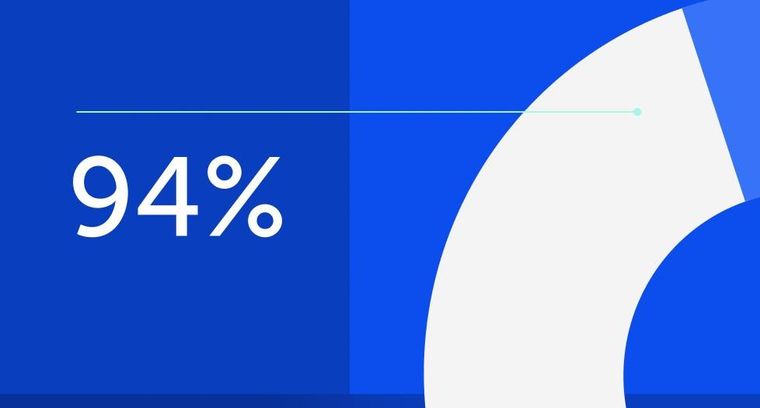
94% of researchers rate our articles as excellent or good
Learn more about the work of our research integrity team to safeguard the quality of each article we publish.
Find out more
ORIGINAL RESEARCH article
Front. Endocrinol., 09 January 2023
Sec. Neuroendocrine Science
Volume 13 - 2022 | https://doi.org/10.3389/fendo.2022.1051882
This article is part of the Research TopicThe HPA Axis and Aging: Individual Features, Age-related PathologyView all 5 articles
The hypothalamic-pituitary-adrenal (HPA) axis is a key adaptive neuroendocrine system, dysfunction of which plays an important role in the increasing incidence of stress-dependent age-related pathology. Among the environmental factors effecting increase age-related diseases, great importance is given to disturbances of the light-dark schedule, particularly with increased illumination at night. While disruption of the light-dark schedule has long been recognized as a powerful behavioral stressor, little is known regarding stress reactivity of the HPA under constant light (CL) conditions, especially with aging and depending on the features of stress behavior. The purpose of this investigation was to study the age-related and individual features of the HPA axis response to acute stress exposure (ASE) under chronic CL in nonhuman primates that are known to differ in behavioral responsiveness to stress. Young and old female rhesus monkeys (with control standard behavior or anxiety and depression-like behavior) were exposed to CL (24 h light/day, 330-400 lux for 4 to 8 weeks). Control young and old monkeys were exposed to standard lighting (SL) with natural light during the day and darkness at night. All animals were subjected to ASE (restriction of mobility for 2 hours), functional tests with corticotrophin-releasing hormone and arginine-vasopressin, and study of circadian rhythms of cortisol and pineal melatonin secretion. For the first time an inhibitory effect of CL on the reaction of the adrenal cortex to ASE was revealed in all individuals, regardless of age and preexisting behavior stress reactivity, the mechanisms of which were age-dependent: due to inhibition of the pituitary ACTH secretion in young animals and mainly not affecting the ACTH secretion in old individuals. There were no significant changes in melatonin secretion both in young and old animals. The observed CL inhibition of adrenal cortical reactivity to ASE may be useful to correct increased vulnerability to ASE observed in individuals with preexisting anxiety and depression-like stress behaviors. On the other hand, the CL induced decrease in adrenal stress reactivity of behaviorally normal animals suggests a potential risk of reducing the adaptive capacity of the organism under conditions of continuous light exposure.
The hypothalamic-pituitary-adrenal (HPA) axis is a key neuroendocrine system that underlies the body’s adequate response to environmental stressors. However, its dysfunction, accompanied by disturbances in the production of glucocorticoid hormones, contributes to the development of various stress-dependent diseases, including age-related, for example mental, metabolic, cognitive, cardiovascular, neurodegenerative, etc., the incidence of which is increasing (1–6). Increasing incidence of age-related diseases is largely due to rise of the proportion of aging individuals and life expectancy and also due to expansion of the range of stressful influences in the modern world (urbanization, environmental problems, local military conflicts, increased mental stress at work, etc.). Among the environmental factors effecting increase age-related diseases, great importance is given to disturbances of the light-dark schedule, particularly with increased illumination at night (7–14). Technological advances have transformed modern societies increasing the percentage of time that individuals spend indoors, where the illumination is much lower compared to bright sunlight or even cloudy daylight. At the same time, the illumination on the streets at night has sharply increased due to high levels of artificial light (11, 12, 15). Light pollution, irregular work and activity schedules, among other factors, can produce both transient and chronic disruption various physiological processes such as circadian rhythms of endocrine secretion and behavior, cognition, sleep-wake rhythm, alertness and performance, cardiovascular system, sympathetic activity, metabolic dysregulation, etc., and contribute to the development of age-related stress-dependent pathology or exacerbate it (7, 9, 10, 12, 15–19).
An extensive scientific material has been accumulated, according to which the basis of the negative impact of the light-dark schedule disturbances on physiological processes and health are damages in the circadian system. Thus, numerous literature data indicate the inhibitory effects of night lighting on pineal secretion of melatonin (MEL) (12, 13, 17, 20–23). A lot of attention has been paid to the functioning of the circadian system for the HPA axis in the normal conditions and under constant light (CL) but little is known regarding stress reactivity of the HPA axis in CL conditions.
So, it is assumed that the circadian regulation of glucocorticoid secretion is one of the best examples of bi-directional communication between the suprachiasmatic nucleus (SCN) of the hypothalamus, i.e. a central light-sensitive “master clock” and peripheral oscillators. SCN-mediated direct and indirect (through the subparaventricular nucleus and the dorsomedial hypothalamus) activation of corticotrophin-releasing hormone (CRH) and arginine-vasopressin (AVP) secretion from the hypothalamic paraventricular nucleus (PVN) controls the rhythmic release of adrenocorticotropic hormone (ACTH) from the pituitary gland, which in turn regulates the rhythmic production of glucocorticoids in the adrenal cortex (24–28). This pathway appears to be the main way that switches the light signal to the HPA axis, providing the basis for the circadian release of glucocorticoids with their zenith concentrations early in the morning and their nadir concentrations at night for humans and primates (25, 29, 30). In addition to this pathway, another indirect, extrahypophyseal autonomic pathway has also been identified. With this pathway the SCN transmits photic information to the adrenal medulla and from there through catecholamines to the adrenal cortex (25, 31, 32). Through this autonomic pathway, the SCN appears to predominantly modulate the sensitivity of the adrenal cortex to ACTH in a time-of-day manner (30, 33, 34), in particular by regulating the activity of the peripheral circadian clocks localized in the adrenal cortex (25, 32, 35).
In addition to controlling the circadian secretion of glucocorticoids with the help of the HPA axis and the extrahypophyseal nerve pathway, a number of authors assign certain importance in its regulation to the pineal hormone MEL (36, 37).
The publications on the functioning of the circadian system for the HPA axis under disruption of the light-dark schedule were carried out mainly on rodents that are nocturnal and differ significantly from humans and primates both in the functioning of the HPA axis and in the sensitivity of the optical system to light. This is probably why the data on the effect of CL on the function of the HPA axis are rather contradictory. Most studies point to disruption of the circadian rhythm of glucocorticoid secretion under CL conditions, accompanied mainly by an increase in their blood concentration in rats, mice, and hamsters (19, 38–45) At the same time, in single studies, along with glucocorticoids, an increase in the concentration of ACTH (40) or the absence of alterations in ACTH levels (32, 46) was noted. In contrast to previous works, a small number of studies have found no change (47, 48) or decrease in circulating glucocorticoids in response to CL in rodents (43, 49, 50). In most clinical studies, either no changes were noted in the levels of cortisol (CORT) in response to nighttime light exposure (51–55) or there has been its decrease (56, 57). The increase was observed only for a short time when applying pulses of bright light in the morning hours (52, 53) or as part of complex changes depending on the duration of light exposure (12). Effects of CL on HPA axis function largely appears to depend on the timing, the intensity, and possibly the duration of the light stimulus, as well as the spectral characteristics of light.
Unfortunately, we did not find any data on the features of HPA axis response to stress exposure under CL in the available literature, with the exception of the study that investigated the effect of an artificial light at night on the function of the adrenal cortex and the cardiovascular system in security guards working on the night shift (58). This study revealed an increase in the concentration of CORT and physiological prevalence of the vagal tone on the cardiocirculatory activity before and after the work shifts. We have previously presented data on the inhibitory effect of CL on the response of the HPA axis to the administration of AVP, which, to a certain extent, mimic the stress effect in monkeys (59). Unfortunately, all these investigations were performed on young individuals and did not affect the aging process, as well as individual differences. At the same time, the problem elucidation of stress reactivity of the HPA axis under disruption of the light-dark schedule remains relevant, especially during aging and depending on the features of stress behavior. Its resolve could be a source of information useful for the development of new approaches to prevent the increased vulnerability to stress and stress-dependent pathology noted in individuals with anxiety and depression-like stress behaviors (3, 6, 60–63). On the other hand, its solution is important to understand how chronic constant lighting affects the ability to adapt to stressful environmental factors.
