- 1Integrative Neurobiology Section, National Institute on Drug Abuse, Intramural Research Program, National Institutes on Drug Abuse, Baltimore, MD, United States
- 2Division of Addiction Research and Treatment, Department of Psychiatry, University of Maryland School of Medicine, Baltimore, MD, United States
- 3Pharmacology Unit, Department of Pathology and Experimental Therapeutics, Faculty of Medicine and Health Sciences, Institute of Neurosciences, University of Barcelona, L'Hospitalet de Llobregat, Spain
- 4Neuropharmacology & Pain Group, Neuroscience Program, Bellvitge Institute for Biomedical Research, L'Hospitalet de Llobregat, Spain
- 5Department of Biochemistry and Molecular Biomedicine, Faculty of Biology, Institute of Biomedicine of the University of Barcelona (IBUB), University of Barcelona, Barcelona, Spain
- 6Department of Basic Pharmaceutical Sciences, Fred Wilson School of Pharmacy, High Point, NC, United States
- 7Department of Chemistry and Biochemistry, Rowan University, Glassboro, NJ, United States
- 8Departament of Physiology, Biophysics and Neurosciences, Centro de Investigación y de Estudios Avanzados del Instituto Politécnico Nacional, Mexico City, Mexico
- 9Department of Neurology, Johns Hopkins University School of Medicine, Baltimore, MD, United States
- 10Instituto de Investigaciones en Ingeniería Genética y Biología Molecular, Consejo Nacional de Investigaciones Científicas y Técnicas and, Facultad de Ciencias Exactas y Naturales, Universidad de Buenos Aires, Buenos Aires, Argentina
- 11National Institute on Drug Abuse, National Institutes of Health, Rockville, MD, United States
The functional and pharmacological significance of the dopamine D4 receptor (D4R) has remained the least well understood of all the dopamine receptor subtypes. Even more enigmatic has been the role of the very prevalent human DRD4 gene polymorphisms in the region that encodes the third intracellular loop of the receptor. The most common polymorphisms encode a D4R with 4 or 7 repeats of a proline-rich sequence of 16 amino acids (D4.4R and D4.7R). DRD4 polymorphisms have been associated with individual differences linked to impulse control-related neuropsychiatric disorders, with the most consistent associations established between the gene encoding D4.7R and attention-deficit hyperactivity disorder (ADHD) and substance use disorders. The function of D4R and its polymorphic variants is being revealed by addressing the role of receptor heteromerization and the relatively avidity of norepinephrine for D4R. We review the evidence conveying a significant and differential role of D4.4R and D4.7R in the dopaminergic and noradrenergic modulation of the frontal cortico-striatal pyramidal neuron, with implications for the moderation of constructs of impulsivity as personality traits. This differential role depends on their ability to confer different properties to adrenergic α2A receptor (α2AR)-D4R heteromers and dopamine D2 receptor (D2R)-D4R heteromers, preferentially localized in the perisomatic region of the frontal cortical pyramidal neuron and its striatal terminals, respectively. We also review the evidence to support the D4R as a therapeutic target for ADHD and other impulse-control disorders, as well as for restless legs syndrome.
1 Introduction
Discovered 30 years ago, the dopamine D4 receptor (D4R) initially drew attention due to its strong affinity for the atypical antipsychotic clozapine, an affinity found to be significantly higher than for the previously discovered D1, D2 and D3 receptors (D1R, D2R and D3R) (1). At the time, it was assumed that this high affinity could underlie clozapine’s unique clinical efficacy, which led to a very intense search for selective D4R antagonists. Unfortunately, the newly discovered D4R antagonists that followed were ineffective as antipsychotic drugs in clinical trials and the interest in D4R as a target for drug development waned [reviewed in ref. (2)].
Not only the pharmacological, but also the functional significance of the D4R has remained the most enigmatic of all the dopamine receptor subtypes. The human D4R gene (DRD4) displays a high number of polymorphisms in its coding sequence. The most extensive polymorphism is found in exon 3, a region that encodes the third intracellular loop (3IL) of the receptor (3–5). This polymorphism comprises a variable number of tandem repeats of a 48-base pair sequence, from 2- to 11 repeats. The most common polymorphisms contain 2, 4 or 7 repeats (with allelic frequencies of about 8%, 60% and 20%, respectively) (4), which encode a D4R with the respective number of repeats of a proline-rich sequence of 16 amino acids (D4.2R, D4.4R and D4.7R) (3–5). DRD4 polymorphisms have been associated with individual differences in impulse control-related neuropsychiatric disorders, with the most consistent associations found between the gene encoding D4.7R and attention-deficit hyperactivity disorder (ADHD) (3, 6–8) and substance use disorders (SUDs) (9). It follows that these significant associations should provide clues about the functional and pharmacological significance of the D4R. The main functional and pharmacological properties of the D4R and its different D4R polymorphic variants have been elucidated only recently with studies addressing the role of receptor heteromerization and the relatively avidity of norepinephrine for D4R. This includes the role of pineal D4R in the circadian noradrenergic modulation of melatonin synthesis and release (10), as well as the role of D4R in the inhibitory dopaminergic modulation of frontal cortico-striatal glutamatergic neurotransmission (11). Here we review the evidence to support the role of frontal cortical D4Rs in the moderation of constructs of impulsivity as personality traits. Based on the available evidence, we submit that the D4R should be exploited as a therapeutic target for impulse control-related neuropsychiatric disorders (primarily, ADHD), as well as for restless legs syndrome (RLS).
2 The D4R-modulated frontal-cortico-striatal neuron
D4R is highly expressed in the prefrontal cortex of mammals, including rodents and human and non-human primates, particularly in deep layer neurons (1, 12–14). In contrast with other dopamine receptors (D1R, D2R and in the ventral striatum, D3R), striatal mRNA expression of D4R is much lower (1, 12). However, immunohistochemical studies using different antibodies against different epitopes of the rodent and human D4R produced incongruent results, particularly in relation to its striatal density, indicating a lack of antibody specificity (15–19). Further studies, using a transgenic mouse expressing a fluorescent protein under the transcriptional control of the mouse dopamine D4R gene (Drd4), confirmed its predominant expression in the deep layer neurons of the prefrontal cortex and its lack of expression in the striatum (20). These results agreed with those obtained using in situ hybridization studies and indicate that striatal D4Rs are localized in striatal nerve terminals and, most probably in the terminals from glutamatergic neurons originated in the deep cortical layers.
In fact, significant evidence for the existence of D4Rs in the prefrontal cortex and the striatum of rats and humans has been obtained by radioligand binding experiments (21–25). The D4R labeling strategies included radioligands with significant selectivity for D4R or non-selective D2-like receptor radioligands in the presence of the D2R-D3R antagonist raclopride, which demonstrates a specific low affinity for the D4R (1). The results showed a disproportionally high density of striatal D4R binding sites when compared with the striatal expression of D4R mRNA, in agreement with their putative localization in cortico-striatal terminals. This was confirmed with experiments with frontal cortex ablation, which produced a significant reduction of striatal D4R binding sites (24).
The neocortex is composed of two major neuronal populations: glutamatergic pyramidal (P) neurons (70-80%) and GABAergic interneurons (20%-30%). There are several subtypes of GABAergic interneurons which have been grouped in three main classes, based on their transcriptional similarities and the expression of selective markers (26, 27). The largest class of cortical interneuron is characterized by the expression of the calcium-binding protein parvalbumin (PV). PV neurons target the perisomatic region of P neurons and control their spiking output. The second class expresses the neuropeptide somatostatin, and these neurons preferentially target the dendritic region of P neurons. And the third class expresses the 5-HT3a serotonin receptor, with a common subclass also expressing the calcium binding protein calretinin and the vasoactive intestinal peptide. These are mainly disinhibitory neurons that preferentially target PV+ and somatostatin+ interneurons. It has been postulated that the three subclasses of GABAergic interneurons establish a local cortical circuit that is critically involved in working memory, by which stimulus tuning of persistent activity arises from the concerted action of widespread inhibition mediated by PV+ interneurons and localized disinhibition of P neurons mediated by calretinin-containing interneurons (27, 28). In non-human primates, most P neurons and nearly half of the GABAergic interneurons express D4R. Among the interneurons, D4R are particularly expressed in PV+ interneurons (13, 14).
D4Rs are therefore positioned to exert a significant modulatory influence on frontal cortico-striatal P neurons. Considering the Gi-coupled D4R as mostly inhibitory, those localized in their cortical perisomatic region and striatal terminals should be expected to mediate an inhibitory effect of dopamine, while those localized in PV+ GABAergic interneurons should be expected to produce disinhibition. Nevertheless, a recent electrophysiological study on mouse cortical slices showed that D4R activation induces a more complex set of effects, with a direct slow decreasing effect on the excitability of P neurons and a fast and transient increase followed by a delayed decrease of the excitability of PV+ interneurons (29). The initial effect on PV+ interneurons should in fact lead to an initial potent suppression of PFC network activity and output signal. The effect of the delayed decrease of the excitability of PV+ interneurons on P neurons should be masked by the direct D4R-mediated decrease excitability of P neurons. In summary, activation of frontal cortical D4R should mostly keep a low output signal of the PFC network (29). In addition, a recent study using a combined optogenetic-microdialysis technique demonstrated the ability of D4Rs localized in frontal cortico-striatal terminals to exert a significant inhibitory role of striatal glutamate release (11). Overall, these studies suggest that D4Rs are instrumental for the dopamine-mediated functional inhibition of frontal cortico-striatal neurotransmission, in agreement with the hyperexcitability observed on frontal cortical P neurons in D4R-deficient mice (30). However, as reviewed below, several studies indicate a more complex picture, where D4Rs can also directly mediate the effects of norepinephrine and indirectly modulate the function of adrenoceptors and other dopamine receptor subtypes by heteromerization.
3 Lessons from the pineal gland. D4R heteromerization and D4R as a target for norepinephrine
Apart from the frontal cortex, D4Rs are highly expressed in the retina (31) and by the pinealocytes of the pineal gland (32), which main function is the circadian secretion of the hormone melatonin. This circadian control is mediated by a neuronal circuit that includes the suprachiasmatic and paraventricular nuclei of the hypothalamus, the intermediolateral column of the spinal cord and the superior cervical ganglion, which sends noradrenergic afferents to the pineal gland (33, 34). Darkness-induced norepinephrine release in the pineal gland activates pinealocyte Gs-coupled β1 and Gq-coupled α1B adrenergic receptors (β1R and α1BR), which promotes the synthesis and release of melatonin and its precursor serotonin (33, 34). Several experimental observations indicate that D4Rs play a fundamental role in the circadian adrenergic control of melatonin synthesis by pinealocytes and that this role depends on their circadian expression, their heteromerization-dependent ability to inhibit β1R and α1BR function and their ability to bind and be activated by norepinephrine (10, 32, 35).
D4R expression in the retina and pineal gland varies significantly in a circadian manner, being particularly elevated during the second half of the dark period (32). This increased expression is under the control of pineal β1R and α1BR and requires thyroid hormone (32). We found evidence indicating that D4R can form heteromers with both β1R and α1BR in mammalian transfected cells and in rat pinealocytes, and that the expression of β1R-D4R and α1B-D4R heteromers follows the circadian expression of D4R, being maximal at sunrise (10). In these heteromers, activation of D4R leads to a significant decrease in the ability of its partner adrenoceptor to signal (10), apparently suggesting that, in the pineal gland, dopamine inhibits the effect of norepinephrine. However, there is no clear evidence for a functional significant dopamine release in the pineal gland, where the catecholaminergic input is largely noradrenergic, the noradrenergic afferents from the superior cervical ganglion (33, 34).
