- 1Department of Tumor Biological Treatment, The Third Affiliated Hospital of Soochow University, Changzhou, China
- 2Department of Oncology, Yangzhou University, Yangzhou, China
- 3Department of Oncology, First People’s Hospital of Changzhou, Changzhou, China
Bone metastasis is closely related to the survival rate of cancer patients and reduces their quality of life. The bone marrow microenvironment contains a complex immune cell component with a local microenvironment that is conducive to tumor formation and growth. In this unique immune environment, a variety of immune cells, including T cells, natural killer cells, macrophages, dendritic cells, and myeloid-derived suppressor cells, participate in the process of bone metastasis. In this review, we will introduce the interactions between immune cells and cancer cells in the bone microenvironment, obtain the details of their contributions to the implications of bone metastasis, and discuss immunotherapeutic strategies targeting immune cells in cancer patients with bone metastasis.
Introduction
With the rise in morbidity due to cancer, bone metastasis has become the main reason for the death rate of people affected by carcinoma. The bone is one of the most important pathological process organs for various solid neoplasms, such as breast, lung, and prostate cancer (1). Although improvements have been made in the diagnosis and therapy of neoplasms, bone metastasis remains insurmountable. The formation and evolution of bone metastasis include involved communication occurring among tumor cells, immune cells, and osteocytes (2). In the spinal marrow, osteoblasts or osteoclasts release numerous growth factors that boost the expansion of metastatic tumors, leading to incurable osteoblastic or osteolytic lesions (3). The immune system is the primary defense system against tumor cells, and its effects on spinal metastasis are still unknown. Earlier studies concentrated on the interaction between tumor cells and bone progenitor cells, and recapitulating specific tumor cell–bone microenvironment interactions is lacking in in-vivo models. However, increasing evidence indicates that metastasis might rely on uncommon constraints in the tumor microenvironment (4). The antitumor or protumor impact of the immune microenvironment might rely on the existence of the regional cytokine milieu, tumor-specific interplay, and specific types of immune cells (Figure 1). Within the existing review, the elaborate functions and impacts of different immune cells on bone metastasis will be introduced. In addition, the existing therapeutic methods for bone metastasis will be presented.
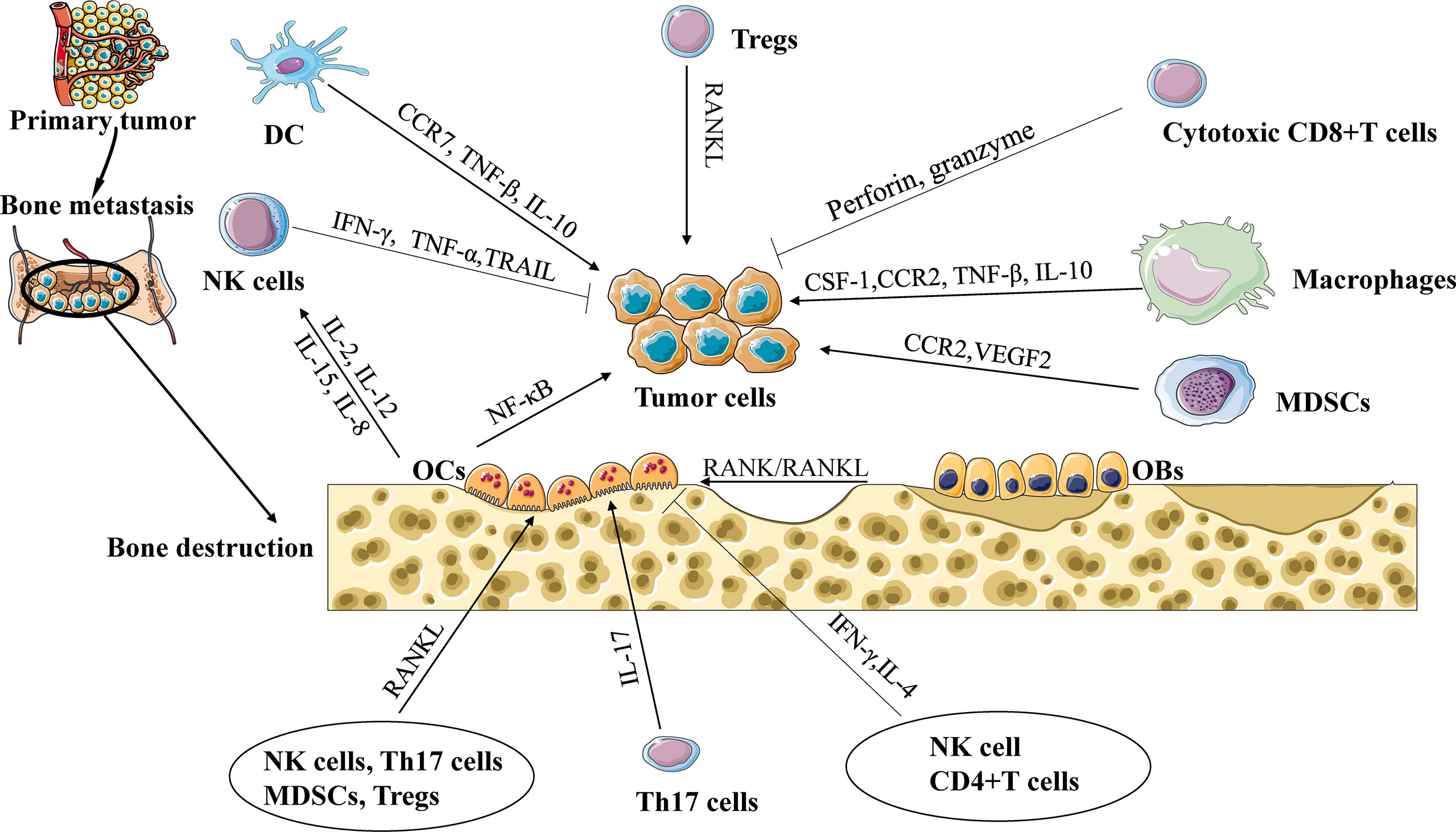
Figure 1 The interaction of immune cells, tumor cells, and osteocytes during bone metastasis. Cytotoxic CD8+ T cells release TNF-α and IFN-γ to eliminate tumor cells. Regulatory T cells (Tregs) promote tumor cell to bone metastasis through the RANK/RANKL axis. Tumor-associated macrophages (TAMs) promote tumor cell to bone metastasis through CCL2/CCR2 or CSF-1/ CSF-1R signaling. Natural killer cells (NK cells) can destroy tumor cells by secreting TNF-α and tumor necrosis factor-related apoptosis-inducing ligand. Dendritic cells (DCs) and TAMs suppress the cytotoxic capacity of CD8+ T cells via TGF-β, and interleukin-10 (IL-10) to promote tumor progression. Myeloid-derived suppressor cells (MDSCs) release chemokines, including vascular endothelial growth factor (VEGF) and CCL2/CCR2 signaling, to promote cancer progression and bone metastasis. IL-17 can also increase bone metastases, while IFN-γ and IL-4 secreted from Th1 and Th2 cells, respectively, can inhibit osteoclast formation and limit bone metastases. NK cells and CD4+ T cells support or repress the production of OCs controlled by the release of TNF-α or IFN-γ.
