- Unité de Biologie Fonctionnelle et Adaptative, UMR8251 CNRS, Université de Paris, Paris, France
The glycoprotein hormones (GPH) are heterodimers composed of a common α subunit and a specific β subunit. They act by activating specific leucine-rich repeat G protein-coupled receptors. However, individual subunits have been shown to elicit responses in cells devoid of the receptor for the dimeric hormones. The α subunit is involved in prolactin production from different tissues. The human chorionic gonadotropin β subunit (βhCG) plays determinant roles in placentation and in cancer development and metastasis. A truncated form of the thyrotropin (TSH) β subunit is also reported to have biological effects. The GPH α- and β subunits are derived from precursor genes (gpa and gpb, respectively), which are expressed in most invertebrate species and are still represented in vertebrates as GPH subunit paralogs (gpa2 and gpb5, respectively). No specific receptor has been found for the vertebrate GPA2 and GPB5 even if their heterodimeric form is able to activate the TSH receptor in mammals. Interestingly, GPA and GPB are phylogenetically and structurally related to cysteine-knot growth factors (CKGF) and particularly to a group of antagonists that act independently on any receptor. This review article summarizes the observed actions of individual GPH subunits and presents the current hypotheses of how these actions might be induced. New approaches are also proposed in light of the evolutionary relatedness with antagonists of the CKGF family of proteins.
1 The Glycoprotein Hormones in Vertebrates: An Overview
1.1 Pituitary Glycoprotein Hormones
The glycoprotein hormones (GPH) are heterodimeric glycoproteins composed of an α subunit that is common to all of them and a β subunit that confers the hormonal specificity to the dimer. The GPH family in vertebrates essentially comprises two gonadotropins, the luteinizing hormone (LH) and follicle-stimulating hormone (FSH) and a thyroid-stimulating hormone (TSH) (1–3). The common α- subunit and LHβ, FSHβ, and TSHβ subunits are each encoded by a single gene (cga, lhb, fshb and tshb, respectively). Both α and β subunits are glycosylated (Figure 1). The α subunit harbors two N-glycosylation sites at conserved positions. Two N-glycosylation sites are also usually present in FSHβ subunit (only one in most teleost species) (4), whereas LHβ and TSHβ subunits have only one. The position of these glycosylation sites is also conserved between the β subunits, one being common to LHβ and FSHβ subunits and the other one common to TSHβ and FSHβ subunits. From a structural point of view, the GPH subunits belong to the superfamily of cystine-knot proteins and particularly to the transforming growth factor β (TGFβ) subfamily of growth factors (5–7). These cystine-knot proteins are characterized by unique cysteine bonding arrangement that confers a flat and elongated structure fostering their association into homo or heterodimers with or without intermolecular disulfide bonding. Each GPH subunit contains four antiparallel β strands tightened with the core cystine-knot motif from which three hairpin loops are deployed (Figure 1). The α and β subunits contain a total of 10 and 12 cysteine residues, respectively, with three pairs of them involved in the cystine-knot motif. GPHs are assembled in a head to tail association so that loops 1 and 3 from one subunit are intertwined with loop 2 of the other subunit. The non-covalently associated GPH heterodimer is stabilized by a « seatbelt » mechanism involving an intramolecular cysteine bridge that closes a carboxy-terminal buckle of the β subunit, encircling loop 2 of the α subunit (8). GPH heterodimers act by activating related but specific receptors (GPHR, namely LHR, FSHR and TSHR) (9–11). Among the large G-protein-coupled receptor superfamily, GPHRs belong to the family of Leucine-rich repeat receptors (LGR type A), characterized by a particularly long extracellular domain that adopts a concave structure to receive the GPH heterodimer with both subunits in contact with the receptor (7, 11). Consequently, none of the GPH subunits is able to satisfactorily (at physiological level) activate any GPHR in a non-heterodimeric association (1). Upon binding, GPHs usually activate the protein Gs-dependent cAMP pathway (12).
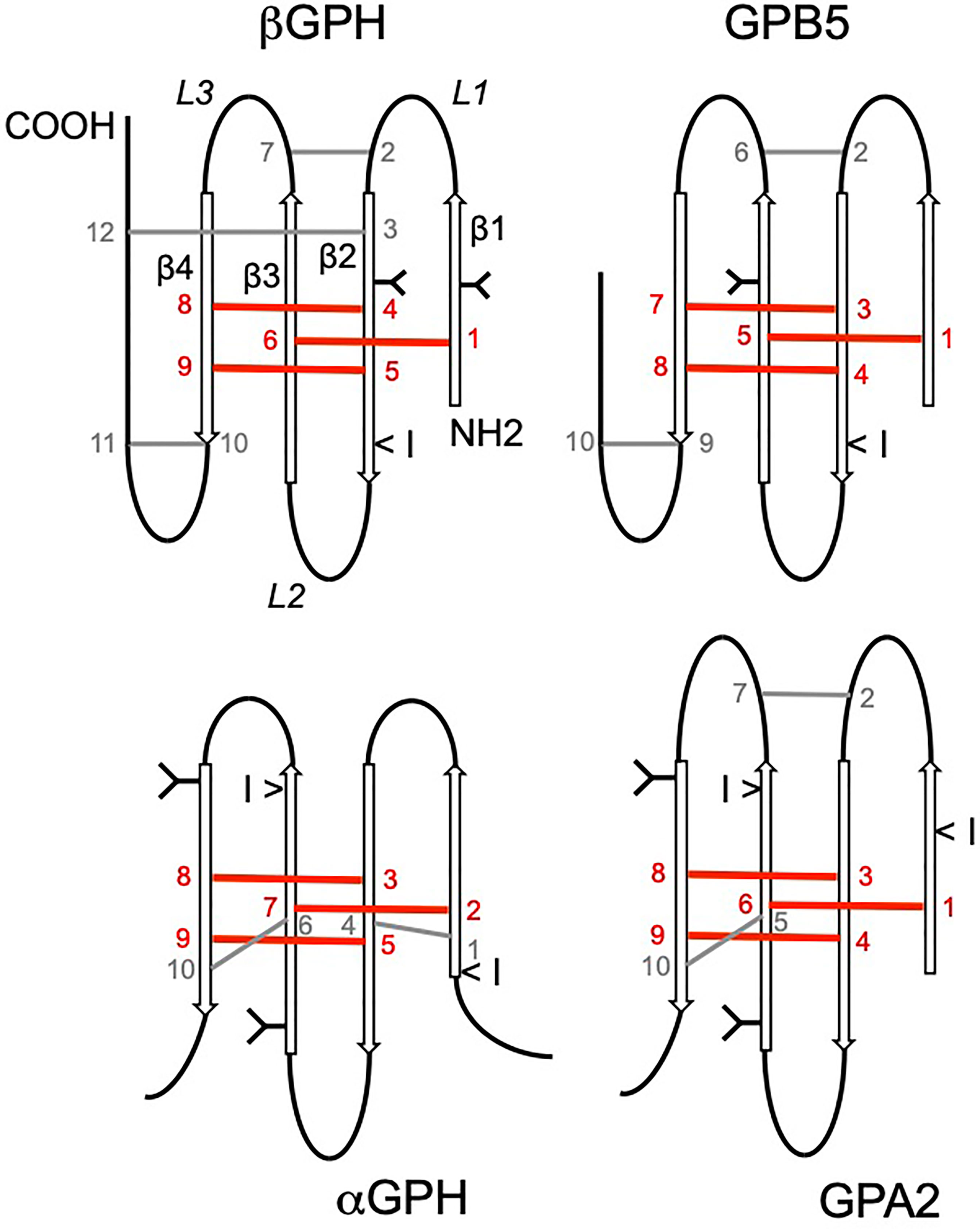
Figure 1 Schematic representation of the glycoprotein hormone (GPH) α- and β subunits and comparison with predicted structure of GPH paralogs GPA2 and GPB5. N-linked glycosylated chains are represented by Y shapes: for GPHβ subunits, the first position (from the N-terminal) is common to LH- and FSHβ subunits whereas the second one is common to TSH- and FSHβ subunits. Location of the conserved intron splicing site is indicated by <I symbol. The conserved cysteine residues are numbered. Disulfide bonds are represented by bars. Bars and cysteine numbering are given in red when involved in the cystine-knot structure and in grey for the additional ones. Location of the β strands (β1–β4) and loops (L1–L3) is indicated.
The thyrotropin and the two gonadotropins are mainly produced by the pituitary and secreted toward the general circulation (13, 14). TSH is essentially produced by a specific cell-type of the pars distalis (PD), the thyrotropes. A second site of synthesis of TSH in birds and mammals is located in the pars tuberalis (PT), a structure connected to the mediobasal hypothalamus (15). The two gonadotropins are usually concomitantly but, independently produced by the same gonadotrope cells in mammals. In teleosts, each hormone is synthesized by a specific cell-type (4). In addition to gonadotropes and thyrotropes, the anterior pituitary gland contains adrenocorticotropin hormone-secreting cells or corticotropes, growth hormone-secreting cells or somatotropes, prolactin-secreting cells or lactotropes as well as non-endocrine cells. Among these latter cells are the folliculostellate cells that form a network connecting them to hormone-secreting cells (14, 16).
In the female (most studies have been performed in mammals), FSH is responsible for the maturation of the follicles through its receptor expressed on granulosa cells. LH activates its receptor on theca cells to stimulate steroid synthesis. Some of these steroids are further transformed in the granulosa cells under the influence of FSH during folliculogenesis and of LH later on. The final stage of maturation of oocyte and granulosa cells and ovulation are activated by LH acting through its receptor, which, at this stage, is expressed on granulosa cells. In the male, LH acts in the testis to stimulate steroidogenesis by the Leydig cells whereas FSH acts on Sertoli cells to control spermatogenesis (1, 17). The main action of the TSH from the PD is to stimulate thyrocytes to produce the thyroid hormones (TH) (18). GPH receptors are also expressed in a number of different tissues and GPHs have been found to be involved in a variety of functions by activating these receptors (19–21).
Although submitted to some control, the pituitary GPHα subunit is usually produced in large amount and the dimer formation is dependent on the rate-limiting β subunits. The expression of each GPHβ subunit is under specific regulations (22–24). The hypothalamus provides the main activators of the GPHs production. These stimulators are short peptides produced by specific neurons that are delivered by pulses into the blood that irrigates the anterior pituitary gland. The gonadotropin-releasing hormone (GnRH) preferentially stimulates the production of lhb or fshb subunit genes according to pulse amplitude and frequency (25). GnRH also stimulates the synthesis of the GPHα subunit in gonadotrope cells, notably when delivered at high pulse frequency (26). The GPHs are tightly regulated by feedback mechanisms arising from their target organs (27). Gonadal steroids exert a sustained retroactive effect on both the hypothalamus and the pituitary gonadotropes to modulate the circulating level of each of the two gonadotropins. Other gonadal (inhibin, activin), metabolic (leptin, fatty acids) or paracrine and autocrine factors contribute to the fine-tuning of LH or FSH production and secretion. Both PD-TSH α and β subunits are stimulated by the thyrotropin-releasing hormone (TRH) in mammals (28, 29) or corticotropin-releasing hormone (CRH) in most non-mammalian vertebrates (30). The expression of PD-TSH is negatively regulated by TH level (22, 31). Numerous paracrine and autocrine factors also participate to achieve an appropriate TH circulating level (32).
1.2 GPHs and Their Receptors Are Indispensable for Species Survival
There are no vertebrate species that were shown to lack any of the GPH subunits or GPH receptors, underscoring their irreplaceable role in reproduction or metabolic homeostasis. Inactivation of the lhb subunit gene in mice revealed postnatal defects that led to infertility of both sexes with reduced testosterone levels and blocked spermatogenesis in males, low estradiol and progesterone levels and defects in folliculogenesis in females (33). Disruption of the LHR drove to similar phenotypes (34–36). Loss of function of either FSHβ subunit or FSHR generated mice with similar phenotypes, i.e. reduced testes volume and altered spermatogenesis but still fertility in males and reduced uterus volume and blocked folliculogenesis leading to infertility in females (37–39). In teleosts, GPHR specificity is not as strict as in mammals and LH can activate the FSHR (4, 40–42) and FSH was also recently shown to activate LHR (43). Consequently, phenotypes of GPH or GPHR-knockout fish are not as deleterious as in mammals. LHβ-null male zebrafish are fertile but females do not spawn (44, 45). FSHβ-deficient zebrafish of both sexes are fertile albeit with delayed gonadal development (45, 46). FSHR knockout male zebrafish are fertile (46) but female zebrafish and medaka displayed arrested growth of follicles at a prepubertal stage (47–49). TSHR-KO mice have non-detectable TH levels, are severely blunted and die after weaning in absence of TH-supplemented diet (50, 51).
1.3 Chorionic Gonadotropin
In addition to the two pituitary gonadotropins and TSH that are present in all vertebrate branches, an additional heterodimeric gonadotropin is produced in primates (1) and in equids (52) mainly by the placenta. In primates, the locus encompassing lhb has been submitted to serial tandem duplication events giving rise to 6 functional lhb-derived genes in human (53–55). Most of them are essentially expressed, together with the GPHα subunit, by syncytiotrophoblastic cells in the placenta to produce the chorionic gonadotropin (CG). In equids, both the cga and lhb genes have acquired the ability to be expressed by specialized placental cells to produce the so-called LH-CG. In human, CG (hCG) appears to be also produced at low level by the pituitary (56–59). The sequence of these duplicated CGβ-encoding genes (cgb) in a given primate species is well conserved between each other and with lhb, thanks to a conservative mechanism of gene conversion that uses parts of lhb as the template (53). The CGβ subunits however differ from the parent LHβ subunit by an extended carboxy-terminal end that results from a single nucleotide deletion allowing the bypass of the stop codon and the incorporation of the entire 3’ untranslated region of the parent lhb (60). The equine LH-CGβ subunit also has a serine-rich carboxy-terminal extension resulting from an independent but similar in effect, frameshift mutation (52). The serine-reach extension allows the attachment of several O-linked glycosylated chains in addition to the N-linked sugar chains harbored by both the GPHα and LHβ or CGβ subunits. It is to be noted also that hCGβ subunit has an additional N-linked glycosylation chain relative to hLHβ subunit providing two carbohydrate chains at the same position as in the FSHβ subunit. These characteristics dramatically increase the half-life of hCG compared to LH. Recently, a new splicing variant of the cga has been characterized in human and other primates (61). This variant includes a 31 amino acid-long Alu-J-type segment few amino acids downstream of the signal peptide. It is reported to be expressed exclusively in the placenta within the same cells as the CGβ subunit and to confer an even longer half-life in the circulation to the dimer it forms with hCGβ subunit. Both equine LH-CG and primate CGs exclusively bind to the LHR, which in these species is called LH-CGR. The equine LH-CG (eLH-CG also called pregnant mare serum gonadotropin or PMSG) is able to bind to mammalian FSHR but only in non-equine species (9, 62). The principal actions of hCG have been extensively reviewed (63–65). Among them and through the LH-CGR, hCG has been demonstrated to stimulate progesterone production by the corpus luteum, promote angiogenesis for the development of the uterine vasculature, stimulate the differentiation of cytotrophoblast cells, and promote quiescence of the uterine myometrium. hCG also contributes to the reduction of the immune response towards the fetus (66).
2 Glycoprotein Hormone Related Proteins
2.1 GPH-Related Proteins in Vertebrates: A Phylogenetic and Structural Relatedness
The GPH α and β subunits are derived from two precursor genes present since the emergence of bilaterians (2, 67, 68). A locus encompassing these precursor genes is assumed to have tandemly duplicated just before the radiation of vertebrates to generate the ancestral GPHα- and β subunit encoding genes (2). The vertebrate forms of these precursor genes where first characterized in human (67) so that they were named gpa2 or gpha2, i.e. GPH α subunit type 2-encoding gene (to take into account the existence of the already known and classical GPH α subunit) and gpb5 or gphb5, i.e. GPHβ subunit type 5 encoding gene (LHβ, FSHβ, TSHβ and CGβ subunits counting for types 1-4). They share numerous structural characteristics with the GPH subunits (67). They have a very similar cysteine residue arrangement including the cystine-knot motif (Figure 1). GPA2 bears two N-linked carbohydrate chains, one of which in a conserved position with the GPHα subunit (69). GPB5 has only one N-glycosylated site, located on a different position as in GPHβ subunits. The main differences concern the carboxy-terminal end of GPB5 that is much shorter than in GPHβ subunits and the absence of the two cysteine residues involved in the so called « seatbelt » mechanism that is crucial for the dimer to be kept tighten once diluted in the general circulation (70, 71). As for the classical GPHs, GPA2 and GPB5 can nevertheless combine into a heterodimer. However, neither human GPA2 was shown to stably associate with any of the human GPHβ subunits, nor GPB5 with the GPHα subunit (72). Of note however, a recombinant heterodimer was produced by combining the lamprey unique (up to now) gonadotropin β subunit with lamprey GPA2. This exotic heterodimer, when expressed as a single chain, was able to activate one of the two identified lamprey GPHRs in vitro (73). No specific receptor has been found yet for the GPA2/GPB5 heterodimer in vertebrates. Nonetheless, the recombinant mammalian GPA2/GPB5 heterodimer is several times more potent than TSH in activating homologous TSHR (69, 72), but does not activate homologous LHR and FSHR. The GPA2/GPB5 heterodimer was consequently named thyrostimulin (72). As for the GPH subunits, GPA2 and GPB5 do not activate TSHR as monomers at physiological level. To date, the role of GPA2 and GPB5 in vertebrates has mostly been investigated according to the thyrostimulin concept, i.e. as a heterodimer activating the TSHR (69, 74–80). No obvious or conserved role has been found or confirmed for the GPA2/GPB5 heterodimer to date. In addition, if the GPA2 knock out has not been described yet, mice lacking GPB5 were healthy with no deleterious phenotype (69, 74, 77).
2.2 GPH-Related Precursor Proteins Outside Vertebrates
The genome of most invertebrate groups contains genes encoding the precursor forms of the αGPH/GPA2 and βGPH/GPB5 subunits (67, 68, 81, 82) that will thereafter be referred to as gpa and gpb, respectively. Although GPHs LH, FSH and TSH are restricted to vertebrates, a gphr related (LGR-1, a type A LGR) gene was identified in several non-vertebrate groups (81, 83, 84) that arose with the emergence of bilaterians (68). The recombinant fly GPA/GPB heterodimer (but not individual subunits) binds to and activates the expressed fly LGR-1 (85), confirming a functional relationship between GPHRs and LGR-1s. In agreement with a functional relationship between GPA (flr-2), GPB (flr-5) and LGR-1 (flr-6) in the nematode Caenorhabditis, they all constitute together with another uncharacterized gene (flr-7), one of the classes of genes involved in resistance to fluoride ions (86). In the fly, ablation of GPB5-expressing cells reduced the survival rate, demonstrating a determinant role of this protein during larval development (87). However, as for vertebrates, no conserved role or role that will be shared in several unrelated species has been put forward to date for the invertebrate GPA/GPB heterodimer (83).
