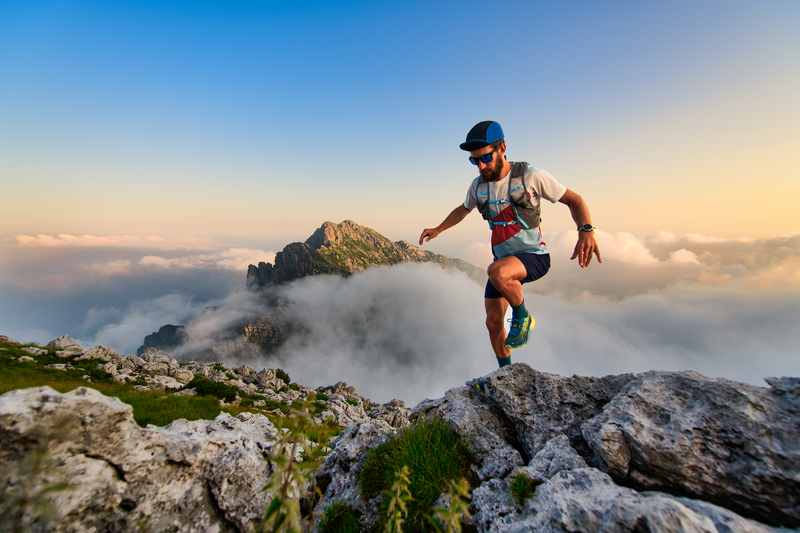
95% of researchers rate our articles as excellent or good
Learn more about the work of our research integrity team to safeguard the quality of each article we publish.
Find out more
REVIEW article
Front. Endocrinol. , 09 January 2023
Sec. Molecular and Structural Endocrinology
Volume 13 - 2022 | https://doi.org/10.3389/fendo.2022.1038874
This article is part of the Research Topic Reviews in Receptor-Receptor Interactions as Novel Targets for Drug Development View all 6 articles
A critical aspect of drug development in the therapy of neuropsychiatric diseases is the “Target Problem”, that is, the selection of a proper target after not simply the etiopathological classification but rather the detection of the supposed structural and/or functional alterations in the brain networks. There are novel ways of approaching the development of drugs capable of overcoming or at least reducing the deficits without triggering deleterious side effects. For this purpose, a model of brain network organization is needed, and the main aspects of its integrative actions must also be established. Thus, to this aim we here propose an updated model of the brain as a hyper-network in which i) the penta-partite synapses are suggested as key nodes of the brain hyper-network and ii) interacting cell surface receptors appear as both decoders of signals arriving to the network and targets of central nervous system diseases. The integrative actions of the brain networks follow the “Russian Doll organization” including the micro (i.e., synaptic) and nano (i.e., molecular) levels. In this scenario, integrative actions result primarily from protein-protein interactions. Importantly, the macromolecular complexes arising from these interactions often have novel structural binding sites of allosteric nature. Taking G protein-coupled receptors (GPCRs) as potential targets, GPCRs heteromers offer a way to increase the selectivity of pharmacological treatments if proper allosteric drugs are designed. This assumption is founded on the possible selectivity of allosteric interventions on G protein-coupled receptors especially when organized as “Receptor Mosaics” at penta-partite synapse level.
Let us open the article with a quote from Sir James Black (Nobel Laureate 1988):
… the benefits that molecular biology will bring to pharmacology are likely, I believe, to be circumscribed by the state of physiological knowledge, models, and concepts (1).
Based on the brain hyper-network (BHN), a model of brain operation previously proposed by us (2), we here present a rational approach to finding therapeutic targets by discussing data obtained at molecular level in the concept of the “Target Problem” (TP).
A crucial point is the assessment of the criteria to characterize a neuropsychiatric disease and to develop the scientific diagnostic approaches to detect the structural and/or functional alterations in the brain areas that could be the cause of the disease (3). From these preliminary steps, the TP would be how to proceed to develop the proper drug-based therapy to address symptoms and/or disease progression without triggering deleterious side effects. Thus, the basic assumption is that one or more brain structures and functions derail from the physiological standard and such disarrangement should be detected based on a model of brain operation.
As the schematic view in Figure 1 illustrates the BHN model for the morpho-functional organization of the central nervous system (CNS) considers the brain as made up by functional modules within plastic boundaries; these modules interact with each other by means of multiple Wiring Transmission (WT) and Volume Transmission (VT) signals (2).
Figure 1 Representation of the brain as a “Brain Hyper-Network” (see the original article (2). The brain as an ″hyper-network″ formed by the integrated assemblage of neural, glia and extracellular molecular networks often organised in compartments of a different size and delimited by plastic boundaries (2). The extracellular molecular network is produced and dynamically modulated by both neurons and glial cells. In turn, the extracellular molecular network plays a role in the formation and dynamic modulation of neuro-glial, intra-neural and intra-glial arches assemblages of components of the three networks form compartments (e.g., functional modules) delimited by plastic boundaries; compartments contain circuits organised according to a ″Russian Doll pattern″ (4). This means that macro-scale, mesoscale, micro-scale, and nano-scale circuits can be described within each Functional. Module. Modified from (2). Abbreviations: EMF electromagnetic fields. For further details, see (2).
According to the BHN model, several structural components at different miniaturization levels should be considered in the investigations of brain complex integrative actions. Indeed, in addition to neural networks other networks such as glial, microglial, extracellular molecular, and brain interstitial fluid (BIF) channel networks must be considered. All these networks are assembled into the BHN via multiple communication modes (5). In addition to the multifaceted aspects of communication modes operating at macro-scale network level, drug development has to explore the location and biochemical features at the meso-scale (cell circuit), micro-scale (cell) and nano-scale (molecular interactions) levels (6). Actually, synaptic contacts play a relevant role at the mesoscale and microscale levels, where neurotransmitter release and decoding processes take place. These highly plastic processes involve the microscale and nanoscale level, that is, cellular and molecular mechanisms occurring at the chemical synapse. Therefore, a detailed analysis of the morpho-functional organization of the CNS especially at the micro-scale and nano-scale level is of relevance to address the topic of drug development in the frame of the TP concept (7).
To clarify the main topic of the present minireview a brief historical summary of the approach that has been followed for decades for drug development focused on the micro-scale and nano-scale levels will be given. In other words, in the context of the TP some main characteristics of the drugs acting as agonists or antagonists on cell surface neuronal receptors will be summarized, with particular reference to G protein-coupled receptors (GPCRs). Subsequently, an expanded view of synaptic contacts will be presented from the perspective of microscale and nanoscale mechanisms at the penta-partite synapse (PPS).
In neuropsychopharmacology the classical view of synapse (see Figure 2, left panel)1 is still the most followed reference framework on which drug discovery and development are based. Indeed, early findings suggested that by acting at the synaptic receptor level, marked changes in integrative brain functions could be achieved ( (17); see (18) for a review on pioneering works discovering dopamine and neurotransmitter effects in brain; see also (19) for a discussion on effects of targeting specific receptors on brain integrative functions). However, frequent failure of drugs in drug development and/or drug side effects, especially during chronic treatments, indicated that the TP was not well resolved by this direct approach (e.g., see (20) for N-methyl-D-aspartate (NMDA) receptor as drug target). A first step forward was the characterization of iso-receptors (i.e. receptor subtypes) at synaptic level, hence the possibility of acting on a more selective target, that is, on a recognition/decoding component of synaptic transmission capable of triggering some peculiar responses at synaptic level (e.g (21). for discovery of dopamine receptor subtypes; see (22) for receptor subtypes as neurotransmitter subtle ways to modulate neuron function; see (23) for dopaminergic transmission and discussion on dopamine receptor subtypes as targets in neuropsychopharmacology; see (24) for serotonergic transmission and discussion on serotonergic receptor subtypes as targets in neuropsychopharmacology). Again, TP was not fully solved with those more selective drugs targeting iso-receptors due to treatment failure and/or side effects, which were less severe than previously (23–25).
Figure 2 Proposed models for the chemical synapse. (A) Several neurotransmitter (NT) inputs like the one indicated in the scheme could impinge on the postsynaptic side. The integrated inputs result in the postsynaptic output: FIRE/NOT FIRE. In principle the pharmacological interventions could be devised on any of these four steps of the chemical synapse. (B) Several NT inputs like the one indicated in the scheme could impinge on the postsynaptic side. The integrated inputs result not only in the postsynaptic output: FIRE/NOT FIRE but also in complex biochemical adjustments. In principle the pharmacological interventions should be devised in a much more complex context. For further details see (8) and the herewith cited bibliography. See also (9–14). NT, neurotransmitter; REC, receptor.
A further important progress was the discovery by Burnstock (26, 27) and by Hokfelt (28–30) of the co-existence and co-release of different neurotransmitters from the same presynaptic terminal (Figure 2 right panel). These breakthrough findings prompted our group to propose that at synaptic level, the co-existence and co-release of neurotransmitters should be accompanied by co-decoding of the released neurotransmitters thanks to i) the assembly of different cell surface receptors complexes (31, 32) and ii) the allosteric interactions derived from receptor-receptor interactions (RRI) (33, 34). RRIs leads to the formation of receptor dimers and multimers, also known as Receptor Mosaics (RMs) (35), the assembly of which into the native conformation may be regulated by other proteins and membrane lipids ( (36) and references therein). As pointed out in several papers, RRIs were found to occur at presynaptic, postsynaptic level and also in glial cells (for reviews see (37–41); see also (42, 43)). The available biochemical evidence on GPCR complexes and on the modulation of their structure and function by cell membrane proteins and lipids could open up new scenarios in the context of the BHN integrative actions and, accordingly, in the pharmacological approaches to neuropsychiatric diseases.