Interaction of tumor cells and immune cells in the bone microenvironment
The onset of neoplasm invasion of the bone leads to decoupled bone loss and formation, an essential process elicited by tumor cells and directed by osteoblasts (OBs) and osteoclasts (OCs) (5). Osteoblasts and osteoclasts are two major cell varieties in the bone microenvironment that promote bone metastasis. Tumor cells release signaling molecules that promote the differentiation of OCs and OBs (6), thus establishing the regeneration of the resultant tumor adhesion, proliferation, and increased bone mass (7). Disseminated tumor cells (DTCs) need to escape immune tolerance by migrating from the primary tumor to the bone. Once DTCs enter the bone marrow, they will proliferate or go dormant (8). In fact, whether DTCs escape from a dormant state is determined by factors released by the bone microenvironment (9), physical factors (10), and the activity of OBs and OCs (11). Evidence indicates that the stem cell released by OCs might trigger the nuclear factor-κB (NF-κB) pathway to induce tumor cell responses (12). Interleukin (IL)-6 plays a functional role in mediating the crosstalk between primary tumors and the bone marrow to promote monocyte-dendritic progenitors to give rise to immunosuppressive macrophages which, in turn, promote metastasis in vivo (13). An elementary molecule connected with the immune system and bone is supported by the specific axis involving RANKL, RANKL, and osteoprotegerin (14). This interaction of RANK/RANKL provokes osteoclast generation, whereas osteoprotegerin (OPG) offsets this impact by interacting with RANK (15). There is proof that interferon-γ (IFN-γ) created by stimulated CD4+ T cells might repress the common activity of osteoclasts (16). In contrast, activated regulatory T cells (Tregs) and type 17 T helper cells (Th17 cells) induce a protumor effect via immune repressions and osteoclast differentiation via RANKL (17).
Effect of immune cells on bone metastasis
T cells
T cells are derived from hematopoietic stem cells and lymphoid precursors kept in the spinal marrow. The thymus is the place where T cells experience early differentiation and are then kept in secondary lymphoid organs, where they are aroused by antigen presentation. Classical T helper 1 (Th1), 2 (Th2), or 17 (Th17) cells or induced T regulatory cells (Tregs) are distinct subsets polarized by naive CD4+ T cells (Th0), as demonstrated by the cytokines they will be recruited to (18). In CD4+ T cells, as latent immune inhibitors, Tregs play a vital role in the balance of the immune system (19). Interestingly, a salient increase in Tregs in the spinal marrow was observed following potential contact with metastatic prostate cancer, which is perceived to inhibit osteoclast formation and bone resorption (20). In addition to having immunological disorder functions, FOXP3+ Tregs are indisputably a significant source of RANKL (21). RANKL is the vital cytokine needed for osteoclast differentiation and tumor cell migrating to the bone (22), indicating that RANKL+ Tregs might stimulate DTC recruitment. Evidence suggests that tumor-specific Th17 cells can promote osteoclast activation and produce RANKL to induce osteolytic bone lesions (17). IL-17 can also increase bone metastases, while IFN-γ and IL-4 secreted from Th1 and Th2 cells, respectively, can inhibit osteoclast formation and limit bone metastases (23). CD8+ T cells can destroy tumor cells by secreting cytotoxic proteins (perforin and granzyme) through the Fas–Fas ligand axis mediating apoptosis (4). Furthermore, non-activated T cells increase osteoclastogenesis, whereas activated T cells are essential effectors in the protective impact against skeletal metastasis (24, 25).
The spinal marrow is a repository for the recruitment of memory CD4+ T cells (26) and CD8+ T cells (27), in which bone-derived cytokines, such as IL-7, influence them. Under the control of IL-7, the bone marrow induce the differentiation of CD4+ and CD8+ T cells from effector to memory T cells. (28). Feuerer et al. suggested that the number of memory CD4+ and CD8+ T cells in the spinal marrow of people with breast neoplasms is increased compared with that in healthy individuals (29).
Macrophages
Macrophages are derived from spinal marrow progenitor cells derived from the yolk sac (30). Similarly, polarized macrophages with a pro-remodeling M2 or pro-inflammatory M1 phenotype were assessed (31). Inflammatory macrophages are attracted to the tumor microenvironment, are referred to as tumor-associated macrophages (TAMs), and are related to unfavorable prognoses in solid cancers (32). T-cell immunoglobulin and mucin domain-containing protein 4 (Tim4+) on TAMs could trap and inhibit CD8+ T-cell cytotoxicity and proliferation for promoting metastasis (33, 34). Macrophages are a major component in the tumor microenvironment arising from spinal marrow-derived monocyte differentiation in response to CC chemokine 2 (CCL2/CCR2) (35) and colony-stimulating factor 1 (CSF-1/CSF-1R). Furthermore, CSF-1 has been verified to be involved in macrophage-driven bone metastasis (36). Cellular adhesion and motility in macrophages are regulated by CCL2–CCR2 signaling (37). Breast tumor cells expressing CCL2 bind to monocyte-derived CCR2+ stromal cells, including macrophages and preosteoclast cells, to promote colonization in the lungs and bone (38). In prostate cancer, TAMs promote the invasion of tumor cells via CCL2–CCR2 signaling (39). In addition, recent studies have demonstrated that bone tumor growth can be weakened by the repression of macrophage-recruiting factors and TAM reprogramming from M2 to M1 (40). TAM-derived transforming growth factor-β (TGF-β) could facilitate the invasion of colorectal cancer cells (41). On the other hand, the final TAM molecular theories in the impetus of skeletal metastasis have not yet been represented. Simultaneously, another type of macrophage, called metastatic-associated macrophages (MAMs), is vital to motivate the generation of growth factors and inhibit T-cell antitumor responses (42).
NK cells
NK cells experience upgrowth and become divided from CD34+ progenitors in the spinal marrow. The FcγIII− receptor (CD16) is expressed by most NK cells, which pushes NK cells to mediate antibody-dependent cellular cytotoxicity (ADCC). NK cells can destroy tumor cells by secreting TNF-α and tumor necrosis factor-related apoptosis-inducing ligand (43), or cytokines, which are capable of reducing tumor cell proliferation and accelerating the inflammatory response, such as IFN-γ. In addition, NK cells are able to secrete chemokines to attract T cells, dendritic cells, and monocytes (44), leading to a specific crosstalk in the adaptive antitumor response (45). Leukemia cells can inhibit NK cells via RANKL signaling (46). However, OCs can be stimulated by NK cells via motivating the RANKL pathway under inflammatory conditions (47). NK cells support or repress the production of OCs controlled by the release of TNF-α or IFN-γ, respectively (48). Furthermore, NK cells appear to produce IFN-γ in response to a target combination of foreign cytokines such as IL-2, IL-12, IL-15, and IL-18.
