- 1Department of Orthopaedic Surgery, Sir Run Run Shaw Hospital, Medical College of Zhejiang University, Hangzhou, China
- 2Department of Orthopedic Surgery, Zhejiang Provincial People’s Hospital, People’s Hospital of Hangzhou Medical College, Hangzhou, China
- 3Key Laboratory of Musculoskeletal System Degeneration and Regeneration Translational Research of Zhejiang Province, Hangzhou, China
- 4Department of Orthopaedic Surgery, The Second Affiliated Hospital, Bengbu Medical College, Bengbu, China
- 5Spine Lab, Department of Orthopedic Surgery, The First Affiliated Hospital, Medical College of Zhejiang University, Hangzhou, China
Mesenchymal stem cell-derived exosomes have been under investigation as potential treatments for a diverse range of diseases, and many animal and clinical trials have achieved encouraging results. However, it is well known that the biological activity of the exosomes is key to their therapeutic properties; however, till date, it has not been completely understood. Previous studies have provided different explanations of therapeutic mechanisms of the exosomes, including anti-inflammatory, immunomodulatory, and anti-aging mechanisms. The pathological effects of oxidative stress often include organ damage, inflammation, and disorders of material and energy metabolism. The evidence gathered from research involving animal models indicates that exosomes have antioxidant properties, which can also explain their anti-inflammatory and cytoprotective effects. In this study, we have summarized the antioxidant effects of exosomes in in vivo and in vitro models, and have evaluated the anti-oxidant mechanisms of exosomes by demonstrating a direct reduction in excessive reactive oxygen species (ROS), promotion of intracellular defence of anti-oxidative stress, immunomodulation by inhibiting excess ROS, and alteration of mitochondrial performance. Exosomes exert their cytoprotective and anti-inflammatory properties by regulating the redox environment and oxidative stress, which explains the therapeutic effects of exosomes in a variety of diseases, mechanisms that can be well preserved among different species.
Introduction
Mesenchymal stem cells (MSCs) are pluripotent stem cells that can be isolated from various adult or foetal tissues, including fatty tissue, bone marrow, and cord blood (1). Owing to the unique ability to regenerate and differentiate into other cells, MSCs are widely used to treat various diseases (2). They are considered a promising option for the treatment of various types of diseases, such as limb ischemia, skin wound, and cartilage defects (3–5). Despite the potential to replace damaged tissues with a vast array of cells, the potential risks like tumorigenesis and tissue transplant rejection, and ethical issues problems associated with stem cell therapy greatly limit their clinical use (6, 7). This risk persists for a long time (8). In addition, the preparation method of stem cells and the best storage method to maintain the viability of stem cells also pose challenges to their application (9).
The previous studies have shown that the indirect interactions between donor cells and somatic cells rely on the release of exosomes (10). The release of exosomes is precisely regulated by cells. Exosomes are produced in multi-vesicular bodies (MVBs) containing intraluminal vesicles (ILVs) in the cells (11). ILVs are ultimately secreted as exosomes with a size range of 30–200 nm, through the fusion of MVBs to the plasma membrane and exocytosis (11, 12). A series of proteins such as endosomal sorting complex required for transport (ESCRT), transmembrane 4 superfamily (M4SF), Rab protein, and soluble N-ethylmaleimide-sensitive fusion protein attachment protein receptor (SNARE) participate in exosome formation, transportation, transfer, and release (13–15). Almost all cell types can release exosomes via the plasma membrane. In addition, exosomes transfer specific protein and genetic information to target cells for intercellular communication (16). The protective lipid bilayer membrane provides exosomes with long-term stability in the microenvironment (17). After release, exosomes can be widely distributed in body fluids (i.e., serum, urine, saliva, breast milk, and semen), and circulate throughout the body through the blood. Target cells can take up exosomes in a variety of ways, including direct fusion, clathrin/caveolin-dependent endocytic pathways, macropinocytosis, phagocytosis, and lipid raft-mediated endocytic pathways (15, 18, 19). The uptake of exosomes by cells is an energy-consuming process (20). In addition, different cells take up exosomes in different ways and often rely on one of the above-mentioned pathways. Therefore, the corresponding uptake behaviour can be inhibited by inhibiting its key proteins such as caveolin and clathrin. At the same time, the pH of the environment is also one of the influencing factors. An acidic environment can enhance the uptake of exosomes, mainly because the acidic environment can increase the expression of caveolin-1 on the surface of exosomes (21, 22). In addition, exosomes may also undergo cell uptake and release cycles in multiple cells to exchange substances. The researchers have also conducted bio-distribution studies on exosomes. After oral administration, exosomes are mainly distributed in the liver, lung, kidney, pancreas, spleen, ovary, colon, and brain, but intravenous administration makes them mainly distributed in the liver, followed by spleen, lung, and gastrointestinal tract (23, 24). Intravenous injection leads to rapid elimination of exosomes from the bloodstream (25), while intranasal administration facilitates the delivery of exosomes to the brain (26, 27). Further in most tissues, macrophages often mediate the uptake of exosomes, and the size of exosomes also affects transportation and bio-distribution. Large-sized exosomes are more likely to accumulate in the bone and liver (28).
The components of exosomes are complex and diverse, including various types of lipids, proteins, mRNAs, and microRNAs, which enable them to act as carriers of various signalling molecules in cells (29–31). Numerous pathological processes have been shown to be related to the exosomes, including tumorigenesis, inflammation, cardiovascular disease, and diabetes (32–35). In addition, exosomes have been reported to perform therapeutic functions and regulate receptor cells through intercellular communication (36, 37). In particular, the application of stem cell-derived exosomes has been proven to maintain functions similar to that of stem cells and avoid the obvious side effects of stem-cell therapy (38). Therefore, exosomes show good therapeutic potential in various diseases. For example, previous reports have confirmed the cell-protective effects of exosomes in the heart, skin, and skeletal muscle diseases (39–41). Recently, the role of exosomes in reducing oxidative and nitrosation damage has attracted a lot of attention. In the pathophysiological process of many diseases, redox environment regulation plays an important role. Numerous studies have evaluated the antioxidant effects of exosome in different disease models, such as the damage caused by hyperglycaemia and obesity (42, 43), alcohol-related brain damage (44), Parkinson’s disease (PD) (45), musculoskeletal diseases (e.g., intervertebral disc degeneration (IVDD), radiation-induced bone loss, osteoarthritis (OA)) (46–48), liver injury (49), ischemia injuries (50), colitis (51), and skin wounds (41) (Figure 2). Further, exosomes can directly alleviate oxidative stress in various types of cells such as glial cells (44), neurons (45), cardiomyocytes (52), endothelial cells (53), immune cells (54), hepatocytes (49), and nucleus pulposus cells (46) in vitro.
Oxidative stress plays a key role in the pathophysiology of many diseases, by causing cell damage, inflammation, and metabolic disorders. In all living cells, similar components are responsible for mediating excessive oxidative stress and unbalanced reduction. Therefore, exosomes can regulate these molecular components which can be used to treat different diseases. The functions of exosomes are conserved across species.
Overview of Oxidative Stress
In 1985, Sie et al. first introduced the concept of oxidative stress to the field of redox biology and medicine (55). Oxidative stress is very common under normal physiological conditions, and low levels of oxidative stress may help prevent ageing (56). Mild oxidative stress does not cause any cell damage. It works synergistically with antioxidants to maintain cell homeostasis and plays a role in host defence, gene transcription, and apoptosis (57).
Antioxidants mainly include antioxidant enzymes, such as catalase (CAT), superoxide dismutase (SOD), glutathione peroxidase (GPX), glutathione-S-transferase, and non-enzymatic antioxidant factors, such as melatonin, carotenoid, and some microelements (57). However, when redox homeostasis is disturbed, peroxides and free radicals are produced, which damage proteins, lipids, and DNA. This process is involved in various diseases, such as cancer (58), PD (59), Alzheimer’s disease (60), colitis (51), diabetes (61), liver diseases (62), and the diseases of the musculoskeletal system (63).
The activation of NADPH oxidase (PHOX) is an important pathway to produce reactive oxygen species (ROS), such as H2O2 (hydrogen peroxide), (superoxide radical), OH- (hydroxyl radical), and NO· (nitric oxide). PHOX is a protein that transfers electrons across biological membranes. Generally, the oxygen is the electron acceptor, the procedure of the electron transfer reaction produces superoxide. Therefore, the production of ROS is the key biological function of PHOX (64).
Four cytosolic subunits (p47phox, p40phox, p67phox, and the small GTPase Rac1/2) and b558, a membrane-bound flavocytochrome form the PHOX. PHOX is activated after the phosphorylation of the cytosolic subunit p47phox. PHOX plays an important role in redox signalling, which can protect vascular functions and regulate eukaryotic initiator factor 2α-mediated stress signalling (64). Conversely, overexpression of PHOX results in the production of excessive ROS, triggering cell damage and apoptosis (65).
The leakage of active oxygen from the mitochondria is considered another source of ROS. Mitochondria are the organelles that produce ATP to provide energy for subcellular processes (66). Several processes involved in cell respiration occur within the mitochondria, including the Krebs cycle, oxidative phosphorylation, and fatty acid β-oxidation. Oxidative phosphorylation plays an important role in the cellular respiration and metabolic pathway and is performed by the inner mitochondrial membrane (IMM) protein complexes and molecules. It mainly includes the following two steps: the electron transport chain (ETC) and the synthesis of ATP. Complex I (NADH-Q reductase, FMN, and Fe-S) is the first proton pump in the electron transport chain. It combines with NADH and transfers the two high-energy electrons on NADH to the FMN prosthetic group to oxidize NADH. FMN is reduced to FMNH2, and then the electrons are transferred to Fe-S. Complex II (succinate-Q reductase, FAD, Cytb) transfers the electrons of FADH2 to the electron transport chain via Fe-S, and the relatively high transfer potential energy of the electrons is harnessed. Complex III (Cytochrome reductase, Cytb, Fe-S, Cytc1) catalyses the electrons transfer process from QH2 to cytochrome c. Complex IV (Cytochrome oxidase, CuA, Cyta, Cyta3, CuB) transfers the electrons of the respiratory substrate directly to molecular oxygen through the cytochrome system, and finally generates H2O, and the ROS obtained here can be used as a cell signalling molecule or oxidative stress factor. The antioxidant pathways that neutralise ROS are outlined in Figure 1.
