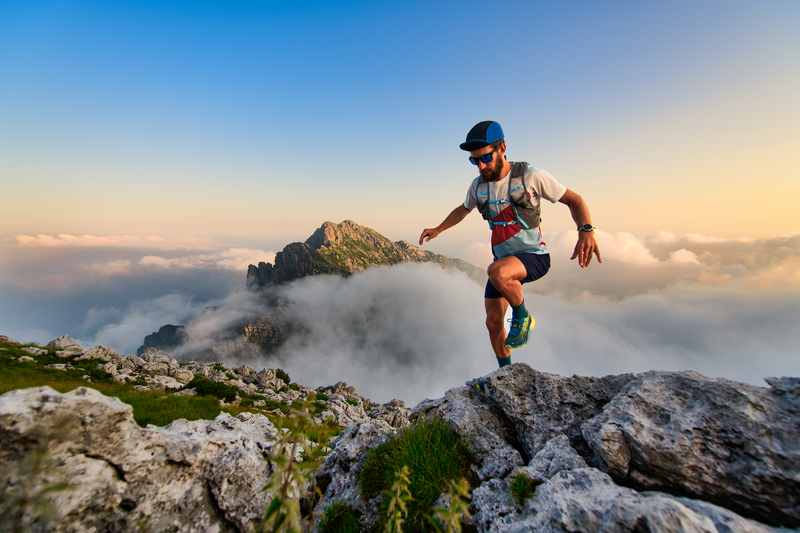
95% of researchers rate our articles as excellent or good
Learn more about the work of our research integrity team to safeguard the quality of each article we publish.
Find out more
MINI REVIEW article
Front. Endocrinol. , 23 December 2021
Sec. Cancer Endocrinology
Volume 12 - 2021 | https://doi.org/10.3389/fendo.2021.808526
This article is part of the Research Topic Double-Edged Swords: Important Factors Connecting Metabolic Disorders and Cancer Development - From Basic Research to Translational Applications View all 11 articles
Hepatocellular carcinoma (HCC) is the most common type of primary liver cancer, which will affect more than a million people by the year 2025. However, current treatment options have limited benefits. Nonalcoholic fatty liver disease (NAFLD) is the fastest growing factor that causes HCC in western countries, including the United States. In addition, NAFLD co-morbidities including obesity, type 2 diabetes mellitus (T2DM), and cardiovascular diseases (CVDs) promote HCC development. Alteration of metabolites and inflammation in the tumor microenvironment plays a pivotal role in HCC progression. However, the underlying molecular mechanisms are still not totally clear. Herein, in this review, we explored the latest molecules that are involved in obesity, T2DM, and CVDs-mediated progression of HCC, as they share some common pathologic features. Meanwhile, several therapeutic options by targeting these key factors and molecules were discussed for HCC treatment. Overall, obesity, T2DM, and CVDs as chronic metabolic disease factors are tightly implicated in the development of HCC and its progression. Molecules and factors involved in these NAFLD comorbidities are potential therapeutic targets for HCC treatment.
Hepatocellular carcinoma is the most common type of primary liver cancer, with a global case number larger than one million by 2025 (1). Factors including hepatitis viral infection, nonalcoholic fatty liver disease (NAFLD), alcohol abuse, and dietary toxins (e.g., aflatoxins) can cause the initiation and development of HCC (2). Both genetic and epigenetic factors can promote HCC progression (3), such as mutation of programmed cell death-1 (PDCD1, rs10204525 C > T mutation) and DNA methylation.
NAFLD is the fastest growing factor that causes HCC in western countries, including the United States (4). Many metabolic disorders, including obesity, type 2 diabetes mellitus (T2DM), and cardiovascular diseases (CVDs), are comorbidities of NAFLD or its advanced stage nonalcoholic steatohepatitis (NASH) (5). Gut microbiota and their-associated factors such as metabolites and components play important roles in the pathogenesis of obesity, T2DM, CVDs, NAFLD, and HCC (6–9). For example, feeding a high fat/high cholesterol diet (HFHC) can lead to fatty liver, NASH, fibrosis, and subsequential HCC in mice (10). Gut microbiota such as genera Mucispirillum and Desulfovibrio were increased, while genera Bifidobacterium and Bacteroides were dramatically decreased in HFHC-fed mice. In addition, gut microbial metabolites taurocholic acid and 3-indolepropionic acid were increased and decreased, separately, during NAFLD-HCC development (10). Overgrowth of nonvirulent lipopolysaccharide (LPS)-producing bacterial strains, such as Enterobacter cloacae, Escherichia coli, and Klebsiella pneumoniae from obese patients with severe fatty liver can induce NAFLD in germ-free mice fed a high-fat diet (HFD), but not HFD alone (11).
Chronic intestinal inflammation and malfunction of gut barrier associated with change of gut microbiota impact enteric hormones, adiposity, insulin resistance, and metabolic functions of intestine and other organs, such as via G protein-coupled receptors (12, 13). Accumulating evidence shows that GPCR signaling pathway play critically important roles in obesity, T2DM, and CVDs (14, 15). For example, phenylacetylglutamine, a gut-microbiota derived metabolite, can modulate cellular functions during CVD via GPCRs, such as α2A, α2B, and β2-adrenergic receptors (16). In addition to gut microbiota, several other factors such as chronic inflammation, insulin resistance, alteration of metabolites have been reported to be associated with obesity, T2DM, CVDs, as well as NAFLD, which can induce HCC initiation and progression. However, in specific situation, the risk factor may have different effect on these comorbidities. For example, genetic risk alleles (e.g., 148Met in the patatin-like phospholipase domain-containing protein 3, PNPLA3) in NAFLD are associated with protecting function in CVDs (17).
Herein, in this review, we summarize the relationships of obesity, T2DM, and CVDs with HCC, and uncover some signaling pathways and treatment options. The causing factors and treatment options of NAFLD-related HCC have been reported in another paper recently (3).
Obesity is a causing factor for many cancers (18), including HCC with a moderate magnitude in obesity-associated cancers. For example, dysbiosis of gut microbiota is shown to be associated with obesity, resulting in an increase of lipoteichoic acid (LTA) (19). LTA, a Gram-positive bacterial membrane component, can promote HCC development by enhancing senescent hepatic stellate cells (HSCs) (19). In addition, LTA function together with deoxycholic acid (DCA), a secondary bile acid produced by gut microbiota, to upregulate the expression of SASP factors and cyclooxygenase-2 (COX2) through Toll-like receptor 2 (TLR2), resulting in COX2-mediated prostaglandin E2 (PGE2) production to inhibit antitumor immunity (19, 20). Furthermore, obesity can modulate intestinal hormone secretion to impact liver function. For example, a high level of glucose-dependent insulinotropic polypeptide (GIP), an intestinal enteroendocrine K cell-secreted hormone, contributes to hepatic steatosis and liver injury by modulating the expression of microRNAs (miRNAs) (21). Overall, obesity can promote HCC progression through modulating metabolites, inflammation, immunity, and autophagy in the tumor microenvironment, as discussed below.
Obesity-mediated metabolic change in the tumor microenvironment can suppress anti-tumor immunity (22). In addition, obesity induces the alteration of gut microbiota, which impacts anti-HCC immune therapies (23). For example, obesity induced by a HFD compromised the effect of cytotoxic CD8+ T cells in the tumor microenvironment by reprogramming fat intake in tumor cells via reducing prolyl hydroxylase-3 (PHD3) expression (7). Obesity can modulate glucose metabolism to promote HCC progression. Saturated fatty acids such as palmitate impact cancer stem cell properties, production of reactive oxidative species, and glucose metabolism to enhance HCC initiation and progression (24, 25). In addition, feeding a HFD promoted the production of lactate when the mice received glucose (25). T-regulatory cells (Tregs) can proliferate in lactate-rich environments, which mediates suppression effector T cell function (26). In addition, feeding a HFD in carcinogen diethylnitrosamine (DEN)-injected promoted the development of HCC compared to mice fed a control diet, with increased secretion of lactate (25).
Low-grade chronic inflammation displays a key role in obesity and metabolic disorder (27). Inflammation impacts the activation of innate and adaptive immunity and modulates the progression of fibrosis and angiogenesis. For example, obesity-induced aberrant biosynthesis of glycosaminoglycan (GAG) which functions as one of the damage-associated molecular patterns (DAMPs) to promote hepatic inflammation and HCC via nuclear factor kappa B (NF-κB) signaling, in tumor suppressor gene exostosin-like 2-deficient mice (28). Adipose tissue caused by obesity can secrete diverse adipokines, such as leptin, adiponectin, and resistin, as well as proinflammatory cytokines, resulting in insulin resistance and chronic low-grade inflammation in different tissues, including liver tissue (29). For example, emerging evidence shows that leptin plays essential roles in cancer development by increasing tumor cell proliferation, metastasis, chemoresistance and promoting angiogenesis via binding its receptor to regulate many downstream signaling pathways (30, 31), as well as promoting NASH and liver fibrosis (32). Obesity impacts adipocytes to secrete proinflammatory cytokines such as interleukin (IL)-6 and tumor necrosis factor-α (TNF-α), and lipotoxicity induces hepatocyte death to activate Kupffer cells to produce those cytokines (33). Obesity-associated HCC development depended on the increased tumor-promoting cytokines IL-6 and TNF, inducing liver inflammation and activation of oncogenic signal transducer and activator of transcription 3 (STAT3) signaling in mice (34). The mRNA expression of Toll-like receptor 4 (TLR4) was positively correlated with IL-6 and IL-10 mRNA expression in obese HCC patients. Treatment with resatorvid, a TLR4 inhibitor, inhibited HCC growth in mice with deletion of phosphatase and tensin homolog (PTEN) in hepatocytes (35).