The purpose of this study was to investigate the age-related and individual features of the HPA axis response to acute stress exposure in CL conditions, as well as the mechanisms underlying them, on the model of rhesus monkey females with control standard behavior and with depression-like and anxiety-like behavior using a two-hour restriction of their mobility (restraint, non-rigid immobilization) in metabolic cages as an acute psycho-emotional stress exposure (ASE), functional tests with CRH and AVP, and the study of circadian rhythms of plasma CORT and MEL in the basal period and on the background of CL. The planning of functional tests with the administration of CRH and AVP was carried out in order to assess the role of each of these neuropeptides in the mechanism of the identified age-related disorders in the HPA axis response to ASE in the conditions of chronic CL. As is well known and noted above (see page 2), CRH and AVP are secreted by neuroendocrine neurons of the hypothalamic PVN into the pituitary portal system. These neurons receive a large number of diverse neural signals from various parts of the brain, which contribute to the endocrine response to stress exposure. CRH and AVP interact with specific receptors on corticotrophs of the anterior pituitary gland (CRH with CRHR1 and AVP with AVP1b, respectively) to induce ACTH secretion into the general circulation. ACTH, after binding to type 2 melanocortin receptor in the adrenal cortex, activates a signaling cascade that usually leads to de novo biosynthesis and release of glucocorticoids (predominantly CORT in humans and nonhuman primates and corticosterone in most rodents) (6, 30). In addition to stress, as noted above (see page 2) CRH and AVP play an important role in the rhythmic release of ACTH and CORT receiving information from the SCN about the environment illumination (28, 30). The administration of CRH or AVP, as in the case of ASE, is also accompanied by an increase in the secretion of ACTH and CORT in young and old monkeys (6). Moreover, significant differences in ACTH response to CRH and AVP tests were found in nonhuman primates differing in stress behavior, with a higher rise in ACTH secretion in individuals with anxiety and depression-like behavior, similar to intergroup differences in ACTH response to ASE in old female rhesus monkeys, and in contrast to the absence of between-group differences in ACTH response to ASE in young monkeys (6, 61).
We demonstrated for the first time an inhibitory effect of CL on the response of the adrenal cortex to acute stress exposure in all individuals, regardless of age and behavioral patterns, the mechanisms of which were age-dependent: due to inhibition of pituitary secretion of ACTH in young animals and mainly without affecting it, in old individuals.
Thirty five young adult (5–8 years) and 23 old (21–33 years) healthy female rhesus monkeys (Macaca mulatta) were used in the experiments. The monkeys originated from the Adler monkey colony (Research Institute of Medical Primatology, Sochi-Adler, Russia). The animals usually were housed in open enclosures (housing 10-15 or 40-50 individuals of various ages, including newborns and elderly animals) or cages designed for group housing (3-5 individuals). During the experiment, the animals were moved into individual metabolic cages in a separate room with narrow windows, natural illumination (usually from 06.00 h to 18.00 h) and controlled temperature (25-28°C). Experimental and control animals were kept in different rooms. The experiments were carried out in summer time (June-August) when ovarian cycles are not typical for this species of laboratory primates. The animals were fed pellets prepared according to the technique of Altromin (Lage). The pellet diet was complemented with fresh vegetables, fruits and water ad libitum. Prior to the experiments, the animals were adapted to the conditions of separate housing and to the procedure of blood sampling for 4 weeks. During the adaptation period, the lighting in the rooms with experimental and control animals usually was about 60-130 lux. However, artificial lighting was additionally turned on during the period of room cleaning and experimental procedures on cloudy days for a short period of time (see Methods section below).
All procedures were obtained approval of the Ethical Committee of the Research Institute of Medical Primatology (Sochi), and all operations in this study were conducted in accordance with the guidelines of the European Convention for the Protection of the Vertebrate Animals Used for Experimental and Other Scientific Purposes” (Strasbourg, 18.III.1986), Directive 2010/63/EU of the European Parliament and the Council of 22 September 2010 (on the protection of animals used for scientific purposes).
A more detailed description of the conditions for keeping the monkeys in the nursery, the assessment of their health and behavior, as well as the preparing animals for experiments were carried out according to standard methods, as described earlier (64).
The data presented in the article were obtained as a result of physiological experiments (acute psycho-emotional stress modeling, experiment with administration of CRH, and experiment with administration of AVP) for 3 years - from 2019 to 2021. In addition, in all animals, the features of the effect of CL on the circadian rhythms of CORT and MEL were studied every year. In the experiments of each year, experimental and control groups of animals were distinguished, as well as the basal period and the actual experimental period, i.e. period of CL and experimental period for control animals with normal light-dark schedule (standard light, SL).
The animals’ behavior was recorded while they were housed in the metabolic cages, both during the period of adaptation, and throughout experimentation. Depending on behavioral features, both young and old animals were divided into two groups: with healthy active adaptive behavior (standard behavior, SB) and with maladaptive depression-like and anxiety-like behavior (DAB). In 2019, 11 animals with DAB and 6 animals with SB were used in experiments; in 2020 - 12 animals with DAB and 10 animals with SB; in 2021 - 8 animals with DAB and 11 animals with SB.
Analysis of the life history of the experimental animals revealed that 2 animals with DAB (№ 40176 - young, 34347- old) and 1 control old animal (№35721) were exposed to severe stress in early childhood (maternal deprivation due to maternal death in the period from 1 month to 9 months) and growing up separately from adult individuals, in the so-called “nursery” in individual cages until age 1.2 years, and then were kept in the cage designed for group housing, together with other immature animals, deprived of mother, and previously lived in the “nursery.”
Twenty two female rhesus monkeys were used in the experiments. The group of young experimental animals included 8 individuals (7.5 ± 0.5 years, 6.0 ± 0.5 years); the group of old experimental animals also included 8 individuals (26.3 ± 1.6 years, 6.1 ± 0.5 kg). The group of control animals included 6 young monkeys (8.0 ± 0.45 years, 6.0 ± 0.2 kg). In this experiment, 8 experimental animals with DAB (young – 5, old – 3), 8 experimental animals with SB (young – 3, old – 5), 4 control animals with DAB, and 2 control animals with SB were used.
After adaptation period, all animals were subjected to ASE: moderate restraint (non-rigid immobilization, restriction of mobility) in a metabolic cage for two hours, as described earlier (61). Restraint was achieved by using a conventional squeeze board to press the animal to the front wall of the metabolic cage. The body and extremities of the animal were not tightly immobilized. Animals were subjected to the stressor at 15.00 h. Blood samples were taken before restraint (0) and 15, 30, 60, and 120 min during application of the stressor, and at 240 min, i.e., 2 h after termination of the stressor exposure.
The adaptation and basal periods were followed by the actual experimental period, during which artificial light (330-400 lux) was turned on in the room with young and old animals of the experimental groups during the day and night continuously for 8 weeks (period of CL). At the same time in the room with young and old animals of the control groups, the light remained the same, i.e. standard (about 60-130 lux) with predominantly natural light during the day and darkness at night (period of SL). It should be noted that during the daytime, the experimental animals were additionally exposed to natural light, similar to that in the room with the control group of animals, that is, 60-130 lux. For chronic CL we used white light-emitting diode lamps with a wavelength of 400-838 nm and with a predominant wavelength range of 513-700 nm (green, yellow, orange, red colors) intended for residential, office, and commercial premises (LED lamp “Navigator” 71 302 NLL-G-T8-18-230-4K-G13, Limited Liability Company “TM Navigator”, Moscow, Russia; made in China – Xiamen Neex Optical Electronic Technology CO., LTD). After 7 weeks of the experimental period all experimental and control animals were exposed ASE in the same way as in the basal period.
Nineteen female rhesus monkeys were used in the experiments, including 6 young experimental (8.0 ± 0.45 years, 5.96 ± 0.24 kg), 5 young control (6.0 ± 0.37 years, 5.0 ± 0.4 kg), 5 old experimental (27.6 ± 0.4 kg) 2.5 years, 5.0 ± 0.3 kg) and 3 old controls (18.0 ± 0 years, 6.7 ± 0.8 kg). In this experiment, 5 experimental animals with DAB (young – 4, old – 1), 6 experimental animals with SB (young – 2, old – 4), 5 control young animals (1 - with DAB and 4 with SB) and 3 control old animals (1 - with DAB and 2 with SB) were used. All experimental and control animals twice in basal period and after 8 weeks, respectively CL period and SL period were injected with CRH in 0.9% NaCl (Сorticotropin Releasing Factor of human, rat, Sigma; intravenously in a dose of 1 μg/kg body weight). Blood samples were taken before (0 min) and 15, 30, 60, 120, and 240 min after the drug administration. Animals were subjected to the injection of CRH at 15.00 h.
Seventeen female rhesus monkeys were used in the experiments, including 5 young experimental (7.6 ± 0.6 years, 6.2 ± 0.6 kg), 5 young control (6.6 ± 0.4 years, 5.7 ± 0.4 kg), 4 old experimental (24.2 ± 1.7 years, 6.7 ± 0.8 kg) and 3 old controls (29.3 ± 2.7 years, 5.7 ± 0.7 kg). In the experiment, 11 animals with DAB and 6 animals with SB were used. All experimental and control animals twice in basal period and after 6 weeks, respectively CL period and SL period, were injected with AVP in 0.9% NaCl (Arg8-Vasopressin, MP Biomedicals, LLC, France) intravenously in a dose of 1 μg/kg body weight. Blood samples were taken before (0 min) and 15, 30, 60, 120, and 240 min after the drug administration. Animals were subjected to the injection of AVP at 15.00 h.
