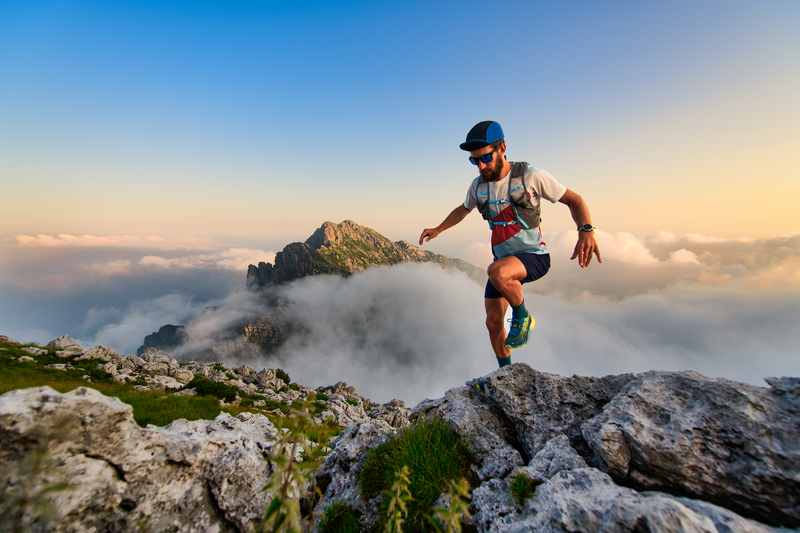
95% of researchers rate our articles as excellent or good
Learn more about the work of our research integrity team to safeguard the quality of each article we publish.
Find out more
REVIEW article
Front. Endocrinol. , 12 August 2021
Sec. Diabetes: Molecular Mechanisms
Volume 12 - 2021 | https://doi.org/10.3389/fendo.2021.720466
This article is part of the Research Topic The Role of Exosomes and Organokines in Metabolic and Endocrine Disease View all 6 articles
Diabetic vascular complications (DVC) including macrovascular and microvascular lesions, have a significant impact on public health, and lead to increased patient mortality. Disordered intercellular cascades play a vital role in diabetic systemic vasculopathy. Exosomes participate in the abnormal signal transduction of local vascular cells and mediate the transmission of metabolic disorder signal molecules in distant organs and cells through the blood circulation. They can store different signaling molecules in the membrane structure and release them into the blood, urine, and tears. In recent years, the carrier value and therapeutic effect of exosomes derived from stem cells have garnered attention. Exosomes are not only a promising biomarker but also a potential target and tool for the treatment of DVC. This review explored changes in the production process of exosomes in the diabetic microenvironment and exosomes’ early warning role in DVC from different systems and their pathological processes. On the basis of these findings, we discussed the future direction of exosomes in the treatment of DVC, and the current limitations of exosomes in DVC research.
Diabetes is a chronic disease that threatens public health, and its incidence is increasing yearly. By 2045, the International Diabetes Federation estimates that there will be 700 million diabetic patients worldwide (1). Cardiovascular complications are the leading cause of death among diabetic patients (2). Due to a lack of early, precise diagnostic markers, many patients with diabetes do not receive early diagnosis and treatment of cardiovascular complications (3). At the same time, many symptomatic treatments for vascular complications are expensive and have unstable therapeutic effects (4, 5). Therefore, it is important to find accurate diagnostic markers for DVC and further improve related pathogenesis research.
Even with effective mitigation of existing cardiovascular risk factors, people with type 2 diabetes have approximately twice the risk of developing cardiovascular disease as those without diabetes (6). Studies indicate that more than 50% of diabetic patients die from vascular diseases, such as coronary artery disease, stroke, and peripheral blood vessel disruptions (7). DVC involves macrovascular and microvascular disease (8). Macrovascular disease mainly involves coronary arteries, of which atherosclerosis is the most common (9). Microangiopathy is a vascular complication unique to diabetic patients that mainly affects the vascular changes in the retina and kidney (10). Previously, researchers believed that hemodynamic changes, glucose metabolism disorders, local hypoxia, and vascular dysfunction caused by inflammation were the main causes of the vascular complications of diabetes (11–13). But evidence demonstrates that the connection between systemic cells and organs is also an indispensable part of diabetic vascular damage (14). Exosomes (a type of small membrane vesicles secreted by cells that deliver nucleic acids and proteins) participate in the occurrence of many systemic diseases (15, 16) by regulating inflammation and metabolism (17). They can also mediate the direct communication between vascular endothelial cells and other organs and cells with the help of blood circulation. In addition to the above effects, the latest view is that exosomes are a medium released by stem cells for systemic therapy (18). Exosomes released by various types of stem cells have been found to alleviate the progression of the disease of diabetes (19). Exosomes are considered potential therapeutic targets and valuable biomarkers for the treatment of DVC (20).
This article focuses on the possibility of exosomes as biomarkers in various DVC in human and animal models. We introduce the special pathological changes of exosomes in diabetic microenvironments and their involvement in vascular complications and summarize the value of exosomes in stem cell therapy for DVC.
Cell-to-cell and organ-to-organ communication are essential during development, normal physiology, or in pathological conditions. Vesicles secreted by cells under passive or active conditions are imperative to signal transduction pathways. The International Society for Extracellular Vesicles (ISEV) guidelines propose extracellular vesicles (EV) as a general term for particles that are naturally released from cells. These particles are defined by a lipid bilayer and cannot be replicated, that is, they do not contain a functional core. The subtypes of EV include endosome-derived exosomes and plasma membrane-derived ectosomes (microparticles/microvesicles) (21).
Exosome formation begins with the formation of early endosomes when cell membranes recess inward and then continue to mature and form late endosomes. Late endosomes wrap specifically sorted nucleic acids, proteins, and other substances in the cytoplasm to form multiple intraluminal vesicles (ILVS) through inward budding. Multivesicular bodies (MVB) are composed of multiple ILVs in late endocytosis (22–24). MVBs mature process involves a specific sorting machine. Endosomal sorting complex required for transport (ESCRT) is an important driver of MVB membrane formation and rupture (22, 23). The ESCRT system is mainly composed of a family of protein complexes: ESCRT -0, - I, - II, and - III, coupled with the accessory proteins tumor susceptibility gene 101 protein and vacuolar protein sorting-associated protein 4 (25, 26). ESCRT-0 subunits are involved in cargo clustering and the ubiquitination of membrane proteins. ESCRT-I/II/III contribute to plasma membrane budding and exosomes releasing events (27, 28). Cells can also produce ILVs and MVB through lipids, ceramides, or tetrapeptides independent of ESCRT (29–32). The mature MVBs may fuse with lysosomes and degrade due to their ubiquitinated cargo, or they may go toward the plasma membrane and release their contents in the extracellular space with the help of the Rab family and attachment protein receptor (SNARE) complex (33).
The exosomes’ primary physiological role is to regulate intercellular communication by transmitting their special cargo, including proteins, lipids, and nucleic acids, in the following three ways. First, exosome membrane proteins can bind to the receptor on the target cell membrane to activate the signal pathways in the target cell. Exosome membrane proteins can be cleaved by some proteases, and the cleaved fragments can bind to cell membrane receptors, thus activating intracellular signaling pathways (34). Third, exosomes directly fuse with the target cell membrane and nonselectively release their contents to regulate target cell signaling (32, 35) (Figure 1).
Figure 1 Schematic diagram of exosomes production and reception. Exosomes are originated from the MVBs, referring to double invagination of the plasma membrane. Early sorting endosomes (ESEs) are formed by plasma membrane invagination, which can be fused with the endoplasmic reticulum (ER) and trans-Golgi network (TGN) to produce late sorting endosomes (LSEs). The second invagination in LSE leads to the formation of intraluminal vesicles (ILV). MVBs are formed from LSEs with several ILVs under two mechanisms, ESCRT-dependent and ESCRT-independent pathway. Some MVBs can be degraded by fusion with autophagy or lysosome, while others can be transported to the plasma membrane and released to the extracellular environment. Exosomes are composed of various proteins, lipids, DNA, and RNA. The released exosomes are mainly uptaken by recipient cells through three pathways, including endocytosis, direct fusion, and receptor-ligand interaction.
There is accumulating evidence that exosome levels are elevated in the blood of diabetic individuals and are involved in diabetes-related pathophysiology, including vascular complications, inflammation, and coagulation changes (36–38). In a cross-sectional study of normoglycemic participants and patients with prediabetes or diabetes mellitus, plasma extracellular vesicles in diabetic patients were higher than those in euglycemic control subjects. Exosome concentration was positively correlated with insulin resistance index (HOMA-IR). In vitro, insulin signaling was reduced, and EVs’ secretion increased after the primary neurons long-term exposure to insulin (36). New research has found that autophagy has the same molecular mechanism as exosomes (39, 40). It has been reported that ATG5-ATG12 and ATG16L1 complexes and LC3 have been detected in endosomes and phagosomes (41–43). The function of these autophagy-related proteins is to ensure that the vesicles are acidified and degraded in the lysosome. Using bafilomycin A1 to inhibit the autophagy pathway can prevent the fusion of endosomes and lysosomes, thereby facilitating the release of exosomes (44). Autophagy pathway of multiple cells is inhibited in the diabetic microenvironment, which may increase exosomes in diabetic patients (45–47).
The abnormal exosomes of stem cells are also an essential factor in DVC. Mesenchymal stem cells (MSCs) can be recruited around blood vessels under normal and pathological conditions. They secrete many factors through the exosome pathway to promote the formation of new blood vessels. Therefore, they are considered an essential part of regulating vascular regeneration (48, 49). In aging or diabetic conditions, the production process of MSC exosomes presents significant changes (50). The Jafar Rezaie team exposed human mesenchymal stem cells to diabetic serum for 7 days. It was observed that the levels of exosomal-related genes CD63, Alix, Rab27a, Rab27b and Rab8b increased significantly. In diabetic patients, the activity of acetylcholinesterase is enhanced, the exocrine volume increases and the zeta potential decreases. Ultrastructural examination revealed that more cytosolic lipid vacuoles accumulated in diabetic cells. The reduction in the zeta potential of exosomes is mainly related to the glycosylation of exosomes, such as lactosamine, mannose and N-linked glycans. It indicates that the abnormal glucose and lipid metabolism of stem cells in the diabetic microenvironment may mediate the modification and content alteration of exosomes (51). More importantly, Jafar Rezaie found that the angiogenesis and recovery ability of human bone marrow mesenchymal stem cells decreased after treated with serum from patients with type 2 diabetes. The survival rate of human bone marrow mesenchymal stem cells and their chemotaxis to vascular endothelial growth factor decreased after 7 days of serum culture in patients with type 2 diabetes. After diabetic serum treatment, the expression of cadherin and NG2 in vascular endothelial cells and the uptake capacity of low-density lipoprotein decreased. The cell migration rate and the activity of matrix metalloproteinase-2 and -9 decreased (52). Another interesting study found that metformin, a common drug used to treat diabetes, increased the production of human glioblastoma cell (U87 MG) exosomes. Metformin reduces the activity of U87 MG human glioblastoma cells and inhibits the expression of cancer-promoting genes related to angiogenesis, carcinogenesis and chemoresistance molecules (53). However, previous reports found that the expression of CD63, Alix, and Rab27A decreased in human endothelial progenitor cells (EPCs) cultured in diabetic serum (54). This suggests that the effect of the diabetic microenvironment on exosome production of different types of stem cells may differ.
Evidence shows that inflammatory system disorders in diabetes induce and accelerate various vascular diseases (55–57). The researchers observed that EVs from diabetic patients were preferentially internalized by circulating monocytes. Further studies have shown that co-incubation of diabetic EVs with monocytes could reduce the expression of genes related to apoptosis and oxidative stress in monocytes (36, 58). It also increased the expression of many proinflammatory cytokines (58–60). These findings suggest that EVs in diabetic patients promote monocyte survival and promote monocyte inflammatory activation. Therefore, an abnormal exosome internalization and activation mechanism may be a significant target in the study of diabetic inflammation activation.
Atherosclerosis is a disease in which abnormal metabolism or blood coagulation causes thickening and hardening of the arterial walls, resulting in narrowing and obstruction of the vascular lumen (61, 62). Diabetes plays a crucial role in accelerating atherosclerosis, with an augmented inflammatory state, more diffuse atherosclerosis, and larger necrotic core areas in the lesion (63). Endothelial and smooth muscle cell dysfunction, attributing to hyperglycemia and insulin resistance, are the major characteristics of diabetic vasculopathy, favoring a pro-inflammatory/thrombotic state that results in atherothrombosis. In the early stage, lipids or glycoproteins are deposited on the arterial intima, and the damaged endothelium recruits blood-circulating mononuclear cells. These differentiate into macrophages that engulf lipids and transform into foam cells. Subsequently, activated endothelium and macrophages release several chemokines and growth factors, leading to smooth muscle proliferation and extracellular matrix protein synthesis. With the accumulation and denaturation of lipids, fibrous cap tissue necrosis, progressive structural remodeling, and inflammatory cell infiltration, the atheromatous plaques begin to form (62, 64). In recent years, numerous investigations suggest that the exosomes in the diabetic microenvironment promote the progression of atherosclerosis via endothelial function, inflammatory pathways, and lipid metabolism (65–67).