A similar situation can be found in the cerebral cortex, particularly of rodents, which receives dense and widespread noradrenergic innervation, whereas dopaminergic terminals are restricted to the prefrontal cortex (36, 37). However, dopamine receptors are expressed throughout the cortex, and their localization exceeds that of the dopaminergic terminals (38–41). It has therefore been suggested that dopamine is either a co-neurotransmitter in noradrenergic neurons (42) or that there is a long-range volume-transmission of catecholamines (43). In fact, asynaptic varicosities represent most cortical norepinephrine releasing sites (37). A more parsimonious mechanism is the possibility that endogenous norepinephrine can also be an endogenous ligand of D4R. Thus, as demonstrated with in vitro experiments, norepinephrine binds and activates D4Rs at submicromolar concentrations (35, 44–46), up to ten times higher than the concentration able to activate β1R or α1BR in pineal cell preparations or pineal tissue (47, 48). Neverthelss, the potency of norepinephrine at activating pineal D4Rs needs to be determined. Altogether, a very plausible mechanism by which pineal D4Rs control melatonin synthesis and release emerges: at the beginning of the dark period, the initial noradrenergic activation of the pineal gland targets β1R and α1BR, favoring a progressive increase in melatonin synthesis, but also D4R expression; whereas at the end of the dark period, the increased expression of D4Rs leads to the formation of β1R-D4R and α1B-D4R heteromers, which allows norepinephrine to preclude β1R and α1BR signaling within the heteromer, thus dwindling melatonin synthesis and release (10).
4 The enigmatic role of DRD4 polymorphic variants. GPCR oligomerization comes to the rescue
The question of the functional significance of the different receptor isoforms encoded by DRD4 polymorphisms has remained enigmatic until recently. Those include electrophysiological and biochemical experiments in frontal cortical slices from D4R knockout mice with rescued expression of human D4.4R and D4.7R by viral transduction (49, 50), and immunohistochemical and in vivo optogenetic-microdialysis experiments in D4.7R knock-in mice expressing a humanized D4R with the 3IL of the human D4.7R (11). Electrophysiological experiments in frontal cortical slices from D4R knockout mice showed an increased ability of a D4R agonist to suppress network bursts and NMDA receptor-mediated excitatory postsynaptic currents from P neurons after viral transduction of human D4.7R, as compared with human D4.4R receptor cDNA (49, 50). Interestingly, biochemical experiments in the same slice preparations revealed that these differences correlated with a more profound D4R agonist-mediated downregulation of NMDA receptor surface expression (i.e., NR1 subunit) in frontal cortical slices virally transduced with D4.7R than with D4.4R (49). The in vivo experiments in D4.7R knock-in mice showed a blunting of methamphetamine-induced cortical activation and ontogenetically and methamphetamine-induced frontal cortico-striatal glutamate release (11). In summary, these studies showed a pronounced gain of function of D4.7R, as compared to D4.4R, in its ability to mediate the inhibitory influence of dopamine on frontal cortico-striatal neurotransmission.
The next enigma to solve was the mechanism behind the functional differences of the products of DRD4 polymorphisms. Thus, in mammalian transfected cells, D4.2R, D4.4R and D4.7R did not show clear pharmacological differences in response to endogenous or exogenous ligands, although an earlier study seemed to indicate that D4.7R signals with less efficiency than D4.4R (51). Nevertheless, by using a functional bioluminescence resonance energy transfer (BRET) technique, we could not find significant differences in the ability of the D4.2R, D4.4R or D4.7R polymorphic variants to promote dopamine-induced activation of any of the five Gi/o protein subtypes (35). Importantly, differences emerged when studying their ability to form heteromers, more specifically with the Gi-coupled D2R and α2AR, preferentially localized in the perisomatic region and nerve terminals of prefrontal cortical P neurons, respectively (52–54).
Apart from D4Rs, a significant proportion of frontal cortical P neurons from layer V also express D2Rs (in monkeys, about 75% and 55%, respectively) (14). Furthermore, in vitro experiments in striatal slices (52) and in vivo optogenetic-microdialysis experiments in rodents (11, 55) have provided evidence for the presence of functional D2R and D4R receptors in cortico-striatal glutamatergic terminals. This provides the framework for the existence of D2R-D4R heteromers in striatal terminals and, possibly, in the perisomatic region of P neurons. The first studies on heteromers of the D4R polymorphic variants, based on BRET and co-immunoprecipitation techniques in transfected mammalian cells, suggested that the D4.7R establishes weaker intermolecular and functional interactions with the D2R (both isoforms, D2LR and D2SR) than the D4.4R (52, 56, 57). At the functional level, co-transfection of D2Rs with D4.4Rs, but not D4.7Rs, led to an increase in MAPK signaling (52, 57), which would seem opposite to the expected D4.7R gain of function. Nevertheless, a dependence on heteromerization of these differences in MAPK signaling was not clearly established. On the other hand, a more recent study using the complemented donor acceptor bioluminescence resonance energy transfer (CODA-RET) technique demonstrated a D4.7R heteromerization-dependent gain of function of the D2R (53).
CODA-RET is a BRET assay that allows the measurement of ligand-induced changes in the interaction between G protein-coupled receptors (GPCRs) forming specific homomers or heteromers with transducer proteins, including G proteins (58). In the CODA-RET assay, two complementary halves of a bioluminescent chromophore, such as Renilla Luciferase are separately fused to two different receptor molecules putatively able to oligomerize and a fluorescent chromophore (such as yellow fluorescent protein) can be fused to a G protein subunit. Ligand-induced changes in CODA-RET measurements imply, first, a successful complementation of Renilla Luciferase and, therefore, oligomerization of the corresponding GPCR units. Second, although CODA-RET does not provide estimates of the degree of oligomerization, such as the affinity of the intermolecular interactions between GPCR subunits or the proportion of subunits forming oligomers, this technique does allow a qualitative measure of the ability of a specific endogenous or exogenous ligand to activate a GPCR homodimer or heterodimer and signal through a specific G protein (58, 59). Thus, using CODA-RET, we were able to disclose, for the first time, a different qualitative profile of several D2-like receptor ligands for D4.4R and D4.7R, but only when forming heteromers with D2R or α2AR (53, 54).
Using CODA-RET, we found two possible mechanisms for the D4.7R gain of function as mediator of an inhibition of frontal cortico-striatal neurotransmission (Figure 1): first, a specific increase in the potency of dopamine for the D2R-D4.7R, but not for D2R-D4.4R heteromer, as compared with the D2R homomer (53); second, a divergent decrease or increase in the constitutive activity of D2R when it was forming D2R-D4.4R or D2R-D4.7R heteromers, respectively (53). In addition, a fraction of D4R should not be expected to form heteromers, but homomers, which should be more prevalent with D4.7R than with D4.4R. In fact, as compared with D4.4R, not only D4.7R seems to be less able to heteromerize with D2R (52, 56, 57), but it also seems to be more able to homomerize (56). Since CODA-RET experiments also demonstrated a significantly higher potency of dopamine for D4.4R-D4.4R and D4.7R-D4.7R homomers than for D2R-D4.4R and the D2R-D4.7R heteromers (53), the expected larger population of D4.7R-D4.7R versus D4.4R-D4.4R homomers should represent a third mechanism involved in the gain of function of D4.7R (Figure 1).
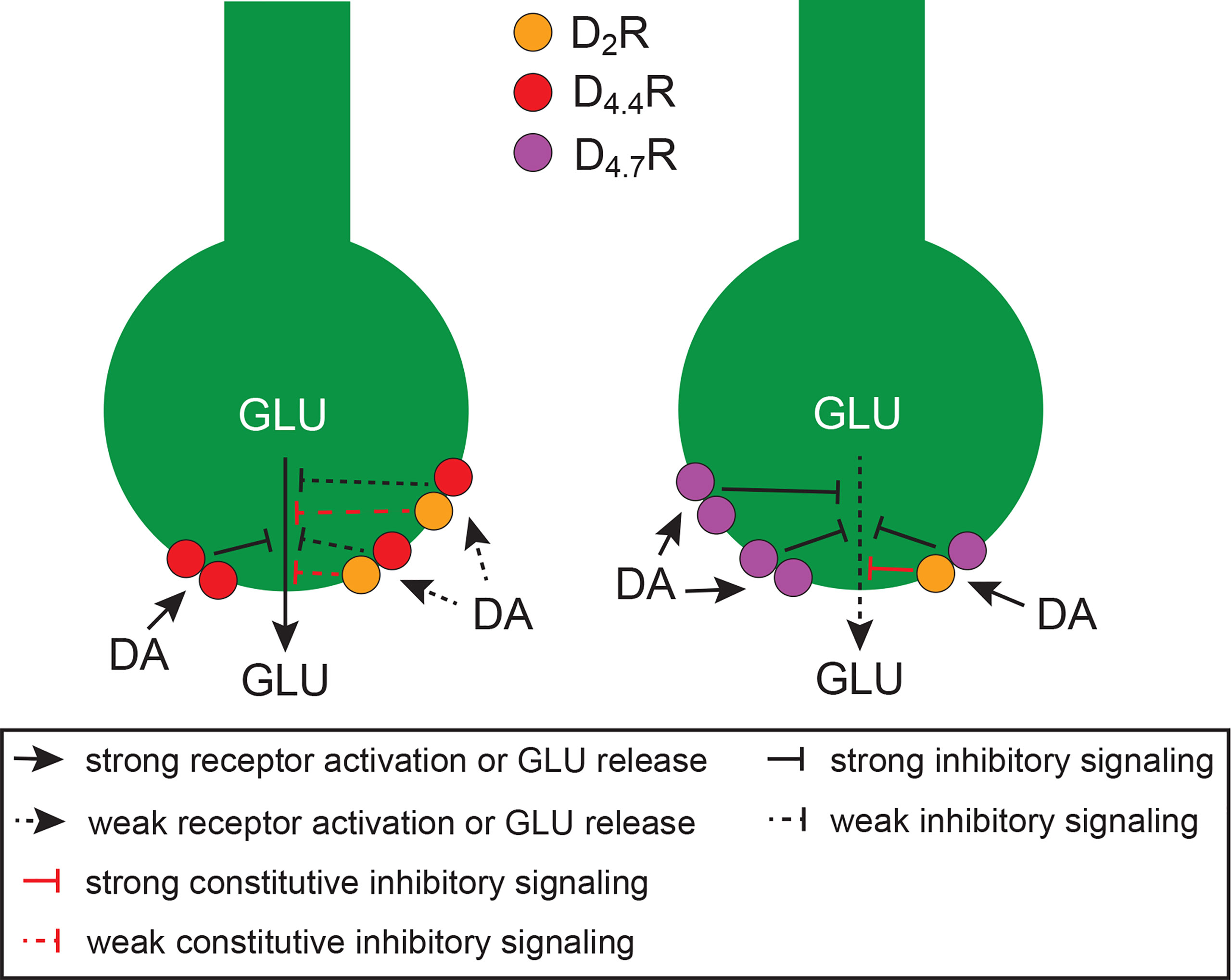
Figure 1 Schematic representation of cortico-striatal glutamatergic terminals and their modulatory D4.4Rs (left terminal) and D4.7Rs (right terminal), which form heteromers with D2Rs. Heteromerization of D2R with D4.7R promotes a gain of function of the dopaminergic-mediated inhibition of glutamate (GLU) release, as compared with heteromerization with D4.4R, since it increases the potency for dopamine (DA) and the constitutive activity of the D2R. In addition, the population of D4.7Rs not forming heteromers with D2Rs is larger than with D4.4Rs, which increases the potency of DA to inhibit GLU release, because of the higher affinity of DA for D4Rs versus D2Rs. The scheme is based on results obtained from experiments in mammalian transfected cells and from in vitro and in vivo experiments in rodents (see text).