To assess the circadian rhythms of CORT and MEL, blood samples were taken from 12 young and 10 old experimental animals, 10 young and 6 old control animals in the basal period and in the CL or SL period (5 weeks) at 09.00 h, 15.00 h, and 22.00 h.
All blood samples were taken from cubital vein of the animals. Blood samples were collected in chilled tubes with EDTA (10.0 mg per 1 ml of blood) as the anticoagulant. At each time point 1.0-2.0 ml of blood was taken. Blood samples were immediately centrifuged at 2000g at +4°C, plasma stored at −70°C for later analysis. Plasma levels of CORT, ACTH, and MEL were measured by immunoenzyme assay using standard hormone kits (AlkorBio, Russia for total CORT; Biomerica Inc., USA for ACTH; IBL international GmbH, Germany for MEL). The ELISA method for the determination of MEL included a stage of preliminary purification on columns with a sorbent. The sensitivity of the assay for CORT was 10.0 nmol/l. The intra-assay and inter-assay variation coefficients (C.V.) for CORT did not exceed 10 and 15%, respectively. The sensitivity of the assay for ACTH was 0.22 pg/ml. The intra-assay and inter-assay variation coefficients for ACTH did not exceed 8 and 10%, respectively. The sensitivity of the assay for MEL was 1.6 pg/ml. The intra-assay and inter-assay variation coefficients for MEL did not exceed 12 and 17%, respectively.
The experimental values are presented in tables and figures as means ± S.E.M. The statistical comparisons of hormone level differences at various time intervals after the start of exposure in comparison with the initial levels of the same hormones and between the corresponding values of hormones under CL and SL and in the basal period and also the age and behavioral group differences were performed using one- and two-way analysis of variances (ANOVA) including post hoc Tukey’s honest significant difference test for paired comparisons (Statistics 10 software package, Stat Soft. Inc., USA). The amplitude of the circadian rhythm of CORT was calculated as the difference between the hormone concentrations at 09.00 and 22.00 h, and the amplitude of the circadian rhythm of MEL was calculated as the difference between its concentrations at 22.00 (or 21.00) and 09.00 h. The areas under curves representing hormone concentration as a function of time (0-240 min, response area) were calculated using the trapezium formula.
Dynamics of ACTH and CORT levels in young female rhesus monkeys (without division by type of behavior) in response to acute stress exposure (ASE) under basal conditions and after 7 weeks of exposure to CL indicates the inhibitory effect of CL on the magnitude of the rise in ACTH and CORT concentrations (Figures 1A, B). In addition, CL reduced the initial levels of СORT (Figure 1B). The areas under curves representing dynamics of ACTH and CORT in response to ASE on the background of CL were also significantly lower than in basal period (Figures 2A, B). At the same time, there were no significant changes in both the areas of response of ACTH and CORT concentrations (see Figures 2C, D) and the dynamics of their response (Figures 3A, B) to ASE in animals of the control group in the basal period and on the background of standard lighting. A decrease in the magnitude of the rise in ACTH in response to ASE under conditions of CL was statistically significant for both animals with DAB and SB (Table 1). The decrease in the magnitude of the rise in the level of СORT on the background of CL was characteristic of animals with DAB (Table 1) and was also observed in animals with SB. Thus, the response area of CORT in three experimental animals with SB in the basal period was 227940, 204960 and 191700 nmol/l×min but under CL it was 214200, 130350 and 124200 nmol/l×min, respectively.
Figure 1 Dynamics of ACTH and CORT levels in peripheral blood plasma in young experimental female rhesus monkeys in the basal period (BP) and on the background of chronic constant lighting (CL) in response to ASE (A, B), administration of CRH (C: -Δ- the animals with SB and -o- with DAB in the basal period; …o… animals with SB and □ with DAB at CL period) (D), and AVP (E, F) (mean ± S.E.M.). ●p <0.05; ●●p<0.01; ●●●p<0.001 - vs. relative values in the basal period.
Figure 2 The areas under the concentration curves of ACTH and CORT in response to ASE, administration of CRH (including animals with SB and DAB for ACTH) and AVP in young experimental (A) ACTH, (B) CORT and control (C) ACTH, (D) CORT female rhesus monkeys in basal period and on the background of CL or standard lighting (SL) (mean ± S.E.M.). ●p <0.05; ●●p<0.01 - vs. the values in basal period; * p <0.05; ** p<0.01 - vs. the relative values in the animals with DAB.
Figure 3 Dynamics of ACTH and CORT levels in peripheral blood plasma in young control female rhesus monkeys in the basal period (BP) and on the background of standard lighting (SL) in response to ASE (A, B), administration of CRH (C, D), and AVP (E, F) (mean ± S.E.M.).
Table 1 Dynamics of ACTH and CORT concentration in peripheral blood plasma and the area under the curve of ACTH and CORT response (0-240 min) to ASE in young experimental female rhesus monkeys with DAB and SB in the basal period and under CL (mean ± S.E.M.).
Thus, the obtained experimental data indicate the inhibitory effect of CL on the response of the HPA axis to ASE by suppressing both ACTH and CORT secretion in all experimental young animals, regardless of behavioral characteristics. In order to study the possible mechanisms of the inhibitory effect of CL on the HPA stress reactivity, we performed functional tests with the administration of CRH and AVP, the main central drivers of the HPA axis, and also evaluated the effect of CL on the circadian rhythm of the activity of the adrenal cortex and the pineal gland.
It was found that in the group of experimental animals (without subdividing them according to the type of behavior), the concentration of ACTH in response to CRH administration on the background of CL did not statistically significantly differ from the corresponding values in the same animals under basal period. However, in the group there was a large individual variability in the response of ACTH to CRH, both on the background of CL and under basal period (Table 2). At the same time, a certain pattern was revealed, according to which, according to the magnitude of the rise in the level of ACTH to the administration of CRH, animals of the experimental group could be divided into 2 subgroups: (1st) with a high response (3 individuals with DAB) and (2nd) with a low response (3 individuals with SB) (Figure 1C). In animals with DAB, the concentration of ACTH on the background of CL 120 and 240 min after the administration of CRH was statistically significantly higher compared to similar values in the basal period. At the same time, the magnitude of the increase in the level of ACTH in animals with SB on the background of CL remained the same as in the basal period (Figure 1C). The response area of ACTH concentration in animals of the 2nd subgroup was significantly lower compared with the corresponding values in animals of the 1st subgroup both in the basal period and on the background of CL (Figure 2A). In control animals, there were no statistically significant changes in the dynamics of ACTH concentration and in response areas to the test with CRH on the background of SL (Figures 2C and 3C).
Table 2 Dynamics of ACTH concentration (pg/ml) in peripheral blood plasma and the area under the curve of ACTH response (0-240 min) to CRH administration in young experimental female rhesus monkeys in the basal period and under CL (mean ± S.E.M.).
An analysis of the dynamics of the CORT level in female rhesus monkeys in response to the administration of CRH under basal conditions and on the background of CL revealed no significant differences in general, except for the CORT concentration 15 min after the administration of CRH. It was statistically significantly lower on the background of CL than in the basal period (Figure 1D). There were no statistically significant differences in the areas of the response of CORT on the background of CL and in the basal period (Figure 2B). In addition, there were no essential differences in the dynamics of CORT concentration and the CORT response area on the background of CL and in the basal conditions in control animals (Figures 2D and 3D).
The dynamics of ACTH level in experimental and control young female rhesus monkeys in response to the administration of AVP in the basal conditions and on the background of CL and SL is shown in Figures 1E and 3E. As can be seen, the CL led to a pronounced decrease in the magnitude of the rise in ACTH concentration in young primates before the start of AVP administration (0 min), as well as 15 and 240 min after its administration (Figure 1E). In addition, in response to the administration of AVP, a statistically significant decrease was observed in the ACTH response area (Figure 2A). At the same time, in control animals, in response to AVP, there were no statistically significant differences in the ACTH response in the basal period and on the background of SL (Figures 2C and 3E).
The magnitude of the concentration of CORT rise in the test with AVP in the animals on the background of CL 60 and 120 min after administration of the drug as well as the area of the CORT response, were significantly lower than in the basal period (Figures 1F and 2B). At the same time, the dynamics of CORT concentration and the response area of CORT in control animals in response to the injection of AVP practically did not differ from similar values in the basal period (Figures 2D and 3F).
Thus, the reaction of the HPA axis to the test with AVP on the background of CL was significantly lower compared to the basal period and was similar to the response of the HPA axis to ASE. It should be noted that in this experiment the majority of experimental (4 individuals out of 5) and control (3 individuals out 5) animals belonged to the DAB type. Therefore, all of the above in the experiment with AVP primarily applies to animals with DAB.
