- Department of Biomedical Sciences, University of Guelph, Guelph, ON, Canada
Epithelial ovarian cancer is predominantly diagnosed at advanced stages which creates significant therapeutic challenges. As a result, the 5-year survival rate is low. Within ovarian cancer, significant tumor heterogeneity exists, and the tumor microenvironment is diverse. Tumor heterogeneity leads to diversity in therapy response within the tumor, which can lead to resistance or recurrence. Advancements in therapy development and tumor profiling have initiated a shift from a “one-size-fits-all” approach towards precision patient-based therapies. Here, we review aspects of ovarian tumor heterogeneity that facilitate tumorigenesis and contribute to treatment failure. These tumor characteristics should be considered when designing novel therapies or characterizing mechanisms of treatment resistance. Individual patients vary considerably in terms of age, fertility and contraceptive use which innately affects the endocrine milieu in the ovary. Similarly, individual tumors differ significantly in their immune profile, which can impact the efficacy of immunotherapies. Tumor size, presence of malignant ascites and vascular density further alters the tumor microenvironment, creating areas of significant hypoxia that is notorious for increasing tumorigenesis, resistance to standard of care therapies and promoting stemness and metastases. We further expand on strategies aimed at improving oxygenation status in tumors to dampen downstream effects of hypoxia and set the stage for better response to therapy.
Epithelial Ovarian Cancer
Epithelial ovarian cancer (EOC) is the most lethal gynecological cancer, and it is the fifth leading cause of cancer related deaths in women (1). A lack of disease-specific symptoms makes early detection difficult, with most women being diagnosed with EOC at an advanced stage (2). At diagnosis, most women have a large primary ovarian tumor, multiple metastatic secondary tumors and abdominal ascites (3). With the advanced stage at diagnosis, it is difficult to effectively treat the disease resulting in a 5-year survival rate for women diagnosed at stages 3 and 4 of 42% and 26% respectively (4).
Ovarian cancer most commonly presents in post-menopausal women at which point there are other age-related physiologic changes. Contributing risk factors include a familial history of EOC, increased lifetime ovulatory events due to nulliparity, undergoing hormone replacement therapy (HRT) and comorbidity factors such as diabetes and obesity (5, 6). Many of these risk factors involve prolonged exposure to steroid hormones. While post-menopausal women experience a decrease in hormone production, prolonged and chronic exposure to these hormones throughout their life contribute to an increased risk of EOC (7). Similarly, post-menopausal women on HRT have a further increase in exposure, enhancing the risk of EOC (8).
Currently, the common treatment protocol for ovarian cancer includes cytoreductive surgical debulking accompanied with chemotherapy, typically carboplatin and paclitaxel (9). Although there is often initial responsiveness to chemotherapy, most women develop chemoresistance and disease recurrence (10). As such, novel therapeutic approaches are needed to prevent chemoresistance and improve treatment success.
The Endocrine System in Ovarian Cancer Tumorigenesis
The ovary is surrounded by a single layer of epithelial cells called the ovarian surface epithelium (OSE). The activity of the OSE is hormone-dependent; the ovary is a primary endocrine organ where both peptide and steroid hormones act on the OSE cells to mediate their activity throughout various reproductive processes. These hormones have an influential role on proliferation and differentiation of OSE cells and aberrant endocrine signaling can inflate the risk of EOC and contribute to its tumorigenesis (11, 12).
Gonadotropin-releasing hormone (GnRH) is a peptide hormone secreted by the hypothalamus. Its primary function is to regulate the production and release of luteinizing hormone (LH) and follicle stimulating hormone (FSH) from the anterior pituitary (11). These hormones, with the addition of human chorionic gonadotrophin (hCG), have been shown to elicit pro-proliferative effects on EOC cells through activation of gonadotropin-response genes, increased growth factor signaling and ovarian production of sex steroids, suggesting an indirect role of GnRH in EOC tumorigenesis (13–15). Additionally, GnRH stimulation of EOC cell-bound GnRH Type I receptors elicit anti-proliferative and anti-apoptotic signals through mechanisms involving activation of phosphotyrosine phosphatase and NFκB, respectively (16, 17). At the level of the ovary, imbalances of inhibin and activin expression in tumor cells may further attribute to EOC tumorigenesis by supporting cell survival and stimulating proliferation (18). These proteins are secreted by the ovary and function primarily to regulate FSH production at the level of the anterior pituitary. Activin directly stimulates FSH from the anterior pituitary and inhibin suppresses activin signaling by binding and sequestering it thereby preventing it from binding to its receptors (11). Additional evidence has suggested paracrine and/or autocrine functions of these proteins in the ovarian tumor microenvironment (TME) with further influences on ovarian steroid synthesis, proliferation and tumor invasion (18–20) although the mechanisms by which these proteins influence these processes remain unclear.
The involvement of steroid hormones in ovarian cancer carcinogenesis is supported by both experimental and epidemiological findings (21, 22). Protective effects of oral contraceptives, particularly progestin-only formulations, as well as multiparity suggest an inverse relationship between progesterone and ovarian cancer risk (23, 24). Experimental findings on progesterone’s role in EOC are conflicting with both positive and negative effects on tumor invasiveness and metastasis (25, 26). Protective effects of breastfeeding and pregnancy suggest a similar inverse relationship between estrogen levels and EOC risk (11, 22). Experimental findings support a pro-tumorigenic role of estrogen and suggests estrogen may elicit EOC cell proliferation through increases in growth factor receptor expression, stimulation and/or increased expression of c-myc (27–29). Elevated risk associated with previous polycystic ovarian syndrome diagnosis and treatment with Danazol, a therapy used in the treatment of endometriosis, suggests a pro-tumorigenic role of androgens in EOC (22, 30). Elevated levels of dihydrotestosterone and testosterone are correlated with an increase in tumor volume in EOC and elevated dihydrotestosterone alone can increase IL-6 mRNA and protein levels and suppress the anti-proliferative effects of TGF-β1 resulting in EOC cell proliferation (31, 32).
With the growing body of epidemiological and experimental evidence, an association between the endocrine status of the patient and ovarian cancer tumorigenesis is well-supported as summarized in Figure 1. However, the specific mechanisms in which hormones impart their effects remain unclear. Future research is needed to elucidate the role of hormonal stimulation in EOC risk and progression.
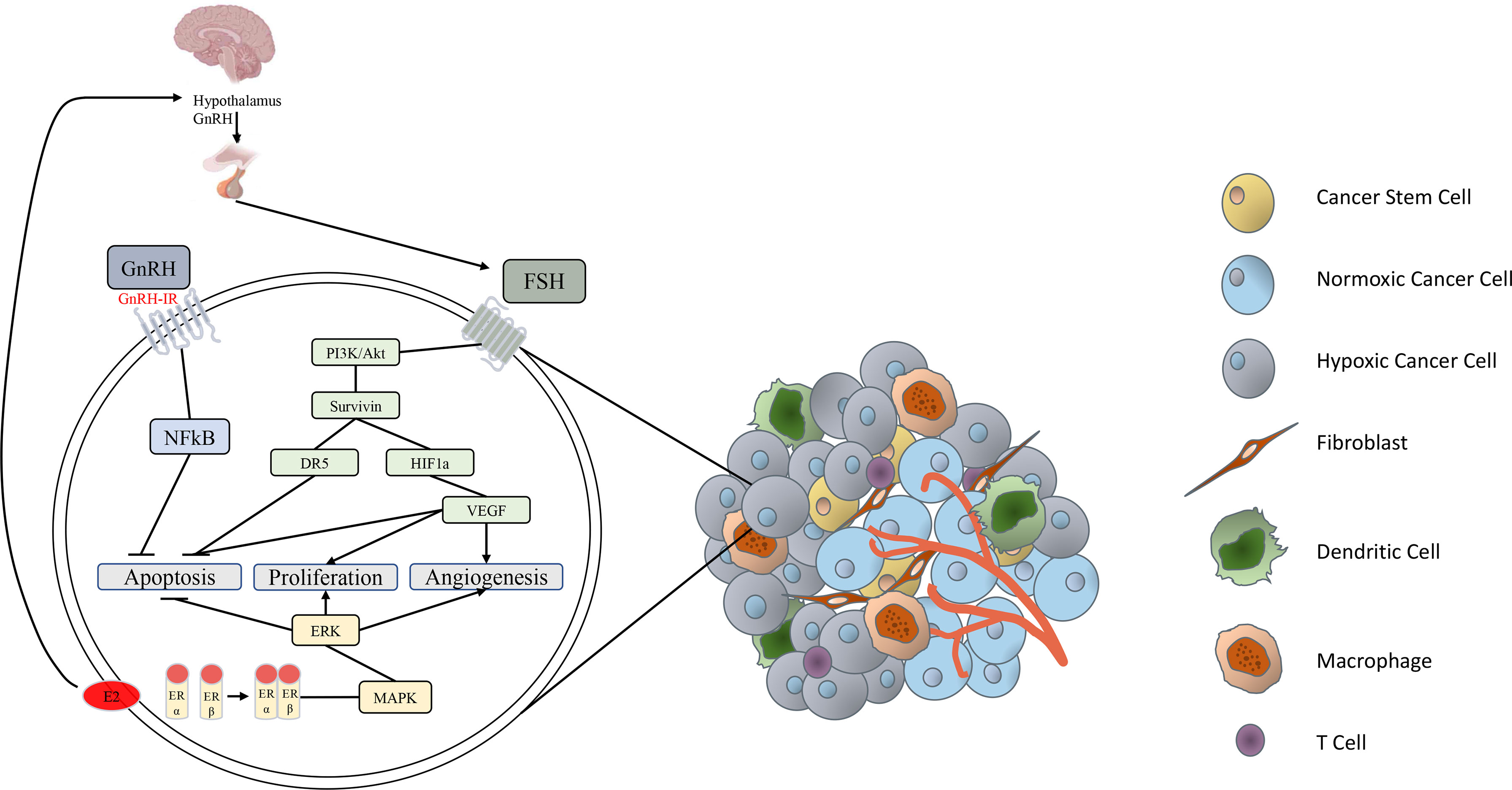
Figure 1 Simplified schematic of endocrine factors involved in epithelial ovarian cancer progression. GnRH secreted from the hypothalamus stimulates release of FSH from the anterior pituitary. FSH binds to the FSH receptor to activate the PI3K/Akt pathway, stimulating the expression of survivin (33). Survivin activates HIF-1α, resulting in secretion of VEGF, which inhibits apoptosis, stimulates proliferation and induces angiogenesis in various carcinomas (34). FSH-induced survivin upregulation is also associated with inhibition of death receptor DR5 and de-activation of an intracellular death-induced silencing complex (33). GnRH binding to the GnRH-I receptor activates intracellular NFkB, which inhibits ovarian cancer cell apoptosis (17, 35). Estrogen has direct effects by inducing activation of the proliferative, survival and angiogenic MAPK/ERK signaling pathway (36, 37). Estrogen also has an indirect pro-tumor effect by stimulating subsequent release of GnRH and FSH (38, 39).
The Role of Angiogenesis in Ovarian Cancer
Angiogenesis is a naturally occurring process to develop new blood vessels from pre-existing vasculature. Angiogenesis is involved in a number of homeostatic processes including vascular repair in wound healing (40). In response to an injury, pro-angiogenetic stimulators including vascular endothelial growth factor (VEGF), fibroblast growth factor, platelet-derived growth factors, angiopoietins, hypoxia inducible factor (HIF) and many more are activated (41–45). When the wound has been repaired, anti-angiogenic factors including thrombospondin-1, endostatin and platelet factor 4 (46–48) are activated to counteract the pro-angiogenic stimulus. This switch inhibits further angiogenesis and prevents uncontrolled vessel formation. Although angiogenesis is generally quiescent in the adult, in the ovary, cyclical angiogenesis occurs and is an important process that helps regulate ovarian function (49). Perifollicular vascularization occurs during the ovarian cycle to support development and function of the growing follicles (50). Rapid angiogenesis occurs during initial formation of the corpus luteum (51). LH and prostaglandins can specifically trigger the activation of angiogenesis following initiation of ovulatory events to accelerate capillary formation within the follicle and developing corpus luteum (52). Similarly in ovarian cancer, hormonal influences play a role in angiogenesis. Both LH and FSH levels continue to increase in menopausal women and as such promote disease progression of ovarian cancer due to their contribution in promoting tumor angiogenesis (53). In ovarian cancer, elevated levels of LH promote angiogenesis specifically through the PI3K/Akt-mTOR pathway (54). As previously mentioned, EOC primarily occurs in post-menopausal women, and as such some post-menopausal women are on HRT. Estrogen HRT is known to be a contributing risk factor to the onset of ovarian cancer. This is partially due to the fact that estrogen is known to enhance tumor growth as well as drive angiogenesis, primarily through the mediation of bone marrow-derived cells, endothelial cells and directly downregulating thrombospondin-1 expression (55, 56).