During the mitochondrial respiratory transport chain, the activation of nicotinamide adenine dinucleotide phosphate oxidase (NOX), xanthine oxidase (XO) generated the superoxide radical anion . The superoxide radical anion has two destinations. It can be converted into hydrogen peroxide (H2O2) by superoxide dismutase (SOD), or interact with nitric oxide (NO·) to produce peroxynitrite (ONOO-) with the help of the endothelial nitric oxide NO synthase (eNOS). The catalase (CAT) and/or glutathione peroxidase (GPX) enzymes maintain the stability of the physiological concentration of hydrogen peroxide. The reaction of excess hydrogen peroxide generates hydroxyl radicals with redox metals can generate hydroxyl radicals. The H2O2 removal and redox regulation are dominated by peroxiredoxins (PRX). Peroxiredoxins in the figure are represented by green.
Antioxidant Properties of Exosomes in Various Systems
Exosomes contain various types of lipids, proteins, mRNA and microRNA, etc., which enable the exosomes to as carriers of various signal transduction pathways in cells (67). Exosomes have been found to have therapeutic effects in multiple disorders. In this section, we summarize the anti-oxidative stress activity of exosomes in different systems and the mechanism of their antioxidant function (Figure 2 and Table 1).
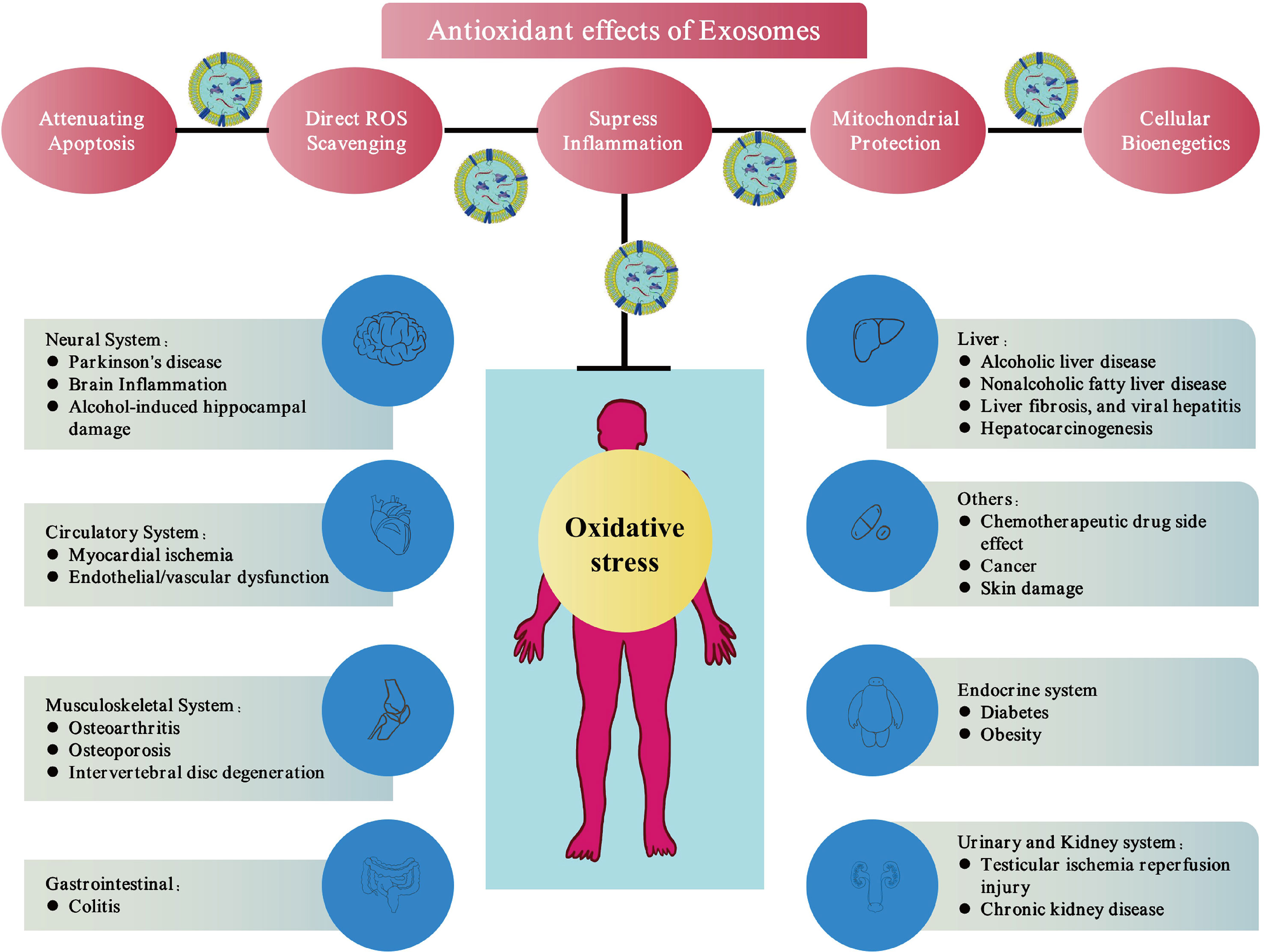
Figure 2 Exosomes therapies via antioxidant effects. Exosomes can eliminate free radicals in cells and donate mitochondrial-related proteins, directly or indirectly up-regulate the antioxidant capacity of cells and improve cell bioenergetics. These mechanisms reduce oxidative stress, which show promising antioxidant properties of exosomes.
Nervous System
As life expectancy increases, ageing-related diseases, such as neurodegenerative diseases have further increased. PD is one of the fastest-growing ageing-related neurological diseases in developed countries (80). Brain tissues from the PD patients exhibit reduced levels of oxidoreductase, CAT, SOD, and other antioxidants (81–83). Due to the blood-brain barrier, the commonly used antioxidant catalase cannot be delivered to the brain (84). Application of the emerging nano-delivery systems, have drawbacks like the toxicity of nanomaterials and quick drug removal by the mononuclear phagocyte system (85). Exosomes are thought to have the ability to cross the blood-brain barrier and can avoid clearance by the immune system due to the membrane layer (26, 27).
Two different studies have investigated the application of catalase encapsulated by exosomes in PD. Kojima et al. constructed human MSC-derived exosomes containing catalase mRNA (45). They used 6-hydroxydopamine (6-OHDA) to produce cytotoxic levels of ROS and damage to neurons. The results showed that the exosomes they designed could rescue neurotoxicity and reduce inflammation. The expression of neuro-inflammation-related factors in the brain such as glial fibrillary acidic protein (GFAP) (86), allograft inflammatory factor 1 (Iba1) (87), tumour necrosis factor α (TNFα) (88), and CD11b (26), were attenuated by the exosomes. Haney et al. conducted a similar study. The exosomes derived from mouse macrophages consisting of catalase directly incorporated into the exosomes (26). The exosomal formulations of catalase they produced could be located within the neurons and microglia, confirming that exosomes can cross the blood-brain barrier. At the same time, the exosomal preparation significantly reduces inflammation in the brain and improved the survival of neurons.
In addition to catalase delivery, studies have also reported that anti-inflammatory drugs delivered by exosomes can play a role in oxidative stress regulation (27). Zhuang et al. used exosomes from EL-4 cells (mouse lymphoma cell line) for curcumin encapsulation and used it to treat lipopolysaccharide (LPS)-induced brain inflammation model. The results showed that by intranasal administration, engineered exosomes can significantly inhibit the number of inflammatory microglia.
Apart from the drug encapsulation, exosomes can play an anti-oxidative stress role by themselves in neurodegenerative diseases. Ezquer et al. used ethanol to induce excessive oxidative stress and neuro-inflammation in rats (9). According to previous reports, alcohol can inhibit the levels of glutamate transporter 1 (GLT1), leading to an increase in the number of inflammatory microglia. Intranasal administration of MSC-derived exosomes significantly increased the expression of GLT1 and rescued the brain oxidative stress damage caused by alcohol.
Digestive System
Increased oxidant stress is recognized as a key factor in most chronic liver diseases, such as viral hepatitis, liver fibrosis, non-alcoholic fatty liver disease, and alcoholic liver disease (89). The progression of hepatocarcinogenesis is often accompanied by the imbalance of intracellular oxidative stress (90). More and more researchers currently use antioxidants as therapeutic agents (91–94). Indeed, there is increasing evidence showing that the therapeutic effects of MSCs are driven by the release of exosomes (16). Moreover, several studies have demonstrated that human MSC may induce tumour growth, whereas MSC-derived exosomes are biologically safe (95).
Several studies have investigated the application of exosomes in liver diseases. Yan et al. isolated human umbilical cord MSC-derived exosomes (hucMSC-Ex) to rescue acute liver injury and liver fibrosis induced by CCl4 and H2O2 (49). They found that hucMSC-Ex relieve CCl4 and H2O2 induced liver injury both in vitro and in vivo, which might be related to the delivery of glutathione peroxidase 1 (GPX1) to eliminate excess ROS and inhibition of oxidative stress-induced apoptosis via upregulation of extracellular-regulated kinase 1/2 (ERK1/2) and B-cell lymphoma-2 (Bcl-2) and downregulation of the inhibitor kappa B kinase β (IKKβ)/nuclear transcription factor kappa B (NF-κB)/caspase-9/caspase-3 pathway. Knockout of GPX1 in hucMSCs abolished the antioxidant and anti-apoptotic capabilities of HucMSC-Ex and weakened its hepatoprotective effects in vitro and in vivo. Jiang et al. performed a similar study (68), where a commonly used hepatoprotective agent (bifendate) was compared to exosomes to evaluate the antioxidant effect of exosomes in liver injury. Interestingly, HucMSC-Exs exhibit a stronger antioxidant effect in the pathological process of liver tumours induced by CCl4. Damania et al. showed that MSC-derived exosomes reduced oxidative stress in in vitro liver injury models (69).