In view of the involvement of D4R and α2AR in impulsivity and ADHD (see next section), their concurrent localization in frontal cortical P neurons (see below) and the demonstrated ability of D4R to form functional heteromers with other adrenoceptors in the pineal gland (see above), we investigated the possible existence of functionally significant α2AR-D4.4R and α2AR-D4.7R heteromers in the brain. Using several biophysical and biochemical techniques and using heteromer-specific disruptive peptides, we demonstrated the ability of both D4.4R and D4.7R to heteromerize with α2AR both in mammalian transfected cells and in the mouse cerebral cortex (54). The results of BRET experiments indicated that, akin to D2R-D4R heteromers, the D4.7R variant was less able to heteromerize with α2AR than D4.4R. Furthermore, results from radioligand-binding, CODA-RET and signaling experiments indicated that heteromerization with D4.7R, but not with D4.4R, increases the potency of norepinephrine at activating α2AR. Furthermore, D4.4R, but not D4.7R activation, allosterically inhibited α2AR-mediated signaling in their respective heteromers (54). Thus, dopamine should be able to promote a significant inhibitory effect of α2AR signaling though α2AR-D4.4R, but not α2AR-D4.7R heteromers. Furthermore, as elaborated below, D4R can also be activated by endogenous norepinephrine in the cerebral cortex, and high concentrations should determine a significant inhibition of α2AR signaling by the α2AR-D4.4R, but not by the α2AR-D4.7R heteromer. If the main functional output of α2AR-D4R heteromers is a decrease in excitability of P neurons (see below, section 6) this could provide an additional mechanism for the gain of function of D4.7R in its inhibitory control of frontal cortico-striatal neurotransmission (Figure 2).
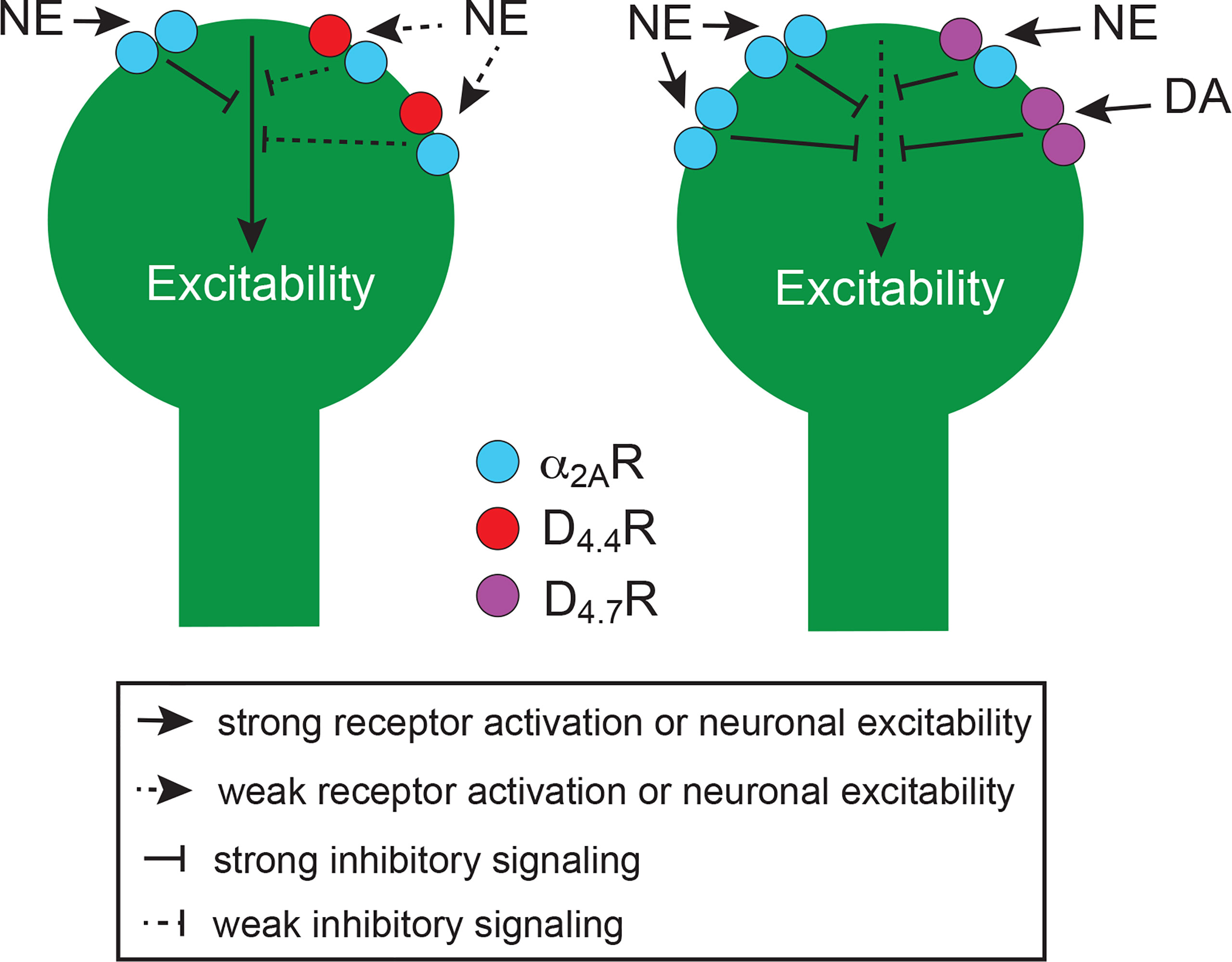
Figure 2 Schematic representation of the perisomatic region of frontal cortical P neurons and their modulatory D4.4Rs (left neuron) and D4.7Rs (right neuron), which form heteromers with α2ARs. Heteromerization of α2AR with D4.7R promotes a gain of function of the noradrenergic-mediated inhibition of neuronal excitability, as compared with heteromerization with D4.4R, since it increases the potency of norepinephrine (NE). In addition, high concentrations of NE promote a decrease in the potency of NE in the α2AR-D4.4R but not in the α2AR-D4.7R heteromer (see text). Furthermore, the population of D4.7Rs not forming heteromers with α2AR is larger than with D4.4Rs, which also increases the population of α2AR not forming heteromers and therefore the potency of NE to decrease neuronal excitability. The increased proportion of D4.7Rs not forming heteromers additionally increases the ability of DA to decrease neuronal excitability. The scheme is based on results obtained from experiments in mammalian transfected cells and from in vitro experiments in rodents (see text).
5 D4R as a moderator of the personality traits action and choice impulsivity
The most popular models of personality are the Big-Three and the Big-Five models, operationalized by the Multidimensional Personality Questionnaire (MPQ) (60) and the NEO Personality Inventory-Revised (NEO-PI-R) psychometric tests (61), respectively. The MPQ measures three orthogonal traits: positive emotionality (PEM), negative emotionality (NEM) and constraint (CON) (60, 62). PEM and NEM incorporate dispositions toward positive and negative emotions, respectively, and are linked conceptually to the brain systems underlying appetitive-approach and defensive-withdrawal behaviors. Constraint (CON) encompasses dimensions related to behavioral restraint, the opposite end of which implies disinhibition. NEO-PI-R (61) measures neuroticism (N), extraversion (E), openness (O), agreeableness (A) and conscientiousness (C). N and E highly correlate with NEM and PEM respectively, generally constituting the same personality constructs (63, 64). O captures interest toward experience, and A implies an empathic personality. Finally, C is a spectrum of constructs that describes individual differences in the propensity to be self-controlled, responsible to others, hardworking, orderly, and rule abiding (65).
Impulsivity is defined as a predisposition toward rapid, unplanned reactions to internal or external stimuli with little regard for the negative consequences to the individual or others (66). Impulsivity has been decomposed into “rapid-response” or “action” impulsivity, and “cognitive” or “choice” impulsivity (67–69). Action impulsivity (AI) is defined as a diminished ability to inhibit prepotent responses, or a failure of volitional motor inhibition or disinhibition (68). Choice impulsivity (CI) implies a tendency to accept small immediate or likely rewards at the expense of large delayed or unlikely rewards (69). Excessive CI overlaps conceptually with impairment in decision-making and particularly with temporal or delay discounting (70). Delay discounting is the phenomenon by which a delayed outcome of a choice reduces the subjective value of a reward and constitutes an operational measure of the degree of CI (71, 72). We have previously maintained that AI constitutes the same concept as strong disinhibition, the opposite end of the personality trait CON (9), and that the trait C encapsulates both dimensions of impulsivity (AI and CI) (73). In fact, a significant correlation between measures of CON and C has been reported (63, 64). We have also argued that the same as AI, CI fulfills the criteria to be considered as a personality trait (73). The substantial overlap of several of these personality traits with maladaptive behaviors and specifically, with mental health disorders, justifies the sustained search for their neural underpinnings as therapeutic targets. But this has been a significant challenge for the field of neuropsychiatry.
The ‘endophenotype’ concept has provided an invaluable approach for the identification of genes that predispose or indemnify individuals from mental and psychiatric disorders. The endophenotype concept is understood as simpler clues to genetic underpinnings than the disease syndrome itself and involves the genetic analysis of any of a variety of biological markers (cognitive, neurophysiological, anatomical, biochemical, etc.) of the disease. The concept promotes the view that psychiatric diagnoses can be decomposed or deconstructed into more tractable intermediate phenotypes by virtue of their assumed proximity to the genetic antecedents of the disease (74, 75). Based on the results of studies that have linked the structure of psychopathology to the structure of personality, as defined by the MPQ or NEO-PI-R (76), we argued previously that specific personality traits constitute endophenotypes of mental health disorders, such as SUD (9).
We initially identified PEM/E (from the MPQ and NEO-PI-R assessments, respectively), NEM/N (again, from the two assessments, respectively), and CON (the inverse measure of AI), as tied to specific brain circuits and genes (9). PEM/E is modulated by the function of the central dopaminergic system and is moderated by the D2R gene. NEM/N is modulated by the glutamatergic outputs from the right anterior cingulate cortex and ventromedial prefrontal cortex to the amygdala and insula and is moderated by the serotonin transporter gene. AI is modulated by a circuit including the glutamatergic neurons arising in the pre-supplementary motor area and right inferior frontal gyrus and innervating the dorsal striatum and the subthalamic nucleus and is moderated by the genes of the D4R and the dopamine transporter (9) (Figure 3). Individuals with low PEM/E, high NEM/N and high AI would be most vulnerable (least resilient) to develop SUD. Conversely, individuals with high PEM/E, low NEM/N and low AI would be least vulnerable (most resilient) to SUD (9).