The blood plasma CORT at different times of the day in experimental female rhesus monkeys (combined group of animals with SB and DAB) showed a pronounced decrease at 15.00 compared to similar values in the basal period (Figure 4A). In addition, in experimental animals, an increase in the level of CORT at 22.00 was noted with a corresponding decrease in the amplitude of the circadian rhythm (Figure 4A). In control animals, there was a tendency to a decrease in the level of CORT at 15.00 and an increase at 22.00 on the background of SL, which, however, were accompanied by a statistically significant decrease in the amplitude of the circadian rhythm (Figure 4B). Apparently, CL is not a specific factor that induces an increase in the concentration of CORT at night, but its presence enhances this process.
Figure 4 The concentration of CORT and MEL at different times of the day and the amplitude of their circadian rhythms in behaviorally combined groups of young experimental (n=12; A, C) and control (n=10; B, D) female rhesus monkeys in the basal conditions and under the CL or SL conditions (mean ± S.E.M.). ● p<0.05; ●● р< 0.01 vs. the values in basal period.
The plasma MEL at different times of the day in experimental and control female rhesus monkeys (combined groups of animals with SB and DAB) demonstrated no significant changes at 09.00, 15.00 and 22.00, as well as in the amplitude of its circadian rhythm on the background of CL, as well as on the background of SL (Figures 4С, D).
The CL had no significant effect on the dynamics of ACTH concentration and the response area of ACTH to ASE in the behaviorally combined group of old animals (Figures 5A and 6A) and also in the animals with SB (Table 3). However, a slight decrease in the concentration of ACTH was observed in two animals with DAB (Table 3). At the same time, in contrast to ACTH, the magnitude of the rise in CORT level in response to ASE on the background of CL, including the response area of CORT, was significantly lower than in the basal period (Figures 5B and 6B).
Figure 5 Dynamics of ACTH and CORT levels in peripheral blood plasma in old experimental female rhesus monkeys in the basal period (BP) and on the background of chronic constant lighting (CL) in response to ASE (A, B), administration of CRH (C, D), and AVP (E, F) (mean ± S.E.M.). ●p <0.05; ●●p<0.01 - vs. relative values in the basal period.
Figure 6 The areas under the concentration curves of ACTH (A) and CORT (B) in response to ASE, administration of CRH and AVP in old experimental female rhesus monkeys in basal period and on the background of CL (mean ± S.E.M.) ●●p<0.01 - vs. the values in basal period.
Table 3 Dynamics of ACTH concentration (pg/ml) in peripheral blood plasma and the area under the curve of ACTH response (0-240 min) to ASE in old female rhesus monkeys with SB and DAB in the basal period and under CL (mean ± S.E.M.).
Thus, in old animals, in contrast to young animals, there was no significant decrease in the ACTH response to ASE induced by CL. At the same time, CL induced a significant decrease in both the basal level of CORT and the magnitude of its rise in response to ASE for all animals, regardless of behavior. Apparently, CL in old animals inhibits the magnitude of the rise in the concentration of CORT in response to ASE, mainly without affecting the secretion of ACTH. In order to elucidate the mechanisms underlying the inhibitory effect of CL on the stress reactivity of the adrenal cortex in old female rhesus monkeys, as in the case of young animals, we studied the response of ACTH and CORT to tests with the administration of CRH and AVP, as well as the circadian rhythms of CORT and MEL in basal conditions and on the background of CL.
As shown by a functional test with CRH, in old experimental female rhesus monkeys on the background of CL, there were no significant changes in the magnitude of the rise in ACTH concentration compared to the basal period, except for the point of 240 min, where a statistically significant increase in the level of ACTH was noted (Figure 5C). There were no statistically significant changes in the ACTH response area (Figure 6А). In control animals, there were also no significant changes in the ACTH response to the test with CRH on the background of standard illumination (Figures 7А and 8A). The response of CORT to the administration of CRH on the background of CL in old female rhesus monkeys, as in the case of young females, did not undergo significant changes, except for the significantly lower values of the CORT level 15 min after the administration of CRH, compared to the response of CORT in basal period (Figure 5D). The response area of CORT to the injection of CRH did not undergo statistically significant changes in experimental animals (Figure 6B). There were no significant changes in the dynamics of the plasma CORT and the response area to the administration of CRH in the control group of animals on the background of SL (Figures 7В and 8B).
Figure 7 Dynamics of ACTH and CORT levels in peripheral blood plasma in old control female rhesus monkeys in the basal period (BP) and on the background of standard lighting (SL) in response to administration of CRH (A, B) and AVP (C, D) (mean ± S.E.M).
Figure 8 The areas under the concentration curves of ACTH and CORT in response to administration of CRH (A, B) and AVP (A, B) in old control female rhesus monkeys in basal period and on the background of standard lighting (SL) (mean ± S.E.M.).
In the experiment, 5 old female rhesus monkeys were used (3 individuals with DAB and 2 individuals with SB). In contrast to young animals, the CL did not lead to a significant decrease in the magnitude of the rise in ACTH concentration and the area response to the administration of AVP in old female rhesus monkeys (Figures 5E and 6A). There were no significant changes in the ACTH response to the administration of AVP in control old animals (Figures 7С and 8A). At the same time, it should be noted that polymorphism was observed in the reaction of ACTH to the administration of AVP under CL. So, in 3 old experimental animals, as well as in young female rhesus monkeys, a decrease in the magnitude of the increase in ACTH concentration was observed in response to the administration of AVP. Thus, the response areas of ACTH concentration in the basal period and under CL were respectively: 20880, 20784 and 5602 pg/ml×min and 4512, 10728 and 3912 pg/ml×min. Similar to the dynamics of the level of ACTH, the concentration of CORT in the peripheral blood plasma in old experimental female rhesus monkeys in response to the AVP administration under CL did not undergo significant changes compared to the basal period (Figures 5F and 6B). There were no significant changes in the response of CORT to AVP in control animals on the background of SL (Figures 7D and 8B). It should be noted that in old animals with a pronounced decrease in the magnitude of the rise in plasma ACTH on the background of CL, there was also a slight decrease in the level of CORT. Thus, the response area of ACTH for 240 min in the animals under discussion was 225600, 203520, 214800 nmol/l×min, respectively, in the basal period and 199440, 182160 and 189600 nmol/l×min under CL.
As in young female rhesus monkeys, the concentration of CORT in old animals on the background of CL was statistically significantly lower compared to the basal period at 15.00 and was characterized by a tendency to increase at 22.00 (Figure 9A). In control animals, significant changes in the concentration of CORT at different times of the day on the background of SL were not detected (Figure 9B). Significant changes in the circadian rhythm of MEL on the background of CL in old female rhesus monkeys, like young females, were not revealed either in comparison with the basal period or in comparison with control animals (Figures 9C, D).
Figure 9 The plasma concentration of CORT and MEL at different times of the day and the amplitude of their circadian rhythms in behaviorally combined groups of old experimental (n=10; A, C) and control (n=6; B, D) female rhesus monkeys in the basal conditions and under the CL or SL conditions (mean ± S.E.M.). ●● р< 0.01 vs. the values in basal period.
As a result of the research, we demonstrated for the first time an inhibitory effect of CL on the response of the adrenal cortex to ASE applied in the afternoon (start at 15.00) in young mature and old female rhesus monkeys. Inhibition of the function of the adrenal cortex under CL was characteristic of both for animals with DAB and with control SB. Although the inhibition of the function of the adrenal cortex was detected in all the examined animals, regardless of age and behavior, the mechanism of the inhibitory effect of CL on the stress reactivity of the adrenal cortex, apparently, is age-dependent. So, young mature female rhesus monkeys were characterized by an inhibitory effect of CL both on the magnitude of the rise in CORT secretion and on the magnitude of the rise in ACTH levels and its absence in control animals with normal light-dark schedule. At the same time, a decrease in the magnitude of the rise in the level of ACTH preceded a decrease in the magnitude of the rise in plasma CORT (see Figures 1-3). Therefore, it can be considered that the inhibitory effect of CL on the secretion of CORT in young monkeys is due to the inhibition of ACTH secretion.
Apparently, the inhibitory effect of CL on stress activation of ACTH secretion in young animals is conditioned by the SCN-mediated inhibition of activation of AVP secretion from the hypothalamic PVN that controls the release of ACTH from the pituitary gland. This is indicated, on the one hand, by the literature data on the existence of such a neuronal pathway, through which information about the illumination of the environment is transmitted from the SCN to the adrenal cortex through the activation of CRH/AVP containing neurons of the medial parvocellular PVN and modulation of the circadian secretion of CRH and AVP, as well as pituitary ACTH and glucocorticoids by the adrenal cortex (24, 26, 28, 30). On the other hand, the inhibitory effect of CL on stress activation of ACTH secretion is confirmed by our results on the test with AVP, which revealed an inhibitory effect of CL on the magnitude of the rise in ACTH secretion and its absence in control animals with normal light-dark schedule (see Figures 1-3). CRH, apparently, does not play an important role in the mechanism of inhibition of HPA axis stress reactivity by CL, since the test with CRH not only did not reveal a decrease in the magnitude of the rise in ACTH levels in the behaviorally combined group of young animals (see Table 2), but even stimulated a significant elevated ACTH levels in animals with DAB (see Figures 1 and 2). It should be noted that earlier, under conditions of normal light-dark schedule we also noted a higher ACTH response to the test with CRH in young animals with DAB than in animals with SB (6). In addition, a higher rise in ACTH levels in response to CRH administration was characteristic of animals with DAВ compared with animals with SB in the basal period in our experiment (see Figures 1 and 2).