Dendritic cells
DCs originate from common myeloid progenitors (CMPs), which differentiate into common dendritic cell progenitors (CDPs) in the absence of the transcription factor Nur77, resulting in the generation of plasmacytoid DCs (pDCs) and conventional DCs (cDCs) (49). The transportation of cDCs, which are divided into cDC1 and cDC2, to lymph nodes and the production of a systemic antineoplastic immune response are controlled by C-C chemokine receptor 7 (CCR7) expression (50). The cDC1 subset has the unique capability to cross-present a foreign antigen, thereby activating Foxp3+ CD8+ Tregs (51, 52). cDC2s are responsible for presenting foreign antigens to CD4+ T cells and shaping the polarization of cells (53). Essentially, a consanguineous correlation between cDC2 abundance and non-Treg CD4+ T-cell infiltration into head and neck squamous carcinomas is demonstrated. For example, longer progression-free survival was associated with supernal cDC2s and poor Treg infiltration (54). pDCs seem to be primarily tolerated in the background of cancer and are associated with a negative prognosis (55). The upregulation of MHC molecules and costimulatory molecules is induced by enabled pDCs, which can still activate CD4+ and CD8+ T cells. It has also been found that tumor-infiltrating DCs and TAMs suppress the cytotoxic capacity of CD8+ T cells via the production of TGF-β and IL-10 (2). Similarly, pDCs recruit other immunonegative immune cells involving Tregs, and myeloid-derived suppressor cells (MDSCs) promote, but do not protect, tumor progression and metastasis (56). Subsequently, Sawant et al. reported an increasing number of pDCs in the spinal marrow of mice inoculated with breast 4T1 carcinoma cells (57); thus, therapeutically targeting pDCs might hold promise for treating bone metastasis.
MDSCs
As a heterogeneous group of immature myeloid cells, MDSCs are derived from the spinal marrow. MDSCs are made up of two large groups: granulocytic or polymorphonuclear MDSCs (PMN-MDSCs) and monocytic MDSCs (M-MDSCs). Importantly, breast, ovarian, and gastric human neoplasms cultured in vitro secrete CCL2, and MDSCs from these patients express the relevant CCR2 and migrate toward these chemokines in vitro (58). Deletion of CCL2 in a mouse model of spontaneous colorectal neoplasm diminished the number of colonic MDSCs (59). PD-L1+ M-MDSCs can differentiate into osteoclasts and are potent suppressors of T-cell activation (25). In addition to effector T-cell groups, these data suggest that MDSCs might affect the expansion and activation of Tregs and conversely mediate immunosuppression. MDSCs from the bone microenvironment with bone metastases can subsequently differentiate into functional osteoclasts, and without bone metastases, they fail to differentiate into osteoclasts, which illustrates that tumor cells residing in the bone microenvironment lead to an increased quantity of activated osteoclasts (60, 61). Enhanced levels of MDSCs were found in the blood of patients with breast (62) and prostate neoplasms (63), which is correlated with the tumor stage. The higher the level of circulating MDSCs in patients with breast and prostate cancer, the lower the overall survival (64). Considering the potent impacts of MDSCs on destroying host immunity and quickening bone damage, MDSCs might become a latent therapeutic target for bone metastasis.
Potential of modulating the immune system in the treatment of bone metastasis
Targeting T cells
T cells can express receptors, such as cytotoxic T lymphocyte-associated protein 4 (CTLA-4) or apoptotic process protein 1 (PD-1), and when they interact with ligands, T cells lose activity. The monoclonal antibodies that intercept CTLA-4, PD-1, or PD-L1 show significant clinical results in patients with multiple neoplasms involving advanced melanoma (65) and non-small cell lung neoplasms (66). The anti-CTLA-4 antagonists and anti-PD-1 antibody nivolumab can block the inhibitory function of Tregs in vitro, as demonstrated in mice (67). Tim-4 inhibition significantly improves antitumor effectiveness in mouse models of anti-PD-1 treatment (34). Emerging data suggest that the androgen receptor is a negative regulator of CD8+ T cells in responding to anti-PD-1/PD-L1 treatment (68). Moreover, the usage of sunitinib and sorafenib which aims at VEGFR2 decreases the percentage of Tregs in foreign blood (69). To boost the immune response against tumors, CD8+ T cells are stimulated by vaccination or engineering T cells to express tumor-specific T-cell receptors (TCRs) or chimeric antigen receptors (CARs) (4). Engineered T cells were still detectable 9 months after transplantation in three patients in a phase I trial, and the number of cancer cells in two patients with refractory advanced myeloma was reduced. This result highlights the feasibility and therapeutic capacity of engineering cancer-specific T cells to attack cancer in the bone microenvironment (70). Clinical studies have not shown whether bone metastases can be reduced or eliminated by engineered T cells.
Targeting macrophages
In view of the crucial character of macrophages in influencing bone metastasis, targeting macrophages would be an essential approach for skeletal metastasis therapy. Several therapeutic antibodies and molecules alone or in combination with other treatments are used to target TAMs. These treatments include depletion, reprogramming, and molecular targeting.
Inhibiting CSF-1/CSF-1R signaling and using liposomes containing clodronate are the most studied therapeutic approaches to remove TAMs from the tumor microenvironment. Anti-CD115 antibody (CSF-1R antibody) treatment reduced the number of TAMs and bone destruction in a breast neoplasm mouse model (71). TGF-β1 and VEGFA in tumor cells were downregulated when we depleted macrophages in squamous cell carcinoma models (72), which demonstrated that VEGFA-dependent angiogenesis was reduced after TAM ablation.
Overwhelming studies have suggested that targeting the NF-κB/CCL2 signal might be beneficial for blocking TAM recruitment (73). In agreement with this, the use of celecoxib to suppress NF-κB and the downregulation of CCL2 attenuated TAM recruitment and increased the apoptosis of tumor cells in malignant glioma (74).
Oligonucleotide delivery technology is another common method to reshape TAMs, involving charge-altering released transporters and other nanoparticles. To restore the negative effects of TAMs, sunitinib and sorafenib aimed to limit STAT3 or STAT6 in macrophages, subsequently distorting macrophage polarization (75, 76).