3 Unconventional Actions of GPHs or Their Subunits
A number of observations made in vertebrates shows that some of the GPH subunits are produced and released as monomers or homo-multimers and may induce cellular responses independently of GPHR activation and even in cells that do not express GPH receptors. This review article will summarize results obtained for the individual GPH subunits (Table 1), and will also question possible actions for individual GPA and GPB in invertebrates and for their vertebrate derivatives. TSH also presents structural variants that behave in unconventional ways that will be briefly presented in this review.
3.1 Occurrence and Actions of the Free GPHα Subunit
3.1.1 GPHα Subunit and Lactotrope Differentiation and Prolactin Production
During the ontogeny of the pituitary gland in mice and human, the GPHα subunit production starts long before that of any GPHβ subunits (88–91). This free GPHα subunit is released from human fetal pituitary tissue in culture in large amount, exceeding by 200 times the amount of LH and FSH (92). In human fetuses, the circulating level of the α subunit peaks at ten times the level of heterodimeric glycoprotein hormones (93–96). Even in adults, the α subunit is released as a non heterodimeric form (97–100), circulating in the blood in parallel with LH during the menstrual cycle (101, 102) and increasing according to GnRH stimulation in women (97) as well as in men (103). The free α subunit differs from that associated in GPH heterodimers by presenting additional saccharide branching resulting in a slightly heavier molecular mass (104, 105). Homodimers of the α subunit were characterized in both β subunit producing and non-producing cells (106). The α subunits are more tightly associated in the α homodimer than in the heterodimers (106). While the synthesis of large excess of α subunit allows the regulation of the heterodimer production to rely on the rate-limiting β subunit, the released free, heavily glycosylated α subunit may also serve other purposes and possibly, play a role by itself.
Purified α subunit was indeed shown to be involved in stimulating lactotrope differentiation in rat fetal pituitary explants. This action was prevented by the addition of an antiserum against the α subunit but not by an antiserum against the LHβ subunit (107). The α subunit was further demonstrated to have trophic effects on lactotrope cells expansion and production of prolactin on 14-day-old rat pituitary cells allowed to re-aggregate after dispersal (108). In support for a local paracrine action of the GPHα subunit on lactotropes, a preferential connection between gonadotropes and lactotrope cells has been evidenced in the rat (109–111). A physiological role of the α subunit on lactotrope differentiation or proliferation is also supported by the abundance of lactotrope cells in the pituitary gland of anencephalic human fetuses that lack GnRH innervation (112) and present high amount of GPHα subunit (113). Conversely, mice inactivated for the α subunit gene are almost devoid of lactotrope cells (114, 115). The α subunit also specifically stimulated the release of prolactin in late developing ovine fetal pituitary explants, but only at a time when lactotrope cells proliferate (116), suggesting that in ovine, the α subunit does not accelerate lactotrope differentiation but increases prolactin production from fully differentiated cells. In hamster, a correlation was found between an increase in α subunit expression and prolactin expression after a prolonged short photoperiod exposure (117). Outside mammals, the α subunit was also shown (albeit at relative high doses) to stimulate prolactin release in adult bullfrog pituitary cells in culture (118). It thus seems that the GPHα subunit may endorse a role on lactotrope differentiation and/or in stimulating pituitary prolactin production at least in some tetrapods. It must then act on lactotrope cells by activating or antagonizing a signaling pathway that should be conserved in tetrapods. In teleost fish, effect of the GPHα subunit on prolactin production has not been looked for to my knowledge and lactotropes appear much earlier than β subunit-expressing gonadotropes and thyrotropes during pituitary ontogenesis (119). Consequently, the GPHα subunit is unlikely to play a role on lactotrope differentiation in teleosts except if, similarly as in mammals, it is produced long before the GPHβ subunits in some sort of progenitor cells, which have still to be evidenced.
The pituitary gland is not the only tissue that synthesizes prolactin (120, 121). This is also the case for the human decidual cells of the endometrium notably during pregnancy (122). The α subunit is also produced in excess (relative to the hCGβ subunit) by trophoblast cells of the placenta and is secreted in free form all along pregnancy (121, 123–125). Despite the fact that the decidual and pituitary lactotrope cells make use a different prolactin gene promoter (126), purified α subunit was shown to stimulate the release of prolactin from decidual cells isolated from term placenta at doses within the range of physiological level (127). Intact hCG was also able to elicit a response but at doses high enough for a contamination with dissociated or free α subunit to be responsible for the effect. Decidualization of endometrial stromal cells also occurs during the luteal phase of the menstrual cycle (126) and, remarkably, these decidualizing cells are able to dissociate hCG into its subunits (128). The free or dissociated α subunit acts synergistically with progesterone to stimulate the transdifferentiation of dispersed stromal cells in culture into decidual cells as monitored by morphological change and the amount of prolactin release (128, 129). The isolated α subunit was twenty times more potent than the intact hCG, with a dose-dependent, saturable and biphasic stimulation pattern (128). Myometrium also appears to be one of the multiple extrapituitary sites of prolactin production and the α subunit was demonstrated to stimulate the synthesis and secretion of prolactin from explant cultures of myometrium tissue either from pre- or post-menopausal women at equimolar concentration as intact hCG (130). It is then established that the GPH α subunit by itself is able to enhance prolactin release in different mammalian cell types in which the prolactin gene expression is controlled by different promoters. Excessive free α subunit serum level was linked to molar pregnancies (124) even if this alteration might as well be a consequence rather than a cause for this disorder.
3.1.2 GPH α Subunit in Men
In men, in addition to the pituitary, the α subunit was shown to be produced by fibroblasts in the prostate stroma (131) and in rare epithelial cells presenting neurite-like extensions organizing a network toward the lumen of the acini (132). These cells do not produce any GPHβ subunit. The free α subunit is released into the seminal fluid at extremely high levels similar to those reached in extra-embryonic coelomic fluid of pregnant women and is present mostly as monomer but also as αα homodimer or even tetramer (132). Seminal level of the free α subunit was shown to be lower in men presenting semen abnormalities, suggesting it may play a role in the latest stages of spermatogenesis (133). Also, the GPH α subunit reduced the growth of stromal smooth muscle cells and it was speculated that the decreasing production of the α subunit along ageing could favor transdifferentiation of fibroblasts into smooth muscle cells and the development of benign prostate hyperplasia (131).
3.1.3 GPH α Subunit in Cancer
Very high GPHα-subunit expression (up to thousand times its level in normal breast tissue) is detected in more than 30% of breast tumors (134). The GPHα-subunit expression is limited to tumor cells positive for the estrogen receptor α for which it could be a reliable marker of functionality. Histopathological observation of α subunit-expressing cells suggested that it could also be, together with progesterone and estrogen-receptor expression, a marker of low tumor aggressiveness (134). However, the role of the α subunit in breast cancer development has still to be unveiled.
3.2 Occurrence and Unconventional Actions of the CGβ Subunit
Unconventional, paracrine actions of hCG have been reported for more than twenty years and were recently reviewed (63, 135–137) and only effects that are not suspected to be dependent on a LH-CGR activation or to result from possible contaminants present in the preparations used will be mentioned below.
3.2.1 CGβ Subunit and Pregnancy
In addition to the heterodimeric CG, the human placenta also releases, although in much lower amount, each of its α and β subunits in free form (138). While the GPHα-subunit serum level increases continuously during pregnancy, both hCG and the free hCGβ subunit peak at 8-10 weeks of gestation (125). The ratio between βhCG and dimeric hCG was highest at the beginning of pregnancy, decreasing until 12-13 weeks when it was maintained at about 0.5% (123). In human, the placenta is characterized as hemochorial with the trophoblast entering deep inside the uterus myometrium and establishing direct contact with the maternal blood. The primitive syncytium is the first cell type that presents invasive behavior (139). The early mononucleated villous cytotrophoblast (VCT) cells are derived from the trophoectoderm and form an epithelium that covers the floating villi. They then fuse into multinucleated syncytiotrophoblast (ST) that will be renewed all along pregnancy. Later on, the highly proliferating extravillous trophoblast (eVCT) cells develop at the tip of anchoring chorionic villi, forming multi-layered columns of cells. As they further differentiate, eVCT cells break away from the column and invade decidua whereas cells in the column differentiate into giant multinucleated cells that spread to cover the embryonic sac (139–141). hCG is produced in these two different types, ST and eVCT, of trophoblast cells. Interestingly, the eVCT cells were shown to produce a hyperglycosylated form of hCG, hCG-H (142, 143). An antibody was raised, antibody B152, that selectively recognizes hCG-H but not regular hCG (144, 145). hCG-H represents the major if not the only form of hCG that circulates in the mother serum at the beginning of the first trimester of gestation (146). It is questioned whether the eVCT cells are the first cells to produce hCG-H as the primitive syncytium already has an invasive behavior and hCG-H is the major form expressed at that time of implantation (135).
Trophoblast invasion, as measured by migration of eVCT-derived HIPEC65 cells, was stimulated by eVCT-conditioned medium but not by ST-condition medium containing the same amount of hCG. The stimulatory effect was strongly decreased when hCG was depleted from eVCT-conditioned medium using an anti-hCG antibody able to recognize either or not hyperglycosylated forms of hCG (147). Since regular hCG from ST did not stimulate HIPEC65 cell migration, hCG-H from eVCT was unlikely to act through the LH-CGR (136). Moreover, hCG-H was shown to activate LH-CGR with a much lower potency than hCG (138, 148). The importance of hCG-H on trophoblast cell invasion was more recently confirmed. Firstly, treatment of first trimester placental villous tips cultured on collagen droplets with the antibody B152 significantly decreased outgrowth area. Secondly, invasion of JEG-3 choriocarcinoma cells (that produce hCG-H, see below) through a gold electrode in a Boyden chamber assay was similarly inhibited when co-incubated with the B152 antibody (149). In a different approach using JEG-3 cell migration through a basement membrane as an invasion assay, both hCG and hCG-H as well as, most importantly, their purified β subunits were shown to stimulate cell invasion (150). The isolated β subunits were shown to be unable to efficiently activate LH-CGR (148), giving strength to a mechanism of cell invasion independent on LH-CGR expression. Importantly, LH-CGR knockdown of JEG-3 cells did not alter the stimulatory effect of hCG and its β subunit on cell invasiveness. Together, these results demonstrate that hCG-H as well as the β subunit of hCG and hCG-H are able to stimulate trophoblast invasion through an LH-CGR-independent pathway. For these functions, hCG-H action can be reproduced by the β subunit alone, showing that the α subunit is not involved in its action.
Gestation implies a modification of the immune response in the mother to tolerate the blastocyst implantation. hCG was proposed to be a player in this mechanism by stimulating interleukin IL-8 production by peripheral blood mononuclear cells (151). The effect, which was significant only with high doses of recombinant hCG (more than 10 UI/ml), was prevented when the preparation was pre-incubated with an anti-hCG antibody. Intact hCG at high doses (more than 1 µg/ml) but not deglycosylated hCG nor LH or FSH, stimulated the proliferation of uterine natural killer (uNK) cells (152). The verified absence of LH-CGR expression in these two latter cell types (and the high doses required for the effect to be observed) suggested that, here again, LH-CGR-independent mechanisms had to be accounted for.
In agreement with all these LH-CGR-independent actions, LHR knocked-out mice transplanted with pieces of wild type ovary at a prepubertal stage were able to become pregnant, showing that extragonadal LHR expression was not necessary (153). Similarly in human, expression of LH-CGR in uterus or other mother tissues was shown to be dispensable for successful pregnancy. A patient with biallelic inactivating mutations of LH-CGR became pregnant using donor eggs (the actual protocol was not given) and delivered a healthy child, showing that successful implantation and termed pregnancy could be achieved in absence of conventional action of regular hCG in non-placental tissues (154). Only the embryonic tissues were able to express a functional receptor for conventional actions of hCG in this patient. On the other hand, clinical investigation demonstrated that a proportion of hCG-H relative to regular hCG under 50% on the day of implantation in urine (155) or 11 days after blastocyst transfer in serum (156) was predictive for pregnancy failures. Specifically, low hCG-H proportion during the first trimester of gestation was associated with preeclampsia, which is characterized by an impaired invasion of the cytotrophoblast into the myometrium (157). Since hCG-H is the major form of hCG produced at the beginning of pregnancy, these results are consistent with a determinant role of hCG-H specifically in the first steps of placental development, a role that is demonstrated to rely on a LH-CGR-independent mechanism. An elegant hypothesis was developed that draws a link between the successive mutations that allowed the hCGβ subunit to increase the number of its O-glycosylated residues and its biopotency during primate evolution and the depth of implantation leading to hemochorial placentation in human (63).
3.2.2 CGβ Subunit in Males
If the GPHα subunit is synthesized by the prostate gland and released as a free form in the seminal plasma, the β subunit of hCG is produced, presumably by Leydig cells, in the interstitium of the testis (132). The β-hCG is secreted as a free form and found at high level in the seminal plasma (132, 158, 159). The role of the free β-hCG has not been elucidated but a positive correlation was made between its level in semen and sperm count or total motile sperm density (158, 159).
3.2.3 CGβ Subunit and Cancer
Results reported above show that the β subunit of hCG and, particularly its hyperglycosylated form, has the capacity to stimulate cell migration and invasion and to alter immune responses. These are properties that are also necessary for cancer cell dispersion and metastasis. More specifically, the eVCT cells that form the anchoring villi share numerous similarities with cells harboring tumorigenic phenotype and the further differentiated invading eVCT cells behave more so as to metastatic cells with the notable difference that their invasive capacities are tightly regulated (140). Choriocarcinoma (including JEG-3 cells) and germ cell malignancies have been shown to produce the hyperglycosylated form of hCG, hCG-H (160). Many other cancers, notably epithelial tumors, produce the β subunit, but not the α subunit of hCG, with variable proportion of the hyperglycosylated form over the regular form (161–163). Interestingly, the β subunits of hCG and hCG-H by themselves but not regular hCG were shown to be as potent as hCG-H in promoting cell proliferation on choriocarcinoma, germ and non-germ cancer cell lines that produce at least one of these hCG forms. It clearly indicated that the effect did not require activation of LH-CGR (163). Also, purified β-hCG dose-dependently increased cell number of different bladder carcinoma cell lines, an effect that appeared to result from a reduced apoptosis rate (164). Inversely, inhibition of growth was observed in bladder cancer cell lines that produce β-hCG when an anti-β-hCG antibody was added to the culture medium (165). Similarly, knockdown by shRNA of β-hCG expression in a bladder cancer cell line resulted in significant growth inhibition (166). Also, enhanced apoptosis was obtained in uterine cervical carcinoma cells by silencing their β-hCG production (167). The involvement of β-hCG and specifically its hyperglycosylated form on tumor growth was further demonstrated using a xenograft model with JEG-3 cells transplanted into nude mice. When these transplanted mice were administered the antibody B152, oncostasis occurred whereas tumor increased in size in control mice (168).
In addition to its inhibitory effect on apoptosis, which allows multiplication of cancer cells, β-hCG expression level was positively correlated with metastatic behavior of human cancer cells transplanted into nude mice (169). It was further demonstrated that either β-hCG overexpression or incubation with purified β-hCG was able to alter the shape of two different prostate carcinoma cell lines, reduce cell adhesion and lower E-cadherin expression, a recognized suppressor of tumor invasion and metastasis (170) and stimulate their migratory and invasive capabilities (171). Pro-metastatic roles were also attributed to β-hCG in several other cancer cell lines (172–174). BRCA1 (for Breast Cancer 1) was shown to down regulate β-hCG expression in breast cancer cells so that cancer cells harboring inactivating mutation in BRCA1 have high level of β-hCG (175). In agreement with a role of β-hCG in promoting metastasis, knockdown of β-hCG in BRCA1 mutant HCC1937 cells by siRNA significantly reduced their migration and invasion capacities (175). There was no mention of the α subunit expression in this later study as in too many of the studies using supplementation, overexpression or knockdown of the β subunit. But the α subunit expression is linked to estrogen receptor α in breast tumors (134), which is absent in HCC1937 cells so that the effect cannot be attributed to the dimeric CG.
Overall, it is now admitted that β-hCG expression in cancer tissues and elevated β-hCG serum level are poor prognosis for cancer patients (176). Experiments aiming at lowering the level of circulating hCG as a reversible anti-fertility vaccine had been realized by coupling a composite CG consisting of an ovine α subunit and hCGβ subunit coupled to a toxoid to boost the immune response (177, 178). While the vaccine was highly effective in women exhibiting anti-hCG antibody, only 60-80% of women receiving the treatment generated satisfying amount of antibody (179). This however prompted experiments intended to evaluate the use of immunotherapy against β-hCG to treat β-hCG-expressing tumors. In a phase II trial, patients with colorectal cancer were treated with the specific carboxy-terminal peptide of β-hCG conjugated with diphtheria toxoid to enhance immunogenicity. Vaccination induced anti-hCG antibodies in 72% of patients and those who developed anti-hCG antibody levels above the median value had a significantly better survival rate (45 weeks vs 24 weeks) than those with levels below the median value (180). Different strategies have since been explored to use anti-β-hCG vaccination to treat β-hCG expressing cancers that aim at further enhancing the immunogenicity of the vaccine (180–190). None seemed to reach the safety or efficacy level needed to be included as a therapeutic tool.
3.3 Unconventional Actions of TSH and Its β Subunit
3.3.1 TSH From the Pars Tuberalis
As mentioned earlier, in addition to the TSH synthesized in the PD, another form of TSH is produced by cells in the PT (15). While PD-TSH is stimulated by hypothalamic TRH and submitted to a TH direct feed-back action as mentioned earlier, TSH expressing cells of the PT are devoid of TRH and TH receptors (191). PT-TSH synthesis is instead triggered by melatonin in mammals (192). The released TSH is then delivered in a retrograde pathway to activate TSHR located in the ependymal layer of the infundibular recess to participate in the regulation of reproductive function, metabolism and immune responses according to changes in day length (15, 193, 194). PT-TSH is also constitutively secreted into the general circulation but, surprisingly, this TSH has low biological activity (195). It was shown that PT-TSH binds to serum immunoglobulin IgG2b and albumin to form an extremely stable macro-TSH complex that protects it from binding to TSHR in the thyroid and reduces its degradation rate. The different behavior of the two TSHs was demonstrated to result from differences in their glycosylation status with the presence of tri-antennary and tetra-antennary N-glycans associated with sialic acid specifically on PT-TSH (195). In agreement, patients with primary hypothyroidism (who lack PD-TSH) were shown to secrete a highly sialylated TSH with low bioactivity (196). The PT-TSH extracted from the PT is able to activate the TSHR in vitro and it is the complex that it forms in the general circulation that prevents it from activating the receptor. The unconventional action in this case relies on the fact that this TSH does not have endocrine capabilities. Its retrograde pathway has not been deciphered yet (193) but it is highly unlikely that PT-TSH reaches the mediobasal hypothalamus via the blood circulation.