Hyung et al. confirmed that human hepatic progenitor cell (CdH)-derived exosomes (EXO-hCdHs) significantly reduce the oxidative stress response and delay hepatocyte cell death (70). The data showed that EXO-hCdHs inhibited oxidation-induced cell death in hepatocytes. Consistently, EXO-hCdHs activated nuclear factor erythroid 2-related factor 2 (NRF2) expression and induced downstream regulators. NRF2 is an emerging cellular antioxidant regulator, which can induce the expression of antioxidant-related genes to further regulate cellular oxidative stress (96) and protect against an imbalance of ROS (97).
In addition, exosomes attenuated the severity of colitis (51). The therapeutic effect of BMSC-derived exosomes in colitis is related to the suppression of oxidative disturbance, which is manifested by decreased activities of myeloperoxidase and malondialdehyde (MDA), as well as increased levels of SOD and glutathione. These studies indicate that exosomes are promising candidates for the treatment of oxidative stress-related digestive system diseases.
Circulatory System
Atherosclerosis, hypertension, and macrovascular disease, blood vessel damage are common in individuals of advanced age (98). Many researchers have demonstrated that excessive oxidative stress can cause endothelial/vascular dysfunction (99). Therefore, reducing oxidative stress is a very important therapeutic strategy for improving vascular function and treating cardiovascular diseases in the elderly.
In the case of hypoxia/reoxygenation (H/R)-induced injury, senescent endothelial cells have a higher rate of apoptosis, excessive ROS, insufficient nitric oxide (NO), upregulated nicotinamide adenine dinucleotide phosphate oxidase 2 (NOX2), downregulate angiotensin-converting enzyme 2 (ACE2) and eNOS, and compromised tube formation ability. The endothelial progenitor cells triggered by ACE2 can protect endothelial cells through the release of exosomes (53). This effect may be due to the presence of miR-18a, which subsequently downregulates the NOX2/ROS pathway.
Ribeiro-Rodrigues et al. found that the exosomes of cardiomyocytes under ischemic conditions can promote the formation of cardiovascular blood vessels (100). In another similar study, Li et al. collected coronary artery serum exosomes from patients with myocardial ischemia and found that this type of exosomes could promote endothelial cell proliferation and migration, and tube formation (50). They also found that exosomes induced by such ischemic conditions had low levels of miR-939-5p. As miR-939-5p has the ability to downregulate the inducible nitric oxide synthase (iNOS) and NO (related to endothelial cell proliferation and tube formation), ischemia-induced exosomes have the ability to promote blood vessel formation.
Exosomes obtained from cardiomyocytes subjected to hypoxia or inflammation have been found to show anti-oxidative stress function. Wang et al. isolated exosomes from the cardiomyocytes preconditioned using hypoxia and it was found that the exosomes obtained after this treatment had a stronger repair capability and significantly upregulated circHIPK3 than in the exosomes derived from unconditioned cells (72). Previous studies have confirmed that the circRNAs can be transferred to target cells via exosomes (52). It was also shown that exosomes rich in circHIPK3 can reduce levels of apoptosis and oxidative stress in cardiac vascular endothelial cells (shown by the decrease in MDA levels and increased SOD activity), upregulate the anti-apoptotic protein Bcl-2, and downregulate the pro-apoptotic proteins Bax and cleaved caspase-3.
In another study (71), the authors used TNFα to stimulate cardiomyocytes and isolated the subsequently produced exosomes. The exosomes obtained in this way are rich in a variety of miRNAs (microRNA-27a, microRNA-28a, and microRNA-34a). The results show that such miRNA-rich exosomes can cause an imbalance in the NRF2/antioxidant response element (ARE) signalling pathway. As the NRF2/ARE signalling pathway plays an important role in preventing oxidative damage to the cardiomyocytes (101), the authors believe that this type of exosome is related to the oxidative stress of cardiomyocytes.
In addition to the changes in exosomes caused by hypoxia, ischemia, and inflammation, the body’s hormone levels can also regulate the cardiovascular system through exosomes. Melatonin is a type of indole neuroendocrine hormone necessary for maintaining physiological functions (102). Previous studies have shown that melatonin improves the cardiovascular system through the direct downregulation of excessive ROS and indirect antioxidant activity. A study by Feng et al. found that melatonin could stimulate vascular smooth muscle cells to release exosomes containing miR-204/miR-211, which might be able to target bone morphogenetic protein 2 (BMP2), thereby reducing vascular calcification and ageing (73).
Musculoskeletal System
Degenerative diseases of the musculoskeletal system, such as IVDD, OA, and osteoporosis, have had a huge impact on society, and the quality of life of middle-aged and elderly people suffering from these diseases is significantly decreased (80).
Previous studies have shown that the onset of IVDD is closely related to ROS and oxidative stress (103). The study by Xia et al. investigated the effect of MSC-derived exosomes on oxidative stress in degenerating intervertebral discs (46). Mitochondria are the primary ROS-producing organelles and are also the source of organelle damage caused by ROS (104). Excessive mitochondrial ROS production can cause cells to lose homeostasis. Exosomes play an important role in mitochondrial communication between cells and can mediate information transmission between cells (75, 105, 106). They found that exosomes could inhibit the H2O2 induced cell apoptosis and the expression of inflammatory factors (iNOS and interleukin-6). At the same time, exosomes can reduce the production of intracellular ROS and structural abnormalities in mitochondria. This anti-oxidative stress effect may be due to the action of a variety of mitochondria-related proteins in exosomes.
OA is the most common joint disease worldwide (107). Similar to IVDD, many studies have confirmed that inflammation is associated with oxidative stress damage in arthritic chondrocytes which has been reviewed previously (108). Interleukin-1β (IL-1β), a pro-inflammatory factor, stimulates chondrocytes to produce iNOS and NO. Tofiño-Vian et al. found that the use of exosomes derived from adipose tissue-derived MSCs can reduce the levels of nitrite in the medium and the mRNA expression of iNOS in OA chondrocytes (48). Similar to the study of intervertebral discs, Chen et al. investigated the effects of primary chondrocyte exosomes and bone marrow MSC-derived exosomes on the expression of ROS in degenerative chondrocytes in two studies (74, 75). They found that these two types of exosomes can reduce the structural abnormalities of mitochondria and the intracellular ROS production, thereby having a therapeutic effect on cartilage degeneration.
BMSC-derived exosomes have also shown good prospects in bone loss-related diseases. Two studies have found that exosomes can also delay osteoporosis by inhibiting osteoclast metabolism, promote local angiogenesis to prevent femoral head necrosis (109, 110). Zuo et al. found that bone loss caused by radiotherapy is accompanied by excessive oxidative stress, DNA damage, and chromosomal aberrations. BMSC-derived exosomes can reduce the oxidative stress damage of BMSCs caused by irradiation by reducing ROS production, promoting the expression of antioxidant proteins (such as catalase, SOD1, and SOD2) (47), and accelerating DNA repair after radiation.
Other Systems
In addition to the diseases of the above-mentioned systems, exosomes can also exert anti-cancer effects by regulating abnormal oxidative stress metabolism in the tumour microenvironment. Furthermore, a series of studies have applied exosomes to urinary diseases and immune deficiency diseases caused by oxidative stress.
Badawy et al. found that camel milk-derived exosomes showed anticancer effects (77). The milk-derived exosomes significantly increased the activities of antioxidant enzymes (SOD, GPX, and CAT), and decreased the levels of the lipid peroxidation marker MDA and the expression of the oxidative stress marker iNOS in tumour tissues. Zhuang et al. have also reported that a STAT3 inhibitor delivered by exosomes can efficiently inhibit tumour growth (27).
The oxidative stress-related and immunosuppressive effects of chemotherapeutic drugs are well established (111, 112). In an earlier study, Ibrahim et al. have confirmed that cyclophosphamide (CTX) decreased CAT, SOD, and GPX levels, and induced immunosuppression in rats (54). However, compared with CTX-treated animals, the camel milk exosomes significantly reduced the level of lipid peroxidation marker MDA and increased the activity of antioxidant enzymes SOD, GPX, and CAT. In addition, camel milk exosomes can normalize biochemical and immunological parameters.
The progression in chronic kidney disease often leads to loss of kidney function, resulting in ineffective homeostasis. Yoon et al. reported that exosomes derived from melatonin-treated MSCs (MT exosomes) can significantly improve the pathophysiology of chronic kidney disease (79). They demonstrated that the PrPc protein (a highly conserved and ubiquitous glycoprotein) in exosomes improves the immunomodulatory effect and up-regulates antioxidant proteins in the cell (113, 114), thus improving the regenerative potential of MSCs and attenuating ischemia-induced oxidative stress.
Testicular ischemia-reperfusion injury (IRI) is the main pathophysiological process of surgical reduction after testicular torsion. A large number of oxygen free radicals and inflammatory cytokines play an important role in the pathophysiological process of IRI (115, 116). Zhang et al. found that the treatment of BMSC-derived exosomes can increase the SOD activity of ischemia-reperfusion-injured testicular tissue and decrease MDA content (78). This preliminary study indicated that BMSC-derived exosomes can reduce testicular IRI to protect spermatogenesis.
Diseases such as diabetes and obesity are metabolism-related diseases, which are often accompanied by increased inflammation and oxidative stress (117, 118). For example, in diabetic ulcers, the number of ROS-releasing macrophages and neutrophils increases (119, 120), often leading to wounds that cannot heal. A previous study reported that the amputation rate in diabetic patients was 10-20 times higher than that of non-diabetic patients (121). Shiekh et al. extracted exosomes derived from adipose-derived stem cells (ADSCs) and combined them with a scaffold capable of releasing oxygen (42). The composite structure of the scaffold can significantly enhance the healing of diabetic wounds. At the same time, their research showed that ADSC-derived exosomes can reduce the effects of hyperglycaemia by reducing oxidative stress, thereby increasing the metabolic activity of cells. Exosomes can not only promote the healing of diabetic wounds, but Wang et al. found that exosomes can also reduce skin oxidative stress damage caused by ultraviolet rays (76). In their study, MSC-derived exosomes reduced ROS production, DNA damage, and mitochondrial changes, in a process regulated by the NRF2 defence system.