The process of angiogenesis is crucial in the development and progression of solid tumors. Without angiogenesis, tumors would not be able to grow larger than 1-2mm3 (57). By initiating angiogenesis, the tumor can stimulate the formation of blood vessels to supply oxygen and nutrients and to facilitate metabolic waste removal (58). To stimulate blood vessel formation, the tumor undergoes an “angiogenic switch” in which pro-angiogenic factors are over-expressed while angiogenesis inhibitors are concomitantly suppressed (59). Angiogenesis does not occur uniformly throughout the solid tumor and most tumors have a mosaic pattern of blood vessels (60). This lack of equal distribution of blood vessels contributes to normoxic regions within the tumor that are highly vascularized allowing oxygen to diffuse to the tumor cells. Typically following the angiogenic switch, the pro-angiogenic stimulus is aggressive, resulting in the rapid formation of tumor vessels (61). As a result of the rapid vascularization, many tumor vessels have altered morphology, with blind-ends, constrictions, shunts and other malformations (62). In addition, tumor vessels typically lack smooth muscle cell coverage and are considered immature (63). As a result of these malformations, tumor vessels are very inefficient in perfusing the tumor, resulting in widespread hypoxia (64). Hypoxic tumor cells can stimulate VEGF expression which activates neighbouring endothelial cells, further increasing angiogenesis within the TME and formation of dysfunctional tumor vessels (65). This reduced vascular perfusion represents a significant impediment to cancer therapy success as the treatment compounds cannot reach the interior of the tumor. Hypoperfusion and the resultant hypoxia is associated with the development of chemoresistance, which is a major problem in ovarian cancer patients (66, 67). Women with EOC that develop chemoresistance have demonstrated a specific angiogenic gene signature which may facilitate therapies targeted in these patients (68).
Tumor vasculature is also important in facilitating tumor metastasis (69). Due to the excessive stimulation by pro-angiogenic factors such as VEGF, tumor vessels are highly fenestrated, with increased space between endothelial cells (70). Due to the increased fenestration, tumor cells can more easily penetrate and enter the vasculature, enhancing metastasis (71). Excessively fenestrated tumor vessels also contribute to fluid extravasation and accumulation in the perivascular space which increases interstitial fluid pressure (IFP) (72). Elevated IFP creates an additional barrier to therapy uptake due to the elevated pressures within the tumor (73). High IFP can also cause the collapse of intratumoral lymphatic vessels, which can obstruct proper lymphatic drainage (74). A combination of hyperpermeable vasculature and lack of lymphatic drainage seen in EOC results in leakage in to the peritoneum through an osmotic effect (75). This contributes to the accumulation of ascites in the abdomen, and the release of tumor cells into the ascites increases metastasis (76).
Hypoxia as a Feature of Ovarian Cancer Progression
As tumors grow and become less oxygenated and hypoxic, cancer cells develop mechanisms to survive under lower oxygen tension (77). The immediate molecular response to low oxygen is the stabilization of HIFs. HIFs in turn, will activate a number of survival pathways that promote proliferation, angiogenesis and invasion while concurrently inhibiting apoptotic cancer cell death (78, 79). In opposition to this, normal, non-cancerous cells typically respond to hypoxia by undergoing senescence, arresting mitosis and dying by apoptosis if there is DNA damage (80, 81).
Hypoxia is a key inducer of angiogenesis and an activator of the angiogenic switch during tumor development (82). This event is a tip in the balance of pro-angiogenic and anti-angiogenic factors within the TME in response to stimuli, favouring a transition from angiogenic dormancy to a vascularized tumor. HIF-1 is a heterodimer, consisting of a constitutively expressed HIF-1β subunit and a regulatory HIF-1α subunit (83). In response to tissue hypoxia, HIF-1α stabilizes, accumulates and translocates to the nucleus. Once translocated to the nucleus, HIF-1α binds to conserved hypoxia response elements to activate hypoxia-sensitive genes such as VEGF by binding to their promoter regions (84). Culture of ovarian cancer cells under hypoxic conditions results in a concomitant increase in expression of HIF-1α and VEGF (85) and inhibition of HIF-1α results in a significant decrease in VEGF production and tumor angiogenesis (86). HIF-1α also targets and upregulates the stanniocalcin (STC) gene, known for being an anti-hypercalcemic glycoprotein hormone, although not much research has been focused on STC in ovarian cancer tumorigenesis (87). STC1 overexpression has been linked with increased proliferation, migration and colony formation in human ovarian cancer cell lines as well as increased expression of cell cycle regulation proteins and increased expression of anti-apoptotic proteins, hindering apoptosis (88). Under hypoxic conditions, HIF-1α regulates increased STC2 expression to facilitate increased ovarian cancer tumor cell proliferation (89). Both STC1 and STC2 promote epithelial to mesenchymal transition in hypoxic ovarian cancer cells and contribute to their invasion and metastasis as well (90, 91). Additionally, estrogen and progestin can directly regulate HIF-1α through the PI3K/Akt-mTOR signaling pathway and contribute to tumor metastasis in ovarian cancer (92). In EOC, HIF-1α expression is upregulated and its increased expression is linked to poor survival (93). HIF-2α on the other hand dimerizes with HIF-1β and binds to hypoxia response elements similarly to HIF-1α. In EOC, women with advanced disease, either stage 3 or 4, have a specific HIF profile characteristic of elevated nuclear expression of HIF-1α and elevated cytoplasmic HIF-2α expression, and this specific profile is associated with a poor prognostic outcome (94).
Several hypoxia-mediated changes within the TME may explain the link between poor prognosis and HIF-1α expression. Hypoxia contributes to the selection and activation of an ovarian cancer stem cell niche and cancer stem cells (CSCs) appear to favour hypoxic sites within tumors (95). CSCs are undifferentiated, or less-differentiated cells that drive tumorigenesis and give rise to the large population of cells that comprise majority of the tumor (96). CSCs can create a significant therapeutic challenge as they can evade and resist chemotherapy due to their stem cell qualities. CSCs are quiescent, or very slowly cycling, which renders them resistant to therapies that target rapidly dividing cells (97). CSCs also upregulate survival signaling pathways, making them harder to kill with conventional cytotoxic therapy (98). CSCs act as a cell reservoir, and can be a major contributor to cancer recurrence (99), including that seen in ovarian cancer (100). It has been suggested that the correlation of poor patient outcome with hypoxia may be related to the enhanced presence of CSCs (101). The Notch, Wnt and Hedgehog pathways are important in the maintenance, self-renewal and resistance of CSCs (102, 103). In ovarian cancer, HIF-1α activates Notch1 signaling, which increases the activity of the Sox2 promoter, creating a CSC phenotype and drives drug resistance in ovarian cancer stem cells (104). In ovarian cancer cells, exposure to hypoxia increases expression of CD44, CD133, Oct3/4 and Sox2, which are known markers of ovarian CSCs (105). The other isoform of HIF, HIF-2α, also targets Notch, Oct4 and Sox2 (106), suggesting that HIF-2α is also important in maintaining stemness in the hypoxic TME. HIF-1α also upregulates the expression of Sirtuin type 1, which is known to promote CSC-like features in ovarian cancer cells (107).
A unique feature of peritoneal tumors, including ovarian cancer, is the presence of ascites fluid. This complex mixture of soluble factors accumulates due to leaky tumor vasculature as well as disrupted lymphatic patency (108). Given that ascites worsens tumor access to oxygen, ascites accumulation promotes the negative responses of the tumor to low oxygen (109). In addition, the flow of ascites fluid current dictates the direction of EOC secondary tumor dissemination within the abdominal cavity (108). Malignant ascites also contains factors which induce immunosuppression and enhance survival – creating an ideal environment for tumor dissemination to other abdominal organs by evading the immune system.
Hypoxia Alters the Immune Environment in Ovarian Cancer
The tumor immune microenvironment (TIME), which encompasses not only malignant transformed cells, but also normal cells such as epithelial cells, fibroblasts, endothelial cells, muscle cells and immune cells in EOC has been described as ‘highly permissive’ to tumor growth, metastasis and therapy resistance (110–112). While tumor subtypes such as melanoma and lung cancer present with high levels of interferon and T cell infiltrates and are thus excellent candidates for immunotherapy, the TIME in ovarian tumors contains a distinct suppressive phenotype populated by immature myeloid cells, anergic T cells and T regulatory (Treg) cells (113). As previously discussed, ascites fluid is rich in immunosuppressive cytokines and therefore drives production of these suppressive cells and acts as an ideal conduit in the spread of tumor nodules to other organs (114). Ascites also contains significantly higher proportions of T cells expressing checkpoints such as LAG‐3+, PD‐1+, TIM+ and CTLA‐4+ compared to peripheral blood (115).
Low oxygen partial pressure within the tumor activates hypoxia-dependent signaling, where HIFs are stabilized. HIF-1 maintains elevated myeloid-derived suppressor cell levels and regulates their function and maturation (116). Myeloid-derived suppressor cells in turn lead to the production of immunosuppressive cytokines, of which TGF-β, IL-6 and IL-8 contribute to high immunosuppression in advanced ovarian cancer (117). Hypoxic areas also attract and polarize M2 type tumor-associated macrophages (118). The function of this subtype of macrophages is to promote tissue repair, which involves immune tolerance and modulation (119). M2 macrophages are present in higher proportions in advanced stages of ovarian cancer compared to early stages of the disease (120), indicating that they may be linked to disease progression. In addition, a higher M2/M1 tumor-associated macrophage ratio is an indicator of positive prognosis in EOC and has been reliable in predicting patient survival time in other cancers (121). Hypoxia also upregulates CCL28, a chemokine for Treg cells, in a HIF-dependent manner (122). Tregs in turn challenge anti-tumor immune responses by downregulating effector T cells (123). A high CD8+/Treg ratio is a significant predictor of prognosis in ovarian cancer (124). As the most potent antigen-presenting cell, dendritic cells (DCs) play a major role in tumor immunosurveillance. Prolonged exposure of DCs to hypoxia leads to cell death of these antigen presenting cells – a process that can be prevented by inhibiting HIF-1α (125). Additionally, expression of HIF-1α diminishes the ability of DCs to produce IL-12 – an important cytokine for the development of cytotoxic T cells (126). STC1 has been shown to interact with and decrease membrane exposure of calreticulin, impairing phagocytic responses of antigen presenting cells, including DCs and macrophages, to ultimately inhibit antigen presentation to facilitate T cell activation (127). Additionally, STC1 inhibits macrophage infiltration, further hindering an immune response against the tumor (128). Altogether, while malignant cells thrive in the absence of oxygen, immune cells which would ideally produce an anti-tumor response against tumor associated antigens, frequently become anergic or die in response to this environment (129). This system imbalance creates a pro-tumorigenic environment and hinders patient response to immunotherapies.
The fundamental basis of successful immunotherapy to treat cancer is positive immunogenicity of the specific tumor subtype, which considers presence of tumor associated antigens and effective presentation of these antigens. The immunosuppressive TIME as well as low mutation rate, which impairs neo-antigen formation, challenges the use of immune-based therapies in ovarian cancer (130–132). Findings have demonstrated that ovarian tumors are typically “cold” meaning that they lack cytotoxic T cell infiltration. Goode et al. demonstrated a distinct dose-response relationship between the number of CD8+ infiltrates and patient survival time in high-grade serous ovarian cancer (133). The prognostic impact of tumor infiltrating lymphocytes (TILs) trafficking suggests that the factors which regulate TIL infiltration are vital when improving current therapies or seeking new targets. In EOC, T cells face physical barriers such as vascular access, which impede their access to tumor cells. Abnormal vasculature within EOC tumors leads to downstream hypoxia, which has been named a common biological determinant of immune suppression in solid tumors (134). Indeed, mono-immunotherapy in ovarian cancer has yielded modest results (135, 136). Battaglia et al. found that assessment of immune status prior to treatment with a CA-125 targeted monoclonal antibody may predict treatment sensitivity (137). EOC patients vary with respect to levels of TILs and individual tumor samples have pronounced heterogeneity in immune profile (138, 139). In light of these findings, more recent studies into EOC immunotherapy focus on personalized and combination strategies. The anti-angiogenic compound Avastin has shown promising results in combination with immunotherapy in light of reducing molecules such as VEGF, thereby enhancing inflammation. Between patients, previous exposure to chemotherapy, disease histotype and ascites profile are each indicators of immune therapy success (133, 140, 141). These findings illustrate that immunotherapy may be a successful treatment strategy for EOC in the future, although the tumor microenvironment must be considered in therapy design.