3.3.2 A Truncated TSHβ Subunit
A decade ago was discovered a splice variant of the tshb gene in mice (197) and human (198). This variant is made of the 3’ end of the last intron of tshb that now encodes a nine-amino acid-long stretch that presents features in agreement with a signal peptide (199) and the last exon of tsb, generating an N-terminally truncated β subunit. This protein lacks amino acids encoded by the first encoding exon and notably one each of the three paired-cysteine residues involved in the cystine-knot plus one that normally buckles the carboxyl end « seatbelt » of the β subunit around the α subunit (Figure 1). In addition, it lacks the unique glycosylation site conserved in TSHβ subunits. The cystine knot is a key feature for the folding and conformation of the GPH subunits. In addition, the carbohydrate side chain has been shown to be involved in storage and secretion of the dimer, in receptor binding and activation and in metabolic clearance of GPHs (7). It seems thus very unlikely that this truncated variant may associate with an α subunit to generate a dimer able to activate the TSHR. Yet, molecular dynamics simulation of monomeric TSHβ subunits in complex with the extracellular domain of the TSHR seems to indicate that the full-length β subunit and the truncated peptide dock in a rather similar way (200, 201). And accordingly, both forms were shown to elicit a response as recombinant monomers on cells expressing the TSHR (197, 201, 202). But for the TSHβ variant to activate the receptor, it needs to be secreted and that was not shown for the recombinant variant peptide as a monomer (201). Release of the TSHβ variant was reported from naturally expressing cells or transfected cells but the released amount appeared to be very low. It was shown that TSHβ variant is expressed in pituitary (at very low level compared with full length βTSH), in splenic and peripheral blood leucocytes, bone marrow hematopoietic cells and bone marrow-derived macrophages (197, 198, 202–204). Bone marrow cells were shown to express very little amount of the GPHα subunit (198) if any (202). Even if some role has been attributed to the truncated variant from macrophages in osteoprotection (202), in immune response with the variant expressed in leucocytes homing to the thyroid after systemic bacterial infection (199, 203) and in the pathology of Hashimoto’s Thyroiditis patients (205), the way this variant may achieve these functions remains to be deciphered. It has to be reminded that the TSHβ variant consists of part of the last intron directly attached to the last exon of the tshb. So that the PCR-amplified product from cDNA cannot be distinguished from that obtained from genomic DNA. The mRNA preparation must then be exempt of genomic DNA contamination. In addition, no specific antiserum was raised against the variant so that the immunologically detected protein using anti-TSH antibody (in cell sorting or immuno-histochemistry, for example) also recognizes genuine TSH. It will then be determinant for future studies to at least raise a specific antiserum against this variant. The pituitary cells expressing the TSHβ variant were not identified but as TH treatment induces a decrease in its pituitary expression (200) and as PT thyrotrope cells do not express TH receptors (191), the TSHβ variant is most likely produced by PD thyrotrope cells.
3.4 GPA2 and GPB5: Individual Paracrine Factors or Heterodimeric Hormones?
The thyrostimulin concept for the GPA2/GPB5 heterodimer was proposed on the basis of its highly efficient activation of mammalian TSHR (72). However, GPA2/GPB5 heterodimer and TSH appeared to dock to partially overlapping sites on the TSHR and large excess of TSH could not displace the GPA2/GPB5 heterodimer when this later was much more potent than TSH to displace the binding of TSH (69). Mammalian gonadotropins have also been found to potentially activate TSHR even if only the high plasma levels that hCG reaches at the first trimester of pregnancy (or in hCG-expressing cancer patients) have physiological impact on TH production by the thyroid (206). In addition, TSHR is unique among GPHRs in that it is submitted to several post-translational modifications including possible cleavage of a 50 amino acid-long segment of the region linking the extracellular domain to the transmembrane domain, with only 3 disulfide bondings for the extracellular domain to be kept attached to the rest of the receptor (207, 208). The cleavage and possible detachment of the extracellular domain may induce the generation of autoantibodies that can have either stimulating or blocking impact on TSHR activity causing thyroid pathologies like Graves disease (209). It indicates that TSHR, in mammals at least, is more permissive than the other GPHRs to alternative ligand-induced activation. However, in the elephant shark, a cartilaginous fish species, the recombinant GPA2/GPB5 dimer does not bind to homologous TSHR (or any homologous GPHR) expressed on mammalian cells (210). It thus remains to be investigated whether the thyrostimulin concept holds in other groups of vertebrates. In addition, for the TSHR to be activated by the GPA2/GPB5 dimer in mammals, the dimer has to be available. But, likely because of the shorter carboxy-terminal end of GPB5 compared to GPHβ subunits and the absence of the « seatbelt » mechanism (70) the stability of the GPA2/GPB5 heterodimer was demonstrated to be far much labile than for the GPHs (72, 211). Consequently, a GPA2/GPB5 dimer seems more likely to act as an autocrine or paracrine factor than as an endocrine factor, whatever the target receptor may be. Interestingly, the tissue distribution of GPB5 was shown to be far less extended than for GPA2 in human and rat (67, 69, 72, 212, 213). GPA2 and GPB5 may be both expressed in a number of tissues but the pituitary gland was the only tissue in which co-expression of GPA2 and GPB5 has been observed within the same cells to date. However, the cell type involved is not very clear yet: corticotrophs in human (72), unidentified cells but not corticotrophs in rat (69). In addition, the GPA2 mRNA expression level was shown to be up to 100 times higher than that of GPB5 in tissues that expressed the two genes (69, 213). No reliable antiserum has been raised against the GPA2/GPB5 heterodimer or the monomeric glycoproteins allowing quantitation so that the tissue concentrations at the protein level cannot be compared. The difference in tissue distribution however strongly suggests that individual subunits rather than heterodimers may be the real players in most tissues.
GPA2 and GPB5 are both glycosylated in vertebrates and mutation experiments on both glycoproteins have shown that, as for the classical GPHs, the presence of the carbohydrate chains impact on secretion and activation abilities of the dimer (211). In contrast, the number and position of potential N-linked glycosylated chains are highly variable in invertebrate GPA and GPB proteins. In most hexapods, either GPA or GPB are devoid of potential N-glycosylation site (82). Remarkably, in sea hare or tick, none of the proteins displays a potential site. This questions the ability of these proteins to be secreted. In addition, GPA and GPB may show differential expression patterns, notably during development (82, 214, 215). As mentioned above, fly GPA/GPB heterodimer activates fly LGR-1 to stimulate cAMP production (85). Recent research performed in mosquito demonstrated that recombinant GPA and GPB form heterodimers but only when proteins are attached to each other by a linker segment. Unexpectedly, incubation with crude cellular extracts from cells expressing the tethered proteins led to a switch from a constitutive activity to an inhibition of the mosquito LGR-1 as measured by the cAMP level (216). The conditioned medium had no effect, suggesting the dimer is poorly released or that the biological activity of the secreted dimer is prevented by factors present in the medium. It has to be noted also that a recombinant tethered amphioxus GPA/GPB heterodimer was able to activate the amphioxus LGR-1 receptor (217) but only at very high doses (100 to 1000 ng/ml), hardly compatible with an endocrine effect.
4 Proposed Mechanisms of Action for the hCGβ Subunit
Both GPH α subunit and hCGβ subunit are able to induce a cellular response by themselves without the need to be in a heterodimeric form. None of the subunits are able to efficiently activate any GPHR (1, 148) and some of the cellular responses have been obtained on cells that do not express LH-CGR. Both GPHα (105, 106) and hCGβ subunits (218) were shown to form homodimers. The glycosylation pattern of uncombined α subunit differs from that of the dissociated α subunit (105). Whether the free α subunit is acting as a monomer or a homodimer has not been clarified and no mechanism of its action has been proposed yet. In contrast, the monomeric recombinant hCGβ subunit was shown to be as active as the homodimeric ββ-hCG in stimulating bladder cancer cell growth (219). It thus appears that hCG-H and the β subunits of hCG or hCG-H either as monomer or homodimer exhibit activities that are not reproduced by the regular heterodimeric hCG. Obviously, the hyperglycosylated state of the β subunit in hCG-H reveals a structure that is buried inside the regular hCG but seemingly accessible in the free β subunits. And accordingly, the hCG-H-specific antibody B152 recognizes a core-2 O-glycan structure on a specific serine residue and surrounding residues in the carboxy-terminal end of β-hCG-H that does not appear accessible in regular heterodimeric hCG (145).
4.1 β-hCG-H Alters TGFβ Signaling
The GPH α- and β-subunit structure is characterized by a cystine-knot core motif that has the same general characteristics as in the TGFβ family of peptides (5, 6). This super-family of cystine-knot growth factors (CKGFs) includes five groups of factors in vertebrates : 1- TGFβs together with bone morphogenic factors (BMPs), growth and differentiation factors (GDFs) and activin-inhibin subunits, 2- nerve growth factors (NGF), brain-derived neurotrophic factor (BDNF) and neurotrophic factors 3 and 4 (NTF3, NTF4), 3- platelet-derived growth factors (PDGF), 4- vascular endothelial growth factors (VEGF) and 5- the DAN (differential screening-selected gene aberrant in neuroblastoma) or CAN (Cerberus and Dan) family of BMP antagonists (68). A sixth group of CKGFs, bursicon α and β subunits, has been lost in chordates (see below). Apart from BMP antagonists of the DAN/CAN family, CKGFs play their role through activation of kinase receptor complexes (220). Usually, CKGF dimers bind to a family-specific type-II receptor, a transmembrane receptor with intrinsic serine-threonine kinase activity that, upon binding, recruits and phosphorylates a given type-I receptor of the activin-receptor-like kinase (ALK) type that ultimately phosphorylates one of the SMAD (Similar to Drosophila Mothers Against Decapentaplegic) proteins (220). In addition to the SMAD pathway, TGFβ is also able to act through the extracellular signal-regulated kinase (ERK)/Akt pathway (221). Among these CKGFs, TGFβ is known to be deregulated in many cancers and have dual role during cancer progression. In early stages like in healthy cells, TGFβ promotes cell-cycle arrest and apoptosis and thus plays a tumor-suppressor role. However, in later stages, highly activated TGFβ signaling favors tumorigenesis and metastasis by activating an epithelial-to-mesenchymal transition causing cell evasion and by suppressing immune response (222). These latter properties recall those described for hCG-H or its β subunit and it was suggested that hCG-H or β-hCG could act by interfering with the TGFβ signaling (164). In support, TGFβ type II-receptor was shown to be co-precipitated with an anti-hCGβ subunit antibody from the breast cancer HCC1937 cell line (175). It was shown that overexpression of β-hCG in ovarian cancer cells or addition of purified β-hCG into their culture medium was able to activate ERK independently on LH-CGR presence (223), supporting a possible involvement of TGFβ signalization in β-hCG action. Similar effects of hCG on ERK were demonstrated in various cancer cells (174, 224, 225). However, in many instances, TGFβ-like effects described after exogenous hCG treatments may have been misinterpreted as many preparations of hCG or its β subunit were shown to be contaminated with co-purified TGFβ (226) or with other growth factors like EGF (227), which is also demonstrated to stimulate trophoblast invasion (228). In addition, TGFβ was shown to oppose rather than to mimic the effect of recombinant hCGβ subunit on carcinoma cell apoptosis (164). Moreover, opposite to hCG (149), TGFβ was demonstrated to dose-dependently inhibit the invasive capacity of extravillous trophoblast cells isolated from placental explants at 8-10 weeks of gestation (229, 230). Thus, hCG would better fit an antagonistic role on TGFβ signaling. More work is needed to figure out how hCG-H or the hCGβ subunit may interfere with TGFβ signaling with both similar and opposite effects.
4.2 Other Proposed Mechanisms
It was also proposed that hCG could enhance trophoblast invasion by altering the insulin-like growth factor II (IGFII)/mannose 6-phosphate receptor (M6PR) recycling, allowing action of IGFII (231). IGFII is abundantly synthesized by trophoblast cells (232) and was shown to activate trophoblast invasion via IGFII/M6PR (233). The mechanism of how hCG could act on receptor recycling was not deciphered. Another again mechanism was proposed for the stimulation of proliferation of uNK cells by hCG (152). It was shown that the hCG action was prevented when deglycosylated hormone was used or when cells were incubated in the presence of excess mannose whose receptor was co-localized with hCG on the cell surface. Similarly, the stimulating effect of hCG on IL8 production by peripheral blood mononuclear cells was attenuated in the presence of excess mannose, suggesting here again that hCG could bind to C-type lectins expressed on cell surface (151). Such a type of interaction would make sense when considering the highly glycosylated form of hCG-H that is expressed at time of implantation and invasion and that is responsible for the LH-CGR-independent action of hCG. However, since the β subunit of regular hCG is equally active as its hyperglycosylated form in promoting cancer cell proliferation (163), other mechanisms have to be looked for. Also, it has to be reminded that for both effects on uNK cell proliferation and peripheral blood mononuclear cells, very high levels of hCG were needed so it has to be demonstrated that such high levels are reached at the site of implantation.
5 Phylogenetic Studies Open Up Ways for Alternative Modes of Action for the Individual GPH-Related Proteins
The closest phylogenetically relatives for GPA and GPB (see Figure 2) were identified as the CKGF members bursicon, burs (or bursicon α) and pburs (for partner of burs, or bursicon β) (68) that heterodimerize to activate a type-B LGR (LGR-2) (234). Both bursicon subunit genes have disappeared from chordate genomes whereas genes for three vertebrate type-B LGR receptors, lgr4-6 are found (84, 215). In absence of bursicon these receptors were first thought to be orphan in chordates. The three of them were later found to bind with high affinity all four R-spondin members which are potent Wnt enhancers (235). This is an example of molecular exaptation: a receptor has lost its natural ligand but a new ligand comes and uses the receptor. It is to be noted that, in addition to the change in ligand, LGR4-6 also change their signalization as binding of R-spondins on LGRs does not activate protein G-induced signalization (235). Bursicon burs/pburs heterodimer is involved in cuticle melanization and sclerotization after molting and in adult wing expansion in insects (234). Interestingly, bursicon subunits can also associate as burs/burs and pburs/pburs homodimers and both homodimers were shown to stimulate the secretion of several anti-microbial proteins, independently on LGR-2 (236, 237). It thus appears that the ability of homodimers to activate a different signaling system as the heterodimeric ligand does not seem restricted to the GPH-GPHR system.
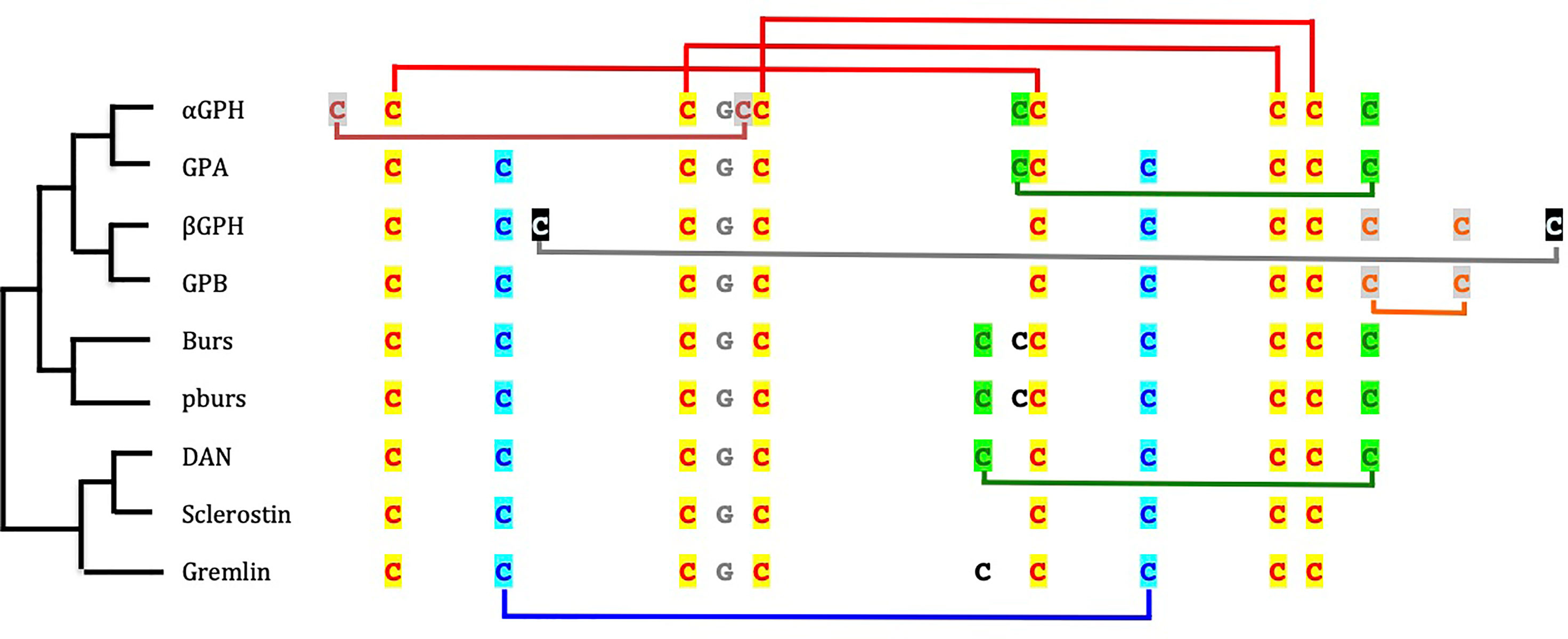
Figure 2 Cysteine residue arrangement of glycoprotein hormone (GPH) subunits and cystine-knot growth factors. The phylogenetic relationships [after (67)] between sequence types are schematically represented on the left side. Disulfide bridges between cysteine residues involved in the cystine-knot are depicted in red on top of the sequences. The other cysteine residues linked by disulfide bridges are given in different colors according to their position in the folded protein. The cysteine residues in black are not involved in intramolecular bridge. The glycine residue conserved in all cystine-knot growth factor is indicated in grey. GPA and GPB are the precursor forms in invertebrates of GPH α- and GPHβ subunits, respectively; Burs is for bursicon α and pburs for bursicon β; DAN is for differential screening-selected gene aberrant in neuroblastoma.