Endothelial dysfunction is the basic mechanism of early atherosclerosis in diabetes mellitus. An analysis of patients with atherosclerosis showed that cargo proteins levels in plasma endothelial cell-derived exosomes (EDEs), such as VCAM-1, vWF, PDGF-BB, angiopoietin-1, were significantly higher relative to those of matched control subjects. This is related to the different functions of endothelial cells, such as adhesiveness, antithrombosis, survival and proliferation, transport, metabolism, and the vascular collagen structure (68). Moreover, reduced nitric oxide (NO) bioavailability enables hyperglycemia to impair vascular endothelial function (69). Furchgott and Lgnarro proposed that NO can be used as an endothelial vasodilator to reflect the vascular endothelium function (70, 71). In the early stage of atherosclerosis, NO secretion decreases after vascular endothelial injury, which leads to the increased expression of adhesion molecules, the adhesion of macrophages, LDL oxidation, and smooth muscle proliferation to accelerate atherosclerosis progression (72, 73). Huina Zhang et al. transferred exosomes from the blood of db/db diabetic mice into db/m+ non-diabetic mice and found that exosomes can be delivered to the aortic endothelial cells of non-diabetic db/m+ mice and damage endothelial cell function. Further analysis on exosomal proteins in the endothelial cells of diabetic mouse aortas found that exosome protein signals played a major role in this process. Proteomics analysis showed that arginase 1 in the exosomes of the db/db group was significantly increased (74). The main substrates for NO synthesis are L-arginine and hydroxyl L-arginine. Arginase 1 can specifically degrade NO substrates and reduce NO production in endothelial cells (75). This study demonstrated that lesions in the aortic endothelium could affect other normal cell parts via certain regulatory proteins carried by exosomes.
A disordered local microenvironment is an important condition for plaque formation. Exosomes from mature dendritic cells (DCs) are involved in increasing endothelial inflammation and atherosclerosis via the NF-κB pathway mediated by tumor necrosis factor-α (TNF-α) located in the exosome membrane (76). Naive and M2-polarized macrophage-derived exosomes, which carry and transfer miR-146b-5p, miR-378-3p, and miR-99a-5p, have a protective role in the suppression of the inflammatory response via NF-κB and TNF-α signaling. They also reduce necrotic lesion areas in the atheroma (77).
Additionally, recent studies have revealed that inflammasome activation is one of the pathologic mechanisms of diabetic vascular endothelial dysfunction (78–80). NLRP3 inflammasome is activated in the coronary endothelial cells of early diabetic mice (81). The NLRP3 inflammasome is a multimeric protein complex that mediates caspase-1 activation and the secretion of pro-inflammatory cytokines IL-1β/IL-18 in response to a stimulation (82). Inflammatory body products are not secreted through specific Golgi-mediated direction and transportation, but they are segregated by the membrane and secreted in extracellular vesicles. Exosomes mediate the release of inflammatory body products into the extracellular space (82, 83). In a diabetic mouse model induced by streptozotocin (STZ), endothelial-specific lysosomal acid ceramidase (AC) knockout mice (Asah1fl/fl/ECcre) significantly elevated the formation of NLRP3 inflammasomes and activation in coronary artery ECs (CECs). A metabolic enzyme of ceramide, AC is involved in the transportation of MVBs to lysosomes, thereby regulating the fate of MVBs. In in vitro experiments, AC knockout CECS can reduce the interaction between MVB and lysosomes, promote exosome production, increase the release of IL-1β exosomes under high glucose stimulation, and promote an inflammatory reaction, which leads to vascular inflammation and atherosclerosis (84). Therefore, regulating inflammatory responses mediated by exosomes may be an effective way to treat diabetic atherosclerosis.
Improving the plaque stability of advanced plaque and reducing the core of necrosis are the main goals of the current treatment of atherosclerosis. Studies have shown that atherosclerotic plaques in subjects with type 2 diabetes are more likely to rupture. As a hedgehog (hh) signaling molecule, sonic hedgehog (shh) plays a critical role in normal embryonic development and the maintenance of adult vasculature and neovascularization (85). Hh signaling contributes to the progression of atherosclerosis, and its inhibition resulted in a reduction in the plasma cholesterol content. These effects were related to a reduced uptake of modified lipoproteins mediated by scavenger receptors on plaque macrophages (86). Adipocytes are an important endocrine cell involved in controlling appetite and satiety, regulating energy metabolism, and effecting insulin sensitivity (87). Adipocyte dysfunction regulates multiple diabetic complications via insulin resistance and tissue inflammation (88). Insulin resistance adipocyte-derived exosomes contribute to vasa vasorum angiogenesis via the shh signaling pathway in endothelial cells ex vivo, and further promote plaque burden and plaque vulnerability in diabetic ApoE-/- mice (66). Generally, exosomes play an important role in regulating endothelial injury, inflammatory activation, and plaque instability during atherosclerosis development.
DR is a common complication of diabetes. Pathological angiogenesis and leakage of the blood-retinal barrier are characteristic changes in diabetic patients (89). Diabetic retinopathy is usually asymptomatic in its early stages. As the disease progresses, patients experience decreased vision due to macular edema, vitreous hemorrhage, and pathological neovascularization. In severe patients, fibrous tissue proliferation in the vascular scar can cause detachment by pulling the retina (90). Current studies on the molecular mechanisms of DR have revealed the critical role of neurovascular crosstalk disorders in retinal injury (91, 92). As an important carrier of cell crosstalk, the role of exosomes in DR is gradually being recognized.
Müller cells are the most abundant glial cells in the retina, and they play an irreplaceable role in maintaining the blood-retinal barrier and the function of neurons (93). Müller cells have a dual role in DR. On the one hand, high glucose can damage the normal transport function of Müller cells and affect retinal homeostasis (94). On the other hand, the angiogenic factors released by Müller cells and the excessive proliferation of Müller cells are considered to be the central effector cells of the proliferative diabetic retina (95). It is well known that the pathogenesis and progression of diabetes are related to the dysfunction of pancreatic beta cells (96). Tengku Ain Kamalden et al. found that the level of miR-15a in the plasma of diabetic patients is positively correlated with the severity of retinopathy. miR-15a is a crucial molecule that regulates insulin secretion by pancreatic β cells. The exosomes released by pancreatic β cells carry miR-15a into the retina and activate Müller cell apoptosis through the Akt3 pathway (97). In contrast, Zhang Wei’s team found that exosomes isolated from the plasma of diabetic rats can promote the migration and proliferation of Müller cells. In further mechanism studies, it was confirmed that diabetic plasma exosomes enhanced fibronectin and connective tissue growth factor (CTGF) expression in Müller cells, activated Yes-related proteins and jointly promoted the proliferation and fibrosis of Müller cells. These results suggest that exosomes regulate the functions of Müller cells through very complex and delicate pathways. To clarify the specific pathway requires more careful sorting and identification of exosomes.
The retinal pigment epithelium (RPE) is located between the choroid and the retina, forming an external blood-retinal barrier. RPE regulates the translation of epithelial cells and the stability of tight junctions. The regulation of retinal pigment epithelium in diabetic retinopathy is a direction that cannot be ignored (98). Researchers exposed retinal pigment epithelial cell line-19 (ARPE-19) to a high-glucose (HG) medium and detected many exosomes carrying VEGF and ROS. Melanin receptor 5 (MCR5) agonist treatment can significantly reduce the production of ROS and VEGF-carrying exosomes in the retinal pigment epithelium and reduce the angiogenesis caused by ARPE19 induced by high glucose (99). Nevertheless, in another study, Shun Gu et al. found that the release of miR-202-5p-containing exosomes from ARPE19 cells treated with HG can effectively prevent proliferative diabetic retinopathy. The ingestion of these exosomes inhibited the growth, migration and tube formation of human umbilical vein endothelial cells. The miR-202-5p in exosomes can also inhibit endothelial-mesenchymal transition through the TGF/Smad pathway (100).
Vascular inflammation and activation of the complement system play an important role in vascular barrier leakage (101, 102). Complement can be activated by a classical pathway, selective pathway, and lectin pathway. All complement pathways can activate the production of C3/C5 invertase and form a membrane attack complex (MAC) (103). Previous studies in DR patients and diabetic rat models have found that MAC is extensively deposited in retinal endothelial cells, leading to retinal endothelial cell death and increased retinal vessel leakage (104, 105). Huang C et al. found that the content of exosomes in the plasma of diabetic patients increased significantly. Exosomes carry IgG bind Clq to activate the classical complement pathway to form MAC attack retinal blood vessels. This study makes up for the limitations of previous studies on exosomes in DR (106).
The incidence of Diabetic kidney disease (DKD) is increasing globally, and 20-40% of diabetic patients suffer from DKD. DKD is a major cause of chronic kidney disease and end-stage kidney disease (107, 108). During the 10-20 years from hyperglycemia to renal failure, a variety of mechanisms are involved in damage to the vascular system and the glomerulus (109). Disorders of glucose and lipid metabolism, podocyte injury, basement membrane thickening, mesangial dilatation, and glomerulosclerosis are the characteristic manifestations of DKD (110, 111). The American Diabetes Association defines DKD as a progressive kidney disease related to diabetes with a glomerular filtration rate < 60 or with proteinuria (112).
Early identification and intervention can reduce and delay the progression of diabetic nephropathy into end-stage renal disease (113). There was no obvious clinical manifestation during an early-stage renal injury in diabetic patients (114). Although proteinuria is the gold standard for a renal disease diagnosis, it is affected by many factors (115). Most patients have irreversible renal damage when they have apparent clinical manifestations (116), and renal replacement therapy may be the only option once the disease progresses to end-stage renal disease (117). Accurate, early biomarkers of DKD are important.
Urine is a noninvasive body fluid that reflects kidney function (118). In previous studies, many markers (including proteins and RNA) reflecting renal function and DKD progression have been found in the urine (119). However, recent studies have found that the detection of urine exosome-related molecules is more accurately reflective of the progress of DKD (120). Exosomes are also carriers of many proteins and microRNAs in the urine (121). In the ultra-early stages of DKD, circulating exosomes cannot pass through the glomerulus to enter the urine, and the exosomes in urine are mainly derived from the kidney’s constituent cells (120). Therefore, exosome content in the urine is not easily disturbed by some peak proteins and can better mirror the progression of diabetic nephropathy.
MiRNA is a small RNA with a length of about 20-24 nucleotides, which can bind to mRNA and regulate the expression of multiple target genes. It plays a vital role in immune regulation, insulin secretion, cell differentiation, and other physiological and pathological processes (122, 123). Exosomal nucleic acids exist in a remarkably stable form due to the protective effects of exosomes from RNase activity. Federica-Barutta used two-step differential centrifugation to provide the first evidence that urine exosomes contain a large amount of MiRNAs. Subsequently, many studies have confirmed that urinary microRNAs are related to the degree of lesions in DKD, which is valuable in early diagnosis and therapeutic targeting.
Researchers first examined the reported microRNAs associated with DKD in urine exosomes. MiR-215, miR-192, and miR-194 were enriched in the kidney and played a role in DKD pathogenesis (124). Yijie Jia et al. classified diabetic patients into three groups of no albuminuria, microalbuminuria, and massive albuminuria according to different albuminuria levels. They found that the levels of miR-192 in urinary exosomes was higher than the levels of miR-194 and miR-215, but miR-192 levels in the massive albuminuria group decreased. In patients with normal albuminuria and microalbuminuria, miR-192 was positively correlated with proteinuria and transforming growth factor (TGF-β). Receiver operating characteristic curve analysis shows that miR-192 is superior to miR-215 and miR-194 in distinguishing between the normal albuminuria and microalbuminuria group. MiR-192 has more predictive value in early renal function impairment in diabetic patients (125).
Yijun Xie et al. tested 5 Type 2 diabetes (T2D) patients without kidney disease and 5 T2D patients with massive proteinuria. A total of 496 types of UExo-derived miRNAs were differentially expressed in DKD patients (>2 times). After PCR verification, three up-regulated miRNAs (miR-877-3p, miR-362-3p and miR-150-5p) were found. These miRNAs are involved in the regulation of AMPK, p53 and mTOR pathways in DKD (126). Barutta F et al. evaluated miRNA expression in urine exosomes of patients with diabetes and early kidney disease. The results showed that miR-145 and miR-130a were enriched in urine exosomes of DKD patients, while miR-424 and miR-155 were reduced. In animal models of diabetic nephropathy, the levels of miR-145 in glomeruli and urine exosomes are elevated, and a high glucose environment can increase the content of miR-145 in mesangial exosomes (127).