Targeting NK cells
The selection of NK-cell sources and the means of enhancing NK-cell function in vivo are the key factors influencing NK-cell therapy. IL-2 and IL-15 are viewed as essential cytokines that upregulate the viability of NK cells. Treating human foreign blood mononuclear cells with IL-2 results in the expansion of a group of lymphokine-activated killer (LAK) cells, which consist primarily of T cells and NK cells and are highly cytotoxic to tumor cells (77). Furthermore, IL-2 decreased the amount and size of metastases in mouse models of pulmonary osteosarcoma when injected repeatedly at low doses following adoptive LAK cell transfer (78). In initial studies in syngeneic mouse models of several neoplasms, recombinant IL-15 was well tolerated and expanded NK and CD8+ T-cell groups, which promoted tumor suppression and reduced metastasis (79).
Targeting dendritic cells
The capability of DCs to elicit robust and direct adaptive immune responses has been exploited for neoplasm immunotherapy, and targeting DCs may provide a way to improve immune responses. There is evidence that antibodies against vascular endothelial growth factor enhance antitumor immune responses by offsetting DC suppression (80, 81).
DC vaccination is the injection of mature DCs loaded with tumor antigens ex vivo into cancer patients. Whether this is clinically feasible has not been established, especially given the lack of circulating mature cDC1s in human foreign blood (55, 82). In glioblastoma, a phase III trial (NCT00045968) will evaluate the efficacy of a whole-cell DC vaccine unified with tumor resection, temozolomide, and radiotherapy, which showed safety and potential efficacy in earlier results (83).
Unlike injecting exogenously expanded and activated cDCs, injecting an incremental number of cDCs within tumors is another method to increase the cumulative function of the group. Preclinical studies have shown that systemic injection of Flt3L results in systemic expansion of the cDC1 population, enhances the number of these cells within B16 melanomas, and prominently destroys tumor growth (84). The anti-CD123-directed diphtheria toxin tagraxofusp-erzs was able to eliminate the pDC population in acute myeloid leukemia (85). This means that it is being studied clinically in several types of tumors, including metastatic breast cancer and non-Hodgkin’s lymphoma (NCT03789097, NCT01976585).
Targeting myeloid-derived suppressor cells
Several lines of evidence manifest a close connection between MDSC accumulation and clinical outcome in cancer patients (86). The frequency of M-MDSCs is conversely interrelated with the treatment effect of chemotherapy in cervical and colorectal neoplasms (87, 88). The number of PMN-MDSCs is negatively correlated with the response to chemotherapy in colorectal cancer (88). In patients with unresectable melanoma, the percentages of circulating M-MDSCs and PMN-MDSCs are inversely associated with objective clinical responses to ipilimumab (anti-CTLA-4) (89, 90). Recent studies in mouse tumor models indicate that inhibition of MDSCs during immunotherapy improves the treatment effect (91, 92). Lu et al. supplemented a combination of low-dose adjuvant epigenetic modifiers in a mouse model of lung metastasis, which disrupts the formation of the pre-metastatic microenvironment by suppressing the migration of MDSCs and promoting MDSC differentiation into an interstitial macrophage-like phenotype (86). Fewer circulating MDSCs with lower iNOS and arginase expression and a greater number of spontaneously generated tumor-specific T cells are found in head and neck cancer and multiple myeloma patients who are treated with tadalafil (90, 93).
Conclusions
In conclusion, the complex interactions among tumor cells, immune cells, and osteocytes in the spinal marrow microenvironment demand further research into the mechanisms that trigger bone metastasis. The function and number of immune cells in the bone microenvironment influence the efficacy of the anticancer immune response (Table 1). A comprehensive understanding of the roles and functions of T cells, macrophages, NK cells, DCs, and MDSCs in the bone microenvironment is essential for more effective treatments for bone metastasis and brings new promise to patients with bone metastasis.
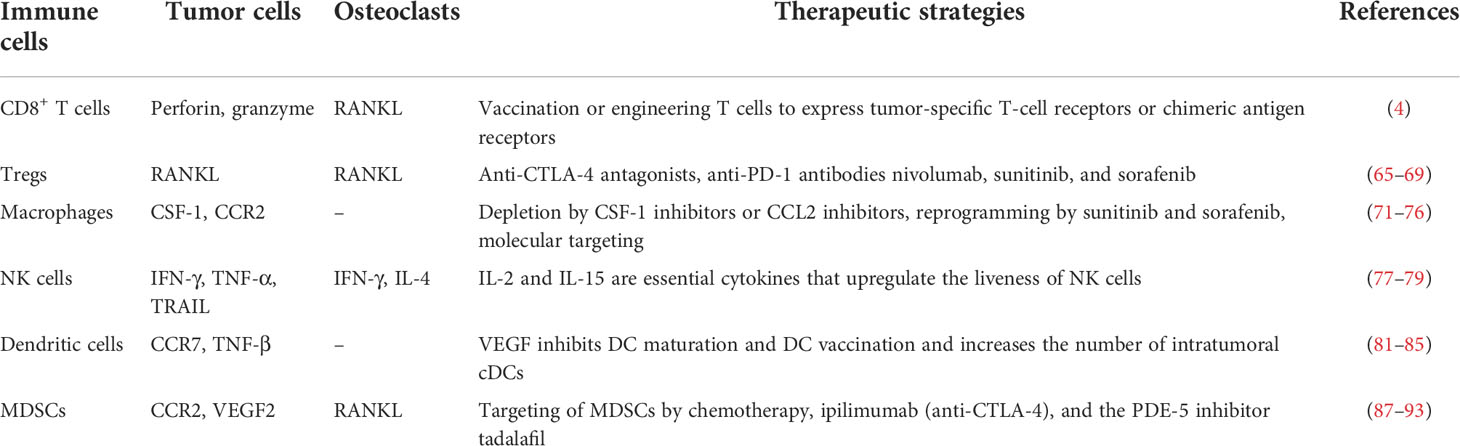
Table 1 The interaction of immune cells, tumor cells, and osteoclasts in the bone microenvironment and their therapeutic strategies.
Author contributions
The manuscript was conceptualized by NH and JJ. NH wrote the majority of the manuscript and produced the figure and table. JJ critically revised the manuscript. All authors contributed to the article and approved the submitted version.
Funding
This work was supported by the National Key R&D Program (grant number 2018YFC1313400); the Joint Research Fund for Overseas Chinese, Hong Kong and Macao Scholars (grant number 31729001); and the National Natural Science Foundation of China (grant numbers 81972869, 81902386).
Conflict of interest
The authors declare that the research was conducted in the absence of any commercial or financial relationships that could be construed as a potential conflict of interest.
Publisher’s note
All claims expressed in this article are solely those of the authors and do not necessarily represent those of their affiliated organizations, or those of the publisher, the editors and the reviewers. Any product that may be evaluated in this article, or claim that may be made by its manufacturer, is not guaranteed or endorsed by the publisher.