The Impact of Hypoxia on Therapy Resistance
Hypoxia has been associated with the development of chemoresistance through a variety of mechanisms. One of the mechanisms by which hypoxia induces chemoresistance is through alteration of cancer cell metabolism. In response to hypoxia, ovarian cancer cells undergo a metabolic switch, with changes in the glycolytic pathway that promotes resistance to carboplatin (142–144). As ovarian tumors become hypoxic, there is an upregulation of glycolytic enzymes to metabolize glucose, resulting in the formation of lactate (145). The reduction in pH from lactate accumulation inhibits the efficacy of chemotherapy agents (144). HIF-1α appears to be a central regulator of cellular metabolism. HIF-1α regulates many of the enzymes involved in glucose catabolism and regulates lactate production through activation of lactate dehydrogenase A and the lactate transporter MCT4 (146). As HIF-1α drives cells toward anaerobic glycolysis, it supports the metabolic switch seen in ovarian cancer cells. Inhibition of HIF-1α is a strategy that has been employed to redirect cells to oxidative phosphorylation, resulting in the production of cytotoxic levels of reactive oxygen species and cancer cell apoptosis (147). Specifically targeting HIF-1α through antisense as an anti-cancer strategy has shown efficacy in xenograft models of ovarian cancer (148). HIF-1α also contributes to the development of platinum resistance through induction of cancer cell autophagy (149). Autophagy is a process that assists in maintaining cell viability during times of stress. Under stressful conditions, autophagy is rapidly activated to reduce cellular growth and increase catabolic lysis of unnecessary proteins and organelles. However, persistent or excessive autophagy can lead to the induction of cell death (150). Activation of autophagy in ovarian cancer is associated with the development of chemoresistance through the induction of the MAPK/ERK survival pathways (151). HIF-1α initiates autophagy in ovarian cancer cells exposed to hypoxia, and these cells develop resistance to cisplatin-induced apoptosis (149). In the challenging, hypoxic and nutrient-poor TME, autophagy may be an important mechanism employed by tumor cells to survive and develop chemotherapy resistance. HIF-1α has also been found to directly induce expression of the STC1 gene, producing STC in human ovarian cancer cell lines (152). STC has been shown to regulate a number of oncogenic effects in different tumor subtypes such as triggering angiogenesis through upregulation of VEGF in gastric cancer (153), as well as exacerbating chemoresistance, invasion and metastasis in breast cancer (154–156). Although the mechanistic details behind STC in ovarian cancer have not been heavily studied, its expression is highest in the ovaries, particularly during pregnancy and lactation (157). In addition, dysregulated levels of STC have been linked to poor outcome in patients, lending merit to further investigations into its functions (88).
Hypoxia also regulates the expression and function of a multitude of microRNAs (miRNAs). MiRNAs are small, approximately 19-25 nucleotides endogenous non-coding RNAs that regulate gene expression (158) in humans, as well as a wide array of organisms (159, 160). MiRNAs have been implicated in the onset, progression and therapy resistance in ovarian cancer (161). Hypoxia is known to regulate the expression of several miRNAs in ovarian cancer. Hypoxia in ovarian cancer is associated with altered levels of circulating miRNAs and these expression profiles are associated with the risk of developing ovarian cancer. In one study, 36 miRNAs were overexpressed, and 101 miRNAs were downregulated in women at high-risk of ovarian cancer, compared to those with low risk (162). HIF-1α hypoxia-induced overexpression of miR-210 in EOC has been shown to promote proliferation and migration while inhibiting apoptosis of EOC in vitro (163, 164). Interestingly, deletion of miR-210 is also associated with dysregulated cell cycle and progression of ovarian cancer (165), suggesting that miRNA effects may be context-specific. In addition to regulating tumorigenic processes, hypoxia-induced miRNA expression has been implicated in the acquisition of drug resistance in ovarian cancer. Under hypoxic conditions, the HIF-1α pathway increases expression of miR-27a and is associated with the development of paclitaxel resistance through downregulation of the apoptosis-related protein APAF1 (166). Hypoxia has also been implicated in drug resistance in patients with high HIF-1α expression through upregulation of expression of miR-223 and activation of the PTEN-PI3K/Akt-mTOR pathway, resulting in multi-drug resistance and disease recurrence (167). MiRNA expression in response to hypoxia in EOC appears to regulate numerous important tumorigenic processes and response to therapy, although the mechanisms involved are still largely unclear.
Reversal of Hypoxia as an Approach to Personalized Therapy
HIF-1 expression alone is not an indicative prognostic marker onRESA the disease progression in ovarian cancer, however, overexpression of HIF-1 in combination with the presence of non-functional p53 is associated with a more aggressive phenotype and a poorer prognosis (168). A gain-of-function mutation of the p53 gene is characteristic of the most common subtype of EOC, high-grade serous ovarian cancer (169), therefore ovarian cancer patients with a high HIF-1 expression profile may benefit from therapies directly targeting HIF-1. There are many novel therapeutic agents targeting HIF-1 activity that are currently in clinical trials. These therapeutic agents are designed to directly inhibit HIF-1 activity through a multitude of various targets including mTOR (170), COX2 (171), epidermal growth factor receptor EGFR (172), heat shock protein 90 (173), topoisomerase I (174) or at the post-transcriptional level (175). Herceptin is a monoclonal antibody that specifically targets human epidermal growth factor receptor 2 (HER2) and is currently an FDA approved therapy for the treatment of early and late stage breast cancer patients with HER2 overexpression (176). The mechanisms of action of Herceptin are not fully understood, however, many outcomes of treatment with Herceptin have been observed. Herceptin prevents HER2 and Src tyrosine kinase from clustering, inhibiting activation of PI3K/Akt-mTOR and MAPK/ERK signaling pathways (177). Herceptin also induces tumor cell apoptosis and cell cycle G1 arrest (178, 179). Overexpression of HER2 in tumor cells is known to be affiliated with increased VEGF expression and increased angiogenesis (180). Herceptin decreases expression of pro-angiogenic factors and subsequently increases expression of anti-angiogenic factors (181) as well as reduces endothelial cell migration (182). In ovarian cancer, HER2 is expressed in up to 66% of EOC cases and is associated with a poorer prognosis (183, 184). While not much research has been focused on the use of Herceptin in treating ovarian cancer, there may be a subset of ovarian cancer patients with HER2 overexpression that may benefit from Herceptin incorporated into their treatment plan, but this needs to be explored in greater detail. Alternatively, targeting downstream effects of HIF-1α are also potential therapeutic avenues. STC1 and STC2 are upregulated and promote tumorigenesis and disease progression in a HIF dependent manner as previously discussed and may be a future target of interest. Recent studies targeting STC1 with a vector expressing a suicide gene under a STC promoter inhibited and arrested cell growth in lung cancer cell lines (185) although further research is still required before its potential clinical use.
Hypoxia-activated prodrugs were designed to specifically target the hypoxic tumor cells within a solid tumor. Their mechanism of action work by their enzymatic reduction to reactive oxygen species under hypoxic conditions, with capacity to re-oxidize in oxygenated environments. In pre-clinical and clinical settings, hypoxia activated prodrugs have shown promising efficacy in successfully attacking hypoxic cells of solid tumors (186–188). This approach has been more efficacious when combined with chemotherapy and radiotherapy (189, 190), as well as anti-angiogenic therapies (191–193). Many solid tumor cancers, including EOC, are known to have overexpressed folate receptors on the tumor cell surfaces (194). Folate-based redox-responsive nanoparticles (NPs) and designed to target and bind to the folate receptor with high affinity. NPs are capable of releasing therapies solely in the presence of hypoxia due to their design of being cleaved only in the absence of oxygen (195). Folate acid-tagged NPs can be loaded with cancer therapies, such as chemotherapy and immunotherapy and rapidly release these drugs under hypoxic conditions compared to normoxic regions (195–198). Anti-angiogenic application of NPs are also being employed through use of gold, silver and silicate-based NPs. Their mechanism of action work to inhibit VEGF and other pro-angiogenic factors as well as induce production of reactive oxygen species causing vessel constriction and thereby inhibiting proliferation and migration of endothelial cells, which ultimately halts tumor cell growth (199–202).
Anti-angiogenic therapies can normalize tumor vasculature, uniformly increase oxygen delivery and reverse the hypoxic areas of the tumor. This allows for better uptake and distribution of therapeutic drugs to the tumor (203). Many anti-angiogenic therapies work by inhibiting VEGF, either by directly binding to VEGF or inhibiting the VEGF receptor. By inhibiting only angiogenesis, these therapies do not interfere with normal pre-existing vasculature in patients. The anti-angiogenic therapy Avastin is an FDA approved monoclonal antibody used in combination with chemotherapy primarily when recurrence of EOC occurs (204). This includes patients that are both platinum-sensitive and platinum-resistant (205). Avastin works by binding to VEGF with high specificity and therefore prevents it from binding to the VEGF receptor. Combination of Avastin with chemotherapy at the first onset of platinum resistance in ovarian cancer patients demonstrates the greatest outcome for overall patient survival, compared to Avastin or chemotherapy alone (206). Additionally, anti-angiogenic therapies when combined with NPs in platinum resistant patients are also being investigated for clinical safety and their potential efficacy (207). Other anti-angiogenic therapies targeting the VEGF receptor show similar efficacy, especially when used in combination with other cancer therapies, including chemotherapy (208, 209) and immune checkpoint inhibitors (210). More recently, the combination of Avastin and the poly (ADP-ribose) polymerase (PARP) inhibitor, olaparib, have been FDA approved for combination use in patients who responded to chemotherapy or in patients that carry a BRCA1 or BRCA2 mutation (211). Due to the nature of its use, rapid tumor resistance to Avastin often occurs due to upregulation and reliance on pathways other than VEGF by the tumor (212, 213). As such other new anti-angiogenic therapies are being developed to overcome this issue and demonstrate promising potential. Treatment with 3TSR, a novel compound derived from the anti-angiogenic regions of thrombospondin-1, has demonstrated its ability to reduce primary ovarian tumor size, occurrence of metastatic tumors and decrease abdominal ascites accumulation in a murine EOC model when used in combination with chemotherapy (214) and oncolytic viruses (215). Neferine, a bisbenzylisoquinoline alkaloid derivative from lotus seed embryos, demonstrates anti-angiogenic properties in chemoresistant EOC by inducing autophagy and inhibiting macrophage maturation (216).
Estrogen receptors are specifically located on smooth muscle pericytes within blood vessels and estrogen is known to inhibit proliferation of vascular smooth muscle cells (217, 218). Additionally, estrogen is known to be anti-inflammatory and vasoprotective in young women compared to its pro-inflammatory and vasotoxic effects in older women, partially due to altered estrogen signaling pathways with age (219). Tamoxifen, a selective estrogen receptor modulator hormonal therapy, is known to exhibit its anti-angiogenic properties by inhibiting platelet activation (220). Although this hasn’t been studied much in relation to cancer, the presence of estrogen receptors on pericytes and the hormonal status of a woman (menopausal with or without HRT) may result in some patients being less responsive to anti-angiogenic vessel normalization therapies. The addition of HRT may aid in increased efficacy of anti-angiogenic therapies, and this should be taken into consideration when designing a treatment plan for patients with hormone-dependent cancers.
Reversing hypoxia within the TME will not directly contribute to tumor cell death, however, we can utilize this as an advantage to create more personalized combinational therapeutic approaches. The presence of hypoxia and poor tumor vasculature is characteristic to many solid tumors including EOC (221). In light of this, women with ovarian cancer may benefit from initial therapies that normalize the tumor vasculature and reverse a hypoxia followed by administration of a more specific therapy, such as chemotherapy, a PARP inhibitor or oncolytic virus, based on a patient-by-patient basis. By normalizing the tumor vasculature first, we may be able to increase uptake of these therapies by the tumor, have better distribution of therapies throughout the tumor cells and ultimately reduce chemoresistance and increase apoptotic cell death of the entire tumor. This will ultimately lead to more efficacious treatments and increased patient survival.
Conclusion
Hypoxia-induced changes in the endocrine environment and immune status of the tumor play an influential role in promoting tumorigenesis in ovarian cancer. By understanding the changes that occur in the ovarian TME, we can develop novel therapies that target these changes to improve efficacy and reduce therapy resistance. An approach to personalized treatment strategies on a patient-by-patient basis may ultimately improve the way we treat women with ovarian cancer.
Author Contributions
The article was conceptualized by JP. MP, KM, CJ, and JP contributed to the writing and editing of the review. All authors contributed to the article and approved the submitted version.
Conflict of Interest
The authors declare that the research was conducted in the absence of any commercial or financial relationships that could be construed as a potential conflict of interest.
Publisher’s Note
All claims expressed in this article are solely those of the authors and do not necessarily represent those of their affiliated organizations, or those of the publisher, the editors and the reviewers. Any product that may be evaluated in this article, or claim that may be made by its manufacturer, is not guaranteed or endorsed by the publisher.
Abbreviations
CSC, Cancer Stem Cell; DC, Dendritic Cell; EOC, Epithelial Ovarian Cancer; FSH, Follicle Stimulating Hormone; GnRH, Gonadotropin-Releasing Hormone; hCG, Human Chorionic Gonadotrophin; HER2, Human Epidermal Growth Factor Receptor 2; HIF, Hypoxia Inducible Factor; HRT, Hormone Replacement Therapy; LH, Luteinizing Hormone; NP, Nanoparticle; OSE, Ovarian Surface Epithelium; PARP, Poly (ADP-ribose) Polymerase; STC, Stanniocalcin; TIME, Tumor Immune Microenvironment; TME, Tumor Microenvironment; Treg, T Regulatory Cell; VEGF, Vascular Endothelial Growth Factor.