The next closest phylogenetically relative in line (68) appears to be the DAN-CAN family of BMP antagonists (Figure 2). These antagonists were also shown to have the closest structural relationships with the GPH subunits in terms of cysteine residues arrangement (70, 238). This family includes 1- Dan or Nbl1 (for neuroblastoma suppression of tumorigenicity 1), 2- Gremlin, Cerberus, PRDC (protein related to DAN and Cerberus) and Coco/Dante that form a subgroup and 3- SOST (Sclerostin) and USAG-1 (Uterine sensitization-associated gene-1) gathered into another subgroup (239). These proteins are known to be highly expressed and involved during development. Except for SOST and USAG-1, which are active as monomers, DAN family members act as non-covalently linked homodimers. Most of them were shown to bind to selected BMP-GDF members to prevent them from activating their receptor. Gremlin additionally interacts with VEGF receptor 2 whereas SOST and USAG-1 antagonizes Wnt signaling by binding to co-receptors (239, 240). A tentative modeling of their interaction with BMP members has been proposed showing two homodimers of the antagonist binding perpendicularly at each tip of the elongated growth factor (239). So, the secreted peptides of the DAN family of BMP antagonists are able to play their role without activating a specific receptor. Interestingly, some of the DAN family members have been shown to harbor several accessible and effective sulfated polysaccharide heparin and heparan-sulfate binding sites (241). Binding of these factors to heparan-sulfate proteoglycans of the extracellular matrix or to cell surface hampers their diffusion away from the site of secretion and contribute to reaching and maintaining the concentration needed for their action. Whether such sites also exist for the GPH individual subunits and related proteins remain to be explored. Nonetheless, the close structural similarity between GPH-related subunits and these family of antagonists, their ability to form homodimers and their apparent absence of specific receptors open up new exploratory fields for the mechanistic study of their actions. It would be interesting, for example, to search for cystine-knot factors with which GPH subunits could interact and if these interactions could be related to some of their actions.
6 Evolutionary Considerations
In invertebrate genomes, the genes encoding GPA and GPB are most commonly found close to each other without intervening gene (68, 82), suggesting that the physical promiscuity between these two genes is under evolutionary pressure. Furthermore, no species has been reported that lacks only either GPA or GPB but some invertebrates, notably among hymenoptera like the parasitic wasp (242), honey and bumble bees (243) and several ants (244), but also the unrelated leech (245) and myriapod (246, 247), appear to lack both GPA and GPB and, interestingly, the LGR-1-type receptor also. The absence of the receptor gene but presence of GPA and GPB genes has not been reported to date. This strongly suggests that the evolutionary conservation of these two proteins and the receptor are interdependent. One would indeed expect that if GPA or GPB had determinant individual LGR-1-independent roles, it should have been conserved even in the absence of its heterodimeric partner or the receptor for the heterodimer. So, it looks like, contrary to what was expected from available functional and expression distribution data, a possible receptor-independent individual role for GPA and GPB would not be the evolutionary drive for their conservation.
Interestingly, the tandem organization of GPA and GPB genes observed in most invertebrate species was maintained before the duplication of the locus just prior to the vertebrate emergence as evidenced by comparative synteny analysis between vertebrate and amphioxus (a pre-vertebrate chordate) genomes (2). The two rounds of whole genome duplications (1R and 2R) that occurred before the radiation of vertebrates (248) led to the conservation of only two GPB5-related genes and one GPA2 gene in extent vertebrates (Figure 3). One of the two GPB5 genes (GPB5a) was maintained close to the unique GPA2 gene (2, 249), indicating that the evolutionary constraints that maintained this genes in a tight locus in invertebrates were still probably in place in early vertebrates. No additional GPB-related genes were conserved after the third, teleost-specific whole genome duplication (3R). The GPB5a form was lost in tetrapods but is still present, close to GPA2 in coelacanth (which emerged just prior to the tetrapod radiation) and in teleosts (249).
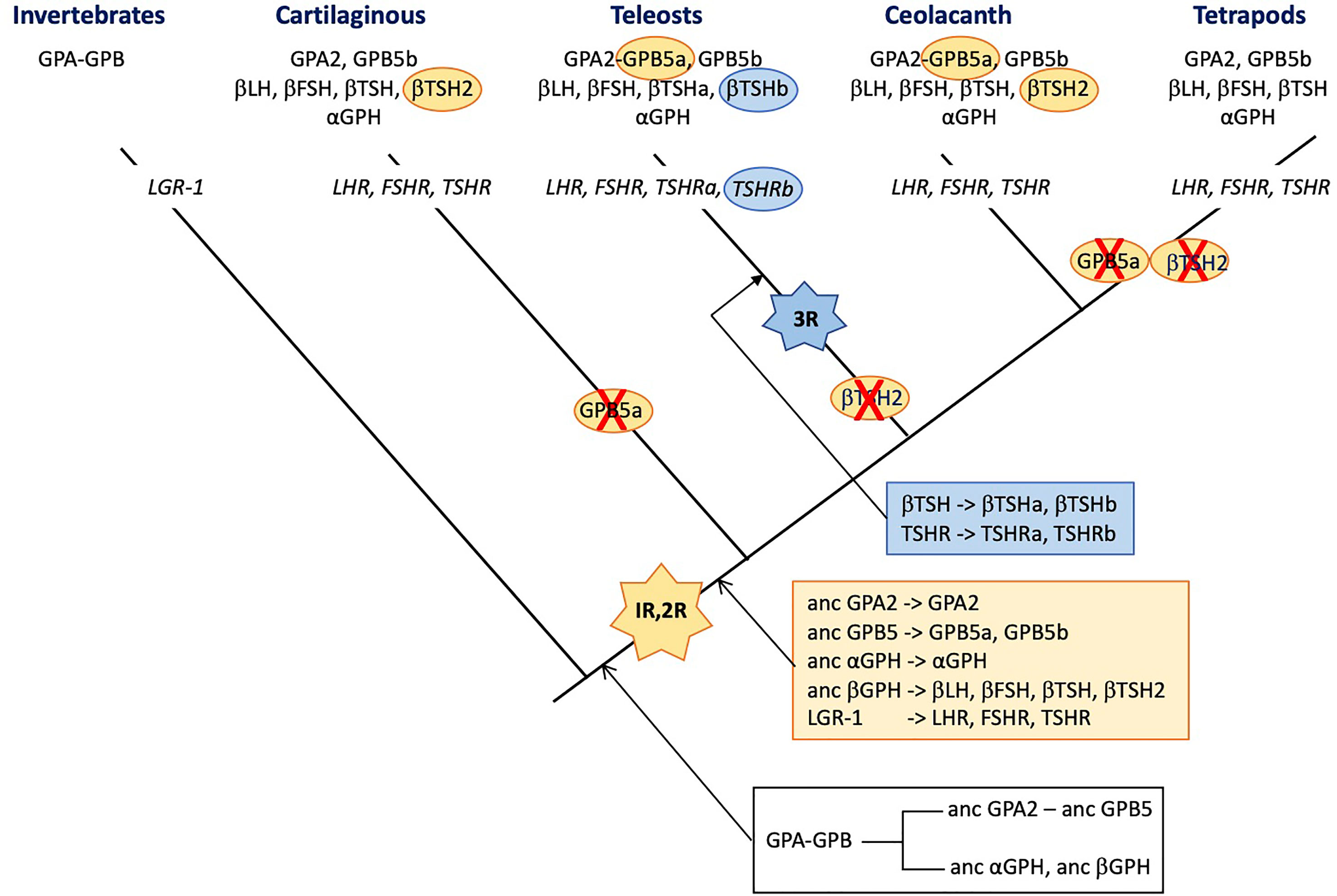
Figure 3 Schematic representation of the distribution of glycoprotein hormone (GPH) subunit-related genes and receptors (in italic) presented on a simplified phylogenetic tree. The initial tandem duplication of GPA and GPB giving rise to the ancestral forms of GPA2 and GPB5 and of α- and β-GPH just prior to the vertebrate expansion is indicated in the black box underneath the tree. The genes issued from the two rounds of genomic duplication that occurred in founder vertebrates are depicted in the orange box. Those issued from the teleost-specific third round of genomic duplication are presented in the blue box. The hyphen in GPA-GPB and GPA2-GPB5a indicates that the genes are organized in tandem. The red cross indicates loss of the gene. 1R, 2R and 3R represent whole genome duplication events. LH, luteinizing hormone; FSH, follicle stimulating hormone; TSH, thyroid stimulating hormone; GPA, invertebrate representative of the precursor for vertebrate GPA2 and GPH α subunit; GPB, invertebrate representative of the precursor for vertebrate GPB5 and GPHβ subunits. GPA2 and GPB5, vertebrate representatives of GPA and GPB, respectively; LGR, leucine-reach repeat receptor; LHR, FSHR and TSHR, receptors for LH, FSH and TSH, respectively.
Usually, gene duplicates accumulate detrimental mutations leading to pseudogenization within few million years. Advantageous mutations conferring new functions to the duplicate can exert positive selection allowing the duplicate gene to be maintained for new functions (neofunctionalization) or to take in charge parts of the ancestral gene function (subfunctionalization) (250). There are no vertebrate species lacking any of the GPH subunits or GPH receptors. However, the duplication of the locus comprising GPA and GPB just prior to the vertebrate emergence that generated the precursors of GPA2, GPB5 and GPH α and β subunits, and the two rounds of whole genome duplications (1R and 2R) that occurred in founder vertebrates may have open opportunities for new roles to be acquired by the generated paralogs (250). According to the current hypothesis (2, 3), the ancestral GPHβ subunit generated four paralogs through the 1R and 2R, the β subunits of LH and FSH on the one hand and of TSH and its sister gene TSH2 on the other hand (251) (see Figure 3). LH-, FSH- and TSH-β subunits allowed new functions to be acquired by combining to the GPHα subunit as heterodimers and their encoding gene was maintained. The TSH2β subunit sequence is well conserved with only few amino acid replacements and shows the normal cysteine arrangement and unique glycosylation site as compared to the classical βTSH, suggesting it can form heterodimers with the GPH α subunit. This βTSH2-encoding gene was lost in tetrapods and before the radiation of teleosts but is still present in cartilaginous fish, the coelacanth and the dipnoi (another group that emerged just before the terrestrialization). The duplication of tshβ was not accompanied by a duplication of the tshr (or the duplicated receptor was not maintained), so it is tempting to assume that TSH2 did not acquire a specific role, different from the classical TSH, that would have given a driving force for its evolutionary conservation. In agreement, the elephant shark recombinant TSH2 activated the shark TSHR only when the two subunits were linked to each other (210), questioning the stability of the TSH2β/GPHα heterodimer and the possible role and mode of action of TSH2. No data are yet available for coelacanth or dipnoi TSH2.
Interestingly, after the 3R that occurred just prior to the radiation of teleosts, among the GPHβ subunits, only the duplicated tshb gene was maintained. According to the nomenclature used in post 3R teleosts, they are referred to as tshbb (encoding βTSHb) and tshba (encoding the classical βTSHa). The gene tsh2b had disappeared before the radiation of teleosts (249). In the pituitary, the teleost βTSHb appears to be synthesized in a different area as βTSHa, in few cells close to the pituitary stalk, a location that is somewhat similar to the PT in mammals (252). They are submitted to differential regulation of expression and notably, CRH stimulates the TSHa β subunit whereas it has no effect on βTSHb. The similarity with the mammalian PT-TSH is reinforced by the role that TSHb appears to play in the regulation of physiological functions according to the photoperiod (253). TSHb but not TSHa was also shown to be involved in the metamorphic events determinant for the pre-adaptation of salmon to the sea water environment (252) and in the adaptation to different ecosystems in stickleback (254). This time, the 3R-issued duplicated tshr gene was also maintained (251) so that teleosts are provided with two TSHR (TSHRa and TSHRb). The new tshrb was translocated to a new genomic environment in teleosts soon after its duplication and is thus susceptible to be submitted to new cis-regulatory factors (251). In accordance, the tissue distribution of the novel teleost TSHRb was shown to be much more extended than for the classical TSHRa and notably encompass several brain areas where TSHb is also expressed (251). This pleads for a possible paracrine action of the novel TSHb. It is not known whether both teleost TSH can activate both teleost TSHR. It is to be noted that βTSHb acquired a second glycosylation site, at the same position as in βLH so that βTSHb shares the same two glycosylation site positions as in βFSH. Whether this second glycosylation site would alter the binding specificities of TSHb remains to be explored. The conservation of the duplicated TSH-TSHR system seems to indicate that it has acquired an advantageous function that allowed it to be maintained during evolution of teleosts. It is thus tempting to assume that teleosts and mammals have opted for different ways to divide the different TSH functions (subfunctionalization). They both produce TSH in different cell types allowing differential regulation but in teleosts, a second and possibly specific TSH receptor was used whereas in mammals, the photoperiodic-related PT-TSH was modified so that it could not interfere with the homeostasis-controlling PD-TSH. It would be interesting to look for a possible paracrine role of TSH2 in the regulation of photoperiod or other environment-related adaptations in cartilaginous fish.
Ancestral gpa2 and cga genes are the only genes for which none of the whole genomic duplications either at the root of vertebrates (1R and 2R) or before the radiation of teleosts (3R) generated duplicates that were conserved to extant vertebrates (Figure 3). Some species in teleosts do have duplicated genes for the GPH α subunit but phylogenetic analysis showed that they resulted from relatively recent and independent pseudo-tetraploidization events (255). The whole genomic duplications generated five GPHβ subunits (the β subunits of LH, FSH, TSH, TSH2 and of the teleost TSHb). LH, FSH and TSH (and TSHb) acquired specific functions and were maintained (neofunctionalization). It can be hypothesized that TSH2 endorsed only parts of the function (subfunctionalization) of the ancestral TSH issued from the 1R. When this subdivision of the function was not advantageous, the TSH2 β subunit-encoding gene evolved into pseudogenization and disappeared from the genomes of descendant lineages. Given that αGPH has to bind to each of these newly generated β subunits (including β-hCG in primates), one can wonder why the αGPH sister genes have never been conserved. This is all the more surprising as specific functions are now attributed to the individual α subunit. Neo- or subfunctionalization should have represented strong driving forces on the GPH α subunit and would have favored the α GPH duplicates to be maintained. It thus appears that the strongest conservative driving forces would be related to the heterodimerization process which imposes the same constraints on the GPH α subunit whatever the newly duplicated GPHβ subunit in order to generate a heterodimer able to bind and activate a related receptor. The functions that the GPH α subunit may play as monomer or homodimer would not exert strong-enough driving forces for the conservation of a duplicated, neofunctionalizing form. The new CGβ subunit that appeared with the radiation of primates evolved by enriching its glycosylation pattern allowing a deeper implantation and better oxygenation of the fetus blood (63). Here, the neofunctionalization is in progress with the conservation of an increasing number of CGβ subunit genes.
7 Conclusion
Individual actions have been clearly demonstrated for the GPH α subunit in mammals as well as for the hCGβ subunit in human. The mechanisms of these actions have not been deciphered yet but it is demonstrated that the receptors for the GPH heterodimers are not involved. In invertebrates, GPA, GPB and the GPHR together appear to be under selective evolutionary pressure but individual roles for GPA and GPB can also be predicted. In vertebrates, the thyrostimulin concept of GPA2/GPB5 heterodimer activating the TSHR does not seem to be the driving force for their evolutionary conservation notably as it does not function in a cartilaginous fish. Individual actions are then to be looked for each GPA2 and GPB5 proteins also. Paracrine or autocrine actions rather than endocrine actions are favored for all these factors. Individual functions of the GPH subunits have been shown to be related to modifications of their glycosylation status. For the α subunit specific glycosylation seems to favor homodimerization. The heavily glycosylated chain in hCG exposes specific sites favoring actions via the individual β subunit. Also, the PT-TSH-specific glycosylation pattern prevents it from activating the undesired receptor. Close structural relationships have been established between GPH subunits and CKGFs and particularly with members of the DAN family of BMP antagonists that act in a receptor-independent manner. This should stimulate new approaches for future investigations on the mechanisms of receptor-independent actions of individual GPH subunits.
Author Contributions
The author confirms being the sole contributor of this work and has approved it for publication.
Conflict of Interest
The author declares that the research was conducted in the absence of any commercial or financial relationships that could be construed as a potential conflict of interest.
Publisher’s Note
All claims expressed in this article are solely those of the authors and do not necessarily represent those of their affiliated organizations, or those of the publisher, the editors and the reviewers. Any product that may be evaluated in this article, or claim that may be made by its manufacturer, is not guaranteed or endorsed by the publisher.
Acknowledgments
I gratefully acknowledge Pr Joëlle Cohen-Tannoudji and Drs Sylvie Dufour, Karine Rousseau and Raymond Counis for enlightening discussions and for their critical reading of the manuscript.