In recent years, researchers have used bioinformatics technology to screen out further many miRNAs related to DKD. Eissa S et al. initially screened the differential expression and pathway enrichment analysis of miRNA in urine exosomes based on the syber-green PCR array. Among them, miR-15b, miR-636 and miR-34a are the three most significantly up-regulated miRNAs in DKD. Validation of qRT-PCR in larger independent subjects showed that miR-15b, miR-636 and miR-34a were all up-regulated in urine exosomes of DKD patients. It is worth noting that there is a positive correlation between these miRNAs and the ratio of serum creatinine and urine protein creatinine. The sensitivity of these urinary exosomal miRNAs detection to the diagnosis of DKD is 100% (128).
In another subsequent study, the Eissa S team used public miRNA databases to apply a combined target prediction algorithm. The PCR detection of differential expression in urine exosomes of 210 clinical participants showed that the expression levels of miR-133b, miR-30a and miR-342were higher than normal levels. A more interesting result is that there are still 39.3% miR-133b, 19.6% miR-342 and 17.9% positive rates in patients with normal albuminuria. This research indicates that these miRNAs may have changed before the patient developed albuminuria (129).
There are many other factors besides diabetes that can lead to chronic renal insufficiency. To further explore more accurate markers of DKD, Jinnan Zang et al. established a cohort study comparing urinary exosomes MiRs in patients with T2DKD and T2DM (normal renal function), as well as chronic kidney disease (CCKD). They found that let-7e-5p, miR-23b-3p and miR-21-5p were higher in T2DKD patients than CCKD patients, while the expression of miR-30b-5p and miR-125b-5p was reduced. Compared with T2DM patients in independent validation experiments, miR-21-5p was up-regulated in the T2DKD and CCKD cohorts. MiR-30b-5p has decreased expression in T2DKD and CCKD (130). Interstitial transformation of epithelial cells is a key step in renal fibrosis. MiR-21-5p in the renal cortex of diabetic mice increased inhibitory phosphatase, phosphatase and tensin homolog deleted (PTEN) and fibronectin levels (131). Overexpression of miR-21-5p promoted TGF-β-induced epithelial-mesenchymal transition (132). miR-30b-5p is abundantly expressed in prostate cancer patients, and its expression level is lower in T2DKD patients. The miR-30 family have a protective effect in kidney cells. The reduction of miR-30 family expression is also associated with renal fibrosis (133). Although the changes of miR-30b-5p and miR-21-5p are not unique to T2DKD, the degree of imbalance of miR-30b-5p and miR-21-5p in T2DKD is more obvious than that of CCKD. The definition of DKD patients is not distinguished from CCD by pathological testing methods. The different course of DKD patients is also the reason for the difficulty in finding biomarkers. How to group patients more accurately may be a breakthrough point for in-depth research in the future.
In addition to carrying miRNAs, exosomes also contain various proteins that participate in multiple signal pathways of DKD. Irene Zubiri et al. detected 352 proteins in human urinary exosomes using proteomic techniques for the first time. Quantitative analysis revealed significant changes in 25 proteins in DKD. Further screening and analysis confirmed that there were differences in the expression of histone-lysine N-methyltransferase (MLL), α-microglobulin/bikunin precursor (AMBP), and voltage-dependent anion-selective channel protein 1 (VDAC1) (134). MLL3 is a histone methyltransferase firstly identified in exosomes (135) that is associated with proliferator-activated receptorγ (PPARγ) downstream activation. PPARγ agonists prevent a variety of kidney diseases, including DKD, through systemic and renal action (136). AMBP is a membrane glycoprotein expressed in the liver and kidneys that inhibits serine protease activity (137). Previous studies have found that compared with healthy people, patients with type 2 diabetes have lower levels of AMBP in their urine (138). The decreased synthesis of AMBP in the liver and kidneys of diabetic patients or the obstruction of exosome inclusion into AMBP may explain the decrease of urinary exosomes. VDAC 1 has been reported in exosomes secreted by both urine and B cells (139). In this study, VDAC1 levels were reduced in urine foreign bodies isolated from DKD samples (140). VDAC1 has the activity of NADH-ferricyanide reductase. In previous studies, the up-regulation of VDAC1 in the kidneys of diabetic rats was related to podocyte apoptosiss (141). The decrease in the presence of exosome VDAC1 may be related to apoptosis regulation by exosomal secretion. Due to the limitations of previous studies’ techniques and methodology, it was difficult to detect many low-abundance proteins. After removing high-abundance proteins, protein techniques can help locate potential biomarkers and therapeutic targets for DKD. This study shows us a better screening process of exosome protein markers.
Gelatinase and ceruloplasmin are two types of proteins that have been identified in urine and are closely related to the development of DKD (120, 142, 143). There is ample evidence in laboratory animals and humans that gelatinase is reduced in early and late DKD kidney tissues. The symptoms of diabetic nephropathy in mice were significantly aggravated after the deletion of gelatinase (144). Ceruloplasmin, which promotes inflammation in response to hyperglycemia and advanced glycation end products, is activated in renal tissues of patients with confirmed DKD (145). Krishnamurthy P. Gudehithlu and his team compared changes in gelatinase and ceruloplasmin levels in the urine and exosomes from DKD patients and healthy subjects. They found that the changes to gelatinase and ceruloplasmin in urinary exosomes of DKD patients were consistent with the differences in renal tissue. In contrast, the activity of these enzymes in whole urine samples from patients with DKD differed from that in renal tissues. These studies suggest that protein markers found in urinary exosomes may better reflect the kidney’s underlying changes than proteins measured in whole urine samples (120).
Aquaporins (AQPs) expressed on the plasma membrane of renal tubular epithelial cells are often dysregulated in diabetic nephropathy (146, 147). Linear regression analysis of the histological diagnosis and exosomal excretion of AQP5 and AQP2 in the urine (UAQP5 and UAQP2) of patients with non-diabetic proteinuric nephropathy (NDN) and diabetic nephropathy showed that UAQP5 and UAQP2 were positively correlated with the histological type of diabetic nephropathy (148). AQPS is a complete membrane protein. The excretion of AQP2 and other top plasma membrane proteins through exosome formation was demonstrated by immunoelectron microscopy and nano atomization liquid chromatography-tandem mass spectrometry (149). It has been suggested that exosomes can mediate the transport and secretion of cell membrane proteins in the diseased kidney.
The activation of the TGF-β/Smad is recognized as a classic signal for pathological changes of DKD (150). In recent years, various evidence has confirmed that exosomes of diabetic patients can affect many aspects of the TGF-β/Smad cascade signal. In the HG environment, the secretion of exosomes by macrophages was significantly increased. Exosomes carry TGF-β mRNA into mesangial cells, mediating the proliferation and activation of mesangial cells through the TGF-β/Smad pathway and promoting renal fibrosis (151). TGF-β superfamily receptors are classified into type I and type II receptors. TGF-β can phosphorylate Smad3 protein when it binds to type II receptor on the cell surface, regulating cell proliferation-related genes (152). The up-regulation of RII transcription is one of the mechanisms leading to the TGF-β signal activation. Akiko Sakurai et al. found that podocytes release exosomes containing the epithelial cell-specific transcription factor3 (ELF3) transcription factor in high glucose culture, promoting TGF-β/Smad signaling by up-regulating RII transcription. There was a linear correlation between the ELF3 content in exosomes and the decrease of glomerular filtration rate (153).
Podocytes are located on the surface of glomerular capillaries. They are highly differentiated epithelial cells that work with endothelium to maintain normal glomerular filtration function (154). Podocyte damage is a hallmark pathological manifestation of early DKD. Podocyte injury can lead to a variety of cellular and structural changes in the glomerulus (155). In vitro studies, exosomes released by macrophages cultured with high glucose disrupted podocyte function by inducing apoptosis and TGF-β pathway and reduced the expression of protective proteins such as nephrin, podocin and Wilms tumor protein (WT1) (156). WT1, as a podocyte-derived signal transduction factor family member, is closely related to the development and function of the urinary system (157). Clinical trials have found that the content of WT1 in urinary exosomes is related to multiple indicators that reflect the decline of kidney function (serum creatinine, albumin/creatinine ratio, urine protein/creatinine ratio and eGFR) (158). WT1 in urine exosomes is considered to be a marker of podocyte damage in DKD. These results indicate that exosomes can participate in TGF-β/Smad signal transduction in multiple pathways.
At present, the treatment of various diabetic complications aims to control the metabolic and hemodynamic changes related to this condition and slow disease progression. The treatment methods for vascular injury are very limited (159). Stem cells can self-renew and differentiate into multiple lineages to produce specific cell types (160). They are considered a potential treatment for DVC due to their ability to reconstruct damaged, lost, and aged tissues (161–163). Moreover, in recent years, research indicates that that exosomes secreted by stem cells may be an effective weapon for treating diabetic vascular diseases.
A Safwat et al. injected rabbit adipose-derived mesenchymal stem cell (ADSC) exosomes into mice eyes 4, 8, and 12 weeks after the diabetic model was established by systemic, subconjunctival, and intraocular injection. They found that ADSC-derived exosomes can effectively alleviate retinal edema, the cell structure destruction in each layer in the STZ group. MiR-222 in exosomes can reduce abnormal angiogenesis by regulating STAT5a (164).
Kanna Nagaishi et al. found that after intravenous transplantation of bone marrow mesenchymal stem cells, minimal donor mesenchymal stem cells were observed in the kidneys (165). Further studies have found that bone marrow MSCs can inhibit ICAM-1 and TNF-α through paracrine exosomes and reduce the excessive infiltration of macrophages (166). At the same time, MSC-derived exosomes inhibited the mesenchymal transition and fibrosis of the renal tubular epithelial cell phenotype by reducing the expression of TGF-β (167, 168).
Autophagy has a protective effect on hyperglycemia-induced renal injury (169). Rapamycin (mTOR), the core component of cell growth signals that enhances protein translation, can inhibit autophagy when its activity is enhanced. Increased mTORC1 activity has been observed in human and animal diabetic nephropathy (170–172). Nesrine Ebrahim et al. found that bone marrow mesenchymal stem cells-derived exosomes can enhance autophagy by inhibiting the mTOR signaling pathway in the diabetic nephropathy model. Additionally, the team also found that MSC-derived exosomes significantly increased the expression of autophagy-related proteins, Beclin-1 and LC3. The histological morphology of the kidney of mice treated with MSC-derived exosomes was restored, and fibrosis markers in the kidney tissue was reduced (173).
The occurrence and development of DKD are related to podocyte injury (174). VEGF produced by podocytes is unfavorable for the treatment of DKD (175). MiR-16-5p can inhibit the expression of VEGF. Hyperglycemia reduces the production of miR-16-5p by podocytes and promotes the release of VEGF. After over-expressing MiR-16-5p in human embryonic stem cells, MiR-16-5p can be transferred to podocytes treated with high glucose through the exosomal pathway, reducing the degree of podocyte apoptosis and the expression of VEGF (176).
Approximately 15% of diabetic patients worldwide experience wound healing difficulties and diabetic foot ulcers (DFU), of which 5-24% require amputation (177). The slow reconstruction of blood vessels and inflammation make wound healing difficult in diabetic patients (178, 179). Exosomes also play an essential role in stem cell transplantation for diabetic wound angiogenesis (180). Cell-free treatment of exosomes is a highly stable, non-immune therapy that provides easy access to the lesion and has clinical value (181, 182). Evidence describes the therapeutic mechanism of MSCs-exos in diabetic wound healing. Arsalan Shabbir et al. found that MSCs-exos can be internalized by fibroblasts from normal donors and chronic wound patients and can enhance the proliferation and migration of fibroblasts in a dose-dependent manner. The uptake of MSC-exos by human umbilical vein endothelial cells also leads to increased endothelial cell lumen formation. The MSC-exos treatment activates Akt, ERK and STAT3 signaling pathways and induces the production of various growth factors (183). In another experiment, it was also confirmed that MSCs-exosomes extracted from blood accelerate wound healing in diabetic mice. MSCs-exosomes can induce macrophage polarization, enhanced angiogenesis and collagen deposition by affecting the NF-ĸB signaling pathway and up-regulation of VEGF. The expression of PTEN protein in the MSC-derived exosomes pretreated with melatonin was up-regulated, and the M2 polarization of macrophages was induced by inhibiting the phosphorylation of AKT to promote wound repair (184).
EPCs are the progenitor cells of endothelial cells, which play a crucial part in angiogenesis and diabetic wound repair (185). J Zhang et al. injected human umbilical cord blood EPC exosomes into diabetic rats and found that EPC-Exos can be integrated into endothelial cells and enhance endothelial cell proliferation, migration and blood vessel formation by activating the Erk1/2 signaling pathway (186). Chun-Yuan Chen et al. collected exosomes secreted by human urine-derived stem cells. USC-Exos protein profile screening showed that the highly expressed pro-angiogenic protein 1 (DMBT1) enhanced the angiogenic activity of endothelial cells and promoted wound healing in STZ mice (187).
Nrf2 has a protective effect on oxidative stress. Patients with Nrf2 gene mutation are more likely to have diabetic complications, including peripheral neuropathy, nephropathy, retinopathy, foot ulcer, and microvascular disease (188). Xue Li et al. used the exosomes of Nrf2 overexpressing ADSCs to treat diabetic rats and found that the foot wound ulcer area was significantly reduced. Increased levels of granulation tissue formation, angiogenesis and growth factors, as well as decreased levels of inflammatory and oxidative stress-related proteins were detected in the wound bed. This experiment shows that the target gene-modified stem cells can make their exosomes play a more effective therapeutic role (189).