References
1. Bray F, Ferlay J, Soerjomataram I, Siegel RL, Torre LA, Jemal A. Global Cancer Statistics 2018: GLOBOCAN Estimates of Incidence and Mortality Worldwide for 36 Cancers in 185 Countries. CA Cancer J Clin (2018) 68:394–424. doi: 10.3322/caac.21492
2. Badgwell D, Bast RC. Early Detection of Ovarian Cancer. Dis Markers (2007) 23:397–410. doi: 10.1155/2007/309382
3. Jacobs IJ, Menon U. Progress and Challenges in Screening for Early Detection of Ovarian Cancer. Mol Cell Proteomics (2004) 3:355–66. doi: 10.1074/mcp.R400006-MCP200
4. Torre LA, Trabert B, DeSantis CE, Miller KD, Samimi G, Runowicz CD, et al. Ovarian Cancer Statistics, 2018. CA Cancer J Clin (2018) 68:284–96. doi: 10.3322/caac.21456
5. Rizzuto I, Behrens RF, Smith LA. Risk of Ovarian Cancer in Women Treated With Ovarian Stimulating Drugs for Infertility. Cochrane Database Syst Rev (2019). doi: 10.1002/14651858.cd008215.pub3
6. Yarmolinsky J, Relton CL, Lophatananon A, Muir K, Menon U, Gentry-Maharaj A, et al. Appraising the Role of Previously Reported Risk Factors in Epithelial Ovarian Cancer Risk: A Mendelian Randomization Analysis. PloS Med (2019) 16:e1002893. doi: 10.1371/journal.pmed.1002893
7. Shafrir AL, Babic A, Gates Kuliszewski M, Rice MS, Townsend MK, Hecht JL, et al. Estrogen Receptor-β Expression of Ovarian Tumors and Its Association With Ovarian Cancer Risk Factors. Cancer Epidemiol Biomarkers Prev (2020) 29:2211–9. doi: 10.1158/1055-9965.EPI-20-0618
8. Qian F, Rookus MA, Leslie G, Risch HA, Greene MH, Aalfs CM, et al. Mendelian Randomisation Study of Height and Body Mass Index as Modifiers of Ovarian Cancer Risk in 22,588 BRCA1 and BRCA2 Mutation Carriers. Br J Cancer (2019) 121:180–92. doi: 10.1038/s41416-019-0492-8
9. Orr B, Edwards RP. Diagnosis and Treatment of Ovarian Cancer. Hematol Oncol Clin North Am (2018) 32:943–64. doi: 10.1016/j.hoc.2018.07.010
10. Aleksandra Kujawa K, Lisowska KM. Ovarian Cancer – From Biology to Clinic. Postepy Hig Med Dosw (2015) 69:1275–90. doi: 10.5604/17322693.1184451
11. Auersperg N, Wong AST, Choi K-C, Kang SK, Leung PCK. Ovarian Surface Epithelium: Biology, Endocrinology, and Pathology*. Endocr Rev (2001) 22:255–88. doi: 10.1210/edrv.22.2.0422
12. Lukanova A, Kaaks R. Endogenous Hormones and Ovarian Cancer: Epidemiology and Current Hypotheses. Cancer Epidemiol Biomarkers Prev (2005) 14:98–107.
13. Stewart SL, Querec TD, Ochman AR, Gruver BN, Bao R, Babb JS, et al. Characterization of a Carcinogenesis Rat Model of Ovarian Preneoplasia and Neoplasia. Cancer Res (2004) 64:8177–83. doi: 10.1158/0008-5472.CAN-04-1702
14. Zhang Z, Jia L, Feng Y, Zheng W. Overexpression of Follicle-Stimulating Hormone Receptor Facilitates the Development of Ovarian Epithelial Cancer. Cancer Lett (2009) 278:56–64. doi: 10.1016/j.canlet.2008.12.024
15. Lenhard M, Tsvilina A, Schumacher L, Kupka M, Ditsch N, Mayr D, et al. Human Chorionic Gonadotropin and Its Relation to Grade, Stage and Patient Survival in Ovarian Cancer. BMC Cancer (2012) 12:2. doi: 10.1186/1471-2407-12-2
16. Cheung LW, Yung S, Chan TM, Leung PC, Wong AS. Targeting Gonadotropin-Releasing Hormone Receptor Inhibits the Early Step of Ovarian Cancer Metastasis by Modulating Tumor-Mesothelial Adhesion. Mol Ther (2013) 21:78–90. doi: 10.1038/mt.2012.187
17. Gründker C, Emons G. Role of Gonadotropin-Releasing Hormone (GnRH) in Ovarian Cancer. Reprod Biol Endocrinol (2003) 1:65. doi: 10.1186/1477-7827-1-65
18. Walentowicz P, Krintus M, Sadlecki P, Grabiec M, Mankowska-Cyl A, Sokup A, et al. Serum Inhibin A and Inhibin B Levels in Epithelial Ovarian Cancer Patients. PloS One (2014) 9:e90575. doi: 10.1371/journal.pone.0090575
19. McNeilly AS. Diagnostic Applications for Inhibin and Activins. Mol Cell Endocrinol (2012) 359:121–5. doi: 10.1016/j.mce.2011.06.017
20. Marino FE, Risbridger G, Gold E. The Inhibin/Activin Signalling Pathway in Human Gonadal and Adrenal Cancers. Mol Hum Reprod (2014) 20:1223–37. doi: 10.1093/molehr/gau074
21. Modugno F, Laskey R, Smith AL, Andersen CL, Haluska P, Oesterreich S. Hormone Response in Ovarian Cancer: Time to Reconsider as a Clinical Target? Endocr Relat Cancer (2012) 19:R255–79. doi: 10.1530/ERC-12-0175
22. Permuth-Wey J, Sellers TA. Epidemiology of Ovarian Cancer. Methods Mol Biol (2009) 472:413–37. doi: 10.1007/978-1-60327-492-0_20
23. Bosetti C, Negri E, Trichopoulos D, Franceschi S, Beral V, Tzonou A, et al. Vecchia C La. Long-Term Effects of Oral Contraceptives on Ovarian Cancer Risk. Int J Cancer (2002) 102:262–5. doi: 10.1002/ijc.10696
24. Han KH, Kim M-K, Kim HS, Chung HH, Song YS. Protective Effect of Progesterone During Pregnancy Against Ovarian Cancer. J Cancer Prev (2013) 18:113–22. doi: 10.15430/jcp.2013.18.2.113
25. Kim O, Park EY, Kwon SY, Shin S, Emerson RE, Shin YH, et al. Targeting Progesterone Signaling Prevents Metastatic Ovarian Cancer. Proc Natl Acad Sci USA (2020) 117:31993–2004. doi: 10.1073/pnas.2013595117
26. Peluso JJ, Liu X, Saunders MM, Claffey KP, Phoenix K. Regulation of Ovarian Cancer Cell Viability and Sensitivity to Cisplatin by Progesterone Receptor Membrane Component-1. J Clin Endocrinol Metab (2008) 93:1592–9. doi: 10.1210/jc.2007-2771
27. Cunat S, Hoffmann P, Pujol P. Estrogens and Epithelial Ovarian Cancer. Gynecol Oncol (2004) 94:25–32. doi: 10.1016/j.ygyno.2004.03.026
28. Ataei N, Aghaei M, Panjehpour M. Evidences for Involvement of Estrogen Receptor Induced ERK1/2 Activation in Ovarian Cancer Cell Proliferation by Cadmium Chloride. Toxicol Vitr (2019) 56:184–93. doi: 10.1016/j.tiv.2019.01.015
29. Pellegrini C, Gori I, Achtari C, Hornung D, Chardonnens E, Wunder D, et al. The Expression of Estrogen Receptors as Well as GREB1, C-MYC, and Cyclin D1, Estrogen-Regulated Genes Implicated in Proliferation, Is Increased in Peritoneal Endometriosis. Fertil Steril (2012) 98:1200–08. doi: 10.1016/j.fertnstert.2012.06.056
30. Cottreau CM, Ness RB, Modugno F, Allen GO, Goodman MT. Endometriosis and Its Treatment With Danazol or Lupron in Relation to Ovarian Cancer. Clin Cancer Res (2003) 9:5142–4.
31. Evangelou A, Jindal SK, Brown TJ, Letarte M. Down-Regulation of Transforming Growth Factor β Receptors by Androgen in Ovarian Cancer Cells. Cancer Res (2000) 60:929–35.
32. Syed V, Ulinski G, Mok SC, Ho SM. Reproductive Hormone-Induced, STAT3-Mediated Interleukin 6 Action in Normal and Malignant Human Ovarian Surface Epithelial Cells. J Natl Cancer Inst (2002) 94:617–29. doi: 10.1093/jnci/94.8.617
33. Huang Y, Jin H, Liu Y, Zhou J, Ding J, Cheng KW, et al. FSH Inhibits Ovarian Cancer Cell Apoptosis by Up-Regulating Survivin and Down-Regulating PDCD6 and DR5. Endocr Relat Cancer (2011) 18:13–26. doi: 10.1677/ERC-09-0308
34. Tian QG, Wu YT, Liu Y, Zhang J, Song ZQ, Gao WF, et al. Expressions and Correlation Analysis of HIF-1α, Survivin and VEGF in Patients With Hepatocarcinoma. Eur Rev Med Pharmacol Sci (2018) 22:3378–85. doi: 10.26355/EURREV_201806_15159
35. Meyer C, Sims AH, Morgan K, Harrison B, Muir M, Bai J, et al. Transcript and Protein Profiling Identifies Signaling, Growth Arrest, Apoptosis, and NF-κb Survival Signatures Following GNRH Receptor Activation. Endocr Relat Cancer (2013) 20:123–36. doi: 10.1530/ERC-12-0192
36. Zhang G, Cheng Y, Zhang Q, Li X, Zhou J, Wang J, et al. ATX-LPA Axis Facilitates Estrogen-Induced Endometrial Cancer Cell Proliferation via MAPK/ERK Signaling Pathway. Mol Med Rep (2018) 17:4245–52. doi: 10.3892/mmr.2018.8392
37. Wang T, Jin J, Qian C, Lou J, Lin J, Xu A, et al. Estrogen/ER in Anti-Tumor Immunity Regulation to Tumor Cell and Tumor Microenvironment. Cancer Cell Int (2021) 21:295. doi: 10.1186/s12935-021-02003-w
38. Fan X, Gabbi C, Kim HJ, Cheng G, Andersson LC, Warner M, et al. Gonadotropin-Positive Pituitary Tumors Accompanied by Ovarian Tumors in Aging Female Erβ-/- Mice. Proc Natl Acad Sci USA (2010) 107:6453–8. doi: 10.1073/pnas.1002029107
39. Chen FCK, Oskay-Özcelik G, Bühling KJ, Köpstein U, Mentze M, Lichtenegger W, et al. Prognostic Value of Serum and Ascites Levels of Estradiol, FSH, LH and Prolactin in Ovarian Cancer. Anticancer Res (2009) 29:1575–8.