BHN has as crucial nodes the PPS, which acts as complex computational structures formed by neural (pre- and post-synaptic), astrocytic, microglial and extracellular matrix molecular networks; in coordinated fashion they compute by signals spreading via the BIF channels (Figures 3, 4). It was originally proposed the concept of tripartite synapse (45, 46), where pre- and post-synaptic structures, and the perisynaptic astrocyte processes (PAPs) ensheathing the synapse, integrate their functions. It became then apparent that a comprehensive view of the synapse includes the extracellular matrix playing not only plastic but also signal-modifying roles at the tetra-partite synapse (44, 47–50). Furthermore, the involvement of microglial cells that can transiently contact the synapse and extracellular matrix and send signals to be integrated in PPS was recognized; in particular, microglia appear to be involved in fine tuning of neural circuits in pathological conditions (51–56). Therefore, it is proposed that PPSs are likely basic computational modules playing crucial integrative tasks in healthy and in diseased conditions.
Figure 3 Glial cells, extracellular matrix and neurons build up penta-partite synapses (PPSs): penta-partite synapses are crucial components of the Brain Hyper-Network (BHN) nodes and are surrounded by Brain Interstitial Fluid (BIF) channels that allow a control of signals exchange between the synapse and the extra-cellular fluid. It should be noted that some highly pervasive signals as the electromagnetic field do not need the BIF channels to reach the PPS components. While neurons, astrocytes and extracellular matrix are structural components of the synapse, microglia can be mainly involved in transient functional regulating roles at the synapse in normal brain homeostasis and in disease. Modified from (2). BHN, Brain Hyper-Network; BIF, Brain Interstitial; Fluid, GNs glial networks; NNs, neural networks.
Figure 4 Schematic representation of the main morpho-functional features of the penta-partite synapse (PPS). Astrocytes, extracellular matrix, and neurons together with microglia build up PPS. VT signals can reach the PPSs via the Brain Interstitial Fluid channels (BIF channels) impinging on them. Besides the classical pre- and post-synaptic sides, of basic importance for the integrative function of the PPSs are the several molecular components indicated in the scheme, and microglial cytokines/chemokines involved in brain homeostasis and in homeostasis loss in disease. Modified from (2, 44). CAM cell adhesion molecules, EMF, electromagnetic field; HMN, horizontal molecular network; NT, neurotransmitter; VMN, vertical molecular network leading to signal transduction; VT, volume transmission; WT, wiring transmission.
By affecting the perviousness of BIF channels controlling the signals impinging on the synapses, perisynaptic astrocytic processes regulate the multiplicity of events at the PPS. In addition, other highly pervasive signals can reach the PPSs. In this context some cues should be mentioned, namely electromagnetic fields , biophotons, oxygen and carbon dioxide, and the gaseous transmitter nitric oxide, since they can modulate morpho-functional aspects of the various networks mentioned above, deeply affecting penta-partite synapses (57–60). In particular, as far as electromagnetic fields are concerned, they are generated by neural activity and exert a direct action on the voltage sensor of the voltage-gated calcium channels that are involved in the neurotransmitter release (61–64). In this context the concept of broadcasted neuro-connectomes has been proposed to describe how these highly pervasive signals may affect information handling of brain networks especially at high miniaturization levels (2). Broadcasted neuro-connectomes, indeed, could modulate nanoscale “computational nodes” such as the RMs at the PPSs (Figure 4) (2, 44, 58).2
Membrane receptor complexes were, therefore, proposed as key integrators capable of converting multiple extracellular signals into appropriate cellular biochemical responses (37, 41, 65). These aspects, opening new possibilities to addressing the TP, will be to some extent detailed in the section that follows.3
Different postsynaptic decoding processes can result from the corelease of different neurotransmitters (signals) from the presynaptic terminals. Usually, chemically different transmitters are packaged in separate populations of synaptic vesicles, hence multiple signals can be released in response to a proper stimulation. However, multiple signals can be also released in the case in which two or more chemically different transmitters are present in the same vesicle (66). The regulatory mechanisms leading to co-transmitter packaging are still not completely clarified (67–70). These transmitter signals can be recognized by different decoding mechanisms both at pre- and post-synaptic level (66, 71).
These mechanisms involve molecular networks (see (72) for a review), also known as Horizontal Molecular Networks (HMNs) (Figure 4); they are located in membrane microdomains of presynaptic and postsynaptic elements, and in the membrane of associated glial cells. HMNs can operate as ‘‘intelligent devices” since they can sense not only extra-cellular and the intra-cellular environments but also the different components of the multi-facet structure of the penta-partite chemical synapse such as, e.g., components of the Extracellular Molecular Network (Figures 1, 3).
A crucial role in HMNs may be played by GPCR complexes. A given GPCR or different GPCRs can be present in the plasma membrane as monomers, dimers or oligomers and these different molecular arrangements have important functional implications. In fact, in view of the allostery phenomenon, GPCRs at HMNs level operate as highly plastic integrative units since allosterism resulting from RRIs can be differentially modulated (2, 40, 72–75). As a matter of fact, “allostery” can be described as the biochemical phenomenon that allows the transmission, inside and between associated macromolecules, of information from the site where the effector binds to a distant functional site where the GPCR agonist binds (76). Thus, a complex regulation of the activity of macromolecules allows the appearance, via the macromolecular assembly, of novel properties (see (34) and the bibliography herewith mentioned). Therefore, in this context is often cited the famous Monod’s sentence stating that “allostery” for protein functions should be considered as “the second secret of life,” since it is only second as importance to the genetic code (77–79). As our group has discussed in previous papers, the actions of the orthosteric and allosteric ligands of the GPCRs that form an assembly provide the cellular decoding apparatus with sophisticated dynamics (80) in terms of binding modulation, G-protein-mediated signaling and selectivity, receptor desensitization, and switching to β-arrestin-dependent signaling (see (81) for a recent review).
In this context, the consequences of receptor complex formation deserve consideration in GPCR-based drug discovery; there are several allosteric modulators in clinical trials (82) thus showing the potential of allosteric modulators of GPCRs in multiple CNS disorders (83). Apart from decreased adverse side effects (83), allosteric ligands can provide greater receptor subtype selectivity as well as temporal selectivity (82). In this respect, novel specific allosteric sites susceptible of being allosterically targeted may appear in the quaternary structure resulting from the assembly of the receptor protomers. Selective ligands may exist for a specific receptor structure, which is only found in a heteromeric context; this possibility further expands the possibilities of modulating the decoding processes (see (84) for a review). Indeed, those allosteric binding sites are attractive targets for drug development (40, 72, 74, 85). In the absence of crystal structures of GPCR heteromers except for the extracellular domains in the heteromers of class C GPCRs (86–89) there are reliable tetrameric models reported for class A GPCR heteromers (90–95).
It is clear that to surmise protein-protein interactions , evidence of their co-localization (i.e., close proximity (<10 nm)) at cell membrane level has to be demonstrated. Hence, co-localization of two GPCRs and the interplay of their decoding processes are preliminary experimental evidence needed to assess RRI (31, 73, 96). Thus, early evidence on RRIs was indirect evidence based on a coarse co-localization of two receptors obtained by computer-assisted immunocytochemical image analyses. Biochemical approaches led to results that showed that, in membrane preparations from discrete brain regions, the ligand binding to one of the two receptors in a heteromer modulated the binding to the other receptor ( (96, 97); see (81) for a review). Such evidence was further supported by functional studies carried out in vivo assessing the functional relevance of the in vitro findings in both physiological conditions and in animal models of CNS disease (31, 98–101). In the last few decades, several biophysical techniques have been developed that allow a direct demonstration not only of the close (<10 nm) spatial proximity but also of direct interactions between receptors in neuronal and glial cell membranes. In this context experimental approaches of particular relevance are: energy transfer-based methods, bimolecular luminescence or fluorescence complementation, total internal reflection fluorescence microscopy, fluorescence correlation spectroscopy, coimmunoprecipitation, assays based on bivalent ligands and in situ proximity ligation assays ( (41, 102) and herewith included bibliography). We are aware that some associations of receptors as heterodimers found in heterologous systems of expression might not exist in vivo, and that the addressing of receptors in specific locations of the cells allows for avoiding some heteromers. Therefore, it seems of crucial importance to obtain evidence for RRIs of native GPCRs in heteromers through both physicochemical and functional approaches.
Let us briefly examine some peculiar aspects of the RRIs due to GPCRs oligomerization centered in the RMs concept in cells forming the PPS.