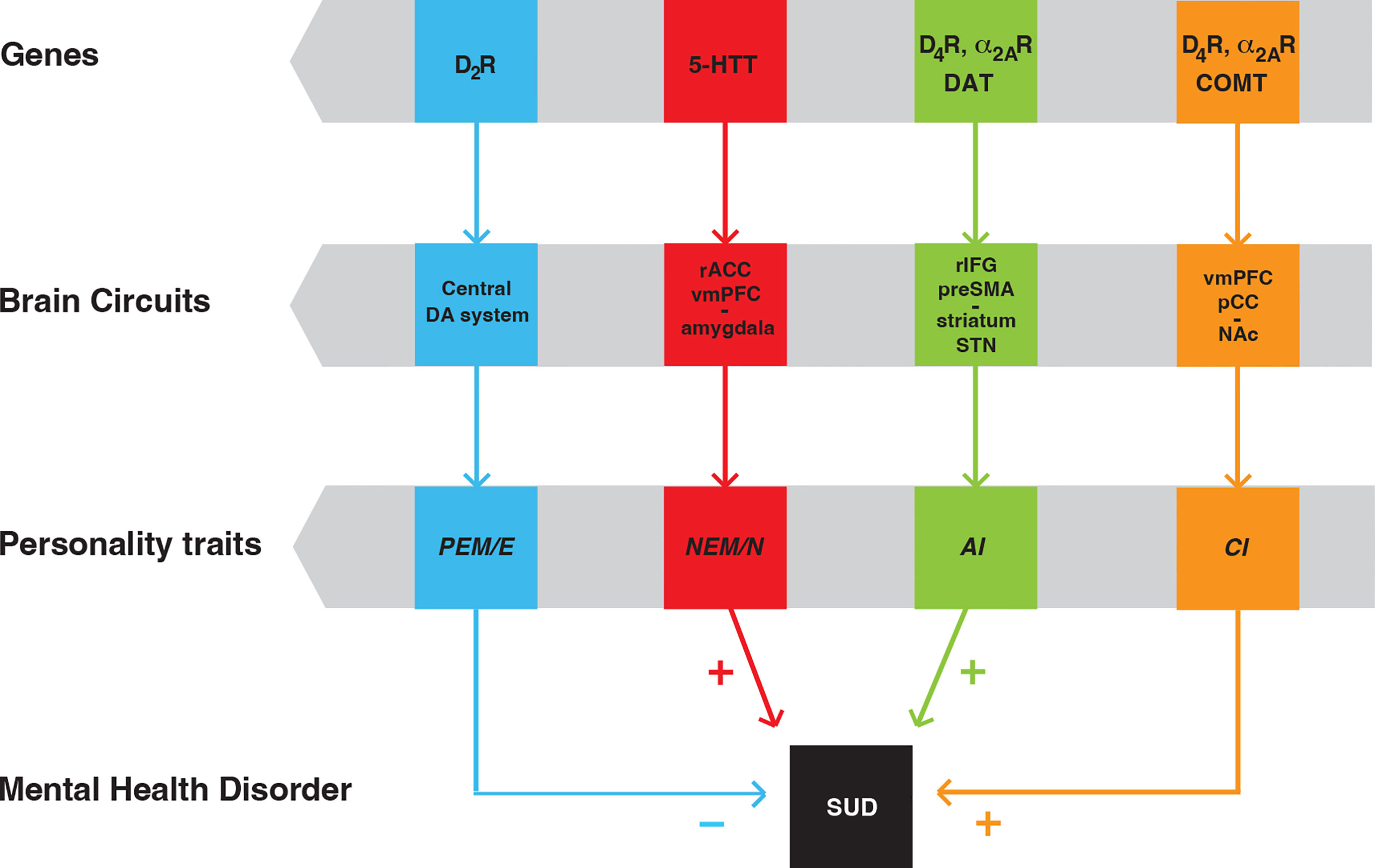
Figure 3 Scheme of personality traits as endophenotypes of SUD, with their linkage to specific brain circuits and genes. Positive emotionality/extroversion (PEM/E) is modulated by the central dopaminergic (DA) system and is moderated by the D2R gene. Negative emotionality/neuroticism (NEM/N) is modulated by a circuit that involves the right anterior cingulate cortex (rACC), ventromedial prefrontal cortex (vmPFC) and the amygdala and is moderated by the gene of the serotonin transporter (5-HTT). Action impulsivity (AI) is modulated by a circuit that includes the pre-supplementary motor area (preSMA), the right inferior frontal gyrus (rIFG), the striatum and the subthalamic nucleus (STN). Choice impulsivity (CI) is modulated by a circuit that includes the ventromedial prefrontal cortex (vmPFC), the posterior cingular cortex (pCC) and the nucleus accumbens (NAc). AI and CI are moderated by the genes of the dopamine transported (DAT) and the enzyme cathecol-O-methyltransferase (COMT), respectively. In addition, AI and CI are both moderated by the genes of D4R and α2AR. “+” and “-” indicate that individuals with low PEM, high NEM, high AI and high CI are most vulnerable (least resilient) to develop SUD [modified from ref (71)].
To our initial analysis, we added CI as an additional personality trait, modulated by a circuit including glutamatergic neurons from the ventromedial prefrontal cortex and the posterior cingular cortex that innervate the ventral striatum (nucleus accumbens) and, as with AI, is also moderated by the D4R gene, and by the gene of the enzyme catechol-O-methyltransferase (73) (Figure 3). The fact that D4R moderates both AI and CI provide a clue for the apparent orthogonality of the C trait, as it encapsulates both traits (see above), which constitute endophenotypes for ADHD (76–80) and SUD (9, 73). Not surprisingly, as for SUD, low C is a consistent finding in ADHD (81), which if left untreated, constitutes a risk factor for SUD (82–84).
The same as for the D4R gene, polymorphisms of the α2AR gene may confer vulnerability to developing ADHD as well as symptoms of impulse control disorders (85, 86). However, a large meta-analysis did not find a consistent significant association (87). Yet, when studied at the intermediate phenotype level, as an endophenotype, a clear significant association was established between α2AR gene polymorphisms and AI (88). Additionally, the α2AR agonist guanfacine, which is currently used in the symptomatic treatment of ADHD (89), significantly decreases delay discounting in nonhuman primates (90). Our recent study on α2AR-D4R heteromers brings together two key receptors involved in the pathogenesis and treatment of ADHD, since α2AR-D4R heteromers represent a significant population of both catecholaminergic receptors in the mouse cerebral cortex (54).
6 D4R antagonists as a plausible treatment for ADHD and other impulse-control disorders
Apart from the association with D4.7R, several other preclinical and clinical findings converge on the involvement of D4R and its heteromers in ADHD and other impulse-control disorders and, therefore, on its possible utility as a therapeutic target for those disorders. At the preclinical level, several animal models of ADHD with varied face, construct, and predictive validity have been developed, particularly in rodents (91). The complexity of the clinical symptoms and pathology of ADHD has been very challenging for the development of those models and, unfortunately, the lack of knowledge about the etiology and pathogenetic mechanisms of the disorder hampers their construct validity.
The most consistent pathogenetic finding in ADHD is a frontal cortical hypoactivity (92, 93), for which there is not yet a clearly accepted explanation. Although it might seem counterintuitive that a decrease and not an increase in cortico-striatal glutamatergic transmission is associated with impulsivity and ADHD, this could probably be explained by considering the well-established differential effects of the activation of the direct and indirect striatal efferent pathways. Classically, the direct and indirect pathways have been conceptualized as gas and brake pedals of the output signals of the basal ganglia (“Go” and “NoGo” pathways), respectively (94). It therefore seems that a sufficient decrease in the activation of the indirect pathway (releasing the gas pedal) determines an increase in the basal ganglia output, irrespective of a concomitant decreased activation of the direct pathway. Considering a more cognitive conceptualization, which includes the processing of information of relevant and irrelevant stimuli (95), a blunted cortico-striatal neurotransmission affecting the activity of both the direct and indirect striatal efferent pathways should decrease their respective ability to increase the reactivity to reward-related stimuli and to suppress the reactivity to nonrewarded- or aversive-related stimuli. As previously proposed (11), the outcome should be an increased “interest” for irrelevant stimuli and a reduced inhibition of irrelevant responses, which could be important in explaining not only the impulsivity, but also the attentional deficit of ADHD.
Genetic manipulations of the D4R in the experimental animal have provided significant correlative information supporting the role of a decrease in striatal glutamatergic transmission in ADHD. As mentioned before, D4R-knockout mice showed hyperexcitability of frontal cortical P neurons (30), while the gain of function provided by D4.7R induced the opposite effect, with a decrease in cortico-striatal glutamatergic transmission (11). In addition, one of the classical animal models of ADHD, the rodent with neonatal lesions with 6-OH-dopamine, showed an ADHD-like phenotype, including locomotor hyperactivity, paradoxical hypolocomotor response to amphetamine and methylphenidate and poor behavioral inhibition, which was counteracted by genetic or pharmacological blockade of the D4R (96, 97). Furthermore, it was also shown that locomotor hyperactivity in 6-OH-dopamine-lesioned rats correlate with increases in the striatal density of D4R (97).
The 6-OH-dopamine ADHD rodent model also demonstrated predictive value, since apart from amphetamine and methylphenidate, selective norepinephrine uptake inhibitors were effective at counteracting locomotor hyperactivity (98). In fact, apart from amphetamine and methylphenidate, the inhibitor of the norepinephrine transporter atomoxetine and the α2AR agonists guanfacine and clonidine are the most accepted pharmacological treatments for ADHD (89). In the cortex, α2ARs are preferentially localized postsynaptically, in P neurons of the deep layers (99, 100), therefore, potentially co-localized with D4R. In fact, as mentioned before, we have recently provided experimental evidence indicating that α2AR-D4R heteromers represent a significant population of both receptors in the mouse cerebral cortex (54). Two different and opposite neuronal effects, both dependent on Gi protein-mediated decrease in cAMP formation, have been described upon activation of cortical postsynaptic α2AR: an excitatory effect, dependent on the inactivation of hyperpolarization-activated cyclic nucleotide-gated (HCN) channels (101), and an inhibitory effect, dependent on the inactivation of AMPA receptors (102, 103). These results indicate the existence of two different functional populations of α2AR. It is assumed that the excitatory effect mediates the therapeutic effect of α2AR agonists, by counteracting the cortical frontal hypoactivity of ADHD (100). It was then suggested that the inhibitory effect would be mediated by a different population of α2AR, which could provide a protective mechanism upon overstimulation by high levels of norepinephrine released under stress conditions (102).
It is plausible, and experimentally testable, that D4Rs mostly heteromerize and modulate the population of α2ARs that mediate the inhibitory effect of P neuronal function. This could explain at least part of the protective effect of D4.4R in ADHD, as well as the D4.7R-mediated increased vulnerability to develop ADHD. Thus, the same as with pineal β1R-D4R and α1B-D4R heteromers, the cortical α2AR-D4.4R heteromer could function as a norepinephrine concentration-sensing device, where high concentrations activate D4.4R and counteract the effect of the activation of the α2AR in the heteromer, diminishing the α2AR-mediated inhibitory effect on P neuronal function. On the other hand, the increase in the potency of norepinephrine for α2AR in the α2AR-D4.7R heteromer would facilitate the α2AR-mediated inhibitory effect on P neuronal function (Figure 2). As in cortico-striatal terminals, activation of D4R localized in the perisomatic region of P neurons not forming heteromers (or possible forming heteromers with D2R) should directly promote an inhibition of the activity of P neurons (Figure 2). D4R antagonists should then be considered as possible therapeutic targets for ADHD, as predicted by the positive results obtained in rodents with neonatal 6-OH-dopamine lesions (96, 97), specially, when considering their additional ability to counteract the dopamine-mediated inhibition of glutamate release by cortico-striatal terminals.