Low-grade inflammation accompanied by obesity often results in the infiltration of immune cells into adipose tissue (122). The inflammation of adipose tissue leads to the downregulation of α-ketoglutarate (αKG), which is a target for melatonin inhibition of adipose inflammation. A study by Liu et al. demonstrated that the melatonin increased the release of adipose-derived exosomal αKG, which in turn attenuated signal transduction and activation of transduction-3 (STAT3)/NF-κB signalling pathway by its receptor, oxoglutarate receptor 1 (OXGR1), in adipocytes (43). Therefore, melatonin may have the ability to prevent and treat systemic inflammatory diseases caused by obesity through exosomes.
Role of Exosomes in Inflammation and Degeneration
Under normal physiological conditions classically activated (M1) macrophages secrete pro-inflammatory factors such as TNFα, interleukin-6, and IL-1β, while alternatively activated (M2) macrophages show an anti-inflammatory phenotype (123, 124). Additionally, regulatory T cells (Tregs) and type 2 T helper (Th2) cells secrete anti-inflammatory factors (125). These cells maintain the homeostasis of the immune microenvironment. When cells are stimulated by harmful factors such as toxic chemicals, heat, abnormal pressure, tumours, ageing, or degeneration the cellular inflammatory response triggers a series of reactions (126). The inflammation-related cells secrete exosomes that contain pathogen-related and injury-related molecules and pathogenic antigens (127). In addition, the proteases and glycosidases in these exosomes also cause tissue destruction. This indicates that exosomes play a key role in the process of inflammation (127). Therefore, exosomes are important targets for the treatment of inflammation-related diseases in the future.
In addition to inflammation-related diseases, exosomes also have treatment potential in the degenerative diseases. For example, in PD, glial cell-derived exosomes can transmit beneficial or harmful information to neurons through their internal cargo (128). As exosomes can penetrate the blood-brain barrier, the exosomes released by neuronal cells can be detected in serum or plasma. Since exosomal constituents can provide information about their source cells, exosomes have the potential to become a new type of disease diagnostic markers (129). Moreover, researchers have revealed the relationship between endothelial cell inflammation and brain degeneration by analysing endothelium-derived exosomes isolated from plasma (130).
Many studies have confirmed the ability of MSCs to inhibit inflammation. As MSCs mainly exert biological effects by secreting exosomes, an increasing amount of research is focused on exploring the anti-inflammatory effect of MSC-derived exosomes. The study of this mechanism has shown that MSC-derived exosomes can induce polarization of macrophages from M1 type to M2 type (131–133), thereby changing the phenotype of macrophages from pro-inflammatory to anti-inflammatory. Some investigators have also found that MSC-derived exosomes can regulate the function of T cells, inducing the transition from type 1 T helper (Th1) cells to Th2 cells (134). MSC-derived exosomes have also become a next-generation treatment strategy for degenerative diseases such as PD (135), OA (136), IVDD (137), and macular and retinal degeneration (138). These abilities of MSC-derived exosomes are mainly exerted by the constituent proteins, mRNA, and miRNA, which attenuate chronic inflammation, reduce apoptosis and stimulate proliferation (139).
Future Perspectives
According to the presented literature review, it can be seen that the anti-oxidative stress properties of exosomes have been confirmed in multiple systems, which proves the potential of exosomes as a therapeutic agent against oxidative stress. However, before further application, the following hurdles still need to be overcome. First, exosomes are highly heterogeneous, showing a high degree of diversity in the size of exosomes derived from different cell sources, the internal biological components, and their effect on the function of the recipient cells (28). Exosomes derived from the MSCs, a common source of exosomes, exhibit huge differences in the expression of MSC membrane markers and proteomic characteristics even when derived from the same tissue (140). The cell microenvironment and internal biology may also affect the content of exosomes and their biomarkers (29–31, 52). Based on an in-depth understanding of their heterogeneity, it is necessary to accurately characterize the exosomes used in different experiments.
Secondly, how exosomes affect the function of recipient cells requires further study. At present, most studies have focused on the components of exosomes (such as proteins, miRNAs, and circRNAs) that exert antioxidant effects on recipient cells. However, the behaviour of these components after entering the cell needs further investigation. The location of these components in the cell and whether they are degraded by lysosomes is unknown. Existing studies have shown that exosomes may be taken up by cells in different ways. For example, human melanoma cells take up cargo in exosomes through membrane fusion (21), while in neurosecretory PC12 cells (derived from rat adrenal medullary tumours), exosomes are more dependent on clathrin-dependent endocytosis for uptake (19). How different types of exosomes enter the cell and deliver cargos (i.e., membrane fusion, macropinocytosis, phagocytosis, and clathrin-dependent endocytosis), and what factors affect this process, need to be further investigated (141, 142).
Thirdly, although it is assumed that exosomes can functionally deliver their internal miRNA to target cells there is still a lack of direct evidence for exosomes mediated miRNA transfer. As lipoproteins are inevitably mixed with exosomes in the current exosome extraction process and lipoproteins also have the function of transferring miRNA (143), it is necessary to purify exosomes to determine their efficiency of miRNA delivery.
Lastly, the current research on exosomes is limited to cellular or animal models, and there are very few human studies. Although the studies reviewed in this article demonstrate the potential of exosomes to resist oxidative stress at the cellular and organ levels, more clinical trials need to be conducted to test the feasibility of this strategy.
Conclusion
The findings summarized here demonstrate that MSC-derived exosomes and exosomal formulations show excellent antioxidant properties. Growing evidence shows that exosomes can eliminate excessive ROS in cells and deliver mitochondrial protective proteins, thereby improving the antioxidant capacity of cells and enhancing cell viability. Future research should focus on elucidating the specific differences in the antioxidant mechanisms of exosomes in different diseases. Similarly, the in the future, research should be focused on targeted tissue engineering of exosomes, such as increasing the content of specific antioxidant enzymes or mitochondria related proteins in exosomes to further enhance the efficacy of exosomes. The MSC-derived exosome-based therapy has promising application prospects in multiple systemic diseases.
Author Contributions
Conceptualization, PC and CX. Writing—review and editing, CX, ZD, YJ, and PC. All authors contributed to the article and approved the submitted version.
Funding
The study was supported by the National Key R&D Program of China (Grant No. 2020YFC1107104), the National Nature Science Fund of China (Grant No. 81802147, and 81772387), and the Natural Science Fund of Zhejiang Province (Grant No. LY21H060002).
Conflict of Interest
The authors declare that the research was conducted in the absence of any commercial or financial relationships that could be construed as a potential conflict of interest.
Publisher’s Note
All claims expressed in this article are solely those of the authors and do not necessarily represent those of their affiliated organizations, or those of the publisher, the editors and the reviewers. Any product that may be evaluated in this article, or claim that may be made by its manufacturer, is not guaranteed or endorsed by the publisher.
References
1. Squillaro T, Peluso G, Galderisi U. Clinical Trials With Mesenchymal Stem Cells: An Update. Cell Transplant (2016) 25(5):829–48. doi: 10.3727/096368915X689622
2. Minguell JJ, Erices A, Conget P. Mesenchymal Stem Cells. Exp Biol Med (Maywood) (2001) 226(6):507–20. doi: 10.1177/153537020122600603
3. Maharlooei MK, Bagheri M, Solhjou Z, Jahromi BM, Akrami M, Rohani L, et al. Adipose Tissue Derived Mesenchymal Stem Cell (AD-MSC) Promotes Skin Wound Healing in Diabetic Rats. Diabetes Res Clin Pract (2011) 93(2):228–34. doi: 10.1016/j.diabres.2011.04.018
4. Nammian P, Asadi-Yousefabad SL, Daneshi S, Sheikhha MH, Tabei SMB, Razban V. Comparative Analysis of Mouse Bone Marrow and Adipose Tissue Mesenchymal Stem Cells for Critical Limb Ischemia Cell Therapy. Stem Cell Res Ther (2021) 12(1):58. doi: 10.1186/s13287-020-02110-x
5. Ryu DJ, Jeon YS, Park JS, Bae GC, Kim JS, Kim MK. Comparison of Bone Marrow Aspirate Concentrate and Allogenic Human Umbilical Cord Blood Derived Mesenchymal Stem Cell Implantation on Chondral Defect of Knee: Assessment of Clinical and Magnetic Resonance Imaging Outcomes at 2-Year Follow-Up. Cell Transplant (2020) 29:963689720943581. doi: 10.1177/0963689720943581
6. Barkholt L, Flory E, Jekerle V, Lucas-Samuel S, Ahnert P, Bisset L, et al. Risk of Tumorigenicity in Mesenchymal Stromal Cell-Based Therapies–Bridging Scientific Observations and Regulatory Viewpoints. Cytotherapy (2013) 15(7):753–9. doi: 10.1016/j.jcyt.2013.03.005
7. Veceric-Haler Z, Cerar A, Perse M. (Mesenchymal) Stem Cell-Based Therapy in Cisplatin-Induced Acute Kidney Injury Animal Model: Risk of Immunogenicity and Tumorigenicity. Stem Cells Int (2017) 2017:7304643. doi: 10.1155/2017/7304643
8. Park SJ, Kim HJ, Kim W, Kim OS, Lee S, Han SY, et al. Tumorigenicity Evaluation of Umbilical Cord Blood-Derived Mesenchymal Stem Cells. Toxicol Res (2016) 32(3):251–8. doi: 10.5487/TR.2016.32.3.251
9. Gonzalez Villarreal C, Said Fernandez S, Soto Dominguez A, Padilla Rivas G, Garza Trevino E, Rodriguez Rocha H, et al. Bone Marrow Mesenchymal Stem Cells: Improving Transgene Expression Level, Transfection Efficiency and Cell Viability. J BUON (2018) 23(6):1893–903.