It is certainly possible to have receptor colocalization without receptor heteromerization and it is also possible to have heteromerization without allosteric RRIs, when subunit interaction does not cause conformational changes in other subunits of the complex (103). However, RMs especially at the PPS are likely crucial nodes for the BHN, since these plastic mosaics can also undergo a reshuffling, including addition of new proteins (“tesserae” of the mosaic via, e.g., the Roamer-Type of VT (5, 74, 104)) or alteration in their topology and order of diffusion of the allosteric signaling in the mosaic (for a discussion of the topic and the possible role of “Hub Receptors” in the RMs see below) (40, 74, 75, 105).
RRIs not only cause marked effects on the recognition/decoding processes of dimers with respect to the monomers but also it has been demonstrated that iso-receptor dimers can have a clear-cut shift in recognition/decoding characteristics with respect to the iso-receptor monomers. For example, k-δ and δ-μ opioid receptor heterodimers show a shift (with respect to the k, δ or μ monomers) in receptor affinity and in the cell response to opioid molecules (106, 107). Another remarkable finding has been the switch detected for the D1R-D2R heteromer with a change from Gs (dopamine D1R) and Gi/o (dopamine D2R) to a Gq/11 coupling (108). Thus, also simple RMs, such the dimers, display previously unsuspected properties with respect to the component monomers; this capability underlines the likely crucial role of RRIs, especially at the PPS where they are components of a “intelligent interface” communicating the extra- and intra-cellular environments. As a matter of fact, several mechanisms allow different integrative processes in RMs formed by three or more GPCRs and this fact has led us to propose the existence of an “Hub Receptor” that in some way coordinates the activation of the various components adding a new plastic capability to the mosaic (105, 109). Thus, it has been proposed to define a GPCR in a RM as a “Hub Receptor” if it has the following characteristics:
1. it interacts with multiple partners either receptors of the RM or proteins associated with the plasma membrane;
2. it can control, at least to some extent, the sequential order according to which the individual components of the mosaic are involved in the integrative action of the mosaic.
This scenario adds a new layer to brain connectivity since brain-wide connectivity can now be described at macro-scale, meso-scale, micro-scale and nano-scale levels (for the connectome concept see (110, 111)). We have been specially interested and involved in the investigations of the integrative mechanisms for reciprocal releasing and decoding processes of multiple signals at meso-scale, micro-scale and nano-scale level in particular considering the PPSs and the RMs (2, 6, 112, 113).
In this context, our aim has been to correlate the morpho-structural characteristics of the BHN computational nodes with the manner WT and VT multi-faceted communication modes allow integrative actions. As pointed out above, it is suggested that further research should be carried out to investigate the possible crucial role that allosteric interactions play in RMs located in neuronal and glial cells, especially in the PPS.
Crosstalk between neurons and glial cells occurs thanks to both WT and VT, as evidenced by, for instance, adenosine receptors-mediated signaling. As a matter of fact, adenosine is released by neurons and astrocytes allowing complex interactions among RMs containing the four adenosine receptors that may be present in both cell types (114, 115). Adenosine receptors are considered targets for several peripheral diseases, mainly cardiovascular (116, 117), but also for various neuropsychiatric diseases (118, 119). It should be underlined that although adenosine storage in synaptic vesicles and modes for release are not fully clarified (120–123), the compound can modulate neurotransmitter release/reuptake and neurotransmission itself by, among other, actions involving neuronal excitability and synaptic plasticity (124–129). In agreement with multi-faceted adenosine actions, it has been demonstrated that adenosine receptors , especially via RRIs, can modulate glia–neuron and glia–glia intercellular communication, with significant consequences not only on synaptic activity but also on brain network integrative functions. Thus, it is obvious the potential relevance of investigations on the allosteric modulators of RMs containing adenosine receptors localized both in neuronal and glia cell surface (41–43, 94, 130–135).
This important topic will be discussed in the next section with an emphasis on the potential implications for the design of new pharmacological approaches to combat neuropsychiatric diseases (42, 43, 74, 133, 136, 137).
As pointed out above and by several groups (83, 129) a new approach to advance in solving the TP is to develop allosteric modulators capable of selectively favor or reduce the selected iso-receptor response to its orthosteric ligand. Thus, allosteric modulators especially of iso-receptors in RMs represent a new therapeutic approach with the added value of probably reducing side effects compared to classical receptor agonists and antagonists.
To provide therapeutic benefit, allosteric modulators can be considered based on three properties that were outlined by Korkutata et al. (129)
1. affinity modulation of the binding of the orthosteric ligand to the receptor;
2. modulation of the receptor decoding signaling triggered by the orthosteric ligand;
3. possible effects of the allosteric modulator on the receptor conformation and/or membrane location in the absence of the orthosteric ligand.
The latter property is often underestimated even though it may play an important role in the formation and location of RMs at the cell membrane level.
Allosteric modulators can have at least three different effects on the orthosteric binding sites as far as agonist/antagonist affinity and efficacy are concerned, namely can be positive, negative or neutral allosteric modulators (138). Neutral allosteric modulators have no effects on the orthosteric ligand affinity/efficacy but by occupying the allosteric binding site inhibits the activity of positive/negative allosteric modulators.
Summing up, since the integrative actions of signals by RMs are based on inter-GPCRs interactions, allosteric modulators can play important roles in at least partially solving the TP. In other words, allosteric modulators could offer a new perspective to solve the “selectivity problem”, i.e., how to hit only the proper target avoiding side effects due to targeting receptors in cells located within not altered brain areas. In view of the possible crucial role of RMs at this BHN node, the most suitable “allosteric drugs” would be those acting on GPCRs (tesserae) of the RMs especially at PPS. In this context it is important to briefly report some experimental evidence supporting the relevance of the allosteric modulations of adenosine receptors in RMs resulting in marked modulatory shifts in their integrative actions.
One example is provided by results obtained in CHO cells stably co-transfected with dopamine D2 and adenosine A2A receptors. The interaction of these two receptors was one of the first reported for class A GPCRs (139, 140).
In CHO cells expressing the A2A-D2 receptor heteromer, homocysteine (Hcys) selectively reduced the internalization of these heteromers induced by D2 receptor agonists stimulation. It is important to underline that Hcys did not disrupt or prevent the heteromerization of A2A and D2 receptors, suggesting that Hcys probably behaves as a modulator of the allosteric process of energy transmission between the two partners (see Figure 5) (74, 105, 141). Hence, Hcys acts as an allosteric modulator that specifically binds to an ad hoc (allosteric) binding site made available by the structure of the heteromer.
Figure 5 On the possible existence of modifiers along the allosteric communication channels in receptor heteromers. Allosteric communication in receptor heteromers (Receptor Mosaics, RMs) can be modulated by modifiers. Along the allosteric pathway connecting A2A with D2 receptors, homocysteine (Hcys) can modulate the heteromer integrative action. Left panel: the Hcys allosteric binding site is an epitope on the ICL3 of D2-R, hence it can efficiently modulate two out of three of the domains involved in the binding pocket of the D2 receptor (for experimental procedures see (141)). This finding can be of great importance since indicates a new pharmacological approach to produce a biasing action on RMs involved in PD. Lan et al., 2006 (142); Agnati et al., 2006 (141). DA, dopamine; ECL, extracellular loop; ICL, intracellular loop; PD, Parkinson’s disease; TM, transmembrane.
Notably, the allosteric modulation of Hcys previously reported in heterologous systems of expression was demonstrated for the associations of native receptors in heterodimers in astrocytes where Hcys reduced D2 receptor-mediated inhibition of glutamate release without altering the A2A-D2 receptor interaction; in fact, the A2A receptor-mediated antagonism of dopaminergic actions was maintained (see Figure 6). These findings, as discussed also in recent published papers, open the possibility to explore novel, glia-mediated strategies to address neurodegenerative and functional BHN disorders such as Parkinson’s disease (136, 143). As a matter of fact, inhibition of astrocytic D2 receptor-mediated signaling by A2A receptor agonists may contribute to striatal glutamatergic transmission dysfunction by increasing the extracellular glutamate levels. Thus, astrocytic A2A-D2 receptor heteromers can be a proper target to control, also by means of allosteric modulators, striatal glutamate transmission in Parkinson’s disease.
Figure 6 Representation of astrocytic A2A-D2 receptor heteromers in the striatum. A confocal image showing immunofluorescence staining of an astrocyte with the astrocyte marker Glial Fibrillary Acidic Protein (GFAP) in a striatal slice from adult rat. The presence of A2A-D2 receptor heteromers was demonstrated in striatal astrocytes both on perisynaptic processes and astrocyte branchlets (42, 137). Activation of the A2A receptor in the heteromer prevented the effect of D2 receptor activation (42); intracellular homocysteine (Hcys) behaved as an allosteric antagonist of the D2 receptor while maintaining A2A-D2 interaction (136). The heteromers were involved in the control of glutamate release from the processes (42, 136). The findings suggest that A2A-D2 receptor heteromer may play crucial roles to control glutamatergic transmission in striatal functional modules, supporting exploration of strategies targeting at the heteromers to address neurodegenerative and functional striatal disorders. Yellow arrows: allosteric antagonism; red arrow: inhibition of vesicular glutamate release.
Therefore, Hcys D2 silencing at the astrocytic level could be effective targets to be considered in the context of the classical treatments aimed, inter alia, to reduce the neuro-inflammatory cascade for the Parkinson’s disease onset (74).