40. Greaves NS, Ashcroft KJ, Baguneid M, Bayat A. Current Understanding of Molecular and Cellular Mechanisms in Fibroplasia and Angiogenesis During Acute Wound Healing. J Dermatol Sci (2013) 72:206–17. doi: 10.1016/j.jdermsci.2013.07.008
41. Shibuya M. Vascular Endothelial Growth Factor (VEGF) and Its Receptor (VEGFR) Signaling in Angiogenesis: A Crucial Target for Anti- and Pro-Angiogenic Therapies. Genes Cancer (2011) 2:206–17. doi: 10.1177/1947601911423031
42. Cross MJ, Claesson-Welsh L. FGF and VEGF Function in Angiogenesis: Signalling Pathways, Biological Responses and Therapeutic Inhibition. Trends Pharmacol Sci (2001) 22:201–7. doi: 10.1016/S0165-6147(00)01676-X
43. Raica M, Cimpean AM. Platelet-Derived Growth Factor (PDGF)/PDGF Receptors (PDGFR) Axis as Target for Antitumor and Antiangiogenic Therapy. Pharmaceuticals (2010) 3:572–99. doi: 10.3390/ph3030572
44. Fagiani E, Christofori G. Angiopoietins in Angiogenesis. Cancer Lett (2013) 328:18–26. doi: 10.1016/j.canlet.2012.08.018
45. Shi YH, Fang WG. Hypoxia-Inducible Factor-1 in Tumour Angiogenesis. World J Gastroenterol (2004) 10:1082–7. doi: 10.3748/wjg.v10.i8.1082
46. Miao X, Rahman MFU, Jiang L, Min Y, Tan S, Xie H, et al. Thrombin-Reduced miR-27b Attenuates Platelet Angiogenic Activities In Vitro via Enhancing Platelet Synthesis of Anti-Angiogenic Thrombospondin-1. J Thromb Haemost (2018) 16:791–801. doi: 10.1111/jth.13978
47. Walia A, Yang JF, Huang YH, Rosenblatt MI, Chang JH, Azar DT. Endostatin’s Emerging Roles in Angiogenesis, Lymphangiogenesis, Disease, and Clinical Applications. Biochim Biophys Acta - Gen Subj (2015) 1850:2422–38. doi: 10.1016/j.bbagen.2015.09.007
48. Lord MS, Cheng B, Farrugia BL, McCarthy S, Whitelock JM. Platelet Factor 4 Binds to Vascular Proteoglycans and Controls Both Growth Factor Activities and Platelet Activation. J Biol Chem (2017) 292:4054–63. doi: 10.1074/jbc.M116.760660
49. Fraser HM, Lunn SF. Angiogenesis and Its Control in the Female Reproductive System. Br Med Bull (2000) 56:787–97. doi: 10.1258/0007142001903364
50. Hazzard TM, Stouffer RL. Angiogenesis in Ovarian Follicular and Luteal Development. Best Pract Res Clin Obstet Gynaecol (2000) 14:883–900. doi: 10.1053/beog.2000.0133
51. Gospodarowicz D, Thakral KK. Production of a Corpus Luteum Angiogenic Factor Responsible for Proliferation of Capillaries and Neovascularization of the Corpus Luteum. Proc Natl Acad Sci USA (1978) 75:847–51. doi: 10.1073/pnas.75.2.847
52. Trau HA, Davis JS, Duffy DM. Angiogenesis in the Primate Ovulatory Follicle Is Stimulated by Luteinizing Hormone via Prostaglandin E2. Biol Reprod (2015) 92:15. doi: 10.1095/biolreprod.114.123711
53. Schiffenbauer YS, Abramovitch R, Meir G, Nevo N, Holzinger M, Itin A, et al. Loss of Ovarian Function Promotes Angiogenesis in Human Ovarian Carcinoma. Proc Natl Acad Sci USA (1997) 94:13203–8. doi: 10.1073/pnas.94.24.13203
54. Liao H, Zhou Q, Gu Y, Duan T, Feng Y. Luteinizing Hormone Facilitates Angiogenesis in Ovarian Epithelial Tumor Cells and Metformin Inhibits the Effect Through the mTOR Signaling Pathway. Oncol Rep (2012) 27:1873–78. doi: 10.3892/or.2012.1745
55. Zhuo Y, Li X, Zheng Q, Fan X, Ma W, Chen J, et al. Estrogen Enhances Tumor Growth and Angiogenesis Indirectly via Mediation of Bone Marrow-Derived Cells as Well as Directly Through Stimulation of Tumor and Endothelial Cells. Oncol Rep (2018) 40:2147–56. doi: 10.3892/or.2018.6631
56. Hyder SM, Liang Y, Wu J. Estrogen Regulation of Thrombospondin-1 in Human Breast Cancer Cells. Int J Cancer (2009) 125:1045–53. doi: 10.1002/ijc.24373
57. Zuazo-Gaztelu I, Casanovas O. Unraveling the Role of Angiogenesis in Cancer Ecosystems. Front Oncol (2018) 8:248. doi: 10.3389/fonc.2018.00248
58. Nishida N, Yano H, Nishida T, Kamura T, Kojiro M. Angiogenesis in Cancer. Vasc Heal Risk Manag (2006) 2:213–9. doi: 10.2147/vhrm.2006.2.3.213
59. Hanahan D, Weinberg RA. Hallmarks of Cancer: The Next Generation. Cell (2011) 144:646–74. doi: 10.1016/j.cell.2011.02.013
60. Nagy JA, Chang SH, Shih SC, Dvorak AM, Dvorak HF. Heterogeneity of the Tumor Vasculature. Semin Thromb Hemost (2010) 36:321–31. doi: 10.1055/s-0030-1253454
61. Lugano R, Ramachandran M, Dimberg A. Tumor Angiogenesis: Causes, Consequences, Challenges and Opportunities. Cell Mol Life Sci (2020) 77:1745–70. doi: 10.1007/s00018-019-03351-7
62. Nagy JA, Chang SH, Dvorak AM, Dvorak HF. Why are Tumour Blood Vessels Abnormal and Why Is It Important to Know? Br J Cancer (2009) 100:865–69. doi: 10.1038/sj.bjc.6604929
63. Morikawa S, Baluk P, Kaidoh T, Haskell A, Jain RK, McDonald DM. Abnormalities in Pericytes on Blood Vessels and Endothelial Sprouts in Tumors. Am J Pathol (2002) 160:985–1000. doi: 10.1016/S0002-9440(10)64920-6
64. Kimura H, Braun RD, Ong ET, Hsu R, Secomb TW, Papahadjopoulos D, et al. Fluctuations in Red Cell Flux in Tumor Microvessels Can Lead to Transient Hypoxia and Reoxygenation in Tumor Parenchyma. Cancer Res (1996) 56:5522–8.
65. Liu Y, Cox SR, Morita T, Kourembanas S. Hypoxia Regulates Vascular Endothelial Growth Factor Gene Expression in Endothelial Cells: Identification of a 5’ Enhancer. Circ Res (1995) 77:638–43. doi: 10.1161/01.RES.77.3.638
66. Selvendiran K, Bratasz A, Kuppusamy ML, Tazi MF, Rivera BK, Kuppusamy P. Hypoxia Induces Chemoresistance in Ovarian Cancer Cells by Activation of Signal Transducer and Activator of Transcription 3. Int J Cancer (2009) 125:2198–204. doi: 10.1002/ijc.24601
67. Zhang K, Kong X, Feng G, Xiang W, Chen L, Yang F, et al. Investigation of Hypoxia Networks in Ovarian Cancer via Bioinformatics Analysis. J Ovarian Res (2018) 11:16. doi: 10.1186/s13048-018-0388-x
68. Trachana SP, Pilalis E, Gavalas NG, Tzannis K, Papadodima O, Liontos M, et al. The Development of an Angiogenic Protein “Signature” in Ovarian Cancer Ascites as a Tool for Biologic and Prognostic Profiling. PloS One (2016) 11:e0156403. doi: 10.1371/journal.pone.0156403
69. Schaaf MB, Garg AD, Agostinis P. Defining the Role of the Tumor Vasculature in Antitumor Immunity and Immunotherapy Article. Cell Death Dis (2018) 9:115. doi: 10.1038/s41419-017-0061-0
70. Dudley AC. Tumor Endothelial Cells. Cold Spring Harb Perspect Med (2012) 2:a006536. doi: 10.1101/cshperspect.a006536
71. Martin T, Ye L, Sanders A, Lane J, Jiang W. Cancer Invasion and Metastasis : Molecular and Cellular Perspective Cancer Invasion and Metastasis : The Role of Cell Adhesion Molecules. Cancer Invasion Metastasis Mol Cell Perspect (2014) 9:135–68.
72. Zhang B, Hu Y, Pang Z. Modulating the Tumor Microenvironment to Enhance Tumor Nanomedicine Delivery. Front Pharmacol (2017) 8:952. doi: 10.3389/fphar.2017.00952
73. Böckelmann LC, Schumacher U. Targeting Tumor Interstitial Fluid Pressure: Will It Yield Novel Successful Therapies for Solid Tumors? Expert Opin Ther Targets (2019) 23:1005–14. doi: 10.1080/14728222.2019.1702974
74. Rofstad EK, Huang R, Galappathi K, Andersen LMK, Wegner CS, Hauge A, et al. Functional Intratumoral Lymphatics in Patient-Derived Xenograft Models of Squamous Cell Carcinoma of the Uterine Cervix: Implications for Lymph Node Metastasis. Oncotarget (2016) 7:56986–97. doi: 10.18632/oncotarget.10931
75. Gossmann A, Helbich TH, Mesiano S, Shames DM, Wendland MF, Roberts TPL, et al. Magnetic Resonance Imaging in an Experimental Model of Human Ovarian Cancer Demonstrating Altered Microvascular Permeability After Inhibition of Vascular Endothelial Growth Factor. Am J Obstetrics Gynecol (2000) 183:956–63. doi: 10.1067/mob.2000.107092
76. Penet MF, Krishnamachary B, Wildes FB, Mironchik Y, Hung CF, Wu TC, et al. Ascites Volumes and the Ovarian Cancer Microenvironment. Front Oncol (2018) 8:595. doi: 10.3389/fonc.2018.00595
77. Rofstad EK, Gaustad JV, Egeland TAM, Mathiesen B, Galappathi K. Tumors Exposed to Acute Cyclic Hypoxic Stress Show Enhanced Angiogenesis, Perfusion and Metastatic Dissemination. Int J Cancer (2010) 127:1535–46. doi: 10.1002/ijc.25176
78. Iommarini L, Porcelli AM, Gasparre G, Kurelac I. Non-Canonical Mechanisms Regulating Hypoxia-Inducible Factor 1 Alpha in Cancer. Front Oncol (2017) 7:286. doi: 10.3389/fonc.2017.00286
79. Singh D, Arora R, Kaur P, Singh B, Mannan R, Arora S. Overexpression of Hypoxia-Inducible Factor and Metabolic Pathways: Possible Targets of Cancer. Cell Biosci (2017) 7:62. doi: 10.1186/s13578-017-0190-2
80. Qiu Y, Li P, Ji C. Cell Death Conversion Under Hypoxic Condition in Tumor Development and Therapy. Int J Mol Sci (2015) 16:25536–51. doi: 10.3390/ijms161025536
81. Welford SM, Giaccia AJ. Hypoxia and Senescence: The Impact of Oxygenation on Tumor Suppression. Mol Cancer Res (2011) 9:538–44. doi: 10.1158/1541-7786.MCR-11-0065
82. Varone E, Decio A, Chernorudskiy A, Minoli L, Brunelli L, Ioli F, et al. The ER Stress Response Mediator ERO1 Triggers Cancer Metastasis by Favoring the Angiogenic Switch in Hypoxic Conditions. Oncogene (2021) 40:1721–36. doi: 10.1038/s41388-021-01659-y
83. Wang GL, Jiang BH, Rue EA, Semenza GL. Hypoxia-Inducible Factor 1 Is a Basic-Helix-Loop-Helix-PAS Heterodimer Regulated by Cellular O2 Tension. Proc Natl Acad Sci USA (1995) 92:5510–4. doi: 10.1073/pnas.92.12.5510
84. Semenza GL. Signal Transduction to Hypoxia-Inducible Factor 1. Biochem Pharmacol (2002) 64:993–8. doi: 10.1016/S0006-2952(02)01168-1
85. Zhu G, Saed GM, Deppe G, Diamond MP, Munkarah AR. Hypoxia Up-Regulates the Effects of Prostaglandin E 2 on Tumor Angiogenesis in Ovarian Cancer Cells. Gynecol Oncol (2004) 94:422–6. doi: 10.1016/j.ygyno.2004.05.010
86. Bryant CS, Munkarah AR, Kumar S, Batchu RB, Shah JP, Berman J, et al. Reduction of Hypoxia-Induced Angiogenesis in Ovarian Cancer Cells by Inhibition of HIF-1 Alpha Gene Expression. Arch Gynecol Obstet (2010) 282:677–83. doi: 10.1007/s00404-010-1381-9
87. Yeung HY, Lai KP, Chan HY, Mak NK, Wagner GF, Wong CKC. Hypoxia-Inducible Factor-1-Mediated Activation of Stanniocalcin-1 in Human Cancer Cells. Endocrinology (2005) 146:4951–60. doi: 10.1210/en.2005-0365
88. Liu G, Yang G, Chang B, Mercado-Uribe I, Huang M, Zheng J, et al. Stanniocalcin 1 and Ovarian Tumorigenesis. J Natl Cancer Inst (2010) 102:812–27. doi: 10.1093/jnci/djq127
89. Law AYS, Wong CKC. Stanniocalcin-2 Is a HIF-1 Target Gene That Promotes Cell Proliferation in Hypoxia. Exp Cell Res (2010) 316:466–76. doi: 10.1016/j.yexcr.2009.09.018
90. Yang Y, Yin S, Li S, Chen Y, Yang L. Stanniocalcin 1 in Tumor Microenvironment Promotes Metastasis of Ovarian Cancer. Onco Targets Ther (2019) 12:2789–98. doi: 10.2147/OTT.S196150
91. Law AYS, Wong CKC. Stanniocalcin-2 Promotes Epithelial-Mesenchymal Transition and Invasiveness in Hypoxic Human Ovarian Cancer Cells. Exp Cell Res (2010) 316:3425–34. doi: 10.1016/j.yexcr.2010.06.026
92. Hua K, Din J, Cao Q, Feng W, Zhang Y, Yao L, et al. Estrogen and Progestin Regulate HIF-1α Expression in Ovarian Cancer Cell Lines via the Activation of Akt Signaling Transduction Pathway. Oncol Rep (2009) 21:893–8. doi: 10.3892/or_00000300
93. Daponte A, Ioannou M, Mylonis I, Simos G, Minas M, Messinis IE, et al. Prognostic Significance of Hypoxia-Inducible Factor 1 Alpha(HIF-1alpha) Expression in Serous Ovarian Cancer: An Immunohistochemical Study. BMC Cancer (2008) 8:335. doi: 10.1186/1471-2407-8-335
94. Osada R, Horiuchi A, Kikuchi N, Yoshida J, Hayashi A, Ota M, et al. Expression of Hypoxia-Inducible Factor 1α, Hypoxia-Inducible Factor 2α, and Von Hippel-Lindau Protein in Epithelial Ovarian Neoplasms and Allelic Loss of Von Hippel-Lindau Gene: Nuclear Expression of Hypoxia-Inducible Factor 1α Is an Independent Prognostic Factor in Ovarian Carcinoma. Hum Pathol (2007) 38:1310–20. doi: 10.1016/j.humpath.2007.02.010
95. Das B, Tsuchida R, Malkin D, Koren G, Baruchel S, Yeger H. Hypoxia Enhances Tumor Stemness by Increasing the Invasive and Tumorigenic Side Population Fraction. Stem Cells (2008) 26:1818–30. doi: 10.1634/stemcells.2007-0724
96. Yu Z, Pestell TG, Lisanti MP, Pestell RG. Cancer Stem Cells. Int J Biochem Cell Biol (2012) 44:2144–51. doi: 10.1016/j.biocel.2012.08.022
97. Richichi C, Brescia P, Alberizzi V, Fornasari L, Pelicci G. Marker-Independent Method for Isolating Slow-Dividing Cancer Stem Cells in Human Glioblastoma. Neoplasia (United States) (2013) 15:840–7. doi: 10.1593/neo.13662
98. Clarke MF, Fuller M. Stem Cells and Cancer: Two Faces of Eve. Cell (2006) 124:1111–5. doi: 10.1016/j.cell.2006.03.011
99. Schöning JP, Monteiro M, Gu W. Drug Resistance and Cancer Stem Cells: The Shared But Distinct Roles of Hypoxia-Inducible Factors HIF1α and HIF2α. Clin Exp Pharmacol Physiol (2017) 44:153–61. doi: 10.1111/1440-1681.12693
100. Zuber E, Schweitzer D, Allen D, Parte S, Kakar SS. Stem Cells in Ovarian Cancer and Potential Therapies. Proc Stem Cell Res Oncog (2020) 8:e1001.
