaling molecules, the regulatory effects of endogenous NO, H2S and SO2 on many systems in the body and their mechanisms of action on various diseases have been elucidated, but their regulatory functions and mechanisms in the endocrine system have not been fully understood (60). Recent studies at home and abroad have shown that NO, H2S and SO2 act by similar but slightly different mechanisms and that the mechanisms of action differ in different systems.
In cells, NO is produced by nitric oxide synthase in three forms (neuronal NO synthase, nNOS or NOS I); inducible NO synthase, i NOS or NOS II and endothelial NO synthase, eNOS or NOS III (61). These enzymes use arginine as a substrate to produce NO and citrulline. The three NOS have similar molecular structures: an N-terminal oxygenase region, a calmodulin-binding region and a C-terminal reductase region. e NOS and nNOS are both activated by a rise in intracellular calcium. eNOS is highly expressed in vascular endothelial cells, while n NOS is mainly found in the nervous system, where the synthesis and release of NO from neurons is defined as a function of the presence of a coenzyme. The neurons that synthesize and release NO are defined as nitroergic neurons. In the mammalian autonomic nervous system, nNOS is usually found in parasympathetic postganglionic neurons. iNOS was first identified in macrophages and is induced in macrophages and other cells and tissues by bacterial lipopolysaccharides and growth inhibitors. iNOS is metabolized in the body mainly to NO2- and NO3-, with a small amount excreted by respiration.
In the endocrine system, NOS, as the main synthesizing enzyme of NO, plays an important role in maintaining the homeostasis of the endocrine system. These results suggest that NO can inhibit the development of atherosclerosis and other diseases.
The affinity of NO for iron in proteins can inhibit the catalytic activity of many key iron-containing enzymes, including iron-sulfur cluster-dependent enzymes that catalyse the mitochondrial respiratory chain, nucleotide reductase, and cis-aconitase. In addition, NO generated by NOS can directly interfere with the synthesis of DNA in target cells, leading to DNA strand breaks and fragmentation. In parasitic microorganisms and specific tumour cells, these effects may contribute to the cytostatic and toxic effects of NO.
NO activates GC in vascular smooth muscle cells to increase the cGMP concentration and decrease the intracellular free Ca2+ concentration, resulting in vasodilation. In addition, NO inhibits cell proliferation and counteracts vasoconstrictive substances. NO regulates a large number of mitochondrial haemoglobin proteins by a similar mechanism. The s-nitrosylation modification is an important way in which NO exerts its biological function. This is a posttranslational modification similar to phosphorylation and acetylation, in which NO or peroxynitrite acts on the cysteine sulfhydryl group (-SH) of a protein to produce -SNO. In addition, S-nitrosylated glyceraldehyde-3-phosphate dehydrogenase activates downstream apoptotic signals and regulates cell and tumour growth.
Therefore, future research should focus on the use of NO’s affinity for proteins to inhibit tumour cell growth in tumour cells. At the same time, the focus should also be on the inhibitory effect of NO on the development of diseases such as atherosclerosis. The regulatory effects of NO on the endocrine system and some cells can be used to inhibit tumour growth or inhibit disease progression.
H2S in humans is produced by the enzymatic reactions of cystathionine and homocysteine as substrates, cystathionine beta synthase (CBS), cystathionine gamma lyase (CSE), and 3-mercaptopyruvate transsulfurase (3MST). The distribution of these enzymes is tissue specific, with CBS highly expressed in the nervous system, CSE mainly in cardiovascular tissue, and both in the liver and kidney. In cellular mitochondria, H2S is rapidly oxidized to thiosulfate (S2O32-) and subsequently converted to sulfite (SO32-) and the more stable sulfate (SO42-).