As platelet-rich plasma (PRP) contains many growth factors that promote tissue regeneration and wound healing (including new blood vessel formation), PRP has been widely used to treat chronic wounds (190). Exosomes encapsulate many platelet growth factors. VEGF and bFGF carried by PRP-exos have pro-angiogenic effects in normal ECs via PI3K/Akt signaling (191). Wound healing begins with the proliferation of fibroblasts (192). PDGFBB promotes the proliferation of fibroblasts through the extracellular signal-regulated kinase (Erk) pathway (193). Additionally, the expression of bFGF, PDGF-BB, and TGF-β in PRP-exons was significantly enriched compared with the PRP supernatant. After PRP-exos treatment, the downstream target protein YAP of Rho GTPase (RhoA) is dephosphorylated, allowing it to transfer to the nucleus, increasing fibroblasts’ migration and promoting faster wound healing (194).
In addition to the exosomes released from stem cells, macrophages-derived exosomes also have a promising effect in mediating the pro-angiogenic effects in diabetic wounds via inhibiting the inflammatory response. Liu W et al. found that macrophages-derived exosomes can activate P-AKT and reduce MMP-9 levels, significantly reduce pro-inflammatory cytokines secretion, and promote the proliferation and migration of endothelial cells to improve wound healing in diabetes mellitus. Furthermore, it reduced TNF-α, IL-1β, and iNOS expression and increased the expression of IL-10 and Arg-1 (59).
After stem cell transplantation, it is unnecessary to reach the lesions for cell replacement to treat diabetic vascular damage. Exosomes may help stem cells slow down pathological changes in blood vessels throughout the body via blood circulation. These results have provided new suggestions for stem cell therapy.
In the past diagnosis of DVC, we mainly relied on abnormal biochemical indicators produced after different organ vascular system diseases (195). However, these biochemical indicators’ values do not change significantly in the early stage of the disease and can be interfered with by many factors (196). Different types of cells can secrete exosomes. The membrane structure of exosomes prevents enzyme degradation of its contents. The outer membrane and contents of exosomes are derived from the source cell, which is a marker of pathological changes in the source cell and also a signal mediator. Many studies have confirmed that exosomes are more related to vascular complications than the signal molecules detected in the total body fluid. However, the experimenters found that the level of exosomes in the urine of patients with early diabetic nephropathy increased, the number of exosomes in the urine of end-stage diabetic nephropathy decreased (197, 198). Therefore, the influence of exosomes processing in different stages of DVC is also a challenge for converting exosomes into biomarkers.
Diabetes is a chronic disease of the whole body. Abnormal glucose metabolism and inflammation activation are the main causes of diabetic complications. Metabolic disorders in multiple organs are the main feature of diabetes. The vascular system is the most critical channel for the exchange of nutrients and signal molecules. Both paracrine and remote signals are essential ways for diabetic organs to regulate vascular disease. Exosomes connect the crosstalk between cells and blood vessels in organs and connect the regulation of blood vessels by the body’s immune system. By summarizing previous studies, we found that exosomes can activate classical pathological pathways and provide opportunities for miRNAs and microproteins to participate in diseases. It also provides new targets and perspectives for the treatment of DVC in the future.
Stem cells have always been regarded as a very promising strategy in the treatment of diabetic complications. Several studies have confirmed that stem cells can improve diabetes complications through systemic effects. In this article, we summarized the mechanism of various stem cells to treat DVC through exosomes. These results suggest that stem cells are no longer purely cell replacement therapy and are more likely to be the source of signal release for tissue repair. The treatment of stem cell exosomes will be an important direction for diabetes and vascular diseases in the future.
In summary, this review emphasizes the diagnostic and therapeutic value of exosomes in DVC (Figure 2). However, due to the current definition of DVC, it is difficult to distinguish pathologically from similar diseases completely. The results of many exosome-related biomarker studies still need to be further confirmed. Another shortcoming in the current exosome research is the classification of the source of exosomes. Although the technology of extracting exosomes from body fluids is becoming more and more mature. However, the method of determining the source cells of exosome will be the key to pushing exosomes research to a higher level in the future. Therefore, in the study of exosomes and diabetic vascular complications, it is necessary to clarify the internal relationship between the pathological changes dominant in different stages and the changes in exosomes. In addition, exosomes from different sources represent different characteristics. By improving exosomes marking methods to clarify the distribution, uptake and half-life of exosomes, the relationship between exosomes in different cells and organs can be better clarified. It can also better avoid the non-targeted uptake and side effects of exosome therapy. In general, stem cell exosomes are a promising direction for the treatment of diabetic vascular complications, but challenges still exist in the process of clinical application in the future.
Figure 2 Schematic representation of exosomes regulating the pathological process of diabetic vascular complications. Exosomes mediate atherosclerosis and plaque rupture by regulating the production of no in aortic endothelium, increasing adhesion molecules, inflammatory transformation of macrophages, and endothelial proliferation in diabetes. In the retina, exosomes promote angiogenesis, destroy endothelial cells and increase leakage. At the same time, exosomes mediate the apoptosis of Müller cells in the early stage of DR and the proliferation and fibrosis of Müller cells in the late stage of DR. Exosomes carrying proteins and microRNAs mediate podocyte injury, basement membrane thickening, mesangial dilatation, and glomerulosclerosis in diabetic patients. Exosomes derived from stem cells can accelerate wound healing by regulating the proliferation and migration of fibroblasts and vascular endothelial cells.
BH and YL conceived the manuscript. AC, HW, and YS wrote the manuscript. CZ and YQ draw the figure. YZ and YW wrote the table. All authors contributed to the article and approved the submitted version.
This work was supported by National Natural Science Foundation of China (no. 82071336 to YL and no.82090044 to BH), Natural Science Foundation of Hubei Province (no. 2020CF763 to YL), National Natural Science Foundation of China (no. 81820108010 to BH, no. 81901212 to YZ, and no. 81901214 to YW), and National Key R&D Program of China (no. 2018YFC1312200).
The authors declare that the research was conducted in the absence of any commercial or financial relationships that could be construed as a potential conflict of interest.
All claims expressed in this article are solely those of the authors and do not necessarily represent those of their affiliated organizations, or those of the publisher, the editors and the reviewers. Any product that may be evaluated in this article, or claim that may be made by its manufacturer, is not guaranteed or endorsed by the publisher.
The Supplementary Material for this article can be found online at: https://www.frontiersin.org/articles/10.3389/fendo.2021.720466/full#supplementary-material
1. Nair GG, Tzanakakis ES, Hebrok M. Emerging Routes to the Generation of Functional Beta-Cells for Diabetes Mellitus Cell Therapy. Nat Rev Endocrinol (2020) 16(9):506–18. doi: 10.1038/s41574-020-0375-3
2. Marcovecchio ML, Dalton RN, Daneman D, Deanfield J, Jones TW, Neil HAW, et al. A New Strategy for Vascular Complications in Young People With Type 1 Diabetes Mellitus. Nat Rev Endocrinol (2019) 15(7):429–35. doi: 10.1038/s41574-019-0198-2
3. Marshall S, Flyvbjerg AJB. Prevention and Early Detection of Vascular Complications of Diabetes. BMJ (2006) 333(7566):475–80. doi: 10.1136/bmj.38922.650521.80
4. American Diabetes A. Economic Costs of Diabetes in the U.S. @ in 2017. Diabetes Care (2018) 41(5):917–28. doi: 10.2337/dci18-0007
5. Rawshani A, Rawshani A, Sattar N, Franzén S, McGuire D, Eliasson B, et al. Relative Prognostic Importance and Optimal Levels of Risk Factors for Mortality and Cardiovascular Outcomes in Type 1 Diabetes Mellitus. Circulation (2019) 139(16):1900–12. doi: 10.1161/circulationaha.118.037454
6. Holman R, Sourij H, Califf RJL. Cardiovascular Outcome Trials of Glucose-Lowering Drugs or Strategies in Type 2 Diabetes. Lancet (2014) 383(9933):2008–17. doi: 10.1016/s0140-6736(14)60794-7
7. Laakso M, Kuusisto J. Insulin Resistance and Hyperglycaemia in Cardiovascular Disease Development. Nat Rev Endocrinol (2014) 10(5):293–302. doi: 10.1038/nrendo.2014.29
8. Cole JB, Florez JC. Genetics of Diabetes Mellitus and Diabetes Complications. Nat Rev Nephrol (2020) 16(7):377–90. doi: 10.1038/s41581-020-0278-5
9. Rask-Madsen C, King GL. Vascular Complications of Diabetes: Mechanisms of Injury and Protective Factors. Cell Metab (2013) 17(1):20–33. doi: 10.1016/j.cmet.2012.11.012
10. Fioretto P, Dodson PM, Ziegler D, Rosenson RS. Residual Microvascular Risk in Diabetes: Unmet Needs and Future Directions. Nat Rev Endocrinol (2009) 6(1):19–25. doi: 10.1038/nrendo.2009.213
11. Paneni F, Beckman JA, Creager MA, Cosentino F. Diabetes and Vascular Disease: Pathophysiology, Clinical Consequences, and Medical Therapy: Part I. Eur Heart J (2013) 34(31):2436–43. doi: 10.1093/eurheartj/eht149
12. Tang J, Kern TS. Inflammation in Diabetic Retinopathy. Prog Retin Eye Res (2011) 30(5):343–58. doi: 10.1016/j.preteyeres.2011.05.002
13. Hodgkinson CP, Laxton RC, Patel K, Ye S. Advanced Glycation End-Product of Low Density Lipoprotein Activates the Toll-Like 4 Receptor Pathway Implications for Diabetic Atherosclerosis. Arterioscler Thromb Vasc Biol (2008) 28(12):2275–81. doi: 10.1161/ATVBAHA.108.175992