10. Lai RC, Arslan F, Lee MM, Sze NS, Choo A, Chen TS, et al. Exosome Secreted by MSC Reduces Myocardial Ischemia/Reperfusion Injury. Stem Cell Res (2010) 4(3):214–22. doi: 10.1016/j.scr.2009.12.003
11. Huang L, Ma W, Ma Y, Feng D, Chen H, Cai B. Exosomes in Mesenchymal Stem Cells, a New Therapeutic Strategy for Cardiovascular Diseases? Int J Biol Sci (2015) 11(2):238–45. doi: 10.7150/ijbs.10725
12. Kourembanas S. Exosomes: Vehicles of Intercellular Signaling, Biomarkers, and Vectors of Cell Therapy. Annu Rev Physiol (2015) 77:13–27. doi: 10.1146/annurev-physiol-021014-071641
13. Hurley JH. ESCRT Complexes and the Biogenesis of Multivesicular Bodies. Curr Opin Cell Biol (2008) 20(1):4–11. doi: 10.1016/j.ceb.2007.12.002
14. Ostrowski M, Carmo NB, Krumeich S, Fanget I, Raposo G, Savina A, et al. Rab27a and Rab27b Control Different Steps of the Exosome Secretion Pathway. Nat Cell Biol (2010) 12(1):19–301–13. doi: 10.1038/ncb2000
15. McKelvey KJ, Powell KL, Ashton AW, Morris JM, McCracken SA. Exosomes: Mechanisms of Uptake. J Circ Biomark (2015) 4:7. doi: 10.5772/61186
16. Mathieu M, Martin-Jaular L, Lavieu G, Thery C. Specificities of Secretion and Uptake of Exosomes and Other Extracellular Vesicles for Cell-to-Cell Communication. Nat Cell Biol (2019) 21(1):9–17. doi: 10.1038/s41556-018-0250-9
17. Nagashima S, Jirintai S, Takahashi M, Kobayashi T, Tanggis, Nishizawa T, et al. Hepatitis E Virus Egress Depends on the Exosomal Pathway, With Secretory Exosomes Derived From Multivesicular Bodies. J Gen Virol (2014) 95(Pt 10):2166–75. doi: 10.1099/vir.0.066910-0
18. Svensson KJ, Christianson HC, Wittrup A, Bourseau-Guilmain E, Lindqvist E, Svensson LM, et al. Exosome Uptake Depends on ERK1/2-Heat Shock Protein 27 Signaling and Lipid Raft-Mediated Endocytosis Negatively Regulated by Caveolin-1. J Biol Chem (2013) 288(24):17713–24. doi: 10.1074/jbc.M112.445403
19. Tian T, Zhu YL, Zhou YY, Liang GF, Wang YY, Hu FH, et al. Exosome Uptake Through Clathrin-Mediated Endocytosis and Macropinocytosis and Mediating miR-21 Delivery. J Biol Chem (2014) 289(32):22258–67. doi: 10.1074/jbc.M114.588046
20. Horibe S, Tanahashi T, Kawauchi S, Murakami Y, Rikitake Y. Mechanism of Recipient Cell-Dependent Differences in Exosome Uptake. BMC Cancer (2018) 18(1):47. doi: 10.1186/s12885-017-3958-1
21. Parolini I, Federici C, Raggi C, Lugini L, Palleschi S, De Milito A, et al. Microenvironmental pH is a Key Factor for Exosome Traffic in Tumor Cells. J Biol Chem (2009) 284(49):34211–22. doi: 10.1074/jbc.M109.041152
22. Iessi E, Logozzi M, Lugini L, Azzarito T, Federici C, Spugnini EP, et al. Acridine Orange/exosomes Increase the Delivery and the Effectiveness of Acridine Orange in Human Melanoma Cells: A New Prototype for Theranostics of Tumors. J Enzyme Inhib Med Chem (2017) 32(1):648–57. doi: 10.1080/14756366.2017.1292263
23. Morishita M, Takahashi Y, Nishikawa M, Takakura Y. Pharmacokinetics of Exosomes-An Important Factor for Elucidating the Biological Roles of Exosomes and for the Development of Exosome-Based Therapeutics. J Pharm Sci (2017) 106(9):2265–9. doi: 10.1016/j.xphs.2017.02.030
24. Murphy DE, de Jong OG, Brouwer M, Wood MJ, Lavieu G, Schiffelers RM, et al. Extracellular Vesicle-Based Therapeutics: Natural Versus Engineered Targeting and Trafficking. Exp Mol Med (2019) 51(3):1–12. doi: 10.1038/s12276-019-0223-5
25. Smyth T, Kullberg M, Malik N, Smith-Jones P, Graner MW, Anchordoquy TJ. Biodistribution and Delivery Efficiency of Unmodified Tumor-Derived Exosomes. J Control Release (2015) 199:145–55. doi: 10.1016/j.jconrel.2014.12.013
26. Haney MJ, Klyachko NL, Zhao Y, Gupta R, Plotnikova EG, He Z, et al. Exosomes as Drug Delivery Vehicles for Parkinson's Disease Therapy. J Control Release (2015) 207:18–30. doi: 10.1016/j.jconrel.2015.03.033
27. Zhuang X, Xiang X, Grizzle W, Sun D, Zhang S, Axtell RC, et al. Treatment of Brain Inflammatory Diseases by Delivering Exosome Encapsulated Anti-Inflammatory Drugs From the Nasal Region to the Brain. Mol Ther (2011) 19(10):1769–79. doi: 10.1038/mt.2011.164
28. Zhang H, Freitas D, Kim HS, Fabijanic K, Li Z, Chen H, et al. Identification of Distinct Nanoparticles and Subsets of Extracellular Vesicles by Asymmetric Flow Field-Flow Fractionation. Nat Cell Biol (2018) 20(3):332–43. doi: 10.1038/s41556-018-0040-4
29. Keerthikumar S, Chisanga D, Ariyaratne D, Al Saffar H, Anand S, Zhao K, et al. ExoCarta: A Web-Based Compendium of Exosomal Cargo. J Mol Biol (2016) 428(4):688–92. doi: 10.1016/j.jmb.2015.09.019
30. Pathan M, Fonseka P, Chitti SV, Kang T, Sanwlani R, Van Deun J, et al. Vesiclepedia 2019: A Compendium of RNA, Proteins, Lipids and Metabolites in Extracellular Vesicles. Nucleic Acids Res (2019) 47(D1):D516–9. doi: 10.1093/nar/gky1029
31. van Balkom BW, Eisele AS, Pegtel DM, Bervoets S, Verhaar MC. Quantitative and Qualitative Analysis of Small RNAs in Human Endothelial Cells and Exosomes Provides Insights Into Localized RNA Processing, Degradation and Sorting. J Extracell Vesicles (2015) 4:26760. doi: 10.3402/jev.v4.26760
32. Ko KW, Yoo YI, Kim JY, Choi B, Park SB, Park W, et al. Attenuation of Tumor Necrosis Factor-Alpha Induced Inflammation by Umbilical Cord-Mesenchymal Stem Cell Derived Exosome-Mimetic Nanovesicles in Endothelial Cells. Tissue Eng Regener Med (2020) 17(2):155–63. doi: 10.1007/s13770-019-00234-7
33. Xu Z, Zeng S, Gong Z, Yan Y. Exosome-Based Immunotherapy: A Promising Approach for Cancer Treatment. Mol Cancer (2020) 19(1):160. doi: 10.1186/s12943-020-01278-3
34. Zhang TR, Huang WQ. Angiogenic Exosome-Derived microRNAs: Emerging Roles in Cardiovascular Disease. J Cardiovasc Transl Res (2020) 14(5):824–40. doi: 10.1007/s12265-020-10082-9
35. Wu Y, Huang Q, Bu S. Cross Talk Between Exosomes and Pancreatic Beta-Cells in Diabetes. Arch Physiol Biochem (2020) 1–10. doi: 10.1080/13813455.2020.1760303
36. Meldolesi J. Exosomes and Ectosomes in Intercellular Communication. Curr Biol (2018) 28(8):R435–44. doi: 10.1016/j.cub.2018.01.059
37. Mathivanan S, Ji H, Simpson RJ. Exosomes: Extracellular Organelles Important in Intercellular Communication. J Proteomics (2010) 73(10):1907–20. doi: 10.1016/j.jprot.2010.06.006
38. Kalluri R, LeBleu VS. The Biology, Function, and Biomedical Applications of Exosomes. Science (2020) 367(6478):eaau6977. doi: 10.1126/science.aau6977
39. Li N, Rochette L, Wu Y, Rosenblatt-Velin N. New Insights Into the Role of Exosomes in the Heart After Myocardial Infarction. J Cardiovasc Transl Res (2019) 12(1):18–27. doi: 10.1007/s12265-018-9831-z
40. Choi JS, Yoon HI, Lee KS, Choi YC, Yang SH, Kim IS, et al. Exosomes From Differentiating Human Skeletal Muscle Cells Trigger Myogenesis of Stem Cells and Provide Biochemical Cues for Skeletal Muscle Regeneration. J Control Release (2016) 222:107–15. doi: 10.1016/j.jconrel.2015.12.018
41. An Y, Lin S, Tan X, Zhu S, Nie F, Zhen Y, et al. Exosomes From Adipose-Derived Stem Cells and Application to Skin Wound Healing. Cell Prolif (2021) 54(3):e12993. doi: 10.1111/cpr.12993
42. Shiekh PA, Singh A, Kumar A. Exosome Laden Oxygen Releasing Antioxidant and Antibacterial Cryogel Wound Dressing OxOBand Alleviate Diabetic and Infectious Wound Healing. Biomaterials (2020) 249:120020. doi: 10.1016/j.biomaterials.2020.120020
43. Liu Z, Gan L, Zhang T, Ren Q, Sun C. Melatonin Alleviates Adipose Inflammation Through Elevating Alpha-Ketoglutarate and Diverting Adipose-Derived Exosomes to Macrophages in Mice. J Pineal Res (2018) 64(1):e12455. doi: 10.1111/jpi.12455
44. Ezquer F, Quintanilla ME, Morales P, Santapau D, Ezquer M, Kogan MJ, et al. Intranasal Delivery of Mesenchymal Stem Cell-Derived Exosomes Reduces Oxidative Stress and Markedly Inhibits Ethanol Consumption and Post-Deprivation Relapse Drinking. Addict Biol (2019) 24(5):994–1007. doi: 10.1111/adb.12675
45. Kojima R, Bojar D, Rizzi G, Hamri GC, El-Baba MD, Saxena P, et al. Designer Exosomes Produced by Implanted Cells Intracerebrally Deliver Therapeutic Cargo for Parkinson's Disease Treatment. Nat Commun (2018) 9(1):1305. doi: 10.1038/s41467-018-03733-8
46. Xia C, Zeng Z, Fang B, Tao M, Gu C, Zheng L, et al. Mesenchymal Stem Cell-Derived Exosomes Ameliorate Intervertebral Disc Degeneration via Anti-Oxidant and Anti-Inflammatory Effects. Free Radic Biol Med (2019) 143:1–15. doi: 10.1016/j.freeradbiomed.2019.07.026