However, a randomized, double-blind, crossover study with the selective D4R antagonist L-745,870 (also named MK-0929) in adults with ADHD did not demonstrate a significant effect over placebo (104). To our knowledge, this is the only published study addressing the possible clinical efficacy of D4R antagonists in ADHD. Obvious limitations, such as the small number of patients, short duration of each treatment period and lack of quantitative cognitive assessments of inhibitory control and other executive functions cannot be ignored. Importantly, D4.7R polymorphism characterization was not included in this study, which may also explain the negative findings. Apart from the above reviewed preclinical role of D4R as a very significant mediator of the effect of dopamine and norepinephrine on the function of frontal cortical P neuronal function, there is the unequivocal association of D4.7R to ADHD. It would therefore be important to carry out more clinical studies with D4R antagonists, which should also include addressing their ability to modify the underlying endophenotypes AI and CI (9, 73).
7 D4R agonists as plausible treatment of restless legs syndrome
The term akathisia is used to define an urgent need to move. Thus, the primary component of akathisia is a sensory experience which acts as a “drive” or “motivational state” that compels the subject to move. This is objectively perceived by an observer as restlessness or motor hyperactivity (105). Akathisia is also implicit in the description of the symptoms of the very prevalent sensorimotor disorder RLS, where, more often, the sensory experience is an urgent need to specifically move one’s legs (106). Brain iron deficiency (BID), more often without concomitant peripheral iron deficiency, is recognized as the main initial pathogenetic mechanism in RLS (107, 108). BID seems then to trigger a series of pathogenetic mechanisms, including an increase in the motor-cortical and thalamic excitability, conceptualized as a hyperglutamatergic state, that seems to underly RLS symptomatology (108).
BID in rodents is a well-accepted animal model of RLS, which can be induced by providing a severe iron-deficient diet during the postweaning period. The model has both construct and face validity since it recapitulates several biochemical and behavioral findings of RLS (109, 110). Using the optogenetic-microdialysis method, an increase in the sensitivity of cortico-striatal terminals to release glutamate could be demonstrated in the rat with BID, since a lower frequency of optogenetic stimulation was necessary to induce striatal glutamate release as compared to controls (55). This increased sensitivity seems to be related to a BID-induced alteration in the expression of adenosine receptor subtypes in the cortico-striatal glutamatergic terminals, with a downregulation of adenosine A1 receptors (A1Rs) and a relative upregulation of adenosine A2A receptors (A2ARs) (111, 112). Using the optogenetic-microdialysis method, evidence was also obtained for the ability of the equilibrative nucleoside transporter dipyridamole, which increases the extracellular levels of adenosine, to significantly inhibit cortico-striatal glutamate in control rats and in rats with BID (113). The results of these experiments indicated a possible therapeutic effect of dipyridamole, which was recently demonstrated by two clinical studies, an open trial, and a randomized, placebo-controlled crossover study (114, 115). These studies provided a new therapeutic approach for RLS and significantly validated the cortico-striatal glutamatergic terminals as targets for the treatment of RLS.
Using the same model, perfusion of the most prescribed drugs in RLS, pramipexole, ropinirole and gabapentin, all counteracted the ability of optogenetic stimulation to induce glutamate release, both in controls and in rats with BID (55). By binding to the α2δ subunit of voltage-dependent calcium channels localized in glutamatergic terminals, gabapentin reduces their function and trafficking, therefore decreasing striatal glutamate release (116). Pramipexole and ropinirole are non-selective D2-like receptor agonists with a slightly higher affinity for D3R. It has therefore been suggested that their therapeutic effect is related to their preferential affinity for D3R (117). However, the effect of pramipexole on the optogenetically induced glutamate release was not antagonized by a selective D3R antagonist, but by D2R and D4R antagonists (55). These results, therefore, provided a significant support to the key mediation of D2R and D4R in the local striatal inhibitory control of dopamine on cortico-striatal glutamate release.
The predictive value offered by the conceptual framework of an increased sensitivity of cortico-striatal terminals in RLS points to D4R agonists as a possible new treatment for RLS. This could possibly avoid secondary effects of the currently used dopaminergic compounds, which very commonly lead not only to disappearance of their therapeutic effect, but to an increase in the RLS symptoms, known as “augmentation” (118). Thus, it is conceivable that augmentation is secondary to activation of postsynaptic dopamine receptors, similar to the mechanism involved in L-DOPA-induced dyskinesia, a common complication of the treatment with dopaminergic compounds in Parkinson’s disease (119).
8 Conclusions
We reviewed the evidence conveying a significant role of D4R in the dopaminergic and noradrenergic modulation of the frontal cortico-striatal pyramidal neuron, with implications for the moderation of constructs of impulsivity as personality traits. We also reviewed the evidence strongly supporting that these D4Rs should be exploited as therapeutic targets for ADHD and other impulse-control disorders and for RLS. Special emphasis was placed on the concept of receptor heteromerization, which has played a fundamental role in the understanding of D4R function and in the understanding of the different functional differences between D4R polymorphic variants.
Particularly striking is the fact that the most common polymorphic variants, D4.4R and D4.7R (with allelic frequencies of about 60% and 20%, respectively) (4), confer significantly different functional and pharmacological properties to α2AR-D4R and D2R-D4R heteromers, which mediate a dopamine- and norepinephrine-dependent fine-tune modulation of the frontal cortico-striatal glutamatergic neuronal function. This can explain the differential effect of D4R polymorphisms in the moderation of the personality traits AI and CI and their role as endophenotypes of impulse-control disorders, including ADHD and SUD. More specifically, it can explain the association of D4.7R with impulse-control disorders. The demonstrated mediation of a stronger inhibition of cortico-striatal glutamatergic transmission mediated by D4.7R (in D4.7R knock-in mouse expressing a humanized D4R with the 3IL of the human D4.7R) (11), would then disclose a mechanism determining an increase in AI and CI.
Reviewing the results from experimental models of ADHD and RLS, it becomes evident that selective D4R antagonists and agonists could be respectively effective. Although a single clinical study with a D4R antagonist resulted negative in the treatment of ADHD (104), we believe that the reviewed preclinical evidence calls for additional clinical studies. Apart from addressing the ability of D4R antagonists to modify the underlying endophenotypes AI and CI, the role of D4R polymorphisms should also be addressed. Similarly, to our knowledge, there are no studies concerning the frequency of the different D4R polymorphic variants in RLS patients, and a prediction could be made about an expected lower frequency of D4.7R. As mentioned above, we also found a different qualitative profile of several D2-like receptor ligands for D4.4R and D4.7R, but only when forming heteromers with D2R or αAR (52, 53). Therefore, when searching for new D4R ligands, D4R polymorphisms and D4R heteromers (D2R-D4R and α2AR-D4R heteromers) should be considered as targets, which could provide a more effective and individualized treatment. Importantly, in this review we only considered the most prevalent and studied D4R polymorphisms, and more studies need to be performed to evaluate the specific properties of less common yet prevalent polymorphisms.
Finally, in this review we have not discussed the role of D4R localized in brain areas other than the frontal cortico-striatal pyramidal neuron and the pinealocytes. The globus pallidus and the lateral habenula are additional regions where D4Rs have been shown to play a significant role in the mediation of inhibitory transmission by dopamine or norepinephrine, respectively (120–122). The present review emphasizes the need to find the heteromeric partners of the D4R and to establish the differential functional and pharmacological role of its polymorphic variants.
Author contributions
All authors listed have made a substantial, direct, and intellectual contribution to the work and approved it for publication.
Funding
S.F. is supported by the intramural funds of the National Institute on Drug Abuse. E. M., V.C.-A. and V.C. are supported by “Ministerio de Ciencia e Innovación/Agencia Estatal de Investigación’ (MCIN/AEI/10.13039/501100011033) and FEDER (SAF2017–87629-R and PID2020–113938RB-I00). V.C.-A also acknowledges the Spanish MCIN/AEI/10.13039/501100011033 for a ‘Juan de la Cierva’ fellowship (FJC2019-041020-I).
Conflict of interest
The authors declare that the research was conducted in the absence of any commercial or financial relationships that could be construed as a potential conflict of interest.
Publisher’s note
All claims expressed in this article are solely those of the authors and do not necessarily represent those of their affiliated organizations, or those of the publisher, the editors and the reviewers. Any product that may be evaluated in this article, or claim that may be made by its manufacturer, is not guaranteed or endorsed by the publisher.
Abbreviations
A, agreeableness; A1R and A2AR, adenosine A1 and A2A receptor; ADHD, attention-deficit hyperactivity disorder; α1BR, α2AR and β1R, adrenergic α1B, α2A and β1 receptor; AI, action impulsivity; BID, brain iron deficiency; BRET, bioluminescence resonance energy transfer; C, conscientiousness; CI, choice impulsivity; CODA-RET, complemented donor acceptor bioluminescence resonance energy transfer; CON, constraint; D1R, D2R, D3R and D4R, dopamine D1, D2, D3 and D4 receptor; DRD4, human D4R gene; D4.2R, D4.4R and D4.7R, products of DRD4 polymorphic variants with 2, 4 and 7 tandem repeats; E, extraversion; GPCR, G protein-coupled receptor; 3IL, third intracellular loop; MPQ, Multidimensional Personality Questionnaire; N, neuroticism; NEM, negative emotionality; NEO-PI-R, NEO Personality Inventory-Revised; O, openness; PEM, positive emotionality; P neuron, pyramidal neuron; PV, parvalbumin; RLS, restless legs syndrome; SUD, substance use disorder.
References
1. Van Tol HH, Bunzow JR, Guan HC, Sunahara RK, Seeman P, Niznik HB, et al. Cloning of the gene for a human dopamine D4 receptor with high affinity for the antipsychotic clozapine. Nature (1991) 350(6319):610–4. doi: 10.1038/350610a0
2. Lindsley CW, Hopkins CR. Return of D4 dopamine receptor antagonists in drug discovery. J Med Chem (2017) 60(17):7233–43. doi: 10.1021/acs.jmedchem.7b00151
3. LaHoste GJ, Swanson JM, Wigal SB, Glabe C, Wigal T, King N, et al. Dopamine D4 receptor gene polymorphism is associated with attention deficit hyperactivity disorder. Mol Psychiatry (1996) 1(2):121–4.