14. Ebrahimpour S, Zakeri M, Esmaeili A. Crosstalk Between Obesity, Diabetes, and Alzheimer’s Disease: Introducing Quercetin as an Effective Triple Herbal Medicine. Ageing Res Rev (2020) 62:101095. doi: 10.1016/j.arr.2020.101095
15. Martinez MC, Andriantsitohaina R. Extracellular Vesicles in Metabolic Syndrome. Circ Res (2017) 120(10):1674–86. doi: 10.1161/CIRCRESAHA.117.309419
16. Guay C, Kruit JK, Rome S, Menoud V, Mulder NL, Jurdzinski A, et al. Lymphocyte-Derived Exosomal MicroRNAs Promote Pancreatic β Cell Death and May Contribute to Type 1 Diabetes Development. Cell Metab (2019) 29(2):348–61.e6. doi: 10.1016/j.cmet.2018.09.011
17. Akbari A, Rezaie J. Potential Therapeutic Application of Mesenchymal Stem Cell-Derived Exosomes in SARS-CoV-2 Pneumonia. Stem Cell Res Ther (2020) 11(1):356. doi: 10.1186/s13287-020-01866-6
18. Zhang Z, Buller B, Chopp M. Exosomes - Beyond Stem Cells for Restorative Therapy in Stroke and Neurological Injury. Nat Rev Neurol (2019) 15(4):193–203. doi: 10.1038/s41582-018-0126-4
19. Sun Y, Shi H, Yin S, Ji C, Zhang X, Zhang B, et al. Human Mesenchymal Stem Cell Derived Exosomes Alleviate Type 2 Diabetes Mellitus by Reversing Peripheral Insulin Resistance and Relieving β-Cell Destruction. ACS Nano (2018) 12(8):7613–28. doi: 10.1021/acsnano.7b07643
20. Mori MA, Ludwig RG, Garcia-Martin R, Brandao BB, Kahn CR. Extracellular miRNAs: From Biomarkers to Mediators of Physiology and Disease. Cell Metab (2019) 30(4):656–73. doi: 10.1016/j.cmet.2019.07.011
21. Thery C, Witwer KW, Aikawa E, Alcaraz MJ, Anderson JD, Andriantsitohaina R, et al. Minimal Information for Studies of Extracellular Vesicles 2018 (MISEV2018): A Position Statement of the International Society for Extracellular Vesicles and Update of the MISEV2014 Guidelines. J Extracell Vesicles (2018) 7(1):1535750. doi: 10.1080/20013078.2018.1535750
22. Mathieu M, Martin-Jaular L, Lavieu G, Théry C. Specificities of Secretion and Uptake of Exosomes and Other Extracellular Vesicles for Cell-to-Cell Communication. Nat Cell Biol (2019) 21(1):9–17. doi: 10.1038/s41556-018-0250-9
23. van Niel G, D’Angelo G, Raposo G. Shedding Light on the Cell Biology of Extracellular Vesicles. Nat Rev Mol Cell Biol (2018) 19(4):213–28. doi: 10.1038/nrm.2017.125
24. Bebelman MP, Smit MJ, Pegtel DM, Baglio SR. Biogenesis and Function of Extracellular Vesicles in Cancer. Pharmacol Ther (2018) 188:1–11. doi: 10.1016/j.pharmthera.2018.02.013
25. Henne William M, Buchkovich Nicholas J, Emr Scott D. The ESCRT Pathway. Dev Cell (2011) 21(1):77–91. doi: 10.1016/j.devcel.2011.05.015
26. Hurley JH, Hanson PI. Membrane Budding and Scission by the ESCRT Machinery: It’s All in the Neck. Nat Rev Mol Cell Biol (2010) 11(8):556–66. doi: 10.1038/nrm2937
27. Choudhuri K, Llodrá J, Roth EW, Tsai J, Gordo S, Wucherpfennig KW, et al. Polarized Release of T-Cell-Receptor-Enriched Microvesicles at the Immunological Synapse. Nature (2014) 507(7490):118–23. doi: 10.1038/nature12951
29. van Niel G, Charrin S, Simoes S, Romao M, Rochin L, Saftig P, et al. The Tetraspanin CD63 Regulates ESCRT-Independent and -Dependent Endosomal Sorting During Melanogenesis. Dev Cell (2011) 21(4):708–21. doi: 10.1016/j.devcel.2011.08.019
30. Pegtel D, Gould S. Exosomes. Annu Rev Biochem (2019) 88:487–514. doi: 10.1146/annurev-biochem-013118-111902
31. Trajkovic K, Hsu C, Chiantia S, Rajendran L, Wenzel D, Wieland F, et al. Ceramide Triggers Budding of Exosome Vesicles Into Multivesicular Endosomes. Science (2008) 319(5867):1244–7. doi: 10.1126/science.1153124
32. Ibrahim A, Marbán E. Exosomes: Fundamental Biology and Roles in Cardiovascular Physiology. Annu Rev Physiol (2016) 78:67–83. doi: 10.1146/annurev-physiol-021115-104929
33. Mashouri L, Yousefi H, Aref AR, Ahadi AM, Molaei F, Alahari SK. Exosomes: Composition, Biogenesis, and Mechanisms in Cancer Metastasis and Drug Resistance. Mol Cancer (2019) 18(1):75. doi: 10.1186/s12943-019-0991-5
34. Mathivanan S, Ji H, Simpson RJ. Exosomes: Extracellular Organelles Important in Intercellular Communication. J Proteomics (2010) 73(10):1907–20. doi: 10.1016/j.jprot.2010.06.006
35. Turturici G, Tinnirello R, Sconzo G, Geraci F. Extracellular Membrane Vesicles as a Mechanism of Cell-to-Cell Communication: Advantages and Disadvantages. Am J Physiol Cell Physiol (2014) 306(7):C621–33. doi: 10.1152/ajpcell.00228.2013
36. Freeman DW, Noren Hooten N, Eitan E, Green J, Mode NA, Bodogai M, et al. Altered Extracellular Vesicle Concentration, Cargo, and Function in Diabetes. Diabetes (2018) 67(11):2377–88. doi: 10.2337/db17-1308
37. Akbar N, Azzimato V, Choudhury RP, Aouadi M. Extracellular Vesicles in Metabolic Disease. Diabetologia (2019) 62(12):2179–87. doi: 10.1007/s00125-019-05014-5
38. O’Neill S, Bohl M, Gregersen S, Hermansen K, O’Driscoll L. Blood-Based Biomarkers for Metabolic Syndrome. Trends Endocrinol Metab (2016) 27(6):363–74. doi: 10.1016/j.tem.2016.03.012
39. Salimi L, Akbari A, Jabbari N, Mojarad B, Vahhabi A, Szafert S, et al. Synergies in Exosomes and Autophagy Pathways for Cellular Homeostasis and Metastasis of Tumor Cells. Cell Biosci (2020) 10:64. doi: 10.1186/s13578-020-00426-y
40. Hassanpour M, Rezabakhsh A, Rezaie J, Nouri M, Rahbarghazi R. Exosomal Cargos Modulate Autophagy in Recipient Cells via Different Signaling Pathways. Cell Biosci (2020) 10:92. doi: 10.1186/s13578-020-00455-7
41. Guo H, Chitiprolu M, Roncevic L, Javalet C, Hemming F, Trung M, et al. Atg5 Disassociates the VV-ATPase to Promote Exosome Production and Tumor Metastasis Independent of Canonical Macroautophagy. Dev Cell (2017) 43(6):716–30.e7. doi: 10.1016/j.devcel.2017.11.018
42. Martinez J, Malireddi R, Lu Q, Cunha L, Pelletier S, Gingras S, et al. Molecular Characterization of LC3-Associated Phagocytosis Reveals Distinct Roles for Rubicon, NOX2 and Autophagy Proteins. Nat Cell Biol (2015) 17(7):893–906. doi: 10.1038/ncb3192
43. Murrow L, Malhotra R, Debnath J. ATG12-ATG3 Interacts With Alix to Promote Basal Autophagic Flux and Late Endosome Function. Nat Cell Biol (2015) 17(3):300–10. doi: 10.1038/ncb3112
44. Alvarez-Erviti L, Seow Y, Schapira AH, Gardiner C, Sargent IL, Wood MJ, et al. Lysosomal Dysfunction Increases Exosome-Mediated Alpha-Synuclein Release and Transmission. Neurobiol Dis (2011) 42(3):360–7. doi: 10.1016/j.nbd.2011.01.029
45. Cheng Z. The FoxO-Autophagy Axis in Health and Disease. Trends Endocrinol Metab (2019) 30(9):658–71. doi: 10.1016/j.tem.2019.07.009
46. Lim H, Lim YM, Kim KH, Jeon YE, Park K, Kim J, et al. A Novel Autophagy Enhancer as a Therapeutic Agent Against Metabolic Syndrome and Diabetes. Nat Commun (2018) 9(1):1438. doi: 10.1038/s41467-018-03939-w
47. Kim KH, Lee MS. Autophagy–A Key Player in Cellular and Body Metabolism. Nat Rev Endocrinol (2014) 10(6):322–37. doi: 10.1038/nrendo.2014.35
48. Li Y, Han C, Wang J, Zhou J, Liang C, Ranganna K, et al. Exosomes Mediate the Beneficial Effects of Exercise. Adv Exp Med Biol (2017) 1000:333–53. doi: 10.1007/978-981-10-4304-8_18
49. Fujita Y, Kawamoto A. Stem Cell-Based Peripheral Vascular Regeneration. Adv Drug Delivery Rev (2017) 120:25–40. doi: 10.1016/j.addr.2017.09.001
50. Ahmadi M, Rezaie J. Ageing and Mesenchymal Stem Cells Derived Exosomes: Molecular Insight and Challenges. Cell Biochem Funct (2021) 39(1):60–6. doi: 10.1002/cbf.3602
51. Rezaie J, Nejati V, Khaksar M, Oryan A, Aghamohamadzadeh N, Shariatzadeh M, et al. Diabetic Sera Disrupted the Normal Exosome Signaling Pathway in Human Mesenchymal Stem Cells In Vitro. Cell Tissue Res (2018) 374(3):555–65. doi: 10.1007/s00441-018-2895-x
52. Rezaie J, Mehranjani MS, Rahbarghazi R, Shariatzadeh MA. Angiogenic and Restorative Abilities of Human Mesenchymal Stem Cells Were Reduced Following Treatment With Serum From Diabetes Mellitus Type 2 Patients. J Cell Biochem (2018) 119(1):524–35. doi: 10.1002/jcb.26211
53. Soraya H, Sani NA, Jabbari N, Rezaie J. Metformin Increases Exosome Biogenesis and Secretion in U87 MG Human Glioblastoma Cells: A Possible Mechanism of Therapeutic Resistance. Arch Med Res (2021) 52(2):151–62. doi: 10.1016/j.arcmed.2020.10.007
54. Hassanpour M, Cheraghi O, Siavashi V, Rahbarghazi R, Nouri M. A Reversal of Age-Dependent Proliferative Capacity of Endothelial Progenitor Cells From Different Species Origin in In Vitro Condition. J Cardiovasc Thorac Res (2016) 8(3):102–6. doi: 10.15171/jcvtr.2016.22
55. Donath M, Dinarello C, Mandrup-Poulsen T. Targeting Innate Immune Mediators in Type 1 and Type 2 Diabetes. Nat Rev Immunol (2019) 19(12):734–46. doi: 10.1038/s41577-019-0213-9
56. Hotamisligil G. Foundations of Immunometabolism and Implications for Metabolic Health and Disease. Immunity (2017) 47(3):406–20. doi: 10.1016/j.immuni.2017.08.009
57. Lackey D, Olefsky J. Regulation of Metabolism by the Innate Immune System. Nat Rev Endocrinol (2016) 12(1):15–28. doi: 10.1038/nrendo.2015.189
58. Rutman AK, Negi S, Gasparrini M, Hasilo CP, Tchervenkov J, Paraskevas S. Immune Response to Extracellular Vesicles From Human Islets of Langerhans in Patients With Type 1 Diabetes. Endocrinology (2018) 159(11):3834–47. doi: 10.1210/en.2018-00649
59. Li M, Wang T, Tian H, Wei G, Zhao L, Shi Y. Macrophage-Derived Exosomes Accelerate Wound Healing Through Their Anti-Inflammation Effects in a Diabetic Rat Model. Artif Cells Nanomed Biotechnol (2019) 47(1):3793–803. doi: 10.1080/21691401.2019.1669617
60. Tesovnik T, Kovač J, Pohar K, Hudoklin S, Dovč K, Bratina N, et al. Extracellular Vesicles Derived Human-miRNAs Modulate the Immune System in Type 1 Diabetes. Front Cell Dev Biol (2020) 8:202. doi: 10.3389/fcell.2020.00202
61. Herrington W, Lacey B, Sherliker P, Armitage J, Lewington S. Epidemiology of Atherosclerosis and the Potential to Reduce the Global Burden of Atherothrombotic Disease. Circ Res (2016) 118(4):535–46. doi: 10.1161/CIRCRESAHA.115.307611
62. Bentzon Jacob F, Otsuka F, Virmani R, Falk E. Mechanisms of Plaque Formation and Rupture. Circ Res (2014) 114(12):1852–66. doi: 10.1161/CIRCRESAHA.114.302721
63. Virmani R, Burke AP, Farb A, Kolodgie FD. Pathology of the Vulnerable Plaque. J Am Coll Cardiol (2006) 47(8 Suppl):C13–8. doi: 10.1016/j.jacc.2005.10.065
64. Falk E. Pathogenesis of Atherosclerosis. J Am Coll Cardiol (2006) 47(8 Suppl):C7–12. doi: 10.1016/j.jacc.2005.09.068
65. Bai S, Yin Q, Dong T, Dai F, Qin Y, Ye L, et al. Endothelial Progenitor Cell-Derived Exosomes Ameliorate Endothelial Dysfunction in a Mouse Model of Diabetes. BioMed Pharmacother (2020) 131:110756. doi: 10.1016/j.biopha.2020.110756