The absence of significant changes in the dynamics of the rise of the CORT level in response to the injection of CRH on the background of CL compared with the basal period in most of the studied time intervals, as well as in the area of the CORT response (see Figures 1D and 2B) along with a significantly higher rise ACTH secretion in DAB animals in CL conditions (see Figure 1C) appears to be due to the inhibitory effect of CL on sensitivity of the adrenal cortex to ACTH. Indeed, a number of authors have shown that SCN, which receives light/dark information from the environment, has a direct effect on the regulation of glucociorticoid circadian rhythms mediated by neural projections into the hypothalamic PVN. PVN, in turn, switches the light signal to the HPA axis, providing a basis for diurnal fluctuations of glucocorticoids with their peak concentrations early in the morning and their nadir levels at night for humans and nonhuman primates (15, 25, 29, 30, 65). In addition, SCN appears to modulate the sensitivity of the adrenal cortex to ACTH in a time-of-day manner, possibly mediated by an extrahypophysial autonomic pathway using peripheral circadian clocks localized in the adrenal cortex (25, 32, 35).
Available literature data on the effect of CL on CRH are rare. But they indicate a mismatch in the rhythms of the concentration of CRH in the hypothalamus or CRH mRNA expression in the PVN, ACTH and corticosterone in the blood plasma (66, 67). Apparently, the classical scheme of regulation of the HPA axis function, in particular under stress exposure, is disturbed in conditions of CL, and the regulation of glucocorticoid secretion using the extrahypophyseal autonomous pathway becomes important, accompanied by a disorder of the sensitivity of adrenal corticocytes to ACTH, as well as the sensitivity of pituitary corticotrophs to AVP, which can lead to the altered response of the adrenals to stress exposure.
In old animals an inhibitory effect of CL on the magnitude of the CORT level rise in response to ASE was noted mainly in the absence of corresponding changes in the rise of plasma ACTH (see Figures 5, 6). Apparently, in old animals, the inhibitory effect of CL on stress reactivity of the adrenal cortex is due to a decrease in the sensitivity of the adrenal cortex to ACTH, which is modulated by indirect extrahypophyseal autonomic pathway from the SCN to the adrenal cortex in a time-of-day manner (25, 30–35). This conclusion is also supported by the results of tests with CRH and AVP, which did not reveal statistically significant inhibitory effects of CL on the secretion of ACTH and CORT in old animals (Figures 5 and 6). In addition, in old animals under CL conditions, a more pronounced circadian decrease in the concentration of CORT at 15.00 was revealed than in the basal period, that is, the time of the onset of stress exposure (see Figure 9).
However, it should be noted that in some old animals under chronic CL there was a mild decrease in the magnitude of the rise in ACTH concentration in response to ASE and AVP administration along with a decrease in the magnitude of the rise in the CORT level (see Results for old monkeys). In this regard, it can be assumed that although in old animals CL inhibits mainly a sensitivity of the adrenal cortex to ACTH via the extrahypophyseal autonomic tract, in some individuals, a decrease in the secretion of CORT in response to ASE observed due to inhibition of ACTH secretion, that is, similar to that in young animals.
It should be noted that, although in the groups of young and old female rhesus monkeys, in response to the injection of CRH, there was no decrease in ACTH (and even an increase in young animals with DAB), and no decrease in the response area of CORT and its levels in most of the studied intervals was observed, there was a statistically significant decrease in the concentration of CORT 15 min after the administration of CRH in all animals (see Figures 1 and 5). Most likely, exogenous CRH on the background of CL can briefly decrease the sensitivity of the adrenal glands to ACTH in young animals and the adrenals to ACTH or the splanchnic nerve in most old animals.
Since a number of studies assign MEL an important role in the regulation of circadian secretion of CORT (36, 37), and numerous data indicate that a light at night leads to a rapid and pronounced decrease in the secretion of MEL (12, 13, 21–23, 68, 69), we tried to evaluate the age-related and individual features of pineal MEL secretion under CL in our experiment. Therefore, when planning the experiment, we assumed that CL may be accompanied by a decrease in MEL secretion, and this phenomenon could be the main one in the supposed impairment of HPA axis stress reactivity. However, contrary to the expected decrease in the secretion of MEL under chronic CL, we have never revealed a significant decrease in the concentration of MEL at night in either young or old animals, regardless of the behavioral characteristics (see Figures 4 and 9). Therefore, we believe that the inhibitory effect of CL on HPA axis stress reactivity in our experiments is not due to a decrease in MEL.
Our finding that there were no significant changes in pineal MEL secretion under CL is consistent with a number of publications that also did not reveal a decrease in MEL secretion as a result of artificial light at night in humans and diurnal animal species (12, 13, 20, 70–72). In addition, a significant role was reported for the duration, brightness, features of the spectral characteristics of night lighting and the places of contact of illumination with the human and animal body (ocular or extraocular illumination) on the characteristics of pineal secretion of MEL (12, 20, 70). In particular, it was found that a decrease in the secretion of MEL under night lighting is characteristic only when the retina is exposed to blue, but not red light (13, 16, 70, 73, 74). The absence of an expected decrease in MEL secretion under night light, that we found, could be due to changes in the spectrum of polychromatic white light emitted by the discussed LED lamps (see Methods) compared to sunlight. Indeed, according to the characteristics of the LED lamps we use, provided by the distributor, the spectrum emitted by these lamps was characterized by 16 times less activity for short-wave blue light and only 5 times less activity for long-wave red light compared to the spectrum of natural sunlight. In addition, our use of a prolonged (several weeks) CL can neutralize the melatonin-inhibiting effect of green light, as long-term green light illumination has been shown to rapidly desensitize melatonin suppression (75).
It should be noted that, despite the absence of changes in the concentration of MEL in young and old female rhesus monkeys at different times of the day (09.00, 15.00, 22.00) under CL, we found a significant decrease in the concentration of CORT on the background of CL in all animals in 15.00, as well as its increase in young animals at 22.00. In addition, in young animals there was a statistically significant decrease in the amplitude of the circadian rhythm of CORT (the difference between its concentration at 09.00h and 22.00h) (Figures 4 and 9). These data, as well as the above data on a decrease in the stress reactivity of the HPA axis under CL, indicate the damaging effect of CL on the function of the HPA axis.
The identified age-related differences in the function of the HPA axis under CL may be dependent on the age-related changes in the axis “retina – SCN - tissue clock genes”, which were noted by various authors (20, 76–78). In particular, age-related changes have been described for the biochemistry and morphology of SCN (20, 79), the expression of a number of biologically active peptides of SCN (vasoactive intestinal polypeptide; AVP) (80, 81) and an age-related decrease in the amplitude of circadian rhythms of electrical activity in SCN (82). According to some authors, both constriction cause progressive age-related losses in circadian photoreception in terms of phase shifts and melatonin suppression (76, 83).
The revealed phenomenon of the inhibitory effect of CL on the stress reactivity of HPA axis may be of significant practical importance. On the one hand, the findings suggest that chronic CL with LED lamps designed for residential, office and commercial environments may impairs the response of the HPA axis to stress exposure. This finding, apparently, can be used to correct increased vulnerability to stress in individuals with preexisting anxiety and depression stress behaviors. Thus, it is known that increased stress reactivity of the HPA axis often detected in persons with an anxiety and depression-prone behavior, as well as patients with some types of clinically diagnosed depression (6, 60, 62, 63). Perhaps, short-term lighting courses in the evening or at night would be effective to correct increased vulnerability the HPA axis to acute stress. Currently, light therapy at night already is carried out for the treatment of psychiatric diseases, in particular depression (15, 84, 85), sleep disorders (15, 86), neurodegenerative diseases (87, 88). Perhaps it is based, at least in part, on the inhibitory effect of light on the stress reactivity of the HPA axis that we have identified. On the other hand, the CL induced decrease in stress reactivity of the adrenals in behaviorally normal animals suggests a potential risk of reducing the adaptive capacity of the organism under continuous CL conditions. Thus, a decrease in adaptive abilities was noted among residents of the Far North and members of expeditions in Antarctica, as well as among people who work the night shift for a long time (89–94).
Thus, experiments on the study of the features of the HPA axis response to acute stress under conditions of chronic round-the-clock illumination (CL) on the model of young adult and old female rhesus monkeys revealed a pronounced decrease in the magnitude of the rise in the CORT level in response to acute stress exposure (ASE), the mechanisms of which were age-dependent. Young animals were characterized by an inhibitory effect of CL on the adrenal cortex function due to inhibition of the pituitary ACTH secretion. In turn, the inhibitory effect of CL on ACTH secretion seems to be due to the inhibition of the ACTH-stimulating effects of vasopressin. At the same time, in the majority of old animals, an inhibitory effect of CL directly on the function of the adrenal cortex without the participation of the adenohypophysis were revealed. The inhibitory effect of CL on the function of the adrenal cortex was detected in all animals, regardless of behavior, although a number of individual differences were also noted, apparently not of a fundamental nature for the revealed phenomenon. The observed CL inhibition of adrenal cortical reactivity to ASE may be useful to correct increased vulnerability to ASE observed in individuals with preexisting anxiety and depression-like stress behaviors. On the other hand, the CL induced decrease in adrenal stress reactivity of behaviorally normal animals suggests a potential risk of reducing the adaptive capacity of the organism under conditions of continuous light exposure.