101. Yoshida Y, Takahashi K, Okita K, Ichisaka T, Yamanaka S. Hypoxia Enhances the Generation of Induced Pluripotent Stem Cells. Cell Stem Cell (2009) 5:237–41. doi: 10.1016/j.stem.2009.08.001
102. Sun X, Lv X, Yan Y, Zhao Y, Ma R, He M, et al. Hypoxia-Mediated Cancer Stem Cell Resistance and Targeted Therapy. BioMed Pharmacother (2020) 130:110623. doi: 10.1016/j.biopha.2020.110623
103. Takebe N, Harris PJ, Warren RQ, Ivy SP. Targeting Cancer Stem Cells by Inhibiting Wnt, Notch, and Hedgehog Pathways. Nat Rev Clin Oncol (2011) 8:97–106. doi: 10.1038/nrclinonc.2010.196
104. Seo EJ, Kim DK, Jang IH, Choi EJ, Shin SH, Lee SI, et al. Hypoxia-NOTCH1-SOX2 Signaling Is Important for Maintaining Cancer Stem Cells in Ovarian Cancer. Oncotarget (2016) 7:55624–38. doi: 10.18632/oncotarget.10954
105. Liang D, Ma Y, Liu J, Trope CG, Holm R, Nesland JM, et al. The Hypoxic Microenvironment Upgrades Stem-Like Properties of Ovarian Cancer Cells. BMC Cancer (2012) 12:201. doi: 10.1186/1471-2407-12-201
106. Keith B, Johnson RS, Simon MC. HIF1 α and HIF2 α: Sibling Rivalry in Hypoxic Tumour Growth and Progression. Nat Rev Cancer (2012) 12:9–22. doi: 10.1038/nrc3183
107. Qin J, Liu Y, Lu Y, Liu M, Li M, Li J, et al. Hypoxia-Inducible Factor 1 Alpha Promotes Cancer Stem Cells-Like Properties in Human Ovarian Cancer Cells by Upregulating SIRT1 Expression. Sci Rep (2017) 7:10592. doi: 10.1038/s41598-017-09244-8
108. Tan DS, Agarwal R, Kaye SB. Mechanisms of Transcoelomic Metastasis in Ovarian Cancer. Lancet Oncol (2006) 7:925–34. doi: 10.1016/S1470-2045(06)70939-1
109. Klemba A, Bodnar L, Was H, Brodaczewska KK, Wcislo G, Szczylik CA, et al. Hypoxia-Mediated Decrease of Ovarian Cancer Cells Reaction to Treatment: Significance for Chemo-and Immunotherapies. Int J Mol Sci (2020) 21:9492. doi: 10.3390/ijms21249492
110. Binnewies M, Roberts EW, Kersten K, Chan V, Fearon DF, Merad M, et al. Understanding the Tumor Immune Microenvironment (TIME) for Effective Therapy. Nat Med (2018) 24:541–50. doi: 10.1038/s41591-018-0014-x
111. Baci D, Bosi A, Gallazzi M, Rizzi M, Noonan DM, Poggi A, et al. The Ovarian Cancer Tumor Immune Microenvironment (Time) as Target for Therapy: A Focus on Innate Immunity Cells as Therapeutic Effectors. Int J Mol Sci (2020) 21:3125. doi: 10.3390/ijms21093125
112. Worzfeld T, von Strandmann EP, Huber M, Adhikary T, Wagner U, Reinartz S, et al. The Unique Molecular and Cellular Microenvironment of Ovarian Cancer. Front Oncol (2017) 7:24. doi: 10.3389/fonc.2017.00024
113. Drakes ML, Stiff PJ. Regulation of Ovarian Cancer Prognosis by Immune Cells in the Tumor Microenvironment. Cancers (Basel) (2018) 10:302. doi: 10.3390/cancers10090302
114. Gong Y, Yang J, Wang Y, Xue L, Wang J. Metabolic Factors Contribute to T-Cell Inhibition in the Ovarian Cancer Ascites. Int J Cancer (2020) 147:1768–77. doi: 10.1002/ijc.32990
115. Rådestad E, Klynning C, Stikvoort A, Mogensen O, Nava S, Magalhaes I, et al. Immune Profiling and Identification of Prognostic Immune-Related Risk Factors in Human Ovarian Cancer. Oncoimmunology (2019) 8:e1535730. doi: 10.1080/2162402X.2018.1535730
116. Corzo CA, Condamine T, Lu L, Cotter MJ, Youn JI, Cheng P, et al. HIF-1α Regulates Function and Differentiation of Myeloid-Derived Suppressor Cells in the Tumor Microenvironment. J Exp Med (2010) 207:2439–53. doi: 10.1084/jem.20100587
117. Toutirais O, Chartier P, Dubois D, Bouet F, Lévêque J, Catros-Quemener V, et al. Constitutive Expression of TGF-Bêta1, Interleukin-6 and Interleukin-8 by Tumor Cells as a Major Component of Immune Escape in Human Ovarian Carcinoma. Eur Cytokine Netw (2003) 14:246–55.
118. Murdoch C, Giannoudis A, Lewis CE. Mechanisms Regulating the Recruitment of Macrophages Into Hypoxic Areas of Tumors and Other Ischemic Tissues. Blood (2004) 104:2224–34. doi: 10.1182/blood-2004-03-1109
119. Mills CD. M1 and M2 Macrophages: Oracles of Health and Disease. Crit Rev Immunol (2012) 32:463–88. doi: 10.1615/CritRevImmunol.v32.i6.10
120. Yuan X, Zhang J, Li D, Mao Y, Mo F, Du W, et al. Prognostic Significance of Tumor-Associated Macrophages in Ovarian Cancer: A Meta-Analysis. Gynecol Oncol (2017) 147:181–7. doi: 10.1016/j.ygyno.2017.07.007
121. Pantano F, Berti P, Guida FM, Perrone G, Vincenzi B, Amato MMC, et al. The Role of Macrophages Polarization in Predicting Prognosis of Radically Resected Gastric Cancer Patients. J Cell Mol Med (2013) 17:1415–21. doi: 10.1111/jcmm.12109
122. Ren L, Yu Y, Wang L, Zhu Z, Lu R, Yao Z. Hypoxia-Induced CCL28 Promotes Recruitment of Regulatory T Cells and Tumor Growth in Liver Cancer. Oncotarget (2016) 7:75763–73. doi: 10.18632/oncotarget.12409
123. Ohara M, Yamaguchi Y, Matsuura K, Murakami S, Arihiro K, Okada M. Possible Involvement of Regulatory T Cells in Tumor Onset and Progression in Primary Breast Cancer. Cancer Immunol Immunother (2009) 58:441–7. doi: 10.1007/s00262-008-0570-x
124. Sato E, Olson SH, Ahn J, Bundy B, Nishikawa H, Qian F, et al. Intraepithelial CD8+ Tumor-Infiltrating Lymphocytes and a High CD8+/regulatory T Cell Ratio are Associated With Favorable Prognosis in Ovarian Cancer. Proc Natl Acad Sci USA (2005) 102:18538–43. doi: 10.1073/pnas.0509182102
125. Naldini A, Morena E, Pucci A, Miglietta D, Riboldi E, Sozzani S, et al. Hypoxia Affects Dendritic Cell Survival: Role of the Hypoxia-Inducible Factor-1α and Lipopolysaccharide. J Cell Physiol (2012) 227:587–95. doi: 10.1002/jcp.22761
126. Hammami A, Abidin BM, Heinonen KM, Stäger S. HIF-1α Hampers Dendritic Cell Function and Th1 Generation During Chronic Visceral Leishmaniasis. Sci Rep (2018) 8:3500. doi: 10.1038/s41598-018-21891-z
127. Lin H, Kryczek I, Li S, Green MD, Ali A, Hamasha R, et al. Stanniocalcin 1 Is a Phagocytosis Checkpoint Driving Tumor Immune Resistance. Cancer Cell (2021) 39:480–93.e6. doi: 10.1016/j.ccell.2020.12.023
128. Leung CCT, Wong CKC. Effects of Stanniocalcin-1 Overexpressing Hepatocellular Carcinoma Cells on Macrophage Migration. PloS One (2020) 15:e0241932. doi: 10.1371/journal.pone.0241932
129. Chen L, Endler A, Shibasaki F. Hypoxia and Angiogenesis: Regulation of Hypoxia-Inducible Factors via Novel Binding Factors. Exp Mol Med (2009) 41:849–57. doi: 10.3858/emm.2009.41.12.103
130. Alexandrov LB, Nik-Zainal S, Wedge DC, Aparicio SAJR, Behjati S, Biankin AV, et al. Signatures of Mutational Processes in Human Cancer. Nature (2013) 500:415–21. doi: 10.1038/nature12477
131. Cai DL, Jin LP. Immune Cell Population in Ovarian Tumor Microenvironment. J Cancer (2017) 8:2915–23. doi: 10.7150/jca.20314
132. Ojalvo LS, Thompson ED, Wang TL, Meeker AK, Shih IM, Fader AN, et al. Tumor-Associated Macrophages and the Tumor Immune Microenvironment of Primary and Recurrent Epithelial Ovarian Cancer. Hum Pathol (2018) 74:135–47. doi: 10.1016/j.humpath.2017.12.010
133. Goode EL, Block MS, Kalli KR, Vierkant RA, Chen W, Fogarty ZC, et al. Dose-Response Association of CD8+ Tumor-Infiltrating Lymphocytes and Survival Time in High-Grade Serous Ovarian Cancer. JAMA Oncol (2017) 3:e173290. doi: 10.1001/jamaoncol.2017.3290
134. Pietrobon V, Marincola FM. Hypoxia and the Phenomenon of Immune Exclusion. J Transl Med (2021) 19:9. doi: 10.1186/s12967-020-02667-4
135. Hamanishi J, Mandai M, Ikeda T, Minami M, Kawaguchi A, Murayama T, et al. Safety and Antitumor Activity of Anti-PD-1 Antibody, Nivolumab, in Patients With Platinum-Resistant Ovarian Cancer. J Clin Oncol (2015) 33:4015–22. doi: 10.1200/JCO.2015.62.3397
136. Ghisoni E, Imbimbo M, Zimmermann S, Valabrega G. Ovarian Cancer Immunotherapy: Turning Up the Heat. Int J Mol Sci (2019) 20:2927. doi: 10.3390/ijms20122927
137. Battaglia A, Fossati M, Buzzonetti A, Scambia G, Fattorossi A. A Robust Immune System Conditions the Response to Abagovomab (Anti-Idiotypic Monoclonal Antibody Mimicking the CA125 Protein) Vaccination in Ovarian Cancer Patients. Immunol Lett (2017) 191:35–9. doi: 10.1016/j.imlet.2017.09.006
138. Zhang L, Conejo-Garcia JR, Katsaros D, Gimotty PA, Massobrio M, Regnani G, et al. Intratumoral T Cells, Recurrence, and Survival in Epithelial Ovarian Cancer. N Engl J Med (2003) 348:203–13. doi: 10.1056/nejmoa020177
139. Duechler M, Peczek L, Szubert M, Suzin J. Influence of Hypoxia Inducible Factors on the Immune Microenvironment in Ovarian Cancer. Anticancer Res (2014) 34:2811–9.
140. Böhm S, Montfort A, Pearce OMT, Topping J, Chakravarty P, Everitt GLA, et al. Neoadjuvant Chemotherapy Modulates the Immune Microenvironment in Metastases of Tubo-Ovarian High-Grade Serous Carcinoma. Clin Cancer Res (2016) 22:3025–36. doi: 10.1158/1078-0432.CCR-15-2657
141. Giuntoli RL, Webb TJ, Zoso A, Rogers O, Diaz-Montes TP, Bristow RE, et al. Ovarian Cancer-Associated Ascites Demonstrates Altered Immune Environment: Implications for Antitumor Immunity. Anticancer Res (2009) 29:2875–84.