References
1. Coleman RE. Clinical features of metastatic bone disease and risk of skeletal morbidity. Clin Cancer Res (2006) 12(20 Pt 2):6243s–9s. doi: 10.1158/1078-0432.CCR-06-0931
2. D'Amico L, Roato I. The impact of immune system in regulating bone metastasis formation by osteotropic tumors. J Immunol Res (2015) 2015:143526. doi: 10.1155/2015/143526
3. Kingsley LA, Fournier PG, Chirgwin JM, Guise TA. Molecular biology of bone metastasis. Mol Cancer Ther (2007) 6(10):2609–17. doi: 10.1158/1535-7163.MCT-07-0234
4. Janssen LME, Ramsay EE, Logsdon CD, Overwijk WW. The immune system in cancer metastasis: Friend or foe? J Immunother Cancer (2017) 5(1):79. doi: 10.1186/s40425-017-0283-9
5. Bussard KM, Gay CV, Mastro AM. The bone microenvironment in metastasis; what is special about bone? Cancer Metastasis Rev (2008) 27(1):41–55. doi: 10.1007/s10555-007-9109-4
6. Suominen MI, Wilson T, Kakonen SM, Scholz A. The mode-of-Action of targeted alpha therapy radium-223 as an enabler for novel combinations to treat patients with bone metastasis. Int J Mol Sci (2019) 20(16)3899. doi: 10.3390/ijms20163899
7. Loberg RD, Logothetis CJ, Keller ET, Pienta KJ. Pathogenesis and treatment of prostate cancer bone metastases: Targeting the lethal phenotype. J Clin Oncol (2005) 23(32):8232–41. doi: 10.1200/JCO.2005.03.0841
8. Ghajar CM. Metastasis prevention by targeting the dormant niche. Nat Rev Cancer (2015) 15(4):238–47. doi: 10.1038/nrc3910
9. Oskarsson T, Batlle E, Massague J. Metastatic stem cells: Sources, niches, and vital pathways. Cell Stem Cell (2014) 14(3):306–21. doi: 10.1016/j.stem.2014.02.002
10. Lou Y, McDonald PC, Oloumi A, Chia S, Ostlund C, Ahmadi A, et al. Targeting tumor hypoxia: Suppression of breast tumor growth and metastasis by novel carbonic anhydrase ix inhibitors. Cancer Res (2011) 71(9):3364–76. doi: 10.1158/0008-5472.CAN-10-4261
11. Chiang AC, Massague J. Molecular basis of metastasis. N Engl J Med (2008) 359(26):2814–23. doi: 10.1056/NEJMra0805239
12. Winkler IG, Barbier V, Nowlan B, Jacobsen RN, Forristal CE, Patton JT, et al. Vascular niche e-selectin regulates hematopoietic stem cell dormancy, self renewal and chemoresistance. Nat Med (2012) 18(11):1651–7. doi: 10.1038/nm.2969
13. Magidey-Klein K, Cooper TJ, Kveler K, Normand R, Zhang T, Timaner M, et al. Il-6 contributes to metastatic switch via the differentiation of monocytic-dendritic progenitors into prometastatic immune cells. J Immunother Cancer (2021) 9(6):e002856. doi: 10.1136/jitc-2021-002856
14. Santini D, Perrone G, Roato I, Godio L, Pantano F, Grasso D, et al. Expression pattern of receptor activator of nfkappab (Rank) in a series of primary solid tumors and related bone metastases. J Cell Physiol (2011) 226(3):780–4. doi: 10.1002/jcp.22402
15. Tipton DA, Seshul BA, Dabbous MK. Effect of bisphosphonates on the production of mediators of osteoclastogenesis (Rankl, opg, and il-6) by human gingival fibroblasts. J Periodontal Res (2011) 46(1):39–47. doi: 10.1111/j.1600-0765.2010.01306.x
16. Xu Z, Hurchla MA, Deng H, Uluckan O, Bu F, Berdy A, et al. Interferon-gamma targets cancer cells and osteoclasts to prevent tumor-associated bone loss and bone metastases. J Biol Chem (2009) 284(7):4658–66. doi: 10.1074/jbc.M804812200
17. Monteiro AC, Leal AC, Goncalves-Silva T, Mercadante AC, Kestelman F, Chaves SB, et al. T Cells induce pre-metastatic osteolytic disease and help bone metastases establishment in a mouse model of metastatic breast cancer. PloS One (2013) 8(7):e68171. doi: 10.1371/journal.pone.0068171
18. Luckheeram RV, Zhou R, Verma AD, Xia B. Cd4(+)T cells: Differentiation and functions. Clin Dev Immunol (2012) 2012:925135. doi: 10.1155/2012/925135
19. Najafi M, Farhood B, Mortezaee K. Contribution of regulatory T cells to cancer: A review. J Cell Physiol (2019) 234(6):7983–93. doi: 10.1002/jcp.27553
20. Zhao E, Wang L, Dai J, Kryczek I, Wei S, Vatan L, et al. Regulatory T cells in the bone marrow microenvironment in patients with prostate cancer. Oncoimmunology (2012) 1(2):152–61. doi: 10.4161/onci.1.2.18480
21. Tan W, Zhang W, Strasner A, Grivennikov S, Cheng JQ, Hoffman RM, et al. Tumour-infiltrating regulatory T cells stimulate mammary cancer metastasis through rankl-rank signalling. Nature (2011) 470(7335):548–53. doi: 10.1038/nature09707
22. Li B, Wang P, Jiao J, Wei H, Xu W, Zhou P. Roles of the rankl-rank axis in immunity-implications for pathogenesis and treatment of bone metastasis. Front Immunol (2022) 13:824117. doi: 10.3389/fimmu.2022.824117
23. Mendoza-Reinoso V, McCauley LK, Fournier PGJ. Contribution of macrophages and T cells in skeletal metastasis. Cancers (2020) 12(4):1014. doi: 10.3390/cancers12041014
24. Mathan T, Figdor CG, Buschow SI. Human plasmacytoid dendritic cells: From molecules to intercellular communication network. Front Immunol (2013) 4:372. doi: 10.3389/fimmu.2013.00372
25. Arellano DL, Juarez P, Verdugo-Meza A, Almeida-Luna PS, Corral-Avila JA, Drescher F, et al. Bone microenvironment-suppressed T cells increase osteoclast formation and osteolytic bone metastases in mice. J Bone Miner Res (2022) 37(8):1446–63. doi: 10.1002/jbmr.4615
26. Okhrimenko A, Grun JR, Westendorf K, Fang Z, Reinke S, von Roth P, et al. Human memory T cells from the bone marrow are resting and maintain long-lasting systemic memory. Proc Natl Acad Sci U.S.A. (2014) 111(25):9229–34. doi: 10.1073/pnas.1318731111
27. Mazo IB, Honczarenko M, Leung H, Cavanagh LL, Bonasio R, Weninger W, et al. Bone marrow is a major reservoir and site of recruitment for central memory Cd8+ T cells. Immunity (2005) 22(2):259–70. doi: 10.1016/j.immuni.2005.01.008
28. Li J, Huston G, Swain SL. Il-7 promotes the transition of Cd4 effectors to persistent memory cells. J Exp Med (2003) 198(12):1807–15. doi: 10.1084/jem.20030725
29. Feuerer M, Rocha M, Bai L, Umansky V, Solomayer EF, Bastert G, et al. Enrichment of memory T cells and other profound immunological changes in the bone marrow from untreated breast cancer patients. Int J Cancer J Int Du Cancer (2015) 92(1):96–105.