66. Wang F, Chen FF, Shang YY, Li Y, Wang ZH, Han L, et al. Insulin Resistance Adipocyte-Derived Exosomes Aggravate Atherosclerosis by Increasing Vasa Vasorum Angiogenesis in Diabetic ApoE(-/-) Mice. Int J Cardiol (2018) 265:181–7. doi: 10.1016/j.ijcard.2018.04.028
67. Hulsmans M, Holvoet P. MicroRNA-Containing Microvesicles Regulating Inflammation in Association With Atherosclerotic Disease. Cardiovasc Res (2013) 100(1):7–18. doi: 10.1093/cvr/cvt161
68. Goetzl EJ, Schwartz JB, Mustapic M, Lobach IV, Daneman R, Abner EL, et al. Altered Cargo Proteins of Human Plasma Endothelial Cell-Derived Exosomes in Atherosclerotic Cerebrovascular Disease. FASEB J (2017) 31(8):3689–94. doi: 10.1096/fj.201700149
69. Creager MA, Lüscher TF, Cosentino F, Beckman JA. Diabetes and Vascular Disease: Pathophysiology, Clinical Consequences, and Medical Therapy: Part I. Circulation (2003) 108(12):1527–32. doi: 10.1161/01.Cir.0000091257.27563.32
70. Cherry P, Furchgott R, Zawadzki J, Jothianandan D. Role of Endothelial Cells in Relaxation of Isolated Arteries by Bradykinin. Proc Natl Acad Sci USA (1982) 79(6):2106–10. doi: 10.1073/pnas.79.6.2106
71. Ignarro L, Byrns R, Buga G, Wood K. Endothelium-Derived Relaxing Factor From Pulmonary Artery and Vein Possesses Pharmacologic and Chemical Properties Identical to Those of Nitric Oxide Radical. Circ Res (1987) 61(6):866–79. doi: 10.1161/01.res.61.6.866
72. Herman A, Moncada S. Therapeutic Potential of Nitric Oxide Donors in the Prevention and Treatment of Atherosclerosis. Eur Heart J (2005) 26(19):1945–55. doi: 10.1093/eurheartj/ehi333
73. Förstermann U, Xia N, Li H. Roles of Vascular Oxidative Stress and Nitric Oxide in the Pathogenesis of Atherosclerosis. Circ Res (2017) 120(4):713–35. doi: 10.1161/circresaha.116.309326
74. Zhang H, Liu J, Qu D, Wang L, Wong CM, Lau CW, et al. Serum Exosomes Mediate Deliveryof Arginase 1 as a Novel Mechanism for Endothelial Dysfunction in Diabetes. Proc Natl Acad Sci USA (2018) 115(29):E6927–36. doi: 10.1073/pnas.1721521115
75. Berkowitz D, White R, Li D, Minhas K, Cernetich A, Kim S, et al. Arginase Reciprocally Regulates Nitric Oxide Synthase Activity and Contributes to Endothelial Dysfunction in Aging Blood Vessels. Circulation (2003) 108(16):2000–6. doi: 10.1161/01.Cir.0000092948.04444.C7
76. Gao W, Liu H, Yuan J, Wu C, Huang D, Ma Y, et al. Exosomes Derived From Mature Dendritic Cells Increase Endothelial Inflammation and Atherosclerosis via Membrane TNF-α Mediated NF-κb Pathway. J Cell Mol Med (2016) 20(12):2318–27. doi: 10.1111/jcmm.12923
77. Bouchareychas L, Duong P, Covarrubias S, Alsop E, Phu TA, Chung A, et al. Macrophage Exosomes Resolve Atherosclerosis by Regulating Hematopoiesis and Inflammation via MicroRNA Cargo. Cell Rep (2020) 32(2):107881. doi: 10.1016/j.celrep.2020.107881
78. Ferreira N, Bruder-Nascimento T, Pereira C, Zanotto C, Prado D, Silva J, et al. NLRP3 Inflammasome and Mineralocorticoid Receptors Are Associated With Vascular Dysfunction in Type 2 Diabetes Mellitus. Cells (2019) 8(12):1595. doi: 10.3390/cells8121595
79. Zhang J, Xia L, Zhang F, Zhu D, Xin C, Wang H, et al. A Novel Mechanism of Diabetic Vascular Endothelial Dysfunction: Hypoadiponectinemia-Induced NLRP3 Inflammasome Activation. Biochim. Biophys Acta Mol Basis Dis (2017) 1863(6):1556–67. doi: 10.1016/j.bbadis.2017.02.012
80. Shin J, Lee E, Park T, Kim W. Damage-Associated Molecular Patterns and Their Pathological Relevance in Diabetes Mellitus. Ageing Res Rev (2015) 24:66–76. doi: 10.1016/j.arr.2015.06.004
81. Chen Y, Wang L, Pitzer A, Li X, Li P, Zhang Y. Contribution of Redox-Dependent Activation of Endothelial Nlrp3 Inflammasomes to Hyperglycemia-Induced Endothelial Dysfunction. J Mol Med (Berl) (2016) 94(12):1335–47. doi: 10.1007/s00109-016-1481-5
82. Kelley N, Jeltema D, Duan Y, He Y. The NLRP3 Inflammasome: An Overview of Mechanisms of Activation and Regulation. Int J Mol Sci (2019) 20(13):3328. doi: 10.3390/ijms20133328
83. Cypryk W, Nyman TA, Matikainen S. From Inflammasome to Exosome-Does Extracellular Vesicle Secretion Constitute an Inflammasome-Dependent Immune Response? Front Immunol (2018) 9:2188. doi: 10.3389/fimmu.2018.02188
84. Yuan X, Bhat OM, Lohner H, Zhang Y, Li PL. Endothelial Acid Ceramidase in Exosome-Mediated Release of NLRP3 Inflammasome Products During Hyperglycemia: Evidence From Endothelium-Specific Deletion of Asah1 Gene. Biochim Biophys Acta Mol Cell Biol Lipids (2019) 1864(12):158532. doi: 10.1016/j.bbalip.2019.158532
85. Lavine KJ, Kovacs A, Ornitz DM. Hedgehog Signaling is Critical for Maintenance of the Adult Coronary Vasculature in Mice. J Clin Invest (2008) 118(7):2404–14. doi: 10.1172/jci34561
86. Beckers L, Heeneman S, Wang L, Burkly LC, Rousch MM, Davidson NO, et al. Disruption of Hedgehog Signalling in ApoE -/- Mice Reduces Plasma Lipid Levels, But Increases Atherosclerosis Due to Enhanced Lipid Uptake by Macrophages. J Pathol (2007) 212(4):420–8. doi: 10.1002/path.2193
87. Blüher M. Adipose Tissue Dysfunction Contributes to Obesity Related Metabolic Diseases. Best Pract Res Clin Endocrinol Metab (2013) 27(2):163–77. doi: 10.1016/j.beem.2013.02.005
88. Klöting N, Blüher M. Adipocyte Dysfunction, Inflammation and Metabolic Syndrome. Rev Endocr Metab Disord (2014) 15(4):277–87. doi: 10.1007/s11154-014-9301-0
89. Vujosevic S, Aldington SJ, Silva P, Hernández C, Scanlon P, Peto T, et al. Screening for Diabetic Retinopathy: New Perspectives and Challenges. Lancet Diabetes Endocrinol (2020) 8(4):337–47. doi: 10.1016/s2213-8587(19)30411-5
90. Cheung N, Mitchell P, Wong TY. Diabetic Retinopathy. Lancet (2010) 376(9735):124–36. doi: 10.1016/s0140-6736(09)62124-3
91. Cerani A, Tetreault N, Menard C, Lapalme E, Patel C, Sitaras N, et al. Neuron-Derived Semaphorin 3A is an Early Inducer of Vascular Permeability in Diabetic Retinopathy via Neuropilin-1. Cell Metab (2013) 18(4):505–18. doi: 10.1016/j.cmet.2013.09.003
92. Liu C, Ge H, Liu B, Dong R, Shan K, Chen X, et al. Targeting Pericyte-Endothelial Cell Crosstalk by Circular RNA-Cpwwp2a Inhibition Aggravates Diabetes-Induced Microvascular Dysfunction. Proc Natl Acad Sci USA (2019) 116(15):7455–64. doi: 10.1073/pnas.1814874116
93. Bringmann A, Pannicke T, Grosche J, Francke M, Wiedemann P, Skatchkov S, et al. Müller Cells in the Healthy and Diseased Retina. Prog Retin Eye Res (2006) 25(4):397–424. doi: 10.1016/j.preteyeres.2006.05.003
94. Cuenca N, Fernández-Sánchez L, Campello L, Maneu V, de la Villa P, Lax P, et al. Cellular Responses Following Retinal Injuries and Therapeutic Approaches for Neurodegenerative Diseases. Prog Retin Eye Res (2014) 43:17–75. doi: 10.1016/j.preteyeres.2014.07.001
95. Dyer M, Cepko C. Control of Müller Glial Cell Proliferation and Activation Following Retinal Injury. Nat Neurosci (2000) 3(9):873–80. doi: 10.1038/78774
96. Smith M, Simmons K, Cambier J. B Cells in Type 1 Diabetes Mellitus and Diabetic Kidney Disease. Nat Rev Nephrol (2017) 13(11):712–20. doi: 10.1038/nrneph.2017.138
97. Kamalden T, Macgregor-Das A, Kannan S, Dunkerly-Eyring B, Khaliddin N, Xu Z, et al. Exosomal MicroRNA-15a Transfer From the Pancreas Augments Diabetic Complications by Inducing Oxidative Stress. Antioxid Redox Signal (2017) 27(13):913–30. doi: 10.1089/ars.2016.6844
98. Tonade D, Kern T. Photoreceptor Cells and RPE Contribute to the Development of Diabetic Retinopathy. Prog Retin Eye Res (2020) 83:100919. doi: 10.1016/j.preteyeres.2020.100919
99. Maisto R, Oltra M, Vidal-Gil L, Martínez-Gil N, Sancho-Pellúz J, Filippo C, et al. ARPE-19-Derived VEGF-Containing Exosomes Promote Neovascularization in HUVEC: The Role of the Melanocortin Receptor 5. Cell Cycle (2019) 18(4):413–24. doi: 10.1080/15384101.2019.1568745
100. Gu S, Liu Y, Zou J, Wang W, Wei T, Wang X, et al. Retinal Pigment Epithelial Cells Secrete miR-202-5p-Containing Exosomes to Protect Against Proliferative Diabetic Retinopathy. Exp Eye Res (2020) 201:108271. doi: 10.1016/j.exer.2020.108271
101. Joussen A, Murata T, Tsujikawa A, Kirchhof B, Bursell S, Adamis A. Leukocyte-Mediated Endothelial Cell Injury and Death in the Diabetic Retina. Am J Pathol (2001) 158(1):147–52. doi: 10.1016/s0002-9440(10)63952-1
102. Propson N, Roy E, Litvinchuk A, Köhl J, Zheng H. Endothelial C3a Receptor Mediates Vascular Inflammation and Blood-Brain Barrier Permeability During Aging. J Clin Invest (2021) 131(1):e140966. doi: 10.1172/jci140966
103. Flyvbjerg A. Diabetic Angiopathy, the Complement System and the Tumor Necrosis Factor Superfamily. Nat Rev Endocrinol (2010) 6(2):94–101. doi: 10.1038/nrendo.2009.266
104. Yanai R, Thanos A, Connor KM. Complement Involvement in Neovascular Ocular Diseases. Adv Exp Med Biol (2012) 946:161–83. doi: 10.1007/978-1-4614-0106-3_10
105. Acosta J, Hettinga J, Flückiger R, Krumrei N, Goldfine A, Angarita L, et al. Molecular Basis for a Link Between Complement and the Vascular Complications of Diabetes. Proc Natl Acad Sci USA (2000) 97(10):5450–5. doi: 10.1073/pnas.97.10.5450
106. Huang C, Fisher K, Hammer S, Navitskaya S, Blanchard G, Busik JJD. Plasma Exosomes Contribute to Microvascular Damage in Diabetic Retinopathy by Activating the Classical Complement Pathway. Diabetes (2018) 67(8):1639–49. doi: 10.2337/db17-1587
107. Tuttle KR, Bakris GL, Bilous RW, Chiang JL, de Boer IH, Goldstein-Fuchs J, et al. Diabetic Kidney Disease: A Report From an ADA Consensus Conference. Diabetes Care (2014) 37(10):2864–83. doi: 10.2337/dc14-1296
108. Kim SS, Kim JH, Kim IJ. Current Challenges in Diabetic Nephropathy: Early Diagnosis and Ways to Improve Outcomes. Endocrinol Metab (Seoul) (2016) 31(2):245–53. doi: 10.3803/EnM.2016.31.2.245
109. Vallon V, Thomson SC. Renal Function in Diabetic Disease Models: The Tubular System in the Pathophysiology of the Diabetic Kidney. Annu Rev Physiol (2012) 74:351–75. doi: 10.1146/annurev-physiol-020911-153333
110. Qin X, Zhao Y, Gong J, Huang W, Su H, Yuan F, et al. Berberine Protects Glomerular Podocytes via Inhibiting Drp1-Mediated Mitochondrial Fission and Dysfunction. Theranostics (2019) 9(6):1698–713. doi: 10.7150/thno.30640
111. You YH, Quach T, Saito R, Pham J, Sharma K. Metabolomics Reveals a Key Role for Fumarate in Mediating the Effects of NADPH Oxidase 4 in Diabetic Kidney Disease. J Am Soc Nephrol (2016) 27(2):466–81. doi: 10.1681/ASN.2015030302