Plasma S100 calcium-binding protein A4 (S100A4) levels were positively associated with insulin resistance in prepubertal non-diabetic obese children, which has been shown to be associated with inflammation (36). Plasma levels of FGF-21 are higher in obese adolescents than lean controls, especially in those with fatty liver (37). Feeding a high fat, high sucrose (HFHS) diet, fibroblast growth factor 21 (FGF21) deficient mice developed advanced steatosis and liver fibrosis, with liver inflammation compared to wild-type mice (38).
Obesity modulates intrahepatic immunity to induce an immunotolerant microenvironment, which results in HCC progression. For example, obese mice had a higher frequency of PD-1+ T cells in the liver compared to control mice (39). In addition, the expression of Ki67 in hepatic T cells in obese mice was reduced compared to that in control mice, post-ex vivo stimulation with anti-mouse CD3 antibody, as well as reduced interferon (IFN)-γ and TNF-α production, indicating functional exhaustion. The frequency of PD-1+ T cells and Ki67+ T cells in the peripheral blood of obese (BMI ≥ 30) volunteers were increased and decreased, respectively, compared to that in non-obese (BMI < 30) human healthy controls following ex vivo stimulation (39). Similarly, a recent study showed that PD1+CD8+ T cells were increased in the livers of mice with NASH, promoting NASH-HCC progression (40). In addition, Ma et al. reported that obesity caused hepatic lipid accumulation and loss of CD4+ T cells, which plays a critical role in NAFLD-HCC progression (41). A recent study from this group also showed that loss of liver CD4+ T cells impaired immunotherapies such as RNA vaccine (M30) and anti-OX40 antibody-mediated treatment against tumor cell growth in the liver (42). Furthermore, there are several other subtypes of T cells play important roles in the NALFD or NAFLD-HCC pathogenesis (43).
Autophagy-related protein 4b (Apg4b)-deficient mice, with limited autophagy function, showed a bodyweight gain compared to wild-type mice when the mice were challenged with a high-fat diet consisting of 42% fat or with a standard rodent diet with 30% sucrose supplementation in drinking water (44). Apg4b-deficient mice also displayed more accumulation of visceral and hepatic fat, low glucose tolerance, and reduced insulin responses. By modulating autophagy, lipid metabolism, endoplasmic reticulum (ER) stress, and mitochondrial dysfunction can be regulated to ameliorate obesity-associated pathogenesis (45).
Excessive production of reactive oxygen species (ROS) plays a pivotal role in the pathogenesis of obesity, which promotes obesity-related metabolic disorders, including diabetes, NAFLD, and HCC (45). Autophagy can be turned off to rescue ROS-induced cell damage (46). Furthermore, autophagy-related genes were also shown to be aberrantly expressed in cholangiocarcinoma (47), the secondary most liver cancer. Treatment with hydroxychloroquine can induce cell apoptosis and inhibit cholangiocarcinoma cell proliferation by increasing ROS accumulation through inhibiting autophagy (47).
T2DM has been reported to be an independent factor that is associated with increased risk for HCC for both men and women in the U.S (48)., as well as Asian countries. For example, in Japan, the concurrence of HCC with T2DM and obesity is reported in hepatitis B virus surface antigen-negative or hepatitis C virus antibody-negative patients (49). Another report also showed that HCC-caused mortality was higher than other cancer-associated death in T2DM patients in Japan (50). Some differentially methylated genes (DMGs) were co-expressed in HCC and T2DM, such as ST3 beta-galactoside alpha-2,3-sialyltransferase 2 (ST3GAL2) and glycerophosphodiester phosphodiesterase domain containing 2 (GDPD2). And also, these DMGs were implicated in the signaling pathways including biosynthesis of glycosaminoglycan and unsaturated fatty acids (51). In addition, patients with NAFLD showed a higher risk of incident T2DM than those without NAFLD, even more in those with advanced high NAFLD fibrosis scores (52). Even after transarterial chemoembolization (TACE), a shorter interval time of progression and higher risk of cancer-specific mortality were found in HCC patients with T2DM who underwent TACE than patients without T2DM, especially in patients with cirrhosis (53).
A recent study showed that the prevalence of NAFLD in T2DM patients was 100%, while the prevalence of NASH was 96.82% (54). This study also showed that the HOMA-IR score (homeostatic model assessment for insulin resistance) was significantly higher in NASH patients than that in NALFD patients, which may cause a higher co-incidence of NASH with T2DM. Factors in T2DM pathogenesis impact HCC initiation and progression, including insulin/insulin-like growth factor (IGF) related factors (55, 56), proinflammatory cytokines (57), oxidative stress (58), gut microbiota dysbiosis (8, 59), angiogenesis (60), cell apoptosis (61), autophagy (62), which are summarized in Table 1.
Cardiovascular diseases (CVDs) are a group of disorders of the heart and blood vessels. CVDs and their outcomes include myocardial infarction, angina, transient ischemic attack, stroke, claudication, and heart failure (79). Chronic liver disease can increase the development of CVD events. For example, a meta-analysis of studies on the effects of NAFLD and the risk of CVD showed that patients with NAFLD have a significantly increased risk of fatal and non-fatal CVD incidences than those without NAFLD (80). Hepatokines such as α2-HS-glycoprotein secreted in the liver during NAFLD contribute to both CVDs and T2DM as distinct pathogenic factors from skeletal muscle and adipose tissue (81). Not only liver diseases can impact the progression of CVDs, but cardiovascular complications can in turn affect hepatic function and disease progression (66). There are many co-factors of CVDs and HCC, such as inflammation (63–65), oxidative stress (66, 67), gut microbiota and their relative metabolites (68–70), metabolic dysfunction (71–73), cell apoptosis (74–76), viral infections (77), and autophagy (78) (Table 1). Due to their correlation, medicines for the treatment of heart disease could be applied to treat liver disease. For example, statins, β-Hydroxy β-methylglutaryl-CoA (HMG-CoA) reductase inhibitors with function to reduce the risk of CVD morbidity and mortality, show a beneficial effect on liver disease, including NASH and HCC (82, 83).
Obesity, T2DM, and CVDs share some signaling pathways to promote NAFLD-related HCC progression. Thus, some examples of canonical signaling pathways and new findings will be discussed in the following context.
Wnt/β-catenin signaling is implicated in adipose tissue lipogenesis (84), activation of hepatic stellate cells (HSCs) or liver fibrosis (85), and ischemic myocardium (86). In primary liver cancers, including HCC, Wnt/β-catenin signaling is often activated to induce cancer cell growth and metastasis (87).
In obesity-associated HCC, liver inflammation and ER stress are associated with higher expression of inositol-requiring enzyme 1α (IRE1α). IRE1α, the unfolded protein response (UPR) signal transducer in ER, can activate nuclear factor kappa B kinase subunit beta (IKK-β)/NF-κB signaling pathway to promote TNF and IL-6 expression, resulting in HCC progression (88).
The expression of miR-34a was upregulated in fatty liver and palmitate acid (PA)-treated BNL CL.2 cells, which can induce hepatocyte senescence via downregulating cyclin-dependent kinase 6 (CDK6) expression (89). Hydrodynamic injection of miR-15a/16-1 (containing the miR-15a and miR-16-1) can prevent HCC in both protein kinase B (AKT)/Ras and c-Myc mice with overexpression of activated forms of AKT and NRas oncogenes (AKT/Ras) or c-Myc, via suppressing Tregs function to increase the effect of cytotoxic T cells (90). As the most abundant miRNAs in the liver, miR-122 has been reported to be significantly suppressed in HCC cell lines and tumor tissues. Overexpression of miR-122 can increase HCC cell radiosensitivity and sensitivity to chemotherapy medicines (91).
Proteomics analysis showed that hypoxia-inducible factor-1 alpha (HIF-1α), a transcription factor, is a binding protein of fatty acid-binding protein 5 (FABP5) (92). In addition, fatty acid (e.g., oleic acid) can activate FABP5/HIF-1α signaling to modulate lipid metabolism reprogramming to promote HCC progression, as well as the proliferation of HCC cells.
Signal transducer and activator of transcription 3 (STAT3) signaling pathway is involved in apoptosis, migration, and epithelial-mesenchymal transition (EMT) of HCC cells (93, 94). Surgical procedure-induced overexpression of IL-11 promoted tumor cell growth and recurrence of HCC via activating STAT3 signaling, while blocking IL-11/STAT3 signaling dampened HCC recurrence after surgical resection (95).