30. Gomez Perdiguero E, Klapproth K, Schulz C, Busch K, Azzoni E, Crozet L, et al. Tissue-resident macrophages originate from yolk-Sac-Derived erythro-myeloid progenitors. Nature (2015) 518(7540):547–51. doi: 10.1038/nature13989
31. Manolagas SC. Birth and death of bone cells: Basic regulatory mechanisms and implications for the pathogenesis and treatment of osteoporosis. Endocr Rev (2000) 2):2. doi: 10.1210/edrv.21.2.0395
32. Takeya M, Komohara Y. Role of tumor-associated macrophages in human malignancies: Friend or foe? Pathol Int (2016) 66(9):491–505. doi: 10.1111/pin.12440
33. Rodriguez PC, Ruffell B. Cavity macrophages stop anti-tumor T cells. Cancer Cell (2021) 39(7):900–2. doi: 10.1016/j.ccell.2021.06.007
34. Chow A, Schad S, Green MD, Hellmann MD, Allaj V, Ceglia N, et al. Tim-4(+) cavity-resident macrophages impair anti-tumor Cd8(+) T cell immunity. Cancer Cell (2021) 39(7):973–88.e9. doi: 10.1016/j.ccell.2021.05.006
35. Qian BZ, Li J, Hui Z, Kitamura T, Zhang J, Campion LR, et al. Ccl2 recruits inflammatory monocytes to facilitate breast-tumour metastasis. Nature (2012) 475(7355):222–5. doi: 10.1038/nature10138
36. Sullivan AR, Pixley FJ. Csf-1r signaling in health and disease: A focus on the mammary gland. J Mammary Gland Biol Neoplasia (2014) 19(2):149–59. doi: 10.1007/s10911-014-9320-1
37. Jimenez-Sainz MC. Signaling pathways for monocyte chemoattractant protein 1-mediated extracellular signal-regulated kinase activation. Mol Pharmacol (2003) 64(3):773–82. doi: 10.1124/mol.64.3.773
38. Lu X, Kang Y. Chemokine (C-c motif) ligand 2 engages Ccr2+ stromal cells of monocytic origin to promote breast cancer metastasis to lung and bone. J Biol Chem (2009) 284(42):29087–96. doi: 10.1074/jbc.M109.035899
39. Izumi K, Fang LY, Mizokami A, Namiki M, Li L, Lin WJ, et al. Targeting the androgen receptor with sirna promotes prostate cancer metastasis through enhanced macrophage recruitment Via Ccl2/Ccr2-induced Stat3 activation. EMBO Mol Med (2013) 5(9):1383–401. doi: 10.1002/emmm.201202367
40. Battafarano G, Rossi M, Marampon F, Del Fattore A. Cellular and molecular mediators of bone metastatic lesions. Int J Mol Sci (2018) 19(6):1709. doi: 10.3390/ijms19061709
41. Liu C, Zhang W, Wang J, Si T, Xing W. Tumor-associated macrophage-derived transforming growth factor-beta promotes colorectal cancer progression through Hif1-Trib3 signaling. Cancer Sci (2021) 112(10):4198–207. doi: 10.1111/cas.15101
42. Kitamura T, Qian BZ, Soong D, Cassetta L, Pollard JW. Ccl2-induced chemokine cascade promotes breast cancer metastasis by enhancing retention of metastasis-associated macrophages. J Exp Med (2015) 212(7):1043–59. doi: 10.1084/jem.20141836
43. Plenker D, Bertrand M, de Langen AJ, Riedel R, Lorenz C, Scheel AH, et al. Structural alterations of met trigger response to met kinase inhibition in lung adenocarcinoma patients. Clin Cancer Res (2018) 24(6):1337–43. doi: 10.1158/1078-0432.CCR-17-3001
44. Bottcher JP, Bonavita E, Chakravarty P, Blees H, Cabeza-Cabrerizo M, Sammicheli S, et al. Nk cells stimulate recruitment of Cdc1 into the tumor microenvironment promoting cancer immune control. Cell (2018) 172(5):1022–37.e14. doi: 10.1016/j.cell.2018.01.004
45. Ardolino M, Zingoni A, Cerboni C, Cecere F, Soriani A, Iannitto ML, et al. Dnam-1 ligand expression on Ag-stimulated T lymphocytes is mediated by ros-dependent activation of DNA-damage response: Relevance for nk-T cell interaction. Blood (2011) 117(18):4778–86. doi: 10.1182/blood-2010-08-300954
46. Schmiedel BJ, Werner A, Steinbacher J, Nuebling T, Buechele C, Grosse-Hovest L, et al. Generation and preclinical characterization of a fc-optimized gitr-ig fusion protein for induction of nk cell reactivity against leukemia. Mol Ther (2013) 21(4):877–86. doi: 10.1038/mt.2013.11
47. Soderstrom K, Stein E, Colmenero P, Purath U, Muller-Ladner U, de Matos CT, et al. Natural killer cells trigger osteoclastogenesis and bone destruction in arthritis. Proc Natl Acad Sci U.S.A. (2010) 107(29):13028–33. doi: 10.1073/pnas.1000546107
48. Takayanagi H, Ogasawara K, Hida S, Chiba T, Taniguchi T. T-Cell-Mediated regulation of osteoclastogenesis by signalling cross-talk between rankl and ifn-gamma. Nature (2000) 408(6812):600–5. doi: 10.1038/35046102
49. Gardner A, Ruffell B. Dendritic cells and cancer immunity. Trends Immunol (2016) 37(12):855–65. doi: 10.1016/j.it.2016.09.006
50. Roberts EW, Broz ML, Binnewies M, Headley MB, Nelson AE, Wolf DM, et al. Critical role for Cd103+/Cd141+ dendritic cells bearing Ccr7 for tumor antigen trafficking and priming of T cell immunity in melanoma. Cancer Cell (2016) 30(2):324–36. doi: 10.1016/j.ccell.2016.06.003
51. Veglia F, Gabrilovich DI. Dendritic cells in cancer: The role revisited. Curr Opin Immunol (2017) 45:43–51. doi: 10.1016/j.coi.2017.01.002
52. Joeris T, Gomez-Casado C, Holmkvist P, Tavernier SJ, Silva-Sanchez A, Klotz L, et al. Intestinal Cdc1 drive cross-tolerance to epithelial-derived antigen Via induction of Foxp3+Cd8+ tregs. Sci Immunol (2021) 6(60):eabd3774. doi: 10.1126/sciimmunol.abd3774
53. Sittig SP, Bakdash G, Weiden J, Skold AE, Tel J, Figdor CG, et al. A comparative study of the T cell stimulatory and polarizing capacity of human primary blood dendritic cell subsets. Mediators Inflammation (2016) 2016:3605643. doi: 10.1155/2016/3605643