112. Xu WC, Qian G, Liu AQ, Li YQ, Zou HQ. Urinary Extracellular Vesicle: A Potential Source of Early Diagnostic and Therapeutic Biomarker in Diabetic Kidney Disease. Chin Med J (Engl) (2018) 131(11):1357–64. doi: 10.4103/0366-6999.232801
113. Kikuchi K, Saigusa D, Kanemitsu Y, Matsumoto Y, Thanai P, Suzuki N, et al. Gut Microbiome-Derived Phenyl Sulfate Contributes to Albuminuria in Diabetic Kidney Disease. Nat Commun (2019) 10(1):1835. doi: 10.1038/s41467-019-09735-4
114. Mulder S, Hamidi H, Kretzler M, Ju W. An Integrative Systems Biology Approach for Precision Medicine in Diabetic Kidney Disease. Diabetes Obes Metab (2018) 20 Suppl 3:6–13. doi: 10.1111/dom.13416
115. Kelly D, Rothwell PM. Disentangling the Multiple Links Between Renal Dysfunction and Cerebrovascular Disease. J Neurol Neurosurg Psychiatry (2020) 91(1):88–97. doi: 10.1136/jnnp-2019-320526
116. Remuzzi G, Benigni A, Remuzzi A. Mechanisms of Progression and Regression of Renal Lesions of Chronic Nephropathies and Diabetes. J Clin Invest (2006) 116(2):288–96. doi: 10.1172/JCI27699
117. Uwaezuoke SN. The Role of Novel Biomarkers in Predicting Diabetic Nephropathy: A Review. Int J Nephrol Renovasc Dis (2017) 10:221–31. doi: 10.2147/IJNRD.S143186
118. Alvarez ML, Khosroheidari M, Kanchi Ravi R, DiStefano JK. Comparison of Protein, microRNA, and mRNA Yields Using Different Methods of Urinary Exosome Isolation for the Discovery of Kidney Disease Biomarkers. Kidney Int (2012) 82(9):1024–32. doi: 10.1038/ki.2012.256
119. Miranda KC, Bond DT, McKee M, Skog J, Paunescu TG, Da Silva N, et al. Nucleic Acids Within Urinary Exosomes/Microvesicles are Potential Biomarkers for Renal Disease. Kidney Int (2010) 78(2):191–9. doi: 10.1038/ki.2010.106
120. Gudehithlu KP, Garcia-Gomez I, Vernik J, Brecklin C, Kraus M, Cimbaluk DJ, et al. In Diabetic Kidney Disease Urinary Exosomes Better Represent Kidney Specific Protein Alterations Than Whole Urine. Am J Nephrol (2015) 42(6):418–24. doi: 10.1159/000443539
121. Pisitkun T, Johnstone R, Knepper MA. Discovery of Urinary Biomarkers. Mol Cell Proteomics (2006) 5(10):1760–71. doi: 10.1074/mcp.R600004-MCP200
122. Fehlmann T, Lehallier B, Schaum N, Hahn O, Keller A. Common Diseases Alter the Physiological Age-Related Blood microRNA Profile. Nat Commun (2020) 11(1):5958. doi: 10.1038/s41467-020-19665-1
123. Chang TC, Mendell JT. microRNAs in Vertebrate Physiology and Human Disease. Annu Rev Genomics Hum Genet (2007) 8(1):215. doi: 10.1146/annurev.genom.8.080706.092351
124. Sun Y. Development of a Micro-Array to Detect Human and Mouse microRNAs and Characterization of Expression in Human Organs. Nucleic Acids Res (2004) 32(22):e188–e. doi: 10.1093/nar/gnh186
125. Jia Y, Guan M, Zheng Z, Zhang Q, Tang C, Xu W, et al. miRNAs in Urine Extracellular Vesicles as Predictors of Early-Stage Diabetic Nephropathy. J Diabetes Res (2016) 2016:7932765. doi: 10.1155/2016/7932765
126. Xie Y, Jia Y, Xie C, Hu F, Xue M, Xue Y. Urinary Exosomal MicroRNA Profiling in Incipient Type 2 Diabetic Kidney Disease. J Diabetes Res (2017) 2017:1–10. doi: 10.1155/2017/6978984
127. Federica B, Marinella T, Alessandro C, Laura A, Silvia P, Serena G, et al. Urinary Exosomal MicroRNAs in Incipient Diabetic Nephropathy. PloS One (2013) 8(11):e73798. doi: 10.1371/journal.pone.0073798
128. Eissa S, Matboli M, Aboushahba R, Bekhet MM, Soliman Y. Urinary Exosomal microRNA Panel Unravels Novel Biomarkers for Diagnosis of Type 2 Diabetic Kidney Disease. J Diabetes Complications (2016) 30(8):1585–92. doi: 10.1016/j.jdiacomp.2016.07.012
129. Eissa S, Matboli M, Bekhet MJB. Biomedecine P, Pharmacotherapie. Clinical Verification of a Novel Urinary microRNA Panal: 133b, -342 and -30 as Biomarkers for Diabetic Nephropathy Identified by Bioinformatics Analysis. BioMed Pharmacother (2016) 83:92–9. doi: 10.1016/j.biopha.2016.06.018
130. Zang J, Maxwell A, Simpson D, McKay GJ. Differential Expression of Urinary Exosomal MicroRNAs miR-21-5p and miR-30b-5p in Individuals With Diabetic Kidney Disease. Sci Rep (2019) 9(1):10900. doi: 10.1038/s41598-019-47504-x
131. Dey N, Das F, Mariappan M, Mandal C, Ghosh-Choudhury N, Kasinath B, et al. MicroRNA-21 Orchestrates High Glucose-Induced Signals to TOR Complex 1, Resulting in Renal Cell Pathology in Diabetes. J Biol Chem (2011) 286(29):25586–603. doi: 10.1074/jbc.M110.208066
132. Gao S, Chen D, Zou L, Huang L, Dai R, Jie Z, et al. MiR-21 Overexpression Enhances TGF-β1-Induced Epithelial-to-Mesenchymal Transition by Target RECK in Hepatic Oval Cells. Int J Clin Exp Pathol (2016) 9(4):4779–85. doi: 10.1016/j.mce.2014.05.018
133. Wu J, Zheng C, Fan Y, Zeng C, Chen Z, Qin W, et al. Downregulation of MicroRNA-30 Facilitates Podocyte Injury and Is Prevented by Glucocorticoids. J Am Soc Nephrol (2014) 25(1):92–104. doi: 10.1681/ASN.2012111101
134. Zubiri I, Posada-Ayala M, Sanz-Maroto A, Calvo E, Martin-Lorenzo M, Gonzalez-Calero L, et al. Diabetic Nephropathy Induces Changes in the Proteome of Human Urinary Exosomes as Revealed by Label-Free Comparative Analysis. J Proteomics (2014) 96:92–102. doi: 10.1016/j.jprot.2013.10.037
135. Clayton A, Turkes A, Dewitt S, Steadman R, Mason M, Hallett M. Adhesion and Signaling by B Cell-Derived Exosomes: The Role of Integrins. FASEB J (2004) 18(9):977–9. doi: 10.1096/fj.03-1094fje
136. Lee J, Saha P, Yang Q, Lee S, Park J, Suh Y, et al. Targeted Inactivation of MLL3 Histone H3-Lys-4 Methyltransferase Activity in the Mouse Reveals Vital Roles for MLL3 in Adipogenesis. Proc Natl Acad Sci USA (2008) 105(49):19229–34. doi: 10.1073/pnas.0810100105
137. Grewal J, Tsai J, Khan S. Oxalate-Inducible AMBP Gene and its Regulatory Mechanism in Renal Tubular Epithelial Cells. Biochem J (2005) 387:609–16. doi: 10.1042/bj20041465
138. Riaz S, Skinner V, Srai SK. Effect of High Dose Thiamine on the Levels of Urinary Protein Biomarkers in Diabetes Mellitus Type 2. J Pharm BioMed Anal (2011) 54(4):817–25. doi: 10.1016/j.jpba.2010.11.008
139. Buschow S, van Balkom B, Aalberts M, Heck A, Wauben M, Stoorvogel WJI, et al. MHC Class II-Associated Proteins in B-Cell Exosomes and Potential Functional Implications for Exosome Biogenesis. Immunol Cell Biol (2010) 88(8):851–6. doi: 10.1038/icb.2010.64
140. Lawen A, Ly JD, Lane DJ, Zarschler K, Messina A, De Pinto V. Voltage-Dependent Anion-Selective Channel 1 (VDAC1)–A Mitochondrial Protein, Rediscovered as a Novel Enzyme in the Plasma Membrane. Int J Biochem Cell Biol (2005) 37(2):277–82. doi: 10.1016/j.biocel.2004.05.013
141. Shoshan-Barmatz V, De Pinto V, Zweckstetter M, Raviv Z, Keinan N, Arbel N. VDAC, A Multi-Functional Mitochondrial Protein Regulating Cell Life and Death. Mol Aspects Med (2010) 31(3):227–85. doi: 10.1016/j.mam.2010.03.002
142. Cornish TC, Bagnasco SM, Macgregor AM, Lu J, Selvin E, Halushka MK. Glomerular Protein Levels of Matrix Metalloproteinase-1 and Tissue Inhibitor of Metalloproteinase-1 Are Lower in Diabetic Subjects. J Histochem Cytochem (2009) 57(11):995–1001. doi: 10.1369/jhc.2009.954107
143. Duan S, Chen J, Wu L, Nie G, Sun L, Zhang C, et al. Assessment of Urinary NGAL for Differential Diagnosis and Progression of Diabetic Kidney Disease. J Diabetes Complications (2020) 34(10):107665. doi: 10.1016/j.jdiacomp.2020.107665
144. Takamiya Y, Fukami K, Yamagishi S, Kaida Y, Nakayama Y, Obara N, et al. Experimental Diabetic Nephropathy Is Accelerated in Matrix Metalloproteinase-2 Knockout Mice. Nephrol Dial Transplant (2013) 28(1):55–62. doi: 10.1093/ndt/gfs387
145. Bonventre JV. Can We Target Tubular Damage to Prevent Renal Function Decline in Diabetes? Semin Nephrol (2012) 32(5):452–62. doi: 10.1016/j.semnephrol.2012.07.008
146. Andersen H, Friis UG, Hansen PB, Svenningsen P, Henriksen JE, Jensen BL. Diabetic Nephropathy is Associated With Increased Urine Excretion of Proteases Plasmin, Prostasin and Urokinase and Activation of Amiloride-Sensitive Current in Collecting Duct Cells. Nephrol Dial Transplant (2015) 30(5):781–9. doi: 10.1093/ndt/gfu402
147. Cheung PW, Bouley R, Brown D. Targeting the Trafficking of Kidney Water Channels for Therapeutic Benefit. Annu Rev Pharmacol Toxicol (2020) 60:175–94. doi: 10.1146/annurev-pharmtox-010919-023654
148. Rossi L, Nicoletti M, Carmosino M, Mastrofrancesco L, Di Franco A, Indrio F, et al. Urinary Excretion of Kidney Aquaporins as Possible Diagnostic Biomarker of Diabetic Nephropathy. J Diabetes Res (2017) 2017:4360357. doi: 10.1155/2017/4360357
149. Pisitkun T, Shen R, Knepper M. Identification and Proteomic Profiling of Exosomes in Human Urine. Proc Natl Acad Sci USA (2004) 101(36):13368–73. doi: 10.1073/pnas.0403453101
150. He X, Cheng R, Huang C, Takahashi Y, Yang Y, Benyajati S, et al. A Novel Role of LRP5 in Tubulointerstitial Fibrosis Through Activating TGF-β/Smad Signaling. Signal Transduct Target Ther (2020) 5(1):45. doi: 10.1038/s41392-020-0142-x
151. Zhu QJ, Zhu M, Xu X, Meng X, Wu YG. Exosomes From High Glucose–Treated Macrophages Activate Glomerular Mesangial Cells via TGF-β1/Smad3 Pathway In Vivo and In Vitro. FASEB J (2019) 33(8):9279–90. doi: 10.1096/fj.201802427RRR
152. Kang J, Saunier E, Akhurst R, Derynck R. The Type I TGF-Beta Receptor is Covalently Modified and Regulated by Sumoylation. Nat Cell Biol (2008) 10(6):654–64. doi: 10.1038/ncb1728
153. Sakurai A, Ono H, Ochi A, Matsuura M, Doi T. Involvement of Elf3 on Smad3 Activation-Dependent Injuries in Podocytes and Excretion of Urinary Exosome in Diabetic Nephropathy. PloS One (2019) 14(5):e0216788. doi: 10.1371/journal.pone.0216788
154. Fu Y, Sun Y, Wang M, Hou Y, Huang W, Zhou D, et al. Elevation of JAML Promotes Diabetic Kidney Disease by Modulating Podocyte Lipid Metabolism. Cell Metab (2020) 32(6):1052–62.e8. doi: 10.1016/j.cmet.2020.10.019
155. Carney E. Diabetic Nephropathy: Restoring Podocyte Proteostasis in DN. Nat Rev Nephrol (2017) 13(9):514. doi: 10.1038/nrneph.2017.111
156. Wang YY, Tang LQ, Wei W. Berberine Attenuates Podocytes Injury Caused by Exosomes Derived From High Glucose-Induced Mesangial Cells Through Tgfβ1-PI3K/AKT Pathway. Eur J Pharmacol (2018) 824:185–92. doi: 10.1016/j.ejphar.2018.01.034
157. Hideharu A, Akiko S, Hiroyuki O, Sanae H, Sakiya Y, Arisa O, et al. Urinary Exosomal mRNA of WT1 as Diagnostic and Prognostic Biomarker for Diabetic Nephropathy. J Med Invest (2018) 65(3.4):208–15. doi: 10.2152/jmi.65.208
158. Kalani A, Mohan A, Godbole MM, Bhatia E, Gupta A, Sharma RK, et al. Wilm’s Tumor-1 Protein Levels in Urinary Exosomes From Diabetic Patients With or Without Proteinuria. PloS One (2013) 8(3):e60177. doi: 10.1371/journal.pone.0060177