142. Alharbi M, Lai A, Sharma S, Kalita-De Croft P, Godbole N, Campos A, et al. Extracellular Vesicle Transmission of Chemoresistance to Ovarian Cancer Cells Is Associated With Hypoxia-Induced Expression of Glycolytic Pathway Proteins, and Prediction of Epithelial Ovarian Cancer Disease Recurrence. Cancers (Basel) (2021) 13:3388. doi: 10.3390/cancers13143388
143. Kato Y, Ozawa S, Miyamoto C, Maehata Y, Suzuki A, Maeda T, et al. Acidic Extracellular Microenvironment and Cancer. Cancer Cell Int (2013) 13:89. doi: 10.1186/1475-2867-13-89
144. Thews O, Nowak M, Sauvant C, Gekle M. Hypoxia-Induced Extracellular Acidosis Increases P-Glycoprotein Activity and Chemoresistance in Tumors In Vivo via P38 Signaling Pathway. Adv Exp Med Biol (2011) 701:115–22. doi: 10.1007/978-1-4419-7756-4_16
145. Yu L, Chen X, Sun X, Wang L, Chen S. The Glycolytic Switch in Tumors: How Many Players Are Involved? J Cancer (2017) 8:3430–40. doi: 10.7150/jca.21125
146. Semenza GL. HIF-1: Upstream and Downstream of Cancer Metabolism. Curr Opin Genet Dev (2010) 20:51–6. doi: 10.1016/j.gde.2009.10.009
147. Pathania D, Millard M, Neamati N. Opportunities in Discovery and Delivery of Anticancer Drugs Targeting Mitochondria and Cancer Cell Metabolism. Adv Drug Delivery Rev (2009) 61:1250–75. doi: 10.1016/j.addr.2009.05.010
148. Lu T, Tang J, Shrestha B, Heath BR, Hong L, Lei YL, et al. Up-Regulation of Hypoxia-Inducible Factor Antisense as a Novel Approach to Treat Ovarian Cancer. Theranostics (2020) 10:6959–76. doi: 10.7150/thno.41792
149. Long F, Liu W, Jia P, Wang H, Jiang G, Wang T. HIF-1α-Induced Autophagy Contributes to Cisplatin Resistance in Ovarian Cancer Cells. Pharmazie (2018) 73:533–6. doi: 10.1691/ph.2018.8514
150. Sui X, Chen R, Wang Z, Huang Z, Kong N, Zhang M, et al. Autophagy and Chemotherapy Resistance: A Promising Therapeutic Target for Cancer Treatment. Cell Death Dis (2013) 4:e838. doi: 10.1038/cddis.2013.350
151. Wang J, Wu GS. Role of Autophagy in Cisplatin Resistance in Ovarian Cancer Cells. J Biol Chem (2014) 289:17163–73. doi: 10.1074/jbc.M114.558288
152. Zhao F, Yang G, Feng M, Cao Z, Liu Y, Qiu J, et al. Expression, Function and Clinical Application of Stanniocalcin-1 in Cancer. J Cell Mol Med (2020) 24:7686–96. doi: 10.1111/jcmm.15348
153. He LF, Wang TT, Gao QY, Zhao GF, Huang YH, Yu LK, et al. Stanniocalcin-1 Promotes Tumor Angiogenesis Through Up-Regulation of VEGF in Gastric Cancer Cells. J BioMed Sci (2011) 18:81. doi: 10.1186/1423-0127-18-39
154. Murai R, Tanaka M, Takahashi Y, Kuribayashi K, Kobayashi D, Watanabe N. Stanniocalcin-1 Promotes Metastasis in a Human Breast Cancer Cell Line Through Activation of PI3K. Clin Exp Metastasis (2014) 31:787–94. doi: 10.1007/s10585-014-9668-z
155. Han J, Jeon M, Shin I, Kim S. Elevated STC-1 Augments the Invasiveness of Triple-Negative Breast Cancer Cells Through Activation of the JNK/c-Jun Signaling Pathway. Oncol Rep (2016) 36:1764–71. doi: 10.3892/or.2016.4977
156. Chang ACM, Doherty J, Huschtscha LI, Redvers R, Restall C, Reddel RR, et al. STC1 Expression Is Associated With Tumor Growth and Metastasis in Breast Cancer. Clin Exp Metastasis (2015) 32:15–27. doi: 10.1007/s10585-014-9687-9
157. Deol HK, Varghese R, Wagner GF, Dimattia GE. Dynamic Regulation of Mouse Ovarian Stanniocalcin Expression During Gestation and Lactation. Endocrinology (2000) 141:3412–21. doi: 10.1210/endo.141.9.7658
158. Ambros V. MicroRNA Pathways in Flies and Worms: Growth, Death, Fat, Stress, and Timing. Cell (2003) 113:673–6. doi: 10.1016/S0092-8674(03)00428-8
159. Brennecke J, Hipfner DR, Stark A, Russell RB, Cohen SM. Bantam Encodes a Developmentally Regulated microRNA That Controls Cell Proliferation and Regulates the Proapoptotic Gene Hid in Drosophila. Cell (2003) 113:25–36. doi: 10.1016/S0092-8674(03)00231-9
160. Reinhart BJ, Slack FJ, Basson M, Pasquienelll AE, Bettlnger JC, Rougvle AE, et al. The 21-Nucleotide Let-7 RNA Regulates Developmental Timing in Caenorhabditis Elegans. Nature (2000) 403:901–6. doi: 10.1038/35002607
161. Dahiya N, Morin PJ. MicroRNAs in Ovarian Carcinomas. Endocr Relat Cancer (2010) 17:F77–89. doi: 10.1677/ERC-09-0203
162. Pandey R, Woo HH, Varghese F, Zhou M, Chambers SK. Circulating miRNA Profiling of Women at High Risk for Ovarian Cancer. Transl Oncol (2019) 12:714–72. doi: 10.1016/j.tranon.2019.01.006
163. Ishikawa M, Iwasaki M, Zhao H, Saito J, Hu C, Sun Q, et al. Sevoflurane and Desflurane Exposure Enhanced Cell Proliferation and Migration in Ovarian Cancer Cells via Mir-210 and Mir-138 Downregulation. Int J Mol Sci (2021) 22:1826. doi: 10.3390/ijms22041826
164. Li L, Huang K, You Y, Fu X, Hu L, Song L, et al. Hypoxia-Induced miR-210 in Epithelial Ovarian Cancer Enhances Cancer Cell Viability via Promoting Proliferation and Inhibiting Apoptosis. Int J Oncol (2014) 45:2111–20. doi: 10.3892/ijo.2014.2368
165. Giannakakis A, Sandaltzopoulos R, Greshock J, Liang S, Huang J, Hasegawa K, et al. miR-210 Links Hypoxia With Cell Cycle Regulation and Is Deleted in Human Epithelial Ovarian Cancer. Cancer Biol Ther (2008) 7:255–64. doi: 10.4161/cbt.7.2.5297
166. Feng L, Shen F, Zhou J, Li Y, Jiang R, Chen Y. Hypoxia-Induced Up-Regulation of miR-27a Promotes Paclitaxel Resistance in Ovarian Cancer. Biosci Rep (2020) 40:BSR20192457. doi: 10.1042/BSR20192457
167. Zhu X, Shen H, Yin X, Yang M, Wei H, Chen Q, et al. Macrophages Derived Exosomes Deliver miR-223 to Epithelial Ovarian Cancer Cells to Elicit a Chemoresistant Phenotype. J Exp Clin Cancer Res (2019) 38:81. doi: 10.1186/s13046-019-1095-1
168. Birner P, Schindl M, Obermair A, Breitenecker G, Oberhuber G. Expression of Hypoxia-Inducible Factor 1α in Epithelial Ovarian Tumors: Its Impact on Prognosis and on Response to Chemotherapy. Clin Cancer Res (2001) 7:1661–8 doi: 10.1002/1097-0142(20010701)92:1<165::AID-CNCR1305>3.0.CO;2-F
169. Cole AJ, Dwight T, Gill AJ, Dickson KA, Zhu Y, Clarkson A, et al. Assessing Mutant P53 in Primary High-Grade Serous Ovarian Cancer Using Immunohistochemistry and Massively Parallel Sequencing. Sci Rep (2016) 6:26191. doi: 10.1038/srep26191
170. Del Bufalo D, Ciuffreda L, Trisciuoglio D, Desideri M, Cognetti F, Zupi G, et al. Antiangiogenic Potential of the Mammalian Target of Rapamycin Inhibitor Temsirolimus. Cancer Res (2006) 66:5549–54. doi: 10.1158/0008-5472.CAN-05-2825
171. Sui W, Zhang Y, Wang Z, Wang Z, Jia Q, Wu L, et al. Antitumor Effect of a Selective COX-2 Inhibitor, Celecoxib, May be Attributed to Angiogenesis Inhibition Through Modulating the PTEN/PI3K/Akt/HIF-1 Pathway in an H22 Murine Hepatocarcinoma Model. Oncol Rep (2014) 31:2252–60. doi: 10.3892/or.2014.3093
172. Lee JG, Wu R. Erlotinib-Cisplatin Combination Inhibits Growth and Angiogenesis Through C-MYC and HIF-1α in EGFR-Mutated Lung Cancer In Vitro and In Vivo. Neoplasia (United States) (2015) 17:190–200. doi: 10.1016/j.neo.2014.12.008
173. Bohonowych JES, Peng S, Gopal U, Hance MW, Wing SB, Argraves KM, et al. Comparative Analysis of Novel and Conventional Hsp90 Inhibitors on HIF Activity and Angiogenic Potential in Clear Cell Renal Cell Carcinoma: Implications for Clinical Evaluation. BMC Cancer (2011) 11:520. doi: 10.1186/1471-2407-11-520
174. Kummar S, Raffeld M, Juwara L, Horneffer Y, Strassberger A, Allen D, et al. Multihistology, Target-Driven Pilot Trial of Oral Topotecan as an Inhibitor of Hypoxia-Inducible Factor-1α in Advanced Solid Tumors. Clin Cancer Res (2011) 17:5123–31. doi: 10.1158/1078-0432.CCR-11-0682
175. Mabjeesh NJ, Escuin D, LaVallee TM, Pribluda VS, Swartz GM, Johnson MS, et al. 2ME2 Inhibits Tumor Growth and Angiogenesis by Disrupting Microtubules and Dysregulating HIF. Cancer Cell (2003) 3:363–75. doi: 10.1016/S1535-6108(03)00077-1
176. Gajria D, Chandarlapaty S. HER2-Amplified Breast Cancer: Mechanisms of Trastuzumab Resistance and Novel Targeted Therapies. Expert Rev Anticancer Ther (2011) 11:263–75. doi: 10.1586/era.10.226
177. Nagata Y, Lan KH, Zhou X, Tan M, Esteva FJ, Sahin AA, et al. PTEN Activation Contributes to Tumor Inhibition by Trastuzumab, and Loss of PTEN Predicts Trastuzumab Resistance in Patients. Cancer Cell (2004) 6:117–27. doi: 10.1016/j.ccr.2004.06.022
178. Le XF, Pruefer F, Bast RC. HER2-Targeting Antibodies Modulate the Cyclin-Dependent Kinase Inhibitor p27Kip1. via multiple Signaling pathways Cell Cycle (2005) 4:87–95. doi: 10.4161/cc.4.1.1360
179. Henson ES, Hu X, Gibson SB. Herceptin Sensitizes ErbB2-Overexpressing Cells to Apoptosis by Reducing Antiapoptotic Mcl-1 Expression. Clin Cancer Res (2006) 12:845–53. doi: 10.1158/1078-0432.CCR-05-0754
180. Laughner E, Taghavi P, Chiles K, Mahon PC, Semenza GL. HER2 (Neu) Signaling Increases the Rate of Hypoxia-Inducible Factor 1α (HIF-1α) Synthesis: Novel Mechanism for HIF-1-Mediated Vascular Endothelial Growth Factor Expression. Mol Cell Biol (2001) 21:3995–4004. doi: 10.1128/mcb.21.12.3995-4004.2001
181. Izumi Y, Xu L, Di Tomaso E, Fukumura D, Jain RK. Herceptin Acts as an Anti-Angiogenic Cocktail. Nature (2002) 416:279–80. doi: 10.1038/416279b
182. Klos KS, Zhou X, Lee S, Zhang L, Yang W, Nagata Y, et al. Combined Trastuzumab and Paclitaxel Treatment Better Inhibits ErbB-2-Mediated Angiogenesis in Breast Carcinoma Through a More Effective Inhibition of Akt Than Either Treatment Alone. Cancer (2003) 98:1377–85. doi: 10.1002/cncr.11656
183. Medl M, Sevelda P, Czerwenka K, Dobianer K, Hanak H, Hruza C, et al. DNA Amplification of HER-2/Neu and INT-2 Oncogenes in Epithelial Ovarian Cancer. Gynecol Oncol (1995) 59:321–6. doi: 10.1006/gyno.1995.9969
184. Bookman MA, Darcy KM, Clarke-Pearson D, Boothby RA, Horowitz IR. Evaluation of Monoclonal Humanized Anti-HER2 Antibody, Trastuzumab, in Patients With Recurrent or Refractory Ovarian or Primary Peritoneal Carcinoma With Overexpression of HER2: A Phase II Trial of the Gynecologic Oncology Group. J Clin Oncol (2003) 21:283–90. doi: 10.1200/JCO.2003.10.104