Recent studies have shown that H2S signals through S-mercapturised proteins, a process in which H2S acts on cysteine sulfhydryl groups (-SH) of proteins to produce -SSH, which can be detected by a modified biotin-switch method. Under physiological conditions, many proteins in mammalian liver are modified by S-mercapturisation, and approximately 10%-25% of endogenous GADPH, β-tubulin and actin in liver are mercapturised, five to ten times more than by nitroxylation. parkin proteins have cysteine sites that can be S-mercapturised, and under physiological conditions, they can be modified by exogenous hydrogen sulfide. This suggests that reduced parkin S-mercapturization may be involved in the development of PD (62). In contrast, the free thiol group at the cysteine 38 site of NF-κB p65 can be modified by S-mercapturization, which may be a key mechanism for H2S inhibition of oxidized LDL-induced inflammation in macrophages. Further studies may reveal more S-mercapto-modified proteins and their corresponding functional alterations.
Although considerable progress has been made in the study of the mechanism of action of H2S, the molecular targets of its action are still not well understood. Earlier studies have supported the possibility that the ATP-sensitive potassium channel (KATP) may be one of the functional targets of H2S. However, in mice with knockout of the KATP channel subunit Kir6.2, H2S was still able to protect against MPTP-induced dopamine neuron deficiency, suggesting that H2S does not act directly on the KATP channel; therefore, further studies are needed to investigate the mechanism of action of hydrogen sulfide.
Although the molecular targets of H2S have not yet been identified, current studies have found that H2S has an inhibitory effect on dopamine neuron deficits as well as a modulatory effect on validation, and further research should focus on investigating the mechanisms underlying the inhibitory effect of H2S on dopamine neuron deficits, with a view to applying these mechanisms in clinical treatment. At the same time, the mechanism of H2S modulation of inflammation should be further investigated to develop H2S-based inflammation-modulating drugs.
Mammals produce SO2 through the metabolism of sulfur-containing amino acids or through the oxidation of hydrogen sulfide (H2S), catalyzed by aspartate aminotransferase (AAT). Endogenous SO2 is synthesized in both vascular endothelial and smooth muscle cells; mainly in vascular endothelial cells, endogenous SO2 synthesized in vivo can be converted to endogenous sulfite and bisulfite to produce biological effects (63).
Endogenously produced SO2 and SO2 derivatives have important cardiovascular physiological effects in the regulation of vascular tone and cardiac function, and SO2 is also pathophysiologically important in many cardiovascular diseases, such as hypertension, atherosclerosis, pulmonary hypertension, ischaemia−reperfusion injury and myocardial injury. However, the biological mechanisms by which endogenous SO2 regulates different cardiovascular diseases and other cardiovascular effects need further investigation. Studies on the cardioprotective function of SO2 provide new therapeutic strategies based on the regulation of H2S and SO2 production. However, the functional and signaling pathways associated with CSE/CBS/MPST and AAT in the cardiovascular system need to be investigated in more depth. In addition, the design of physiologically concentrated SO2-controlled drugs is urgently needed, as stable and reliable SO2 donors are not only useful research tools but also potential drugs for the treatment of endocrine diseases. Future research will focus on the development of SO2-based drugs for the treatment of endocrine disorders through the effects of SO2 and its derivatives on cardiovascular diseases and the mechanisms that regulate endocrine. To date, many cardiovascular studies with SO2 have been conducted in rats and mice, and clinical evidence is lacking. Studying the effects of H2S and SO2 in large animal models with cardiovascular disease characteristics similar to those of humans would be more useful for translation to clinical trials.
Through a keyword search in Web Of Science, we collected, screened, integrated and summarised previous studies to clarify the regulatory functions and interaction mechanisms of gas signaling molecules in the endocrine system, and analysed their mechanisms of action in various disease processes, providing a basis for identifying targets for intervention and therapeutic strategies for related diseases.
Methodology: WQ, LM, SS, YdZ, HQ, CH, HM, HG, and YzZ. Validation: WQ, LM, SS, YdZ, HQ, CH, HM, HG, and YzZ. Formal analysis: WQ, LM, SS, YdZ, HQ, CH, HM, HG, and YzZ. Investigation: WQ, LM, SS, YdZ, HQ, CH, HM, HG, and YzZ. Resources: WQ, LM, SS, YdZ, HQ, CH, HM, HG, and YzZ. Data curation: WQ and LM. Writing - original draft: WQ. Writing - review & editing: WQ and LM. Supervision: WQ and LM. Project administration: WQ. All authors contributed to the article and approved the submitted version.