The conclusion made about the inhibitory effect of CL on the stress reactivity of the adrenal cortex in old animals has some limitations, since in the experiment with ASE there was no control group, and the comparison of the stress reactivity of the HPA axis in CL conditions was carried out relative to the stress reactivity of the HPA axis in these same animals in the basal period.
The datasets presented in this study can be found in online repositories. The names of the repository/repositories and accession number(s) can be found in the article/Supplementary Material.
The animal study was reviewed and approved by the Ethics Committee of the Research Institute of Medical Primatology, Sochi, Russia.
NG: study design, supervision of performing experiments, analysis of research results, writing the manuscript; OC: performing experiments, statistical analysis of results, preparation of tables and figures; TO: performing experiments, measurement of hormone levels. All authors have approved the final version of manuscript.
This study was funded in accordance with the current scientific plan authorized and supported by the Ministry of Science and High Education of the Russian Federation, project no. AAAA-A18-118032690257-4.
The authors declare that the research was conducted in the absence of any commercial or financial relationships that could be construed as a potential conflict of interest.
All claims expressed in this article are solely those of the authors and do not necessarily represent those of their affiliated organizations, or those of the publisher, the editors and the reviewers. Any product that may be evaluated in this article, or claim that may be made by its manufacturer, is not guaranteed or endorsed by the publisher.
The Supplementary Material for this article can be found online at: https://www.frontiersin.org/articles/10.3389/fendo.2022.1051882/full#supplementary-material
1. Benedict C, Grillo CA. Insulin resistance as a therapeutic target in the treatment of alzheimer’s disease: A state-of-the art review. Front Neurosci (2018) 12:215. doi: 10.3389/fnins.2018.00215
2. Conrad N, Judge A, Tran J, Mohseni H, Hedgecott D, Crespillo AP, et al. Temporal trends and patterns in heart failure incidence: a populationbased study of 4 million individuals. Lancet (2018) 391:572–80. doi: 10.1016/S0140-6736(17)32520-5
3. Faye C, McGowan JC, Denny CA and David DJ. Neurobiological mechanisms of stress resilience and implications for the aged population. Curr Neuropharmacol (2018) 16:234–70. doi: 10.2174/1570159X15666170818095105
4. Menke A. Is the HPA axis as target for depression outdated, or is there a new hope? front. Psychiatry (2019) 10:101. doi: 10.3389/fpsyt.2019.00101
5. Ryan L, Hay M, Huentelman MJ, Duarte A, Rundek T, Levin B, et al. Precision aging: applying precision medicine to the field of cognitive aging. Front Aging Neurosci (2019) 11:128. doi: 10.3389/fnagi.2019.00128
6. Goncharova ND. The HPA axis under stress and aging: individual vulnerability is associated with behavioral patterns and exposure time. BioEssays (2020) 42:2000007. doi: 10.1002/bies.202000007
7. Chang AM, Aeschbach D, Duffy JF, Czeisler CA. Evening use of light-emitting eReaders negatively affects sleep, circadian timing, and next-morning alertness. Proc Natl Acad Sci USA (2015) 112:1232–7. doi: 10.1073/pnas.1418490112
8. Smolensky MH, Sackett-Lundeen LL, Portaluppi F. Nocturnal light pollution and underexposure to daytime sunlight: Complementary mechanisms of circadian disruption and related diseases. Chronobiol Int (2015) 32:1029–48. doi: 10.3109/07420528.2015.1072002
9. Koopman ADM, Rauh SP, van’t Riet E, Groeneveld L, van der Heijden AA, Elders PJ, et al. The association between social jetlag, the metabolic syndrome, and type 2 diabetes mellitus in the general population: the new hoorn study. J Biol Rhythm (2017) 32:359–68. doi: 10.1177/0748730417713572
10. Garcia-Saenz A, Sanchez de Miguel A, Espinosa A, Valentin A, Aragones N, Llorca J, et al. Evaluating the association between artificial light at night exposure and breast and prostate cancer risk in Spain (MCC-Spain study). Environ Health Perspect (2018) 126:4. doi: 10.1289/EHP1837
11. Daugaard S, Markvart J, Bonde JP, Christoffersen J, Garde AH, Hansen AM, et al. Light exposure during days with night, outdoor, and indoor work. Ann Work Expo Health (2019) 63:651–65. doi: 10.1093/annweh/wxy110
12. Rahman SA, Wright KP Jr, Lockley SW, Czeisler CA, Gronfier C. Characterizing the temporal dynamics of melatonin and cortisol changes in response to nocturnal light exposure. Sci Rep (2019) 9:19720. doi: 10.1038/s41598-019-54806-7
13. Fleury J, Masis-Vargas A, Kalsbeek A. Metabolic implications of exposure to light at night: lessons from animal and human studies. Obesity (2020) 28:S18–28. doi: 10.1002/oby.22807
14. Munzel T, Hahad O, Daiber A. The dark side of nocturnal light pollution. outdoor light at night increases risk of coronary heart disease. Eur Heart J (2021) 42:831–4. doi: 10.1093/eurheartj/ehaa866
15. De Nobrega AK, Luz KV, Lyons LC. Resetting the aging clock: implications for managing age-related diseases. In: Guest P, editor. Reviews on new drug targets in age-related disorders. advances in experimental medicine and biology. Cham, Switzerland: Springer (2020). p. 193–266. doi: 10.1007/978-3-030-42667-5_9
16. Wright HR, Lack LC, Kennaway DJ. Differential effects of light wavelength in phase advancing the melatonin rhythm. J Pineal Res (2004) 36:140 –4. doi: 10.1046/j.1600-079X.2003.00108.x
17. Rutters F, Lemmens SG, Adam TC, Bremmer MA, Elders PJ, Nijpels G, et al. Is social jetlag associated with an adverse endocrine, behavioral, and cardiovascular risk profile? J Biol Rhythm (2014) 29:377–83. doi: 10.1177/0748730414550199
18. Figueiro MG, Sahin L, Wood B, Plitnick B. Light at night and measures of alertness and performance: implications for shift workers. Biol Res Nurs (2016) 18:90–100. doi: 10.1177/1099800415572873
19. Jing JN, Wu ZT, Li ML, Wang YK, Tan X, Wang WZ. Constant light exerted detrimental cardiovascular effects through sympathetic hyperactivity in normal and heart failure rats. Front Neurosci (2020) 14:248. doi: 10.3389/fnins.2020.00248
20. Bonmati-Carrion MA, Arguelles-Prieto R, Martinez-Madrid MJ, Reiter R, Hardeland R, Rol MA, et al. Protecting the melatonin rhythm through circadian healthy light exposure. Int J Mol Sci (2014) 15:23448–500. doi: 10.3390/ijms151223448
21. Touitou Y, Reinberg A, Touitou D. Association between light at night, melatonin secretion, sleep deprivation, and the internal clock: health impacts and mechanisms of circadian disruption. Life Sci (2017) 173:94–106. doi: 10.1016/j.lfs.2017.02.008
22. Yonis M, Haim A, Zubidat E. Altered metabolic and hormonal responses in mail rats exposed to acute bright light-at-night associated with global DNA hypo-methylation. J Photochem Photobiol B: Biol (2019) 194:107–18. doi: 10.1016/j.jphotobiol.2019.03.020
23. Rumanova VS, Okuliarova M, Zeman M. Differential effects of constant light and dim light at night on the circadian control of metabolism and behavior. Int J Mol Sci (2020) 21:5478. doi: 10.3390/ijms21155478
24. Buijs RM, Kalsbeek A. Hypothalamic integration of central and peripheral clocks. Nat Rev Neurosci (2001) 2:521–6. doi: 10.1038/35081582
25. Oster H, Damerow S, Kiessling S, Jakubcakova V, Abraham D, Tian J, et al. The circadian rhythm of glucocorticoids is regulated by a gating mechanism residing in the adrenal cortical clock. Cell Metab (2006) 4:163–73. doi: 10.1016/j.cmet.2006.07.002
26. Kalsbeek A, van der Spek R, Lei J, Endert E, Buijs RM, Fliers E. Circadian rhythms in the hypothalamo-pituitary-adrenal (HPA) axis. Mol Cell Endocrinol (2012) 349:20–9. doi: 10.1016/j.mce.2011.06.042
27. Tsang AH, Barclay JL, Oster H. Interactions between endocrine and circadian systems. J Mol Endocrinol (2014) 52:R1–16. doi: 10.1530/JME-13-0118
28. Agorastos A, Nicolaides NC, Bozikas VP, Chrousos GP, Pervanidou P. Multilevel interactions of stress and circadian system: implications for traumatic stress. Front Psychiatry (2020) 10:1003. doi: 10.3389/fpsyt.2019.01003