54. Binnewies M, Mujal AM, Pollack JL, Combes AJ, Hardison EA, Barry KC, et al. Unleashing type-2 dendritic cells to drive protective antitumor Cd4(+) T cell immunity. Cell (2019) 177(3):556–71.e16. doi: 10.1016/j.cell.2019.02.005
55. Wang W, Xu J, Khoury JD, Pemmaraju N, Fang H, Miranda RN, et al. Immunophenotypic and molecular features of acute myeloid leukemia with plasmacytoid dendritic cell differentiation are distinct from blastic plasmacytoid dendritic cell neoplasm. Cancers (2022) 14(14):3375. doi: 10.3390/cancers14143375
56. Shurin MR, Naiditch H, Zhong H, Shurin GV. Regulatory dendritic cells: New targets for cancer immunotherapy. Cancer Biol Ther (2011) 11(11):988–92. doi: 10.4161/cbt.11.11.15543
57. Sawant A, Hensel JA, Chanda D, Harris BA, Siegal GP, Maheshwari A, et al. Depletion of plasmacytoid dendritic cells inhibits tumor growth and prevents bone metastasis of breast cancer cells. J Immunol (2012) 189(9):4258–65. doi: 10.4049/jimmunol.1101855
58. Huang B, Lei Z, Zhao J, Gong W, Liu J, Chen Z, et al. Ccl2/Ccr2 pathway mediates recruitment of myeloid suppressor cells to cancers. Cancer Lett (2007) 252(1):86–92. doi: 10.1016/j.canlet.2006.12.012
59. Chun E, Lavoie S, Michaud M, Gallini CA, Kim J, Soucy G, et al. Ccl2 promotes colorectal carcinogenesis by enhancing polymorphonuclear myeloid-derived suppressor cell population and function. Cell Rep (2015) 12(2):244–57. doi: 10.1016/j.celrep.2015.06.024
60. Zhuang J, Zhang J, Lwin ST, Edwards JR, Edwards CM, Mundy GR, et al. Osteoclasts in multiple myeloma are derived from gr-1+Cd11b+Myeloid-Derived suppressor cells. PloS One (2012) 7(11):e48871. doi: 10.1371/journal.pone.0048871
61. Danilin S, Merkel AR, Johnson JR, Johnson RW, Edwards JR, Sterling JA. Myeloid-derived suppressor cells expand during breast cancer progression and promote tumor-induced bone destruction. Oncoimmunology (2012) 1(9):1484–94. doi: 10.4161/onci.21990
62. Gonda K, Shibata M, Ohtake T, Matsumoto Y, Tachibana K, Abe N, et al. Myeloid-derived suppressor cells are increased and correlated with type 2 immune responses, malnutrition, inflammation, and poor prognosis in patients with breast cancer. Oncol Lett (2017) 14(2):1766–74. doi: 10.3892/ol.2017.6305
63. Idorn M, Kollgaard T, Kongsted P, Sengelo VL, Straten PT. Correlation between frequencies of blood monocytic myeloid-derived suppressor cells, regulatory T cells and negative prognostic markers in patients with castration-resistant metastatic prostate cancer. Cancer Immunol Immunother (2014) 63(11):1177–87. doi: 10.1007/s00262-014-1591-2
64. Ning C, Tan Z, Ma K, Bao L, Yun Z. Increased circulating myeloid-derived suppressor cells correlate with cancer stages, interleukin-8 and -6 in prostate cancer. Int J Clin Exp Med (2014) 7(10):3181–92.
65. Larkin J, Chiarion-Sileni V, Gonzalez R, Grob JJ, Wolchok JD. Five-year survival with combined nivolumab and ipilimumab in advanced melanoma. New Engl J Med (2019) 381(16):1535–46. doi: 10.1056/NEJMoa1910836
66. Hellmann MD, Ciuleanu TE, Pluzanski A, Lee JS, Otterson GA, Audigier-Valette C, et al. Nivolumab plus ipilimumab in lung cancer with a high tumor mutational burden. N Engl J Med (2018) 378(22):2093–104. doi: 10.1056/NEJMoa1801946
67. Wang SD, Li HY, Li BH, Tao X, Zhu T, Sun LL, et al. The role of ctla-4 and pd-1 in anti-tumor immune response and their potential efficacy against osteosarcoma. Int Immunopharmacol (2016) 38:81–9. doi: 10.1016/j.intimp.2016.05.016
68. Guan X, Polesso F, Wang C, Sehrawat A, Hawkins RM, Murray SE, et al. Androgen receptor activity in T cells limits checkpoint blockade efficacy. Nature (2022) 606(7915):791–6. doi: 10.1038/s41586-022-04522-6
69. Busse A, Asemissen AM, Nonnenmacher A, Braun F, Ochsenreither S, Stather D, et al. Immunomodulatory effects of sorafenib on peripheral immune effector cells in metastatic renal cell carcinoma. Eur J Cancer (2011) 47(5):690–6. doi: 10.1016/j.ejca.2010.11.021
70. Stadtmauer EA, Fraietta JA, Davis MM, Cohen AD, June CH. Crispr-engineered T cells in patients with refractory cancer. Science (2020) 367(6481):eaba7365. doi: 10.1126/science.aba7365
71. Fend L, Accart N, Kintz J, Cochin S, Reymann C, Le Pogam F, et al. Therapeutic effects of anti-Cd115 monoclonal antibody in mouse cancer models through dual inhibition of tumor-associated macrophages and osteoclasts. PloS One (2013) 8(9):e73310. doi: 10.1371/journal.pone.0073310
72. Tannahill GM, Curtis AM, Adamik J, Palsson-McDermott EM, McGettrick AF, Goel G, et al. Succinate is an inflammatory signal that induces il-1beta through hif-1alpha. Nature (2013) 496(7444):238–42. doi: 10.1038/nature11986
73. Wu D, Liu X, Mu J, Yang J, Wu F, Zhou H. Therapeutic approaches targeting proteins in tumor-associated macrophages and their applications in cancers. Biomolecules (2022) 12(3):392. doi: 10.3390/biom12030392
74. Shono K, Yamaguchi I, Mizobuchi Y, Kagusa H, Sumi A, Fujihara T, et al. Downregulation of the Ccl2/Ccr2 and Cxcl10/Cxcr3 axes contributes to antitumor effects in a mouse model of malignant glioma. Sci Rep (2020) 10(1):15286. doi: 10.1038/s41598-020-71857-3
75. Rong D, Gong Y, Wen M, Meng Y, Bo Y. The involvement of M2 macrophage polarization inhibition in fenretinide-mediated chemopreventive effects on colon cancer. Cancer Lett (2017) 388:43–53. doi: 10.1016/j.canlet.2016.11.029