Aberrant activation and inhibition of phosphatidylinositol-4,5-bisphosphate 3-kinase (PI3K)/AKT signaling pathway are associated with HCC cell proliferation (96) and apoptosis (97), respectively. One study showed that protein arginine methyltransferase 9 can regulate EMT to increase HCC cell migration and invasion by activating Snail expression through PI3K/AKT signaling pathway (98).
Peroxisome proliferator-activated receptors (PPARs), including PPAR-α (99), PPAR-β/δ (100), and PPAR-γ (101), are involved in HCC growth and metastasis. PPARs can be regulated by microRNAs (miRNAs) to regulate tumor cell proliferation, migration, and invasion. For example, miR-1468 can promote HCC progression by activating PPAR-γ/AKT signaling pathway (102).
Vascular endothelial growth factor (VEGF) or its receptor (VEGFR) plays an important role in angiogenesis in HCC. A cohort study in Turkish showed that HCC patients with low levels of serum VEGF-A (<100 pg/mL) had a higher overall survival (OS) rate compared to patients with high levels of serum VEGF-A (≥100 pg/mL), indicating as an independent predictor for OS in HCC patients (103). Treatment of bioactive compound VS 8 can induce human HCC cell line HepG2 cell apoptosis and inhibit the expression of EMT-induced transcription factors in CD44+CD133+ cancer stem cells, by inhibiting VEGF/VEGFR-2 signaling pathway (104).
Prevention and treatment options for HCC include lifestyle change, dietary supplement, modulation of gut microbiota, anti-inflammation and anti-oxidative stress medicines, anti-obesity and anti-diabetic treatments, anti-angiogenesis, as well as natural products-mediated therapies, which are summarized in Figure 1.
Unhealthy lifestyles, such as over-nutrition, smoking, drinking, and lack of exercise, are risk factors causing cancer development and progression (105). One study showed that consumption of caffeinated coffee with an extra two cups daily was positively associated with reduction in the risk of HCC, which was also shown with consumption of decaffeinated coffee to some extent (106). Another study showed that intermediate-salt (6-10 g/day) or high-salt (>10 g/day) intake displayed a higher risk to develop primary liver cancer compared to low-salt intake (<6 g/day), after adjusting other potential cofactors such as fatty liver, hypertension, and diabetes (107). Lifestyle intervention can effectively result in low body fat mass, reduction of visceral adiposity, and a decrease of metabolic diseases, including NAFLD, CVD, and T2DM (108, 109); therefore, change of lifestyle plays an essential role in preventing HCC development.
Environmental or dietary exposure to aflatoxin B1, a genotoxic hepatocarcinogen, can also drive a high risk of HCC (110, 111). Aflatoxin contamination in food has been reported in food products, such as groundnuts, maize, wheat, and cocoa, which is associated with fungal growth (112). Therefore, consumption with fresh and non-contaminated food is also critically important to reduce potential risk of HCC.
Supplement of eicosapentaenoic acid (EPA), an omega-3 polyunsaturated fatty acid, reduced the development of obesity-related HCC in mice via suppressing the expression of STAT3 to inhibit tumor growth (113). Another study also omega-3 supplementation can decrease hepatic de novo lipogenesis while increasing fatty acid oxidation (114). Both in vitro and in vivo studies show that Se and selenoproteins exert immunomodulatory function against HCC by modulating oxidative stress, inflammation, angiogenesis, cell proliferation, and apoptosis (115).
Strategies via modulating gut microbiota are able to change anti-cancer immune response and inhibit factors causing HCC development, including bariatric surgery (BS) and fecal microbiota transplantation (FMT). For example, BS can inhibit the onset of NASH and HCC in a large propensity-matched cohort study after 7.1 years of follow-up (116), which can ameliorate NASH features including steatosis, hepatocyte ballooning, and lobular inflammation (117). Another meta-analysis with a comprehensive literature review also showed that BS was associated with a decreased HCC risk (118). Another review paper explored the potential of FMT in the prevention of NAFLD/NASH and improving the anti-cancer immune response (119).
We and other researchers show that natural anti-oxidative and anti-inflammatory product astaxanthin can modulate intrahepatic and systemic inflammation and oxidative stress to inhibit NASH and liver fibrosis (120). Molecular mechanism study showed that metformin treatment inhibited the expression of IL-12-mediated proliferation, migration, and invasion of HCC cells and attenuated ectopic IL-22 expression-caused HCC progression by activating the Hippo signaling pathway (121). In addition, it has been shown that astaxanthin inhibits the alcoholic fatty liver disease (AFLD) via modulating gut microbiota, resulting in a decrease of phyla Bacteroidetes and Proteobacteria and genera Butyricimonas, Bilophila, and Parabacteroides, while inducing an increase of phylum Verrucomicrobia and genus Akkermansia compared to control group (122).
Aspirin, a nonsteroidal anti-inflammatory drug, can reduce pain, fever, and reduce the risk of a heart attack. A prospective study showed that daily aspirin use inhibited the progression of NASH and advanced fibrosis in NAFLD patients (123). In addition, Ricciotti et al. reported that as an adjuvant, aspirin has the ability to reduce the recurrence of HCC, which is associated with anti-inflammatory and antiplatelet functions (124).
A retrospective study showed that the incidence of HCC was significantly lower in T2DM patients with cirrhosis with metformin treatment (17.4% in total of 125 patients) compared to patients without metformin treatment (37.4% in total of 128 patients) (125). For HCC patients, metformin treatment extended the median survival time from 3.88 years to 6.9 years (125). Another meta-analysis study showed that metformin treatment can significantly prolong the OS of HCC patients with T2DM after curative therapy (126). Metformin treatment can reduce HCC risk, but the effective dose has racial disparity in HCC patients with T2DM but without chronic liver disease (127).
Several studies showed that the combination of anti-diabetic drug liraglutide with human umbilical cord mesenchymal stem cell (hUC-MSCs) can modulate glycolipid metabolism, insulin resistance, and liver injury in rats with T2DM via inhibiting pancreatic beta-cell apoptosis, TLR4/NF-κB signaling pathway, and oxidative stress (128, 129). Furthermore, cholesterol-lowering drugs such as statin show beneficial effects against HCC development (130) or recurrence (131).
Angiogenesis resulting from an imbalance of factors such as VEGF/VEGFR signaling can advance HCC progression. The approved anti-angiogenic drugs (AAD) such as sorafenib, regorafenib, and lenvatinib have been shown to have a therapeutic effect on HCC (5). However, proteinuria caused by AAD can impact their effect against HCC. Angiotensin-converting enzyme inhibitors have been applied to reduce AAD-related proteinuria (132), but these inhibitors show inhibiting effects to the efficacy of AADs. In addition, some studies showed that T2DM patients with HCC who received metformin are resistant to sorafenib treatment (133, 134), having poor progression-free survival (PFS) and OS.
Treatment with hirsutine, an indole alkaloid isolated from Uncaria rhynchophylla, can attenuate HFD-induced hepatic steatosis, peripheral hyperglycemia, cardiac hypertrophy, and insulin resistance, via activating PI3K/AKT pathway (135). Another study showed that freshly dried mulberry fruits can avoid hyperphagia and reduce body weight gain and visceral fat accumulation, ameliorating hypertrophy of arterial and cardiac walls, aortic collagen fiber, and hepatic lipid accumulation in HFD-fed mice (136).
Furthermore, eradication of viral infection is also helpful to reduce metabolic disorders. For example, hepatitis C virus (HCV) eradication treated with direct-acting antivirals can reduce the incidence of T2DM by improving insulin resistance and restoring glucose homeostasis altered during viral infection (137). In addition, HCV clearance was also independently associated with a decreased risk of cardiovascular events (138), as well as major cardiovascular events in prediabetic patients (139). Therefore, anti-HCV treatment is helpful for metabolic disease-associated progression of HCC.
NAFLD comorbidities obesity, T2DM, and CVDs are risk factors that contribute to HCC initiation and progression. The incidence of metabolic disease-associated HCC is increased in the past decade, due to the increase of NAFLD and its comorbidities. Pathogenic factors such as abnormal metabolites, inflammatory factors, and immune modulations are underlying mechanisms for metabolic dysfunction associated with HCC pathogenesis. Currently, many treatment options show promising effects in HCC. However, the benefit of treatments such as sorafenib is still limited. In addition, an inappropriate combination of treatments even may reduce the effect of monotherapy. More clinical trials are awaited to explore the potential treatments for metabolic disease-associated HCC. A better understanding of the underlying mechanism of how these metabolic dysfunctions promote HCC initiation and progression is helpful to provide precision medicine care personally.
Conceptualization and data collection: CZ, SL, and MY. Original draft preparation, review, and editing: CZ, SL, and MY. All authors contributed to the article and approved the submitted version.
The authors declare that the research was conducted in the absence of any commercial or financial relationships that could be construed as a potential conflict of interest.