29. Bartlang MS, Lundkvist GB. Stress and the central circadian clock. In: Fink G, editor. Stress: Neuroendocrinology and Neurobiology: Handbook of stress series, Volume 2. Academic Press (2017). p. 38593. doi: 10.1016/B978-0-12-802175-0.00038-3
30. Nicolaides NC, Charmandari E, Kino T, Chrousos GP. Stress related and circadian secretion and target tissue actions of glucocorticoids: impact on health. Front Endocrinol (Lausanne) (2017) 8:70. doi: 10.3389/fendo.2017.00070
31. Buijs RM, Wortel J, Van Heerikhuize JJ, Feenstra MG, Ter Horst GJ, Romijn HJ, et al. Anatomical and functional demonstration of a multisynaptic suprachiasmatic nucleus adrenal (cortex) pathway. Eur J Neurosci (1999) 11:1535–44. doi: 10.1046/j.1460-9568.1999.00575.x
32. Ishida A, Mutoh T, Ueyama T, Bando H, Masubuchi S, Nakahara D, et al. Light activates the adrenal gland: timing of gene expression and glucocorticoid release. Cell Metab (2005) 2:297–307. doi: 10.1016/j.cmet.2005.09.009
33. Ulrich-Lai YM, Arnhold MM, Engeland WC. Adrenal splanchnic innervation contributes to the diurnal rhythm of plasma corticosterone in rats by modulating adrenal sensitivity to ACTH. Am J Physiol Regul Integr Comp Physiol (2006) 290:R1128–1135. doi: 10.1152/ajpregu.00042.2003
34. Chung S, Son GH, Kim K. Circadian rhythm of adrenal glucocorticoid: its regulation and clinical implications. Biochim Biophys Acta (2011) 1812:581–91. doi: 10.1016/j.bbadis.2011.02.003
35. Oster H, Challet E, Ott H, Arvat E, de Kloet RE, Dijk DJ, et al. The functional and and clinical significance of the 24-hour rhythm of circulating glucocorticoids. Endocr Rev (2017) 38:3–45. doi: 10.1210/er.2015-1080
36. Torres-Farfan C, Richter HG, Rojas-Garcia P, Vergara M, Forcelledo ML, Valladares LE, et al. mt1 melatonin receptor in the primate adrenal gland: Inhibition of adrenocorticotropin-stimulated cortisol production by melatonin. J Clin Endocrinol Metab (2003) 88:450–8. doi: 10.1210/jc.2002-021048
37. Wu YH, Zhou JN, Balesar R, Unmehopa U, Bao A, Jockers B, et al. Distribution of MT1 melatonin receptor immunoreactivity in the human hypothalamus and pituitary gland: Colocalization of MT1 with vasopressin, oxytocin, and corticotropin-releasing hormone. J Comp Neurol (2006) 499:897–910. doi: 10.1002/cne.2115290
38. Scheving LE, Pauly JE. Effect of light on corticosterone levels in plasma of rats. Am J Physiol Legacy Content (1996) 210:1112–7. doi: 10.1152/ajplegacy.1966.210.5.1112
39. Abilio VC, Freitas FM, Dolnikoff MS, Castrucci AML, Filho RF. Effects of continuous exposure to light on behavioral dopaminergic supersensitivity. Biol Psychiatry (1999) 45:1622–9. doi: 10.1016/S0006-3223(98)00305-9
40. Mohawk JA, Pargament JM, Lee TM. Circadian dependence of corticosterone release to light exposure in the rat. Physiol Behav (2007) 92:800–6. doi: 10.1016/j.physbeh.2007.06.009
41. Cailotto C, Lei J, van der Vliet J, van Heijningen C, van Eden CG, Kalsbeek A, et al. Effects of nocturnal light on (clock) gene expression in peripheral organs: a role for the autonomic innervation of the liver. PloS One (2009) 4:1–12. doi: 10.1371/journal.pone.0005650
42. Dauchy RT, Dauchy EM, Tirrell RP, Hill CR, Davidson LK, Greene MW, et al. Dark-phase light contamination disrupts circadian rhythms in plasma measures of endocrine physiology and metabolism in rats. Comp Med (2010) 60:348–56.
43. Fonken LK, Workman JL, Walton JC, Weil ZM, Morris JS, Haim A, et al. Light at night increases body mass by shifting the time of food intake. Proc Natl Acad Sci (2010) 107:18664–9. doi: 10.1073/pnas.1008734107
44. Bedrosian TA, Galan A, Vaughn CA, Weil ZM and Nelson RJ. Light at night alters daily patterns of cortisol and clock proteins in female siberian hamsters. J Endocrinol (2013) 25:590–6. doi: 10.1111/jne.12036
45. Wilson AL, Downs CT. Light interference and melatonin affects digestion and glucocorticoid metabolites in striped mouse. Biol Rhythm Res (2015) 6:929–39. doi: 10.1080/09291016.2015.1066546
46. Kiessling S, Sollars PJ, Pickard GE. Light stimulates the mouse adrenal through a retinohypothalamic pathway independent of an effect on the clock in the suprachiasmatic nucleus. PloS One (2014) 9:e92959. doi: 10.1371/journal.pone.0092959
47. Opperhuizen A-L, Stenvers DJ, Jansen RD, Foppen E, Fliers E, Kalsbeek A. Light at night acutely impairs glucose tolerance in a time-, intensity- and wavelength-dependent manner in rats. Diabetologia (2017) 60:1333–43. doi: 10.1007/s00125-017-4262-y
48. Russart KLG, Nelson RJ. Light at night as an environmental endocrine disruptor. Physiol Behav (2018) 190:82–9. doi: 10.1016/j.physbeh.2017.08.029
49. Fonken LK, Finy MS, Walton JC, Weil ZM, Workman JL, Ross J, et al. Influence of light at night on murine anxiety and depressive-like responses. Behav Brain Res (2009) 205:349–54. doi: 10.1016/j.bbr.2009.07.001
50. Dauchy RT, Wren MA, Dauchy EM, Hoffman AE, Hanifin JP, Warfield B, et al. The influence of red light exposure at night on circadian metabolism and physiology in sprague-dawley rats. J Am Assoc Lab Anim Sci (2015) 54:40–50.
51. Talen BE, Morkrid L, Kjellman BF, Wetterberg L. Cortisol in light treatment of seasonal and non-seasonal depression: relationship between melatonin and cortisol. Acta Psychiatr Scand (1997) 96:385–94. doi: 10.1111/j.1600-0447.1997.tb09934.x
52. Leproult R, Colecchia EF, L’Hermite-Balériaux M and Van Cauter E. Transition from dim to bright light in the morning induces an immediate elevation of cortisol levels. J Clin Endocrinol Metab (2001) 86:151–7. doi: 10.1210/jc.86.1.151
53. Scheer FAJL, Buijs RM. Light affects morning salivary cortisol in humans. J Clin Endocrinol Metab (1999) 84:3395–8. doi: 10.1210/jcem.84.9.6102
54. Rüger M, Gordijn MC, Beersma DG, de Vries B and Daan S. Time-of-day-dependent efects of bright light exposure on human psychophysiology: comparison of daytime and nighttime exposure. Am J Physiol Regul Integr Comp Physiol (2006) 290:R1413–20. doi: 10.1152/ajpregu.00121.2005
55. Jung CM, Khalsa SBS, Scheer FAJL, Cajochen C, Lockley SW, Czeisler CA, et al. Acute efects of bright light exposure on cortisol levels. J Biol Rhythms (2010) 25:208–16. doi: 10.1177/0748730410368413
56. Kostoglou-Athanassiou I, Treacher DF, Wheeler MJ and Forsling ML. Bright light exposure and pituitary hormone secretion. Clin Endocrinol (1998) 48:73–9. doi: 10.1046/j.1365-2265.1998.00355.x
57. Jung CM, Khalsa SBS, Scheer FAJL, Cajochen C, Lockley SW, Czeisler CA, et al. Acute effects of bright light exposure on cortisol levels. J Biol Rhythms (2010) 25:208–16. doi: 10.1177/0748730410368413
58. Cannizzaro E, Cirrincione L, Mazzucco W, Scorciapino A, Catalano C, Ramaci T, et al. Night-time shift work and related stress responses: a study on security guards. Int J Environ Res Public Health (2020) 17:562. doi: 10.3390/ijerph17020562
59. Goncharova ND, Chigarova OA, Oganyan TE. Effect of constant illumination on the function of the hypothalamic-pituitary-adrenal axis in nonhuman primates. Bul Exp Biol Med (2021) 171:778–82. doi: 10.1007/s10517-021-05315-z
60. Ancelin ML, Scali J, Norton J, Ritchie K, Dupuy AM, Chaudieu I, et al. Heterogeneity in HPA axis dysregulation and serotonergic vulnerability to depression. Psychoneuroendocrinology (2017) 77:90–4. doi: 10.1016/j.psyneuen.2016.11.016
61. Goncharova ND, Oganyan TE. Age-related differences in stress responsiveness of the hypothalamic-pituitary-adrenal axis of nonhuman primates with various types of adaptive behavior. Gen Comp Endocrinol (2018) 258:163–72. doi: 10.1016/j.ygcen.2017.08.007
62. Juruena MF, Bocharova M, Agustini B, Young AH. Atypical and non-atypical depression: Is HPA axis function a biomarker? a systematic review. J Affect Disord (2018) 233:45–67. doi: 10.1016/j.jad.2017.09.052
63. Lupien SJ, Juster RP, Raymond C, Marin MF. The effects of chronic stress on the human brain: from neurotoxicity, to vulnerability, to opportunity. Front Neuroendocrinol (2018) 49:91–105. doi: 10.1016/j.yfrne.2018.02.001