76. Hu L, Liu Y, Kong X, Wu R, Peng Q, Zhang Y, et al. Fusobacterium nucleatum facilitates M2 macrophage polarization and colorectal carcinoma progression by activating Tlr4/Nf-Kappab/S100a9 cascade. Front Immunol (2021) 12:658681. doi: 10.3389/fimmu.2021.658681
77. Williams R, Cooley S, Bachanova V, Waldmann TA, Verneris MR. Role of recipient Cd8+ T cell exhaustion in the rejection of adoptively transferred haploidentical nk cells. Blood (2016) 128(22):503–. doi: 10.1126/science.6332379
78. Mule J, Shu S, Schwarz S, Rosenberg S. Adoptive immunotherapy of established pulmonary metastases with lak cells and recombinant interleukin-2. Science (1984) 225(4669):1487–9. doi: 10.1126/science.6332379
79. Tang F, Zhao L, Jiang Y, Ba D, Cui L, He W. Activity of recombinant human interleukin-15 against tumor recurrence and 1Metastasis in mice. Cell Mol Immunol (2008) 5(3):189–96. doi: 10.1038/cmi.2008.23
80. Zhao H, Wu L, Yan G, Chen Y, Zhou M, Wu Y, et al. Inflammation and tumor progression: Signaling pathways and targeted intervention. Signal Transduct Target Ther (2021) 6(1):263. doi: 10.1038/s41392-021-00658-5
81. Long J, Hu Z, Xue H, Wang Y, Chen J, Tang F, et al. Vascular endothelial growth factor (Vegf) impairs the motility and immune function of human mature dendritic cells through the vegf receptor 2-Rhoa-Cofilin1 pathway. Cancer Sci (2019) 110(8):2357–67. doi: 10.1111/cas.14091
82. Perez CR, De Palma M. Engineering dendritic cell vaccines to improve cancer immunotherapy. Nat Commun (2019) 10(1):5408. doi: 10.1038/s41467-019-13368-y
83. Liau LM, Ashkan K, Tran DD, Campian JL, Trusheim JE, Cobbs CS, et al. First results on survival from a Large phase 3 clinical trial of an autologous dendritic cell vaccine in newly diagnosed glioblastoma. J Transl Med (2018) 16(1):142. doi: 10.1186/s12967-018-1507-6
84. Salmon H, Idoyaga J, Rahman A, Leboeuf M, Remark R, Jordan S, et al. Expansion and activation of Cd103(+) dendritic cell progenitors at the tumor site enhances tumor responses to therapeutic pd-L1 and braf inhibition. Immunity (2016) 44(4):924–38. doi: 10.1016/j.immuni.2016.03.012
85. Xiao W, Chan A, Waarts MR, Mishra T, Liu Y, Cai SF, et al. Plasmacytoid dendritic cell expansion defines a distinct subset of Runx1-mutated acute myeloid leukemia - sciencedirect. (2021) 137(10):1377–1391. doi: 10.1182/blood.2020007897
86. Lu Z, Zou J, Li S, Topper MJ, Tao Y, Zhang H, et al. Epigenetic therapy inhibits metastases by disrupting premetastatic niches. Nature (2020) 579(7798):284–90. doi: 10.1038/s41586-020-2054-x
87. Kawano M, Mabuchi S, Matsumoto Y, Sasano T, Takahashi R, Kuroda H, et al. The significance of G-csf expression and myeloid-derived suppressor cells in the chemoresistance of uterine cervical cancer. Sci Rep (2015) 5(1):18217. doi: 10.1038/srep18217
88. Tada K, Kitano S, Shoji H, Nishimura T, Shimada Y, Nagashima K, et al. Pretreatment immune status correlates with progression-free survival in chemotherapy-treated metastatic colorectal cancer patients. Cancer Immunol Res (2016), 4(7):592–9. doi: 10.1158/2326-6066.CIR-15-0298
89. Sadefeldman M, Kanterman J, Klieger Y, Ishshalom E, Olga M, Saragovi A, et al. Clinical significance of circulating Cd33+Cd11b+Hla-dr- myeloid cells in patients with stage iv melanoma treated with ipilimumab. Clin Cancer Res (2016) 22(23):5661–72. doi: 10.1158/1078-0432.CCR-15-3104
90. Martens A, Wistuba-Hamprecht K, Geukes Foppen M, Yuan J, Postow MA, Wong P, et al. Baseline peripheral blood biomarkers associated with clinical outcome of advanced melanoma patients treated with ipilimumab. Clin Cancer Res (2016) 22(12):2908–18. doi: 10.1158/1078-0432.CCR-15-2412
91. Iida Y, Harashima N, Motoshima T, Komohara Y, Eto M, Harada M. Contrasting effects of cyclophosphamide on anti-Ctl-Associated protein 4 blockade therapy in two mouse tumor models. Cancer Sci (2017) 108(10):1974–84. doi: 10.1111/cas.13337
92. Kamran N, Kadiyala P, Saxena M, Candolfi M, Li Y, Moreno-Ayala MA, et al. Immunosuppressive myeloid cells' blockade in the glioma microenvironment enhances the efficacy of immune-stimulatory gene therapy. Mol Ther (2017) 25(1):232–48. doi: 10.1016/j.ymthe.2016.10.003
Keywords: immune system, immunotherapy, bone microenvironment, immune response, bone metastasis
Citation: He N and Jiang J (2022) Contribution of immune cells to bone metastasis pathogenesis. Front. Endocrinol. 13:1019864. doi: 10.3389/fendo.2022.1019864
Received: 15 August 2022; Accepted: 13 September 2022;
Published: 29 September 2022.
Edited by:
Narattaphol Charoenphandhu, Mahidol University, ThailandReviewed by:
Giacomina Brunetti, University of Bari Aldo Moro, ItalyCopyright © 2022 He and Jiang. This is an open-access article distributed under the terms of the Creative Commons Attribution License (CC BY). The use, distribution or reproduction in other forums is permitted, provided the original author(s) and the copyright owner(s) are credited and that the original publication in this journal is cited, in accordance with accepted academic practice. No use, distribution or reproduction is permitted which does not comply with these terms.
*Correspondence: Jingting Jiang, jiangjingting@suda.edu.cn