All claims expressed in this article are solely those of the authors and do not necessarily represent those of their affiliated organizations, or those of the publisher, the editors and the reviewers. Any product that may be evaluated in this article, or claim that may be made by its manufacturer, is not guaranteed or endorsed by the publisher.
1. Llovet JM, Kelley RK, Villanueva A, Singal AG, Pikarsky E, Roayaie S, et al. Hepatocellular Carcinoma. Nat Rev Dis Primers (2021) 7(1):6. doi: 10.1038/s41572-020-00240-3
2. Yang JD, Hainaut P, Gores GJ, Amadou A, Plymoth A, Roberts LR. A Global View of Hepatocellular Carcinoma: Trends, Risk, Prevention and Management. Nat Rev Gastroenterol Hepatol (2019) 16(10):589–604. doi: 10.1038/s41575-019-0186-y
3. Zhang C, Yang M. The Emerging Factors and Treatment Options for NAFLD-Related Hepatocellular Carcinoma. Cancers (Basel) (2021) 13(15):3740. doi: 10.3390/cancers13153740
4. Huang DQ, El-Serag HB, Loomba R. Global Epidemiology of NAFLD-Related HCC: Trends, Predictions, Risk Factors and Prevention. Nat Rev Gastroenterol Hepatol (2021) 18(4):223–38. doi: 10.1038/s41575-020-00381-6
5. Zhang C, Yang M. Current Options and Future Directions for NAFLD and NASH Treatment. Int J Mol Sci (2021) 22(14):7571. doi: 10.3390/ijms22147571
6. Canfora EE, Meex RCR, Venema K, Blaak EE. Gut Microbial Metabolites in Obesity, NAFLD and T2DM. Nat Rev Endocrinol (2019) 15(5):261–73. doi: 10.1038/s41574-019-0156-z
7. Tang WH, Kitai T, Hazen SL. Gut Microbiota in Cardiovascular Health and Disease. Circ Res (2017) 120(7):1183–96. doi: 10.1161/circresaha.117.309715
8. Ponziani FR, Bhoori S, Castelli C, Putignani L, Rivoltini L, Del Chierico F, et al. Hepatocellular Carcinoma Is Associated With Gut Microbiota Profile and Inflammation in Nonalcoholic Fatty Liver Disease. Hepatology (2019) 69(1):107–20. doi: 10.1002/hep.30036
9. Ren Z, Li A, Jiang J, Zhou L, Yu Z, Lu H, et al. Gut Microbiome Analysis as a Tool Towards Targeted Non-Invasive Biomarkers for Early Hepatocellular Carcinoma. Gut (2019) 68(6):1014–23. doi: 10.1136/gutjnl-2017-315084
10. Zhang X, Coker OO, Chu ESH, Fu K, Lau HCH, Wang Y-X, et al. Dietary Cholesterol Drives Fatty Liver-Associated Liver Cancer by Modulating Gut Microbiota and Metabolites. Gut (2021) 70(4):761. doi: 10.1136/gutjnl-2019-319664
11. Fei N, Bruneau A, Zhang X, Wang R, Wang J, Rabot S, et al. Endotoxin Producers Overgrowing in Human Gut Microbiota as the Causative Agents for Nonalcoholic Fatty Liver Disease. mBio (2020) 11(1):e03263–19. doi: 10.1128/mBio.03263-19
12. Patterson E, Ryan PM, Cryan JF, Dinan TG, Ross RP, Fitzgerald GF, et al. Gut Microbiota, Obesity and Diabetes. Postgrad Med J (2016) 92(1087):286–300. doi: 10.1136/postgradmedj-2015-133285
13. Yang M, Zhang C-Y. G Protein-Coupled Receptors as Potential Targets for Nonalcoholic Fatty Liver Disease Treatment. World J Gastroenterol (2021) 27(8):677–91. doi: 10.3748/wjg.v27.i8.677
14. Oliveira de Souza C, Sun X, Oh D. Metabolic Functions of G Protein-Coupled Receptors and β-Arrestin-Mediated Signaling Pathways in the Pathophysiology of Type 2 Diabetes and Obesity. Front Endocrinol (Lausanne) (2021) 12:715877. doi: 10.3389/fendo.2021.715877
15. Plouffe B, Thomsen ARB, Irannejad R. Emerging Role of Compartmentalized G Protein-Coupled Receptor Signaling in the Cardiovascular Field. ACS Pharmacol Trans Sci (2020) 3(2):221–36. doi: 10.1021/acsptsci.0c00006
16. Nemet I, Saha PP, Gupta N, Zhu W, Romano KA, Skye SM, et al. A Cardiovascular Disease-Linked Gut Microbial Metabolite Acts via Adrenergic Receptors. Cell (2020) 180(5):862–77.e22. doi: 10.1016/j.cell.2020.02.016
17. Stefan N, Häring HU, Cusi K. Non-Alcoholic Fatty Liver Disease: Causes, Diagnosis, Cardiometabolic Consequences, and Treatment Strategies. Lancet Diabetes Endocrinol (2019) 7(4):313–24. doi: 10.1016/s2213-8587(18)30154-2
18. Colditz GA, Peterson LL. Obesity and Cancer: Evidence, Impact, and Future Directions. Clin Chem (2018) 64(1):154–62. doi: 10.1373/clinchem.2017.277376
19. Loo TM, Kamachi F, Watanabe Y, Yoshimoto S, Kanda H, Arai Y, et al. Gut Microbiota Promotes Obesity-Associated Liver Cancer Through PGE2-Mediated Suppression of Antitumor Immunity. Cancer Discov (2017) 7(5):522–38. doi: 10.1158/2159-8290.cd-16-0932
20. Yoshimoto S, Loo TM, Atarashi K, Kanda H, Sato S, Oyadomari S, et al. Obesity-Induced Gut Microbial Metabolite Promotes Liver Cancer Through Senescence Secretome. Nature (2013) 499(7456):97–101. doi: 10.1038/nature12347
21. Góralska J, Raźny U, Polus A, Dziewońska A, Gruca A, Zdzienicka A, et al. Malczewska-Malec: Enhanced GIP Secretion in Obesity Is Associated With Biochemical Alteration and miRNA Contribution to the Development of Liver Steatosis. Nutrients (2020) 12(2):476. doi: 10.3390/nu12020476
22. Ringel AE, Drijvers JM, Baker GJ, Catozzi A, García-Cañaveras JC, Gassaway BM, et al. Obesity Shapes Metabolism in the Tumor Microenvironment to Suppress Anti-Tumor Immunity. Cell (2020) 183(7):1848–66.e26. doi: 10.1016/j.cell.2020.11.009
23. Zhang C, Yang M, Ericsson AC. The Potential Gut Microbiota-Mediated Treatment Options for Liver Cancer. Front Oncol (2020) 10:524205. doi: 10.3389/fonc.2020.524205
24. Chong LW, Tsai CL, Yang KC, Liao CC, Hsu YC. Targeting Protein Palmitoylation Decreases Palmitate−Induced Sphere Formation of Human Liver Cancer Cells. Mol Med Rep (2020) 22(2):939–47. doi: 10.3892/mmr.2020.11172
25. Broadfield LA, Duarte JAG, Schmieder R, Broekaert D, Veys K, Planque M, et al. Fat Induces Glucose Metabolism in Nontransformed Liver Cells and Promotes Liver Tumorigenesis. Cancer Res (2021) 81(8):1988–2001. doi: 10.1158/0008-5472.Can-20-1954
26. Angelin A, Gil-de-Gómez L, Dahiya S, Jiao J, Guo L, Levine MH, et al. Foxp3 Reprograms T Cell Metabolism to Function in Low-Glucose, High-Lactate Environments. Cell Metab (2017) 25(6):1282–93.e7. doi: 10.1016/j.cmet.2016.12.018
27. Saltiel AR, Olefsky JM. Inflammatory Mechanisms Linking Obesity and Metabolic Disease. J Clin Invest (2017) 127(1):1–4. doi: 10.1172/jci92035
28. Nadanaka S, Hashiguchi T, Kitagawa H. Aberrant Glycosaminoglycan Biosynthesis by Tumor Suppressor EXTL2 Deficiency Promotes Liver Inflammation and Tumorigenesis Through Toll-Like 4 Receptor Signaling. FASEB J (2020) 34(6):8385–401. doi: 10.1096/fj.201902076R
29. Rajesh Y, Sarkar D. Association of Adipose Tissue and Adipokines With Development of Obesity-Induced Liver Cancer. Int J Mol Sci (2021) 22(4):2163. doi: 10.3390/ijms22042163
30. Lin TC, Hsiao M. Leptin and Cancer: Updated Functional Roles in Carcinogenesis, Therapeutic Niches, and Developments. Int J Mol Sci (2021) 22(6):2870. doi: 10.3390/ijms22062870
31. Ribatti D, Belloni AS, Nico B, Di Comite M, Crivellato E, Vacca A. Leptin-Leptin Receptor Are Involved in Angiogenesis in Human Hepatocellular Carcinoma. Peptides (2008) 29(9):1596–602. doi: 10.1016/j.peptides.2008.05.011