64. Goncharova ND, Chigarova OA, Oganyan TE, Rudenko NS. Glucocorticoid negative feedback in regulation of the hypothalamic-pituitary-adrenal axis in rhesus monkeys with various types of adaptive behavior: individual and age-related differences. Front Endocrinol (2019) 10:24. doi: 10.3389/fendo.2019.00024
65. Churilov AN, Milton JG. Modeling pulsativity in the hypothalamic–pituitary–adrenal hormonal axis. Sci Rep (2020) 12:8480. doi: 10.1038/s41598-022-12513-w
66. Ikeno T, Deats SP, Soler J, Lonstein JS, Yan L. Decreased daytime illumination leads to anxiety-like behaviors and HPA axis dysregulation in the diurnal grass rat (Arvicanthis niloticus). Behav Brain Res (2016) 300:77–84. doi: 10.1016/j.bbr.2015.12.004
67. Fischman AJ, Kastin AJ, Graf MV, Moldow RL. Constant light and dark affect the circadian rhythm of the hypothalamic-pituitary-adrenal axis. Neuroendocrinology (1988) 47:309–16. doi: 10.1159/000124930
68. Reiter RJ, Tan DX, Sanchez-Barcelo E, Mediavilla MD, Gitto E, Korkmaz A. Circadian mechanisms in the regulation of melatonin synthesis: disruption with light at night and the pathophysiological consequences. J Exp Int Med (2011) 1:13–22. doi: 10.5455/jeim.101210.ir.001
69. Zubidat AE, Haim A. Artificial light-at-night - a novel lifestyle risk factor for metabolic disorder and cancer morbidity. J Basic Clin Physiol Pharmacol (2017) 28:295–313. doi: 10.1515/jbcpp-2016-0116
70. Hebert M, Martin SK, Eastman CI. Nocturnal melatonin secretion is not suppressed by light exposure behind the knee in humans. Neurosci Lett (1999) 274:127–30. doi: 10.1016/S0304-3940(99)00685-0
71. Figueiro MG, Rea MS. The effects of red and blue lights on circadian variations in cortisol, alpha amylase, and melatonin. Int J Endocrinol (2010) 2010:829351. doi: 10.1155/2010/829351
72. De Rui M, Middleton B, Sticca A, Gatta A, Amodio P, Skene DJ, et al. Sleep and circadian rhythms in hospitalized patients with decompensated cirrhosis: effect of light therapy. Neurochem Res (2014) 40:284 –92. doi: 10.1007/s11064-014-1414-z
73. Gabel V, Maire M, Reichert CF, Chellappa SL, Schmidt C, Hommes V, et al. Effects of artificial dawn and morning blue light on daytime cognitive performance, well -being, cortisol and melatonin levels. Chronobiol Int (2013) 30:988 –97. doi: 10.3109/07420528.2013.793196
74. Mien IH, Chua ECP, Lau P, Tan LC, Lee ITG, Yeo SC, et al. Effects of exposure to intermittent versus continuous red light on human circadian rhythms, melatonin suppression, and pupillary constriction. PloS One (2014) 9:e96532. doi: 10.1371/journal.pone.0096532
75. Gooley JJ, Rajaratnam SM, Brainard GC, Kronauer RE, Czeisler CA, Lockley SW. Spectral responses of the human circadian system depend on the irradiance and duration of exposure to light. Sci Transl Med (2010) 2:31–3. doi: 10.1126/scitranslmed.3000741
76. Turner PL, Mainster MA. Circadian photoreception: Ageing and the eye’s important role in systemic health. Br J Ophthalmol (2008) 92:1439–44. doi: 10.1136/bjo.2008.141747
77. Herbst K, Sander B, Lund-Andersen H, Broendsted AE, Kessel L, Hansen MS, et al. Intrinsically photosensitive retinal ganglion cell function in relation to age: A pupillometric study in humans with special reference to the age-related optic properties of the lens. BMC Ophthalmol (2012) 12:4. doi: 10.1186/1471-2415-12-4
78. Najjar RP, Chiquet C, Teikari P, Cornut PL, Claustrat B, Denis P, et al. Aging of non-visual spectral sensitivity to light in humans: Compensatory mechanisms? PloS One (2014) 9:e85837. doi: 10.1371/journal.pone.0085837
79. Hood S, Amir S. The aging clock: circadian rhythms and later life. J Clin Invest (2017) 127:437–46. doi: 10.1172/JCI90328
80. Aujard F, Cayetanot F, Bentivoglio M, Perret M. Age-related effects on the biological clock and its behavioral output in a primate. Chronobiol Int (2006) 23:451–60. doi: 10.1080/07420520500482090
81. Cayetanot F, Bentivoglio M, Aujard F. Arginine-vasopressin polypeptide rhythms in the suprachiasmatic nucleus of the mouse lemur reveal aging-related alterations of circadian pacemaker neurons in a non-human primate. Eur J Neurosci (2005) 22:902–10. doi: 10.1111/j.1460-9568.2005.04268.x
82. Gibson EM, Williams WP, Kriegsfeld LJ. Aging in the circadian system: Considerations for health, disease prevention and longevity. Exp Gerontol (2009) 44:51–6. doi: 10.1016/j.exger.2008.05.007
83. Revell VL, Skene DJ. Impact of age on human non-visual responses to light. Sleep Biol Rhythm (2010) 8:84–94. doi: 10.1111/j.1479-8425.2009.00418.x
84. Tuunainen A, Kripke DF, Endo T. Light therapy for non-seasonal depression. Cochrane Database Syst Rev (2004) 2:CD004050. doi: 10.1002/14651858.CD004050.pub2
85. Kaladchibachi S, Fernandez F. Precision light for the treatment of psychiatric disorders. Neural Plasticity (2018) 2018:5868570. doi: 10.1155/2018/5868570
86. van Maanen A, Meijer AM, van der Heijden KB, Oort FJ. The effects of light therapy on sleep problems: a systematic review and meta-analysis. Sleep Med Rev (2016) 29:52–62. doi: 10.1016/j.smrv.2015.08.009
87. Rutten S, Vriend C, van den Heuvel OA, Smit JH, Berendse HW, van der Werf YD. Bright light therapy in parkinson’s disease: an overview of the background and evidence. Parkinson's Dis (2012) 2012:1–9. doi: 10.1155/2012/767105
88. Videnovic A, Klerman EB, Wang W, Marconi A, Kuhta T, Zee PC. Timed light therapy for sleep and daytime sleepiness associated with Parkinson disease: a randomized clinical trial. JAMA Neurol (2017) 74:411–8. doi: 10.1001/jamaneurol.2016.5192
89. Olson JJ. Antarctica: a review of recent medical research. Trends Pharmacol Sci (2002) 23:487–90. doi: 10.1016/s0165-6147(02)02087-4
90. Bishop SL. Evaluating teams in extreme environments: from issues to answers. Aviation Space Environ Med (2004) 75:C14–21.
91. Chen N, Wu Q, Li H, Zhang T and Xu C. Different adaptations of Chinese winterover expeditioners during prolonged Antarctic and sub-Antarctic residence. Int J Biometeorol (2016) 60:737–47. doi: 10.1007/s00484-015-1069-8
92. James SM, Honn KA, Gaddameedhi S, Van Dongen HP. Shift work: disrupted circadian rhythms and sleep–implications for health and well-being. Curr Sleep Med Rep (2017) 3:104–12. doi: 10.1007/s40675-017-0071-6
93. Koshy A, Cuesta M, Boudreau P, Cermakian N and Boivin DB. Disruption of central and peripheral circadian clocks in police officers working at night. FASEB J (2019) 33:6789–800. doi: 10.1096/fj.201801889r
Keywords: acute stress, the HPA axis, melatonin, constant light, aging, behavior, rhesus monkeys
Citation: Goncharova N, Chigarova O and Oganyan T (2023) Age-related and individual features of the HPA axis stress responsiveness under constant light in nonhuman primates. Front. Endocrinol. 13:1051882. doi: 10.3389/fendo.2022.1051882
Received: 11 October 2022; Accepted: 20 December 2022;
Published: 09 January 2023.
Edited by:
Donna Toufexis, University of Vermont, United StatesReviewed by:
Asamanja Chattoraj, Kazi Nazrul University, IndiaCopyright © 2023 Goncharova, Chigarova and Oganyan. This is an open-access article distributed under the terms of the Creative Commons Attribution License (CC BY). The use, distribution or reproduction in other forums is permitted, provided the original author(s) and the copyright owner(s) are credited and that the original publication in this journal is cited, in accordance with accepted academic practice. No use, distribution or reproduction is permitted which does not comply with these terms.
*Correspondence: Nadezhda Goncharova, bmRnb25jaGFyb3ZhQG1haWwucnU=
Disclaimer: All claims expressed in this article are solely those of the authors and do not necessarily represent those of their affiliated organizations, or those of the publisher, the editors and the reviewers. Any product that may be evaluated in this article or claim that may be made by its manufacturer is not guaranteed or endorsed by the publisher.
Research integrity at Frontiers
Learn more about the work of our research integrity team to safeguard the quality of each article we publish.