47. Zuo R, Liu M, Wang Y, Li J, Wang W, Wu J, et al. BM-MSC-Derived Exosomes Alleviate Radiation-Induced Bone Loss by Restoring the Function of Recipient BM-MSCs and Activating Wnt/beta-Catenin Signaling. Stem Cell Res Ther (2019) 10(1):30. doi: 10.1186/s13287-018-1121-9
48. Tofino-Vian M, Guillen MI, Perez Del Caz MD, Silvestre A, Alcaraz MJ. Microvesicles From Human Adipose Tissue-Derived Mesenchymal Stem Cells as a New Protective Strategy in Osteoarthritic Chondrocytes. Cell Physiol Biochem (2018) 47(1):11–25. doi: 10.1159/000489739
49. Yan Y, Jiang W, Tan Y, Zou S, Zhang H, Mao F, et al. hucMSC Exosome-Derived GPX1 Is Required for the Recovery of Hepatic Oxidant Injury. Mol Ther (2017) 25(2):465–79. doi: 10.1016/j.ymthe.2016.11.019
50. Li H, Liao Y, Gao L, Zhuang T, Huang Z, Zhu H, et al. Coronary Serum Exosomes Derived From Patients With Myocardial Ischemia Regulate Angiogenesis Through the miR-939-Mediated Nitric Oxide Signaling Pathway. Theranostics (2018) 8(8):2079–93. doi: 10.7150/thno.21895
51. Yang J, Liu XX, Fan H, Tang Q, Shou ZX, Zuo DM, et al. Extracellular Vesicles Derived From Bone Marrow Mesenchymal Stem Cells Protect Against Experimental Colitis via Attenuating Colon Inflammation, Oxidative Stress and Apoptosis. PLoS One (2015) 10(10):e0140551. doi: 10.1371/journal.pone.0140551
52. Lasda E, Parker R. Circular RNAs Co-Precipitate With Extracellular Vesicles: A Possible Mechanism for circRNA Clearance. PLoS One (2016) 11(2):e0148407. doi: 10.1371/journal.pone.0148407
53. Zhang C, Wang J, Ma X, Wang W, Zhao B, Chen Y, et al. ACE2-EPC-EXs Protect Ageing ECs Against Hypoxia/Reoxygenation-Induced Injury Through the miR-18a/Nox2/ROS Pathway. J Cell Mol Med (2018) 22(3):1873–82. doi: 10.1111/jcmm.13471
54. Ibrahim HM, Mohammed-Geba K, Tawfic AA, El-Magd MA. Camel Milk Exosomes Modulate Cyclophosphamide-Induced Oxidative Stress and Immuno-Toxicity in Rats. Food Funct (2019) 10(11):7523–32. doi: 10.1039/C9FO01914F
55. Sies H, Cadenas E. Oxidative Stress: Damage to Intact Cells and Organs. Philos Trans R Soc Lond B Biol Sci (1985) 311(1152):617–31. doi: 10.1098/rstb.1985.0168
56. Gems D, Partridge L. Stress-Response Hormesis and Aging: "That Which Does Not Kill Us Makes Us Stronger". Cell Metab (2008) 7(3):200–3. doi: 10.1016/j.cmet.2008.01.001
57. Jiang T, Sun Q, Chen S. Oxidative Stress: A Major Pathogenesis and Potential Therapeutic Target of Antioxidative Agents in Parkinson's Disease and Alzheimer's Disease. Prog Neurobiol (2016) 147:1–19. doi: 10.1016/j.pneurobio.2016.07.005
58. Hayes JD, Dinkova-Kostova AT, Tew KD. Oxidative Stress in Cancer. Cancer Cell (2020) 38(2):167–97. doi: 10.1016/j.ccell.2020.06.001
59. Henchcliffe C, Beal MF. Mitochondrial Biology and Oxidative Stress in Parkinson Disease Pathogenesis. Nat Clin Pract Neurol (2008) 4(11):600–9. doi: 10.1038/ncpneuro0924
60. Tramutola A, Lanzillotta C, Perluigi M, Butterfield DA. Oxidative Stress, Protein Modification and Alzheimer Disease. Brain Res Bull (2017) 133:88–96. doi: 10.1016/j.brainresbull.2016.06.005
61. Zhang P, Li T, Wu X, Nice EC, Huang C, Zhang Y. Oxidative Stress and Diabetes: Antioxidative Strategies. Front Med (2020) 14(5):583–600. doi: 10.1007/s11684-019-0729-1
62. Ramos-Tovar E, Muriel P. Molecular Mechanisms That Link Oxidative Stress, Inflammation, and Fibrosis in the Liver. Antioxidants (Basel) (2020) 9(12):1279. doi: 10.3390/antiox9121279
63. Scicchitano BM, Pelosi L, Sica G, Musaro A. The Physiopathologic Role of Oxidative Stress in Skeletal Muscle. Mech Ageing Dev (2018) 170:37–44. doi: 10.1016/j.mad.2017.08.009
64. Sies H, Berndt C, Jones DP. Oxidative Stress. Annu Rev Biochem (2017) 86:715–48. doi: 10.1146/annurev-biochem-061516-045037
65. Lee HN, Surh YJ. Resolvin D1-Mediated NOX2 Inactivation Rescues Macrophages Undertaking Efferocytosis From Oxidative Stress-Induced Apoptosis. Biochem Pharmacol (2013) 86(6):759–69. doi: 10.1016/j.bcp.2013.07.002
66. Mollazadeh H, Tavana E, Fanni G, Bo S, Banach M, Pirro M, et al. Effects of Statins on Mitochondrial Pathways. J Cachexia Sarcopenia Muscle (2021) 12(2):237–51. doi: 10.1002/jcsm.12654
67. Tkach M, Thery C. Communication by Extracellular Vesicles: Where We Are and Where We Need to Go. Cell (2016) 164(6):1226–32. doi: 10.1016/j.cell.2016.01.043
68. Jiang W, Tan Y, Cai M, Zhao T, Mao F, Zhang X, et al. Human Umbilical Cord MSC-Derived Exosomes Suppress the Development of CCl4-Induced Liver Injury Through Antioxidant Effect. Stem Cells Int (2018) 2018:6079642. doi: 10.1155/2018/6079642
69. Damania A, Jaiman D, Teotia AK, Kumar A. Mesenchymal Stromal Cell-Derived Exosome-Rich Fractionated Secretome Confers a Hepatoprotective Effect in Liver Injury. Stem Cell Res Ther (2018) 9(1):31. doi: 10.1186/s13287-017-0752-6
70. Hyung S, Jeong J, Shin K, Kim JY, Yim JH, Yu CJ, et al. Exosomes Derived From Chemically Induced Human Hepatic Progenitors Inhibit Oxidative Stress Induced Cell Death. Biotechnol Bioeng (2020) 117(9):2658–67. doi: 10.1002/bit.27447
71. Tian C, Gao L, Zimmerman MC, Zucker IH. Myocardial Infarction-Induced microRNA-Enriched Exosomes Contribute to Cardiac Nrf2 Dysregulation in Chronic Heart Failure. Am J Physiol Heart Circ Physiol (2018) 314(5):H928–39. doi: 10.1152/ajpheart.00602.2017
72. Wang Y, Zhao R, Liu W, Wang Z, Rong J, Long X, et al. Exosomal Circhipk3 Released From Hypoxia-Pretreated Cardiomyocytes Regulates Oxidative Damage in Cardiac Microvascular Endothelial Cells via the miR-29a/IGF-1 Pathway. Oxid Med Cell Longev (2019) 2019:7954657. doi: 10.1155/2019/7954657
73. Xu F, Zhong JY, Lin X, Shan SK, Guo B, Zheng MH, et al. Melatonin Alleviates Vascular Calcification and Ageing Through Exosomal miR-204/miR-211 Cluster in a Paracrine Manner. J Pineal Res (2020) 68(3):e12631. doi: 10.1111/jpi.12631
74. Zheng L, Wang Y, Qiu P, Xia C, Fang Y, Mei S, et al. Primary Chondrocyte Exosomes Mediate Osteoarthritis Progression by Regulating Mitochondrion and Immune Reactivity. Nanomedicine (Lond) (2019) 14(24):3193–212. doi: 10.2217/nnm-2018-0498
75. Chen P, Zheng L, Wang Y, Tao M, Xie Z, Xia C, et al. Desktop-Stereolithography 3D Printing of a Radially Oriented Extracellular Matrix/Mesenchymal Stem Cell Exosome Bioink for Osteochondral Defect Regeneration. Theranostics (2019) 9(9):2439–59. doi: 10.7150/thno.31017
76. Wang T, Jian Z, Baskys A, Yang J, Li J, Guo H, et al. MSC-Derived Exosomes Protect Against Oxidative Stress-Induced Skin Injury via Adaptive Regulation of the NRF2 Defense System. Biomaterials (2020) 257:120264. doi: 10.1016/j.biomaterials.2020.120264
77. Badawy AA, El-Magd MA, AlSadrah SA. Therapeutic Effect of Camel Milk and Its Exosomes on MCF7 Cells In Vitro and In Vivo. Integr Cancer Ther (2018) 17(4):1235–46. doi: 10.1177/1534735418786000
78. Zhang W, Yang C, Guo W, Guo X, Bian J, Zhou Q, et al. Rotective Effect of Bone Marrow Mesenchymal Stem Cells-Derived Exosomes Against Testicular Ischemia-Reperfusion Injury in Rats. Nan Fang Yi Ke Da Xue Xue Bao (2018) 38(8):910–6. doi: 10.3969/j.issn.1673-4254.2018.08.02
79. Yoon YM, Lee JH, Song KH, Noh H, Lee SH. Melatonin-Stimulated Exosomes Enhance the Regenerative Potential of Chronic Kidney Disease-Derived Mesenchymal Stem/Stromal Cells via Cellular Prion Proteins. J Pineal Res (2020) 68(3):e12632. doi: 10.1111/jpi.12632
80. Disease GBD, Injury I, Prevalence C. Global, Regional, and National Incidence, Prevalence, and Years Lived With Disability for 354 Diseases and Injuries for 195 Countries and Territories, 1990-2017: A Systematic Analysis for the Global Burden of Disease Study 2017. Lancet (2018) 392(10159):1789–858. doi: 10.1016/S0140-6736(18)32279-7
81. Ambani LM, Van Woert MH, Murphy S. Brain Peroxidase and Catalase in Parkinson Disease. Arch Neurol (1975) 32(2):114–8. doi: 10.1001/archneur.1975.00490440064010
82. Riederer P, Sofic E, Rausch WD, Schmidt B, Reynolds GP, Jellinger K, et al. Transition Metals, Ferritin, Glutathione, and Ascorbic Acid in Parkinsonian Brains. J Neurochem (1989) 52(2):515–20. doi: 10.1111/j.1471-4159.1989.tb09150.x
83. Abraham S, Soundararajan CC, Vivekanandhan S, Behari M. Erythrocyte Antioxidant Enzymes in Parkinson's Disease. Indian J Med Res (2005) 121(2):111–5.