4. Chang FM, Kidd JR, Livak KJ, Pakstis AJ, Kidd KK. The world-wide distribution of allele frequencies at the human dopamine D4 receptor locus. Hum Genet (1996) 98(1):91–101. doi: 10.1007/s004390050166
5. Wang E, Ding YC, Flodman P, Kidd JR, Kidd KK, Grady DL, et al. The genetic architecture of selection at the human dopamine receptor D4 (DRD4) gene locus. Am J Hum Genet (2004) 74(5):931–44. doi: 10.1086/420854
6. Faraone SV, Perlis RH, Doyle AE, Smoller JW, Goralnick JJ, Holmgren MA, et al. Molecular genetics of attention-deficit/hyperactivity disorder. Biol Psychiatry (2005) 57(11):1313–23. doi: 10.1016/j.biopsych.2004.11.024
7. Li D, Sham PC, Owen MJ, He L. Meta-analysis shows significant association between dopamine system genes and attention deficit hyperactivity disorder (ADHD). Hum Mol Genet (2006) 15(14):2276–84. doi: 10.1093/hmg/ddl152
8. Gizer IR, Ficks C, Waldman ID. Candidate gene studies of ADHD: a meta-analytic review. Hum Genet (2009) 126(1):51–90. doi: 10.1007/s00439-009-0694-x
9. Belcher AM, Volkow ND, Moeller FG, Ferré S. Personality traits and vulnerability or resilience to substance use disorders. Trends Cognit Sci (2014) 18(4):211–7. doi: 10.1016/j.tics.2014.01.010
10. González S, Moreno-Delgado D, Moreno E, Pérez-Capote K, Franco R, Mallol J, et al. Circadian-related heteromerization of adrenergic and dopamine D4 receptors modulates melatonin synthesis and release in the pineal gland. PloS Biol (2012) 10(6):e1001347. doi: 10.1371/journal.pbio.1001347
11. Bonaventura J, Quiroz C, Cai NS, Rubinstein M, Tanda G, Ferré S. Key role of the dopamine D4 receptor in the modulation of corticostriatal glutamatergic neurotransmission. Sci Adv (2017) 3(1):e1601631. doi: 10.1126/sciadv.1601631
12. Meador-Woodruff JH, Damask SP, Wang J, Haroutunian V, Davis KL, Watson SJ. Dopamine receptor mRNA expression in human striatum and neocortex. Neuropsychopharmacology (1996) 15(1):17–29. doi: 10.1016/0893-133X(95)00150-C
13. Noaín D, Avale ME, Wedemeyer C, Calvo D, Peper M, Rubinstein M. Identification of brain neurons expressing the dopamine D4 receptor gene using BAC transgenic mice. Eur J Neurosci (2006) 24(9):2429–38. doi: 10.1111/j.1460-9568.2006.05148.x
14. de Almeida J, Mengod G. D2 and D4 dopamine receptor mRNA distribution in pyramidal neurons and GABAergic subpopulations in monkey prefrontal cortex: implications for schizophrenia treatment. Neuroscience (2010) 170(4):1133–9. doi: 10.1016/j.neuroscience.2010.08.025
15. Ariano MA, Wang J, Noblett KL, Larson ER, Sibley DR. Cellular distribution of the rat D4 dopamine receptor protein in the CNS using anti-receptor antisera. Brain Res (1997) 752(1-2):26–34. doi: 10.1016/s0006-8993(96)01422-9
16. Defagot MC, Malchiodi EL, Villar MJ, Antonelli MC. Distribution of D4 dopamine receptor in rat brain with sequence-specific antibodies. Brain Res Mol Brain Res (1997) 45(1):1–12. doi: 10.1016/s0169-328x(96)00235-5
17. Khan ZU, Gutiérrez A, Martín R, Peñafiel A, Rivera A, de la Calle A. Differential regional and cellular distribution of dopamine D2-like receptors: an immunocytochemical study of subtype-specific antibodies in rat and human brain. J Comp Neurol (1998) 402(3):353–71. doi: 10.1002/(sici)1096-9861(19981221)402:3<353::aid-cne5>3.0.co;2-4
18. Mauger C, Sivan B, Brockhaus M, Fuchs S, Civelli O, Monsma F Jr.Development and characterization of antibodies directed against the mouse D4 dopamine receptor. Eur J Neurosci (1998) 10(2):529–37. doi: 10.1046/j.1460-9568.1998.00056.x
19. Rivera A, Cuéllar B, Girón FJ, Grandy DK, de la Calle A, Moratalla R. Dopamine D4 receptors are heterogeneously distributed in the striosomes/matrix compartments of the striatum. J Neurochem (2002) 80(2):219–29. doi: 10.1046/j.0022-3042.2001.00702.x
20. Noaín D, Avale ME, Wedemeyer C, Calvo D, Peper M, Rubinstein M. Identification of brain neurons expressing the dopamine D4 receptor gene using BAC transgenic mice. Eur J Neurosci (2006) 24(9):2429–38. doi: 10.1111/j.1460-9568.2006.05148.x
21. Murray AM, Hyde TM, Knable MB, Herman MM, Bigelow LB, Carter JM, et al. Distribution of putative D4 dopamine receptors in postmortem striatum from patients with schizophrenia. J Neurosci (1995) 15(3 Pt 2):2186–91. doi: 10.1523/JNEUROSCI.15-03-02186.1995
22. Primus RJ, Thurkauf A, Xu J, Yevich E, McInerney S, Shaw K, et al. Localization and characterization of dopamine D4 binding sites in rat and human brain by use of the novel, D4 receptor-selective ligand [3H]NGD 94-1. J Pharmacol Exp Ther (1997) 282(2):1020–7.
23. Tarazi FI, Kula NS, Baldessarini RJ. Regional distribution of dopamine D4 receptors in rat forebrain. Neuroreport (1997) 8(16):3423–6. doi: 10.1097/00001756-199711100-00001
24. Tarazi FI, Campbell A, Yeghiayan SK, Baldessarini RJ. Localization of dopamine receptor subtypes in corpus striatum and nucleus accumbens septi of rat brain: comparison of D1-, D2-, and D4-like receptors. Neuroscience (1998) 83(1):169–76. doi: 10.1016/s0306-4522(97)00386-2
25. de la Garza R 2nd, Madras BK. [(3)H]PNU-101958, a D(4) dopamine receptor probe, accumulates in prefrontal cortex and hippocampus of non-human primate brain. Synapse (2000) 37(3):232–44. doi: 10.1002/1098-2396(20000901)37:3<232::AID-SYN7>3.0.CO;2-7
26. Lim L, Mi D, Llorca A, Marín O. Development and functional diversification of cortical interneurons. Neuron (2018) 100(2):294–313. doi: 10.1016/j.neuron.2018.10.009
27. Wang XJ, Yang GR. A disinhibitory circuit motif and flexible information routing in the brain. Curr Opin Neurobiol (2018) 49:75–83. doi: 10.1016/j.conb.2018.01.002
28. Wang XJ, Tegnér J, Constantinidis C, Goldman-Rakic PS. Division of labor among distinct subtypes of inhibitory neurons in a cortical microcircuit of working memory. Proc Natl Acad Sci USA (2004) 101(5):1368–73. doi: 10.1073/pnas.0305337101
29. Zhong P, Yan Z. Distinct physiological effects of dopamine D4 receptors on prefrontal cortical pyramidal neurons and fast-spiking interneurons. Cereb Cortex (2016) 26(1):180–91. doi: 10.1093/cercor/bhu190
30. Rubinstein M, Cepeda C, Hurst RS, Flores-Hernandez J, Ariano MA, Falzone TL, et al. Dopamine D4 receptor-deficient mice display cortical hyperexcitability. J Neurosci (2001) 21(11):3756–63. doi: 10.1523/JNEUROSCI.21-11-03756.2001
31. Klitten LL, Rath MF, Coon SL, Kim JS, Klein DC, Møller M. Localization and regulation of dopamine receptor D4 expression in the adult and developing rat retina. Exp Eye Res (2008) 87(5):471–7. doi: 10.1016/j.exer.2008.08.004
32. Kim JS, Bailey MJ, Weller JL, Sugden D, Rath MF, Møller M, et al. Thyroid hormone and adrenergic signaling interact to control pineal expression of the dopamine receptor D4 gene (Drd4). Mol Cell Endocrinol (2010) 314(1):128–35. doi: 10.1016/j.mce.2009.05.013
33. Ganguly S, Coon SL, Klein DC. Control of melatonin synthesis in the mammalian pineal gland: The critical role of serotonin acetylation. Cell Tissue Res (2002) 309(1):127–37. doi: 10.1007/s00441-002-0579-y
34. Maronde E, Stehle JH. The mammalian pineal gland: known facts, unknown facets. Trends Endocrinol Metab (2007) 18(4):142–9. doi: 10.1016/j.tem.2007.03.001
35. Sánchez-Soto M, Bonifazi A, Cai NS, Ellenberger MP, Newman AH, Ferré S, et al. Evidence for noncanonical neurotransmitter activation: Norepinephrine as a dopamine D2-like receptor agonist. Mol Pharmacol (2016) 89(4):457–66. doi: 10.1124/mol.115.101808
36. Descarries L, Lemay B, Doucet G, Berger B. Regional and laminar density of the dopamine innervation in adult rat cerebral cortex. Neuroscience (1987) 21(3):807–24. doi: 10.1016/0306-4522(87)90038-8
37. Séguéla P, Watkins KC, Geffard M, Descarries L. Noradrenaline axon terminals in adult rat neocortex: An immunocytochemical analysis in serial thin sections. Neuroscience (1990) 35(2):249–64. doi: 10.1016/0306-4522(90)90079-j
38. Lidow MS, Goldman-Rakic PS, Gallager DW, Rakic P. Distribution of dopaminergic receptors in the primate cerebral cortex: Quantitative autoradiographic analysis using [3H]raclopride, [3H]spiperone and [3H]SCH23390. Neuroscience (1991) 40(3):657–71. doi: 10.1016/0306-4522(91)90003-7
39. Richfield EK, Young AB, Penney JB. Comparative distributions of dopamine d-1 and d-2 receptors in the cerebral cortex of rats, cats, and monkeys. J Comp Neurol (1989) 286(4):409–26. doi: 10.1002/cne.902860402
40. Goldsmith SK, Joyce JN. Dopamine D2 receptor expression in hippocampus and parahippocampal cortex of rat, cat, and human in relation to tyrosine hydroxylase-immunoreactive fibers. Hippocampus (1994) 4(3):354–73. doi: 10.1002/hipo.450040318
41. Wedzony K, Chocyk A, Maćkowiak M, Fijał K, Czyrak A. Cortical localization of dopamine D4 receptors in the rat brain–immunocytochemical study. J Physiol Pharmacol (2000) 51(2):205–21.
42. Devoto P, Flore G. On the origin of cortical dopamine: is it a co-transmitter in noradrenergic neurons? Curr Neuropharmacol (2006) 4(2):115–25. doi: 10.2174/157015906776359559
43. Agnati LF, Guidolin D, Guescini M, Genedani S, Fuxe K. Understanding wiring and volume transmission. Brain Res Rev (2010) 64(1):137–59. doi: 10.1016/j.brainresrev.2010.03.003
44. Lanau F, Zenner MT, Civelli O, Hartman DS. Epinephrine and norepinephrine act as potent agonists at the recombinant human dopamine D4 receptor. J Neurochem (1997) 68(2):804–12. doi: 10.1046/j.1471-4159.1997.68020804.x
45. Newman-Tancredi A, Audinot-Bouchez V, Gobert A, Millan MJ. Noradrenaline and adrenaline are high affinity agonists at dopamine D4 receptors. Eur J Pharmacol (1997) 319(2-3):379–83. doi: 10.1016/s0014-2999(96)00985-5
46. Cummings DF, Ericksen SS, Goetz A, Schetz JA. Transmembrane segment five serines of the D4 dopamine receptor uniquely influence the interactions of dopamine, norepinephrine, and Ro10-4548. J Pharmacol Exp Ther (2010) 333(3):682–95. doi: 10.1124/jpet.109.164962
47. Schaad NC, Klein DC. Characterization of alpha 2-adrenergic receptors on rat pinealocytes. Endocrinology (1992) 130(5):2804–10. doi: 10.1210/endo.130.5.1315259
48. Roseboom PH, Klein DC. Norepinephrine stimulation of pineal cyclic AMP response element-binding protein phosphorylation: Primary role of a beta-adrenergic receptor/cyclic AMP mechanism. Mol Pharmacol (1995) 47(3):439–49.