159. Satirapoj B, Adler SG. Comprehensive Approach to Diabetic Nephropathy. Kidney Res Clin Pract (2014) 33(3):121–31. doi: 10.1016/j.krcp.2014.08.001
160. Liu Y, Tang SC. Recent Progress in Stem Cell Therapy for Diabetic Nephropathy. Kidney Dis (Basel) (2016) 2(1):20–7. doi: 10.1159/000441913
161. Cha BH, Kim JS, Bello A, Lee GH, Kim DH, Kim BJ, et al. Efficient Isolation and Enrichment of Mesenchymal Stem Cells From Human Embryonic Stem Cells by Utilizing the Interaction Between Integrin Alpha5beta1 and Fibronectin. Adv Sci (Weinh) (2020) 7(17):2001365. doi: 10.1002/advs.202001365
162. Nikfarjam S, Rezaie J, Zolbanin NM, Jafari R. Mesenchymal Stem Cell Derived-Exosomes: A Modern Approach in Translational Medicine. J Transl Med (2020) 18(1):449. doi: 10.1186/s12967-020-02622-3
163. Babaei M, Rezaie J. Application of Stem Cell-Derived Exosomes in Ischemic Diseases: Opportunity and Limitations. J Transl Med (2021) 19(1):196. doi: 10.1186/s12967-021-02863-w
164. Safwat A, Sabry D, Ragiae A, Amer E, Mahmoud RH, Shamardan RM. Adipose Mesenchymal Stem Cells-Derived Exosomes Attenuate Retina Degeneration of Streptozotocin-Induced Diabetes in Rabbits. J Circ Biomark (2018) 7:1849454418807827. doi: 10.1177/1849454418807827
165. Nagaishi K, Mizue Y, Chikenji T, Otani M, Nakano M, Konari N, et al. Mesenchymal Stem Cell Therapy Ameliorates Diabetic Nephropathy via the Paracrine Effect of Renal Trophic Factors Including Exosomes. Sci Rep (2016) 6:34842. doi: 10.1038/srep34842
166. Tang SC, Leung JC, Chan LY, Tsang AW, Lai KN. Activation of Tubular Epithelial Cells in Diabetic Nephropathy and the Role of the Peroxisome Proliferator-Activated Receptor-Gamma Agonist. J Am Soc Nephrol (2006) 17(6):1633–43. doi: 10.1681/ASN.2005101113
167. Sugimoto H, LeBleu VS, Bosukonda D, Keck P, Taduri G, Bechtel W, et al. Activin-Like Kinase 3 is Important for Kidney Regeneration and Reversal of Fibrosis. Nat Med (2012) 18(3):396–404. doi: 10.1038/nm.2629
168. Hills CE, Squires PE. The Role of TGF-Beta and Epithelial-to Mesenchymal Transition in Diabetic Nephropathy. Cytokine Growth Factor Rev (2011) 22(3):131–9. doi: 10.1016/j.cytogfr.2011.06.002
169. De Rechter S, Decuypere JP, Ivanova E, van den Heuvel LP, De Smedt H, Levtchenko E, et al. Autophagy in Renal Diseases. Pediatr Nephrol (2016) 31(5):737–52. doi: 10.1007/s00467-015-3134-2
170. Lloberas N, Cruzado JM, Franquesa M, Herrero-Fresneda I, Torras J, Alperovich G, et al. Mammalian Target of Rapamycin Pathway Blockade Slows Progression of Diabetic Kidney Disease in Rats. J Am Soc Nephrol (2006) 17(5):1395–404. doi: 10.1681/ASN.2005050549
171. Mori H, Inoki K, Masutani K, Wakabayashi Y, Komai K, Nakagawa R, et al. The mTOR Pathway is Highly Activated in Diabetic Nephropathy and Rapamycin has a Strong Therapeutic Potential. Biochem Biophys Res Commun (2009) 384(4):471–5. doi: 10.1016/j.bbrc.2009.04.136
172. Godel M, Hartleben B, Herbach N, Liu S, Zschiedrich S, Lu S, et al. Role of mTOR in Podocyte Function and Diabetic Nephropathy in Humans and Mice. J Clin Invest (2011) 121(6):2197–209. doi: 10.1172/JCI44774
173. Ebrahim N, Ahmed IA, Hussien NI, Dessouky AA, Farid AS, Elshazly AM, et al. Mesenchymal Stem Cell-Derived Exosomes Ameliorated Diabetic Nephropathy by Autophagy Induction Through the mTOR Signaling Pathway. Cells (2018) 7(12):226. doi: 10.3390/cells7120226
174. Zhang X, Song Z, Guo Y, Zhou M. The Novel Role of TRPC6 in Vitamin D Ameliorating Podocyte Injury in STZ-Induced Diabetic Rats. Mol Cell Biochem (2015) 399(1-2):155–65. doi: 10.1007/s11010-014-2242-9
175. Bus P, Scharpfenecker M, Priscilla V, Wolterbeek R, Bruijn JA, Baelde HJJD. The VEGF-A Inhibitor sFLT-1 Improves Renal Function by Reducing Endothelial Activation and Inflammation in a Mouse Model of Type 1 Diabetes. Diabetologia (2017) 30(9):1813–21. doi: 10.1007/s00125-017-4322-3
176. Duan Y-R, Chen B-P, Chen F, Yang S-X, Zhu C-Y, Ma YL, et al. Exosomal microRNA-16-5p From Human Urine-Derived Stem Cells Ameliorates Diabetic Nephropathy Through Protection of Podocyte. J Cell Mol Med (2019). doi: 10.1111/jcmm.14558
177. Alexiadou K, Doupis J. Management of Diabetic Foot Ulcers. Diabetes Ther (2012) 3(1):4–. doi: 10.1007/s13300-012-0004-9
178. Wicks K, Torbica T, Mace KA. Myeloid Cell Dysfunction and the Pathogenesis of the Diabetic Chronic Wound. Semin Immunol (2014) 26(4):341–53. doi: 10.1016/j.smim.2014.04.006
179. Acosta JB, del Barco DG, Vera DC, Savigne W, Lopez-Saura P, Guillen Nieto G, et al. The Pro-Inflammatory Environment in Recalcitrant Diabetic Foot Wounds. Int Wound J (2008) 5(4):530–9. doi: 10.1111/j.1742-481X.2008.00457.x
180. Hu P, Yang Q, Wang Q, Shi C, Wang D, Armato U, et al. Mesenchymal Stromal Cells-Exosomes: A Promising Cell-Free Therapeutic Tool for Wound Healing and Cutaneous Regeneration. Burns Trauma (2019) 7:38. doi: 10.1186/s41038-019-0178-8
181. Joo HS, Suh JH, Lee HJ, Bang ES, Lee JM. Current Knowledge and Future Perspectives on Mesenchymal Stem Cell-Derived Exosomes as a New Therapeutic Agent. Int J Mol Sci (2020) 21(3):727. doi: 10.3390/ijms21030727
182. Vu NB, Nguyen HT, Palumbo R, Pellicano R, Fagoonee S, Pham PV. Stem Cell-Derived Exosomes for Wound Healing: Current Status and Promising Directions. Minerva Med (2020) 112(3):384–400. doi: 10.23736/s0026-4806.20.07205-5
183. Shabbir A, Cox A, Rodriguez-Menocal L, Salgado M, Van Badiavas E. Mesenchymal Stem Cell Exosomes Induce Proliferation and Migration of Normal and Chronic Wound Fibroblasts, and Enhance Angiogenesis In Vitro. Stem Cells Dev (2015) 24(14):1635–47. doi: 10.1089/scd.2014.0316
184. Liu W, Yu M, Xie D, Wang L, Ye C, Zhu Q, et al. Melatonin-Stimulated MSC-Derived Exosomes Improve Diabetic Wound Healing Through Regulating Macrophage M1 and M2 Polarization by Targeting the PTEN/AKT Pathway. Stem Cell Res Ther (2020) 11(1):259. doi: 10.1186/s13287-020-01756-x
185. Kaushik K, Das AJC. Endothelial Progenitor Cell Therapy for Chronic Wound Tissue Regeneration. Cytotherapy (2019) 21(11):1137–50. doi: 10.1016/j.jcyt.2019.09.002
186. Li X, Xie X, Lian W, Shi R, Han S, Zhang H, et al. Exosomes From Adipose-Derived Stem Cells Overexpressing Nrf2 Accelerate Cutaneous Wound Healing by Promoting Vascularization in a Diabetic Foot Ulcer Rat Model. Exp Mol Med (2018) 50(4):1–14. doi: 10.1038/s12276-018-0058-5
187. Chen C, Rao S, Ren L, Hu X, Tan Y, Hu Y, et al. Exosomal DMBT1 From Human Urine-Derived Stem Cells Facilitates Diabetic Wound Repair by Promoting Angiogenesis. Theranostics (2018) 8(6):1607–23. doi: 10.7150/thno.22958
188. Li M, Yu H, Pan H, Zhou X, Ruan Q, Kong D, et al. Nrf2 Suppression Delays Diabetic Wound Healing Through Sustained Oxidative Stress and Inflammation. Front Pharmacol (2019) 10:1099. doi: 10.3389/fphar.2019.01099
189. Ks A, Yj A, Xw A, Jz B, Kl A, Jing WA, et al. Exosomes From Adipose-Derived Stem Cells Alleviate the Inflammation and Oxidative Stress via Regulating Nrf2/HO-1 Axis in Macrophages - ScienceDirect. Free Radic Biol Med (2021) 165:54–66. doi: 10.1016/j.freeradbiomed.2021.01.023
190. Hesseler MJ, Shyam N. Platelet-Rich Plasma and its Utility in Medical Dermatology: A Systematic Review. J Am Acad Dermatol (2019) 81(3):834–46. doi: 10.1016/j.jaad.2019.04.037
191. Zhang W, Jiang H, Kong Y. Exosomes Derived From Platelet-Rich Plasma Activate YAP and Promote the Fibrogenic Activity of Müller Cells via the PI3K/Akt Pathway. Exp Eye Res (2020) 193:107973. doi: 10.1016/j.exer.2020.107973
192. Dekoninck S, Blanpain C. Stem Cell Dynamics, Migration and Plasticity During Wound Healing. Nat Cell Biol (2019) 21(1):18–24. doi: 10.1038/s41556-018-0237-6
193. Sun Q, Liu L, Mandal J, Molino A, Stolz D, Tamm M, et al. PDGF-BB Induces PRMT1 Expression Through ERK1/2 Dependent STAT1 Activation and Regulates Remodeling in Primary Human Lung Fibroblasts. Cell Signal (2016) 28(4):307–15. doi: 10.1016/j.cellsig.2016.01.004
194. Guo SC, Tao SC, Yin WJ, Qi X, Yuan T, Zhang CQ. Exosomes Derived From Platelet-Rich Plasma Promote the Re-Epithelization of Chronic Cutaneous Wounds via Activation of YAP in a Diabetic Rat Model. Theranostics (2017) 7(1):81–96. doi: 10.7150/thno.16803
195. Kaštelan S, Orešković I, Bišćan F, Kaštelan H. Gverović Antunica AJBm. Inflammatory and Angiogenic Biomarkers in Diabetic Retinopathy. Karger Publishers (2000) 30(3):030502. doi: 10.11613/bm.2020.030502
196. Gregg EW, Sattar N, Ali MK. The Changing Face of Diabetes Complications. Diabetologia (2016) 4(6):537–47. doi: 10.1007/s00125-019-05075-6
197. Wen J, Ma Z, Livingston M, Zhang W, Yuan Y, Guo C, et al. Decreased Secretion and Profibrotic Activity of Tubular Exosomes in Diabetic Kidney Disease. Am J Physiol Renal Physiol (2020) 319(4):F664–F73. doi: 10.1152/ajprenal.00292.2020
Keywords: diabetic vascular complications (DVC), exosome, atherosclerosis, diabetic retinopathy (DR), diabetic kidney disease (DKD), stem cells
Citation: Chen A, Wang H, Su Y, Zhang C, Qiu Y, Zhou Y, Wan Y, Hu B and Li Y (2021) Exosomes: Biomarkers and Therapeutic Targets of Diabetic Vascular Complications. Front. Endocrinol. 12:720466. doi: 10.3389/fendo.2021.720466
Received: 04 June 2021; Accepted: 22 July 2021;
Published: 12 August 2021.
Edited by:
Undurti Narasimha Das, UND Life Sciences LLC, United StatesReviewed by:
Jafar Rezaie, Urmia University of Medical Sciences, IranCopyright © 2021 Chen, Wang, Su, Zhang, Qiu, Zhou, Wan, Hu and Li. This is an open-access article distributed under the terms of the Creative Commons Attribution License (CC BY). The use, distribution or reproduction in other forums is permitted, provided the original author(s) and the copyright owner(s) are credited and that the original publication in this journal is cited, in accordance with accepted academic practice. No use, distribution or reproduction is permitted which does not comply with these terms.
*Correspondence: Yanan Li, bHluLmh1c3RAcXEuY29t; Bo Hu, aHVib0BodXN0LmVkdS5jbg==
†These authors have contributed equally to this work and share first authorship
Disclaimer: All claims expressed in this article are solely those of the authors and do not necessarily represent those of their affiliated organizations, or those of the publisher, the editors and the reviewers. Any product that may be evaluated in this article or claim that may be made by its manufacturer is not guaranteed or endorsed by the publisher.
Research integrity at Frontiers
Learn more about the work of our research integrity team to safeguard the quality of each article we publish.