It is obvious that each component of the BHN can be target of a pharmacological treatment, but components that operate in an integrative mode can be more suitable targets for drug development. Proper pharmacological treatments may be those triggering synergistic effects to compensate the morpho-functional alterations of CNS diseases. For example, actions on the cell surface (e.g., Lipid Rafts) can affect RMs assemblage and composition, hence their recognition and decoding processes of signals. RMs can be modulated by, for instance, electromagnetic fields and also by the Roamer Type VT since, as mentioned above, micro-vesicles can transfer/exchange GPCRs. However, the evaluation of drug effectiveness and side effects can be made more difficult in poly-therapies involving pharmacological and non-pharmacological approaches.
According to the proposal discussed in the paper, a rational possible approach to the “Target Problem” is based on:
1. careful investigations by means of different diagnostic techniques of the BHN structures that are altered in their integrative mechanisms;
2. detection of biochemical targets (especially at the PPS level) that play a crucial role in the functional dysregulation, with special emphasis on GPCRs;
3. development of allosteric modulators that acting on the target GPCR-containing macromolecular complexes in the RMs restore the proper function of the PPS.
As far as the development of drugs acting on selected GPCRs is concerned, the development of drugs acting on dimers, hence of bivalent ligands has been also taken into account (144–153). A bivalent ligand consists of two pharmacophoric entities linked by an appropriate spacer. In this way it should be possible to target GPCR heteromers by adequate, potent, and receptor-selective pharmacophores. A potential drawback could be the hydrolysis of the compound before reaching the CNS.
Furthermore, it is worth evaluating the efficacy of drugs targeting heteromers composed of GPCRs for neurotransmitters/gliotransmitters and GPCRs for cytokines or chemokines. The combination of these different type of receptors in the RMs in the PPS, allow novel possibilities for the development of very selective allosteric modulators; this strategy might acquire relevance in the integrated signaling at PPS, i.e. when activated microglial cells are in proximity of neuronal and astrocytic synaptic structures (51, 52, 55, 56). In fact, heteromers formed by chemokine and opioid receptors in the plasma membrane of lymphocytes or cell models provide novel functional properties in response to opioids and to chemokines ( (154–156); see also (157)).
Summing up, pharmacology aimed at opposing neuropsychiatric diseases requires revisiting the target selection criteria and including the integrative modules that are morphologically and/or functionally altered in the disease. In this sense, experimental investigations should be developed that seek procedures capable of restoring, at least partially, the physiological conditions without leading to serious side effects. The development of allosteric modulators selective for structural domains in GPCR heteromers appears as a very promising strategy.
All authors contributed to the article and approved the submitted version.
This work was supported by FFABR (Fondo di finanziamento per le attività base di ricerca; MIUR-Italy) to MM, by grant PID2020-126600OB-I00 funded by Spanish MCIN/AEI/10.13039/501100011033 to RF and, as appropriate, by “ERDF A way of making Europe”, by the “European Union” or by the “European Union Next Generation EU/PRTR” to RF, by the University of Bologna (RFO University of Bologna to PC) and by FFABR (Fondo di finanziamento per le attività base di ricerca; MIUR-Italy) to CC.
The authors declare that the research was conducted in the absence of any commercial or financial relationships that could be construed as a potential conflict of interest.
All claims expressed in this article are solely those of the authors and do not necessarily represent those of their affiliated organizations, or those of the publisher, the editors and the reviewers. Any product that may be evaluated in this article, or claim that may be made by its manufacturer, is not guaranteed or endorsed by the publisher.
1. Black J. Foreword. In: Noble D, Boyd CAR, editors. Logic of life: The challenge of integrative physiology. New York: Oxford University Press (1993).
2. Agnati LF, Marcoli M, Maura G, Woods A, Guidolin D. The brain as a “hyper-network”: The key role of neural networks as main producers of the integrated brain actions especially via the “broadcasted” neuroconnectomics. J Neural Transmiss (2018) 125(6):883–97. doi: 10.1007/s00702-018-1855-7
3. Lima AA, Mridha MF, Das SC, Kabir MM, Islam MR, Watanobe Y. A comprehensive survey on the detection, classification, and challenges of neurological disorders. Biology (2022) 11(3):469. doi: 10.3390/biology11030469
4. Agnati LF, Fuxe K. New concepts on the structure of the neuronal networks: The miniaturization and hierarchical organization of the central nervous system: Hypothesis. Biosci Rep (1984) 4(2):93–8. doi: 10.1007/BF01120304
5. Agnati LF, Guidolin D, Guescini M, Genedani S, Fuxe K. Understanding wiring and volume transmission. Brain Res Rev (2010) 64(1):137–59. doi: 10.1016/j.brainresrev.2010.03.003
6. Guidolin D, Marcoli M, Maura G, Agnati LF. New dimensions of connectomics and network plasticity in the central nervous system. Rev Neurosci (2017) 28(2):113–32. doi: 10.1515/revneuro-2016-0051
7. De Luca C, Colangelo AM, Virtuoso A, Alberghina L, Papa M. Neurons, glia, extracellular matrix and neurovascular unit: A systems biology approach to the complexity of synaptic plasticity in health and disease. Int J Mol Sci (2020) 21(4):1539. doi: 10.3390/ijms21041539
8. Agnati LF, Genedani S, Leo G, Rivera A, Guidolin D, Fuxe K. One century of progress in neuroscience founded on golgi and cajal's outstanding experimental and theoretical contributions. Brain Res Rev (2007) 55(1):167–89. doi: 10.1016/j.brainresrev.2007.03.004
9. Ramón y Cajal S. Neue darstellung vom histologischen bau des central nervensystem. Arch Anat Entwick (1893) 1893:319–428.
10. Foster M, Sherringhton CS. A textbook of physiology. with C.S. sherrington. part three: The central nervous system. 7th Vol. 1897. . London: Macmillan (1897).
11. Dale H. Some recent extensions of the chemical transmission of the effects of nerve impulses. Nobel Lecture (1936). doi: 10.1101/SQB.1936.004.01.015
12. Hökfelt T, Elfvin LG, Elde R, Schultzberg M, Goldstein M, Luft R. Occurrence of somatostatin-like immunoreactivity in some peripheral sympathetic noradrenergic neurons. Proc Natl Acad Sci USA (1977) 74:3587–91. doi: 10.1073/pnas.74.8.3587
13. Hökfelt T, Rehfeld JF, Skirboll L, Ivemark B, Goldstein M, Markey K. Evidence for coexistence of dopamine and CCK in mesolimbic neurones. Nature (1980) 285:476–8. doi: 10.1038/285476a0
14. Burnstock G. Do some nerve cells release more than one transmitter? Neuroscience (1976) 1(4):239–48. doi: 10.1016/0306-4522(76)90054-3
15. McCulloch WS, Pitts W. A logical calculus of the ideas immanent in nervous activity. Bull Math Biophys (1943) 5(4):115–33. doi: 10.1007/BF02478259
16. Moreno-Díaz R, Moreno-Díaz A. On the legacy of WS McCulloch. Biosystems (2007) 88(3):185–90. doi: 10.1016/j.biosystems.2006.08.010
17. Kebabian JW, Greengard P. Dopamine-sensitive adenyl cyclase: Possible role in synaptic transmission. Science (1971) 174(4016):1346–9. doi: 10.1126/science.174.4016.1346
18. Snyder SH. What dopamine does in the brain. Proc Natl Acad Sci USA (2011) 108(47):18869–71. doi: 10.1073/pnas.1114346108
19. Giessing C, Thiel CM. Pro-cognitive drug effects modulate functional brain network organization. Front Behav Neurosci (2012) 6:53. doi: 10.3389/fnbeh.2012.00053
20. Lipton SA. Failures and successes of NMDA receptor antagonists: Molecular basis for the use of open-channel blockers like memantine in the treatment of acute and chronic neurologic insults. NeuroRx (2004) 1(1):101–10. doi: 10.1602/neurorx.1.1.101
21. Kebabian JW, Calne DB. Multiple receptors for dopamine. Nature (1979) 277(5692):93–6. doi: 10.1038/277093a0
22. Snyder SH. Drug and neurotransmitter receptors in the brain. Science (1984) 224(4644):22–31. doi: 10.1126/science.6322304
23. Charvin D, Medori R, Hauser RA, Rascol O. Therapeutic strategies for Parkinson disease: Beyond dopaminergic drugs. Nat Rev Drug Discovery (2018) 17(11):804–22. doi: 10.1038/nrd.2018.136
24. Carhart-Harris R, Nutt D. Serotonin and brain function: A tale of two receptors. J Psychopharmacol (2017) 31(9):1091–120. doi: 10.1177/0269881117725915
25. Miller G. Is pharma running out of brainy ideas? Science (2010) 329(5991):502–4. doi: 10.1126/science.329.5991.502
27. Burnstock G. Do some nerve cells release more than one transmitter? In: Smith AD, Llinas R, Kostyuk PG, editors. Commentaries in the neurosciences. Oxford: Pergamon Press (1980). p. 151–60. doi: 10.1016/B978-0-08-025501-9.50011-9
28. Hökfelt T, Holets VR, Staines W, Meister B, Melander T, Schalling M, et al. Coexistence of neuronal messengers–an overview. Prog Brain Res (1986) 68:33–70. doi: 10.1016/s0079-6123(08)60230-7
29. Hökfelt T. Coexistence of neuromessenger molecules-a perspective. In: Gutiérrez R, editor. Co-Existence and co-release of classical neurotransmitters: Ex uno plures. New York: Springer Science + Business Media (2009). p. 1–13. doi: 10.1007/978-0-387-09622-3_1
30. Hökfelt T, Barde S, Xu Z-QD, Kuteeva E, Rüegg J, Le Maitre E, et al. Neuropeptide and small transmitter coexistence: Fundamental studies and relevance to mental illness. Front Neural Circuits (2018) 106:106. doi: 10.3389/fncir.2018.00106
31. Agnati LF, Fuxe K, Zoli M, Rondanini C, Ogren S. New vistas on synaptic plasticity: the receptor mosaic hypothesis of the engram. Med Biol (1982) 60(4):183–90.