32. Jiménez-Cortegana C, García-Galey A, Tami M, Del Pino P, Carmona I, López S, et al. Role of Leptin in Non-Alcoholic Fatty Liver Disease. Biomedicines (2021) 9(7):762. doi: 10.3390/biomedicines9070762
33. Parthasarathy G, Revelo X, Malhi H. Pathogenesis of Nonalcoholic Steatohepatitis: An Overview. Hepatol Commun (2020) 4(4):478–92. doi: 10.1002/hep4.1479
34. Park EJ, Lee JH, Yu GY, He G, Ali SR, Holzer RG, et al. Dietary and Genetic Obesity Promote Liver Inflammation and Tumorigenesis by Enhancing IL-6 and TNF Expression. Cell (2010) 140(2):197–208. doi: 10.1016/j.cell.2009.12.052
35. Nguyen J, Jiao J, Smoot K, Watt GP, Zhao C, Song X, et al. Toll-Like Receptor 4: A Target for Chemoprevention of Hepatocellular Carcinoma in Obesity and Steatohepatitis. Oncotarget (2018) 9(50):29495–507. doi: 10.18632/oncotarget.25685
36. Anguita-Ruiz A, Mendez-Gutierrez A, Ruperez AI, Leis R, Bueno G, Gil-Campos M, et al. The Protein S100A4 as a Novel Marker of Insulin Resistance in Prepubertal and Pubertal Children With Obesity. Metabolism (2020) 105:154187. doi: 10.1016/j.metabol.2020.154187
37. Giannini C, Feldstein AE, Santoro N, Kim G, Kursawe R, Pierpont B, et al. Circulating Levels of FGF-21 in Obese Youth: Associations With Liver Fat Content and Markers of Liver Damage. J Clin Endocrinol Metab (2013) 98(7):2993–3000. doi: 10.1210/jc.2013-1250%JTheJournalofClinicalEndocrinology&Metabolism
38. Singhal G, Kumar G, Chan S, Fisher FM, Ma Y, Vardeh HG, et al. Deficiency of Fibroblast Growth Factor 21 (FGF21) Promotes Hepatocellular Carcinoma (HCC) in Mice on a Long Term Obesogenic Diet. Mol Metab (2018) 13:56–66. doi: 10.1016/j.molmet.2018.03.002
39. Wang Z, Aguilar EG, Luna JI, Dunai C, Khuat LT, Le CT, et al. Paradoxical Effects of Obesity on T Cell Function During Tumor Progression and PD-1 Checkpoint Blockade. Nat Med (2019) 25(1):141–51. doi: 10.1038/s41591-018-0221-5
40. Pfister D, Núñez NG, Pinyol R, Govaere O, Pinter M, Szydlowska M, et al. NASH Limits Anti-Tumour Surveillance in Immunotherapy-Treated HCC. Nature (2021) 592(7854):450–6. doi: 10.1038/s41586-021-03362-0
41. Ma C, Kesarwala AH, Eggert T, Medina-Echeverz J, Kleiner DE, Jin P, et al. NAFLD Causes Selective CD4(+) T Lymphocyte Loss and Promotes Hepatocarcinogenesis. Nature (2016) 531(7593):253–7. doi: 10.1038/nature16969
42. Heinrich B, Brown ZJ, Diggs LP, Vormehr M, Ma C, Subramanyam V, et al. Steatohepatitis Impairs T-Cell-Directed Immunotherapies Against Liver Tumors in Mice. Gastroenterology (2021) 160(1):331–45.e6. doi: 10.1053/j.gastro.2020.09.031
43. Zhang C, Yang M. Targeting T Cell Subtypes for NAFLD and NAFLD-Related HCC Treatment: An Opinion. Front Med (2021) 8:789859. doi: 10.3389/fmed.2021.789859
44. Fernández Á F, Bárcena C, Martínez-García GG, Tamargo-Gómez I, Suárez MF, Pietrocola F, et al. Autophagy Couteracts Weight Gain, Lipotoxicity and Pancreatic β-Cell Death Upon Hypercaloric Pro-Diabetic Regimens. Cell Death Dis (2017) 8(8):e2970. doi: 10.1038/cddis.2017.373
45. Pietrocola F, Bravo-San Pedro JM. Targeting Autophagy to Counteract Obesity-Associated Oxidative Stress. Antioxid (Basel) (2021) 10(1):102. doi: 10.3390/antiox10010102
46. Forte M, Bianchi F, Cotugno M, Marchitti S, Stanzione R, Maglione V, et al. An Interplay Between UCP2 and ROS Protects Cells From High-Salt-Induced Injury Through Autophagy Stimulation. Cell Death Dis (2021) 12(10):919. doi: 10.1038/s41419-021-04188-4
47. Chen J, Pan Q, Bai Y, Chen X, Zhou Y. Hydroxychloroquine Induces Apoptosis in Cholangiocarcinoma via Reactive Oxygen Species Accumulation Induced by Autophagy Inhibition. Front Mol Biosci (2021) 8:720370. doi: 10.3389/fmolb.2021.720370
48. Simon TG, King LY, Chong DQ, Nguyen LH, Ma Y, VoPham T, et al. Diabetes, Metabolic Comorbidities, and Risk of Hepatocellular Carcinoma: Results From Two Prospective Cohort Studies. Hepatology (2018) 67(5):1797–806. doi: 10.1002/hep.29660
49. Umetsu S, Mizukami H, Saito T, Uchida C, Igawa A, Kudo K, et al. Diabetes, an Independent Poor Prognostic Factor of Non-B Non-C Hepatocellular Carcinoma, Correlates With Dihydropyrimidinase-Like 3 Promoter Methylation. Sci Rep (2020) 10(1):1156. doi: 10.1038/s41598-020-57883-1
50. Shima T, Uto H, Ueki K, Kohgo Y, Yasui K, Nakamura N, et al. Hepatocellular Carcinoma as a Leading Cause of Cancer-Related Deaths in Japanese Type 2 Diabetes Mellitus Patients. J Gastroenterol (2019) 54(1):64–77. doi: 10.1007/s00535-018-1494-7
51. Wei H, Wang J, Li W, Ma R, Xu Z, Luo Z, et al. The Underlying Pathophysiology Association Between the Type 2-Diabetic and Hepatocellular Carcinoma. J Cell Physiol (2019) 234(7):10835–41. doi: 10.1002/jcp.27919
52. Mantovani A, Byrne CD, Bonora E, Targher G. Nonalcoholic Fatty Liver Disease and Risk of Incident Type 2 Diabetes: A Meta-Analysis. Diabetes Care (2018) 41(2):372–82. doi: 10.2337/dc17-1902
53. Liu G, Xia F, Fan G, Yu J, Bao L, Zhang C, et al. Type 2 Diabetes Mellitus Worsens the Prognosis of Intermediate-Stage Hepatocellular Carcinoma After Transarterial Chemoembolization. Diabetes Res Clin Pract (2020) 169:108375. doi: 10.1016/j.diabres.2020.108375
54. Masarone M, Rosato V, Aglitti A, Bucci T, Caruso R, Salvatore T, et al. Liver Biopsy in Type 2 Diabetes Mellitus: Steatohepatitis Represents the Sole Feature of Liver Damage. PloS One (2017) 12(6):e0178473. doi: 10.1371/journal.pone.0178473
55. Sakurai Y, Kubota N, Takamoto I, Obata A, Iwamoto M, Hayashi T, et al. Role of Insulin Receptor Substrates in the Progression of Hepatocellular Carcinoma. Sci Rep (2017) 7(1):5387. doi: 10.1038/s41598-017-03299-3
56. Tovar V, Cornella H, Moeini A, Vidal S, Hoshida Y, Sia D, et al. Tumour Initiating Cells and IGF/FGF Signalling Contribute to Sorafenib Resistance in Hepatocellular Carcinoma. Gut (2017) 66(3):530–40. doi: 10.1136/gutjnl-2015-309501
57. Hamouda HA, Mansour SM, Elyamany MF. Vitamin D Combined With Pioglitazone Mitigates Type-2 Diabetes-Induced Hepatic Injury Through Targeting Inflammation, Apoptosis, and Oxidative Stress. Inflammation (2021). doi: 10.1007/s10753-021-01535-7
58. Zhang Y, Wang H, Zhang L, Yuan Y, Yu D. Codonopsis Lanceolata Polysaccharide CLPS Alleviates High Fat/High Sucrose Diet-Induced Insulin Resistance via Anti-Oxidative Stress. Int J Biol Macromol (2020) 145:944–9. doi: 10.1016/j.ijbiomac.2019.09.185
59. Gurung M, Li Z, You H, Rodrigues R, Jump DB, Morgun A, et al. Role of Gut Microbiota in Type 2 Diabetes Pathophysiology. EBioMedicine (2020) 51:102590. doi: 10.1016/j.ebiom.2019.11.051
60. Kawaguchi T, Nakano D, Okamura S, Shimose S, Hayakawa M, Niizeki T, et al. Spontaneous Regression of Hepatocellular Carcinoma With Reduction in Angiogenesis-Related Cytokines After Treatment With Sodium-Glucose Cotransporter 2 Inhibitor in a Cirrhotic Patient With Diabetes Mellitus. Hepatol Res (2019) 49(4):479–86. doi: 10.1111/hepr.13247