References
1. Pierce JG, Parsons TF. Glycoprotein Hormones: Structure and Function. Annu Rev Biochem (1981) 50:465–95. doi: 10.1146/annurev.bi.50.070181.002341
2. Dos Santos S, Mazan S, Venkatesh B, Cohen-Tannoudji J, Quérat B. Emergence and Evolution of the Glycoprotein Hormone and Neurotrophin Gene Families in Vertebrates. BMC Evol Biol (2011) 11:332. doi: 10.1186/1471-2148-11-332
3. Dufour S, Quérat B, Tostivint H, Pasqualini C, Vaudry H, Rousseau K. Origin and Evolution of the Neuroendocrine Control of Reproduction in Vertebrates, With Special Focus on Genome and Gene Duplications. Physiol Rev (2020) 100:869–943. doi: 10.1152/physrev.00009.2019
4. Levavi-Sivan B, Bogerd J, Mañanós EL, Gómez A, Lareyre JJ. Perspectives on Fish Gonadotropins and Their Receptors. Gen Comp Endocrinol (2010) 165:412–37. doi: 10.1016/j.ygcen.2009.07.019
5. Lapthorn AJ, Harris DC, Littlejohn A, Lustbader JW, Canfield RE, Machin KJ, et al. Crystal Structure of Human Chorionic Gonadotropin. Nature (1994) 369:455–61. doi: 10.1038/369455a0
6. Wu H, Lustbader JW, Liu Y, Canfield RE, Hendrickson WA. Structure of Human Chorionic Gonadotropin at 2.6 Å Resolution From MAD Analysis of the Selenomethionyl Protein. Structure (1994) 2:545–58. doi: 10.1016/S0969-2126(00)00054-X
7. Hearn MTW, Gomme PT. Molecular Architecture and Biorecognition Processes of the Cystine Knot Protein Superfamily: Part I. The Glycoprotein Hormones. J Mol Recognit (2000) 13:223–78. doi: 10.1002/1099-1352(200009/10)13:5<223::AID-JMR501>3.0.CO;2-L
8. Xing Y, Myers RV, Cao D, Lin W, Jiang M, Bernard MP, et al. Glycoprotein Hormone Assembly inn the Endoplasmic Reticulum: III. The Seatbelt and Its Latch Site Determine the Assembly Pathway. J Biol Chem (2004) 279:35449–57. doi: 10.1074/jbc.M403054200
9. Combarnous Y. Molecular Basis of the Specificity of Binding of Glycoprotein Hormones to Their Receptors. Endocr Rev (1992) 13:670–91. doi: 10.1210/edrv-13-4-670
10. Vassart G, Pardo L, Costagliola S. A Molecular Dissection of the Glycoprotein Hormone Receptors. Trends Biochem Sci (2004) 29:119–26. doi: 10.1016/j.tibs.2004.01.006
11. Jiang X, Liu H, Chen X, Chen PH, Fischer D, Sriraman V, et al. Structure of Follicle-Stimulating Hormone in Complex With the Entire Ectodomain of Its Receptor. Proc Natl Acad Sci USA (2012) 109:12491–6. doi: 10.1073/pnas.1206643109
12. Jiang X, Dias JA, He X. Structural Biology of Glycoprotein Hormones and Their Receptors: Insights to Signaling. Mol Cell Endocrinol (2014) 382:424–51. doi: 10.1016/j.mce.2013.08.021
13. Mollard P, Hodson DJ, Lafont C, Rizzoti K, Drouin J. A Tridimensional View of Pituitary Development and Function. Trends Endocrinol Metab (2012) 23:261–9. doi: 10.1016/j.tem.2012.02.004
14. Le Tissier PR, Hodson DJ, Lafont C, Fontanaud P, Schaeffer M, Mollard P. Anterior Pituitary Cell Networks. Front Neuroendocrinol (2012) 33:252–66. doi: 10.1016/j.yfrne.2012.08.002
15. Yasuo S, Korf HW. The Hypophysial Pars Tuberalis Transduces Photoperiodic Signals via Multiple Pathways and Messenger Molecules. Gen Comp Endocrinol (2011) 172:15–22. doi: 10.1016/j.ygcen.2010.11.006
16. Fauquier T, Guérineau NC, McKinney RA, Bauer K, Mollard P. Folliculostellate Cell Network: A Route for Long-Distance Communication in the Anterior Pituitary. Proc Natl Acad Sci USA (2001) 98:8891–6. doi: 10.1073/pnas.151339598
17. Bousfield GR, Perry WM, Ward DN. Knobil E, Niell JD, editors. The Physiology of Reproduction. New York: Raven Press. (1994) p. 1749–92.
18. Vassart G, Dumont JE. The Thyrotropin Receptor and the Regulation of Thyrocyte Function and Growth. Endocr Rev (1992) 13:596–611. doi: 10.1210/edrv-13-3-596
19. Blair HC, Wells A, Isales CM. Pituitary Glycoprotein Hormone Receptors in Non-Endocrine Organs. Trends Endocrinol Metab (2007) 18:227–33. doi: 10.1016/j.tem.2007.06.001
20. Williams GR. Extrathyroidal Expression of TSH Receptor. Ann Endocrinol (Paris) (2011) 72:68–73. doi: 10.1016/j.ando.2011.03.006
21. Zaidi M, New MI, Blair HC, Zallone A, Baliram R, Davies TF, et al. Actions of Pituitary Hormones Beyond Traditional Targets. J Endocrinol (2018) 237:R83–98. doi: 10.1530/JOE-17-0680
22. Shupnik MA, Ridgway EC, Chin WW. Molecular Biology of Thyrotropin. Endocr Rev (1989) 10:459–75. doi: 10.1210/edrv-10-4-459
23. Kaiser UB, Jakubowiak A, Steinberger A, Chin WW. Differential Effects of Gonadotropin-Releasing Hormone (GnRH) Pulse Frequency on Gonadotropin Subunit and GnRH Receptor Messenger Ribonucleic Acid Levels In Vitro. Endocrinology (1997) 138:1224–31. doi: 10.1210/endo.138.3.4968
24. Coss D. Regulation of Reproduction via Tight Control of Gonadotropin Hormone Levels. Mol Cell Endocrinol (2018) 463:116–30. doi: 10.1016/j.mce.2017.03.022
25. Stamatiades GA, Kaiser UB. Gonadotropin Regulation by Pulsatile GnRH: Signaling and Gene Expression. Mol Cell Endocrinol (2018) 463:131–41. doi: 10.1016/j.mce.2017.10.015
26. Haisenleder DJ, Dalkin AC, Ortolano GA, Marshall J, Shupnik MA. A Pulsatile Gonadotropin-Releasing Hormone Stimulus is Required to Increase Transcription of the Gonadotropin Subunit Genes: Evidence for Differential Regulation of Transcription by Pulse Frequency In Vivo. Endocrinology (1991) 128:509–17. doi: 10.1210/endo-128-1-509
27. Thackray VG, Mellon PL, Coss D. Hormones in Synergy: Regulation of the Pituitary Gonadotropin Genes. Mol Cell Endocrinol (2010) 314:192–203. doi: 10.1016/j.mce.2009.09.003
28. Shupnik MA, Greenspan SL, Ridgway EC. Transcriptional Regulation of Thyrotropin Subunit Genes by Thyrotropin-Releasing Hormone and Dopamine in Pituitary Cell Culture. J Biol Chem (1986) 261:12675–9. doi: 10.1016/S0021-9258(18)67144-3
29. Galas L, Raoult E, Tonon M-C, Okada R, Jenks BG, Castaño JP, et al. TRH Acts as a Multifunctional Hypophysiotropic Factor in Vertebrates. Gen Comp Endocrinol (2009) 164:40–50. doi: 10.1016/j.ygcen.2009.05.003
30. De Groef B, van der Geyten S, Darras VM, Kühn ER. Role of Corticotropin-Releasing Hormone as a Thyrotropin-Releasing Factor in Non-Mammalian Vertebrates. Gen Comp Endocrinol (2006) 146:62–8. doi: 10.1016/j.ygcen.2005.10.014
31. Chiamolera MI, Wondisford FE. Thyrotropin-Releasing Hormone and the Thyroid Hormone Feedback Mechanism. Endocrinology (2009) 150:1091–6. doi: 10.1210/en.2008-1795
32. Pazos-Moura CC, Ortiga-Carvalho TM, Gaspar de Moura E. The Autocrine/Paracrine Regulation of Thyrotropin Secretion. Thyroid (2003) 13:167–75. doi: 10.1089/105072503321319477
33. Ma X, Dong Y, Matzuk MM, Kumar TR. Targeted Disruption of Luteinizing Hormone -Subunit Leads to Hypogonadism, Defects in Gonadal Steroidogenesis, and Infertility. Proc Natl Acad Sci (2004) 101:17294–9. doi: 10.1073/pnas.0404743101
34. Lei ZM, Mishra S, Zou W, Xu B, Foltz M, Li X, et al. Targeted Disruption of Luteinizing Hormone/Human Chorionic Gonadotropin Receptor Gene. Mol Endocrinol (2001) 15:184–200. doi: 10.1210/mend.15.1.0586
35. Zhang F-P, Poutanen M, Wilbertz J, Huhtaniemi I. Normal Prenatal But Arrested Postnatal Sexual Development of Luteinizing Hormone Receptor Knockout (LuRKO) Mice. Mol Endocrinol (2001) 15:172–83. doi: 10.1210/mend.15.1.0582
36. Lin DX, Lei ZM, Li X, Rao CV. Targeted Disruption of LH Receptor Gene Revealed the Importance of Uterine LH Signaling. Mol Cell Endocrinol (2005) 234:105–16. doi: 10.1016/j.mce.2004.09.011
37. Kumar TR, Wang Y, Lu N, Matzuk MM. Follicle Stimulating Hormone is Required for Ovarian Follicle Maturation But Not Male Fertility. Nat Genet (1997) 15:201–4. doi: 10.1038/ng0297-201
38. Dierich A, Sairam MR, Monaco L, Fimia GM, Gansmuller A, LeMeur M, et al. Impairing Follicle-Stimulating Hormone (FSH) Signaling In Vivo: Targeted Disruption of the FSH Receptor Leads to Aberrant Gametogenesis and Hormonal Imbalance. Proc Natl Acad Sci (1998) 95:13612–7. doi: 10.1073/pnas.95.23.13612
39. Abel M, Huhtaniemi I, Pakarinen P, Kumar T, Charlton H. Age-Related Uterine and Ovarian Hypertrophy in FSH Receptor Knockout and FSHbeta Subunit Knockout Mice. Reproduction (2003) 125:165–73. doi: 10.1530/rep.0.1250165
40. Swanson P, Dickey JT, Campbell B. Biochemistry and Physiology of Fish Gonadotropins. Fish Physiol Biochem (2003) 28:53–9. doi: 10.1023/B:FISH.0000030476.73360.07
41. Bogerd J, Granneman JCM, Schulz RW, Vischer HF. Fish FSH Receptors Bind LH: How to Make the Human FSH Receptor to be More Fishy? Gen Comp Endocrinol (2005) 142:34–43. doi: 10.1016/j.ygcen.2004.12.008
42. Li J, Cheng CHK. Evolution of Gonadotropin Signaling on Gonad Development: Insights From Gene Knockout Studies in Zebrafish. Biol Reprod (2018) 99:686–94. doi: 10.1093/biolre/ioy101
43. Xie Y, Chu L, Liu Y, Sham KWY, Li J, Cheng CHK. The Highly Overlapping Actions of Lh Signaling and Fsh Signaling on Zebrafish Spermatogenesis. J Endocrinol (2017) 234:233–46. doi: 10.1530/JOE-17-0079
44. Chu L, Li J, Liu Y, Hu W, Cheng CHK. Targeted Gene Disruption in Zebrafish Reveals Noncanonical Functions of LH Signaling in Reproduction. Mol Endocrinol (2014) 28:1785–95. doi: 10.1210/me.2014-1061
45. Zhang Z, Zhu B, Ge W. Genetic Analysis of Zebrafish Gonadotropin (FSH and LH) Functions by TALEN-Mediated Gene Disruption. Mol Endocrinol (2015) 29:76–98. doi: 10.1210/me.2014-1256
46. Chu L, Li J, Liu Y, Cheng CHK. Gonadotropin Signaling in Zebrafish Ovary and Testis Development: Insights From Gene Knockout Study. Mol Endocrinol (2015) 29:1743–58. doi: 10.1210/me.2015-1126
47. Murozumi N, Nakashima R, Hirai T, Kamei Y, Ishikawa-Fujiwara T, Todo T, et al. Loss of Follicle-Stimulating Hormone Receptor Function Causes Masculinization and Suppression of Ovarian Development in Genetically Female Medaka. Endocrinology (2014) 155:3136–45. doi: 10.1210/en.2013-2060
48. Zhang Z, Lau S-W, Zhang L, Ge W. Disruption of Zebrafish Follicle-Stimulating Hormone Receptor (Fshr) But Not Luteinizing Hormone Receptor (Lhcgr) Gene by TALEN Leads to Failed Follicle Activation in Females Followed by Sexual Reversal to Males. Endocrinology (2015) 156:3747–62. doi: 10.1210/en.2015-1039
49. Takahashi A, Kanda S, Abe T, Oka Y. Evolution of the Hypothalamic-Pituitary-Gonadal Axis Regulation in Vertebrates Revealed by Knockout Medaka. Endocrinology (2016) 157:3994–4002. doi: 10.1210/en.2016-1356
50. Marians RC, Ng L, Blair HC, Unger P, Graves PN, Davies TF. Nonlinear Partial Differential Equations and Applications: Defining Thyrotropin-Dependent and -Independent Steps of Thyroid Hormone Synthesis by Using Thyrotropin Receptor-Null Mice. Proc Natl Acad Sci (2002) 99:15776–81. doi: 10.1073/pnas.242322099
51. Postiglione MP, Parlato R, Rodriguez-Mallon A, Rosica A, Mithbaokar P, Maresca M, et al. Nonlinear Partial Differential Equations and Applications: Role of the Thyroid-Stimulating Hormone Receptor Signaling in Development and Differentiation of the Thyroid Gland. Proc Natl Acad Sci (2002) 99:15462–7. doi: 10.1073/pnas.242328999
52. Sherman GB, Wolfe MW, Farmerie TA, Clay CM, Threadgill DS, Sharp DC, et al. A Single Gene Encodes the Beta-Subunits of Equine Luteinizing Hormone and Chorionic Gonadotropin. Mol Endocrinol (1992) 6:951–9. doi: 10.1210/mend.6.6.1379674
53. Maston GA, Ruvolo M. Chorionic Gonadotropin Has a Recent Origin Within Primates and an Evolutionary History of Selection. Mol Biol Evol (2002) 19:320–35. doi: 10.1093/oxfordjournals.molbev.a004085
54. Rull K, Hallast P, Uuskula L, Jackson J, Punab M, Salumets A, et al. Fine-Scale Quantification of HCG Beta Gene Transcription in Human Trophoblastic and Non-Malignant Non-Trophoblastic Tissues. Mol Hum Reprod (2008) 14:23–31. doi: 10.1093/molehr/gam082
55. Hallast P, Laan M. Evolution of the Chorionic Gonadotropin Beta Genes in Primates. In: John L, editor. Encyclopedia of Life Science (ELS). Chichester: Wiley & Sons. (2009) p. 1–12.