84. Pardridge WM. Drug Transport Across the Blood-Brain Barrier. J Cereb Blood Flow Metab (2012) 32(11):1959–72. doi: 10.1038/jcbfm.2012.126
85. Peng Q, Zhang S, Yang Q, Zhang T, Wei XQ, Jiang L, et al. Preformed Albumin Corona, a Protective Coating for Nanoparticles Based Drug Delivery System. Biomaterials (2013) 34(33):8521–30. doi: 10.1016/j.biomaterials.2013.07.102
86. Guerriero F, Sgarlata C, Francis M, Maurizi N, Faragli A, Perna S, et al. Neuroinflammation, Immune System and Alzheimer Disease: Searching for the Missing Link. Aging Clin Exp Res (2017) 29(5):821–31. doi: 10.1007/s40520-016-0637-z
87. Tripanichkul W, Jaroensuppaperch EO. Ameliorating Effects of Curcumin on 6-OHDA-Induced Dopaminergic Denervation, Glial Response, and SOD1 Reduction in the Striatum of Hemiparkinsonian Mice. Eur Rev Med Pharmacol Sci (2013) 17(10):1360–8.
88. Blaser H, Dostert C, Mak TW, Brenner D. TNF and ROS Crosstalk in Inflammation. Trends Cell Biol (2016) 26(4):249–61. doi: 10.1016/j.tcb.2015.12.002
89. Cichoz-Lach H, Michalak A. Oxidative Stress as a Crucial Factor in Liver Diseases. World J Gastroenterol (2014) 20(25):8082–91. doi: 10.3748/wjg.v20.i25.8082
90. Takaki A, Yamamoto K. Control of Oxidative Stress in Hepatocellular Carcinoma: Helpful or Harmful? World J Hepatol (2015) 7(7):968–79. doi: 10.4254/wjh.v7.i7.968
91. Garcia-Ruiz C, Fernandez-Checa JC. Mitochondrial Oxidative Stress and Antioxidants Balance in Fatty Liver Disease. Hepatol Commun (2018) 2(12):1425–39. doi: 10.1002/hep4.1271
92. Morales-Gonzalez A, Bautista M, Madrigal-Santillan E, Posadas-Mondragon A, Anguiano-Robledo L, Madrigal-Bujaidar E, et al. Nrf2 Modulates Cell Proliferation and Antioxidants Defenses During Liver Regeneration Induced by Partial Hepatectomy. Int J Clin Exp Pathol (2017) 10(7):7801–11.
93. Singal AK, Jampana SC, Weinman SA. Antioxidants as Therapeutic Agents for Liver Disease. Liver Int (2011) 31(10):1432–48. doi: 10.1111/j.1478-3231.2011.02604.x
94. Li S, Tan HY, Wang N, Zhang ZJ, Lao L, Wong CW, et al. The Role of Oxidative Stress and Antioxidants in Liver Diseases. Int J Mol Sci (2015) 16(11):26087–124. doi: 10.3390/ijms161125942
95. Zhang S, Chen L, Liu T, Zhang B, Xiang D, Wang Z, et al. Human Umbilical Cord Matrix Stem Cells Efficiently Rescue Acute Liver Failure Through Paracrine Effects Rather Than Hepatic Differentiation. Tissue Eng Part A (2012) 18(13-14):1352–64. doi: 10.1089/ten.tea.2011.0516
96. Ma Q. Role of Nrf2 in Oxidative Stress and Toxicity. Annu Rev Pharmacol Toxicol (2013) 53:401–26. doi: 10.1146/annurev-pharmtox-011112-140320
97. Wasik U, Milkiewicz M, Kempinska-Podhorodecka A, Milkiewicz P. Protection Against Oxidative Stress Mediated by the Nrf2/Keap1 Axis is Impaired in Primary Biliary Cholangitis. Sci Rep (2017) 7:44769. doi: 10.1038/srep44769
98. Shanahan CM, Crouthamel MH, Kapustin A, Giachelli CM. Arterial Calcification in Chronic Kidney Disease: Key Roles for Calcium and Phosphate. Circ Res (2011) 109(6):697–711. doi: 10.1161/CIRCRESAHA.110.234914
99. Drexler H. Endothelial Dysfunction: Clinical Implications. Prog Cardiovasc Dis (1997) 39(4):287–324. doi: 10.1016/S0033-0620(97)80030-8
100. Ribeiro-Rodrigues TM, Laundos TL, Pereira-Carvalho R, Batista-Almeida D, Pereira R, Coelho-Santos V, et al. Exosomes Secreted by Cardiomyocytes Subjected to Ischaemia Promote Cardiac Angiogenesis. Cardiovasc Res (2017) 113(11):1338–50. doi: 10.1093/cvr/cvx118
101. Zhu H, Jia Z, Misra BR, Zhang L, Cao Z, Yamamoto M, et al. Nuclear Factor E2-Related Factor 2-Dependent Myocardiac Cytoprotection Against Oxidative and Electrophilic Stress. Cardiovasc Toxicol (2008) 8(2):71–85. doi: 10.1007/s12012-008-9016-0
102. Manchester LC, Coto-Montes A, Boga JA, Andersen LP, Zhou Z, Galano A, et al. Melatonin: An Ancient Molecule That Makes Oxygen Metabolically Tolerable. J Pineal Res (2015) 59(4):403–19. doi: 10.1111/jpi.12267
103. Feng C, Yang M, Lan M, Liu C, Zhang Y, Huang B, et al. ROS: Crucial Intermediators in the Pathogenesis of Intervertebral Disc Degeneration. Oxid Med Cell Longev (2017) 2017:5601593. doi: 10.1155/2017/5601593
104. Zhang B, Xu L, Zhuo N, Shen J. Resveratrol Protects Against Mitochondrial Dysfunction Through Autophagy Activation in Human Nucleus Pulposus Cells. Biochem Biophys Res Commun (2017) 493(1):373–81. doi: 10.1016/j.bbrc.2017.09.015
105. Sansone P, Savini C, Kurelac I, Chang Q, Amato LB, Strillacci A, et al. Packaging and Transfer of Mitochondrial DNA via Exosomes Regulate Escape From Dormancy in Hormonal Therapy-Resistant Breast Cancer. Proc Natl Acad Sci U S A (2017) 114(43):E9066–75. doi: 10.1073/pnas.1704862114
106. Phinney DG, Di Giuseppe M, Njah J, Sala E, Shiva S, St Croix CM, et al. Mesenchymal Stem Cells Use Extracellular Vesicles to Outsource Mitophagy and Shuttle microRNAs. Nat Commun (2015) 6:8472. doi: 10.1038/ncomms9472
107. Martel-Pelletier J, Barr AJ, Cicuttini FM, Conaghan PG, Cooper C, Goldring MB, et al. Osteoarthritis. Nat Rev Dis Primers (2016) 2:16072. doi: 10.1038/nrdp.2016.72
108. Loeser RF, Collins JA, Diekman BO. Ageing and the Pathogenesis of Osteoarthritis. Nat Rev Rheumatol (2016) 12(7):412–20. doi: 10.1038/nrrheum.2016.65
109. Ma X, Yang F, Yang S, Rasul A, Li T, Liu L, et al. Number and Distribution of Myofibroblasts and Alpha-Smooth Muscle Actin Expression Levels in Fetal Membranes With and Without Gestational Complications. Mol Med Rep (2015) 12(2):2784–92. doi: 10.3892/mmr.2015.3719
110. Liu X, Li Q, Niu X, Hu B, Chen S, Song W, et al. Exosomes Secreted From Human-Induced Pluripotent Stem Cell-Derived Mesenchymal Stem Cells Prevent Osteonecrosis of the Femoral Head by Promoting Angiogenesis. Int J Biol Sci (2017) 13(2):232–44. doi: 10.7150/ijbs.16951
111. Banton MI, Peachee VL, White KL, Padgett EL. Oral Subchronic Immunotoxicity Study of Ethyl Tertiary Butyl Ether in the Rat. J Immunotoxicol (2011) 8(4):298–304. doi: 10.3109/1547691X.2011.598480
112. Lapointe JM, Valdez RA, Ryan AM, Haley PJ. Evaluation of the Utility of Popliteal Lymph Node Examination in a Cyclophosphamide Model of Immunotoxicity in the Rat. J Immunotoxicol (2016) 13(4):449–52. doi: 10.3109/1547691X.2015.1122117
113. Han YS, Kim SM, Lee JH, Jung SK, Noh H, Lee SH. Melatonin Protects Chronic Kidney Disease Mesenchymal Stem Cells Against Senescence via PrP(C) -Dependent Enhancement of the Mitochondrial Function. J Pineal Res (2019) 66(1):e12535. doi: 10.1111/jpi.12632
114. Lee JH, Han YS, Lee SH. Potentiation of Biological Effects of Mesenchymal Stem Cells in Ischemic Conditions by Melatonin via Upregulation of Cellular Prion Protein Expression. J Pineal Res (2017) 62(2):e12385. doi: 10.1111/jpi.12385
115. Tuglu D, Yuvanc E, Yilmaz E, Gencay IY, Atasoy P, Kisa U, et al. The Antioxidant Effect of Dexmedetomidine on Testicular Ischemia-Reperfusion Injury. Acta Cir Bras (2015) 30(6):414–21. doi: 10.1590/S0102-865020150060000007