61. Michurina SV, Ishchenko IY, Arkhipov SA, Cherepanova MA, Vasendin DV, Zavjalov EL. Apoptosis in the Liver of Male Db/Db Mice During the Development of Obesity and Type 2 Diabetes. Vavilovskii Zhurnal Genet Selektsii (2020) 24(4):435–40. doi: 10.18699/VJ20.43-o
62. Yoo J, Jeong IK, Ahn KJ, Chung HY, Hwang YC. Fenofibrate, a Pparα Agonist, Reduces Hepatic Fat Accumulation Through the Upregulation of TFEB-Mediated Lipophagy. Metabolism (2021) 120:154798. doi: 10.1016/j.metabol.2021.154798
63. Soysal P, Arik F, Smith L, Jackson SE, Isik AT. Inflammation, Frailty and Cardiovascular Disease. Adv Exp Med Biol (2020) 1216:55–64. doi: 10.1007/978-3-030-33330-0_7
64. Suresh D, Srinivas AN, Kumar DP. Etiology of Hepatocellular Carcinoma: Special Focus on Fatty Liver Disease. Front Oncol (2020) 10:601710. doi: 10.3389/fonc.2020.601710
65. Giraud J, Saleh M. Host-Microbiota Interactions in Liver Inflammation and Cancer. Cancers (Basel) (2021) 13(17):4342. doi: 10.3390/cancers13174342
66. Matyas C, Haskó G, Liaudet L, Trojnar E, Pacher P. Interplay of Cardiovascular Mediators, Oxidative Stress and Inflammation in Liver Disease and Its Complications. Nat Rev Cardiol (2021) 18(2):117–35. doi: 10.1038/s41569-020-0433-5
67. Steven S, Frenis K, Oelze M, Kalinovic S, Kuntic M, Bayo Jimenez MT, et al. Vascular Inflammation and Oxidative Stress: Major Triggers for Cardiovascular Disease. Oxid Med Cell Longev (2019) 2019:7092151. doi: 10.1155/2019/7092151
68. Roncal C, Martínez-Aguilar E, Orbe J, Ravassa S, Fernandez-Montero A, Saenz-Pipaon G, et al. Trimethylamine-N-Oxide (TMAO) Predicts Cardiovascular Mortality in Peripheral Artery Disease. Sci Rep (2019) 9(1):15580. doi: 10.1038/s41598-019-52082-z
69. Liu ZY, Tan XY, Li QJ, Liao GC, Fang AP, Zhang DM, et al. Trimethylamine N-Oxide, a Gut Microbiota-Dependent Metabolite of Choline, Is Positively Associated With the Risk of Primary Liver Cancer: A Case-Control Study. Nutr Metab (Lond) (2018) 15:81. doi: 10.1186/s12986-018-0319-2
70. León-Mimila P, Villamil-Ramírez H, Li XS, Shih DM, Hui ST, Ocampo-Medina E, et al. Trimethylamine N-Oxide Levels Are Associated With NASH in Obese Subjects With Type 2 Diabetes. Diabetes Metab (2021) 47(2):101183. doi: 10.1016/j.diabet.2020.07.010
71. Cohain AT, Barrington WT, Jordan DM, Beckmann ND, Argmann CA, Houten SM, et al. An Integrative Multiomic Network Model Links Lipid Metabolism to Glucose Regulation in Coronary Artery Disease. Nat Commun (2021) 12(1):547. doi: 10.1038/s41467-020-20750-8
72. Ponce de León-Ballesteros G, Sánchez-Aguilar HA, Aguilar-Salinas CA, Herrera MF. Reaching LDL-C Targets in Patients With Moderate, High, and Very High Risk for Cardiovascular Disease After Bariatric Surgery According to Different Guidelines. Obes Surg (2021) 31(5):2087–96. doi: 10.1007/s11695-021-05221-3
73. Ormazabal V, Nair S, Elfeky O, Aguayo C, Salomon C, Zuñiga FA. Association Between Insulin Resistance and the Development of Cardiovascular Disease. Cardiovasc Diabetol (2018) 17(1):122. doi: 10.1186/s12933-018-0762-4
74. Mohammadi A, Balizadeh Karami AR, Dehghan Mashtani V, Sahraei T, Bandani Tarashoki Z, Khattavian E, et al. Evaluation of Oxidative Stress, Apoptosis, and Expression of MicroRNA-208a and MicroRNA-1 in Cardiovascular Patients. Rep Biochem Mol Biol (2021) 10(2):183–96. doi: 10.52547/rbmb.10.2.183
75. Chen X, Li X, Xu X, Li L, Liang N, Zhang L, et al. Ferroptosis and Cardiovascular Disease: Role of Free Radical-Induced Lipid Peroxidation. Free Radic Res (2021) 55(4):405–15. doi: 10.1080/10715762.2021.1876856
76. Gao J, Chen X, Wei P, Wang Y, Li P, Shao K. Regulation of Pyroptosis in Cardiovascular Pathologies: Role of Noncoding RNAs. Mol Ther Nucleic Acids (2021) 25:220–36. doi: 10.1016/j.omtn.2021.05.016
77. Guerreiro GTS, Longo L, Fonseca MA, de Souza VEG, Álvares-da-Silva MR. Does the Risk of Cardiovascular Events Differ Between Biopsy-Proven NAFLD and MAFLD? Hepatol Int (2021) 15(2):380–91. doi: 10.1007/s12072-021-10157-y
78. Bu S, Singh KK. Epigenetic Regulation of Autophagy in Cardiovascular Pathobiology. Int J Mol Sci (2021) 22(12):6544. doi: 10.3390/ijms22126544
79. Weiner DE, Tabatabai S, Tighiouart H, Elsayed E, Bansal N, Griffith J, et al. Cardiovascular Outcomes and All-Cause Mortality: Exploring the Interaction Between CKD and Cardiovascular Disease. Am J Kidney Dis (2006) 48(3):392–401. doi: 10.1053/j.ajkd.2006.05.021
80. Targher G, Byrne CD, Lonardo A, Zoppini G, Barbui C. Non-Alcoholic Fatty Liver Disease and Risk of Incident Cardiovascular Disease: A Meta-Analysis. J Hepatol (2016) 65(3):589–600. doi: 10.1016/j.jhep.2016.05.013
81. Stefan N, Häring HU. The Role of Hepatokines in Metabolism. Nat Rev Endocrinol (2013) 9(3):144–52. doi: 10.1038/nrendo.2012.258
82. Alipour Talesh G, Trézéguet V, Merched A. Hepatocellular Carcinoma and Statins. Biochemistry (2020) 59(37):3393–400. doi: 10.1021/acs.biochem.0c00476
83. Kawaguchi Y, Sakamoto Y, Ito D, Ito K, Arita J, Akamatsu N, et al. Statin Use Is Associated With a Reduced Risk of Hepatocellular Carcinoma Recurrence After Initial Liver Resection. Biosci Trends (2017) 11(5):574–80. doi: 10.5582/bst.2017.01191
84. Bagchi DP, Nishii A, Li Z, DelProposto JB, Corsa CA, Mori H, et al. Wnt/β-Catenin Signaling Regulates Adipose Tissue Lipogenesis and Adipocyte-Specific Loss Is Rigorously Defended by Neighboring Stromal-Vascular Cells. Mol Metab (2020) 42:101078. doi: 10.1016/j.molmet.2020.101078
85. Jia WQ, Zhou TC, Dai JW, Liu ZN, Zhang YF, Zang DD, et al. CD73 Regulates Hepatic Stellate Cells Activation and Proliferation Through Wnt/β-Catenin Signaling Pathway. Eur J Pharmacol (2021) 890:173667. doi: 10.1016/j.ejphar.2020.173667
86. Haybar H, Khodadi E, Shahrabi S. Wnt/β-Catenin in Ischemic Myocardium: Interactions and Signaling Pathways as a Therapeutic Target. Heart Fail Rev (2019) 24(3):411–9. doi: 10.1007/s10741-018-9759-z
87. Perugorria MJ, Olaizola P, Labiano I, Esparza-Baquer A, Marzioni M, Marin JJG, et al. Wnt-β-Catenin Signalling in Liver Development, Health and Disease. Nat Rev Gastroenterol Hepatol (2019) 16(2):121–36. doi: 10.1038/s41575-018-0075-9
88. Wu Y, Shan B, Dai J, Xia Z, Cai J, Chen T, et al. Dual Role for Inositol-Requiring Enzyme 1α in Promoting the Development of Hepatocellular Carcinoma During Diet-Induced Obesity in Mice. Hepatology (2018) 68(2):533–46. doi: 10.1002/hep.29871
89. Qin YE, Duan L, He Y, Yuan C, Wang T, Yuan D, et al. Saturated Fatty Acids Promote Hepatocytic Senecence Through Regulation of miR-34a/Cyclin-Dependent Kinase 6. Mol Nutr Food Res (2020) 64:e2000383. doi: 10.1002/mnfr.202000383
90. Liu N, Chang CW, Steer CJ, Wang XW, Song G. MicroRNA-15a/16-1 Prevents Hepatocellular Carcinoma by Disrupting the Communication Between Kupffer Cells and Tregs. Gastroenterology (2021). doi: 10.1053/j.gastro.2021.10.015