56. Hoermann R, Spoettl G, Moncayo R, Mann K. Evidence for the Presence of Human Chorionic Gonadotropin (hCG) and Free β -Subunit of hCG in the Human Pituitary. J Clin Endocrinol Metab (1990) 71:179–86. doi: 10.1210/jcem-71-1-179
57. Birken S, Maydelman Y, Gawinowicz MA, Pound A, Liu Y, Hartree AS. Isolation and Characterization of Human Pituitary Chorionic Gonadotropin. Endocrinology (1996) 137:1402–11. doi: 10.1210/endo.137.4.8625917
58. Dirnhofer S, Hermann M, Hittmair A, Hoermann R, Kapelari K, Berger P. Expression of the Human Chorionic Gonadotropin-Beta Gene Cluster in Human Pituitaries and Alternate Use of Exon 1. J Clin Endocrinol Metab (1996) 81:4212–7. doi: 10.1210/jcem.81.12.8954017
59. Zhang S, Cui Y, Ma X, Yong J, Yan L, Yang M, et al. Single-Cell Transcriptomics Identifies Divergent Developmental Lineage Trajectories During Human Pituitary Development. Nat Commun (2020) 11:5275. doi: 10.1038/s41467-020-19012-4
60. Talmadge K, Vamvakopoulos NC, Fiddes JC. Evolution of the Genes for the β Subunits of Human Chorionic Gonadotropin and Luteinizing Hormone. Nature (1984) 307:37–40. doi: 10.1038/307037a0
61. Chen H, Chen L, Wu Y, Shen H, Yang G, Deng C. The Exonization and Functionalization of an Alu-J Element in the Protein Coding Region of Glycoprotein Hormone Alpha Gene Represent a Novel Mechanism to the Evolution of Hemochorial Placentation in Primates. Mol Biol Evol (2017) 34:3216–31. doi: 10.1093/molbev/msx252
62. Moore WT, Ward DN. Pregnant Mare Serum Gonadotropin. An In Vitro Biological Characterization of the Lutropin-Follitropin Dual Activity. J Biol Chem (1980) 255:6930–6. doi: 10.1016/S0021-9258(18)43664-2
63. Cole LA. hCG, the Wonder of Today’s Science. Reprod Biol Endocrinol (2012) 10:24. doi: 10.1186/1477-7827-10-24
64. Casarini L, Santi D, Brigante G, Simoni M. Two Hormones for One Receptor: Evolution, Biochemistry, Actions, and Pathophysiology of LH and hCG. Endocr Rev (2018) 39:549–92. doi: 10.1210/er.2018-00065
65. Gridelet V, Perrier d’Hauterive S, Polese B, Foidart J-M, Nisolle M, Geenen V. Human Chorionic Gonadotrophin: New Pleiotropic Functions for an “Old” Hormone During Pregnancy. Front Immunol (2020) 11:343. doi: 10.3389/fimmu.2020.00343
66. Schumacher A, Zenclussen AC. Human Chorionic Gonadotropin-Mediated Immune Responses That Facilitate Embryo Implantation and Placentation. Front Immunol (2019) 10:2896. doi: 10.3389/fimmu.2019.02896
67. Hsu SY, Nakabayashi K, Bhalla A. Evolution of Glycoprotein Hormone Subunit Genes in Bilateral Metazoa: Identification of Two Novel Human Glycoprotein Hormone Subunit Family Genes, GPA2 and GPB5. Mol Endocrinol (2002) 16:1538–51. doi: 10.1210/mend.16.7.0871
68. Roch GJ, Sherwood NM. Glycoprotein Hormones and Their Receptors Emerged at the Origin of Metazoans. Genome Biol Evol (2014) 6:1466–79. doi: 10.1093/gbe/evu118
69. Okada SL, Ellsworth JL, Durnam DM, Haugen HS, Holloway JL, Kelley ML, et al. A Glycoprotein Hormone Expressed in Corticotrophs Exhibits Unique Binding Properties on Thyroid-Stimulating Hormone Receptor. Mol Endocrinol (2006) 20:414–25. doi: 10.1210/me.2005-0270
70. Alvarez E, Cahoreau C, Combarnous Y. Comparative Structure Analyses of Cystine Knot-Containing Molecules With Eight Aminoacyl Ring Including Glycoprotein Hormones (GPH) Alpha and Beta Subunits and GPH-Related A2 (GPA2) and B5 (GPB5) Molecules. Reprod Biol Endocrinol (2009) 7:90. doi: 10.1186/1477-7827-7-90
71. Cahoreau C, Klett D, Combarnous Y. Structure-Function Relationships of Glycoprotein Hormones and Their Subunits’s Ancestors. Front Endocrinol (Lausanne) (2015) 6:26. doi: 10.3389/fendo.2015.00026
72. Nakabayashi K, Matsumi H, Bhalla A, Bae J, Mosselman S, Hsu SY, et al. Thyrostimulin, a Heterodimer of Two New Human Glycoprotein Hormone Subunits, Activates the Thyroid-Stimulating Hormone Receptor. J Clin Invest (2002) 109:1445–52. doi: 10.1172/JCI14340
73. Sower SA, Decatur WA, Hausken KN, Marquis TJ, Barton SL, Gargan J, et al. Emergence of an Ancestral Glycoprotein Hormone in the Pituitary of the Sea Lamprey, a Basal Vertebrate. Endocrinology (2015) 156:3026–37. doi: 10.1210/en.2014-1797
74. Macdonald LE, Wortley KE, Gowen LC, Anderson KD, Murray JD, Poueymirou WT, et al. Resistance to Diet-Induced Obesity in Mice Globally Overexpressing OGH/Gpb5. Proc Natl Acad Sci (2005) 102:2496–501. doi: 10.1073/pnas.0409849102
75. Lantz M, Vondrichova T, Capretz A, Nilsson E, Frenander C, Bondeson A-G, et al. Thyrostimulin (a TSH-Like Hormone) Expression in Orbital and Thyroid Tissue. Thyroid (2007) 17:113–8. doi: 10.1089/thy.2006.0155
76. Sun S-C, Hsu P-J, Wu F-J, Li S-H, Lu C-H, Luo C-W. Thyrostimulin, But Not Thyroid-Stimulating Hormone (TSH), Acts as a Paracrine Regulator to Activate the TSH Receptor in Mammalian Ovary. J Biol Chem (2010) 285:3758–65. doi: 10.1074/jbc.M109.066266
77. van Zeijl CJJ, Surovtseva OV, Wiersinga WM, Boelen A, Fliers E. Transient Hypothyroxinemia in Juvenile Glycoprotein Hormone Subunit B5 Knock-Out Mice. Mol Cell Endocrinol (2010) 321:231–8. doi: 10.1016/j.mce.2010.03.002
78. van Zeijl CJJ, Surovtseva OV, Kwakkel J, van Beeren HC, Duncan Bassett JH, Williams GR, et al. Thyrostimulin Deficiency Does Not Alter Peripheral Responses to Acute Inflammation-Induced Nonthyroidal Illness. Am J Physiol Metab (2014) 307:E527–37. doi: 10.1152/ajpendo.00266.2014
79. Bassett JHD, van der Spek A, Logan JG, Gogakos A, Bagchi-Chakraborty J, Murphy E, et al. Thyrostimulin Regulates Osteoblastic Bone Formation During Early Skeletal Development. Endocrinology (2015) 156:3098–113. doi: 10.1210/en.2014-1943
80. Huang W-L, Li Z, Lin T-Y, Wang S-W, Wu F-J, Luo C-W. Thyrostimulin-TSHR Signaling Promotes the Proliferation of NIH:OVCAR-3 Ovarian Cancer Cells via Trans-Regulation of the EGFR Pathway. Sci Rep (2016) 6:27471. doi: 10.1038/srep27471
81. Park J-I, Semyonov J, Chang CL, Hsu SYT. Conservation of the Heterodimeric Glycoprotein Hormone Subunit Family Proteins and the LGR Signaling System From Nematodes to Humans. Endocrine (2005) 26:267–76. doi: 10.1385/ENDO:26:3:267
82. Dos Santos S, Bardet C, Bertrand S, Escriva H, Habert D, Querat B. Distinct Expression Patterns of Glycoprotein Hormone-α2 and -β5 in a Basal Chordate Suggest Independent Developmental Functions. Endocrinology (2009) 150:3815–22. doi: 10.1210/en.2008-1743
83. Rocco DA, Paluzzi J-PV. Functional Role of the Heterodimeric Glycoprotein Hormone, GPA2/GPB5, and Its Receptor, LGR1: An Invertebrate Perspective. Gen Comp Endocrinol (2016) 234:20–7. doi: 10.1016/j.ygcen.2015.12.011
84. Van Hiel MB, Vandersmissen HP, Van Loy T, Vanden Broeck J. An Evolutionary Comparison of Leucine-Rich Repeat Containing G Protein-Coupled Receptors Reveals a Novel LGR Subtype. Peptides (2012) 34:193–200. doi: 10.1016/j.peptides.2011.11.004
85. Sudo S, Kuwabara Y, Park J-I, Hsu SY, Hsueh AJW. Heterodimeric Fly Glycoprotein Hormone-α2 (GPA2) and Glycoprotein Hormone-β5 (GPB5) Activate Fly Leucine-Rich Repeat-Containing G Protein-Coupled Receptor-1 (DLGR1) and Stimulation of Human Thyrotropin Receptors by Chimeric Fly GPA2 and Human Gpb5. Endocrinology (2005) 146:3596–604. doi: 10.1210/en.2005-0317
86. Oishi A, Gengyo-Ando K, Mitani S, Mohri-Shiomi A, Kimura KD, Ishihara T, et al. FLR-2, the Glycoprotein Hormone Alpha Subunit, is Involved in the Neural Control of Intestinal Functions in Caenorhabditis Elegans. Genes to Cells (2009) 14:1141–54. doi: 10.1111/j.1365-2443.2009.01341.x
87. Sellami A, Agricola H-J, Veenstra JA. Neuroendocrine Cells in Drosophila Melanogaster Producing GPA2/GPB5, a Hormone With Homology to LH, FSH and TSH. Gen Comp Endocrinol (2011) 170:582–8. doi: 10.1016/j.ygcen.2010.11.015
88. Asa SL, Kovacs K, Laszlo FA, Domokos I, Ezrin C. Human Fetal Adenohypophysis. Neuroendocrinology (1986) 43:308–16. doi: 10.1159/000124545
89. Stoeckel E, Hindelang C, Klein M-J, Poissonnier M, Félix J-M. Early Expression of the Glycoprotein Hormone Alpha-Subunit in the Pars Tuberalis of the Rat Pituitary Gland During Ontogenesis. Neuroendocrinology (1993) 58:616–24. doi: 10.1159/000126600
90. Japón MA, Rubinstein M, Low MJ. In Situ Hybridization Analysis of Anterior Pituitary Hormone Gene Expression During Fetal Mouse Development. J Histochem Cytochem (1994) 42:1117–25. doi: 10.1177/42.8.8027530
91. Ericson J, Norlin S, Jessell TM, Edlund T. Integrated FGF and BMP Signaling Controls the Progression of Progenitor Cell Differentiation and the Emergence of Pattern in the Embryonic Anterior Pituitary. Development (1998) 125:1005–15. doi: 10.1242/dev.125.6.1005
92. Franchimont P, Pasteels JL. Independent Secretion of Gonadotropic Hormones and of Their Subunits. C R Acad Sci Hebd Seances Acad Sci D (1972) 275:1799–802.
93. Hagen C, MCNeilly AS. Changes in Circulating Levels of LH, FSH, Lhβ- and α Subunit After Gonadotropin-Releasing Hormone, and of TSH, Lhβ- and α Subunit After Thyrotropin-Releasing Hormone. J Clin Endocrinol Metab (1975) 41:466–70. doi: 10.1210/jcem-41-3-466
94. Hagen C, McNeilly AS. The Gonadotrophins and Their Subunits in Foetal Pituitary Glands and Circulation. J Steroid Biochem (1977) 8:537–44. doi: 10.1016/0022-4731(77)90259-X
95. Kaplan SL, Grumbach MM, Aubert ML. α and β Glycoprotein Hormone Subunits (hLH, hFSH, hCG) in the Serum and Pituitary of the Human Fetus. J Clin Endocrinol Metab (1976) 42:995–8. doi: 10.1210/jcem-42-5-995
96. Beck-Peccoz P, Padmanabhan V, Baggiani AM, Cortelazzi D, Buscaglia M, Medri G, et al. Maturation of Hypothalamic-Pituitary-Gonadal Function in Normal Human Fetuses: Circulating Levels of Gonadotropins, Their Common a-Subunit and Free Testosterone, and Discrepancy Between Immunological and Biological Activities of Circulating Follicle-Stimu. J Clin Endocrinol Metab (1991) 73:525–32. doi: 10.1210/jcem-73-3-525
97. Rosemberg E, Bulat G. Immunoreactive α and β Subunits of Follicle Stimulating and Luteinizing Hormones in Peripheral Blood Throughout the Menstrual Cycle and Following Stimulation With Synthetic Gonadotropin Releasing Hormone (GnRH)1. J Endocrinol Invest (1979) 2:233–9. doi: 10.1007/BF03350408
98. Vogel DL, Rowland JA, Sherins RJ. Kinetics of Secretion of Luteinizing Hormone Subunits and Free Alpha, and Effects of Gonadotropin Releasing Hormone. J Androl (1989) 10:180–7. doi: 10.1002/j.1939-4640.1989.tb00083.x
99. Madersbacher S, Stulnig T, Huber LA, Schönitzer D, Dirnhofer S, Wick G, et al. Serum Glycoprotein Hormones and Their Free α Subunit in a Healthy Elderly Population Selected According to the SENIEUR Protocol. Analyses With Ultrasensitive Time Resolved Fluoroimmunoassays. Mech Ageing Dev (1993) 71:223–33. doi: 10.1016/0047-6374(93)90086-7
100. Holdstock JG, Burrin JM. Regulation of Glycoprotein Hormone Free Alpha-Subunit Secretion and Intracellular Alpha-Subunit Content in Primary Pituitary Cells. Endocrinology (1994) 134:685–94. doi: 10.1210/endo.134.2.7507833
101. Hagen C, McNatty KP, McNeilly AS. Immunoreactive α- and β Subunits of Luteinizing Hormone in Human Peripheral Blood and Follicular Fluid Throughout the Menstrual Cycle, and Their Effect on the Secretion Rate of Progesterone by Human Granulosa Cells in Tissue Culture. J Endocrinol (1976) 69:33–46. doi: 10.1677/joe.0.0690033
102. Roger M, Lahlou N, Couzinet B, Chaussain J-L, Scholler R. La Sous-Unite Alpha Libre Des Hormones Glycoproteiques: Donnees Physiologiques Et Pathologiques. J Steroid Biochem (1989) 33:763–9. doi: 10.1016/0022-4731(89)90489-5
103. Spratt DI, Chin WW, Ridgways EC, Crowley WF. Administration of Low Dose Pulsatile Gonadotropin- Releasing Hormone (GnRH) to GnRH-Deficient Men Regulates Free α Subunit Secretion. J Clin Endocrinol Metab (1986) 62:102–8. doi: 10.1210/jcem-62-1-102
104. Nemansky M, Thotakura NR, Lyons CD, Ye S, Reinhold BB, Reinhold VN, et al. Developmental Changes in the Glycosylation of Glycoprotein Hormone Free α Subunit During Pregnancy. J Biol Chem (1998) 273:12068–76. doi: 10.1074/jbc.273.20.12068
105. Casella I, Lindner H, Zenzmaier C, Riitano D, Berger P, Costa T. Non-Gonadotropin-Releasing Hormone-Mediated Transcription and Secretion of Large Human Glycoprotein Hormone α Subunit in Human Embryonic Kidney-293 Cells. Endocrinology (2008) 149:1144–54. doi: 10.1210/en.2007-1529
106. Krause J-M, Berger P, Roig J, Singh V, Merz WE. Rapid Maturation of Glycoprotein Hormone Free α Subunit (Gphα) and Gphαα Homodimers. Mol Endocrinol (2007) 21:2551–64. doi: 10.1210/me.2007-0051
107. Begeot M, Hemming F, Dubois P, Combarnous Y, Dubois M, Aubert M. Induction of Pituitary Lactotrope Differentiation by Luteinizing Hormone Alpha Subunit. Sci (80- ) (1984) 226:566–8. doi: 10.1126/science.6208610
108. Van Bael A, Denef C. Evidence for a Trophic Action of the Glycoprotein Hormone ?-Subunit in Rat Pituitary. J Neuroendocrinol (1996) 8:99–102. doi: 10.1111/j.1365-2826.1996.tb00829.x
109. Allaerts W, Mignon A, Denef C. Selectivity of Juxtaposition Between Cup-Shaped Lactotrophs and Gonadotrophs From Rat Anterior Pituitary in Culture. Cell Tissue Res (1991) 263:217–25. doi: 10.1007/BF00318763
110. Noda T, Kaidzu S, Kikuchi M, Yashiro T. Topographic Affinities of Hormone-Producing Cells in the Rat Anterior Pituitary Gland. Acta Histochem Cytochem (2001) 34:313–9. doi: 10.1267/ahc.34.313
111. Tsukada T, Kouki T, Fujiwara K, Ramadhani D, Horiguchi K, Kikuchi M, et al. Reassembly of Anterior Pituitary Organization by Hanging Drop Three-Dimensional Cell Culture. Acta Histochem Cytochem (2013) 46:121–7. doi: 10.1267/ahc.13015
112. Begeot M, Dubois MP, Dubois PM. Evolution of Lactotropes in Normal and Anencephalic Human Fetuses. J Clin Endocrinol Metab (1984) 58:726–30. doi: 10.1210/jcem-58-4-726
113. Dubois PM, Begeot M, Dubois MP, Herbert DC. Immunocytological Localization of LH, FSH, TSH and Their Subunits in the Pituitary of Normal and Anencephalic Human Fetuses. Cell Tissue Res (1978) 191:249–65. doi: 10.1007/BF00222423
114. Kendall SK, Samuelson LC, Saunders TL, Wood RI, Camper SA. Targeted Disruption of the Pituitary Glycoprotein Hormone Alpha-Subunit Produces Hypogonadal and Hypothyroid Mice. Genes Dev (1995) 9:2007–19. doi: 10.1101/gad.9.16.2007
115. Seuntjens E, Vankelecom H, Quaegebeur A, Vande Vijver V, Denef C. Targeted Ablation of Gonadotrophs in Transgenic Mice Affects Embryonic Development of Lactotrophs. Mol Cell Endocrinol (1999) 150:129–39. doi: 10.1016/S0303-7207(99)00011-8
116. Chabot V, Gauthier C, Combarnous Y, Taragnat C. Stimulating Effect of Glycoprotein Hormone Free Alpha-Subunit and Daily Gonadotropin Releasing Hormone Treatment on Prolactin Release From 50-Day Ovine Foetal Pituitary Explants. J Neuroendocrinol (2001) 13:199–208. doi: 10.1046/j.1365-2826.2001.00614.x
117. Böckers TM, Bockmann J, Salem A, Niklowitz P, Lerchl A, Huppertz M, et al. Initial Expression of the Common α-Chain in Hypophyseal Pars Tuberalis-Specific Cells in Spontaneous Recrudescent Hamsters. Endocrinology (1997) 138:4101–8. doi: 10.1210/endo.138.10.5423
118. Oguchi A, Tanaka S, Yamamoto K, Kikuyama S. Release of α Subunit of Glycoprotein Hormones From the Bullfrog Pituitary: Possible Effect of α Subunit on Prolactin Cell Function. Gen Comp Endocrinol (1996) 102:141–6. doi: 10.1006/gcen.1996.0055
119. Weltzien F-A, Hildahl J, Hodne K, Okubo K, Haug TM. Embryonic Development of Gonadotrope Cells and Gonadotropic Hormones – Lessons From Model Fish. Mol Cell Endocrinol (2014) 385:18–27. doi: 10.1016/j.mce.2013.10.016
120. Ben-Jonathan N, Mershon JL, Allen DL, Steinmetz RW. Extrapituitary Prolactin: Distribution, Regulation, Functions, and Clinical Aspects. Endocr Rev (1996) 17:639–69. doi: 10.1210/edrv-17-6-639
121. Stewart EA. Gonadotropins and the Uterus: Is There a Gonad-Independent Pathway? J Soc Gynecol Investig (2001) 8:319–26. doi: 10.1016/S1071-5576(01)00136-8
122. Marano RJ, Ben-Jonathan N. Minireview: Extrapituitary Prolactin: An Update on the Distribution, Regulation, and Functions. Mol Endocrinol (2014) 28:622–33. doi: 10.1210/me.2013-1349
123. Ozturk M, Bellet D, Manil L, Hennen G, Frydman R, Wands J. Physiological Studies of Human Chorionic Gonadotropin (hCG), αhcg, and βhcg as Measured by Specific Monoclonal Immunoradiometric Assays. Endocrinology (1987) 120:549–58. doi: 10.1210/endo-120-2-549
124. Berkowitz R, Ozturk M, Goldstein D, Bernstein M, Hill L, Wands JR. Human Chorionic Gonadotropin and Free Subunits’ Serum Levels in Patients With Partial and Complete Hydatidiform Moles. Obstet Gynecol (1989) 74:212–6.
125. Reuter A, Gaspard UJ, Deville J-L, Vrind-Gevaert Y, Franchimont P. Serum Concentrations of Human Chorionic Gonadotrophin and Its Alpha and Beta Subunits. 1. During Normal Singleton and Twin Pregnancies. Clin Endocrinol (Oxf) (1980) 13:305–18. doi: 10.1111/j.1365-2265.1980.tb03390.x
126. Gellersen B, Brosens JJ. Cyclic Decidualization of the Human Endometrium in Reproductive Health and Failure. Endocr Rev (2014) 35:851–905. doi: 10.1210/er.2014-1045
127. Blithe D, Richards RG, Skarulis MC. Free Alpha Molecules From Pregnancy Stimulate Secretion of Prolactin From Human Decidual Cells: A Novel Function for Free Alpha in Pregnancy. Endocrinology (1991) 129:2257–9. doi: 10.1210/endo-129-4-2257
128. Nemansky M, Moy E, Lyons CD, Yu I, Blithe DL. Human Endometrial Stromal Cells Generate Uncombined α Subunit From Human Chorionic Gonadotropin, Which Can Synergize With Progesterone to Induce Decidualization. J Clin Endocrinol Metab (1998) 83:575–81. doi: 10.1210/jcem.83.2.4593
129. Moy E, Kimzey LM, Nelson LM, Blithe DL. Glycoprotein Hormone Alpha-Subunit Functions Synergistically With Progesterone to Stimulate Differentiation of Cultured Human Endometrial Stromal Cells to Decidualized Cells: A Novel Role for Free Alpha-Subunit in Reproduction. Endocrinology (1996) 137:1332–9. doi: 10.1210/endo.137.4.8625908
130. Stewart EA, Jain P, Penglase MD, Friedman AJ, Nowak RA. The Myometrium of Postmenopausal Women Produces Prolactin in Response to Human Chorionic Gonadotropin and Alpha-Subunit In Vitro. Fertil Steril (1995) 64:972–6. doi: 10.1016/S0015-0282(16)57912-6
131. Rumpold H, Mascher K, Untergasser G, Plas E, Hermann M, Berger P. Trans -Differentiation of Prostatic Stromal Cells Leads to Decreased Glycoprotein Hormone α Production. J Clin Endocrinol Metab (2002) 87:5297–303. doi: 10.1210/jc.2002-020596
132. Berger P, Gruschwitz M, Spoettl G, Dirnhofer S, Madersbacher S, Gerth R, et al. Human Chorionic Gonadotropin (hCG) in the Male Reproductive Tract. Mol Cell Endocrinol (2007) 260–262:190–6. doi: 10.1016/j.mce.2006.01.021
133. Zenzmaier C, Gerth R, Gruschwitz M, Lindner H, Plas E, Berger P. Decreased Levels of Genuine Large Free hCG Alpha in Men Presenting With Abnormal Semen Analysis. Reprod Biol Endocrinol (2011) 9:114. doi: 10.1186/1477-7827-9-114
134. Bièche I, Parfait B, Le Doussal V, Olivi M, Rio MC, Lidereau R, et al. Identification of CGA as a Novel Estrogen Receptor-Responsive Gene in Breast Cancer: An Outstanding Candidate Marker to Predict the Response to Endocrine Therapy. Cancer Res (2001) 61:1652–168.