49. Qin L, Liu W, Ma K, Wei J, Zhong P, Cho K, et al. The ADHD-linked human dopamine D4 receptor variant D4.7 induces over-suppression of NMDA receptor function in prefrontal cortex. Neurobiol Dis (2016) 95:194–203. doi: 10.1016/j.nbd.2016.07.024
50. Zhong P, Liu W, Yan Z. Aberrant regulation of synchronous network activity by the attention-deficit/hyperactivity disorder-associated human dopamine D4 receptor variant D4.7 in the prefrontal cortex. J Physiol (2016) 594(1):135–47. doi: 10.1113/JP271317
51. Asghari V, Sanyal S, Buchwaldt S, Paterson A, Jovanovic V, Van Tol HH. Modulation of intracellular cyclic AMP levels by different human dopamine D4 receptor variants. J Neurochem (1995) 65(3):1157–65. doi: 10.1046/j.1471-4159.1995.65031157.x
52. González S, Rangel-Barajas C, Peper M, Lorenzo R, Moreno E, Ciruela F, et al. Dopamine D4 receptor, but not the ADHD-associated D4.7 variant, forms functional heteromers with the dopamine D2S receptor in the brain. Mol Psychiatry (2012) 17(6):650–62. doi: 10.1038/mp.2011.93
53. Sánchez-Soto M, Yano H, Cai NS, Casadó-Anguera V, Moreno E, Casadó V, et al. Revisiting the functional role of dopamine D4 receptor gene polymorphisms: Heteromerization-dependent gain of function of the D4.7 receptor variant. Mol Neurobiol (2019) 56(7):4778–85. doi: 10.1007/s12035-018-1413-1
54. Casadó-Anguera V, Moreno E, Sánchez-Soto M, Cai NS, Bonaventura J, Homar-Ruano P, et al. Heteromerization between α2A adrenoceptors and different polymorphic variants of the dopamine D4 receptor determines pharmacological and functional differences. implications for impulsive-control disorders. Pharmacol Res (2021) 170:105745. doi: 10.1016/j.phrs.2021.105745
55. Yepes G, Guitart X, Rea W, Newman AH, Allen RP, Earley CJ, et al. Targeting hypersensitive corticostriatal terminals in restless legs syndrome. Ann Neurol (2017) 82(6):951–60. doi: 10.1002/ana.25104
56. Van Craenenbroeck K, Borroto-Escuela DO, Romero-Fernandez W, Skieterska K, Rondou P, Lintermans B, et al. Dopamine D4 receptor oligomerization–contribution to receptor biogenesis. FEBS J (2011) 278(8):1333–44. doi: 10.1111/j.1742-4658.2011.08052.x
57. Borroto-Escuela DO, Van Craenenbroeck K, Romero-Fernandez W, Guidolin D, Woods AS, Rivera A, et al. Dopamine D2 and D4 receptor heteromerization and its allosteric receptor-receptor interactions. Biochem Biophys Res Commun (2011) 404(4):928–34. doi: 10.1016/j.bbrc.2010.12.083
58. Urizar E, Yano H, Kolster R, Galés C, Lambert N, Javitch JA. CODA-RET reveals functional selectivity as a result of GPCR heteromerization. Nat Chem Biol (2011) 7(9):624–30. doi: 10.1038/nchembio.623
59. Guitart X, Navarro G, Moreno E, Yano H, Cai NS, Sánchez-Soto M, et al. Functional selectivity of allosteric interactions within G protein-coupled receptor oligomers: the dopamine D1-D3 receptor heterotetramer. Mol Pharmacol (2014) 86(4):417–29. doi: 10.1124/mol.114.093096
60. Tellegen A. Brief manual for the multidimensional personality questionnaire. Univ Minnesota Minneapolis (1982).
61. Costa Jr.PT, McCrae RR. The revised NEO personality inventory (NEO-PI-R). SAGE Publications Inc (2008).
62. Tellegen A. Structures of mood and personality and their relevance to assessing anxiety, with an emphasis on self-report. In: Tuma AH, Maser J, editors. Anxiety and the anxiety disorders, vol. 1085) Erlbaum, Hillsdale, NJ (1985). p. 681–706.
63. Church AT. Relating the Tellegen and five-factor models of personality structure. J Pers Soc Psychol (1994) 67(5):898–909. doi: 10.1037//0022-3514.67.5.898
64. Clark LA, Watson D. Temperament: An organizing paradigm for trait psychology. In: John OP, Robins RW, Pervin LA, editors. Handbook of personality: Theory and research. NY: The Guilford Press (2008). p. 265–86.
65. Roberts BW, Lejuez C, Krueger RF, Richards JM, Hill PL. What is conscientiousness and how can it be assessed? Dev Psychol (2014) 50(5):1315–30. doi: 10.1037/a0031109
66. Moeller FG, Barratt ES, Dougherty DM, Schmitz JM, Swann AC. Psychiatric aspects of impulsivity. Am J Psychiatry (2001) 158(11):1783–93.
67. Bari A, Robbins TW. Inhibition and impulsivity: behavioral and neural basis of response control. Prog Neurobiol (2013) 108:44–79. doi: 10.1016/j.pneurobio.2013.06.005
68. Hamilton KR, Littlefield AK, Anastasio NC, Cunningham KA, Fink LHL, Wing VC, et al. Rapid-response impulsivity: Definitions, measurement issues, and clinical implications. Pers Disord (2015) 6(2):168–81. doi: 10.1037/per0000100
69. Hamilton KR, Mitchell MR, Wing VC, Balodis IM, Bickel WK, Fillmore M, et al. Choice impulsivity: Definitions, measurement issues, and clinical implications. Pers Disord (2015) 6(2):182–98. doi: 10.1037/per0000099
70. McClure SM, Laibson DI, Loewenstein G, Cohen JD. Separate neural systems value immediate and delayed monetary rewards. Science (2004) 306(5695):503–7. doi: 10.1126/science
71. Green L, Myerson J. A discounting framework for choice with delayed and probabilistic rewards. Psychol Bull (2004) 130(5):769–92. doi: 10.1037/0033-2909.130.5.769
72. Peters J, Büchel C. The neural mechanisms of inter-temporal decision-making: understanding variability. Trends Cognit Sci (2011) 15(5):227–39. doi: 10.1016/j.tics.2011.03.002
73. Belcher AM, Lejuez CW, Moeller FG, Volkow ND, Ferré S. Choice impusivity. A drug-modifiable personality trait. In: Pickard H, Ahmed SH, editors. The routledge handbook of philosophy and science of addiction. NY: Routledge Taylor and Francis Group (2018). p. 286–98.
74. Gottesman II, Gould TD. The endophenotype concept in psychiatry: etymology and strategic intentions. Am J Psychiatry (2003) 160(4):636–45. doi: 10.1176/appi.ajp.160.4.636
75. Bearden CE, Freimer NB. Endophenotypes for psychiatric disorders: ready for primetime? Trends Genet (2006) 22(6):306–13. doi: 10.1016/j.tig.2006.04.004
76. Kotov R, Gamez W, Schmidt F, Watson D. Linking “big” personality traits to anxiety, depressive, and substance use disorders: A meta-analysis. Psychol Bull (2010) 136(5):768–821. doi: 10.1007/s10802-007-9131-6
77. Alderson RM, Rapport MD, Kofler MJ. Attention-deficit/hyperactivity disorder and behavioral inhibition: A meta-analytic review of the stop-signal paradigm. J Abnorm Child Psychol (2007) 35(5):745–58. doi: 10.1007/s10802-007-9131-6
78. Lipszyc J, Schachar R. Inhibitory control and psychopathology: a meta-analysis of studies using the stop signal task. J Int Neuropsychol Soc (2010) 16(6):1064–76. doi: 10.1017/S1355617710000895
79. Scheres A, Tontsch C, Thoeny AL. Steep temporal reward discounting in ADHD-combined type: acting upon feelings. Psychiatry Res (2013) 209(2):207–13. doi: 10.1016/j.psychres.2012.12.007
80. Patros CH, Alderson RM, Kasper LJ, Tarle SJ, Lea SE, Hudec KL. Choice-impulsivity in children and adolescents with attention-deficit/hyperactivity disorder (ADHD): A meta-analytic review. Clin Psychol Rev (2016) 43:162–74. doi: 10.1016/j.cpr.2015.11.001
81. Gomez R, Corr PJ. ADHD and personality: A meta-analytic review. Clin Psychol Rev (2014) 34(5):376–88. doi: 10.1016/j.cpr.2014.05.002
82. Lee SS, Humphreys KL, Flory K, Liu R, Glass K. Prospective association of childhood attention-deficit/hyperactivity disorder (ADHD) and substance use and abuse/dependence: a meta-analytic review. Clin Psychol Rev (2011) 31(3):328–41. doi: 10.1016/j.cpr.2011.01.006
83. Wilens TE, Martelon M, Joshi G, Bateman C, Fried R, Petty C, et al. Does ADHD predict substance-use disorders? a 10-year follow-up study of young adults with ADHD. J Am Acad Child Adolesc Psychiatry (2011) 50(6):543–53. doi: 10.1016/j.jaac.2011.01.021
84. van Emmerik-van Oortmerssen K, van de Glind G, van den Brink W, Smit F, Crunelle CL, Swets M, et al. Prevalence of attention-deficit hyperactivity disorder in substance use disorder patients: a meta-analysis and meta-regression analysis. Drug Alcohol Depend (2012) 122(1-2):11–9. doi: 10.1016/j.drugalcdep.2011.12.007
85. Roman T, Schmitz M, Polanczyk GV, Eizirik M, Rohde LA, Hutz MH. Is the alpha-2A adrenergic receptor gene (ADRA2A) associated with attention-deficit/hyperactivity disorder? Am J Med Genet B Neuropsychiatr Genet (2003) 120B(1):116–20. doi: 10.1002/ajmg.b.20018
86. Park L, Nigg JT, Waldman ID, Nummy KA, Huang-Pollock C, Rappley M, et al. Association and linkage of alpha-2A adrenergic receptor gene polymorphisms with childhood ADHD. Mol Psychiatry (2005) 10(6):572–80. doi: 10.1038/sj.mp.4001605
87. Gizer IR, Ficks C, Waldman ID. Candidate gene studies of ADHD: a meta-analytic review. Hum Genet (2009) 126(1):51–90. doi: 10.1007/s00439-009-0694-x
88. Cummins TD, Jacoby O, Hawi Z, Nandam LS, Byrne MA, Kim BN, et al. Alpha-2A adrenergic receptor gene variants are associated with increased intra-individual variability in response time. Mol Psychiatry (2014) 19(9):1031–6. doi: 10.1038/mp.2013.140
89. Cortese S. Pharmacologic treatment of attention deficit-hyperactivity disorder. N Engl J Med (2020) 383(11):1050–6. doi: 10.1056/NEJMra1917069
90. Kim S, Bobeica I, Gamo NJ, Arnsten AF, Lee D. Effects of α-2A adrenergic receptor agonist on time and risk preference in primates. Psychopharmacology (2012) 219(2):363–75. doi: 10.1007/s00213-011-2520-0