This work was supported by Macau Science and Technology Development fund [FDCT (0007/2019/AKP, 0021/2020/AGJ, 0011/2020/A1, 0089/2021/A, 0088/2021/A)], The National Natural Science Foundation of China (Nos. 81973320), and Key Technology R&D Program of Science and Technology Commission Foundation of Tianjin (20YFZCSY00460).
The authors declare that the research was conducted in the absence of any commercial or financial relationships that could be construed as a potential conflict of interest.
All claims expressed in this article are solely those of the authors and do not necessarily represent those of their affiliated organizations, or those of the publisher, the editors and the reviewers. Any product that may be evaluated in this article, or claim that may be made by its manufacturer, is not guaranteed or endorsed by the publisher.
1. Aroca A, Gotor C, Romero LC. Hydrogen sulfide signaling in plants: emerging roles of protein persulfidation. Front Plant Sci (2018) 9(2):1369. doi: 10.16289/sfw.2018.01369
2. Huwiler A, Pfeilschifter J. Nitric oxide signalling with a special focus on lipid-derived mediators. Curr phar design (2018) 12(8):252–64. doi: 10.16289/cpd.2018.01369
3. Molina HA. Nitric oxide: Physical, chemical and biological properties. Acta BIOQUIMICA CLINICA LATINOAMERICANA (1996) 30(3):267–73. doi: 10.16289/3r222018.01369
4. Motterlini R, Haas B, Foresti R. Emerging concepts on the anti-inflammatory actions of carbon monoxide-releasing molecules (CO-RMs). Med Gas Res (2017) 2(1):1–12.
5. Motterlini R, Mann BE, Johnson TR, et al. Bioactivity and pharmacological actions of carbon monoxide-releasing molecules. Curr phar design (2018) 9(30):2525–39.
6. Cao Q, Zhang Q, Li XC, et al. Impact of sleep status on lung adenocarcinoma risk: a prospective cohort study. Eur Rev Med Pharmacol Sci (2022) 26(20):7641–8. doi: 10.26355/eurrev_202210_30040
7. Wang YZ, Ngowi EE, Wang D, et al. The potential of hydrogen sulfide donors in treating cardiovascular diseases. Inter J Mol Sci (2022) 22(4):2194. doi: 10.3390/ijms22042194
8. Zafonte RD, Wang L, Arbelaez CA, et al. Medical gas therapy for tissue, organ, and CNS protection: A systematic review of effects mechanisms and challenges. Advanced Sci (2022) 9(13):2104136. doi: 10.1002/advs.202104136
9. Cao Q, Zhang Q, Zhou KX, et al. Lung cancer screening study from a smoking population in kunming. Eur Rev Med Pharmacol Sci (2022) 26(19):7091–8. doi: 10.26355/eurrev_202210_29894
10. Ding H, Chang J, He F, et al. Hydrogen sulfide: an emerging precision strategy for gas therapy. Advanced Healthcare Materials (2022) 11(4):2101984. doi: 10.1002/adhm.202101984
11. Farrugia G, Szurszewski JH. Carbon monoxide, hydrogen sulfide, and nitric oxide as signaling molecules in the gastrointestinal tract. Gastroenterology (2015) 147(2):303–13.
12. Fukuto JM, Collins MD. Interactive endogenous small molecule (gaseous) signaling: implications for teratogenesis. Curr phar design (2018) 13(29):2952–78.
13. Kajimura M, Nakanishi T, Takenouchi T, et al. Gas biology: tiny molecules controlling metabolic systems. Respir Physiol Neurobiol (2017) 184(2):139–48.
14. Kimura H. Signaling molecules: hydrogen sulfide and polysulfide. Antioxidants Redox Signaling (2015) 22(5):362–76. doi: 10.1089/ars.2014.5869
15. Lamattina L, García-Mata C, Graziano M, et al. Nitric oxide: the versatility of an extensive signal molecule. Annu Rev Plant Biol (2018) 54(1):109–36.