116. Turner TT, Tung KS, Tomomasa H, Wilson LW. Acute Testicular Ischemia Results in Germ Cell-Specific Apoptosis in the Rat. Biol Reprod (1997) 57(6):1267–74. doi: 10.1095/biolreprod57.6.1267
117. Yaribeygi H, Sathyapalan T, Atkin SL, Sahebkar A. Molecular Mechanisms Linking Oxidative Stress and Diabetes Mellitus. Oxid Med Cell Longev (2020) 2020:8609213. doi: 10.1155/2020/8609213
118. Fernandez-Sanchez A, Madrigal-Santillan E, Bautista M, Esquivel-Soto J, Morales-Gonzalez A, Esquivel-Chirino C, et al. Inflammation, Oxidative Stress, and Obesity. Int J Mol Sci (2011) 12(5):3117–32. doi: 10.3390/ijms12053117
119. Giacco F, Brownlee M. Oxidative Stress and Diabetic Complications. Circ Res (2010) 107(9):1058–70. doi: 10.1161/CIRCRESAHA.110.223545
120. Lan CC, Wu CS, Huang SM, Wu IH, Chen GS. High-Glucose Environment Enhanced Oxidative Stress and Increased Interleukin-8 Secretion From Keratinocytes: New Insights Into Impaired Diabetic Wound Healing. Diabetes (2013) 62(7):2530–8. doi: 10.2337/db12-1714
121. Moxey PW, Gogalniceanu P, Hinchliffe RJ, Loftus IM, Jones KJ, Thompson MM, et al. Lower Extremity Amputations–a Review of Global Variability in Incidence. Diabetes Med (2011) 28(10):1144–53. doi: 10.1111/j.1464-5491.2011.03279.x
122. Shan B, Wang X, Wu Y, Xu C, Xia Z, Dai J, et al. The Metabolic ER Stress Sensor IRE1alpha Suppresses Alternative Activation of Macrophages and Impairs Energy Expenditure in Obesity. Nat Immunol (2017) 18(5):519–29. doi: 10.1038/ni.3709
123. Ayoub S, Berberi A, Fayyad-Kazan M. Cytokines, Masticatory Muscle Inflammation, and Pain: An Update. J Mol Neurosci (2020) 70(5):790–5. doi: 10.1007/s12031-020-01491-1
124. Cicchese JM, Evans S, Hult C, Joslyn LR, Wessler T, Millar JA, et al. Dynamic Balance of Pro- and Anti-Inflammatory Signals Controls Disease and Limits Pathology. Immunol Rev (2018) 285(1):147–67. doi: 10.1111/imr.12671
125. Opal SM, DePalo VA. Anti-Inflammatory Cytokines. Chest (2000) 117(4):1162–72. doi: 10.1378/chest.117.4.1162
126. Zhang JM, An J. Cytokines, Inflammation, and Pain. Int Anesthesiol Clin (2007) 45(2):27–37. doi: 10.1097/AIA.0b013e318034194e
127. Buzas EI, Gyorgy B, Nagy G, Falus A, Gay S. Emerging Role of Extracellular Vesicles in Inflammatory Diseases. Nat Rev Rheumatol (2014) 10(6):356–64. doi: 10.1038/nrrheum.2014.19
128. Marchetti B, Leggio L, L'Episcopo F, Vivarelli S, Tirolo C, Paterno G, et al. Glia-Derived Extracellular Vesicles in Parkinson's Disease. J Clin Med (2020) 9(6):1941. doi: 10.3390/jcm9061941
129. Leggio L, Paterno G, Vivarelli S, Falzone GG, Giachino C, Marchetti B, et al. Extracellular Vesicles as Novel Diagnostic and Prognostic Biomarkers for Parkinson's Disease. Aging Dis (2021) 12(6):1494–515. doi: 10.14336/AD.2021.0527
130. Elahi FM, Harvey D, Altendahl M, Brathaban N, Fernandes N, Casaletto KB, et al. Elevated Complement Mediator Levels in Endothelial-Derived Plasma Exosomes Implicate Endothelial Innate Inflammation in Diminished Brain Function of Aging Humans. Sci Rep (2021) 11(1):16198. doi: 10.1038/s41598-021-91759-2
131. Murray PJ. Macrophage Polarization. Annu Rev Physiol (2017) 79:541–66. doi: 10.1146/annurev-physiol-022516-034339
132. Zhang Y, Cai Z, Shen Y, Lu Q, Gao W, Zhong X, et al. Hydrogel-Load Exosomes Derived From Dendritic Cells Improve Cardiac Function via Treg Cells and the Polarization of Macrophages Following Myocardial Infarction. J Nanobiotechnology (2021) 19(1):271. doi: 10.1186/s12951-021-01016-x
133. Dou R, Zhang X, Xu X, Wang P, Yan B. Mesenchymal Stem Cell Exosomal tsRNA-21109 Alleviate Systemic Lupus Erythematosus by Inhibiting Macrophage M1 Polarization. Mol Immunol (2021) 139:106–14. doi: 10.1016/j.molimm.2021.08.015
134. Du YM, Zhuansun YX, Chen R, Lin L, Lin Y, Li JG. Mesenchymal Stem Cell Exosomes Promote Immunosuppression of Regulatory T Cells in Asthma. Exp Cell Res (2018) 363(1):114–20. doi: 10.1016/j.yexcr.2017.12.021
135. Si XL, Fang YJ, Li LF, Gu LY, Yin XZ, Jun T, et al. From Inflammasome to Parkinson's Disease: Does the NLRP3 Inflammasome Facilitate Exosome Secretion and Exosomal Alpha-Synuclein Transmission in Parkinson's Disease? Exp Neurol (2021) 336:113525. doi: 10.1016/j.expneurol.2020.113525
136. Ni Z, Kuang L, Chen H, Xie Y, Zhang B, Ouyang J, et al. The Exosome-Like Vesicles From Osteoarthritic Chondrocyte Enhanced Mature IL-1beta Production of Macrophages and Aggravated Synovitis in Osteoarthritis. Cell Death Dis (2019) 10(7):522. doi: 10.1038/s41419-019-1739-2
137. DiStefano TJ, Vaso K, Danias G, Chionuma HN, Weiser JR, Iatridis JC. Extracellular Vesicles as an Emerging Treatment Option for Intervertebral Disc Degeneration: Therapeutic Potential, Translational Pathways, and Regulatory Considerations. Adv Healthc Mater (2021) e2100596. doi: 10.1002/adhm.202100596
138. Adak S, Magdalene D, Deshmukh S, Das D, Jaganathan BG. A Review on Mesenchymal Stem Cells for Treatment of Retinal Diseases. Stem Cell Rev Rep (2021) 17(4):1154–73. doi: 10.1007/s12015-020-10090-x
139. Bao C, He C. The Role and Therapeutic Potential of MSC-Derived Exosomes in Osteoarthritis. Arch Biochem Biophys (2021) 710:109002. doi: 10.1016/j.abb.2021.109002
140. Wilson A, Hodgson-Garms M, Frith JE, Genever P. Multiplicity of Mesenchymal Stromal Cells: Finding the Right Route to Therapy. Front Immunol (2019) 10:1112. doi: 10.3389/fimmu.2019.01112
141. Mosquera J, Garcia I, Liz-Marzan LM. Cellular Uptake of Nanoparticles Versus Small Molecules: A Matter of Size. Acc Chem Res (2018) 51(9):2305–13. doi: 10.1021/acs.accounts.8b00292
142. Yang B, Chen Y, Shi J. Exosome Biochemistry and Advanced Nanotechnology for Next-Generation Theranostic Platforms. Adv Mater (2019) 31(2):e1802896. doi: 10.1002/adma.201802896
Keywords: mesenchymal stem cell, exosome, inflammation, metabolism, oxidative stress
Citation: Xia C, Dai Z, Jin Y and Chen P (2021) Emerging Antioxidant Paradigm of Mesenchymal Stem Cell-Derived Exosome Therapy. Front. Endocrinol. 12:727272. doi: 10.3389/fendo.2021.727272
Received: 18 June 2021; Accepted: 26 October 2021;
Published: 29 November 2021.
Edited by:
Alan Richardson, Keele University, United KingdomReviewed by:
Bruce Alan Bunnell, University of North Texas Health Science Center, United StatesShupeng Li, Peking University, China
Copyright © 2021 Xia, Dai, Jin and Chen. This is an open-access article distributed under the terms of the Creative Commons Attribution License (CC BY). The use, distribution or reproduction in other forums is permitted, provided the original author(s) and the copyright owner(s) are credited and that the original publication in this journal is cited, in accordance with accepted academic practice. No use, distribution or reproduction is permitted which does not comply with these terms.
*Correspondence: Pengfei Chen, cGVuZ2ZlaV9jaGVuQHpqdS5lZHUuY24=
†These authors have contributed equally to this work