91. Xu G, Bu S, Wang X, Ge H. MiR-122 Radiosensitize Hepatocellular Carcinoma Cells by Suppressing Cyclin G1. Int J Radiat Biol (2021) 1–7. doi: 10.1080/09553002.2021.1987561
92. Seo J, Jeong D-W, Park J-W, Lee K-W, Fukuda J, Chun Y-S. Fatty-Acid-Induced FABP5/HIF-1 Reprograms Lipid Metabolism and Enhances the Proliferation of Liver Cancer Cells. Commun Biol (2020) 3(1):638. doi: 10.1038/s42003-020-01367-5
93. Liu Y, Liu L, Zhou Y, Zhou P, Yan Q, Chen X, et al. CKLF1 Enhances Inflammation-Mediated Carcinogenesis and Prevents Doxorubicin-Induced Apoptosis via IL6/STAT3 Signaling in HCC. Clin Cancer Res (2019) 25(13):4141–54. doi: 10.1158/1078-0432.Ccr-18-3510
94. Yao RR, Li JH, Zhang R, Chen RX, Wang YH. M2-Polarized Tumor-Associated Macrophages Facilitated Migration and Epithelial-Mesenchymal Transition of HCC Cells via the TLR4/STAT3 Signaling Pathway. World J Surg Oncol (2018) 16(1):9. doi: 10.1186/s12957-018-1312-y
95. Wang D, Zheng X, Fu B, Nian Z, Qian Y, Sun R, et al. Hepatectomy Promotes Recurrence of Liver Cancer by Enhancing IL-11-STAT3 Signaling. EBioMedicine (2019) 46:119–32. doi: 10.1016/j.ebiom.2019.07.058
96. Gong C, Ai J, Fan Y, Gao J, Liu W, Feng Q, et al. NCAPG Promotes The Proliferation Of Hepatocellular Carcinoma Through PI3K/AKT Signaling. Onco Targets Ther (2019) 12:8537–52. doi: 10.2147/ott.S217916
97. Liu JS, Huo CY, Cao HH, Fan CL, Hu JY, Deng LJ, et al. Aloperine Induces Apoptosis and G2/M Cell Cycle Arrest in Hepatocellular Carcinoma Cells Through the PI3K/Akt Signaling Pathway. Phytomedicine (2019) 61:152843. doi: 10.1016/j.phymed.2019.152843
98. Jiang H, Zhou Z, Jin S, Xu K, Zhang H, Xu J, et al. PRMT9 Promotes Hepatocellular Carcinoma Invasion and Metastasis via Activating PI3K/Akt/GSK-3β/Snail Signaling. Cancer Sci (2018) 109(5):1414–27. doi: 10.1111/cas.13598
99. Zhang Q, Zhang Y, Sun S, Wang K, Qian J, Cui Z, et al. ACOX2 Is a Prognostic Marker and Impedes the Progression of Hepatocellular Carcinoma via Pparα Pathway. Cell Death Dis (2021) 12(1):15. doi: 10.1038/s41419-020-03291-2
100. Li Z, Li H, Zhao ZB, Zhu W, Feng PP, Zhu XW, et al. SIRT4 Silencing in Tumor-Associated Macrophages Promotes HCC Development via Pparδ Signalling-Mediated Alternative Activation of Macrophages. J Exp Clin Cancer Res (2019) 38(1):469. doi: 10.1186/s13046-019-1456-9
101. Feng J, Dai W, Mao Y, Wu L, Li J, Chen K, et al. Simvastatin Re-Sensitizes Hepatocellular Carcinoma Cells to Sorafenib by Inhibiting HIF-1α/PPAR-γ/PKM2-Mediated Glycolysis. J Exp Clin Cancer Res (2020) 39(1):24. doi: 10.1186/s13046-020-1528-x
102. Wu H, Ng R, Chen X, Steer CJ, Song G. MicroRNA-21 Is a Potential Link Between Non-Alcoholic Fatty Liver Disease and Hepatocellular Carcinoma via Modulation of the HBP1-P53-Srebp1c Pathway. Gut (2016) 65(11):1850–60. doi: 10.1136/gutjnl-2014-308430
103. Lacin S, Yalcin S. The Prognostic Value of Circulating VEGF-A Level in Patients With Hepatocellular Cancer. Technol Cancer Res Treat (2020) 19:1533033820971677. doi: 10.1177/1533033820971677
104. Modi SJ, Kulkarni VM. Discovery of VEGFR-2 Inhibitors Exerting Significant Anticancer Activity Against CD44+ and CD133+ Cancer Stem Cells (CSCs): Reversal of TGF-β Induced Epithelial-Mesenchymal Transition (EMT) in Hepatocellular Carcinoma. Eur J Med Chem (2020) 207:112851. doi: 10.1016/j.ejmech.2020.112851
105. Anstee QM, Reeves HL, Kotsiliti E, Govaere O, Heikenwalder M. From NASH to HCC: Current Concepts and Future Challenges. Nat Rev Gastroenterol Hepatol (2019) 16(7):411–28. doi: 10.1038/s41575-019-0145-7
106. Kennedy OJ, Roderick P, Buchanan R, Fallowfield JA, Hayes PC, Parkes J. Coffee, Including Caffeinated and Decaffeinated Coffee, and the Risk of Hepatocellular Carcinoma: A Systematic Review and Dose-Response Meta-Analysis. BMJ Open (2017) 7(5):e013739. doi: 10.1136/bmjopen-2016-013739
107. Sun M, Cui H, Liang M, Wang W, Wang Y, Liu X, et al. Perceived Dietary Salt Intake and the Risk of Primary Liver Cancer: A Population-Based Prospective Study. J Hum Nutr Diet (2020) 33(6):833–40. doi: 10.1111/jhn.12761
108. Romero-Gómez M, Zelber-Sagi S, Trenell M. Treatment of NAFLD With Diet, Physical Activity and Exercise. J Hepatol (2017) 67(4):829–46. doi: 10.1016/j.jhep.2017.05.016
109. Stefan N. Causes, Consequences, and Treatment of Metabolically Unhealthy Fat Distribution. Lancet Diabetes Endocrinol (2020) 8(7):616–27. doi: 10.1016/s2213-8587(20)30110-8
110. Wu HC, Shen J, Siegel A, Santella RM. Environmental Exposure and Clinical Correlates of Hepatocellular Carcinoma in New York City: A Case Only Study. Cancer Causes Control (2021). doi: 10.1007/s10552-021-01494-2
111. Hamid AS, Tesfamariam IG, Zhang Y, Zhang ZG. Aflatoxin B1-Induced Hepatocellular Carcinoma in Developing Countries: Geographical Distribution, Mechanism of Action and Prevention. Oncol Lett (2013) 5(4):1087–92. doi: 10.3892/ol.2013.1169
112. Mahato DK, Lee KE, Kamle M, Devi S, Dewangan KN, Kumar P, et al. Aflatoxins in Food and Feed: An Overview on Prevalence, Detection and Control Strategies. Front Microbiol (2019) 10:2266. doi: 10.3389/fmicb.2019.02266
113. Inoue-Yamauchi A, Itagaki H, Oda H. Eicosapentaenoic Acid Attenuates Obesity-Related Hepatocellular Carcinogenesis. Carcinogenesis (2018) 39(1):28–35. doi: 10.1093/carcin/bgx112
114. Green CJ, Pramfalk C, Charlton CA, Gunn PJ, Cornfield T, Pavlides M, et al. Hepatic De Novo Lipogenesis Is Suppressed and Fat Oxidation Is Increased by Omega-3 Fatty Acids at the Expense of Glucose Metabolism. BMJ Open Diabetes Res Care (2020) 8(1). doi: 10.1136/bmjdrc-2019-000871
115. Wu BK, Chen QH, Pan D, Chang B, Sang LX. A Novel Therapeutic Strategy for Hepatocellular Carcinoma: Immunomodulatory Mechanisms of Selenium and/or Selenoproteins on a Shift Towards Anti-Cancer. Int Immunopharmacol (2021) 96:107790. doi: 10.1016/j.intimp.2021.107790
116. Kwak M, Mehaffey JH, Hawkins RB, Hsu A, Schirmer B, Hallowell PT. Bariatric Surgery Is Associated With Reduction in Non-Alcoholic Steatohepatitis and Hepatocellular Carcinoma: A Propensity Matched Analysis. Am J Surg (2020) 219(3):504–7. doi: 10.1016/j.amjsurg.2019.09.006
117. Lassailly G, Caiazzo R, Buob D, Pigeyre M, Verkindt H, Labreuche J, et al. Bariatric Surgery Reduces Features of Nonalcoholic Steatohepatitis in Morbidly Obese Patients. Gastroenterology (2015) 149(2):379–88; quiz e15-6. doi: 10.1053/j.gastro.2015.04.014
118. Ramai D, Singh J, Lester J, Khan SR, Chandan S, Tartaglia N, et al. Systematic Review With Meta-Analysis: Bariatric Surgery Reduces the Incidence of Hepatocellular Carcinoma. Aliment Pharmacol Ther (2021) 53(9):977–84. doi: 10.1111/apt.16335
119. Delaune V, Orci LA, Lacotte S, Peloso A, Schrenzel J, Lazarevic V, et al. Fecal Microbiota Transplantation: A Promising Strategy in Preventing the Progression of Non-Alcoholic Steatohepatitis and Improving the Anti-Cancer Immune Response. Expert Opin Biol Ther (2018) 18(10):1061–71. doi: 10.1080/14712598.2018.1518424