16. Li ZG, Li E, Chen HY. Sulfur dioxide: An emerging signaling molecule in plants. Front Plant Sci (2022), 1:1538. doi: 10.3389/fpls.2022.891626
17. Motterlini R, Foresti R. Biological signaling by carbon monoxide and carbon monoxide-releasing molecules. Am J Physiology-Cell Physiol (2017) 312(3):C302–13. doi: 10.1152/ajpcell.00360.2016
18. Sarkar S, Ha YS, Soni N, et al. Immobilization of the gas signaling molecule H2S by radioisotopes: Detection, quantification, and in vivo imaging. Angewandte Chemie Inter Edition (2016) 55(32):9365–70. doi: 10.1002/anie.201603813
19. Tan H, Wong H, Bian S. Hydrogen sulfide: a novel signaling molecule in the central nervous system. Neurochem Inter (2017) 56(1):3–10.
20. Chen Y, You Y, Wang Y. Systematic and meta-based evaluation on job satisfaction of village doctors: An urgent need for solution issue. Front Med (2022) 9:856379. doi: 10.3389/fmed.2022.856379
21. Walewska A, Szewczyk A, Koprowski P. Gas signaling molecules and mitochondrial potassium channels. Inter J Mol Sci (2018) 19(10):3227. doi: 10.3390/ijms19103227
22. Wang M, Liao W. Carbon monoxide as a signaling molecule in plants. Front Plant Sci (2016) 7:572. doi: 10.3389/fpls.2016.00572
23. Xu CS, Shao HY, Du B. Study on correlation of signal molecule genes and their receptor-associated genes with rat liver regeneration. Genome (2018) 52(6):505–23.
24. Yang P, Irani K, Mattagajasingh S, et al. Signal transducer and activator of transcription 3α and specificity protein 1 interact to upregulate intercellular adhesion molecule-1 in ischemic–reperfused myocardium and vascular endothelium. Arteriosclerosis thrombosis Vasc Biol (2018) 25(7):1395–400.
25. Yu W, Jin H, Tang C, et al. Sulfur-containing gaseous signal molecules, ion channels and cardiovascular diseases. Br J Pharmacol (2018) 175(8):1114–25. doi: 10.1111/bph.13829
26. Yu Z, Zhang W, Zhang M, et al. Gas signaling molecule hydrogen sulfide attenuates doxorubicin-induced dilated cardiomyopathy. Oncotarget (2017) 8(56):95425. doi: 10.18632/oncotarget.20729
27. Gullotta F, Masi A, Coletta M, et al. CO metabolism, sensing, and signaling. Biofactors (2017) 38(1):1–13.
28. Jin Q, Zhu K, Cui W, et al. Hydrogen gas acts as a novel bioactive molecule in enhancing plant tolerance to paraquat-induced oxidative stress via the modulation of heme oxygenase-1 signalling system. Plant Cell Environ (2011) 36(5):956–69.
29. Li S, Liao R, Sheng X, et al. Hydrogen gas in cancer treatment. Front Oncol (2019), 7:696. doi: 10.3389/fonc.2019.00696
30. Otterbein E. Carbon monoxide: innovative anti-inflammatory properties of an age-old gas molecule. Antioxidants Redox Signaling (2018) 4(2):309–19.
31. Xuan L, Li J, Wang X, et al. Crosstalk between hydrogen sulfide and other signal molecules regulates plant growth and development. Inter J Mol Sci (2022) 21(13):4593.
32. You IS, Sharma S, Fadriquela A, et al. Antioxidant properties of hydrogen gas attenuates oxidative stress in airway epithelial cells. Molecules (2022) 26(21):6375.
33. Baskar R, Bian J. Hydrogen sulfide gas has cell growth regulatory role. Eur J Pharmacol (2017) 656(1-3):5–9.
34. Chen Y, You Y, Shen Y, et al. Village doctors’ dilemma in China: systematic evaluation of job burnout and turnover intention. Front Public Health (2022) 18:856379. doi: 10.3389/fmed.2022.856379
35. Domingos P, Prado M, Wong A, et al. Nitric oxide: a multitasked signaling gas in plants. Mol Plant (2015) 8(4):506–20. doi: 10.1016/j.molp.2014.12.010
36. Fukuto JM, Carrington S, Tantillo D, et al. Small molecule signaling agents: the integrated chemistry and biochemistry of nitrogen oxides, oxides of carbon, dioxygen, hydrogen sulfide, and their derived species. Chem Res Toxicol (2017) 25(4):769–93.