32. Fuxe K, Agnati LF, Benfenati F, Celani M, Zini I, Zoli M, et al. Evidence for the existence of receptor–receptor interactions in the central nervous system. studies on the regulation of monoamine receptors by neuropeptides. In: Goldstein M, Jellinger K, Riederer P, editors. Basic aspects of receptor biochemistry, vol. 18 . Vienna: Springer (1983). p. 165–79. doi: 10.1007/978-3-7091-4408-4_16
34. Motlagh HN, Wrabl JO, Li J, Hilser VJ. The ensemble nature of allostery. Nature (2014) 508(7496):331–9. doi: 10.1038/nature13001
35. Agnati LF, Tarakanov AO, Ferré S, Fuxe K, Guidolin D. Receptor-receptor interactions, receptor mosaics, and basic principles of molecular network organization. J Mol Neurosci (2005) 26(2):193–208. doi: 10.1385/JMN:26:2-3:193
36. Fuxe K, Marcellino D, Borroto-Escuela DO, Frankowska M, Ferraro L, Guidolin D, et al. The changing world of G protein-coupled receptors: From monomers to dimers and receptor mosaics with allosteric receptor–receptor interactions. J Recept Signal Transduct (2010) 30(5):272–83. doi: 10.3109/10799893.2010.506191
37. Agnati L, Fuxe K, Zini I, Lenzi P, Hökfelt T. Aspects on receptor regulation and isoreceptor identification. Med Biol (1980) 58(4):182–7.
38. Franco R, Casadó V, Cortés A, Ferrada C, Mallol J, Woods A, et al. Basic concepts in G-protein-coupled receptor homo-and heterodimerization. ScientificWorldJournal (2007) 7:48–57. doi: 10.1100/tsw.2007.197
39. Franco R, Casadó V, Cortés A, Mallol J, Ciruela F, Ferré S, et al. G-Protein-coupled receptor heteromers: Function and ligand pharmacology. Br J Pharmacol (2008) 153(S1):S90–S8. doi: 10.1038/sj.bjp.0707571
40. Farran B. An update on the physiological and therapeutic relevance of GPCR oligomers. Pharmacol Res (2017) 117:303–27. doi: 10.1016/j.phrs.2017.01.008
41. Guidolin D, Tortorella C, Marcoli M, Cervetto C, Maura G, Agnati LF. Receptor–receptor interactions and glial cell functions with a special focus on G protein-coupled receptors. Int J Mol Sci (2021) 22(16):8656. doi: 10.3390/ijms22168656
42. Cervetto C, Venturini A, Passalacqua M, Guidolin D, Genedani S, Fuxe K, et al. A2A-D2 receptor–receptor interaction modulates gliotransmitter release from striatal astrocyte processes. J Neurochem (2017) 140(2):268–79. doi: 10.1111/jnc.13885
43. Amato S, Averna M, Guidolin D, Pedrazzi M, Pelassa S, Capraro M, et al. Heterodimer of A2A and oxytocin receptors regulating glutamate release in adult striatal astrocytes. Int J Mol Sci (2022) 23(4):2326. doi: 10.3390/ijms23042326
44. Marcoli M, Agnati LF, Benedetti F, Genedani S, Guidolin D, Ferraro L, et al. On the role of the extracellular space on the holistic behavior of the brain. Rev Neurosci (2015) 26(5):489–506. doi: 10.1515/revneuro-2015-0007
45. Araque A, Parpura V, Sanzgiri RP, Haydon PG. Tripartite synapses: Glia, the unacknowledged partner. Trends Neurosci (1999) 22(5):208–15. doi: 10.1016/s0166-2236(98)01349-6
46. Halassa MM, Fellin T, Haydon PG. The tripartite synapse: Roles for gliotransmission in health and disease. Trends Mol Med (2007) 13(2):54–63. doi: 10.1016/j.molmed.2006.12.005
47. Agnati LF, Leo G, Zanardi A, Genedani S, Rivera A, Fuxe K, et al. Volume transmission and wiring transmission from cellular to molecular networks: History and perspectives. Acta Physiol (Oxf) (2006) 187(1-2):329–44. doi: 10.1111/j.1748-1716.2006.01579.x
48. Agnati LF, Zunarelli E, Genedani S, Fuxe K. On the existence of a global molecular network enmeshing the whole central nervous system: Physiological and pathological implications. Curr Prot Pept Sci (2006) 7(1):3–15. doi: 10.2174/138920306775474086
49. Dityatev A, Rusakov DA. Molecular signals of plasticity at the tetrapartite synapse. Curr Opin Neurobiol (2011) 21(2):353–9. doi: 10.1016/j.conb.2010.12.006
50. Guidolin D, Guescini M, Stocchi V, Genedani S, Fuxe K, Agnati LF. Extra-cellular proteins are key elements of a global molecular network enmeshing the whole central nervous system: Physiological and pathological implications. In: Dunn BM, editor. Advances in protein and peptide sciences: Bentham science (2013). p. 792–832. UAE: Bentham Science. doi: 10.2174/9781608054879113010021
51. Miyamoto A, Wake H, Moorhouse AJ, Nabekura J. Microglia and synapse interactions: Fine tuning neural circuits and candidate molecules. Front Cell Neurosci (2013) 7:70. doi: 10.3389/fncel.2013.00070
52. Savage JC, St-Pierre M-K, Carrier M, El Hajj H, Novak SW, Sanchez MG, et al. Microglial physiological properties and interactions with synapses are altered at presymptomatic stages in a mouse model of huntington’s disease pathology. J Neuroinflamm (2020) 17(1):1–18. doi: 10.1186/s12974-020-01782-9
53. Semyanov A, Verkhratsky A. Astrocytic processes: From tripartite synapses to the active milieu. Trends Neurosci (2021) 44(10):781–92. doi: 10.1016/j.tins.2021.07.006
55. Aramideh JA, Vidal-Itriago A, Morsch M, Graeber MB. Cytokine signalling at the microglial penta-partite synapse. Int J Mol Sci (2021) 22(24):13186. doi: 10.3390/ijms222413186
56. Crapser JD, Arreola MA, Tsourmas KI, Green KN. Microglia as hackers of the matrix: Sculpting synapses and the extracellular space. Cell Mol Immunol (2021) 18(11):2472–88. doi: 10.1038/s41423-021-00751-3
57. Tang R, Dai J. Biophoton signal transmission and processing in the brain. J Photochem Photobiol B Biol (2014) 139:71–5. doi: 10.1016/j.jphotobiol.2013.12.008
58. Agnati LF, Guidolin D, Maura G, Marcoli M. Functional roles of three cues that provide nonsynaptic modes of communication in the brain: Electromagnetic field, oxygen, and carbon dioxide. J Neurophysiol (2018) 119(1):356–68. doi: 10.1152/jn.00413.2017
59. Picón-Pagès P, Garcia-Buendia J, Muñoz FJ. Functions and dysfunctions of nitric oxide in brain. Biochim Biophys Acta Mol Basis Dis (2019) 1865(8):1949–67. doi: 10.1016/j.bbadis.2018.11.007
60. Moro C, Liebert A, Hamilton C, Pasqual N, Jeffery G, Stone J, et al. The code of light: Do neurons generate light to communicate and repair? Neural Regener Res (2022) 17(6):1251–2. doi: 10.4103/1673-5374.327332
61. Beyer C, Christen P, Jelesarov I, Fröhlich J. Experimental system for real-time assessment of potential changes in protein conformation induced by electromagnetic fields. Bioelectromagnetics (2013) 34(6):419–28. doi: 10.1002/bem.21795
62. Scholkmann F. Two emerging topics regarding long-range physical signaling in neurosystems: Membrane nanotubes and electromagnetic fields. J Integr Neurosci (2015) 14(02):135–53. doi: 10.1142/S0219635215300115
63. Pall ML. Microwave frequency electromagnetic fields (EMFs) produce widespread neuropsychiatric effects including depression. J Chem Neuroanat (2016) 75:43–51. doi: 10.1016/j.jchemneu.2015.08.001
64. Liboff A. Magnetic correlates in electromagnetic consciousness. Electromagn Biol Med (2016) 35(3):228–36. doi: 10.3109/15368378.2015.1057641
65. Guidolin D, Ciruela F, Genedani S, Guescini M, Tortorella C, Albertin G, et al. Bioinformatics and mathematical modelling in the study of receptor–receptor interactions and receptor oligomerization: Focus on adenosine receptors. Biochim Biophys Acta (2011) 1808(5):1267–83. doi: 10.1016/j.bbamem.2010.09.022
66. Vaaga CE, Borisovska M, Westbrook GL. Dual-transmitter neurons: Functional implications of co-release and co-transmission. Curr Opin Neurobiol (2014) 29:25–32. doi: 10.1016/j.conb.2014.04.010