135. Evans J. Hyperglycosylated hCG: A Unique Human Implantation and Invasion Factor. Am J Reprod Immunol (2016) 75:333–40. doi: 10.1111/aji.12459
136. Fournier T, Guibourdenche J, Evain-Brion D. Review: hCGs: Different Sources of Production, Different Glycoforms and Functions. Placenta (2015) 36(Suppl 1):S60–5. doi: 10.1016/j.placenta.2015.02.002
137. Koistinen H, Stenman U-H. LH/hCG Receptor–Independent Activities of hCG and Hcgβ. In: Cole LA, Butler SA, editors. 100 Years of Human Chorionic Gonadotropin. Amsterdam: Elsevier. p. 261–8.
138. Handschuh K, Guibourdenche J, Cocquebert M, Tsatsaris V, Vidaud M, Evain-Brion D, et al. Expression and Regulation by Pparγ of hCG α- and β Subunits: Comparison Between Villous and Invasive Extravillous Trophoblastic Cells☆. Placenta (2009) 30:1016–22. doi: 10.1016/j.placenta.2009.09.006
139. Knöfler M, Haider S, Saleh L, Pollheimer J, Gamage TKJB, James J. Human Placenta and Trophoblast Development: Key Molecular Mechanisms and Model Systems. Cell Mol Life Sci (2019) 76:3479–96. doi: 10.1007/s00018-019-03104-6
140. Ferretti C, Bruni L, Dangles-Marie V, Pecking AP, Bellet D. Molecular Circuits Shared by Placental and Cancer Cells, and Their Implications in the Proliferative, Invasive and Migratory Capacities of Trophoblasts. Hum Reprod Update (2006) 13:121–41. doi: 10.1093/humupd/dml048
141. Fournier T. Human Chorionic Gonadotropin: Different Glycoforms and Biological Activity Depending on Its Source of Production. Ann Endocrinol (Paris) (2016) 77:75–81. doi: 10.1016/j.ando.2016.04.012
142. Kovalevskaya G, Genbacev O, Fisher S, Caceres E, O’Connor J. Trophoblast Origin of hCG Isoforms: Cytotrophoblasts Are the Primary Source of Choriocarcinoma-Like hCG. Mol Cell Endocrinol (2002) 194:147–55. doi: 10.1016/S0303-7207(02)00135-1
143. Guibourdenche J, Handschuh K, Tsatsaris V, Gerbaud P, Leguy MC, Muller F, et al. Hyperglycosylated hCG is a Marker of Early Human Trophoblast Invasion. J Clin Endocrinol Metab (2010) 95:E240–4. doi: 10.1210/jc.2010-0138
144. Birken S. Specific Measurement of O-Linked Core 2 Sugar-Containing Isoforms of Hyperglycosylated Human Chorionic Gonadotropin by Antibody B152. Tumor Biol (2005) 26:131–41. doi: 10.1159/000086484
145. Birken S, Krichevsky A, O’Connor J, Schlatterer J, Cole L, Kardana A, et al. Development and Characterization of Antibodies to a Nicked and Hyperglycosylated Form of hCG From a Choriocarcinoma Patient: Generation of Antibodies That Differentiate Between Pregnancy hCG and Choriocarcinoma hCG. Endocrine (1999) 10:137–44. doi: 10.1385/ENDO:10:2:137
146. Stenman U-H, Birken S, Lempiäinen A, Hotakainen K, Alfthan H. Elimination of Complement Interference in Immunoassay of Hyperglycosylated Human Chorionic Gonadotropin. Clin Chem (2011) 57:1075–7. doi: 10.1373/clinchem.2010.159939
147. Handschuh K, Guibourdenche J, Tsatsaris V, Guesnon M, Laurendeau I, Evain-Brion D, et al. Human Chorionic Gonadotropin Produced by the Invasive Trophoblast But Not the Villous Trophoblast Promotes Cell Invasion and is Down-Regulated by Peroxisome Proliferator-Activated Receptor-Gamma. Endocrinology (2007) 148:5011–9. doi: 10.1210/en.2007-0286
148. Koistinen H, Koel M, Peters M, Rinken A, Lundin K, Tuuri T, et al. Hyperglycosylated hCG Activates LH/hCG-Receptor With Lower Activity Than hCG. Mol Cell Endocrinol (2019) 479:103–9. doi: 10.1016/j.mce.2018.09.006
149. Evans J, Salamonsen LA, Menkhorst E, Dimitriadis E. Dynamic Changes in Hyperglycosylated Human Chorionic Gonadotrophin Throughout the First Trimester of Pregnancy and Its Role in Early Placentation. Hum Reprod (2015) 30:1029–38. doi: 10.1093/humrep/dev016
150. Lee C-L, Chiu PCN, Hautala L, Salo T, Yeung WSB, Stenman U-H, et al. Human Chorionic Gonadotropin and Its Free β Subunit Stimulate Trophoblast Invasion Independent of LH/hCG Receptor. Mol Cell Endocrinol (2013) 375:43–52. doi: 10.1016/j.mce.2013.05.009
151. Kosaka K, Fujiwara H, Tatsumi K, Yoshioka S, Sato Y, Egawa H, et al. Human Chorionic Gonadotropin (HCG) Activates Monocytes to Produce Interleukin-8 via a Different Pathway From Luteinizing Hormone/HCG Receptor System. J Clin Endocrinol Metab (2002) 87:5199–208. doi: 10.1210/jc.2002-020341
152. Kane N, Kelly R, Saunders PTK, Critchley HOD. Proliferation of Uterine Natural Killer Cells Is Induced by Human Chorionic Gonadotropin and Mediated via the Mannose Receptor. Endocrinology (2009) 150:2882–8. doi: 10.1210/en.2008-1309
153. Pakarainen T. Fertility in Luteinizing Hormone Receptor-Knockout Mice After Wild-Type Ovary Transplantation Demonstrates Redundancy of Extragonadal Luteinizing Hormone Action. J Clin Invest (2005) 115:1862–8. doi: 10.1172/JCI24562
154. Mitri F, Bentov Y, Behan LA, Esfandiari N, Casper RF. A Novel Compound Heterozygous Mutation of the Luteinizing Hormone Receptor –Implications for Fertility. J Assist Reprod Genet (2014) 31:787–94. doi: 10.1007/s10815-014-0249-5
155. Sasaki Y, Ladner DG, Cole LA. Hyperglycosylated Human Chorionic Gonadotropin and the Source of Pregnancy Failures. Fertil Steril (2008) 89:1781–6. doi: 10.1016/j.fertnstert.2007.03.010
156. Brady PC, Farland LV, Racowsky C, Ginsburg ES. Hyperglycosylated Human Chorionic Gonadotropin as a Predictor of Ongoing Pregnancy. Am J Obstet Gynecol (2020) 222:68.e1–68.e12. doi: 10.1016/j.ajog.2019.08.004
157. Keikkala E, Vuorela P, Laivuori H, Romppanen J, Heinonen S, Stenman U-H. First Trimester Hyperglycosylated Human Chorionic Gonadotrophin in Serum - a Marker of Early-Onset Preeclampsia. Placenta (2013) 34:1059–65. doi: 10.1016/j.placenta.2013.08.006
158. Asch RH, Fernandez EO, Siler-Khodr TM, Pauerstein CJ. Peptide and Steroid Hormone Concentrations in Human Seminal Plasma. Int J Fertil (1984) 29:25–32.
159. Caroppo E, Niederberger C, Iacovazzi PA, Correale M, Palagiano A, D’Amato G. Human Chorionic Gonadotropin Free β Subunit in the Human Seminal Plasma: A New Marker for Spermatogenesis? Eur J Obstet Gynecol Reprod Biol (2003) 106:165–9. doi: 10.1016/S0301-2115(02)00231-2
160. Valmu L, Alfthan H, Hotakainen K, Birken S, Stenman U-H. Site-Specific Glycan Analysis of Human Chorionic Gonadotropin -Subunit From Malignancies and Pregnancy by Liquid Chromatography–Electrospray Mass Spectrometry. Glycobiology (2006) 16:1207–18. doi: 10.1093/glycob/cwl034
161. Iles RK. Ectopic Hcgβ Expression by Epithelial Cancer: Malignant Behaviour, Metastasis and Inhibition of Tumor Cell Apoptosis. Mol Cell Endocrinol (2007) 260–262:264–70. doi: 10.1016/j.mce.2006.02.019
162. Iles RK, Delves PJ, Butler SA. Does hCG or Hcgβ Play a Role in Cancer Cell Biology? Mol Cell Endocrinol (2010) 329:62–70. doi: 10.1016/j.mce.2010.07.014
163. Cole LA, Butler S. Hyperglycosylated hCG, Hcgβ and Hyperglycosylated Hcgβ: Interchangeable Cancer Promoters. Mol Cell Endocrinol (2012) 349:232–8. doi: 10.1016/j.mce.2011.10.029
164. Butler SA, Ikram MS, Mathieu S, Iles RK. The Increase in Bladder Carcinoma Cell Population Induced by the Free Beta Subunit of Human Chorionic Gonadotrophin Is a Result of an Anti-Apoptosis Effect and Not Cell Proliferation. Br J Cancer (2000) 82:1553–6. doi: 10.1054/bjoc.2000.1177
165. Gillott D, Iles R, Chard T. The Effects of Beta-Human Chorionic Gonadotrophin on the In Vitro Growth of Bladder Cancer Cell Lines. Br J Cancer (1996) 73:323–6. doi: 10.1038/bjc.1996.56
166. Burczynska B, Booth MJ, Iles RK, Shah A, Shiled A, Butler SA. Stable Knockdown of Hcgβ mRNA Expression in Bladder Cancer Cells Results in Significant Growth Inhibition. Anticancer Res (2013) 33:3611–4.
167. Jankowska A, Gunderson SI, Andrusiewicz M, Burczynska B, Szczerba A, Jarmolowski A, et al. Reduction of Human Chorionic Gonadotropin Beta Subunit Expression by Modified U1 snRNA Caused Apoptosis in Cervical Cancer Cells. Mol Cancer (2008) 7:26. doi: 10.1186/1476-4598-7-26
168. Cole LA, Butler SA. B152 Anti-Hyperglycosylated Human Chorionic Gonadotropin Free β Subunit. A New, Possible Treatment for Cancer. J Reprod Med (2015) 60:13–20.
169. Acevedo HF, Hartsock RJ. Metastatic Phenotype Correlates With High Expression of Membrane-Associated Complete β-Human Chorionic Gonadotropin In Vivo. Cancer (1996) 78:2388–99. doi: 10.1002/(SICI)1097-0142(19961201)78:11<2388::AID-CNCR18>3.0.CO;2-X
170. Janiszewska M, Primi MC, Izard T. Cell Adhesion in Cancer: Beyond the Migration of Single Cells. J Biol Chem (2020) 295:2495–505. doi: 10.1074/jbc.REV119.007759
171. Wu W, Walker AM. Human Chorionic Gonadotropin β (Hcgβ) Down-Regulates E-Cadherin and Promotes Human Prostate Carcinoma Cell Migration and Invasion. Cancer (2006) 106:68–78. doi: 10.1002/cncr.21549
172. Guo X, Liu G, Schauer IG, Yang G, Mercado-Uribe I, Yang F, et al. Overexpression of the β Subunit of Human Chorionic Gonadotropin Promotes the Transformation of Human Ovarian Epithelial Cells and Ovarian Tumorigenesis. Am J Pathol (2011) 179:1385–93. doi: 10.1016/j.ajpath.2011.05.018
173. Liu N, Peng S-M, Zhan G-X, Yu J, Wu W-M, Gao H, et al. Human Chorionic Gonadotropin β Regulates Epithelial-Mesenchymal Transition and Metastasis in Human Ovarian Cancer. Oncol Rep (2017) 38:1464–72. doi: 10.3892/or.2017.5818
174. Wu W, Gao H, Li X, Peng S, Yu J, Liu N, et al. β-hCG Promotes Epithelial Ovarian Cancer Metastasis Through ERK/MMP2 Signaling Pathway. Cell Cycle (2019) 18:46–59. doi: 10.1080/15384101.2018.1558869
175. Sengodan SK, Nadhan R, Nair RS, Hemalatha SK, Somasundaram V, Sushama RR, et al. BRCA1 Regulation on β-hCG: A Mechanism for Tumorigenicity in BRCA1 Defective Breast Cancer. Oncogenesis (2017) 6:e376. doi: 10.1038/oncsis.2017.75
176. Stenman U-H, Alfthan H, Hotakainen K. Human Chorionic Gonadotropin in Cancer. Clin Biochem (2004) 37:549–61. doi: 10.1016/j.clinbiochem.2004.05.008
177. Talwar GP, Singh O, Pal R, Chatterjee N, Sahai P, Dhall K, et al. A Vaccine That Prevents Pregnancy in Women. Proc Natl Acad Sci USA (1994) 91:8532–6. doi: 10.1073/pnas.91.18.8532
178. Talwar GP, Singh O, Gupta SK, Hasnain SE, Pal R, Majumdar SS, et al. The HSD-hCG Vaccine Prevents Pregnancy in Women: Feasibility Study of a Reversible Safe Contraceptive Vaccine. Am J Reprod Immunol (1997) 37:153–60. doi: 10.1111/j.1600-0897.1997.tb00207.x
179. Naz RK, Gupta SK, Gupta JC, Vyas HK, Talwar and GP. Recent Advances in Contraceptive Vaccine Development: A Mini-Review. Hum Reprod (2005) 20:3271–83. doi: 10.1093/humrep/dei256
180. Moulton HM, Yoshihara PH, Mason DH, Iversen PL, Triozzi PL. Active Specific Immunotherapy With a Beta-Human Chorionic Gonadotropin Peptide Vaccine in Patients With Metastatic Colorectal Cancer: Antibody Response is Associated With Improved Survival. Clin Cancer Res (2002) 8:2044–51.