120. Yang M, Kimchi ET, Staveley-O’Carroll KF, Li G. Astaxanthin Prevents Diet-Induced NASH Progression by Shaping Intrahepatic Immunity. Int J Mol Sci (2021) 22(20):11037. doi: 10.3390/ijms222011037
121. Zhao D, Xia L, Geng W, Xu D, Zhong C, Zhang J, et al. Metformin Suppresses Interleukin-22 Induced Hepatocellular Carcinoma by Upregulating Hippo Signaling Pathway. J Gastroenterol Hepatol (2021). doi: 10.1111/jgh.15674
122. Liu H, Liu M, Fu X, Zhang Z, Zhu L, Zheng X, et al. Astaxanthin Prevents Alcoholic Fatty Liver Disease by Modulating Mouse Gut Microbiota. Nutrients (2018) 10(9):1298. doi: 10.3390/nu10091298
123. Simon TG, Henson J, Osganian S, Masia R, Chan AT, Chung RT, et al. Daily Aspirin Use Associated With Reduced Risk For Fibrosis Progression In Patients With Nonalcoholic Fatty Liver Disease. Clin Gastroenterol Hepatol (2019) 17(13):2776–2784.e4. doi: 10.1016/j.cgh.2019.04.061
124. Ricciotti E, Wangensteen KJ, FitzGerald GA. Aspirin in Hepatocellular Carcinoma. Cancer Res (2021) 81(14):3751–61. doi: 10.1158/0008-5472.Can-21-0758
125. Tangjarusritaratorn T, Tangjittipokin W, Kunavisarut T. Incidence and Survival of Hepatocellular Carcinoma in Type 2 Diabetes Patients With Cirrhosis Who Were Treated With and Without Metformin. Diabetes Metab Syndr Obes (2021) 14:1563–74. doi: 10.2147/dmso.S295753
126. Zhou J, Ke Y, Lei X, Wu T, Li Y, Bao T, et al. Meta-Analysis: The Efficacy of Metformin and Other Anti-Hyperglycemic Agents in Prolonging the Survival of Hepatocellular Carcinoma Patients With Type 2 Diabetes. Ann Hepatol (2020) 19(3):320–8. doi: 10.1016/j.aohep.2019.11.008
127. Wang CP, Kuhn J, Shah DP, Schmidt S, Lam YF, MacCarthy D, et al. Metformin Modifies Disparity in Hepatocellular Carcinoma Incidence in Men With Type 2 Diabetes But Without Chronic Liver Diseases. Cancer Med (2019) 8(6):3206–15. doi: 10.1002/cam4.2142
128. Wang W, Wu RD, Chen P, Xu XJ, Shi XZ, Huang LH, et al. Liraglutide Combined With Human Umbilical Cord Mesenchymal Stem Cell Transplantation Inhibits Beta-Cell Apoptosis via Mediating the ASK1/JNK/BAX Pathway in Rats With Type 2 Diabetes. Diabetes Metab Res Rev (2020) 36(2):e3212. doi: 10.1002/dmrr.3212
129. Xu X, Wang W, Lin L, Chen P. Liraglutide in Combination With Human Umbilical Cord Mesenchymal Stem Cell Could Improve Liver Lesions by Modulating TLR4/NF-kB Inflammatory Pathway and Oxidative Stress in T2DM/NAFLD Rats. Tissue Cell (2020) 66:101382. doi: 10.1016/j.tice.2020.101382
130. Kim G, Jang SY, Han E, Lee YH, Park SY, Nam CM, et al. Effect of Statin on Hepatocellular Carcinoma in Patients With Type 2 Diabetes: A Nationwide Nested Case-Control Study. Int J Cancer (2017) 140(4):798–806. doi: 10.1002/ijc.30506
131. Yang SY, Wang CC, Chen KD, Liu YW, Lin CC, Chuang CH, et al. Statin Use Is Associated With a Lower Risk of Recurrence After Curative Resection in BCLC Stage 0-A Hepatocellular Carcinoma. BMC Cancer (2021) 21(1):70. doi: 10.1186/s12885-021-07796-7
132. Zhang S, Cao M, Hou Z, Gu X, Chen Y, Chen L, et al. Angiotensin-Converting Enzyme Inhibitors Have Adverse Effects in Anti-Angiogenesis Therapy for Hepatocellular Carcinoma. Cancer Lett (2021) 501:147–61. doi: 10.1016/j.canlet.2020.12.031
133. Casadei Gardini A, Marisi G, Scarpi E, Scartozzi M, Faloppi L, Silvestris N, et al. Effects of Metformin on Clinical Outcome in Diabetic Patients With Advanced HCC Receiving Sorafenib. Expert Opin Pharmacother (2015) 16(18):2719–25. doi: 10.1517/14656566.2015.1102887
134. Casadei Gardini A, Faloppi L, De Matteis S, Foschi FG, Silvestris N, Tovoli F, et al. Metformin and Insulin Impact on Clinical Outcome in Patients With Advanced Hepatocellular Carcinoma Receiving Sorafenib: Validation Study and Biological Rationale. Eur J Cancer (2017) 86:106–14. doi: 10.1016/j.ejca.2017.09.003
135. Hu W, Li M, Sun W, Li Q, Xi H, Qiu Y, et al. Hirsutine Ameliorates Hepatic and Cardiac Insulin Resistance in High-Fat Diet-Induced Diabetic Mice and. Vitro models Pharmacol Res (2021) 105917. doi: 10.1016/j.phrs.2021.105917
136. Chaiwong S, Chatturong U, Chanasong R, Deetud W, To-On K, Puntheeranurak S, et al. Dried Mulberry Fruit Ameliorates Cardiovascular and Liver Histopathological Changes in High-Fat Diet-Induced Hyperlipidemic Mice. J Tradit Complement Med (2021) 11(4):356–68. doi: 10.1016/j.jtcme.2021.02.006
137. Adinolfi LE, Petta S, Fracanzani AL, Nevola R, Coppola C, Narciso V, et al. Reduced Incidence of Type 2 Diabetes in Patients With Chronic Hepatitis C Virus Infection Cleared by Direct-Acting Antiviral Therapy: A Prospective Study. Diabetes Obes Metab (2020) 22(12):2408–16. doi: 10.1111/dom.14168
138. Adinolfi LE, Petta S, Fracanzani AL, Coppola C, Narciso V, Nevola R, et al. Impact of Hepatitis C Virus Clearance by Direct-Acting Antiviral Treatment on the Incidence of Major Cardiovascular Events: A Prospective Multicentre Study. Atherosclerosis (2020) 296:40–7. doi: 10.1016/j.atherosclerosis.2020.01.010
Keywords: hepatocellular carcinoma, obesity, type 2 diabetes mellitus, cardiovascular diseases, nonalcoholic fatty liver disease, signaling pathway, treatment
Citation: Zhang C, Liu S and Yang M (2021) Hepatocellular Carcinoma and Obesity, Type 2 Diabetes Mellitus, Cardiovascular Disease: Causing Factors, Molecular Links, and Treatment Options. Front. Endocrinol. 12:808526. doi: 10.3389/fendo.2021.808526
Received: 03 November 2021; Accepted: 07 December 2021;
Published: 23 December 2021.
Edited by:
Che-Pei Kung, Washington University School of Medicine in St. Louis, United StatesReviewed by:
Ferdinando Carlo Sasso, Università della Campania Luigi Vanvitelli, ItalyCopyright © 2021 Zhang, Liu and Yang. This is an open-access article distributed under the terms of the Creative Commons Attribution License (CC BY). The use, distribution or reproduction in other forums is permitted, provided the original author(s) and the copyright owner(s) are credited and that the original publication in this journal is cited, in accordance with accepted academic practice. No use, distribution or reproduction is permitted which does not comply with these terms.
*Correspondence: Ming Yang, eWFuZ21pbkBoZWFsdGgubWlzc291cmkuZWR1
†These authors share first authorship
Disclaimer: All claims expressed in this article are solely those of the authors and do not necessarily represent those of their affiliated organizations, or those of the publisher, the editors and the reviewers. Any product that may be evaluated in this article or claim that may be made by its manufacturer is not guaranteed or endorsed by the publisher.
Research integrity at Frontiers
Learn more about the work of our research integrity team to safeguard the quality of each article we publish.