67. El Mestikawy S, Wallén-Mackenzie Å, Fortin GM, Descarries L, Trudeau L-E. From glutamate co-release to vesicular synergy: Vesicular glutamate transporters. Nat Rev Neurosci (2011) 12(4):204–16. doi: 10.1038/nrn2969
68. Hnasko TS, Edwards RH. Neurotransmitter co-release: Mechanism and physiological role. Annu Rev Physiol (2012) 74:225. doi: 10.1146/annurev-physiol-020911-153315
69. Kim S, Wallace ML, El-Rifai M, Knudsen AR, Sabatini BL. Co-Packaging of opposing neurotransmitters in individual synaptic vesicles in the central nervous system. Neuron (2022) 110(8):1371–84.e7. doi: 10.1016/j.neuron.2022.01.007
70. Upmanyu N, Jin J, von der Emde H, Ganzella M, Bösche L, Malviya VN, et al. Colocalization of different neurotransmitter transporters on synaptic vesicles is sparse except for VGLUT1 and ZnT3. Neuron (2022) 110(9):1483–97.e7. doi: 10.1016/j.neuron.2022.02.008
71. Tritsch NX, Granger AJ, Sabatini BL. Mechanisms and functions of GABA co-release. Nat Rev Neurosci (2016) 17(3):139–45. doi: 10.1038/nrn.2015.21
72. Guidolin D, Marcoli M, Tortorella C, Maura G, Agnati LF. G Protein-coupled receptor-receptor interactions give integrative dynamics to intercellular communication. Rev Neurosci (2018) 29(7):703–26. doi: 10.1515/revneuro-2017-0087
73. Agnati L, Guidolin D, Genedani S, Leo G, Woods SA, Ferrè S, et al. Integrative action of receptor mosaics: Relevance of receptor topology and allosteric modulators. J Recept Signal Transduct Res (2008) 28(6):543–65. doi: 10.1080/10799890802590420
74. Agnati LF, Guidolin D, Leo G, Carone C, Genedani S, Fuxe K. Receptor–receptor interactions: A novel concept in brain integration. Prog Neurobiol (2010) 90(2):157–75. doi: 10.1016/j.pneurobio.2009.10.004
75. Agnati LF, Guidolin D, Vilardaga JP, Ciruela F, Fuxe K. On the expanding terminology in the GPCR field: The meaning of receptor mosaics and receptor heteromers. J Recept Signal Transduct Res (2010) 30(5):287–303. doi: 10.3109/10799891003786226
76. Changeux JP, Christopoulos A. Allosteric modulation as a unifying mechanism for receptor function and modulation. Cell (2016) 166:1084–102. doi: 10.1016/j.cell.2016.08.015
77. Monod J. Chance and necessity: an essay on the natural philosophy of modern biology Vol. 199. . New York: Knopf (1971).
78. Fenton AW. Allostery: An illustrated definition for the ‘second secret of life’. Trends Biochem Sci (2008) 33(9):420–5. doi: 10.1016/j.tibs.2008.05.009
79. Cournia Z, Chatzigoulas A. Allostery in membrane proteins. Curr Opin Struct Biol (2020) 62:197–204. doi: 10.1016/j.sbi.2020.03.006
80. Agnati LF, Tarakanov AO, Guidolin D. A simple mathematical model of cooperativity in receptor mosaics based on the “symmetry rule”. BioSystems (2005) 80(2):165–73. doi: 10.1016/j.biosystems.2004.11.004
81. Guidolin D, Tortorella C, Marcoli M, Maura G, Agnati LF. Intercellular communication in the central nervous system as deduced by chemical neuroanatomy and quantitative analysis of images: Impact on neuropharmacology. Int J Mol Sci (2022) 23(10):5805. doi: 10.3390/ijms23105805
82. Hauser AS, Attwood MM, Rask-Andersen M, Schiöth HB, Gloriam DE. Trends in GPCR drug discovery: New agents, targets and indications. Nat Rev Drug Discovery (2017) 16(12):829–42. doi: 10.1038/nrd.2017.178
83. Nickols HH, Conn PJ. Development of allosteric modulators of GPCRs for treatment of CNS disorders. Neurobiol Dis (2014) 61:55–71. doi: 10.1016/j.nbd.2013.09.013
84. Guidolin D, Marcoli M, Tortorella C, Maura G, Agnati LF. Receptor-receptor interactions as a widespread phenomenon: Novel targets for drug development? Front Endocrinol (2019) 10:53. doi: 10.3389/fendo.2019.00053
85. Agnati LF, Baluška F, Barlow PW, Guidolin D. Mosaic, self-similarity logic and biological attraction principles: Three explanatory instruments in biology. Commun Integr Biol (2009) 2(6):552–63. doi: 10.4161/cib.2.6.9644
86. Kunishima N, Shimada Y, Tsuji Y, Sato T, Yamamoto M, Kumasaka T, et al. Structural basis of glutamate recognition by a dimeric metabotropic glutamate receptor. Nature (2000) 407(6807):971–7. doi: 10.1038/35039564
87. Doumazane E, Scholler P, Zwier JM, Trinquet E, Rondard P, Pin JP. A new approach to analyze cell surface protein complexes reveals specific heterodimeric metabotropic glutamate receptors. FASEB J (2011) 25(1):66–77. doi: 10.1096/fj.10-163147
88. Wu H, Wang C, Gregory KJ, Han GW, Cho HP, Xia Y, et al. Structure of a class c GPCR metabotropic glutamate receptor 1 bound to an allosteric modulator. Science (2014) 344(6179):58–64. doi: 10.1126/science.1249489
89. Papasergi-Scott MM, Robertson MJ, Seven AB, Panova O, Mathiesen JM, Skiniotis G. Structures of metabotropic GABAB receptor. Nature (2020) 584(7820):310–4. doi: 10.1038/s41586-020-2469-4
90. Navarro G, Ferré S, Cordomi A, Moreno E, Mallol J, Casadó V, et al. Interactions between intracellular domains as key determinants of the quaternary structure and function of receptor heteromers. J Biol Chem (2010) 285(35):27346–59. doi: 10.1074/jbc.M110.115634
91. Navarro G, Cordomí A, Zelman-Femiak M, Brugarolas M, Moreno E, Aguinaga D, et al. Quaternary structure of a G-protein-coupled receptor heterotetramer in complex with gi and gs. BMC Biol (2016) 14(1):1–12. doi: 10.1186/s12915-016-0247-4
92. Navarro G, Cordomí A, Brugarolas M, Moreno E, Aguinaga D, Pérez-Benito L, et al. Cross-communication between gi and gs in a G-protein-coupled receptor heterotetramer guided by a receptor c-terminal domain. BMC B (2018) 16(1):1–15. doi: 10.1186/s12915-018-0491-x
93. Gnad T, Navarro G, Lahesmaa M, Reverte-Salisa L, Copperi F, Cordomi A, et al. Adenosine/A2B receptor signaling ameliorates the effects of aging and counteracts obesity. Cell Metab (2020) 32(1):56–70.e7. doi: 10.1016/j.cmet.2020.06.006
94. Franco R, Cordomí A, Llinas del Torrent C, Lillo A, Serrano-Marín J, Navarro G, et al. Structure and function of adenosine receptor heteromers. Cell Mol Life Sci (2021) 78(8):3957–68. doi: 10.1007/s00018-021-03761-6
95. Casanovas M, Jiménez-Rosés M, Cordomí A, Lillo A, Vega-Quiroga I, Izquierdo J, et al. Discovery of a macromolecular complex mediating the hunger suppressive actions of cocaine: Structural and functional properties. Addict Biol (2021) 26(5):e13017. doi: 10.1111/adb.13017
96. Benfenati F, Agnati L, Fuxe K. Development of computer-assisted simulation procedure to analyze receptor modulatory processes. J Neural Transm (1988) 71(2):143–58. doi: 10.1007/BF01245256
97. Agnati L, Fuxe K, Zoli M, Pich EM, Benfenati F, Zini I, et al. Aspects on the information handling by the central nervous system: Focus on cotransmission in the aged rat brain. Prog Brain Res (1986) 68:291–301. doi: 10.1016/s0079-6123(08)60245-9
98. Agnati LF, Fuxe K, Benfenati F, Battistini N, Harfstrand A, Tatemot K, et al. Neuropeptide y in vitro selectively increases the number of α2-adrenergic binding sites in membranes of the medulla oblongata of the rat. Acta Physiol Scand (1983) 118(3):293–5. doi: 10.1111/j.1748-1716.1983.tb07273.x
99. Agnati LF, Fuxe K, Benfenati F, Battistini N, Harfstrand A, Hokfelt T, et al. Failure of neuropeptide y in vitro to increase the number of α2-adrenergic binding sites in membranes of medulla oblongata of the spontaneous hypertensive rat. Acta Physiol Scand (1983) 119:309–12. doi: 10.1111/j.1748-1716.1983.tb07344.x
100. Agnati LF, Fuxe K, Giardino L, Calza L, Zoli M, Battistini N, et al. Evidence for cholecystokinin-dopamine receptor interactions in the central nervous system of the adult and old rat. studies on their functional meaning. Ann N Y Acad Sci (1985) 448:315–33. doi: 10.1111/j.1749-6632.1985.tb29927.x