91. Regan SL, Williams MT, Vorhees CV. Review of rodent models of attention deficit hyperactivity disorder. Neurosci Biobehav Rev (2022) 132:621–37. doi: 10.1016/j.neubiorev.2021.11.041
92. Dickstein SG, Bannon K, Castellanos FX, Milham MP. The neural correlates of attention deficit hyperactivity disorder: an ALE meta-analysis. J Child Psychol Psychiatry (2006) 47(10):1051–62. doi: 10.1111/j.1469-7610.2006.01671.x
93. Fernández A, Quintero J, Hornero R, Zuluaga P, Navas M, Gómez C, et al. Complexity analysis of spontaneous brain activity in attention-deficit/hyperactivity disorder: Diagnostic implications. Biol Psychiatry (2009) 65(7):571–7. doi: 10.1016/j.biopsych.2008.10.046
94. Ferré S, Bonaventura J, Zhu W, Hatcher-Solis C, Taura J, Quiroz C, et al. Essential control of the function of the striatopallidal neuron by pre-coupled complexes of adenosine A2A-dopamine D2 receptor heterotetramers and adenylyl cyclase. Front Pharmacol (2018) 9:243. doi: 10.3389/fphar.2018.00243
95. Bromberg-Martin ES, Matsumoto M, Hikosaka O. Dopamine in motivational control: Rewarding, aversive, and alerting. Neuron (2010) 68(5):815–34. doi: 10.1016/j.neuron.2010.11.022
96. Zhang K, Tarazi FI, Baldessarini RJ. Role of dopamine D(4) receptors in motor hyperactivity induced by neonatal 6-hydroxydopamine lesions in rats. Neuropsychopharmacology (2001) 25:624–32. doi: 10.1016/S0893-133X(01)00262-7
97. Avale ME, Falzone TL, Gelman DM, Low MJ, Grandy DK, Rubinstein M. The dopamine D4 receptor is essential for hyperactivity and impaired behavioral inhibition in a mouse model of attention deficit/hyperactivity disorder. Mol Psychiatry (2004) 9(7):718–26. doi: 10.1038/sj.mp.4001474
98. Davids E, Zhang K, Kula NS, Tarazi FI, Baldessarini RJ. Effects of norepinephrine and serotonin transporter inhibitors on hyperactivity induced by neonatal 6-hydroxydopamine lesioning in rats. J Pharmacol Exp Ther (2002) 301(3):1097–102. doi: 10.1124/jpet.301.3.1097
99. Nicholas AP, Pieribone V, Hökfelt T. Distributions of mRNAs for alpha-2 adrenergic receptor subtypes in rat brain: an in situ hybridization study. J Comp Neurol (1993) 328(4):575–94. doi: 10.1002/cne.903280409
100. Arnsten AFT. Guanfacine's mechanism of action in treating prefrontal cortical disorders: Successful translation across species. Neurobiol Learn Mem (2020) 176:107327. doi: 10.1016/j.nlm.2020.107327
101. Wang M, Ramos BP, Paspalas CD, Shu Y, Simen A, Duque A, et al. Alpha2A-adrenoceptors strengthen working memory networks by inhibiting cAMP-HCN channel signaling in prefrontal cortex. Cell (2007) 129(2):397–410. doi: 10.1016/j.cell.2007.03.015
102. Ji XH, Ji JZ, Zhang H, Li BM. Stimulation of alpha2-adrenoceptors suppresses excitatory synaptic transmission in the medial prefrontal cortex of rat. Neuropsychopharmacology (2008) 33(9):2263–71. doi: 10.1038/sj.npp.1301603
103. Yi F, Liu SS, Luo F, Zhang XH, Li BM. Signaling mechanism underlying α2A -adrenergic suppression of excitatory synaptic transmission in the medial prefrontal cortex of rats. Eur J Neurosci (2013) 38(3):2364–73. doi: 10.1111/ejn.12257
104. Rivkin A, Alexander RC, Knighton J, Hutson PH, Wang XJ, Snavely DB, et al. A randomized, double-blind, crossover comparison of MK-0929 and placebo in the treatment of adults with ADHD. J Atten Disord (2012) 16(8):664–74. doi: 10.1177/1087054711423633
105. Ferré S, Guitart X, Quiroz C, Rea W, García-Malo C, Garcia-Borreguero D, et al. Akathisia and restless legs syndrome: Solving the dopaminergic paradox. Sleep Med Clin (202) 16(2):249–67. doi: 10.1016/j.jsmc.2021.02.012
106. Allen RP, Picchietti DL, Garcia-Borreguero D, Ondo WG, Walters AS, Winkelman JW, et al. Restless legs syndrome/Willis-ekbom disease diagnostic criteria: updated international restless legs syndrome study group (IRLSSG) consensus criteria–history, rationale, description, and significance. Sleep Med (2014) 15(8):860–73. doi: 10.1016/j.sleep.2014.03.025
107. Earley CJ, Connor J, Garcia-Borreguero D, Jenner P, Winkelman J, Zee PC, et al. Altered brain iron homeostasis and dopaminergic function in restless legs syndrome (Willis-ekbom disease). Sleep Med (2014) 15(11):1288–301. doi: 10.1016/j.sleep.2014.05.009
108. Ferré S, García-Borreguero D, Allen RP, Earley CJ. New insights into the neurobiology of restless legs syndrome. Neuroscientist (2019) 25(2):113–25. doi: 10.1177/1073858418791763
109. Earley CJ, Jones BC, Ferré S. Brain-iron deficiency models of restless legs syndrome. Exp Neurol (2022) 356:114158. doi: 10.1016/j.expneurol.2022.114158
110. Salminen AV, Clemens S, Garcia-Borreguero D, Ghorayeb I, Li Y, Manconi M, et al. Consensus guidelines on the construct validity of rodent models of restless legs syndrome. Dis Model Mech (2022) 15:dmm049615. doi: 10.1242/dmm.049615
111. Quiroz C, Gulyani S, Ruiqian W, Bonaventura J, Cutler R, Pearson V, et al. Adenosine receptors as markers of brain iron deficiency: Implications for restless legs syndrome. Neuropharmacology (2016) 111:160–8. doi: 10.1016/j.neuropharm.2016.09.002
112. Rodrigues MS, Ferreira SG, Quiroz C, Earley CJ, García-Borreguero D, Cunha RA, et al. Brain iron deficiency changes the stoichiometry of adenosine receptor subtypes in cortico-striatal terminals: Implications for restless legs syndrome. Molecules (2022) 27(5):1489. doi: 10.3390/molecules27051489
113. Ferré S, Quiroz C, Rea W, Guitart X, García-Borreguero D. Adenosine mechanisms and hypersensitive corticostriatal terminals in restless legs syndrome. rationale for the use of inhibitors of adenosine transport. Adv Pharmacol (2019) 84:3–19. doi: 10.1016/bs.apha.2018.12.005
114. Garcia-Borreguero D, Guitart X, Garcia Malo C, Cano-Pumarega I, Granizo JJ, Ferré S. Treatment of restless legs syndrome/Willis-ekbom disease with the non-selective ENT1/ENT2 inhibitor dipyridamole: testing the adenosine hypothesis. Sleep Med (2018) 45:94–7. doi: 10.1016/j.sleep.2018.02.002
115. Garcia-Borreguero D, Garcia-Malo C, Granizo JJ, Randomized FerréSA. Placebo-controlled crossover study with dipyridamole for restless legs syndrome. Mov Disord (2021) 36(10):2387–92. doi: 10.1002/mds.28668
116. Dooley DJ, Taylor CP, Donevan S, Feltner D. Ca2+ channel alpha2delta ligands: novel modulators of neurotransmission. Trends Pharmacol Sci (2007) 28(2):75–82. doi: 10.1016/j.tips.2006.12.006
117. Manconi M, Ferri R, Zucconi M, Clemens S, Giarolli L, Bottasini V, et al. Preferential D2 or preferential D3 dopamine agonists in restless legs syndrome. Neurology (2011) 77(2):110–7. doi: 10.1212/WNL.0b013e3182242d91
118. García-Borreguero D, Williams AM. Dopaminergic augmentation of restless legs syndrome: the scope of the problem. Sleep Med (2011) 12(5):425–6. doi: 10.1016/j.sleep.2011.03.004
119. Lanza K, Meadows SM, Chambers NE, Nuss E, Deak MM, Ferré S, et al. Behavioral and cellular dopamine D1 and D3 receptor-mediated synergy: Implications for l-DOPA-induced dyskinesia. Neuropharmacology (2018) 138:304–14. doi: 10.1016/j.neuropharm.2018.06.024
120. Erlij D, Acosta-García J, Rojas-Márquez M, González-Hernández B, Escartín-Perez E, Aceves J, et al. Dopamine D4 receptor stimulation in GABAergic projections of the globus pallidus to the reticular thalamic nucleus and the substantia nigra reticulata of the rat decreases locomotor activity. Neuropharmacology (2012) 62(2):1111–8. doi: 10.1016/j.neuropharm.2011.11.001
121. Root DH, Hoffman AF, Good CH, Zhang S, Gigante E, Lupica CR, et al. Norepinephrine activates dopamine D4 receptors in the rat lateral habenula. J Neurosci (2015) 35(8):3460–9. doi: 10.1523/JNEUROSCI.4525-13.2015
Keywords: dopamine D4 receptor, polymorphic variants, impulsivity, attention-deficit hyperactivity disorder, restless legs syndrome
Citation: Ferré S, Belcher AM, Bonaventura J, Quiroz C, Sánchez-Soto M, Casadó-Anguera V, Cai N-S, Moreno E, Boateng CA, Keck TM, Florán B, Earley CJ, Ciruela F, Casadó V, Rubinstein M and Volkow ND (2022) Functional and pharmacological role of the dopamine D4 receptor and its polymorphic variants. Front. Endocrinol. 13:1014678. doi: 10.3389/fendo.2022.1014678
Received: 08 August 2022; Accepted: 14 September 2022;
Published: 30 September 2022.
Edited by:
Diego Guidolin, University of Padua, ItalyReviewed by:
Patrick J. Ronan, United States Department of Veterans Affairs, United StatesGuido Maura, University of Genoa, Italy
Copyright © 2022 Ferré, Belcher, Bonaventura, Quiroz, Sánchez-Soto, Casadó-Anguera, Cai, Moreno, Boateng, Keck, Florán, Earley, Ciruela, Casadó, Rubinstein and Volkow. This is an open-access article distributed under the terms of the Creative Commons Attribution License (CC BY). The use, distribution or reproduction in other forums is permitted, provided the original author(s) and the copyright owner(s) are credited and that the original publication in this journal is cited, in accordance with accepted academic practice. No use, distribution or reproduction is permitted which does not comply with these terms.
*Correspondence: Sergi Ferré, sferre@intra.nida.nih.gov