185. Abe K, Kanehira M, Ohkouchi S, Kumata S, Suzuki Y, Oishi H, et al. Targeting Stanniocalcin-1-Expressing Tumor Cells Elicits Efficient Antitumor Effects in a Mouse Model of Human Lung Cancer. Cancer Med (2021) 10:3085–100. doi: 10.1002/cam4.3852
186. Guise CP, Abbattista MR, Anderson RF, Li D, Taghipouran R, Tsai A, et al. Subcellular Location of Tirapazamine Reduction Dramatically Affects Aerobic But Not Anoxic Cytotoxicity. Molecules (2020) 25:4888. doi: 10.3390/molecules25214888
187. Silva VL, Kaassis A, Dehsorkhi A, Koffi CR, Severic M, Abdelhamid M, et al. Enhanced Selectivity, Cellular Uptake, and: In Vitro Activity of an Intrinsically Fluorescent Copper-Tirapazamine Nanocomplex for Hypoxia Targeted Therapy in Prostate Cancer. Biomater Sci (2020) 8:2420–33. doi: 10.1039/c9bm01905g
188. Jameson MB, Rischin D, Pegram M, Gutheil J, Patterson AV, Denny WA, et al. A Phase I Trial of PR-104, a Nitrogen Mustard Prodrug Activated by Both Hypoxia and Aldo-Keto Reductase 1C3, in Patients With Solid Tumors. Cancer Chemother Pharmacol (2010) 65:791–801. doi: 10.1007/s00280-009-1188-1
189. Guo D, Xu S, Yasen W, Zhang C, Shen J, Huang Y, et al. Tirapazamine-Embedded Polyplatinum(Iv) Complex: A Prodrug Combo for Hypoxia-Activated Synergistic Chemotherapy. Biomater Sci (2020) 8:694–701. doi: 10.1039/c9bm01640f
190. Peeters SGJA, Zegers CML, Biemans R, Lieuwes NG, Van Stiphout RGPM, Yaromina A, et al. TH-302 in Combination With Radiotherapy Enhances the Therapeutic Outcome and Is Associated With Pretreatment [18F]HX4 Hypoxia PET Imaging. Clin Cancer Res (2015) 21:2984–92. doi: 10.1158/1078-0432.CCR-15-0018
191. Riedel RF, Meadows KL, Lee PH, Morse MA, Uronis HE, Blobe GC, et al. Phase I Study of Pazopanib Plus TH-302 in Advanced Solid Tumors. Cancer Chemother Pharmacol (2017) 79:611–9. doi: 10.1007/s00280-017-3256-2
192. Abou-Alfa GK, Chan SL, Lin CC, Chiorean EG, Holcombe RF, Mulcahy MF, et al. PR-104 Plus Sorafenib in Patients With Advanced Hepatocellular Carcinoma. Cancer Chemother Pharmacol (2011) 68:539–45. doi: 10.1007/s00280-011-1671-3
193. Abbattista MR, Jamieson SMF, Gu Y, Nickel JE, Pullen SM, Patterson AV, et al. Pre-Clinical Activity of PR-104 as Monotherapy and in Combination With Sorafenib in Hepatocellular Carcinoma. Cancer Biol Ther (2015) 16:610–22. doi: 10.1080/15384047.2015.1017171
194. Scaranti M, Cojocaru E, Banerjee S, Banerji U. Exploiting the Folate Receptor α in Oncology. Nat Rev Clin Oncol (2020) 17:349–59. doi: 10.1038/s41571-020-0339-5
195. Jang EH, Shim MK, Kim GL, Kim SH, Kang H, Kim JH. Hypoxia-Responsive Folic Acid Conjugated Glycol Chitosan Nanoparticle for Enhanced Tumor Targeting Treatment. Int J Pharm (2020) 580:119237. doi: 10.1016/j.ijpharm.2020.119237
196. Guo J, Yu Z, Das M, Huang L. Nano Codelivery of Oxaliplatin and Folinic Acid Achieves Synergistic Chemo-Immunotherapy With 5-Fluorouracil for Colorectal Cancer and Liver Metastasis. ACS Nano (2020) 14:5075–89. doi: 10.1021/acsnano.0c01676
197. Amreddy N, Babu A, Panneerselvam J, Srivastava A, Muralidharan R, Chen A, et al. Chemo-Biologic Combinatorial Drug Delivery Using Folate Receptor-Targeted Dendrimer Nanoparticles for Lung Cancer Treatment. Nanomed Nanotechnology Biol Med (2018) 14:373–84. doi: 10.1016/j.nano.2017.11.010
198. Khan MM, Madni A, Filipczak N, Pan J, Rehman M, Rai N, et al. Folate Targeted Lipid Chitosan Hybrid Nanoparticles for Enhanced Anti-Tumor Efficacy. Nanomed Nanotechnology Biol Med (2020) 28:102228. doi: 10.1016/j.nano.2020.102228
199. Looprasertkul S, Sereemaspun A, Kitkumthorn N, Sooklert K, Sarachana T, Jindatip D. Gold Nanoparticles Affect Pericyte Biology and Capillary Tube Formation. Pharmaceutics (2021) 13:738. doi: 10.3390/pharmaceutics13050738
200. Kemp MM, Kumar A, Mousa S, Dyskin E, Yalcin M, Ajayan P, et al. Gold and Silver Nanoparticles Conjugated With Heparin Derivative Possess Anti-Angiogenesis Properties. Nanotechnology (2009) 20:455104. doi: 10.1088/0957-4484/20/45/455104
201. Pan Y, Ding H, Qin L, Zhao X, Cai J, Du B. Gold Nanoparticles Induce Nanostructural Reorganization of VEGFR2 to Repress Angiogenesis. J BioMed Nanotechnol (2013) 9:1746–56. doi: 10.1166/jbn.2013.1678
202. Setyawati MI, Leong DT. Mesoporous Silica Nanoparticles as an Antitumoral-Angiogenesis Strategy. ACS Appl Mater Interfaces (2017) 9:6690–703. doi: 10.1021/acsami.6b12524
203. Matuszewska K, Pereira M, Petrik D, Lawler J, Petrik J. Normalizing Tumor Vasculature to Reduce Hypoxia, Enhance Perfusion, and Optimize Therapy Uptake. Cancers (Basel) (2021) 13:4444. doi: 10.3390/cancers13174444
204. Chappell NP, Miller CR, Fielden AD, Barnett JC. Is FDA-Approved Bevacizumab Cost-Effective When Included in the Treatment of Platinum-Resistant Recurrent Ovarian Cancer? J Oncol Pract (2016) 12:e775–83. doi: 10.1200/JOP.2015.010470
205. Della Pepa C, Banerjee S. Bevacizumab in Combination With Chemotherapy in Platinum-Sensitive Ovarian Cancer. Onco Targets Ther (2014) 7:1025–32. doi: 10.2147/OTT.S40527
206. Bamias A, Gibbs E, Khoon Lee C, Davies L, Dimopoulos M, Zagouri F, et al. Bevacizumab With or After Chemotherapy for Platinum-Resistant Recurrent Ovarian Cancer: Exploratory Analyses of the AURELIA Trial. Ann Oncol (2017) 28:1842–8. doi: 10.1093/annonc/mdx228
207. O’Malley DM, Matulonis UA, Birrer MJ, Castro CM, Gilbert L, Vergote I, et al. Phase Ib Study of Mirvetuximab Soravtansine, a Folate Receptor Alpha (Frα)-Targeting Antibody-Drug Conjugate (ADC), in Combination With Bevacizumab in Patients With Platinum-Resistant Ovarian Cancer. Gynecol Oncol (2020) 157:379–85. doi: 10.1016/j.ygyno.2020.01.037
208. Guerin E, Man S, Xu P. Kerbel RS. A Model of Postsurgical Advanced Metastatic Breast Cancer More Accurately Replicates the Clinical Efficacy of Antiangiogenic Drugs. Cancer Res (2013) 73:2743–8. doi: 10.1158/0008-5472.CAN-12-4183
209. Lan CY, Wang Y, Xiong Y, Li JD, Shen JX, Li YF, et al. Apatinib Combined With Oral Etoposide in Patients With Platinum-Resistant or Platinum-Refractory Ovarian Cancer (AEROC): A Phase 2, Single-Arm, Prospective Study. Lancet Oncol (2018) 19:1239–46. doi: 10.1016/S1470-2045(18)30349-8
210. Allen E, Jabouille A, Rivera LB, Lodewijckx I, Missiaen R, Steri V, et al. Combined Antiangiogenic and Anti-PD-L1 Therapy Stimulates Tumor Immunity Through HEV Formation. Sci Transl Med (2017) 9:eaak9679. doi: 10.1126/scitranslmed.aak9679
211. Arora S, Balasubramaniam S, Zhang H, Berman T, Narayan P, Suzman D, et al. FDA Approval Summary: Olaparib Monotherapy or in Combination With Bevacizumab for the Maintenance Treatment of Patients With Advanced Ovarian Cancer. Oncologist (2021) 26:e164–72. doi: 10.1002/onco.13551
212. Li L, Nan F, Guo Q, Guan D, Zhou C. Resistance to Bevacizumab in Ovarian Cancer SKOV3 Xenograft Due to EphB4 Overexpression. J Cancer Res Ther (2019) 15:1282–7. doi: 10.4103/0973-1482.204896
213. Zhou Q, Perakis SO, Ulz P, Mohan S, Riedl JM, Talakic E, et al. Cell-Free DNA Analysis Reveals POLR1D-Mediated Resistance to Bevacizumab in Colorectal Cancer. Genome Med (2020) 12:20. doi: 10.1186/s13073-020-0719-6
214. Russell S, Duquette M, Liu J, Drapkin R, Lawler J, Petrik J. Combined Therapy With Thrombospondin-1 Type I Repeats (3TSR) and Chemotherapy Induces Regression and Significantly Improves Survival in a Preclinical Model of Advanced Stage Epithelial Ovarian Cancer. FASEB J (2015) 29:576–88. doi: 10.1096/fj.14-261636
215. Matuszewska K, Santry LA, van Vloten JP, AuYeung AWK, Major PP, Lawler J, et al. Combining Vascular Normalization With an Oncolytic Virus Enhances Immunotherapy in a Preclinical Model of Advanced-Stage Ovarian Cancer. Clin Cancer Res (2019) 25:1624–38. doi: 10.1158/1078-0432.CCR-18-0220
216. Zhang Q, Li Y, Miao C, Wang Y, Xu Y, Dong R, et al. Anti-Angiogenesis Effect of Neferine via Regulating Autophagy and Polarization of Tumor-Associated Macrophages in High-Grade Serous Ovarian Carcinoma. Cancer Lett (2018) 432:144–55. doi: 10.1016/j.canlet.2018.05.049
217. Bonkowski D, Katyshev V, Balabanov RD, Borisov A, Dore-Duffy P. The CNS Microvascular Pericyte: Pericyte-Astrocyte Crosstalk in the Regulation of Tissue Survival. Fluids Barriers CNS (2011) 8:8. doi: 10.1186/2045-8118-8-8
218. Ueda K, Lu Q, Baur W, Aronovitz MJ, Karas RH. Rapid Estrogen Receptor Signaling Mediates Estrogen-Induced Inhibition of Vascular Smooth Muscle Cell Proliferation. Arterioscler Thromb Vasc Biol (2013) 33:1837–43. doi: 10.1161/ATVBAHA.112.300752
219. Miller AP, Chen YF, Xing D, Feng W, Oparil S. Hormone Replacement Therapy and Inflammation: Interactions in Cardiovascular Disease. in. Hypertension (2003) 42:657–63. doi: 10.1161/01.HYP.0000085560.02979.0C
220. Johnson KE, Forward JA, Tippy MD, Ceglowski JR, El-Husayni S, Kulenthirarajan R, et al. Tamoxifen Directly Inhibits Platelet Angiogenic Potential and Platelet-Mediated Metastasis. Arterioscler Thromb Vasc Biol (2017) 37:664–74. doi: 10.1161/ATVBAHA.116.308791
Keywords: ovarian cancer, hypoxia, tumor microenvironment, endocrine cancer, immunotherapy
Citation: Pereira M, Matuszewska K, Jamieson C and Petrik J (2021) Characterizing Endocrine Status, Tumor Hypoxia and Immunogenicity for Therapy Success in Epithelial Ovarian Cancer. Front. Endocrinol. 12:772349. doi: 10.3389/fendo.2021.772349
Received: 08 September 2021; Accepted: 26 October 2021;
Published: 17 November 2021.
Edited by:
Bertrand Duvillie, Institut Curie, FranceReviewed by:
Timm Schreiber, Witten/Herdecke University, GermanyAtsushi P. Kimura, Hokkaido University, Japan
Copyright © 2021 Pereira, Matuszewska, Jamieson and Petrik. This is an open-access article distributed under the terms of the Creative Commons Attribution License (CC BY). The use, distribution or reproduction in other forums is permitted, provided the original author(s) and the copyright owner(s) are credited and that the original publication in this journal is cited, in accordance with accepted academic practice. No use, distribution or reproduction is permitted which does not comply with these terms.
*Correspondence: Jim Petrik, jpetrik@uoguelph.ca