101. Fuxe K, Agnati LF, Martire M, Neumeyer A, Benfenati F, Frey P. Studies of neurotensin-dopamine receptor interactions in striatal membranes of the male rat. the influence of 6-hydroxydopamine-induced dopamine receptor supersensitivity. Acta Physiol Scand (1986) 126(1):147–9. doi: 10.1111/j.1748-1716.1986.tb07798.x
102. Gomes I, Ayoub MA, Fujita W, Jaeger WC, Pfleger KD, Devi LA. G Protein–coupled receptor heteromers. Annu Rev Pharmacol Toxicol (2016) 56:403. doi: 10.1146/annurev-pharmtox-011613-135952
103. Dehner C. Non-allosteric proteins. why do proteins have quaternary structure? In: Wells RD. Bond JS, Klinman J, Masters BSS, editors. Molecular life sciences. New York: Springer (2018). p. 812–8. doi: 10.1007/978-1-4614-1531-2_21
104. Guescini M, Genedani S, Stocchi V, Agnati LF. Astrocytes and glioblastoma cells release exosomes carrying mtDNA. J Neural Transm (Vienna) (2010) 117(1):1–4. doi: 10.1007/s00702-009-0288-8
105. Agnati LF, Guidolin D, Carone C, Dam M, Genedani S, Fuxe K. Understanding neuronal molecular networks builds on neuronal cellular network architecture. Brain Res Rev (2008) 58(2):379–99. doi: 10.1016/j.brainresrev.2007.11.002
106. Jordan BA, Devi LA. G-Protein-coupled receptor heterodimerization modulates receptor function. Nature (1999) 399(6737):697–700. doi: 10.1038/21441
107. George SR, Fan T, Xie Z, Tse R, Tam V, Varghese G, et al. Oligomerization of μ-and δ-opioid receptors: Generation of novel functional properties. J Biol Chem (2000) 275(34):26128–35. doi: 10.1074/jbc.M000345200
108. Rashid AJ, So CH, Kong MM, Furtak T, El-Ghundi M, Cheng R, et al. D1–D2 dopamine receptor heterooligomers with unique pharmacology are coupled to rapid activation of Gq/11 in the striatum. Proc Natl Acad Sci USA (2007) 104(2):654–9. doi: 10.1073/pnas.0604049104
109. Agnati LF, Fuxe K, Woods AS, Genedani S, Guidolin D. Theoretical considerations on the topological organization of receptor mosaics. Curr Protein Pept Sci (2009) 10(6):559–69. doi: 10.2174/138920309789630606
110. Sporns O. The human connectome: Origins and challenges. Neuroimage (2013) 80:53–61. doi: 10.1016/j.neuroimage.2013.03.023
111. Bassett DS, Sporns O. Network neuroscience. Nat Neurosci (2017) 20(3):353–64. doi: 10.1038/nn.4502
112. Agnati LF, Fuxe K. 2014 extracellular-vesicle type of volume transmission and tunnelling-nanotube type of wiring transmission add a new dimension to brain neuro-glial networks. Philos Trans R Soc Lond B Biol Sci (2014) 369(1652):20130505. doi: 10.1098/rstb.2013.0505
113. Franco R, Lillo A, Rivas-Santisteban R, Reyes-Resina I, Navarro G. Microglial adenosine receptors: From preconditioning to modulating the M1/M2 balance in activated cells. Cells (2021) 10(5):1124. doi: 10.3390/cells10051124
114. Borea PA, Gessi S, Merighi S, Vincenzi F, Varani K. Pharmacology of adenosine receptors: The state of the art. Physiol Rev (2018) 98(3):1591–625. doi: 10.1152/physrev.00049.2017
115. Zarrinmayeh H, Territo PR. Purinergic receptors of the central nervous system: Biology, PET ligands, and their applications. Mol Imaging (2020) 19:1536012120927609. doi: 10.1177/1536012120927609
116. Korkutata M, Saitoh T, Feng D, Murakoshi N, Sugiyama F, Cherasse Y, et al. Allosteric modulation of adenosine A2A receptors in mice induces slow-wave sleep without cardiovascular effects. Sleep Med (2017) 40:e181. doi: 10.1016/j.sleep.2017.11.530
117. Korkutata M, Saitoh T, Cherasse Y, Ioka S, Duo F, Qin R, et al. Enhancing endogenous adenosine A2A receptor signaling induces slow-wave sleep without affecting body temperature and cardiovascular function. Neuropharmacology (2019) 144:122–32. doi: 10.1016/j.neuropharm.2018.10.022
118. Ferré S, Díaz-Ríos M, Salamone JD, Prediger RD. New developments on the adenosine mechanisms of the central effects of caffeine and their implications for neuropsychiatric disorders. J Caffeine Adenosine Res (2018) 8(4):121–30. doi: 10.1089/caff.2018.0017
119. Domenici MR, Ferrante A, Martire A, Chiodi V, Pepponi R, Tebano MT, et al. Adenosine A2A receptor as potential therapeutic target in neuropsychiatric disorders. Pharmacol Res (2019) 147:104338. doi: 10.1016/j.phrs.2019.104338
120. Wall M, Dale N. Activity-dependent release of adenosine: A critical re-evaluation of mechanism. Curr Neuropharmacol (2008) 6(4):329–37. doi: 10.2174/157015908787386087
121. Lovatt D, Xu Q, Liu W, Takano T, Smith NA, Schnermann J, et al. Neuronal adenosine release, and not astrocytic ATP release, mediates feedback inhibition of excitatory activity. Proc Natl Acad Sci USA (2012) 109(16):6265–70. doi: 10.1073/pnas.1120997109
122. Corti F, Cellai L, Melani A, Donati C, Bruni P, Pedata F. Adenosine is present in rat brain synaptic vesicles. Neuroreport (2013) 24(17):982–7. doi: 10.1097/WNR.0000000000000033
123. Nguyen MD, Venton BJ. Fast-scan cyclic voltammetry for the characterization of rapid adenosine release. Comput Struct Biotechnol J (2015) 13:47–54. doi: 10.1016/j.csbj.2014.12.006
124. Chin JH. Adenosine receptors in brain: Neuromodulation and role in epilepsy. Ann Neurol (1989) 26:695–8. doi: 10.1002/ana.410260602
125. Ciruela F, Casadó V, Rodrigues RJ, Luján R, Burgueño J, Canals M, et al. Presynaptic control of striatal glutamatergic neurotransmission by adenosine A1-A2A receptor heteromers. J Neurosci (2006) 26:2080–7. doi: 10.1523/JNEUROSCI.3574-05.2006
126. Matos M, Augusto E, Santos-Rodrigues AD, Schwarzschild MA, Chen J-F, Cunha RA, et al. Adenosine A2A receptors modulate glutamate uptake in cultured astrocytes and gliosomes. Glia (2012) 60:702–16. doi: 10.1002/glia.22290
127. Kamikubo Y, Shimomura T, Fujita Y, Tabata T, Kashiyama T, Sakurai T, et al. Functional cooperation of metabotropic adenosine and glutamate receptors regulates postsynaptic plasticity in the cerebellum. J Neurosci (2013) 33:18661–71. doi: 10.1523/JNEUROSCI.5567-12.2013
128. Zhou X, Oishi Y, Cherasse Y, Korkutata M, Fujii S, Lee C-Y, et al. Extracellular adenosine and slow-wave sleep are increased after ablation of nucleus accumbens core astrocytes and neurons in mice. Neurochem Int (2019) 124:256–63. doi: 10.1016/j.neuint.2019.01.020
129. Korkutata M, Agrawal L, Lazarus M. Allosteric modulation of adenosine A2A receptors as a new therapeutic avenue. Int J Mol Sci (2022) 23(4):2101. doi: 10.3390/ijms23042101
130. Ciruela F, Ferré S, Casadó V, Cortés A, Cunha RA, Lluis C, et al. Heterodimeric adenosine receptors: A device to regulate neurotransmitter release. Cell Mol Life Sci (2006) 63(21):2427–31. doi: 10.1007/s00018-006-6216-2
131. Fuxe K, Marcellino D, Rivera A, Diaz-Cabiale Z, Filip M, Gago B, et al. Receptor-receptor interactions within receptor mosaics. impact on neuropsychopharmacology. Brain Res Rev (2008) 58(2):415–52. doi: 10.1016/j.brainresrev.2007.11.007
132. Vecchio EA, Baltos JA, Nguyen ATN, Christopoulos A, White PJ, May LT. New paradigms in adenosine receptor pharmacology: Allostery, oligomerization and biased agonism. Br J Pharmacol (2018) 175(21):4036–46. doi: 10.1111/bph.14337
133. Wydra K, Gawliński D, Gawlińska K, Frankowska M, Borroto-Escuela DO, Fuxe K, et al. Adenosine A2AReceptors in substance use disorders: A focus on cocaine. Cells (2020) 9(6):1372. doi: 10.3390/cells9061372
134. Nguyen KDQ, Vigers M, Sefah E, Seppälä S, Hoover JP, Schonenbach NS, et al. Homo-oligomerization of the human adenosine A2A receptor is driven by the intrinsically disordered c-terminus. Elife (2021) 10:e66662. doi: 10.7554/eLife.66662