37. Gautam K, Negi S, Saini V. Targeting endogenous gaseous signaling molecules as novel host-directed therapies against tuberculosis infection. Free Radical Res (2022) 55(8):903–18.
38. Lee S, Nilius B, Han J. Gaseous signaling molecules in cardiovascular function: from mechanisms to clinical translation. Rev Physiology Biochem Pharmacol (2018) 174(2):81–156. doi: 10.1007/112_2017_7
39. Polhemus DJ, Lefer J. Emergence of hydrogen sulfide as an endogenous gaseous signaling molecule in cardiovascular disease. Circ Res (2015) 114(4):730–7.
40. Singh G, Patel A, Tiwari S, et al. Signaling molecules hydrogen sulfide (H2S) and nitric oxide (NO): role in microalgae under adverse environmental conditions. Acta Physiologiae Plantarum (2022) 44(7):1–15. doi: 10.1007/s11738-022-03404-8
41. Zhang GY, Jin YD. Theoretical analysis of photoacoustic spectrum of NO molecule. Spectrosc Spectral Anal (2017) 30(2):297–300.
42. Juan F, Jia M, Yisong Y, et al. Experimental study on the structure characteristics of CO2 in gas hydrate by solid-state nuclear magnetic resonance and raman spectroscopy. Spectrosc AND SPECTRAL Anal (2022) 42(2):464–9.
43. Wang R, Cai J, Chen K, et al. STAT3-NAV2 axis as a new therapeutic target for rheumatoid arthritis via activating SSH1L/Cofilin-1 signaling pathway. Signal transduction targeted Ther (2022) 7(1):1–4. doi: 10.1038/s41392-022-01050-7
44. Schalley CA, Hornung G, Schrdder D, et al. Mass spectrometry as a tool to probe the gas-phase reactivity of neutral molecules. Inter J mass spectrometry ion processes (1998) 172(3):181–208. doi: 10.1016/S0168-1176(97)00115-8
45. Lin YK, Li Y, Li Y, et al. SCM-198 prevents endometriosis by reversing low autophagy of endometrial stromal cell via balancing ERα and PR signals. Front Endocrinol (2022) 13:11429.
46. Yamada T, Shinohara H, Kamikado T, et al. Spray-jet technique for studying nonvolatile functional molecules: Comparison of different spray-jet inlet systems. Japanese J Appl Phys (2018) 47(2S):1408.
47. Qiang C, Qi Z, Yi Q. Mechanisms of p2x7 receptor involvement in pain regulation: a literature review. Acta Med Mediterr (2022) 38(2):1187–94.
48. Zavahir JS, Nolvachai Y, Marriott J. Molecular spectroscopy–information rich detection for gas chromatography. Trends Analytical Chem (2018) 99:47–65. doi: 10.1016/j.trac.2017.11.014
49. Chatterjee PK. Water-soluble carbon monoxide-releasing molecules: helping to elucidate the vascular activity of the ‘silent killer. Br J Pharmacol (2018) 142(3):391–3.
50. Thomas D. Breathing new life into nitric oxide signaling: a brief overview of the interplay between oxygen and nitric oxide. Redox Biol (2015) 5:225–33. doi: 10.1016/j.redox.2015.05.002
51. Wu Y, Wei Z, Li Y, et al. Perturbation of ephrin receptor signaling and glutamatergic transmission in the hypothalamus in depression using proteomics integrated with metabolomics. Front Neurosci (2019) 13:1359. doi: 10.3389/fnins.2019.01359
52. Ma B, Mao Y, Chang L, et al. S-propargyl-cysteine prevents concanavalin a-induced immunological liver injury in mice. phar Biol (2022) 60(1):1169–76. doi: 10.1080/13880209.2022.2080234
53. Khattak S, Rauf MA, Khan H, et al. Hydrogen sulfide biology and its role in cancer. Molecules (2022) 27(11):3389. doi: 10.3390/molecules27113389
54. Kurokouchi S, Kato S. Influence of ion stimulated gas desorption from residual gas analyzer on partial pressure measurement. J Vacuum Sci Technol A: Vacuum Surfaces Films (2018) 19(6):2820–5.