181. He L-Z, Ramakrishna V, Connolly JE, Wang X-T, Smith PA, Jones CL, et al. A Novel Human Cancer Vaccine Elicits Cellular Responses to the Tumor-Associated Antigen, Human Chorionic Gonadotropin β. Clin Cancer Res (2004) 10:1920–7. doi: 10.1158/1078-0432.CCR-03-0264
182. Yankai Z, Rong Y, Yi H, Wentao L, Rongyue C, Ming Y, et al. Ten Tandem Repeats of β-hCG 109–118 Enhance Immunogenicity and Anti-Tumor Effects of β-hCG C-Terminal Peptide Carried by Mycobacterial Heat-Shock Protein HSP65. Biochem Biophys Res Commun (2006) 345:1365–71. doi: 10.1016/j.bbrc.2006.05.022
183. Yi H, Rong Y, Yankai Z, Wentao L, Hongxia Z, Jie W, et al. Improved Efficacy of DNA Vaccination Against Breast Cancer by Boosting With the Repeat beta-hCG C-Terminal Peptide Carried by Mycobacterial Heat-Shock Protein HSP65. Vaccine (2006) 24:2575–84. doi: 10.1016/j.vaccine.2005.12.030
184. Delves PJ, Iles RK, Roitt IM, Lund T. Designing a New Generation of anti-hCG Vaccines for Cancer Therapy. Mol Cell Endocrinol (2007) 260–262:276–81. doi: 10.1016/j.mce.2006.02.018
185. Xiangbing H, Yankai Z, Ming L, Yong L, Yu Z, Huiyong Z, et al. The Fusion Protein of HSP65 With Tandem Repeats of β-hCG Acting as a Potent Tumor Vaccine in Suppressing Hepatocarcinoma. Int Immunopharmacol (2010) 10:230–8. doi: 10.1016/j.intimp.2009.11.006
186. Morse MA, Bradley DA, Keler T, Laliberte RJ, Green JA, Davis TA, et al. CDX-1307: A Novel Vaccine Under Study as Treatment for Muscle-Invasive Bladder Cancer. Expert Rev Vaccines (2011) 10:733–42. doi: 10.1586/erv.11.20
187. Morse MA, Chapman R, Powderly J, Blackwell K, Keler T, Green J, et al. Phase I Study Utilizing a Novel Antigen-Presenting Cell–Targeted Vaccine With Toll-Like Receptor Stimulation to Induce Immunity to Self-Antigens in Cancer Patients. Clin Cancer Res (2011) 17:4844–53. doi: 10.1158/1078-0432.CCR-11-0891
188. Bose A, Huhtaniemi I, Singh O, Pal R. Synergistic Activation of Innate and Adaptive Immune Mechanisms in the Treatment of Gonadotropin-Sensitive Tumors. PloS One (2013) 8:e61288. doi: 10.1371/journal.pone.0061288
189. Nand KN, Gupta JC, Panda AK, Jain SK. Development of a Recombinant hCG-Specific Single Chain Immunotoxin Cytotoxic to hCG Expressing Cancer Cells. Protein Expr Purif (2015) 106:10–7. doi: 10.1016/j.pep.2014.10.008
190. Khare P, Bose A, Singh P, Singh S, Javed S, Jain SK, et al. Gonadotropin and Tumorigenesis: Direct and Indirect Effects on Inflammatory and Immunosuppressive Mediators and Invasion. Mol Carcinog (2017) 56:359–70. doi: 10.1002/mc.22499
191. Bockmann J, Böckers TM, Winter C, Wittkowski W, Winterhoff H, Deufel T, et al. Thyrotropin Expression in Hypophyseal Pars Tuberalis-Specific Cells is 3,5,3′-Triiodothyronine, Thyrotropin-Releasing Hormone, and Pit-1 Independent. Endocrinology (1997) 138:1019–28. doi: 10.1210/endo.138.3.5007
192. von Gall C, Weaver DR, Moek J, Jilg A, Stehle JH, Korf H-W. Melatonin Plays a Crucial Role in the Regulation of Rhythmic Clock Gene Expression in the Mouse Pars Tuberalis. Ann N Y Acad Sci (2005) 1040:508–11. doi: 10.1196/annals.1327.105
193. Korf H-W. Signaling Pathways to and From the Hypophysial Pars Tuberalis, an Important Center for the Control of Seasonal Rhythms. Gen Comp Endocrinol (2018) 258:236–43. doi: 10.1016/j.ygcen.2017.05.011
194. Dardente H, Wood S, Ebling F, Sáenz de Miera C. An Integrative View of Mammalian Seasonal Neuroendocrinology. J Neuroendocrinol (2019) 31:e12729. doi: 10.1111/jne.12729
195. Ikegami K, Liao X-H, Hoshino Y, Ono H, Ota W, Ito Y, et al. Tissue-Specific Posttranslational Modification Allows Functional Targeting of Thyrotropin. Cell Rep (2014) 9:801–9. doi: 10.1016/j.celrep.2014.10.006
196. Persani L, Borgato S, Romoli R, Asteria C, Pizzocaro A, Beck-Peccoz P. Changes in the Degree of Sialylation of Carbohydrate Chains Modify the Biological Properties of Circulating Thyrotropin Isoforms in Various Physiological and Pathological States 1. J Clin Endocrinol Metab (1998) 83:2486–92. doi: 10.1210/jcem.83.7.4970
197. Vincent BH, Montufar-Solis D, Teng B-B, Amendt BA, Schaefer J, Klein JR. Bone Marrow Cells Produce a Novel Tshβ Splice Variant That Is Upregulated in the Thyroid Following Systemic Virus Infection. Genes Immun (2009) 10:18–26. doi: 10.1038/gene.2008.69
198. Schaefer JS, Klein JR. A Novel Thyroid Stimulating Hormone β Subunit Isoform in Human Pituitary, Peripheral Blood Leukocytes, and Thyroid. Gen Comp Endocrinol (2009) 162:241–4. doi: 10.1016/j.ygcen.2009.04.006
199. Klein JR. Novel Splicing of Immune System Thyroid Stimulating Hormone β Subunit—Genetic Regulation and Biological Importance. Front Endocrinol (Lausanne) (2019) 10:44. doi: 10.3389/fendo.2019.00044
200. Baliram R, Latif R, Morshed SA, Zaidi M, Davies TF. T3 Regulates a Human Macrophage-Derived TSH-β Splice Variant: Implications for Human Bone Biology. Endocrinology (2016) 157:3658–67. doi: 10.1210/en.2015-1974
201. Mezei M, Baliram R, Ali MR, Zaidi M, Davies TF, Latif R. The Human Tshβ Subunit Proteins and Their Binding Sites on the TSH Receptor Using Molecular Dynamics Simulation. Endocrinology (2020) 161:1–11. doi: 10.1210/endocr/bqaa125
202. Baliram R, Chow A, Huber AK, Collier L, Ali MR, Morshed SA, et al. Thyroid and Bone: Macrophage-Derived TSH-β Splice Variant Increases Murine Osteoblastogenesis. Endocrinology (2013) 154:4919–26. doi: 10.1210/en.2012-2234
203. Montufar-Solis D, Klein JR. Splenic Leukocytes Traffic to the Thyroid and Produce a Novel Tshβ Isoform During Acute Listeria Monocytogenes Infection in Mice. PloS One (2016) 11:e0146111. doi: 10.1371/journal.pone.0146111
204. Weber A, Pawar HN, Klein JR. Thyroid Stimulating Hormone β Subunit Splice Variant is Expressed in All Fractional Subsets of Bone Marrow Hematopoietic Cells and Peripheral Blood Leukocytes and is Modulated During Bacterial Infection. Gen Comp Endocrinol (2020) 294:113495. doi: 10.1016/j.ygcen.2020.113495
205. Liu C, Miao J, Zhao Z, Li L, Liu Y, Zhang Y, et al. Functional Human Tshβ Splice Variant Produced by Plasma Cell may be Involved in the Immunologic Injury of Thyroid in the Patient With Hashimoto’s Thyroiditis. Mol Cell Endocrinol (2015) 414:132–42. doi: 10.1016/j.mce.2015.06.009
206. Yoshimura M, Hershman Jerome M. Thyrotropic Action of Human Chorionic Gonadotropin. Thyroid (1995) 5:425–34. doi: 10.1089/thy.1995.5.425
207. Loosfelt H, Pichon C, Jolivet A, Misrahi M, Caillou B, Jamous M, et al. Two-Subunit Structure of the Human Thyrotropin Receptor. Proc Natl Acad Sci USA (1992) 89:3765–9. doi: 10.1073/pnas.89.9.3765
208. Vassart G, Costagliola S. A Physiological Role for the Posttranslational Cleavage of the Thyrotropin Receptor? Endocrinology (2004) 145:1–3. doi: 10.1210/en.2003-1225
209. Davies TF. Thyrotropin Receptor-Associated Diseases: From Adenomata to Graves Disease. J Clin Invest (2005) 115:1972–83. doi: 10.1172/JCI26031
210. Buechi HB, Bridgham JT. Evolution of Specificity in Cartilaginous Fish Glycoprotein Hormones and Receptors. Gen Comp Endocrinol (2017) 246:309–20. doi: 10.1016/j.ygcen.2017.01.007
211. Okajima Y, Nagasaki H, Suzuki C, Suga H, Ozaki N, Arima H, et al. Biochemical Roles of the Oligosaccharide Chains in Thyrostimulin, A Heterodimeric Hormone of Glycoprotein Hormone Subunits Alpha2 (GPA2) and Beta5 (GPB5). Regul Pept (2008) 148:62–7. doi: 10.1016/j.regpep.2008.03.002
212. Li C, Hirooka Y, Habu S, Takagi J, Gotoh M, Nogimori T. Distribution of Thyrostimulin in the Rat: An Immunohistochemical Study. Endocr Regul (2004) 38:131–42.
213. Nagasaki H. Differential Expression of the Thyrostimulin Subunits, Glycoprotein 2 and 5 in the Rat Pituitary. J Mol Endocrinol (2006) 37:39–50. doi: 10.1677/jme.1.01932
214. Heyland A, Plachetzki D, Donelly E, Gunaratne D, Bobkova Y, Jacobson J, et al. Distinct Expression Patterns of Glycoprotein Hormone Subunits in the Lophotrochozoan Aplysia: Implications for the Evolution of Neuroendocrine Systems in Animals. Endocrinology (2012) 153:5440–51. doi: 10.1210/en.2012-1677
215. Vandersmissen HP, Van Hiel MB, Van Loy T, Vleugels R, Vanden Broeck J. Silencing D. Melanogaster Lgr1 Impairs Transition From Larval to Pupal Stage. Gen Comp Endocrinol (2014) 209:135–47. doi: 10.1016/j.ygcen.2014.08.006
216. Rocco DA, Paluzzi J-PV. Expression Profiling, Downstream Signaling, and Inter-Subunit Interactions of GPA2/GPB5 in the Adult Mosquito Aedes Aegypti. Front Endocrinol (Lausanne) (2020) 11:158. doi: 10.3389/fendo.2020.00158
217. Wang P, Liu S, Yang Q, Liu Z, Zhang S. Functional Characterization of Thyrostimulin in Amphioxus Suggests an Ancestral Origin of the TH Signaling Pathway. Endocrinology (2018) 159:3536–48. doi: 10.1210/en.2018-00550
218. Butler S, Laidler P, Kicman A, Chard T, Cowan D, Iles R. The Beta-Subunit of Human Chorionic Gonadotrophin Exists as a Homodimer. J Mol Endocrinol (1999) 22:185–92. doi: 10.1677/jme.0.0220185
219. Butler SA, Iles RK. The Free Monomeric Beta Subunit of Human Chorionic Gonadotrophin (Hcgβ) and the Recently Identified Homodimeric Beta-Beta Subunit (Hcgββ) Both Have Autocrine Growth Effects. Tumor Biol (2004) 25:18–23. doi: 10.1159/000077719
220. Hinck AP, Mueller TD, Springer TA. Structural Biology and Evolution of the TGF-β Family. Cold Spring Harb Perspect Biol (2016) 8:a022103. doi: 10.1101/cshperspect.a022103
221. Vander Ark A, Cao J, Li X. TGF-β Receptors: In and Beyond TGF-β Signaling. Cell Signal (2018) 52:112–20. doi: 10.1016/j.cellsig.2018.09.002
222. Colak S, ten Dijke P. Targeting TGF-β Signaling in Cancer. Trends Cancer (2017) 3:56–71. doi: 10.1016/j.trecan.2016.11.008
223. Głodek A, Jankowska A. CGB Activates ERK and AKT Kinases in Cancer Cells via LHCGR-Independent Mechanism. Tumor Biol (2014) 35:5467–79. doi: 10.1007/s13277-014-1715-5
224. Li Z, Du L, Li C, Wu W. Human Chorionic Gonadotropin β Induces Cell Motility via ERK1/2 and MMP-2 Activation in Human Glioblastoma U87MG Cells. J Neurooncol (2013) 111:237–44. doi: 10.1007/s11060-012-1017-y
225. Li Z, Li C, Du L, Zhou Y, Wu W. Human Chorionic Gonadotropin β Induces Migration and Invasion via Activating ERK1/2 and MMP-2 in Human Prostate Cancer DU145 Cells. PloS One (2013) 8:e54592. doi: 10.1371/journal.pone.0054592
226. Koistinen H, Hautala L, Koli K, Stenman U-H. Absence of TGF-β Receptor Activation by Highly Purified hCG Preparations. Mol Endocrinol (2015) 29:1787–91. doi: 10.1210/me.2015-1187
227. Yarram SJ, Jenkins J, Cole LA, Brown NL, Sandy JR, Mansell JP. Epidermal Growth Factor Contamination and Concentrations of Intact Human Chorionic Gonadotropin in Commercial Preparations. Fertil Steril (2004) 82:232–3. doi: 10.1016/j.fertnstert.2003.11.051
228. Saleh L, Prast J, Haslinger P, Husslein P, Helmer H, Knöfler M. Effects of Different Human Chorionic Gonadotrophin Preparations on Trophoblast Differentiation. Placenta (2007) 28:199–203. doi: 10.1016/j.placenta.2006.02.008
229. Lash GE, Otun HA, Innes BA, Bulmer JN, Searle RF, Robson SC. Inhibition of Trophoblast Cell Invasion by TGFB1, 2, and 3 Is Associated With a Decrease in Active Proteases. Biol Reprod (2005) 73:374–81. doi: 10.1095/biolreprod.105.040337
230. Lunghi L, Ferretti ME, Medici S, Biondi C, Vesce F. Control of Human Trophoblast Function. Reprod Biol Endocrinol (2007) 5:6. doi: 10.1186/1477-7827-5-6
231. Zygmunt M, Herr F, Keller-Schoenwetter S, Kunzi-Rapp K, Münstedt K, Rao CV, et al. Characterization of Human Chorionic Gonadotropin as a Novel Angiogenic Factor. J Clin Endocrinol Metab (2002) 87:5290–6. doi: 10.1210/jc.2002-020642
232. Han VK, Bassett N, Walton J, Challis JR. The Expression of Insulin-Like Growth Factor (IGF) and IGF-Binding Protein (IGFBP) Genes in the Human Placenta and Membranes: Evidence for IGF-IGFBP Interactions at the Feto-Maternal Interface. J Clin Endocrinol Metab (1996) 81:2680–93. doi: 10.1210/jcem.81.7.8675597
233. McKinnon T, Chakraborty C, Gleeson LM, Chidiac P, Lala PK. Stimulation of Human Extravillous Trophoblast Migration by IGF-II Is Mediated by IGF Type 2 Receptor Involving Inhibitory G Protein(s) and Phosphorylation of MAPK. J Clin Endocrinol Metab (2001) 86:3665–74. doi: 10.1210/jcem.86.8.7711
234. Luo C-W, Dewey EM, Sudo S, Ewer J, Hsu SY, Honegger H-W, et al. Bursicon, the Insect Cuticle-Hardening Hormone, Is a Heterodimeric Cystine Knot Protein That Activates G Protein-Coupled Receptor LGR2. Proc Natl Acad Sci (2005) 102:2820–5. doi: 10.1073/pnas.0409916102
235. de Lau W, Peng WC, Gros P, Clevers H. The R-Spondin/Lgr5/Rnf43 Module: Regulator of Wnt Signal Strength. Genes Dev (2014) 28:305–16. doi: 10.1101/gad.235473.113
236. An S, Dong S, Wang Q, Li S, Gilbert LI, Stanley D, et al. Insect Neuropeptide Bursicon Homodimers Induce Innate Immune and Stress Genes During Molting by Activating the NF-κb Transcription Factor Relish. PloS One (2012) 7:e34510. doi: 10.1371/journal.pone.0034510
237. Zhang H, Dong S, Chen X, Stanley D, Beerntsen B, Feng Q, et al. Relish2 Mediates Bursicon Homodimer-Induced Prophylactic Immunity in the Mosquito Aedes Aegypti. Sci Rep (2017) 7:43163. doi: 10.1038/srep43163
238. Avsian-Kretchmer O, Hsueh AJW. Comparative Genomic Analysis of the Eight-Membered Ring Cystine Knot-Containing Bone Morphogenetic Protein Antagonists. Mol Endocrinol (2004) 18:1–12. doi: 10.1210/me.2003-0227
239. Nolan K, Thompson TB. The DAN Family: Modulators of TGF-β Signaling and Beyond. Protein Sci (2014) 23:999–1012. doi: 10.1002/pro.2485
240. Nusse R, Clevers H. Wnt/β-Catenin Signaling, Disease, and Emerging Therapeutic Modalities. Cell (2017) 169:985–99. doi: 10.1016/j.cell.2017.05.016
241. Rider CC, Mulloy B. Heparin, Heparan Sulphate and the TGF-β Cytokine Superfamily. Molecules (2017) 22:713. doi: 10.3390/molecules22050713
242. Hauser F, Neupert S, Williamson M, Predel R, Tanaka Y, Grimmelikhuijzen CJP. Genomics and Peptidomics of Neuropeptides and Protein Hormones Present in the Parasitic Wasp Nasonia Vitripennis ∥. J Proteome Res (2010) 9:5296–310. doi: 10.1021/pr100570j
243. Sadd BM, Barribeau SM, Bloch G, de Graaf DC, Dearden P, Elsik CG, et al. The Genomes of Two Key Bumblebee Species With Primitive Eusocial Organization. Genome Biol (2015) 16:76. doi: 10.1186/s13059-015-0623-3
244. Nygaard S, Zhang G, Schiøtt M, Li C, Wurm Y, Hu H, et al. The Genome of the Leaf-Cutting Ant Acromyrmex Echinatior Suggests Key Adaptations to Advanced Social Life and Fungus Farming. Genome Res (2011) 21:1339–48. doi: 10.1101/gr.121392.111
245. Veenstra JA. Neuropeptide Evolution: Neurohormones and Neuropeptides Predicted From the Genomes of Capitella Teleta and Helobdella Robusta. Gen Comp Endocrinol (2011) 171:160–75. doi: 10.1016/j.ygcen.2011.01.005
246. Veenstra JA. Neuropeptide Evolution: Chelicerate Neurohormone and Neuropeptide Genes may Reflect One or More Whole Genome Duplications. Gen Comp Endocrinol (2016) 229:41–55. doi: 10.1016/j.ygcen.2015.11.019
247. Chipman AD, Ferrier DEK, Brena C, Qu J, Hughes DST, Schröder R, et al. The First Myriapod Genome Sequence Reveals Conservative Arthropod Gene Content and Genome Organisation in the Centipede Strigamia Maritima. PloS Biol (2014) 12:e1002005. doi: 10.1371/journal.pbio.1002005
248. Putnam NH, Butts T, Ferrier DEK, Furlong RF, Hellsten U, Kawashima T, et al. The Amphioxus Genome and the Evolution of the Chordate Karyotype. Nature (2008) 453:1064–71. doi: 10.1038/nature06967
249. Hausken K, Levavi-Sivan B. Synteny and Phylogenetic Analysis of Paralogous Thyrostimulin Beta Subunits (GpB5) in Vertebrates. PloS One (2019) 14:e0222808. doi: 10.1371/journal.pone.0222808
250. Cañestro C, Albalat R, Irimia M, Garcia-Fernàndez J. Impact of Gene Gains, Losses and Duplication Modes on the Origin and Diversification of Vertebrates. Semin Cell Dev Biol (2013) 24:83–94. doi: 10.1016/j.semcdb.2012.12.008
251. Maugars G, Dufour S, Cohen-Tannoudji J, Quérat B. Multiple Thyrotropin β Subunit and Thyrotropin Receptor-Related Genes Arose During Vertebrate Evolution. PloS One (2014) 9:e111361. doi: 10.1371/journal.pone.0111361
252. Fleming MS, Maugars G, Lafont A-G, Rancon J, Fontaine R, Nourizadeh-Lillabadi R, et al. Functional Divergence of Thyrotropin Beta-Subunit Paralogs Gives New Insights Into Salmon Smoltification Metamorphosis. Sci Rep (2019) 9:4561. doi: 10.1038/s41598-019-40019-5
253. Irachi S, Hall DJ, Fleming MS, Maugars G, Björnsson BT, Dufour S, et al. Photoperiodic Regulation of Pituitary Thyroid-Stimulating Hormone and Brain Deiodinase in Atlantic Salmon. Mol Cell Endocrinol (2021) 519:111056. doi: 10.1016/j.mce.2020.111056
254. Kitano J, Lema SC, Luckenbach JA, Mori S, Kawagishi Y, Kusakabe M, et al. Adaptive Divergence in the Thyroid Hormone Signaling Pathway in the Stickleback Radiation. Curr Biol (2010) 20:2124–30. doi: 10.1016/j.cub.2010.10.050
Keywords: glycoprotein hormone, TSH, hCG, CGA, unconventional action, cystine knot, GPA & GPB, thyrostimulin
Citation: Querat B (2021) Unconventional Actions of Glycoprotein Hormone Subunits: A Comprehensive Review. Front. Endocrinol. 12:731966. doi: 10.3389/fendo.2021.731966
Received: 28 June 2021; Accepted: 06 September 2021;
Published: 21 September 2021.
Edited by:
Gustavo M. Somoza, Instituto Tecnológico de Chascomús (INTECH) (CONICET), ArgentinaReviewed by:
Ching-Fong Chang, National Taiwan Ocean University, TaiwanHironori Ando, Niigata University, Japan
Copyright © 2021 Querat. This is an open-access article distributed under the terms of the Creative Commons Attribution License (CC BY). The use, distribution or reproduction in other forums is permitted, provided the original author(s) and the copyright owner(s) are credited and that the original publication in this journal is cited, in accordance with accepted academic practice. No use, distribution or reproduction is permitted which does not comply with these terms.
*Correspondence: Bruno Querat, YnJ1bm8ucXVlcmF0QHUtcGFyaXMuZnI=