135. Prasad K, de Vries EFJ, Elsinga PH, Dierckx RAJO, van Waarde A. Allosteric interactions between adenosine A2A and dopamine D2 receptors in heteromeric complexes: Biochemical and pharmacological characteristics, and opportunities for PET imaging. Int J Mol Sci (2021) 22(4):1719. doi: 10.3390/ijms22041719
136. Cervetto C, Venturini A, Guidolin D, Maura G, Passalacqua M, Tacchetti C, et al. Homocysteine and A2A-D2 receptor-receptor interaction at striatal astrocyte processes. J Mol Neurosci (2018) 65(4):456–66. doi: 10.1007/s12031-018-1120-4
137. Pelassa S, Guidolin D, Venturini A, Averna M, Frumento G, Campanini L, et al. A2A-D2 heteromers on striatal astrocytes: Biochemical and biophysical evidence. Int J Mol Sci (2019) 20(10):2457. doi: 10.3390/ijms20102457
138. Christopoulos A, Changeux J-P, Catterall WA, Fabbro D, Burris TP, Cidlowski JA, et al. International union of basic and clinical pharmacology. XC. multisite pharmacology: Recommendations for the nomenclature of receptor allosterism and allosteric ligands. Pharmacol Rev (2014) 66(4):918–47. doi: 10.1124/pr.114.008862
139. Franco R, Ferré S, Agnati L, Torvinen M, Ginés S, Hillion J, et al. Evidence for adenosine/dopamine receptor interactions: Indications for heteromerization. Neuropsychopharmacology (2000) 23(4 Suppl):S50–S9. doi: 10.1016/S0893-133X(00)00144-5
140. Hillion J, Canals M, Torvinen M, Casadó V, Scott R, Terasmaa A, et al. Coaggregation, cointernalization, and codesensitization of adenosine A2A receptors and dopamine D2Receptors. J Biol Chem (2002) 277(20):18091–7. doi: 10.1074/jbc.M107731200
141. Agnati LF, Genedani S, Leo G, Guidolin D, Filaferro M, Carriba P, et al. Allosteric modulation of dopamine D2 receptors by homocysteine. J Proteome Res (2006) 5(11):3077–83. doi: 10.1021/pr0601382
142. Lan H, DuRand CJ, Teeter MM, Neve KA. Structural determinants of pharmacological specificity between D1 and D2 dopamine receptors. Mol Pharmacol (2006) 69(1):185–94. doi: 10.1124/mol.105.017244
143. Guidolin D, Marcoli M, Tortorella C, Maura G, Agnati LF. Adenosine A2A-dopamine D2 receptor-receptor interaction in neurons and astrocytes: Evidence and perspectives. Prog Mol Biol Transl Sci (2020) 169:247–77. doi: 10.1016/bs.pmbts.2019.11.004
144. Daniels DJ, Kulkarni A, Xie Z, Bhushan RG, Portoghese PS. A bivalent ligand (KDAN-18) containing δ-antagonist and κ-agonist pharmacophores bridges δ2 and κ1 opioid receptor phenotypes. J Med Chem (2005) 48(6):1713–6. doi: 10.1021/jm034234f
145. Daniels DJ, Lenard NR, Etienne CL, Law P-Y, Roerig SC, Portoghese PS. Opioid-induced tolerance and dependence in mice is modulated by the distance between pharmacophores in a bivalent ligand series. Proc Natl Acad Sci USA (2005) 102(52):19208–13. doi: 10.1073/pnas.0506627102
146. Soriano A, Ventura R, Molero A, Hoen R, Casadó V, Cortés A, et al. Adenosine A2A receptor-antagonist/dopamine D2 receptor-agonist bivalent ligands as pharmacological tools to detect A2A-D2 receptor heteromers. J Med Chem (2009) 52(18):5590–602. doi: 10.1021/jm900298c
147. Hiller C, Kühhorn J, Gmeiner P. Class a G-protein-coupled receptor (GPCR) dimers and bivalent ligands. J Med Chem (2013) 56(17):6542–59. doi: 10.1021/jm4004335
148. Joürg M, May LT, Mak FS, Lee KCK, Miller ND, Scammells PJ, et al. Synthesis and pharmacological evaluation of dual acting ligands targeting the adenosine A2A and dopamine D2 receptors for the potential treatment of parkinson’s disease. J Med Chem (2015) 58(2):718–38. doi: 10.1021/jm501254d
149. Busnelli M, Kleinau G, Muttenthaler M, Stoev S, Manning M, Bibic L, et al. Design and characterization of superpotent bivalent ligands targeting oxytocin receptor dimers via a channel-like structure. J Med Chem (2016) 59(15):7152–66. doi: 10.1021/acs.jmedchem.6b00564
150. Morales P, Navarro G, Gómez-Autet M, Redondo L, Fernández-Ruiz J, Pérez-Benito L, et al. Discovery of homobivalent bitopic ligands of the cannabinoid CB2 receptor. Chemistry (2020) 26(68):15839–42. doi: 10.1002/chem.202003389
151. Aceto MD, Harris LS, Negus SS, Banks ML, Hughes LD, Akgün E, et al. MDAN-21: A bivalent opioid ligand containing mu-agonist and delta-antagonist pharmacophores and its effects in rhesus monkeys. Int J Med Chem (2012) 2012:327257. doi: 10.1155/2012/327257
152. Huang B, Onge CMS, Ma H, Zhang Y. Design of bivalent ligands targeting putative GPCR dimers. Drug Discovery Today (2021) 26(1):189–99. doi: 10.1016/j.drudis.2020.10.006
153. Ullmann T, Gienger M, Budzinski J, Hellmann J, Hübner H, Gmeiner P, et al. Homobivalent dopamine D2 receptor ligands modulate the dynamic equilibrium of D2 monomers and homo-and heterodimers. ACS Chem Biol (2021) 16(2):371–9. doi: 10.1021/acschembio.0c00895
154. Suzuki S, Chuang LF, Yau P, Doi RH, Chuang RY. Interactions of opioid and chemokine receptors: Oligomerization of mu, kappa, and delta with CCR5 on immune cells. Exp Cell Res (2002) 280(2):192–200. doi: 10.1006/excr.2002.5638
155. Chen C, Li J, Bot G, Szabo I, Rogers TJ, Liu-Chen L-Y. Heterodimerization and cross-desensitization between the μ-opioid receptor and the chemokine CCR5 receptor. Europ J Pharmacol (2004) 483(2-3):175–86. doi: 10.1016/j.ejphar.2003.10.033
156. Pello OM, Martínez-Muñoz L, Parrillas V, Serrano A, Rodríguez-Frade JM, Toro MJ, et al. Ligand stabilization of CXCR4/δ-opioid receptor heterodimers reveals a mechanism for immune response regulation. Europ J Immunol (2008) 38(2):537–49. doi: 10.1002/eji.200737630
Keywords: allosteric modulators, brain connectomics, drug targets, G-protein coupled receptors (GPCRs), neuropsychiatric disorders, penta-partite synapse, receptor heteromerization, receptor mosaics
Citation: Marcoli M, Agnati LF, Franco R, Cortelli P, Anderlini D, Guidolin D, Cervetto C and Maura G (2023) Modulating brain integrative actions as a new perspective on pharmacological approaches to neuropsychiatric diseases. Front. Endocrinol. 13:1038874. doi: 10.3389/fendo.2022.1038874
Received: 07 September 2022; Accepted: 20 December 2022;
Published: 09 January 2023.
Edited by:
Alain Couvineau, Institut National de la Santé et de la Recherche Médicale (INSERM), FranceReviewed by:
Alicia Rivera, University of Malaga, SpainCopyright © 2023 Marcoli, Agnati, Franco, Cortelli, Anderlini, Guidolin, Cervetto and Maura. This is an open-access article distributed under the terms of the Creative Commons Attribution License (CC BY). The use, distribution or reproduction in other forums is permitted, provided the original author(s) and the copyright owner(s) are credited and that the original publication in this journal is cited, in accordance with accepted academic practice. No use, distribution or reproduction is permitted which does not comply with these terms.
*Correspondence: Manuela Marcoli, bWFudWVsYS5tYXJjb2xpQHVuaWdlLml0; Luigi F. Agnati, bHVpZ2kuYWduYXRpQGdtYWlsLmNvbQ==
†These authors have contributed equally to this work
Disclaimer: All claims expressed in this article are solely those of the authors and do not necessarily represent those of their affiliated organizations, or those of the publisher, the editors and the reviewers. Any product that may be evaluated in this article or claim that may be made by its manufacturer is not guaranteed or endorsed by the publisher.
Research integrity at Frontiers
Learn more about the work of our research integrity team to safeguard the quality of each article we publish.