55. Panthi S, Manandhar S, Gautam K. Hydrogen sulfide, nitric oxide, and neurodegenerative disorders. Trans Neurodegeneration (2018) 7(1):1–8. doi: 10.1186/s40035-018-0108-x
56. Cao Q, Li X, Zhang Q, et al. Big data analysis of manufacturing and preclinical studies of nanodrug-targeted delivery systems: A literature review. BioMed Res Inter (2022), 2:2022. doi: 10.1155/2022/1231446
57. Begara JC. Nitric oxide signalling in a CO2-enriched environment. J Exp Bot (2016) 67(3):560–1. doi: 10.1093/jxb/erw010
58. Fix S, Borden MA, Dayton PA. Therapeutic gas delivery via microbubbles and liposomes. J Controlled Release (2015) 209:139–49. doi: 10.1158/1078-0432.CCR-06-2443
59. Lv S, Li X, Zhao S, et al. The role of the signaling pathways involved in the protective effect of exogenous hydrogen sulfide on myocardial ischemia-reperfusion injury. Front Cell Dev Biol (2021) 12:723569. doi: 10.3389/fcell.2021.723569
60. Yizhun Z, Hu W, Wang R, et al. Sp1S-sulfhydration induced by hydrogen sulfide inhibits inflammation via HDAC6/MyD88/NFB signaling pathway in adjuvant-induced arthritis. Antioxidants (2022) 11(4):732. doi: 10.3390/antiox11040732
61. Masi A, Ascenzi P. H2S: a “double face” molecule in health and disease. Biofactors (2011) 39(2):186–96. doi: 10.1021/acsomega.2c02890
62. Gladwin MT, Raat J, Shiva S, et al. Nitrite as a vascular endocrine nitric oxide reservoir that contributes to hypoxic signaling, cytoprotection, and vasodilation. Am J Physiology-Heart Circulatory Physiol (2018) 291(5):2026–35. doi: 10.1152/ajpheart.00407.2006
Keywords: gas signaling molecules, endocrine, mechanism of action, pathogenesis, signalling molecules
Citation: Qi W, Man L, Suguro S, Zhao Y, Quan H, Huang C, Ma H, Guan H and Zhu Y (2022) Endocrine effects of three common gas signaling molecules in humans: A literature review. Front. Endocrinol. 13:1074638. doi: 10.3389/fendo.2022.1074638
Received: 19 October 2022; Accepted: 23 November 2022;
Published: 09 December 2022.
Edited by:
Yicheng Mao, Fudan University, ChinaReviewed by:
Guangzhu Cao, Kunming University of Science and Technology, ChinaCopyright © 2022 Qi, Man, Suguro, Zhao, Quan, Huang, Ma, Guan and Zhu. This is an open-access article distributed under the terms of the Creative Commons Attribution License (CC BY). The use, distribution or reproduction in other forums is permitted, provided the original author(s) and the copyright owner(s) are credited and that the original publication in this journal is cited, in accordance with accepted academic practice. No use, distribution or reproduction is permitted which does not comply with these terms.
*Correspondence: Yizhun Zhu, MTkwOTg1M2FwYTExMDA5QHN0dWRlbnQubXVzdC5lZHUubW8=
Disclaimer: All claims expressed in this article are solely those of the authors and do not necessarily represent those of their affiliated organizations, or those of the publisher, the editors and the reviewers. Any product that may be evaluated in this article or claim that may be made by its manufacturer is not guaranteed or endorsed by the publisher.
Research integrity at Frontiers
Learn more about the work of our research integrity team to safeguard the quality of each article we publish.